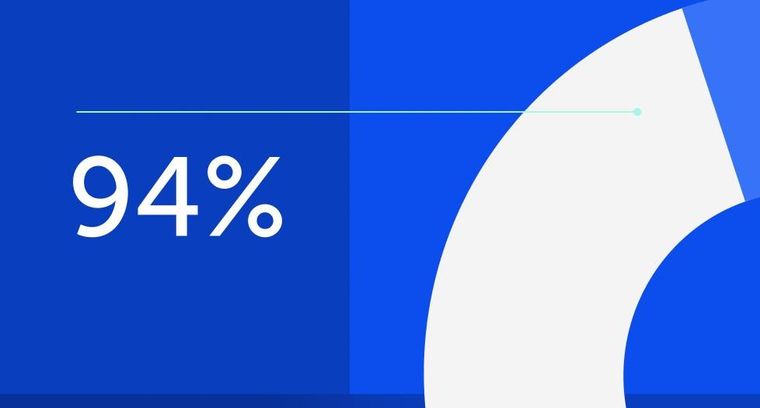
94% of researchers rate our articles as excellent or good
Learn more about the work of our research integrity team to safeguard the quality of each article we publish.
Find out more
REVIEW article
Front. Microbiol., 28 February 2024
Sec. Microbial Symbioses
Volume 15 - 2024 | https://doi.org/10.3389/fmicb.2024.1303010
In recent years, the insect microbiome has become the focus of many actinomycete researchers in their search for novel bioactive compounds with members of the order Hymenoptera at the forefront of the revolution. Hymenoptera encompasses all bees, wasps, ants, and sawflies and is the third largest insect order by species richness. Additionally, Hymenoptera is the most diverse insect order in terms of ecological roles, behaviors, and social systems, thus making it an ideal starting point in the search for symbiotic actinomycetes. The aim of this review is to summarize current knowledge on hymenopteran associations with actinomycetes including information on interactions between actinomycetes and hymenopterans, isolation, and screening methodologies, as well as novel actinomycete species and natural products discovered between early 2013 and 2023. A total of 19 new species were discovered within this time period, with the genus Streptomyces being represented by 11 species while the remaining 8 belonged to rare actinomycetes genera. In addition, 35 novel compounds were reported from hymenopteran-associated actinomycetes within the same time period with the majority originating from Streptomyces strains. The reported novel compounds exhibit a range of biological activities including antibacterial, antifungal, anticancer, anti-enzymatic, and antiproliferative activity, as well as cytotoxicity.
Graphical Abstract. Metabolites of hymenopteran-associated actinomycetes (created using BioRender.com).
Among the most alarming health concerns in the 21st century, is the issue of antimicrobial resistance. Antimicrobial resistance (AMR) occurs in bacteria, fungi, viruses, and parasites, but bacteria are the most concerning organisms due to the frequency of occurrence of resistance (Prestinaci et al., 2015). Currently, AMR is worse than ever with all known antibiotic classes having corresponding
resistant organisms. To understand the magnitude of the problem, the US-CDC’s 2013 annual report on AMR lists three resistant human pathogens (Clostridium difficile, Carbapenem-resistant Enterobacteriaceae, and Drug-resistant Neisseria gonorrhoeae) as urgent threats (CDC, 2013). In comparison, the latest report, which was published in 2019, included two additional pathogens (Carbapenem-resistant Acinetobacter and Candida auris) in the category (CDC, 2019). Furthermore, more than 2.8 million cases of AMR infections and about 36,000 deaths are reported annually in the US alone (CDC, 2019; Kadri, 2020). In addition to the immense loss of lives, the current AMR crisis has placed a heavy burden on the global economy.
Nature has long been a source of inspiration and innovation in the realm of drug discovery and development. From the rainforests to the depths of the oceans, organisms have gifted us with an array of bioactive compounds. Among the diverse organisms that have caught the attention of scientists are microorganisms known as actinomycetes which have gifted mankind with some of the most important antibiotics. Actinomycetes, in this review, refers to members of class actinomycetes of phylum Actinomycetota and comprises of Gram-positive filamentous bacteria with a high Guanine-plus-Cytosine (G+C) content in their genomes. Actinomycetes have long been recognized as prolific producers of natural products, including antibiotics, anticancer agents, immunosuppressants, and enzymes etc (Barka et al., 2015). Their antibiotic production ability is unrivaled with actinomycete-derived antibiotics accounting for 70% of clinically used antibiotics (Subramani and Sipkema, 2019). Traditionally, actinomycetes were isolated from soil and marine environments, which have served as the primary reservoirs for drug discovery. However, diminishing returns in metabolite discovery in the past few decades have spurred scientists to not only revise their methods but also explore alternative reservoirs for actinomycetes (Matsumoto and Takahashi, 2017; Jose et al., 2021). In recent years, a growing body of research has unveiled the tremendous potential of symbiotic actinomycetes, with hymenopteran insects emerging as a particularly promising host group (Poulsen et al., 2011; Nechitaylo et al., 2014; Matarrita-Carranza et al., 2017; Chevrette et al., 2019; Long et al., 2022). Hence, this review focuses on associations between actinomycetes and hymenopteran insects as well as bioactive compounds produced.
Being ubiquitous in nature, microorganisms have developed diverse and unique interactions with a wide range of host organisms, spanning several eukaryotic phyla. Insects are no exception to this rule as the average healthy insect is said to contain more microbial cells than insect cells (Douglas, 2015). Nonetheless, among the multitude of microbial cells that may be present in or on an insect at any given time, very few are symbiotes of the insect. Due to the anatomy and physiology of insects, different regions of the body differ in the ease of invasion by microbes. Regions such as the gut and cuticle are considered open and thus, susceptible to colonization by microorganisms. As such, these regions are dominated by free-living microbes which in a sense, utilize their insect host as a means of dispersal, and microbes that are common among insects and other animals but are rarely isolated from environmental samples (Douglas, 2015). However, several insects have evolved specialized structures to house specific groups of microbes. For example, cuticular structures such as mycetangia in ambrosia beetles are used to store mutualistic fungi which the beetles cultivate as a food source and similarly, antennal gland reservoirs in beewolf wasps and foveae in attine ants are used as culture vessels for mutualistic bacteria of the order Actinomycetales (Currie et al., 2006; Li et al., 2018). These modified structures are not exclusive to the cuticle as the guts of some insect species are also known to exhibit modifications. The midguts of many heteropteran insects for example, are known to contain evaginations known as crypts or caeca which are inhabited by symbiotic bacteria (Engel and Moran, 2013). Ishikawaella capsulata, a mutualistic symbiote of the Japanese stinkbug Megacopta punctatissima, has been shown to inhabit crypts in the midgut region of its host (Fukatsu and Hosokawa, 2002).
Microorganisms that are capable of breaching open regions gain access to less accessible (closed) regions of the insect body. An estimated 10–20% of insect species possess specialized cells, known as bacteriocytes, which mainly function in housing and maintaining symbiotic bacteria (Douglas, 2011). A variation of the bacteriocyte, known as the mycetocyte, is known to contain fungi such as yeasts in some insect species. In addition, gut bacteria can gain access to the hemolymph (which is rich in nutrients) provided they are able to tolerate the conditions (e.g., pH, oxygen content) and bypass the insects’ immune system. Bacteria in tissues such as the hemolymph are usually associated with infections, for example the nematode Steinernema carpocapsae is involved in a mutualistic relationship with the bacterium Xenorhabdus nematophila in which S. carpocapsae invades the gut of an insect larva and subsequently releases X. nematophila at the homocoel (i.e., into the hemolymph) where it compromises the host’s immune system (Singh et al., 2015). Nonetheless, hemolymph-associated mutualistic bacteria have also been reported in some insects. According to Tufts and Bextine (2009), Bacillus cereus and B. thuringiensis are prominent in the hemolymph of imported fire ants (Solenopsis invicta) and the latter is thought to produce toxins that aid in the ants’ defense.
While the majority of actinomycetes exist as free-living cells in soil and water, several actinomycetes have developed associations host organisms such as fungi, plants, and animals. Actinomycete interactions are often mutualistic or commensal in nature, but in rare cases, can be parasitic. In plants, for example, symbiotic actinomycetes of the genera Frankia and Micromonospora form mycorrhizal associations, aiding in nitrogen fixation in exchange for nutrients (Carro et al., 2013). On the contrary, Streptomyces spp. such as S. scabies, S. aureofaciens, S. acidiscabies, and S. ipomoeae, are associated with scab and rot diseases in potatoes, beets, and carrots (Locci, 1994).
Currently, most known mutualisms between Actinomycetota and insects involve members of the class Actinomycetia (Seipke et al., 2012; van der Meij et al., 2017; Baranova et al., 2022). Thus far, actinomycetes have been isolated from insects of the Orders Blattodea, Coleoptera, Dermaptera, Hymenoptera, Lepidoptera, Orthoptera, and Trichoptera (Van Moll et al., 2021; Baranova et al., 2022). Symbiotic associations between actinomycetes and insects are often mutualistic, with both partners contributing toward the wellbeing of each other. The insect host is often tasked with providing a suitable shelter and nutrients to support the growth and reproduction of its symbiote. For example, in leaf-cutter ants, cuticular invaginations on the thorax and legs serve as culture vessels and stores for Pseudonocardia symbiotes whereas antennal gland reservoirs serve the same purpose for the Streptomyces symbiotes of Beewolf wasps (Douglas, 2015). The host insect is also responsible for the transmission of its symbiote either horizontally or vertically, with the latter being often restricted to obligate symbioses. On the other hand, actinomycetes may provide nutritional benefits to their insect hosts as in the case of hemipteran insects. Studies have demonstrated that Rhodnius prolixus and Triatoma infestans, which are both vectors of chagas disease, are nutritionally dependent on actinomycetes of the genera Rhodococcus and Corynebacterium (Kaltenpoth, 2009). Nonetheless, defense is the most well-known and studied service provided by actinomycetes for their insect hosts. This comes as no surprise as actinomycetes are the most prolific producers of antibiotics among microbes. Furthermore, besides producing antagonistic compounds that directly inhibit parasites of their hosts, actinomycetes may also contribute to the host’s defense indirectly. Studies of bacterial-insect mutualisms have identified two main mechanisms of indirect defense; (1) the occupation of susceptible niches in and on the host to outcompete parasites (Koehler, 2014), and (2) the modulation of the insect’s innate immunity (Koehler, 2014; Douglas, 2015).
Hymenoptera comprises more than 150,000 described species, making it the third largest insect order in terms of species richness. Hymenoptera consists of Suborder Symphyta which includes sawflies, horntails, and parasitic wood wasps and Suborder Apocrita which comprises all ant, wasp, and bee species. Along with termites (Order Blattodea), many hymenopterans exhibit eusocialism, a system characterized by the presence of reproductive division of labor, brood care, and an overlap of generation of individuals of the same colony (Matarrita-Carranza et al., 2017). At the same time, solitary species of hymenopterans are also well-known and studied. Hymenopterans are holometabolous insects, meaning they undergo complete metamorphosis (i.e., from egg to larva, larva to pupa and pupa to adult). Furthermore, brood care is common among social hymenopterans while solitary species usually exhibit parasitoidism. Dietary habits are also diverse within Hymenoptera and range from carnivory (insectivory and in rare cases, cannibalism) to herbivory (nectarivory, palynivory, granivory, and frugivory). Furthermore, dietary habits often vary for the different developmental stages (Hyodo et al., 2011; Maák et al., 2020). In some ants for example, larval diets tend to be rich in proteins whereas adult workers require sugar-rich diets (Pohl et al., 2016). These vast variations in lifestyle within the Hymenoptera make it an ideal starting point in the search for novel actinomycetes, unique symbioses and consequently, new bioactive natural products.
