- 1College of Life Sciences, Shihezi University, Shihezi, Xinjiang, China
- 2College of Life Sciences and Food Engineering, Shaanxi Xueqian Normal University, Xi’an, Shaanxi, China
- 3Department of Plant Protection, College of Agriculture, Shihezi University, Shihezi, Xinjiang, China
Yeast is one of the important symbiotic flora in the insect gut. However, little is known about the gut yeast in Helicoverpa armigera (Lepidoptera: Noctuidae) under various dietary conditions. The composition and function of the intestinal yeast community also remain unclear. In this research, we explored the composition of yeast microorganisms in H. armigera larvae under different feeding environments, including apple, pear, tomato, artificial diet (laboratory feeding), Urtica fissa, Helianthus annuus, and Zinnia elegans (wild environment) using high-throughput sequencing. Results showed that a total of 43 yeast OTU readings were obtained, comprising 33 yeast genera and 42 yeast species. The yeast genera with a total content of more than 5% were Hanseniaspora (36.27%), Moesziomyces (21.47%), Trichosporon (16.20%), Wickerhamomyces (12.96%) and Pichia (6.38%). Hanseniaspora was predominant when fed indoors with fruits, whereas Moesziomyces was only detected in the wild group (Urtica fissa, Helianthus annuus, Zinnia elegans) and the artificial diet group. After transferring the larvae from artificial diet to apple, pear and tomato, the composition of intestinal yeast community changed, mainly reflected in the increased relative abundance of Hanseniaspora and the decreased abundance of Trichosporon. Simultaneously, the results of α diversity index indicated that the intestinal yeast microbial diversity of H. armigera fed on wild plants was higher than that of indoor artificial feeding. PCoA and PERMANOVA analysis concluded that there were significant differences in the gut yeast composition of H. armigera larvae on different diets. Our results confirmed that gut yeast communities of H. armigera can be influenced by host diets and may play an important role in host adaptation.
Introduction
The intestines of all metazoan contain an abundant number of commensal microorganisms (Shin et al., 2011; Douglas, 2018). In the long-term co-evolution, insects provide a stable living environment and necessary nutrients for gut microorganisms, while gut microorganisms are also conducive to the survival of insects, significantly impacting nutritional metabolism (Gong et al., 2020), growth and development (Lauzon et al., 2000; Yang et al., 2020), reproductive behavior (Yong et al., 2017), immune disease resistance (Johnston and Rolff, 2015; Su et al., 2019), and other life processes of insects, forming a mutually beneficial symbiotic relationship (Dillon and Dillon, 2004; Shapira, 2016). Gut microorganisms have been widely demonstrated to degrade exogenous toxins (Abdelgaffar et al., 2019; Yang et al., 2020) and affect the transmission efficiency of vector insects (Fukatsu and Hosokawa, 2002).
However, bacteria are not the only inhabitants of the insect gut (Gurung et al., 2019). Mycobiota, especially yeasts, have been shown to be associated with many insects. Yeasts dominate the flora of some insects and establish a symbiotic relationship with the host insects (Malassigné et al., 2021). It has been found that yeast or yeast-like symbionts in insect tissue are involved in amino acid and fatty acid metabolic pathways, and yeast deficiency leads to the instability of host ecosystem regulation and incomplete metamorphosis (Carvalho et al., 2010; Horgan et al., 2021). Insects also lack the ability to synthesize sterols, multivitamins, and enzymes that degrade plant cell wall materials and toxic plant equivalents (Martin, 1979). This deficiency can be compensated for by intestinal symbiotic yeasts (Vega, 2005). For example, in the ground-dwelling beetle family Silphidae, whose species consume animal carcasses (Scott, 1998), the yeast Yarrowia lipolytica and a related species group capable of breaking down diverse carbon sources, including hydrocarbons and lipids (Miller and Alper, 2019), have been isolated (Kaltenpoth and Steiger, 2014). Vogel et al. also suggested that Yarrowia species help inhibit the growth of other microbes by producing antimicrobial substance (Vogel et al., 2017). Therefore, similar to bacteria (Yuan et al., 2021), gut yeast may be the linchpin of the survival mechanism of insects on hosts.
Helicoverpa armigera (Lepidoptera: Noctuidae) is a worldwide pest known to infest over 200 host plants, including cotton, tomato, pepper, potato, and various other crops and wild plants (Zheng et al., 2022). It occurs frequently in large crop-growing countries such as China, India, and Australia. Cotton bollworm larvae mainly eat the buds, flowers and fruits of host plants. After the flower is damaged, the plant cannot be pollinated normally, resulting in reduced production of cash crops such as tomatoes and cotton and greatly reduced the commodity fruit (Tsafack et al., 2013; Gu et al., 2022). Due of its wide distribution, omnivorousness, fast reproduction and strong adaptability (Fitt, 1989), Helicoverpa armigera has caused huge economic losses to global agriculture (Gujar et al., 2021). In 1992, the cumulative occurrence of cotton bollworm in various crops in China reached 21.92 million hectares, causing direct economic losses of 6 billion yuan. The widespread use of conventional insecticides could lead to many problems, for examples, resistance to conventional insecticides, environmental pollution, human health impacts, and injury to beneficial insects. In order to reduce the economic losses caused by cotton bollworm, countries worldwide began to promote the cultivation of genetically modified crops that express the insecticidal protein of Bacillus thuringiensis (Bt). By 2012, the global area of genetically modified crops had reached 191.7 million hectares (James, 2013). However, with the large-scale cultivation of genetically modified crops, the frequency of resistance in cotton bollworm has significantly increased worldwide (Shelton et al., 2002; Jin et al., 2015; Ahmad et al., 2019). Research indicates that the development of cotton bollworm resistance is largely attributed to internal microorganisms and indigenous gut bacteria increasing the tolerance of larvae to B. thuringiensis toxin (Takatsuka and Kunimi, 2000; Dubovskiy et al., 2016). In addition, the resistance of larvae to the entomopathogenic bacterium B. thuringiensis is affected by the maturity of the host plants. Martemyanov’s study showed that the negative effects of B. thuringiensis were eliminated when the larvae fed on mature leaves (Martemyanov et al., 2016). Gut microbial communities play a crucial role in the evolution of insect populations resistant to Bt toxins (Paramasiva et al., 2014). Therefore, gut microorganism is an important entry point to reveal the adaptation mechanism between different hosts of H. armigera.