With regards to symbioses with actinomycetes, few insect groups have been studied as extensively as the Order Hymenoptera (Van Moll et al., 2021; Baranova et al., 2022). Hymenopteran-actinomycete symbioses are predominantly defensive in nature and often follow either of two mechanisms: (1) the protection of the host’s nutritional resources or, (2) the protection of the host and its offspring.
The richness in species of the Order Hymenoptera means that species are often forced to compete over limited resources especially with regards to nutrition (Houadria et al., 2015). Possibly in response to this pressure, certain lineages of hymenopterans have carved out specific nutritional niches in their respective environments. One important adaptation is the development of farming, ranging from plant farming in plant-ants to fungus-farming in attine ants (Chomicki et al., 2019; Shik et al., 2021). While an effective strategy to reduce inter-species competition, farming presents unique challenges, the most prominent of which is parasitism. Nest parasites are generally common in hymenopterans particularly in soil-nesting and social species (Wcislo, 1996; Schmid-Hempel, 2019). Nonetheless, most nest parasites are generalists and thus, can be controlled via behavioral adaptations such as grooming and weeding. Specific lineages of parasites, however, are specialized in infecting nutritional resources, essentially competing with hymenopterans over their food (Nechitaylo et al., 2014; Gotting et al., 2022). It is now known that certain farming hymenopterans recruit actinomycetes for defense (Kaltenpoth et al., 2005; Batey et al., 2020). This defensive function, in all studied cases, is mediated by individual compounds or mixtures produced by the symbiotic actinomycetes (Oh et al., 2009; Fukuda et al., 2021).
Ants of the tribe Attini (Subfamily Myrmicinae, Family Formicidae) are among a handful of insects that are extensively known to cultivate fungi for food (Goldstein and Klassen, 2020). The most primitive form of agriculture emerged in lower attines about 55–65 million years ago and subsequently diversified into other systems (Li et al., 2018; Mueller et al., 2018). Lower agriculture is practiced by members of the basal groups in the Palaeoattini and characteristically involves the cultivation of Basidiomycetes from two distinct clades (Clade 1 and 2) of the Leucocoprineae tribe (Family Agaricaceae) (Mehdiabadi et al., 2012; Mueller et al., 2018). Still within the Paleoattini, a few members of the genus Apterostigma have specialized in the cultivation of the phylogenetically distant coral fungi (Family Pterulaceae) (Cafaro et al., 2011; Douglas, 2022). Ants of the genus Cyphomyrmex (Subfamily Neoattini) are known to cultivate a single clade of single-celled dimorphic leucocoprineaceous fungi (Yeast agriculture) (Douglas, 2022). Finally, higher agriculture encompasses the agricultural systems of the ant genera; Atta, Acromyrmex, Trachymyrmex, and Sericomyrmex which predominantly cultivate fungi from two clades (clade A and B) of the Leucocoprineae tribe (Solomon et al., 2011; Mueller et al., 2018). Trachymyrmex spp. have also been found to cultivate lower-attine fungal cultivars (Mueller et al., 2018). The system of fungiculture practiced by Atta and Acromyrmex species slightly differs from traditional higher agriculture as members of the genera exclusively cultivate fungi on freshly cut leaves as opposed to dead plant material and as such, is appropriately termed leaf-cutter agriculture (Cafaro et al., 2011; Li et al., 2018). Leaf-cutter ants predominantly cultivate Leucocoprinus gongylophorus which is thought to be an obligate symbiote of higher attine ants due to its; (i) strict vertical transmission, (ii) absence in the free-living condition, and (iii) production of nutrient-rich hyphal swellings known as gongylidia that are absent in all other Leucocoprineae (Weber, 1972; Solomon et al., 2011; Mehdiabadi et al., 2012).
Fungi from the genus Escovopsis have specialized in infecting attine ant fungal cultivars and represent the most significant threats to the ants’ food resources (Figure 1; Meirelles et al., 2014; Jiménez-Gómez et al., 2021; Montoya et al., 2021). The fungal garden parasites have attained a level of specialization that is suggestive of co-evolution alongside the ants and their fungal cultivars (Mueller et al., 2018; Douglas, 2022). In response to Escovopsis infections, attine ants have developed adaptations such as fungus grooming, weeding and general nest hygiene as well as the use of metapleural gland secretions (Currie et al., 1999; Currie and Stuart, 2001; Fernández-Marín et al., 2009). Furthermore, some leafcutter fungal cultivars have been shown to be capable of inhibiting Escovopsis associated with lower attines (Birnbaum and Gerardo, 2016). Nonetheless, none of these behavioral and chemical adaptations have proven as effective as the recruitment of mutualistic actinomycetes of the genus Pseudonocardia as biocontrol agents (Figure 1; Goldstein and Klassen, 2020). Depending on the attine ant species, Pseudonocardia symbiotes are localized on different regions and/or structures of the ants’ body. In ants of the genus Apterostigma, Pseudonocardia are contained on the ventral surface of the propleura or metapleural whereas in ants of the genus Trachymyrmex, the symbiotes occur on tubercles which are contained within caeca (Cafaro et al., 2011; Caldera and Currie, 2012). However, in both cases, the area of localization of the Pseudonocardia symbiote is lined by pores associated with exocrine gland cells which are thought to play the role of nourishing the bacterial symbiote (Poulsen et al., 2003). This ability to control nutrient availability, by extension, allows attine ants to regulate the growth of the symbiote as per demand (Currie et al., 2003; Goldstein and Klassen, 2020).
Figure 1. Schematic diagram of the attine ant symbiosis showing interactions between symbiotes (created using BioRender.com).
The effectiveness of Pseudonocardia as biological control for Escovopsis has been demonstrated in vitro through antagonism experiments and in vivo within the context of the attine ant mutualism (Currie et al., 1999; Little and Currie, 2008; Poulsen et al., 2010; Meirelles et al., 2014; Dângelo et al., 2016). There is also evidence to support the vertical transmission and specificity of Pseudonocardia symbiotes among attine ant species (Cafaro et al., 2011; Andersen et al., 2013). Furthermore, Pseudonocardia spp. associated with Trachymyrmex septentrionalis have been shown to be capable of selectively inhibiting other attine ant associated Pseudonocardia using a thiopeptide antibiotic (GE37468) likely acquired from soil bacteria (Chang et al., 2020). Early studies of attine-ant associations postulated the idea that Pseudonocardia are locked in an evolutionary arms race with Escovopsis and that both organisms have been coevolving. However, a reevaluation of this evidence by Mueller (2012) bred an opposing viewpoint which suggests that attine ants may utilize a community of bacteria for garden defense and that non-Pseudonocardia isolated from attine ant biofilms may not be contaminants as previously thought. Furthermore, the author argues that Pseudonocardia are not specialized in the inhibition of Escovopsis and are capable of inhibiting a much broader spectrum of pathogens (Mueller, 2012).
Even more fascinating is the fact that black yeasts of the genus Phialophora and associates (Ascomycota: Chaetothyriales) have been reported to be associated with the attine ant symbiosis (Figure 1; Little and Currie, 2007; Bizarria et al., 2022). Black yeasts are parasites known to derive nutrients from and suppress the growth of attine-ant Pseudonocardia (Little and Currie, 2007). Little and Currie (2008) demonstrated, using infection experiments, that Apterostigma pilosum colonies infected with black yeast are significantly less effective at defending their fungal gardens than uninfected colonies.
Interactions in the complex web that is the attine symbiosis are facilitated by secondary metabolites produced by participating organisms. Several compounds with bioactive properties have been reported from the attine ant symbiosis. Recently, a study of a Trachymyrmex sp. in Brazil identified two new compounds, conocandin B and dentigerumycin F, produced by Escovopsis and Pseudonocardia, respectively. Interestingly, conocandin B was found to upregulate the production of dentigerumycin F and vice versa (Bae et al., 2021).
Pathogens do not only contend over a host’s nutritional resources as the host organism itself can be a source of nutrients for specialized pathogens. For example, the fungus Ophiocordyceps unilateralis is known to hijack ant hosts for its reproduction and dispersal (Evans et al., 2011). Similarly, American foulbrood is a disease in honey bee larvae caused by the bacterium Paenibacillus larvae (Stephan et al., 2020). Actinomycetes, due to their prevalence in hymenopterans and potential as antimicrobial producers, are good candidates for the defense of hosts.
Beewolf wasps or bee-killer wasps are solitary wasps of the tribe Philanthini (Family Crabonidae) that nest in sandy soil and, as their name suggests, primarily prey on honeybees (Kaltenpoth et al., 2005; Nechitaylo et al., 2014). Nests are constructed by female beewolves to house developing larvae and their food, usually over the winter. In summer, fully developed adults emerge from the burrow nests to continue the cycle (Seipke et al., 2012). As such, it makes sense that nest hygiene is very critical for the survival of young beewolves, especially considering that the physical (warmth and high humidity) and biological (exposure of larvae to dead insects) conditions within burrow nests favor the growth of parasites (Kaltenpoth et al., 2012; Koehler et al., 2013). Like fungus-farming ants, beewolf wasps have developed adaptations to manage parasites (Kaltenpoth et al., 2012; Seipke et al., 2012; Nechitaylo et al., 2014; Goettler et al., 2022). One of such adaptations is the use of cephalic-gland secretions to embalm paralyzed prey as food for the developing offspring (Kaltenpoth et al., 2005). Recent reports also show that beewolves emit large amounts of gaseous nitric oxide to protect themselves and their food from pathogenic fungi (Strohm et al., 2019). Additionally, beewolf wasps have also acquired an actinomycete symbiote to aid in defense (Kroiss et al., 2010). Female beewolf wasps inoculate brood cells thoroughly with the whitish-appearing symbiotic actinomycete prior to oviposition. After oviposition and before the commencement of pupation, beewolf larvae inoculate the actinomycete on their cocoon. Kaltenpoth et al. (2012) demonstrated experimentally that the symbiotic actinomycete of beewolf wasps significantly decreases larval mortality during development. The results of the study showed that only one out of 15 (6.7%) larvae without access to the symbiotic actinomycete survived till emergence. In contrast, there was an 83.3% (15/18) chance of emergence in the control group (i.e., larvae with access to the symbiote). Furthermore, in addition to its defensive role, the actinomycete symbiote of beewolves is also believed to serve as a directional cue for larvae during emergence from cocoons (Kaltenpoth et al., 2012; Goettler et al., 2022).
The Philanthini tribe comprises three genera; Trachypus, Philanthus, and Philanthinus, all of which have been found to associate with different biovars of the actinomycete Streptomyces philanthi (Figure 2; Nechitaylo et al., 2014). In all three genera, the Streptomyces symbiote is cultivated and maintained in specialized cuticular invaginations on the antennae (Figure 2; Kaltenpoth et al., 2012; Nechitaylo et al., 2014). These antennal reservoirs are presumably connected to the hemolymph and gland cells through which the Streptomyces symbiotes are thought to obtain nourishment from the host (Kaltenpoth et al., 2012). Evidence strongly suggests that Streptomyces symbiotes are transmitted vertically in beewolf wasps. Larvae of the species have been observed ingesting the symbiote from inoculated nests prior to pupation (Kaltenpoth et al., 2005). The same study also demonstrated a general lack of interest in nest construction by an adult female wasp lacking the symbiote.
Figure 2. Schematic diagram of the beewolf wasp symbiosis showing interactions between symbiotes (created using BioRender.com).
The defensive function of S. philanthi in beewolf wasps is believed to be mediated by a cocktail of antibiotics. Analysis of methanol extracts of Philanthus triangulum cocoons revealed that streptochlorin, piericidin A1, and piericidin B1 were the three most abundant compounds produced by Streptomyces philanthi (Engl et al., 2018). Additionally, the total amount of these three compounds was shown to steadily increase in the 2 weeks following inoculation and persist up till the emergence of the offspring. Furthermore, Boukaew et al. (2013) reported that wheat seed cultures inoculated with S. philanthi RM-1-138 contained up to 36 volatile organic compounds, of which four (dimethyl disulfide, dimethyl trisulfide, geosmin, and benzene ethanol) have been reported to possess antimicrobial properties. These volatile compounds could control rice sheath blight disease, having suppressed the growth of plant pathogenic fungi such as Rhizoctonia solani PTRRC-9, Pyricularia grisea PTRRC-18, Bipolaris oryzae PTRRC-36 and Fusarium fujikuroi PTRRC-16 in vitro (Boukaew et al., 2013). The same S. philanthi strain was later shown to protect soybean seeds from aflatoxin-producing fungi and contain additional compounds such as l-linalool, 2-mercaptoethanol and heneicosane (Boukaew and Prasertsan, 2020).
Formicinae is one of the most diverse ant subfamilies, comprising about 3,030 species widely distributed around the world (Ward et al., 2016).
Carpenter ants (genus Camponotus) are known for their unique ability to excavate galleries within wood to create their nests. Unlike termites, carpenter ants are unable to digest the polymers in wood due to the absence of specialized microbes in their midguts (Brune, 2014). However, carpenter ants are known to be involved in a symbiosis with bacteria of the genus Blochmannia in which the bacteria reside in the host’s midguts and aid in the synthesis of essential and non-essential amino acids (Zientz et al., 2006). Zakalyukina et al. (2019) described the isolation of a Streptomyces violaceochromogenes strain capable of producing nybomycin from Camponotus vagus. Another study by the same author demonstrated the cellulose-degrading ability of actinomycetes isolated from Camponotus vagus (Zakalyukina et al., 2021). Moreover, novel actinomycete species such as Amycolatopsis camponoti, Microbispora camponoti, Nocardia camponoti, Streptomyces capitiformicae, and Streptomyces camponoti among others, have been reported from carpenter ants (Table 1). Studied carpenter ant species include Camponotus vagus, C. japonicus and C. kiusiuensis.
Table 1. Novel species of actinomycetes isolated from hymenopteran insects between January 2013 and May 2023.
Formica is an ant genus comprised of honey dew feeding ants that are widely distributed in the Northern hemisphere (Borowiec et al., 2021). A comparative study of actinomycete abundance in ants by Zakalyukina et al. (2017) showed that actinomycetes are associated with Formica cunicularia, although less frequently than Lasius niger. Zakalyukina et al. (2017) reported the isolation of six Streptomyces spp. from adult worker F. cunicularia. Furthermore, novel compounds have also been reported from Streptomyces spp. associated with Formica yessensis (An et al., 2020; Du et al., 2020). The macrolides formicolide A and B were reported from Streptomyces sp. BA01 while formicin A-C were discovered from Streptomyces sp. SFA33 (Table 2).
Ants of the genus Lasius, commonly known as black garden ants are also known to harbor actinomycetes. A study by Zakalyukina et al. (2014) showed that actinomycetes are equally as abundant in Lasius niger ant tissue as in their nests. Furthermore, Zakalyukina et al. (2017) isolated nine Streptomyces and one Nocardia from adult Lasius niger workers. Two more Streptomyces strains were reported from the same ant species in 2020 (Efimenko et al., 2020). In addition, four novel species of actinomycetes have been discovered from Lasius spp., including three from Lasius fuliginosus and one from Lasius flavus (Table 1).
Like carpenter ants, ants belonging to the genus Polyrhachis are members of the Camponotini tribe. Two novel species of actinomycetes, Micromonospora polyrhachis (Xiang et al., 2014) and Streptomyces polyrhachii (Yu et al., 2013), were isolated from the edible Chinese black ant (Polyrhachis vicina).
Actinomycetes have also been reported from formicine ant genera such as, Oecophylla (Hosmath and Timmappa, 2019), Technomyrmex (Diarra and Osborne-Naikatini, 2023), Petalomyrmex (Hanshew et al., 2015) and Paratrechina (Reyes and Cafaro, 2015; Matarrita-Carranza et al., 2017).
Although much of the focus on actinomycetes within the subfamily Myrmicinae has been directed toward attine ants, actinomycetes have also been reported from non-attine members of the subfamily. The ant genus Allomerus is comprised of ants involved in a unique symbiosis with plants of the genera Cordia and Hirtella (Ruiz-González et al., 2011). Allomerus ants are carnivorous and mainly catch prey via entrapment. The ants build their traps along the stem and branches of their host plant using a combination of debris and fungi of the Order Chaetothyriales (Orivel et al., 2017). The fungus is believed to be deliberately farmed by the ants since different Allomerus species have been shown to associate with a monophyletic group of fungi. Nonetheless, till date, vertical transmission of the fungus has not been demonstrated (Seipke et al., 2013). Furthermore, Seipke et al. (2013) showed that Allomerus ants are associated with bioactive actinomycetes. The authors reported the isolation of three Streptomyces spp. and one Amycolatopsis sp. from Allomerus decemarticulatus, and three Streptomyces spp. from Allomerus octoarticulatus. Additionally, the authors demonstrated that some of the isolates inhibit pathogenic fungi derived from the ants’ nest. However, in a follow up study, the authors found a lack of evidence to support the claim that actinomycetes play a defensive role in Allomerus ants. This study utilized metagenomic analyses and found that actinomycetes occur very rarely on the cuticle of Allomerus ants in comparison to other groups such as Erwinia and Serratia species (Seipke et al., 2013). Therefore, there is insufficient evidence to support the idea that Allomerus ants recruit symbiotes of any kind to defend their fungal galleries; however, in the scenario where a defensive symbiote is likely, Erwinia and Serratia could be the culprits since members of both genera are known to produce antimicrobial compounds.
Exploration of the ant Crematogaster margaritae which nests in domatia of the plant Keetia hispida led to the isolation of 121 Actinomycetota predominantly belonging to Streptomyces. Antifungal assay results showed that 3 out of the 121 isolates inhibit fungi in vitro (Hanshew et al., 2015). In a later study Matarrita-Carranza et al. (2017) reported the isolation of 10 actinomycetes from a member of the same genus (i.e., C. longispina).
Members of the genus Messor, commonly known as harvester ants, have also been explored with regards to association with actinomycetes. Wu et al. (2022) isolated two actinomycetes (Brachybacterium phenoliresistens and Microbacterium sp.) from Messor orientalis tissue and noted strong antifungal activity against plant pathogens. Furthermore, the actinomycete Streptomyces globisporus subsp. globisporus was reported from different colony components of Messor structor and found to be a new producer of albomycin (Zakalyukina et al., 2022b).
Actinomycetes have also been reported from two members of the genus Pheidole (Matarrita-Carranza et al., 2017), and Tetramorium (Diarra and Osborne-Naikatini, 2023).
Despite being among the most diverse ant subfamilies, there are few reports of actinomycetes in dolichoderine ants. Matarrita-Carranza et al. (2017) isolated eight actinomycetes from Tapinoma ramulorum inrectum while our recently work reports the isolation of six actinomycetes from the obligate plant-ant Philidris nagasau (Diarra and Osborne-Naikatini, 2023). A Streptomyces antibioticus strain isolated from Tapinoma simrothi was reported to produce a quercetin 3-O-glucoside derivative with broad-spectrum antimicrobial activity against human pathogenic bacteria and fungi (Nageh Sholkamy et al., 2020).
This subfamily contains only one genus, Paraponera which inhabits tropical rainforests in Central and South America. Paraponera clavata (commonly known as the bullet ant) is the only extant member of the genus and samples of the species have been reported to contain actinomycetes (Matarrita-Carranza et al., 2017).
A study of the bacterial diversity of the gut of five ponerine species including Dinoponera lucida, Pachycondyla curvinodis, Pachycondyla striata, Odontomachus brunneus and O. bauri observed the presence of Actinomycetota in the gut microbiota all five species (Oliveira et al., 2016). A separate but similar study found that Actinomycetota are abundant in the gut microbiome of O. monticola and Ectomomyrmex javanus (Zheng et al., 2021). Moreover, Matarrita-Carranza et al. (2017) also reported the isolation of 25 actinomycetes, five of the isolates were found to exhibit antifungal activity against a Metarhizium sp., from four Odontomachus spp.
With about 32 species residing in plant domatia, Pseudomyrmecinae is the most diverse plant-associated ant subfamily (Chomicki et al., 2015). Although members of the subfamily are predominantly tropical, some also occur in arid and subtropical regions (Ward, 2021). Pseudomyrmecinae contains about 230 species contained within only three genera, two of which have been reported to contain actinomycetes (Seipke et al., 2013; Chomicki et al., 2015; Hanshew et al., 2015).
Hanshew et al. (2015) obtained 60 Actinomycetota isolates from the ant-plant system of Pseudomyrmex penetrator and Tachigali sp., three of which displayed antifungal activity in vitro.
Actinomycetes were first reported in this genus in a study by Seipke et al. (2013) which assessed the bacterial communities of plant-associated Allomerus spp. and Tetraponera penzigi using culture independent techniques. The findings of the study showed that Actinomycetota are abundant in the microbiome of T. penzigi and second only to Proteobacteria. Furthermore, the authors successfully isolated eight actinomycetes (5 Streptomyces spp. and 3 Saccharopolyspora spp.) from T. penzigi, with five isolates displaying antifungal activity. Nonetheless, the authors concluded that, like in Allomerus spp., actinomycetes are unlikely defensive symbiotes of Tetraponera (Seipke et al., 2013). Seipke and colleagues reported the discovery of a novel group of polyketide compounds, formicamycins, from one of the previously isolated Streptomyces strains (Qin et al., 2017). The producer strain, Streptomyces formicae KY5, was isolated from the domatium of an Acacia plant associated with T. penzigi and genome sequencing revealed that it contains 39 biosynthetic gene clusters (BGCs) (Seipke et al., 2013). Formicamycins exhibit potent antimicrobial activity against Gram-positive human pathogens including methicillin resistant Staphylococcus aureus (MRSA) and vancomycin resistant Enterococci (VRE) (Qin et al., 2017).
Decade old studies have shown that honey bees harbor actinomycetes. Promnuan et al. (2009) reported the isolation of Streptomyces, Nonomuraea and Nocardiopsis from three honey bee species (Apis mellifera, Apis cerana and Apis florea) collected in Thailand. In a later study, the same authors described a novel actinomycete (Actinomadura apis) isolated from an A. mellifera hive in Thailand (Promnuan et al., 2011). In addition to hives, actinomycetes have been reported from worker bee tissue (Patil et al., 2010; Promnuan et al., 2021), bee larvae (Promnuan et al., 2021), honey (Promnuan et al., 2021), and pollen (Grubbs et al., 2021; Promnuan et al., 2021). While there is limited evidence to propose a symbiotic association between actinomycetes and honey bees, some bee isolates have been found to inhibit hive pathogens (Promnuan et al., 2009; Grubbs et al., 2021).
Members of this genus are native to the neotropics and are commonly known as orchid bees. In their study of hymenopteran-associated actinomycetes, Matarrita-Carranza and colleagues sampled twelve colonies encompassing six species of the genus Euglossa. Out of the six studied species, actinomycetes were only present in two (i.e., Euglossa heterosticta and Euglossa imperialis).