The composition of the gut microbiota of insects can be affected by many factors, such as host species, genotype, diet, and the host living environment. Diets with different nutritional compositions can affect the longevity of insects (Edgar et al., 2011) and diet is one of the most prominent factors in the formation of intestinal microbial communities (Harris et al., 2019; Moran et al., 2019). But as far as we know, when H. armigera feeds on different fruits and flowers, there are no reports on the composition of gut fungal community, especially the community of gut yeast. To explore the impact of diets on the gut yeast communities of larvaes of H. armigera, we study the composition of intestinal yeasts of H. armigera fed on artificial feed, apple, tomato, pear (laboratory feeding), Helianthus annuus,Urtica fissa and Zinnia elegans (wild environment) by sequencing technology based on D1/D2 domains of the large subunit rRNA gene (26S rRNA). The results revealed the changes of intestinal yeast community in different hosts, and speculated the potential relationship between H. armigera and intestinal yeast community, which provided a new idea for the prevention and control of H. armigera in agriculture.
Materials and methods
Insect sample acquisition
Laboratory-reared cotton bollworms were kept in the artificial feed, at 26 ± 0.5°C, 70 ± 10% relative humidity (Rh), and 15H: 9H photoperiod (L: D) for more than 3 generations at the College of Agriculture, Shehezi University (Yuan et al., 2021). The composition of the artificial feed is shown in Table 1. The fourth-generation eggs were fed artificial feed to the first instar larvae stage, and then transferred to three different fruits (apple, pear, and tomato) to the third instar larvae stage. Cotton bollworms that continued to be fed artificial feed were used as a control group. See Supplementary material 1 for specific feeding processes. Three host fruits were collected from the Botanical Garden of Shihezi University in 2021. Wild third instar larvae were collected from a habitat in Shihezi City (N:44°15′23.14″ E:86°03′9.68″). The larval host plants are Urtica fissa, Helianthus annuus, and Zinnia elegans, respectively.”
DNA extraction
Third instar larvae were first disinfected with 75% ethanol for 60 s, then with 0.5% sodium hypochlorite for 30 s, and finally washed with sterile water three times for 60 s (Wang et al., 2020). Guts were dissected with sterile clamp and washed again in sterile phosphate buffer saline (1 × PBS; pH:7.4). Thirty guts per treatment were collected into sterile centrifuge tubes (Anand et al., 2010), with three replicates per treatments. The FastDNA Spin Kit for Soil (MP Biomedicals, UAS) was used to extract the total DNA of samples.
PCR amplification and high-throughput sequencing
Yeast 26S rRNA was amplified using a pair of specific primers: NL1F (forward primer) (5′-GCATATCAATAAGCGGA GGAAAAG-3′) and NL2R (reverse primer) (5′-CTTGTTCGCTATCGGTCTC-3′) (Keidser et al., 2012). The target DNA bands were amplified on a PCR thermocycler (ABI, California, CA, United States) with an initial denaturation at 98°C for 5 min, followed by 30 cycles of denaturation at 98°C for 30 s, annealing at 52°C for 30 s, and extension at 72°C for 45 s. Final extension was set at 72°C for 45 s. PCR products were analyzed using 1.8% agarose gel electrophoresis and finally purified using the AxyPrep DNA Gel Extraction Kit (Axygen Biosciences, USA). Quantification of the target fragment was performed using the QuantiFluor ™-ST (Promega, USA) (Zhang H. et al., 2018). The Illumina MiSeq PE300 platform (Illumina, USA) was used to perform paired end sequencing (2 × 300) of qualified libraries according to the instructions of Meiji Biomedical Company (Shanghai, China).
Statistical and bioinformatics analysis
The software Trimmomatic (version 0.33, Golm, Germany) was used to filter the quality of the original sequence file, and then the software cutadapt (version 1.9.1, TU Dortmund, Germany) was used to identify and delete primer sequences with high quality readings without primer sequences (Bolger et al., 2014). Through FLASH (version 1.2.7, Baltimore, MD, USA) software, the high-quality readings of each sample are spliced by overlapping, and the resulting splicing sequences are clean reads (Magoč and Salzberg, 2011). We use UCHIME (version 4.2) to identify and delete chimera sequences and obtain the final valid data as a valid read. According to the sequence similarity, the effective Operational Taxonomic Units (OTUs) were clustered at 97% similarity level by software USEARCH (version 10.0) (Edgar et al., 2011). The classification of each D1 domain of the LSU (Ribosomal Large Subunit) rRNA sequence was analyzed by the Ribosomal Database Project (RDP) classifier algorithm (version 2.2). The confidence threshold used in the NCBI database (National Center for Biotechnology Information) was 0.7. Next, we plotted sparse curves to observe the community abundance and sequencing data of each sample (Li et al., 2018). Principal Coordinate Analysis (PCoA) was performed based on Bray Curtis at the OTU level, and the ‘vegan’ and ‘ape’ packages in R (version 4.0.2) were used to analyze the similarity or difference of sample community composition (Schloss et al., 2009). Difference tests between groups in PCoA were analyzed using Analysis of Similarities (ANOSIM) using the vegan package in R. Alpha diversity (CHAO1 estimator, ACE index, Shannon diversity index, and Simpson index) and yeast abundance were analyzed using SPSS 26.0 (IBM, NY, USA) (Zhu et al., 2021). All values are expressed as mean ± SE. When p < 0.05, the difference is considered significant, and when p < 0.01, the difference is considered extremely significant.
Results
Analysis of 26S rRNA high-throughput sequencing
Illumina HiSeq technology was used to sequence 21 samples from 7 treatments. Following splicing and filtering, a total of 1,296,261 clean tags were generated, with an average of approximately 62,727 clean labels per sample. Through cluster analysis based on 97% sequence similarity, we identified 224 OTUs, including 22 phyla, 47 classes, 92 orders, 130 families, 164 genera, and 191 species. Specifically focusing on yeast, we identified 40,703 total reads and 43 OTUs, spanning 2 phyla, 9 classes, 12 orders, 20 families, 33 genera, and 42 species. The sample Shannon index dilution curve (Figure 1) shows that the sequencing depth is saturated, suggesting that the sequencing amount is sufficient and increasing the sample size will not produce more OTUs.