Meliponine bees (Tribe Meliponini, Family Apidae) form the largest grouping of eusocial bees with over 500 described species (Hrncir et al., 2016). The bees are commonly known as stingless bees due to their highly reduced stingers in comparison to other bees. Promnuan et al. (2009) reported the isolation of sixteen Streptomyces from two species of stingless bees, Trigona laeviceps (10 isolates) and Trigona fuscobalteata (6 isolates). Furthermore, the authors reported that six isolates were capable of inhibiting nest pathogens of the bees in vitro. In a 2019 study, seven actinomycetes (six Streptomyces, and one Micromonospora) were isolated from foraging and nurse Melipona scutellaris bees. Two isolates from the study, Streptomyces sp. ICBG1323 and Micromonospora sp. ICBG1321, yielded 15 compounds including lobophorins A, B, CR1, and K and ten anthracyclines (Rodríguez-Hernández et al., 2019). In the same year, Ngalimat et al. (2019) isolated a Streptomyces kunmingensis strain from the Malaysian stingless bee, Heterotrigona itama. Furthermore, two novel hexadepsipeptides were reported from a stingless-bee-associated Streptomyces and found to exhibit strong antibacterial activity against Paenibacillus larvae (Menegatti et al., 2020).
Besides beewolf wasps, actinomycetes have only been isolated from one crabonid genus (Trypoxylon) to our knowledge (Matarrita-Carranza et al., 2017).
To our knowledge, actinomycetes have only been reported from an unidentified member of this family, Pompilidae sp. A (Matarrita-Carranza et al., 2017).
Sceliphrinae is a wasp subfamily that consists of solitary thread-waisted wasps with a cosmopolitan distribution. The subfamily contains six genera, two of which are known to build mud nests (Powell and Taylor, 2017). Nests are usually created by female mud-daubers to house the developing young and their food (paralyzed spiders or insects). The fact that nests are constructed out of mud and often contain paralyzed prey has led scientists to speculate a mechanism for nest hygiene involving actinomycetes (Poulsen et al., 2011; Kumar et al., 2012). Actinomycetes with bioactive properties have been isolated from two mud-dauber wasp species including yellow-black mud-dauber wasps (Sceliphron caementarium) and blue-black mud-dauber wasp (Chalybion californicum) (Poulsen et al., 2011). Additionally, the novel compound Sceliphrolactam was discovered from the fermentation broth of Streptomyces flavogriseus strain e122, isolated from Sceliphron caementarium (Oh et al., 2011). Nonetheless, there is a lack of evidence to suggest that actinomycetes are used by mud-dauber wasps as defensive symbiotes since neither symbiote specificity nor transmission has been demonstrated in the wasps.
Polistinae consists of 25 genera and more than 900 species of eusocial vespid wasps (Gomes and Noll, 2009). Together with some members of the subfamilies Stenogastrinae, and Vespinae, members of Polistinae are social and construct paper nests (Höcherl et al., 2016).
The open-faced nature of paper wasp nests makes them prone to contamination by airborne bacterial and fungal spores leading some scientists to hypothesize defensive mechanisms. Madden et al. (2013), reported the isolation of thirty actinomycetes spanning three actinomycete genera (Streptomyces, Micromonospora, and Actinoplanes) from the nests of paper wasps. However, evidence to support a symbiotic relationship is weak as P. dominulus nests have been shown to be collection points for heavy metals originating from combustion engines and thus, may trap actinomycete spores in a similar manner (Urbini et al., 2006; Madden et al., 2013). Furthermore, Hoggard et al. (2011) showed that paper wasps produce cuticular antimicrobial compounds that could play vital roles in maintaining nest hygiene.
Polybia is a neotropical eusocial wasp genus comprising about 56 species (Prato et al., 2022). Nonetheless, actinomycetes have only been isolated from two species so far. These two species include Polybia plebeja and P. occidentalis from which 12 and 8 isolates were obtained, respectively (Matarrita-Carranza et al., 2017). Seven of the twenty isolates displayed in vitro antifungal activity against the wasp pathogen Hirsutella citriformis (Matarrita-Carranza et al., 2017). Furthermore, five macrocyclic antibiotics with antibacterial, antitumor and antiviral properties were identified from the extract of one of the isolates (i.e., Streptomyces sp. M54) in a later study (Matarrita-Carranza et al., 2021).
Twenty-two and twenty-four actinomycetes were isolated from members of Agelaia, Metapolybia, respectively, by Matarrita-Carranza et al. (2017).
Traditionally, actinomycetes have been isolated from samples using culture-dependent techniques. The appeal of such techniques is that the actinomycete of interest can be mass produced easily and the production of metabolites can be optimized readily. Most metabolite discoveries from actinomycetes have been conducted in this fashion (Figure 3). However, research suggests that less than 1% of actinomycetes have been identified till date because the vast majority of actinomycetes present in samples remain unculturable under conventional conditions (Subramani and Sipkema, 2019). The advent of high throughput culture-independent techniques such as metagenomics and metatranscriptomics in recent decades, have allowed researchers to directly study DNA from environmental samples (eDNA) to gain insights into the diversity, ecological roles, and biosynthetic potential of organisms (Seyedsayamdost et al., 2012).
Figure 3. Illustration of the typical workflow for isolating and bioprospecting hymenopteran-associated actinomycetes using a traditional approach (created using BioRender.com).
Hymenopteran-associated actinomycetes span several taxa including Streptomyces and rare genera. Like with most other environments, the most frequently isolated actinomycetes from hymenopteran samples are members of the genus Streptomyces (Matarrita-Carranza et al., 2017; Chevrette et al., 2019). In fact, among the novel actinomycete species discovered in the past decade, 58% (11 species) belong to genus Streptomyces. The remaining 42% of the novel species belong to rare actinomycete genera such as Actinocorallia, Amycolatopsis, Micromonospora, Microbispora, Nocardia, Promicromonospora, and Virgisporangium (Table 1).
Nonetheless, several other rare genera of actinomycetes have been isolated from hymenopteran samples including Actinomadura (Promnuan et al., 2009, 2021), Actinoplanes (Madden et al., 2013), Kitasatospora Microbacterium (Wu et al., 2022), Nocardiopsis (Promnuan et al., 2009; Kumar et al., 2012), Nocardioides (Hanshew et al., 2015), Nonomuraea (Promnuan et al., 2009), Phytohabitans (Wang et al., 2020), Propionicimonas (Zucchi et al., 2011), Pseudonocardia (Cafaro et al., 2011; Meirelles et al., 2014; Bruner-Montero et al., 2021), Saccharopolyspora (Kumar et al., 2012), Saccharothrix (Matarrita-Carranza et al., 2017), Streptosporangium (Kumar et al., 2012; Matarrita-Carranza et al., 2017), Thermoactinomycetes (Kumar et al., 2012), Tsukamurella (Barke et al., 2010), Verrucosispora (Wang et al., 2020).
Hymenopteran-actinomycete associations represent an important source of natural products with potential applications in human medicine. Such complex symbiotic interactions have a unique potential in drug discovery due to pathogen pressure in hymenopteran insects which selects for association with actinomycete strains that produce efficacious antimicrobials. Chevrette et al. (2019) demonstrated that insect-associated Streptomyces strains exhibit greater inhibitory activity toward bacterial and fungal pathogens compared to soil and plant associated strains. Additionally, compounds isolated from hymenopteran-associated actinomycetes are not limited to inhibiting associated pathogens but also human pathogens thus, making them important in the fight against antimicrobial resistance. For example, dentigerumycin, the main antifungal compound produced by attine ant-associated Pseudonocardia, to combat Escovopsis infections. However, dentigerumycin also displays good inhibitory activity against amphotericin-resistant Candida albicans ATCC200955 (MIC- 1.1 μM) (Oh et al., 2009). At the same time, compounds derived from hymenopteran-actinomycete defensive mutualisms can display remarkable pathogen specificity. Dentigerumycin, for example, selectively inhibits Escovopsis pathogens of attine ants while causing no harm to the mutualistic fungus of the ants (Oh et al., 2009; Jiménez-Gómez et al., 2021). Finally, actinomycete associations with hymenopterans may select for compounds with low toxicity toward animal cells (Chevrette et al., 2019). Thus, making hymenopteran-associated actinomycetes an ideal source for natural products with therapeutic applications. Some novel compounds derived from hymenopteran-associated actinomycetes are shown in Figure 4.
Figure 4. Structures of some bioactive compounds derived from hymenopteran-associated actinomycetes.
Hymenopteran- associated actinomycetes are known to produce a wide range of bioactive natural products ranging from small to large and simple to complex (Figure 4). Compounds reported from hymenopteran-associated actinomycetes include polyketides (Qin et al., 2017; Prado-Alonso et al., 2022), macrolides (Van Arnam et al., 2016; Batey et al., 2020; Ortega et al., 2021; An et al., 2022), macrolactams (Oh et al., 2011; Beemelmanns et al., 2017), non-ribosomal peptides (Bae et al., 2021; Fukuda et al., 2021), ribosomally synthesized and post-translationally modified peptides (Vikeli et al., 2020), sesquiterpenes (Li et al., 2020), p-terphenyls, polyenes (Barke et al., 2010) and monohydroxypyridines among others. In the last decade, thirty-five compounds, shown in Table 2, were discovered from hymenopteran-associated actinomycetes. The genus Streptomyces stands out as the primary reservoir of hymenopteran-associated actinomycete-derived natural products, as evidenced by the discovery of twenty-six novel compounds from it (Table 2). Rare actinomycetes on the other hand, accounted for five novel compounds in the last decade including three compounds discovered from Pseudonocardia spp. (attinimicin, 9-methoxyrebeccamycin, and dentigerumycin F), one each from Amycolatopsis (nocamycin V) and Saccharopolyspora (kyamycin). Ants yielded 14 out of 19 actinomycete producer strains of the novel compounds while bees and wasps accounted for 3 and 2 producer strains, respectively. The biological properties of the novel compounds include inhibitory activities such as antimicrobial, anticancer, antiangiogenic, anti-inflammatory, antimetastatic antiproliferative, cytotoxic, hexokinase inhibition and quinone reductase promotion in vitro (Table 2).
Actinomycetes can be readily isolated from a wide range of hymenopteran insects but symbiotic relationships have so far only been established in attine ants and beewolf wasps. In both attine ants and beewolf wasps, symbiotic actinomycetes primarily perform defensive functions, thus making them uniquely suited to produce bioactive compounds. However, the exploration of hymenopteran-associated actinomycetes for natural product discovery, like with free-living actinomycetes, is challenged by factors such as low cultivability of microorganisms and the cryptic state of many BGCs under conventional conditions. While several methods have been developed to circumvent these issues, applications of these methods in the study of hymenopteran-associated actinomycetes are limited. Nonetheless, significant progress has been made in the past decade and research has yielded several novel strains of hymenopteran-associated actinomycetes with bioactive potential. Future studies should utilize sequence-based techniques such as metagenomics and metabolomics on hymenopterans to screen actinomycetes for bioactive compounds. Metabolomics and transcriptomics can also shed light on the origin of compounds produced by such actinomycetes, possible ecological roles of the compounds, and cues to express cryptic BGCs.
UD: Conceptualization, Data curation, Formal analysis, Investigation, Methodology, Project administration, Writing – original draft. TO-N: Supervision, Writing – review & editing. RS: Conceptualization, Investigation, Supervision, Writing – original draft.
Ramesh Subramani was involved in the conceptualization and planning of this project but tragically passed away in an accident before the completion.
The authors declare that no financial support was received for the research, authorship, and/or publication of this article.
The authors declare that the research was conducted in the absence of any commercial or financial relationships that could be construed as a potential conflict of interest.
All claims expressed in this article are solely those of the authors and do not necessarily represent those of their affiliated organizations, or those of the publisher, the editors and the reviewers. Any product that may be evaluated in this article, or claim that may be made by its manufacturer, is not guaranteed or endorsed by the publisher.
An, J. S., Lee, J. Y., Kim, E., Ahn, H., Jang, Y.-J., Shin, B., et al. (2020). Formicolides A and B, antioxidative and antiangiogenic 20-membered macrolides from a wood ant gut bacterium. J. Nat. Prod. 83, 2776–2784. doi: 10.1021/acs.jnatprod.0c00772
An, J. S., Lim, H.-J., Lee, J. Y., Jang, Y.-J., Nam, S.-J., Lee, S. K., et al. (2022). Hamuramicin C, a cytotoxic bicyclic macrolide isolated from a wasp gut bacterium. J. Nat. Prod. 85, 936–942. doi: 10.1021/acs.jnatprod.1c01075
Andersen, S. B., Hansen, L. H., Sapountzis, P., Sørensen, S. J., and Boomsma, J. J. (2013). Specificity and stability of the Acromyrmex–Pseudonocardia symbiosis. Mol. Ecol. 22, 4307–4321. doi: 10.1111/mec.12380
Bae, M., Mevers, E., Pishchany, G., Whaley, S. G., Rock, C. O., Andes, D. R., et al. (2021). Chemical exchanges between multilateral symbionts. Org. Lett. 23, 1648–1652. doi: 10.1021/acs.orglett.1c00068
Bai, L., Liu, C., Guo, L., Piao, C., Li, Z., Li, J., et al. (2016). Streptomyces formicae sp. nov., a novel actinomycete isolated from the head of Camponotus japonicus Mayr. Antonie Van Leeuwenhoek 109, 253–261. doi: 10.1007/s10482-015-0628-7
Baranova, A. A., Zakalyukina, Y. V., Ovcharenko, A. A., Korshun, V. A., and Tyurin, A. P. (2022). Antibiotics from insect-associated Actinobacteria. Biology 11:1676. doi: 10.3390/biology11111676
Barka, E. A., Vatsa, P., Sanchez, L., Gaveau-Vaillant, N., Jacquard, C., Klenk, H.-P., et al. (2015). Taxonomy, physiology, and natural products of Actinobacteria. Microbiol. Mol. Biol. Rev. 80, 1–43. doi: 10.1128/mmbr.00019-15
Barke, J., Seipke, R. F., Grüschow, S., Heavens, D., Drou, N., Bibb, M. J., et al. (2010). A mixed community of actinomycetes produce multiple antibiotics for the fungus farming ant Acromyrmex octospinosus. BMC Biol. 8:109. doi: 10.1186/1741-7007-8-109
Batey, S. F. D., Greco, C., Hutchings, M. I., and Wilkinson, B. (2020). Chemical warfare between fungus-growing ants and their pathogens. Curr. Opin. Chem. Biol. 59, 172–181.
Beemelmanns, C., Ramadhar, T. R., Kim, K. H., Klassen, J. L., Cao, S., Wyche, T. P., et al. (2017). Macrotermycins A–D, glycosylated macrolactams from a termite-associated Amycolatopsis sp. M39. Org. Lett. 19, 1000–1003. doi: 10.1021/acs.orglett.6b03831
Birnbaum, S. S. L., and Gerardo, N. M. (2016). Patterns of specificity of the pathogen escovopsis across the fungus-growing ant symbiosis. Am. Nat. 188, 52–65. doi: 10.1086/686911
Bizarria, R. Jr., Pagnocca, F. C., and Rodrigues, A. (2022). Yeasts in the attine ant–fungus mutualism: diversity, functional roles, and putative biotechnological applications. Yeast 39, 25–39. doi: 10.1002/yea.3667
Borowiec, M. L., Cover, S. P., and Rabeling, C. (2021). The evolution of social parasitism in Formica ants revealed by a global phylogeny. Proc. Natl. Acad. Sci. 118:e2026029118. doi: 10.1073/pnas.2026029118
Boukaew, S., Plubrukam, A., and Prasertsan, P. (2013). Effect of volatile substances from Streptomyces philanthi RM-1-138 on growth of Rhizoctonia solani on rice leaf. BioControl 58, 471–482. doi: 10.1007/s10526-013-9510-6
Boukaew, S., and Prasertsan, P. (2020). Efficacy of volatile compounds from Streptomyces philanthi RL-1-178 as a biofumigant for controlling growth and aflatoxin production of the two aflatoxin-producing fungi on stored soybean seeds. J. Appl. Microbiol. 129, 652–664. doi: 10.1111/jam.14643
Brune, A. (2014). Symbiotic digestion of lignocellulose in termite guts. Nat. Rev. Microbiol. 12, 168–180. doi: 10.1038/nrmicro3182
Bruner-Montero, G., Wood, M., Horn, H. A., Gemperline, E., Li, L., and Currie, C. R. (2021). Symbiont-mediated protection of acromyrmex leaf-cutter ants from the entomopathogenic fungus Metarhizium anisopliae. mBio 12:e0188521. doi: 10.1128/mBio.01885-21
Cafaro, M. J., Poulsen, M., Little, A. E. F., Price, S. L., Gerardo, N. M., Wong, B., et al. (2011). Specificity in the symbiotic association between fungus-growing ants and protective Pseudonocardia bacteria. Proc. R. Soc. B Biol. Sci. 278, 1814–1822. doi: 10.1098/rspb.2010.2118
Caldera, E. J., and Currie, C. R. (2012). The population structure of antibiotic-producing bacterial symbionts of Apterostigma dentigerum ants: impacts of coevolution and multipartite symbiosis. Am. Nat. 180, 604–617. doi: 10.1086/667886
Cao, T., Mu, S., Lu, C., Zhao, S., Li, D., Yan, K., et al. (2017). Streptomyces amphotericinicus sp. nov., an amphotericin-producing actinomycete isolated from the head of an ant (Camponotus japonicus Mayr). Int. J. Syst. Evol. Microbiol. 67, 4967–4973. doi: 10.1099/ijsem.0.002382
Carro, L., Pujic, P., Trujillo, M. E., and Normand, P. (2013). Micromonospora is a normal occupant of actinorhizal nodules. J. Biosci. 38, 685–693. doi: 10.1007/s12038-013-9359-y
CDC (2019). Antibiotic Resistance Threats in the United States, 2019. Atlanta, GA: U.S. Department of Health and Human Services, CDC.
Chang, P. T., Rao, K., Longo, L. O., Lawton, E. S., Scherer, G., and Van Arnam, E. B. (2020). Thiopeptide defense by an ant’s bacterial symbiont. J. Nat. Prod. 83, 725–729. doi: 10.1021/acs.jnatprod.9b00897
Chevrette, M. G., Carlson, C. M., Ortega, H. E., Thomas, C., Ananiev, G. E., Barns, K. J., et al. (2019). The antimicrobial potential of Streptomyces from insect microbiomes. Nat. Commun. 10:516.
Chomicki, G., Thorogood, C. J., Naikatini, A., and Renner, S. S. (2019). Squamellaria: plants domesticated by ants. Plants People Planet 1, 302–305. doi: 10.1002/ppp3.10072
Chomicki, G., Ward, P. S., and Renner, S. S. (2015). Macroevolutionary assembly of ant/plant symbioses: Pseudomyrmex ants and their ant-housing plants in the Neotropics. Proc. R. Soc. B Biol. Sci. 282:20152200.
Currie, C. R., Bot, A. N. M., and Boomsma, J. J. (2003). Experimental evidence of a tripartite mutualism: bacteria protect ant fungus gardens from specialized parasites. Oikos 101, 91–102. doi: 10.1034/j.1600-0706.2003.12036.x
Currie, C. R., Poulsen, M., Mendenhall, J., Boomsma, J. J., and Billen, J. (2006). Coevolved crypts and exocrine glands support mutualistic bacteria in fungus-growing ants. Science 311, 81–83. doi: 10.1126/science.1119744
Currie, C. R., Scott, J. A., Summerbell, R. C., and Malloch, D. (1999). Fungus-growing ants use antibiotic-producing bacteria to control garden parasites. Nature 398, 701–704. doi: 10.1038/19519
Currie, C. R., and Stuart, A. E. (2001). Weeding and grooming of pathogens in agriculture by ants. Proc. R. Soc. B Biol. Sci. 268, 1033–1039. doi: 10.1098/rspb.2001.1605
Dângelo, R. A. C., de Souza, D. J., Mendes, T. D., Couceiro, J. D. C., and Lucia, T. M. C. D. (2016). Actinomycetes inhibit filamentous fungi from the cuticle of Acromyrmex leafcutter ants. J. Basic Microbiol. 56, 229–237. doi: 10.1002/jobm.201500593
Diarra, U., and Osborne-Naikatini, T. (2023). Isolation, characterization and screening of actinomycetes associated with fijian ant-plant symbioses. Microbiol. Read. Engl. 169:001410.
Douglas, A. E. (2011). Lessons from studying insect symbioses. Cell Host Microbe 10, 359–367. doi: 10.1016/j.chom.2011.09.001
Douglas, A. E. (2015). Multiorganismal insects: diversity and function of resident microorganisms. Annu. Rev. Entomol. 60, 17–34. doi: 10.1146/annurev-ento-010814-020822
Douglas, A. E. (2022). Insects and Their Beneficial Microbes. Princeton, NJ: Princeton University Press.
Du, Y. E., Byun, W. S., Lee, S. B., Hwang, S., Shin, Y.-H., Shin, B., et al. (2020). Formicins, N-Acetylcysteamine-bearing indenone thioesters from a wood ant-associated bacterium. Org. Lett. 22, 5337–5341. doi: 10.1021/acs.orglett.0c01584
Efimenko, T. A., Glukhova, A. A., Demiankova, M. V., Boykova, Y. V., Malkina, N. D., Sumarukova, I. G., et al. (2020). Antimicrobial activity of microorganisms isolated from ant nests of Lasius niger. Life 10:91. doi: 10.3390/life10060091
Engel, P., and Moran, N. A. (2013). The gut microbiota of insects – diversity in structure and function. FEMS Microbiol. Rev. 37, 699–735. doi: 10.1111/1574-6976.12025
Engl, T., Kroiss, J., Kai, M., Nechitaylo, T. Y., Svatoš, A., and Kaltenpoth, M. (2018). Evolutionary stability of antibiotic protection in a defensive symbiosis. Proc. Natl. Acad. Sci. 115, E2020–E2029.
Evans, H. C., Elliot, S. L., and Hughes, D. P. (2011). Ophiocordyceps unilateralis. Commun. Integr. Biol. 4, 598–602. doi: 10.4161/cib.4.5.16721
Fernández-Marín, H., Zimmerman, J. K., Nash, D. R., Boomsma, J. J., and Wcislo, W. T. (2009). Reduced biological control and enhanced chemical pest management in the evolution of fungus farming in ants. Proc. Biol. Sci. 276, 2263–2269. doi: 10.1098/rspb.2009.0184
Fukatsu, T., and Hosokawa, T. (2002). Capsule-transmitted gut symbiotic bacterium of the Japanese common plataspid stinkbug, Megacopta punctatissima. Appl. Environ. Microbiol. 68, 389–396. doi: 10.1128/AEM.68.1.389-396.2002
Fukuda, T. T. H., Helfrich, E. J. N., Mevers, E., Melo, W. G. P., Van Arnam, E. B., Andes, D. R., et al. (2021). Specialized metabolites reveal evolutionary history and geographic dispersion of a multilateral symbiosis. ACS Cent. Sci. 7, 292–299. doi: 10.1021/acscentsci.0c00978
Goettler, W., Kaltenpoth, M., McDonald, S., and Strohm, E. (2022). Comparative morphology of the symbiont cultivation glands in the antennae of female digger wasps of the Genus Philanthus (Hymenoptera: Crabronidae). Front. Physiol. 13:815494. doi: 10.3389/fphys.2022.815494
Goldstein, S. L., and Klassen, J. L. (2020). Pseudonocardia symbionts of fungus-growing ants and the evolution of defensive secondary metabolism. Front. Microbiol. 11:3341. doi: 10.3389/fmicb.2020.621041
Gomes, B., and Noll, F. B. (2009). Diversity of social wasps (Hymenoptera, Vespidae, Polistinae) in three fragments of semideciduous seasonal forest in the northwest of São Paulo State, Brazil. Rev. Bras. Entomol. 53, 428–431.