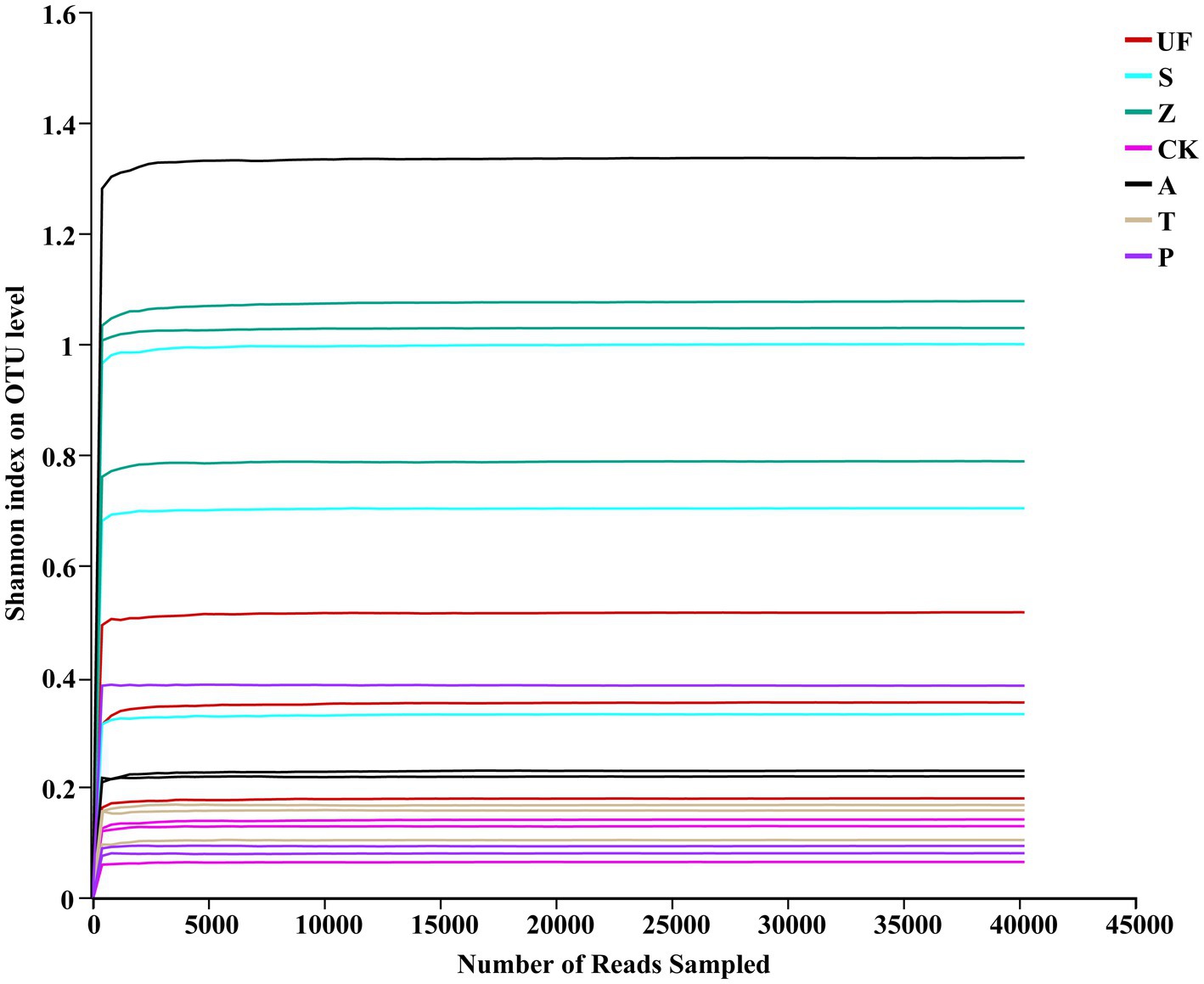
Figure 1. Rarefaction curves of insect gut from different samples. Rarefaction curves of OTUs were clustered for a dissimilarity threshold of 3%. Each sample had three replicates (Replicates are not specifically shown in the legend, but have been involved in the analysis). Samples abbreviations are as in Table 1.
Comparison of intestinal gut yeast groups of Helicoverpa armigera under different hosts
The α diversity index (Table 2) showed that there were differences in the community structure of intestinal yeasts in H. armigera fed on different diets. The abundance of yeasts in the intestinal tract of H. armigera fed on wild plants (UF, S, Z) was notably higher than that of the laboratory artificial fruit feeding group (A, T, P). Particularly, the abundance of yeasts in the intestinal tract of wild H. armigera fed on Zinnia elegans (Z) was significantly higher to the laboratory artificial feeding group (CK, A, T, P), with a Chao 1 index of 17.17 ± 4.37. The richness of UF, S, and Z groups was similar, with no significant difference between CK, A, T, and P groups. According to the difference analysis between groups, the Shannon index of the S group was the highest, indicating significantly higher yeast diversity compared to the A and P groups. However, the diversity of the gut yeast population did not change significantly when the cotton bollworm was transferred from artificial feed to apple, tomato, and pear.
After quality filtering, the representative sequences of OTUs were compared with the NCBI reference database to obtain species classification information corresponding to each OTU. Subsequently, the composition of each sample was quantified at both the genus and species levels. At the genus level (Figure 2A), Hanseniaspora (36.27%), Moesziomyces (21.47%), Trichosporon (16.20%), Wickerhamomyces (12.96%) and Pichia (6.38%) exhibited total relative abundance greater than 5%.