Gotting, K., May, D. S., Sosa-Calvo, J., Khadempour, L., Francoeur, C. B., Berasategui, A., et al. (2022). Genomic diversification of the specialized parasite of the fungus-growing ant symbiosis. Proc. Natl. Acad. Sci. 119:e2213096119. doi: 10.1073/pnas.2213096119
Grubbs, K. J., May, D. S., Sardina, J. A., Dermenjian, R. K., Wyche, T. P., Pinto-Tomás, A. A., et al. (2021). Pollen streptomyces produce antibiotic that inhibits the honey bee pathogen Paenibacillus larvae. Front. Microbiol. 12:632637. doi: 10.3389/fmicb.2021.632637
Guo, L., Liu, C., Zhao, J., Li, C., Guo, S., Fan, J., et al. (2016). Promicromonospora alba sp. nov., an actinomycete isolated from the cuticle of Camponotus japonicas Mayr. Int. J. Syst. Evol. Microbiol. 66, 1340–1345. doi: 10.1099/ijsem.0.000885
Han, C., Liu, C., Zhao, J., Guo, L., Lu, C., Li, J., et al. (2016). Microbispora camponoti sp. nov., a novel actinomycete isolated from the cuticle of Camponotus japonicus Mayr. Antonie Van Leeuwenhoek 109, 215–223. doi: 10.1007/s10482-015-0625-x
Hansen, K. A., Kim, R. R., Lawton, E. S., Tran, J., Lewis, S. K., Deol, A. S., et al. (2022). Bacterial associates of a desert specialist fungus-growing ant antagonize competitors with a nocamycin analog. ACS Chem. Biol. 17, 1824–1830. doi: 10.1021/acschembio.2c00187
Hanshew, A. S., McDonald, B. R., Díaz Díaz, C., Djiéto-Lordon, C., Blatrix, R., and Currie, C. R. (2015). Characterization of Actinobacteria associated with three ant–plant mutualisms. Microb. Ecol. 69, 192–203. doi: 10.1007/s00248-014-0469-3
Höcherl, N., Kennedy, S., and Tautz, J. (2016). Nest thermoregulation of the paper wasp Polistes dominula. J. Therm. Biol. 60, 171–179. doi: 10.1016/j.jtherbio.2016.07.012
Hoggard, S. J., Wilson, P. D., Beattie, A. J., and Stow, A. J. (2011). Social complexity and nesting habits are factors in the evolution of antimicrobial defences in wasps. PLoS One 6:e21763. doi: 10.1371/journal.pone.0021763
Hong, S.-H., Ban, Y. H., Byun, W. S., Kim, D., Jang, Y.-J., An, J. S., et al. (2019). Camporidines A and B: Antimetastatic and anti-inflammatory polyketide alkaloids from a gut bacterium of Camponotus kiusiuensis. J. Nat. Prod. 82, 903–910. doi: 10.1021/acs.jnatprod.8b01000
Hosmath, K., and Timmappa, S. (2019). Comparison between the microbial diversity in carpenter ant (Camponotus) gut and weaver ant (Oecophylla) gut. J. Pure Appl. Microbiol. 13, 2421–2436. doi: 10.22207/JPAM.13.4.58
Houadria, M., Salas-Lopez, A., Orivel, J., Blüthgen, N., and Menzel, F. (2015). Dietary and temporal niche differentiation in tropical ants—can they explain local ant coexistence? Biotropica 47, 208–217.
Hrncir, M., Jarau, S., and Barth, F. G. (2016). Stingless bees (Meliponini): senses and behavior. J. Comp. Physiol. A 202, 597–601. doi: 10.1007/s00359-016-1117-9
Hyodo, F., Takematsu, Y., Matsumoto, T., Inui, Y., and Itioka, T. (2011). Feeding habits of Hymenoptera and Isoptera in a tropical rain forest as revealed by nitrogen and carbon isotope ratios. Insectes Sociaux 58, 417–426.
Jiang, S., Piao, C., Yu, Y., Cao, P., Li, C., Yang, F., et al. (2018). Streptomyces capitiformicae sp. nov., a novel actinomycete producing angucyclinone antibiotics isolated from the head of Camponotus japonicus Mayr. Int. J. Syst. Evol. Microbiol. 68, 118–124. doi: 10.1099/ijsem.0.002468
Jiménez-Gómez, I., Barcoto, M. O., Montoya, Q. V., Goes, A. C., Monteiro, L. S. V. E., Bueno, O. C., et al. (2021). Host susceptibility modulates escovopsis pathogenic potential in the fungiculture of higher attine ants. Front. Microbiol. 12:673444. doi: 10.3389/fmicb.2021.673444
Jose, P. A., Maharshi, A., and Jha, B. (2021). Actinobacteria in natural products research: progress and prospects. Microbiol. Res. 246:126708. doi: 10.1016/j.micres.2021.126708
Kadri, S. S. (2020). Key takeaways from the U.S. CDC’s 2019 antibiotic resistance threats report for frontline providers. Crit. Care Med. 48, 939–945. doi: 10.1097/CCM.0000000000004371
Kaltenpoth, M. (2009). Actinobacteria as mutualists: general healthcare for insects? Trends Microbiol. 17, 529–535. doi: 10.1016/j.tim.2009.09.006
Kaltenpoth, M., Göttler, W., Herzner, G., and Strohm, E. (2005). Symbiotic bacteria protect wasp larvae from fungal infestation. Curr. Biol. 15, 475–479. doi: 10.1016/j.cub.2004.12.084
Kaltenpoth, M., Yildirim, E., Gürbüz, M. F., Herzner, G., and Strohm, E. (2012). Refining the roots of the beewolf-streptomyces symbiosis: antennal symbionts in the rare genus Philanthinus (Hymenoptera. Crabronidae). Appl. Environ. Microbiol. 78, 822–827.
Koehler, S. (2014). Protective Streptomyces in Beewolves: Ecology, Evolutionary History and Specificity of Symbiont-Mediated Defense in Philanthini wasps (Hymenoptera, Crabronidae). Available online at: https://www.semanticscholar.org/paper/Protective-Streptomyces-in-beewolves-%3A-ecology%2C-and-Koehler/969bab39edfcbe74644076f323e04de74b065644 (accessed 16 February, 2023).
Koehler, S., Doubský, J., and Kaltenpoth, M. (2013). Dynamics of symbiont-mediated antibiotic production reveal efficient long-term protection for beewolf offspring. Front. Zool. 10:3. doi: 10.1186/1742-9994-10-3
Kroiss, J., Kaltenpoth, M., Schneider, B., Schwinger, M.-G., Hertweck, C., Maddula, R. K., et al. (2010). Symbiotic Streptomycetes provide antibiotic combination prophylaxis for wasp offspring. Nat. Chem. Biol. 6, 261–263. doi: 10.1038/nchembio.331
Kumar, V., Bharti, A., Gupta, V. K., Gusain, O., and Bisht, G. S. (2012). Actinomycetes from solitary wasp mud nest and swallow bird mud nest: isolation and screening for their antibacterial activity. World J. Microbiol. Biotechnol. 28, 871–880. doi: 10.1007/s11274-011-0884-2
Li, G.-L., Xu, Z.-Y., Li, N., Wang, Z., Tian, T., and Shen, T. (2020). Cornifronone: a cadinane-type sesquiterpene from a mason bee (Osmia cornifrons)–derived Streptomyces sp. J. Chem. Res. 44, 695–698. doi: 10.1177/1747519820918330
Li, H., Sosa-Calvo, J., Horn, H. A., Pupo, M. T., Clardy, J., Rabeling, C., et al. (2018). Convergent evolution of complex structures for ant-bacterial defensive symbiosis in fungus-farming ants. Proc. Natl. Acad. Sci. U. S. A. 115, 10720–10725. doi: 10.1073/pnas.1809332115
Li, Y., Ye, L., Wang, X., Zhao, J., Ma, Z., Yan, K., et al. (2016). Streptomyces camponoticapitis sp. nov., an actinomycete isolated from the head of an ant (Camponotus japonicus Mayr). Int. J. Syst. Evol. Microbiol. 66, 3855–3859. doi: 10.1099/ijsem.0.001276
Little, A. E. F., and Currie, C. R. (2007). Symbiotic complexity: discovery of a fifth symbiont in the attine ant–microbe symbiosis. Biol. Lett. 3, 501–504. doi: 10.1098/rsbl.2007.0253
Little, A. E. F., and Currie, C. R. (2008). Black yeast symbionts compromise the efficiency of antibiotic defenses in fungus-growing ants. Ecology 89, 1216–1222. doi: 10.1890/07-0815.1
Liu, C., Bai, L., Ye, L., Zhao, J., Yan, K., Xiang, W., et al. (2016a). Nocardia lasii sp. nov., a novel actinomycete isolated from the cuticle of an ant (Lasius fuliginosus L). Antonie Van Leeuwenhoek 109, 1513–1520. doi: 10.1007/s10482-016-0753-y
Liu, C., Guan, X., Li, Y., Li, W., Ye, L., Kong, X., et al. (2016b). Nocardia camponoti sp. nov., an actinomycete isolated from the head of an ant (Camponotus japonicas Mayr). Int. J. Syst. Evol. Microbiol. 66, 1900–1905. doi: 10.1099/ijsem.0.000963
Liu, C., Li, Y., Ye, L., Zhao, J., Piao, C., Li, Z., et al. (2016c). Actinocorallia lasiicapitis sp. nov., an actinomycete isolated from the head of an ant (Lasius fuliginosus L.). Int. J. Syst. Evol. Microbiol. 66, 2172–2177. doi: 10.1099/ijsem.0.001005
Liu, C., Liu, S.-H., Zhao, J.-W., Zhang, J., Wang, X.-J., Li, J.-S., et al. (2016d). A new spectinabilin derivative with cytotoxic activity from ant-derived Streptomyces sp. 1H-GS5. J. Asian Nat. Prod. Res. 19, 924–929. doi: 10.1080/10286020.2016.1254200
Liu, C., Han, C., Jiang, S., Zhao, X., Tian, Y., Yan, K., et al. (2018). Streptomyces lasii sp. nov., a novel actinomycete with antifungal activity isolated from the head of an ant (Lasius flavus). Curr. Microbiol. 75, 353–358. doi: 10.1007/s00284-017-1388-6
Liu, S., Xu, M., Zhang, H., Qi, H., Zhang, J., Liu, C., et al. (2015). New cytotoxic spectinabilin derivative from ant-associated Streptomyces sp. 1H-GS5. J. Antibiot. 69, 128–131. doi: 10.1038/ja.2015.99
Locci, R. (1994). Actinomycetes as plant pathogens. Eur. J. Plant Pathol. 100, 179–200. doi: 10.1007/BF01876235
Long, Y., Zhang, Y., Huang, F., Liu, S., Gao, T., and Zhang, Y. (2022). Diversity and antimicrobial activities of culturable actinomycetes from Odontotermes formosanus (Blattaria: Termitidae). BMC Microbiol. 22:80. doi: 10.1186/s12866-022-02501-5
Lu, D.-D., Ren, J.-W., Du, Q.-Q., Song, Y.-J., Lin, S.-Q., Li, X., et al. (2019). p-Terphenyls and actinomycins from a Streptomyces sp. associated with the larva of mud dauber wasp. Nat. Prod. Res. 35, 1869–1873.
Maák, I., Tóth, E., Lenda, M., Lőrinczi, G., Kiss, A., Juhász, O., et al. (2020). Behaviours indicating cannibalistic necrophagy in ants are modulated by the perception of pathogen infection level. Sci. Rep. 10:17906.
Madden, A. A., Grassetti, A., Soriano, J.-A. N., and Starks, P. T. (2013). Actinomycetes with antimicrobial activity isolated from paper wasp (Hymenoptera: Vespidae: Polistinae) nests. Environ. Entomol. 42, 703–710. doi: 10.1603/EN12159
Malmierca, M. G., González-Montes, L., Pérez-Victoria, I., Sialer, C., Braña, A. F., García Salcedo, R., et al. (2018). Searching for glycosylated natural products in actinomycetes and identification of novel macrolactams and angucyclines. Front. Microbiol. 9:39. doi: 10.3389/fmicb.2018.00039
Matarrita-Carranza, B., Moreira-Soto, R. D., Murillo-Cruz, C., Mora, M., Currie, C. R., and Pinto-Tomas, A. A. (2017). Evidence for widespread associations between neotropical hymenopteran insects and actinobacteria. Front. Microbiol. 8:2016. doi: 10.3389/fmicb.2017.02016
Matarrita-Carranza, B., Murillo-Cruz, C., Avendaño, R., Ríos, M. I., Chavarría, M., Gómez-Calvo, M. L., et al. (2021). Streptomyces sp. M54: an actinobacteria associated with a neotropical social wasp with high potential for antibiotic production. Antonie Van Leeuwenhoek 114, 379–398. doi: 10.1007/s10482-021-01520-y
Matsumoto, A., and Takahashi, Y. (2017). Endophytic actinomycetes: promising source of novel bioactive compounds. J. Antibiot. 70, 514–519. doi: 10.1038/ja.2017.20
Mehdiabadi, N. J., Mueller, U. G., Brady, S. G., Himler, A. G., and Schultz, T. R. (2012). Symbiont fidelity and the origin of species in fungus-growing ants. Nat. Commun. 3:840.
Meirelles, L. A., Mendes, T. D., Solomon, S. E., Bueno, O. C., Pagnocca, F. C., and Rodrigues, A. (2014). Broad Escovopsis-inhibition activity of Pseudonocardia associated with Trachymyrmex ants. Environ. Microbiol. Rep. 6, 339–345. doi: 10.1111/1758-2229.12132
Menegatti, C., Lourenzon, V. B., Rodríguez-Hernández, D., da Paixão Melo, W. G., and Ferreira, L. L. G. (2020). Meliponamycins: antimicrobials from stingless bee-associated Streptomyces sp. J. Nat. Prod. 83, 610–616. doi: 10.1021/acs.jnatprod.9b01011
Montoya, Q. V., Martiarena, M. J. S., Bizarria, R., Gerardo, N. M., and Rodrigues, A. (2021). Fungi inhabiting attine ant colonies: reassessment of the genus Escovopsis and description of Luteomyces and Sympodiorosea gens. nov. IMA Fungus 12:23. doi: 10.1186/s43008-021-00078-8
Mueller, U. G. (2012). Symbiont recruitment versus ant-symbiont co-evolution in the attine ant–microbe symbiosis. Curr. Opin. Microbiol. 15, 269–277. doi: 10.1016/j.mib.2012.03.001
Mueller, U. G., Kardish, M. R., Ishak, H. D., Wright, A. M., Solomon, S. E., Bruschi, S. M., et al. (2018). Phylogenetic patterns of ant–fungus associations indicate that farming strategies, not only a superior fungal cultivar, explain the ecological success of leafcutter ants. Mol. Ecol. 27, 2414–2434. doi: 10.1111/mec.14588
Nageh Sholkamy, E., Sayed Ahmed, M., Mohamed Yasser, M., and Mostafa, A. A. (2020). Antimicrobial quercetin 3-O-glucoside derivative isolated from Streptomyces antibioticus strain ess_amA8. J. King Saud Univ. Sci. 32, 1838–1844. doi: 10.1016/j.jksus.2020.01.026
Nechitaylo, T. Y., Westermann, M., and Kaltenpoth, M. (2014). Cultivation reveals physiological diversity among defensive ‘Streptomyces philanthi’ symbionts of beewolf digger wasps (Hymenoptera, Crabronidae). BMC Microbiol. 14:202. doi: 10.1186/s12866-014-0202-x
Ngalimat, M. S., Raja Abd Rahman, R. N. Z., Yusof, M. T., Syahir, A., and Sabri, S. (2019). Characterisation of bacteria isolated from the stingless bee, Heterotrigona itama, honey, bee bread and propolis. PeerJ 7:e7478. doi: 10.7717/peerj.7478
Oh, D.-C., Poulsen, M., Currie, C. R., and Clardy, J. (2009). Dentigerumycin: a bacterial mediator of an ant-fungus symbiosis. Nat. Chem. Biol. 5, 391–393. doi: 10.1038/nchembio.159
Oh, D.-C., Poulsen, M., Currie, C. R., and Clardy, J. (2011). Sceliphrolactam, a polyene macrocyclic lactam from a wasp-associated Streptomyces sp. Org. Lett. 13, 752–755. doi: 10.1021/ol102991d
Oliveira, T. B. D., Ferro, M., Bacci, M., Souza, D. J. D., Fontana, R., Delabie, J. H. C., et al. (2016). Bacterial communities in the midgut of Ponerine ants (Hymenoptera: Formicidae: Ponerinae). Sociobiology 63, 637–644. doi: 10.13102/sociobiology.v63i1.882
Orivel, J., Malé, P.-J., Lauth, J., Roux, O., Petitclerc, F., Dejean, A., et al. (2017). Trade-offs in an ant–plant–fungus mutualism. Proc. R. Soc. B Biol. Sci. 284:20161679. doi: 10.1098/rspb.2016.1679
Ortega, H. E., Lourenzon, V. B., Chevrette, M. G., Ferreira, L. L. G., Alvarenga, R. F. R., Melo, W. G. P., et al. (2021). Antileishmanial macrolides from ant-associated Streptomyces sp. ISID311. Bioorg. Med. Chem. 32:116016. doi: 10.1016/j.bmc.2021.116016
Patil, P. B., Zeng, Y., Coursey, T., Houston, P., Miller, I., and Chen, S. (2010). Isolation and characterization of a Nocardiopsis sp. from honeybee guts. FEMS Microbiol. Lett. 312, 110–118. doi: 10.1111/j.1574-6968.2010.02104.x
Piao, C., Zheng, W., Li, Y., Liu, C., Jin, L., Song, W., et al. (2017). Two new species of the genus Streptomyces: Streptomyces camponoti sp. nov. and Streptomyces cuticulae sp. nov. isolated from the cuticle of Camponotus japonicus Mayr. Arch. Microbiol. 199, 963–970. doi: 10.1007/s00203-017-1353-6
Pohl, S., Frederickson, M. E., Elgar, M. A., and Pierce, N. E. (2016). Colony diet influences ant worker foraging and attendance of Myrmecophilous Lycaenid caterpillars. Front. Ecol. Evol. 4:114. doi: 10.3389/fevo.2016.00114
Poulsen, M., Bot, A. N. M., Currie, C. R., Nielsen, M. G., and Boomsma, J. J. (2003). Within-colony transmission and the cost of a mutualistic bacterium in the leaf-cutting ant Acromyrmex octospinosus. Funct. Ecol. 17, 260–269. doi: 10.1046/j.1365-2435.2003.00726.x
Poulsen, M., Cafaro, M. J., Erhardt, D. P., Little, A. E. F., Gerardo, N. M., Tebbets, B., et al. (2010). Variation in Pseudonocardia antibiotic defence helps govern parasite-induced morbidity in Acromyrmex leaf-cutting ants. Environ. Microbiol. Rep. 2, 534–540. doi: 10.1111/j.1758-2229.2009.00098.x
Poulsen, M., Oh, D.-C., Clardy, J., and Currie, C. R. (2011). Chemical analyses of wasp-associated streptomyces bacteria reveal a prolific potential for natural products discovery. PLoS One 6:e16763. doi: 10.1371/journal.pone.0016763
Powell, E. C., and Taylor, L. A. (2017). Specialists and generalists coexist within a population of spider-hunting mud dauber wasps. Behav. Ecol. 28, 890–898. doi: 10.1093/beheco/arx050
Prado-Alonso, L., Pérez-Victoria, I., Malmierca, M. G., Montero, I., Rioja-Blanco, E., Martín, J., et al. (2022). Colibrimycins, novel halogenated hybrid polyketide synthase-nonribosomal peptide synthetase (PKS-NRPS) compounds produced by Streptomyces sp. strain CS147. Appl. Environ. Microbiol. 88:e0183921. doi: 10.1128/AEM.01839-21
Prato, A., Silva, R. C. D., Mateus, S., and Nascimento, F. S. (2022). A technique for transferring nests of polybia (Hymenoptera: Vespidae: Epiponini) wasps in anthropized environment. Sociobiology 69:e7620
Prestinaci, F., Pezzotti, P., and Pantosti, A. (2015). Antimicrobial resistance: a global multifaceted phenomenon. Pathog. Glob. Health 109, 309–318. doi: 10.1179/2047773215Y.0000000030
Promnuan, Y., Kudo, T., and Chantawannakul, P. (2009). Actinomycetes isolated from beehives in Thailand. World J. Microbiol. Biotechnol. 25, 1685–1689. doi: 10.1007/s11274-009-0051-1
Promnuan, Y., Kudo, T., Ohkuma, M., and Chantawannakul, P. (2011). Actinomadura apis sp. nov., isolated from a honey bee (Apis mellifera) hive, and the reclassification of Actinomadura cremea subsp. rifamycini Gauze et al. 1987 as Actinomadura rifamycini (Gauze et al. 1987) sp. nov., comb. nov. Int. J. Syst. Evol. Microbiol. 61, 2271–2277. doi: 10.1099/ijs.0.026633-0
Promnuan, Y., Kudo, T., Ohkuma, M., and Chantawannakul, P. (2013). Streptomyces chiangmaiensis sp. nov. and Streptomyces lannensis sp. nov., isolated from the South-East Asian stingless bee (Tetragonilla collina). Int. J. Syst. Evol. Microbiol. 63, 1896–1901. doi: 10.1099/ijs.0.045930-0
Promnuan, Y., Promsai, S., Pathom-aree, W., and Meelai, S. (2021). Apis andreniformis associated Actinomycetes show antimicrobial activity against black rot pathogen (Xanthomonas campestris pv. campestris). PeerJ 9:e12097. doi: 10.7717/peerj.12097
Qin, Z., Munnoch, J. T., Devine, R., Holmes, N. A., Seipke, R. F., Wilkinson, K. A., et al. (2017). Formicamycins, antibacterial polyketides produced by Streptomyces formicae isolated from African Tetraponera plant-ants. Chem. Sci. 8, 3218–3227. doi: 10.1039/C6SC04265A
Reyes, R. D. H., and Cafaro, M. J. (2015). Paratrechina longicornis ants in a tropical dry forest harbor specific Actinobacteria diversity. J. Basic Microbiol. 55, 11–21. doi: 10.1002/jobm.201300785
Rodríguez-Hernández, D., Melo, W. G. P., Menegatti, C., Lourenzon, V. B., Nascimento, F. S., and Pupo, M. T. (2019). Actinobacteria associated with stingless bees biosynthesize bioactive polyketides against bacterial pathogens. New J. Chem. 43, 10109–10117. doi: 10.1039/C9NJ01619H
Ruiz-González, M. X., Malé, P.-J. G., Leroy, C., Dejean, A., Gryta, H., Jargeat, P., et al. (2011). Specific, non-nutritional association between an ascomycete fungus and Allomerus plant-ants. Biol. Lett. 7, 475–479. doi: 10.1098/rsbl.2010.0920
Seipke, R. F., Barke, J., Heavens, D., Yu, D. W., and Hutchings, M. I. (2013). Analysis of the bacterial communities associated with two ant–plant symbioses. MicrobiologyOpen 2, 276–283.
Seipke, R. F., Kaltenpoth, M., and Hutchings, M. I. (2012). Streptomyces as symbionts: an emerging and widespread theme? FEMS Microbiol. Rev. 36, 862–876.