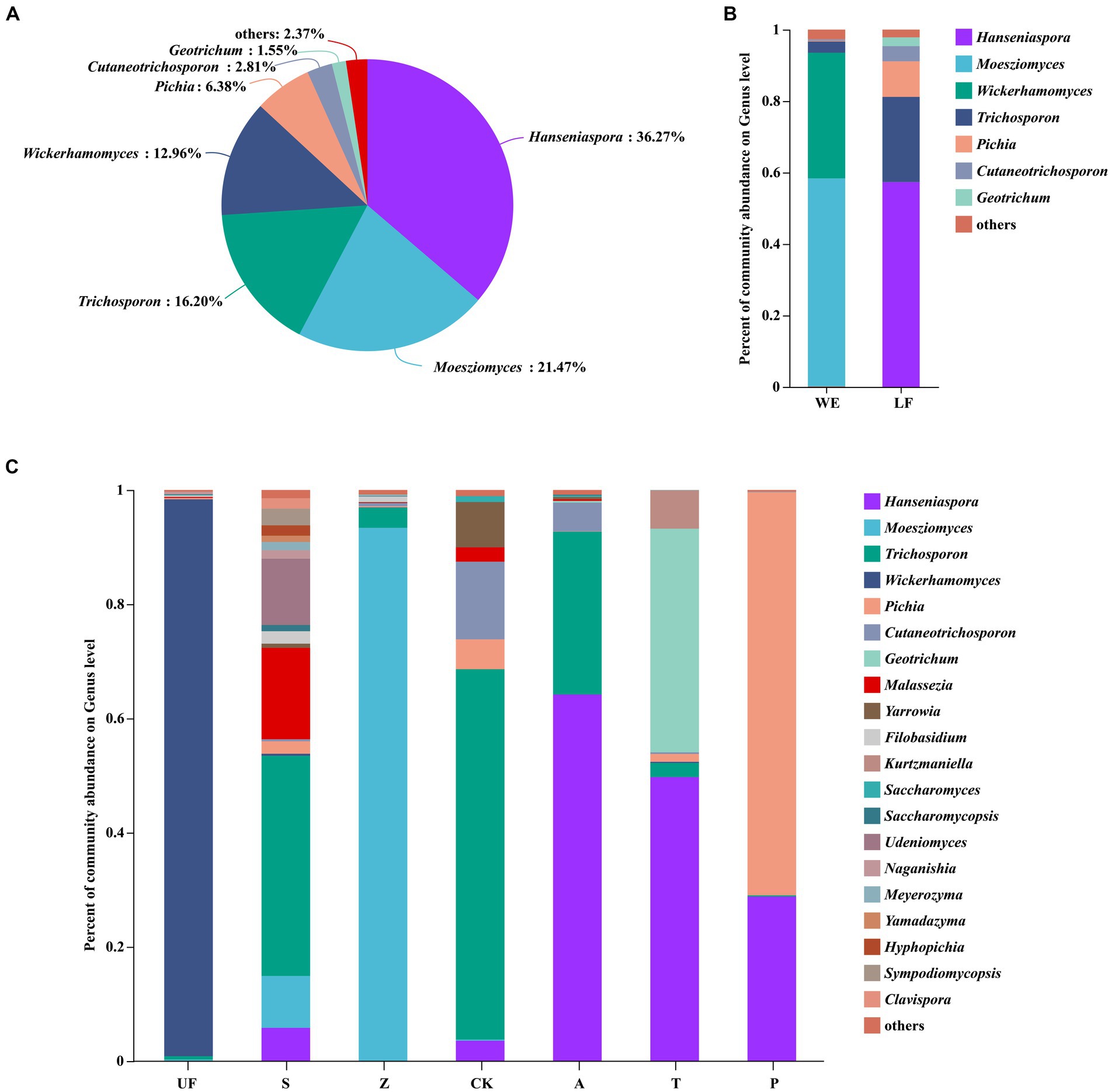
Figure 2. Each sample had three replicates. (A) Yeast community analysis diagram of all samples at the genus level. (B) Relative abundance of yeast in laboratory feeding samples (LF includes A, T, and P samples) and wild environmental samples (WE includes UF, S, Z samples) at the genus level. (C) Relative abundance of yeast at the genus level for each sample.
Hanseniaspora is an absolutely dominant genus, and its relative abundance in artificial feeding samples is generally higher than that in wild sampling groups. The highest relative abundance was observed in the apple feeding group (A) with a value of 77.05 ± 14.18%. The other three groups are: artificial feed feeding group (CK), with a relative abundance of 3.97 ± 6.34%; tomato feeding group (T) at 44.54 ± 11.64%; and pear feeding group (P) at 68.78 ± 41.66%. However, this genus was not detected in the UF group. Moesziomyces, on the other hand, shows lower relative abundance in the artificial feeding samples compared to wild sampling groups. Its highest relative abundance in the Z groups was 90.45 ± 10.94%, whereas it was not detected in the artificial feeding groups (A, T, P). The relative abundance of Trichosporon was higher in the apple feeding group (A) and the feed feeding group (CK). Wickerhamomyces exhibits a relative abundance of more than 5% in the UF group, at 40.04 ± 51.68%, and the relative abundance of Pichia does not show significant differences among the seven groups. Notably, the relative abundance of Hanseniaspora significantly increases in other groups, while the relative abundance of Trichosporon decreases after feeding H. armigera from feed to different fruits (Figures 2B,C; Table 3).