Seyedsayamdost, M. R., Traxler, M.F., Clardy, J., and Kolter, R. (2012) “Old meets new: using interspecies interactions to detect secondary metabolite production in actinomycetes.” in Methods in enzymology, vol. 517, (Academic Press), 89–109. doi: 10.1016/B978-0-12-404634-4.00005-X
Shik, J. Z., Kooij, P. W., Donoso, D. A., Santos, J. C., Gomez, E. B., Franco, M., et al. (2021). Nutritional niches reveal fundamental domestication trade-offs in fungus-farming ants. Nat. Ecol. Evol. 5, 122–134. doi: 10.1038/s41559-020-01314-x
Singh, S., Orr, D., Divinagracia, E., McGraw, J., Dorff, K., and Forst, S. (2015). Role of secondary metabolites in establishment of the mutualistic partnership between Xenorhabdus nematophila and the entomopathogenic nematode Steinernema carpocapsae. Appl. Environ. Microbiol. 81, 754–764. doi: 10.1128/AEM.02650-14
Sit, C. S., Ruzzini, A. C., Van Arnam, E. B., Ramadhar, T. R., Currie, C. R., and Clardy, J. (2015). Variable genetic architectures produce virtually identical molecules in bacterial symbionts of fungus-growing ants. Proc. Natl. Acad. Sci. U. S. A. 112, 13150–13154. doi: 10.1073/pnas.1515348112
Solomon, S. E., Lopes, C. T., Mueller, U. G., Rodrigues, A., Sosa-Calvo, J., Schultz, T. R., et al. (2011). Nesting biology and fungiculture of the fungus-growing ant, Mycetagroicus cerradensis: new light on the origin of higher attine agriculture. J. Insect Sci. 11:12. doi: 10.1673/031.011.0112
Song, Y.-J., Zheng, H.-B., Peng, A.-H., Ma, J.-H., Lu, D.-D., Li, X., et al. (2019). Strepantibins A–C: Hexokinase II inhibitors from a mud dauber wasp associated Streptomyces sp. J. Nat. Prod. 82, 1114–1119. doi: 10.1021/acs.jnatprod.8b00821
Stephan, J. G., de Miranda, J. R., and Forsgren, E. (2020). American foulbrood in a honeybee colony: spore-symptom relationship and feedbacks between disease and colony development. BMC Ecol. 20:15. doi: 10.1186/s12898-020-00283-w
Strohm, E., Herzner, G., Ruther, J., Kaltenpoth, M., and Engl, T. (2019). Nitric oxide radicals are emitted by wasp eggs to kill mold fungi. eLife 8:e43718. doi: 10.7554/eLife.43718
Subramani, R., and Sipkema, D. (2019). Marine rare actinomycetes: a promising source of structurally diverse and unique novel natural products. Mar. Drugs 17:249. doi: 10.3390/md17050249
Tufts, D. M., and Bextine, B. (2009). Identification of bacterial species in the hemolymph of queen Solenopsis invicta (Hymenoptera: Formicidae). Environ. Entomol. 38, 1360–1364. doi: 10.1603/022.038.0502
Urbini, A., Sparvoli, E., and Turillazzi, S. (2006). Social paper wasps as bioindicators: a preliminary research with Polistes dominulus (Hymenoptera Vespidae) as a trace metal accumulator. Chemosphere 64, 697–703. doi: 10.1016/j.chemosphere.2005.11.009
Van Arnam, E. B., Ruzzini, A. C., Sit, C. S., Currie, C. R., and Clardy, J. (2015). A rebeccamycin analog provides plasmid-encoded niche defense. J. Am. Chem. Soc. 137, 14272–14274. doi: 10.1021/jacs.5b09794
Van Arnam, E. B., Ruzzini, A. C., Sit, C. S., Horn, H., Pinto-Tomás, A. A., Currie, C. R., et al. (2016). Selvamicin, an atypical antifungal polyene from two alternative genomic contexts. Proc. Natl. Acad. Sci. 113, 12940–12945. doi: 10.1073/pnas.1613285113
van der Meij, A., Worsley, S. F., Hutchings, M. I., and van Wezel, G. P. (2017). Chemical ecology of antibiotic production by actinomycetes. FEMS Microbiol. Rev. 41, 392–416. doi: 10.1093/femsre/fux005
Van Moll, L., De Smet, J., Cos, P., and Van Campenhout, L. (2021). Microbial symbionts of insects as a source of new antimicrobials: a review. Crit. Rev. Microbiol. 47, 562–579. doi: 10.1080/1040841X.2021.1907302
Vikeli, E., Widdick, D. A., Batey, S. F. D., Heine, D., Holmes, N. A., Bibb, M. J., et al. (2020). In situ activation and heterologous production of a cryptic lantibiotic from an african plant ant-derived saccharopolyspora species. Appl. Environ. Microbiol. 86:e01876-19.
Wang, Z., Peng, A.-H., Lu, D.-D., Song, Y.-J., Wang, C.-L., and Xie, W.-D. (2018). Manumycin-type derivatives from a Streptomyces sp. ASSOCIATED WITH MESON Bee Osmia cornifrons. Nat. Prod. Commun. 13, 673–676.
Wang, Z., Yu, Z., Zhao, J., Zhuang, X., Cao, P., Guo, X., et al. (2020). Community composition, antifungal activity and chemical analyses of ant-derived actinobacteria. Front. Microbiol. 11:201. doi: 10.3389/fmicb.2020.00201
Ward, P. S. (2021). “Pseudomyrmecinae,” in Encyclopedia of Social Insects, ed. C. K. Starr (Cham: Springer).
Ward, P. S., Blaimer, B. B., and Fisher, B. L. (2016). A revised phylogenetic classification of the ant subfamily Formicinae (Hymenoptera: Formicidae), with resurrection of the genera Colobopsis and Dinomyrmex. Zootaxa 4072, 343–357. doi: 10.11646/zootaxa.4072.3.4
Wcislo, W. T. (1996). Parasitism rates in relation to nest site in bees and wasps (Hymenoptera: Apoidea). J. Insect Behav. 9, 643–656. doi: 10.1007/BF02213885
Wu, Y., Liu, Y., Yu, J., Xu, Y., and Chen, S. (2022). Observation of the antimicrobial activities of two actinomycetes in the harvester ant Messor orientalis. Insects 13: 691.
Xiang, W., Yu, C., Liu, C., Zhao, J., Yang, L., Xie, B., et al. (2014). Micromonospora polyrhachis sp. nov., an actinomycete isolated from edible Chinese black ant (Polyrhachis vicina Roger). Int. J. Syst. Evol. Microbiol. 64, 495–500. doi: 10.1099/ijs.0.055863-0
Yamamura, H., Miyazaki, S., Ikoma, K., Nakagawa, Y., Hamada, M., Otoguro, M., et al. (2017). Virgisporangium myanmarense sp. nov., a novel motile actinomycete isolated from an anthill soil in Myanmar. J. Antibiot. 70, 995–999. doi: 10.1038/ja.2017.81
Ye, L., Zhao, S., Li, Y., Jiang, S., Zhao, Y., Li, J., et al. (2017). Streptomyces lasiicapitis sp. nov., an actinomycete that produces kanchanamycin, isolated from the head of an ant (Lasius fuliginosus L.). Int. J. Syst. Evol. Microbiol. 67, 1529–1534. doi: 10.1099/ijsem.0.001756
Yu, C., Liu, C., Wang, X., Zhao, J., Yang, L., Gao, R., et al. (2013). Streptomyces polyrhachii sp. nov., a novel actinomycete isolated from an edible Chinese black ant (Polyrhachis vicina Roger). Antonie Van Leeuwenhoek 104, 1013–1019. doi: 10.1007/s10482-013-0021-3
Zakalyukina, Y. V., Birykov, M. V., Lukianov, D. A., Shiriaev, D. I., Komarova, E. S., Skvortsov, D. A., et al. (2019). Nybomycin-producing Streptomyces isolated from carpenter ant Camponotus vagus. Biochimie 160, 93–99. doi: 10.1016/j.biochi.2019.02.010
Zakalyukina, Y. V., Biryukov, M. V., Golichenkov, M. V., and Netrusov, A. I. (2017). Phenotypic and phylogenetic characterization of actinomycetes isolated from Lasius niger and Formica cunicularia ants. Mosc. Univ. Biol. Sci. Bull. 72, 13–19. doi: 10.3103/S0096392517010072
Zakalyukina, Y. V., Golichenkov, M. V., Brovkina, O. I., and Putyatina, T. S. (2014). Comparative study of actinomycete communities associated with Lasius niger and Formica cunicularia ants and their nests. Mosc. Univ. Biol. Sci. Bull. 69, 118–124. doi: 10.3103/S0096392514030109
Zakalyukina, Y. V., Osterman, I. A., Wolf, J., Neumann-Schaal, M., Nouioui, I., and Biryukov, M. V. (2022a). Amycolatopsis camponoti sp. nov., new tetracenomycin-producing actinomycete isolated from carpenter ant Camponotus vagus. Antonie Van Leeuwenhoek 115, 533–544. doi: 10.1007/s10482-022-01716-w
Zakalyukina, Y. V., Pavlov, N. A., Lukianov, D. A., Marina, V. I., Belozerova, O. A., Tashlitsky, V. N., et al. (2022b). A new albomycin-producing strain of Streptomyces globisporus subsp. globisporus may provide protection for ants Messor structor. Insects 13:1042. doi: 10.3390/insects13111042
Zakalyukina, Y. V., Zaytsev, A. R., and Biryukov, M. V. (2021). Study of cellulose-destroying activity of actinobacteria associated with ants. Vestn. Mosk. Univ. Seriya Biol. 76, 24–32.
Zheng, Z., Hu, X., Xu, Y., Wei, C., and He, H. (2021). Bacterial composition and diversity of the digestive tract of odontomachus monticola emery and Ectomomyrmex javanus Mayr. Insects 12:176. doi: 10.3390/insects12020176
Zientz, E., Beyaert, I., Gross, R., and Feldhaar, H. (2006). Relevance of the endosymbiosis of Blochmannia floridanus and carpenter ants at different stages of the life cycle of the host. Appl. Environ. Microbiol. 72, 6027–6033. doi: 10.1128/AEM.00933-06
Keywords: actinomycetes, hymenoptera, symbiosis, natural products, bioactive compounds
Citation: Diarra U, Osborne-Naikatini T and Subramani R (2024) Actinomycetes associated with hymenopteran insects: a promising source of bioactive natural products. Front. Microbiol. 15:1303010. doi: 10.3389/fmicb.2024.1303010
Received: 27 September 2023; Accepted: 05 February 2024;
Published: 28 February 2024.
Edited by:
Marcelino Gutiérrez, Instituto de Investigaciones Científicas y Servicios de Alta Tecnología, PanamaReviewed by:
Polpass Arul Jose, Harvard University, United StatesCopyright © 2024 Diarra, Osborne-Naikatini and Subramani. This is an open-access article distributed under the terms of the Creative Commons Attribution License (CC BY). The use, distribution or reproduction in other forums is permitted, provided the original author(s) and the copyright owner(s) are credited and that the original publication in this journal is cited, in accordance with accepted academic practice. No use, distribution or reproduction is permitted which does not comply with these terms.
*Correspondence: Umar Diarra, dXNkNjU5OEBnbWFpbC5jb20=
Disclaimer: All claims expressed in this article are solely those of the authors and do not necessarily represent those of their affiliated organizations, or those of the publisher, the editors and the reviewers. Any product that may be evaluated in this article or claim that may be made by its manufacturer is not guaranteed or endorsed by the publisher.
Research integrity at Frontiers
Learn more about the work of our research integrity team to safeguard the quality of each article we publish.