In order to count the number of species in multiple samples and the information of common and unique species, we drew a Venn diagram at the species level (Figure 3). A total of 52 species were detected in all intestinal samples. Further analysis revealed that there were 12 yeast species in wild and laboratory artificial samples (Figure 3A). We also found that each sample had its own unique yeast species. At the species level, group Z had the highest number of detected species, with 22 species, while group T had the lowest, with 10 species (Figure 3B). In all samples, Trichosporon sp. and Cutaneotrichosporon curvatum were common species, and three unique species-Sympodiomycopsis sp., Pichia occidentalis, Clavispora lusitaniae, and Pichia terricola appeared in sample S (Table 4).
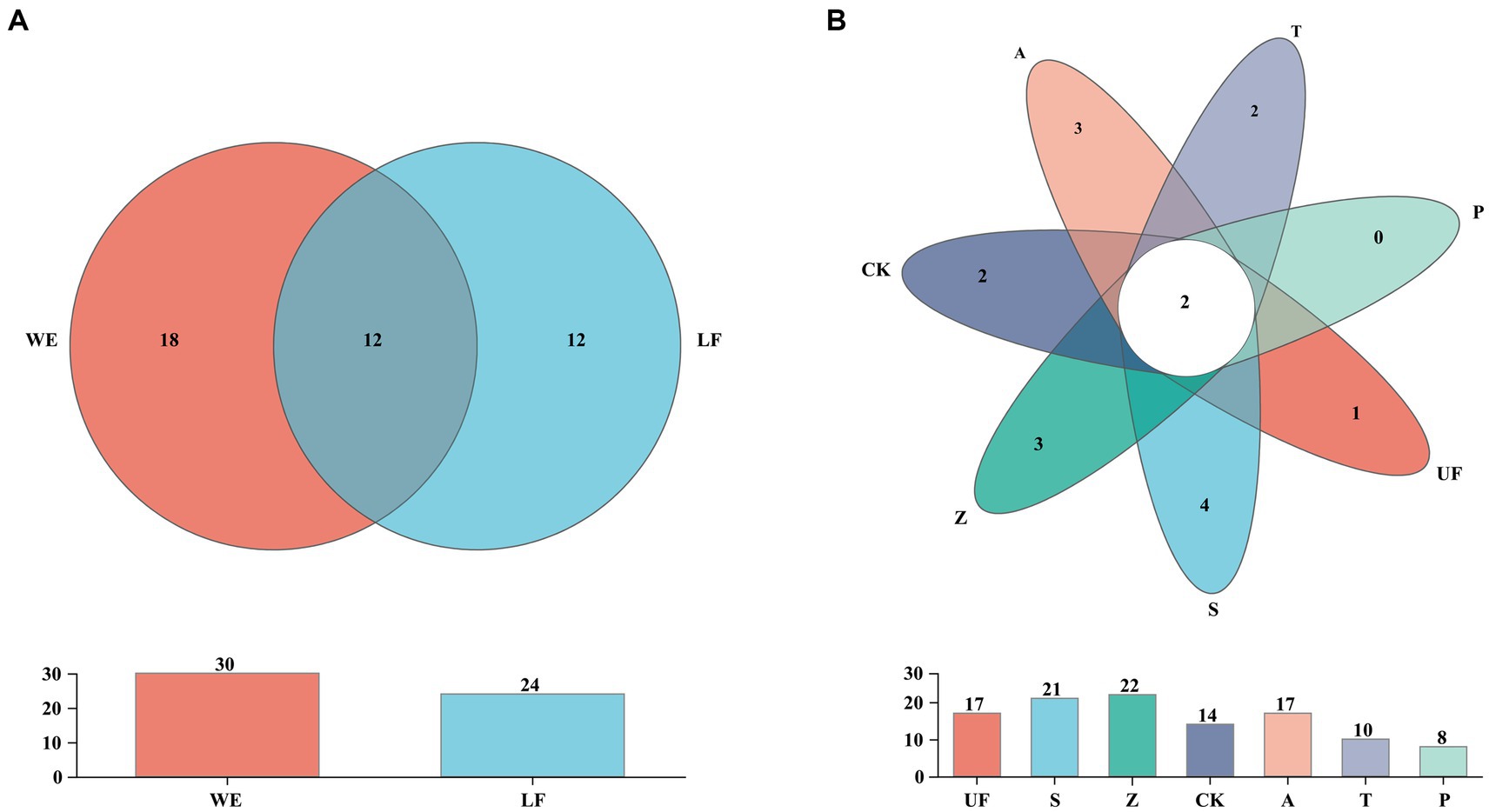
Figure 3. Venn diagram at the species level of samples. (A) Represents laboratory feeding sample (LF includes CK, A, T, and P samples) and wild environmental samples (WE includes UF, S, Z samples). (B) Represents each sample. Each circle with different colors in the diagram represents a group; middle core numbers represent the number of species common to all groups.
The results of species difference analysis, based on the phylum level, showed that the yeast species in the intestinal samples of wild H. armigera were mainly concentrated in Basidiomycetes. In contrast, the laboratory feeding samples were predominantly composed of Ascomycota (Figure 4A). At the genus level (Figure 4B), there were significant differences in 6 genera (p < 0.05) and extremely significant differences in 5 genera (p < 0.01) among the 7 groups. Moesziomyces and Trichosporon mainly found in UF, S, and Z groups, while Hanseniaspora and Trichosporon were primarily detected in CK, A, T, and P.
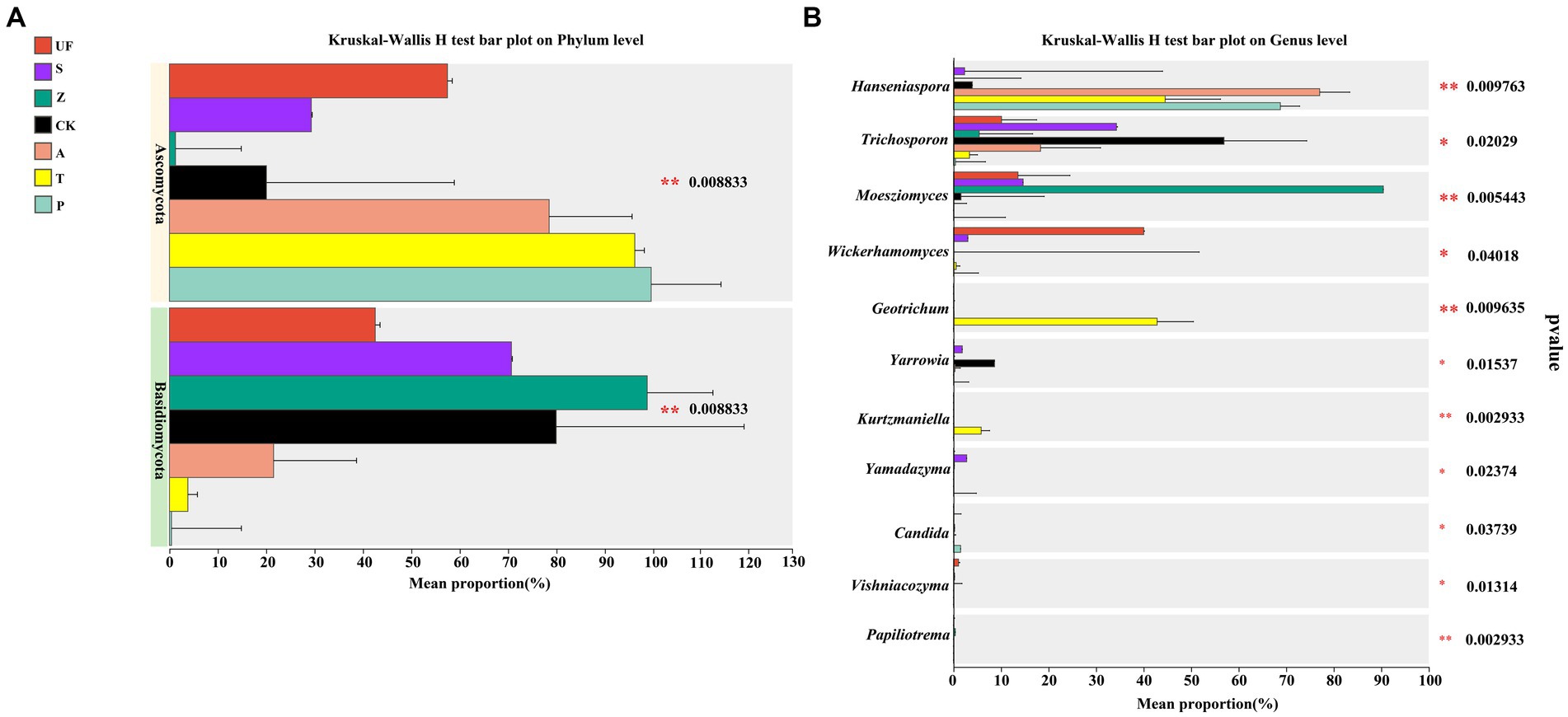
Figure 4. Species difference analysis of seven samples: (A) Phylum level; (B) Genus level. The y-axis represents the classification levels of species, and the x-axis represents the percentage of species average relative abundance in each sample group. Different colors represent different samples. The Kruskal-Wallis rank-sum test was used to show significant differences (*0.01 < p < = 0.05, **0.001 < p < = 0.01, ***p < = 0.001).
To elucidate the dynamic shifts in the intestinal microflora of H. armigera across various hosts, we selected 10 genera with the highest relative abundance for constructing a relative abundance cluster heat map. The clustering is based on the similarity in species abundance, with horizontal clustering representing sample information and vertical clustering indicating species information. Wild plants, including Urtica fissa (UF), Zinnia (Z), and sunflower (S), exhibit distinct clustering on separate branches. Notably, the gut yeast community compositions of H. armigera feeding on pear (P) and tomato (T) are highly similar. Moesziomyces emerges as a major component in the gut microbiome of H. armigera feeding on Zinnia elegans, while the relative abundance of Wickerhamomyces is notably higher in the gut of H. armigera feeding on Urtica than in other groups. Similarly, Hanseniaspora and Trichosporon exhibit elevated relative abundances in the A group (Figure 5).
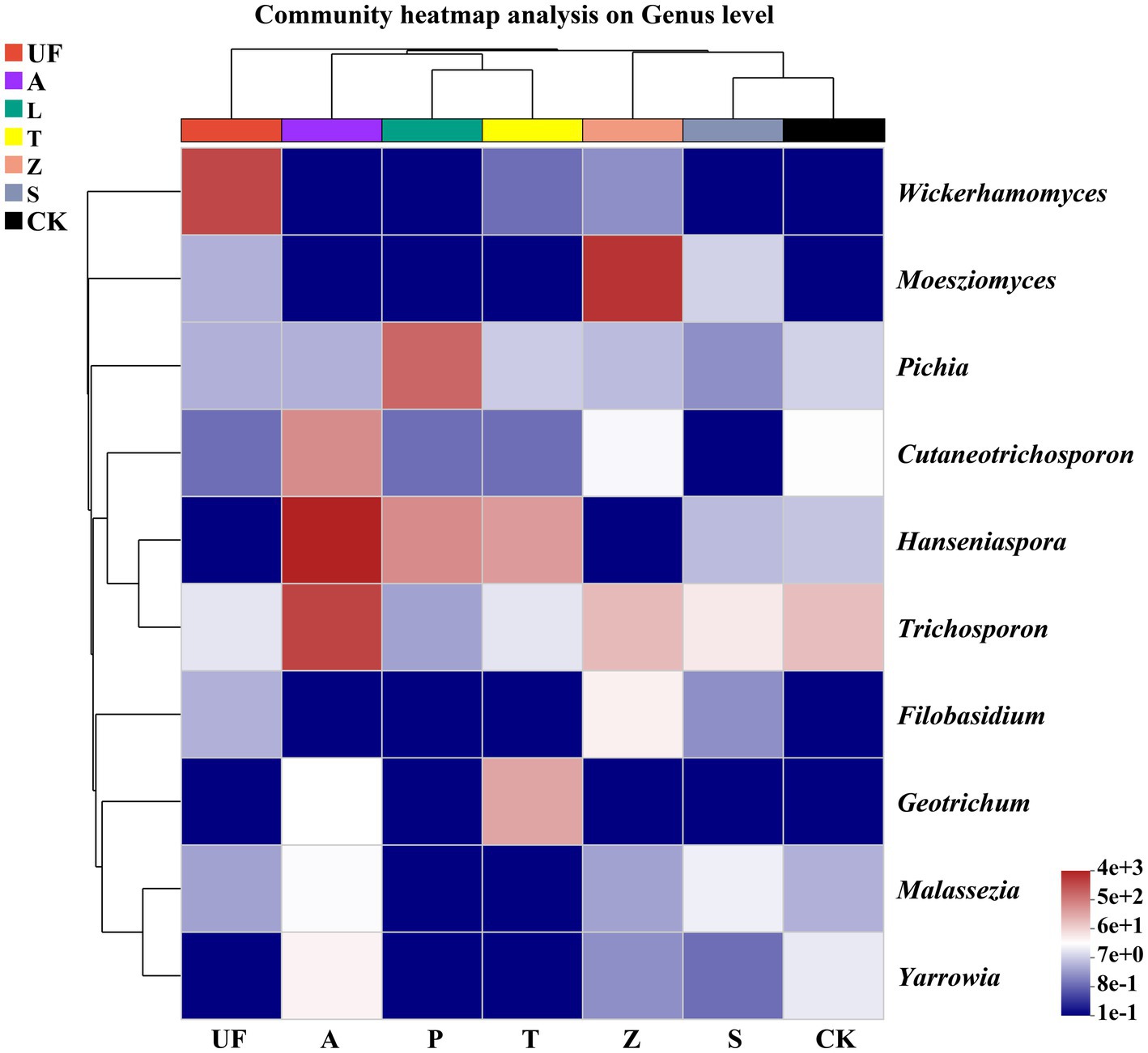
Figure 5. Cluster heat map of the 10 most abundant genera in the yeasts community. The columns represent the samples and the rows represent the yeasts OTUs assigned to the genus level. Dendrograms of hierarchical cluster analysis grouping genera and samples are shown on the left and at the top, respectively. Samples abbreviations are as in Table 1.
Sorted at the OTU level by PCoA to compare community composition similarities between samples. The abscissa and ordinate, respectively, explain the two eigenvalues that contribute the most to the difference between the samples, and the influence degree is 20.89 and 15.49%, respectively, (Figure 6). PCoA1 has relatively small eigenvalues and captures less than 50% of the changes in the input data, so it is not considered to be a very successful PCoA. However, the results of Adonis analysis (Figure 7) showed that the R2 value (R2 = 0.6281, p = 0.001) was greater than 0 and tended to 1, indicating that the difference between the sample groups was greater than that within the sample group. Among the seven insect gut samples, most of the samples in each group clustered together, the three replicates of each sample had good reproducibility, and the degree of separation between each sample group was better, with an AP-value <0.05 (p = 0.001), indicating that there were significant differences in community composition between groups.
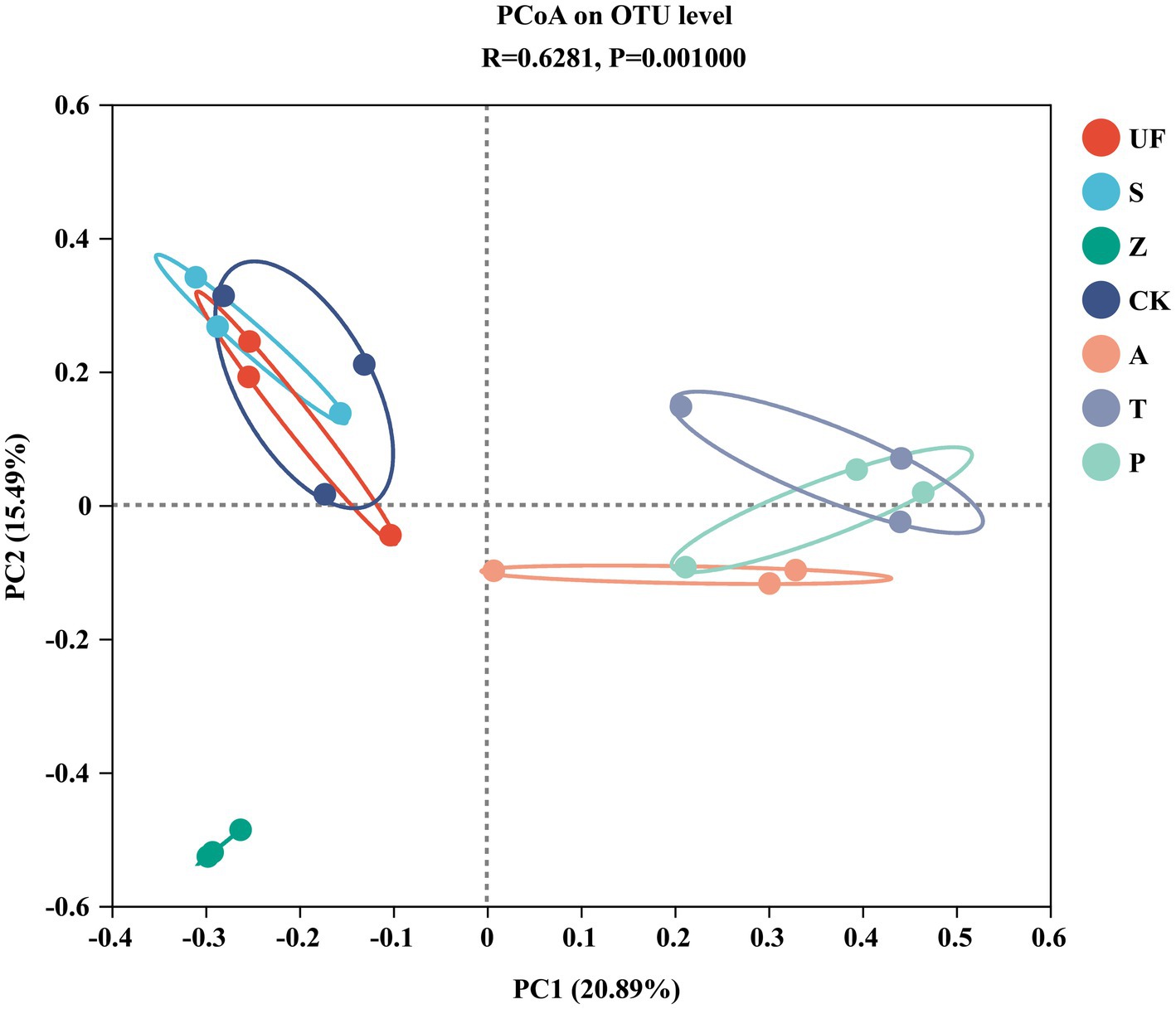
Figure 6. Principal Coordinates analysis (PCoA) based on Bray-Curtis distance method at the OTU level. Samples abbreviations are as in Table 1.
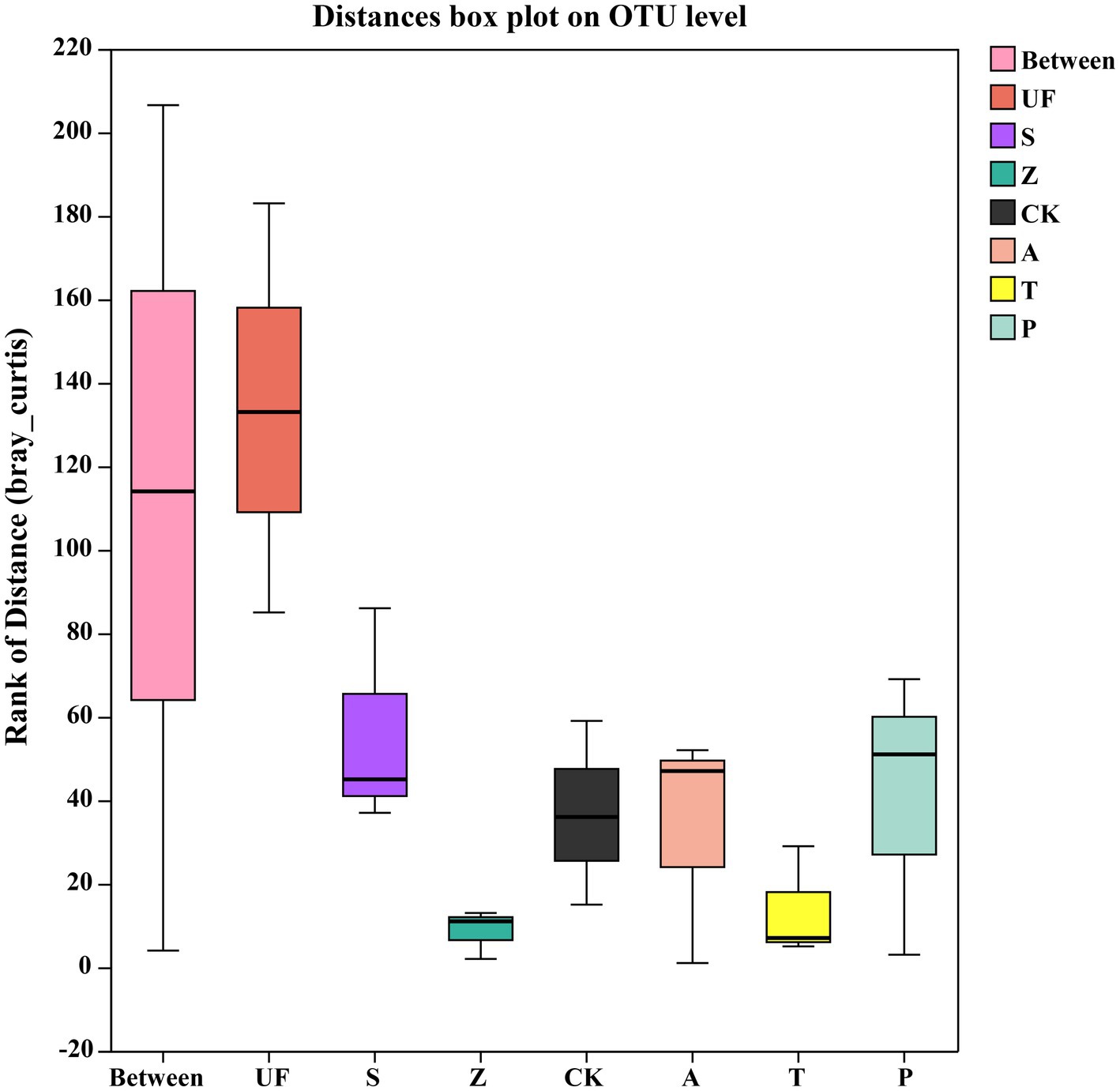
Figure 7. Adonis analysis account for the sample differences by different grouping factors. The ‘Between’ boxes refer to differences between groups, while the others represent differences within their respective groups.
Discussion
Helicoverpa armigera (Lepidoptera: Noctuidae) is an omnivorous and highly migratory agricultural pest. The hatched larvae feed on the tender leaves, flowers, or fruits of plants (Luo et al., 1999). The growth, development, and survival rate of the cotton bollworm are influenced by different host plants (Kim and Lee, 2002). In this research, we have studied the gut yeast diversity and community composition of H. armigera larvae feeding on different wild plants and artificial laboratory fruits by high-throughput sequencing. The experimental results provided a comprehensive understanding of the relationship between H. armigera and its symbiotic yeast. According to our experimental data, we can preliminarily conclude that the diversity and relative abundance of the gut yeast community of H. armigera are affected by the host diet. There is a complex yeast symbiotic community in the gut of H. armigera larvae, which provides a theoretical basis for comprehending the adaptive mechanism of H. armigera and its host plants.
Given the potentially crucial roles of microbial communities in insect physiology and development, extensive research has unveiled the diversity of microbial communities in insects (Douglas, 2007). Some studies indicates that the diversity of fungal communities in the insect gut is influenced by a range of complex factors such as the insect’s habitat environment, diet, developmental stage, and phylogeny. Unlike bacteria, fungi are more strongly influenced by environmental factors, such as different diets and geographical locations, than physiological constraints (Zhang Z. et al., 2018; Park et al., 2019; Ludvigsen et al., 2020). A survey of the gut fungal community of Bactrocera tryoni (Froggatt), Queensland fruit fly larvae feeding on different host fruits revealed that the yeast Pichia had a relative abundance of 90% in the larval gut when feeding on loquats and less than 1% relative abundance in the gut when feeding on pomegranates (Majumder et al., 2020). Insects from different geographical populations typically have distinct gut microbial communities. The gut fungal community of beetles (Coleoptera) is also influenced by their habitat. There are significant differences in the yeast community structure in the guts of beetles between forests and grasslands (Kudo et al., 2019). Reports on the gut microbiota of H. armigera have primarily focused on bacteria. The dominant bacterial phylum in the gut of H. armigera is Actinobacteria (Ranjith et al., 2016). The diversity of symbiotic bacteria in the gut of cotton bollworm larvae feeding on cotton is greater compared to those feeding on artificial feed (Zhao, 2021). In our study, variations in the yeast population composition were observed when cotton bollworm larvae were transitioned from artificial feed to pear, apple, and tomato diets. The yeast diversity in the gut of cotton bollworm larvae feeding on wild plants (UF, S, Z) surpassed that of the laboratory artificial fruit feeding group (CK, A, T, P). Particularly, the yeast diversity in the gut of cotton bollworm larvae feeding on Zinnia elegans was notably higher than that of the laboratory feeding group.
At the phylum level, the gut yeast population of cotton bollworm larvae consuming plants was predominantly composed of Basidiomycetes. In contrast, the gut yeast population of laboratory-fed cotton bollworm larvae consuming fruits was dominated by Ascomycota. At the genus level, Hanseniaspora was the predominant genus detected in H. armigera fed on fruits. This observation may be attributed to the fact that Hanseniaspora is the prevalent yeast genus in the early stages of natural fermentation, commonly found on the epidermis of mature fruits, and capable of secreting various hydrolases. Certain strains of this genus exhibit low ethanol production, facilitating insect digestion of the pulp without causing harm to the host insects (Capece et al., 2005; Viana et al., 2009; Hong and Park, 2013). Moesziomyces, belongs to the phylum Basidiomycota, is primarily distributed in wild larval samples (UF, Z, S), and is rarely detected in laboratory samples. This yeast genus was initially isolated from sediment in Lake Vanda, Antarctica (Li et al., 2019) and later frequently isolated from the surface of plant leaves, serving as a natural source for plant resistance against powdery mildew (Liu et al., 2019). Trichosporon exhibit relatively high abundance in artificial feed (CK), with a relative abundance similarly to Wickerhamomyces in the wild Urticafissa (UF) group. These results strongly suggest a certain correlation between the yeast populations in the cotton bollworm gut and those on the host, indicating potential similarities. The microbial community in the insect gut is influenced by the host’s feeding habits, leading to different dominant microbial lineages (Ayayee et al., 2016; Vilanova et al., 2016; Bing et al., 2018; Liu et al., 2020).
Trichosporon spp. and Cutaneotrichosporon curvatum were found in all seven treatment samples. Trichosporon spp. are versatile organisms that can thrive in diverse environments, including soil, wastewater, sediment, wood pulp, and sludge (Mehta et al., 2021). They have the capability to utilize their cells or enzyme systems for bioremediation, breaking down pollutants and external substances. This occurs particularly when using uric acid, ethylamine, aniline, and aromatic compounds as the exclusive energy source (Godjevargova and Gabrovska, 2003; Bergauer et al., 2005). Another species, Cutaneotrichosporon curvatum, exhibits the capacity to utilize unconventional carbon sources for oil production and is proficient in lipid accumulation (Péter et al., 2019). Several yeast species found in the wild samples display special function. Metschnikowia pulcherrima demonstrates the ability to produce various acidic proteases and microbial lipids (Li et al., 2021). Additionally, Papiliotrema laurentii in group Z stands out as an oleaginous yeast with a diverse range of metabolic carbon sources, enabling the production of enzymes and high lipid concentrations (de Almeida et al., 2022). Similarly, Sporobolomyces roseus can produce zeatin (Streletskii et al., 2019) and degrade amino acids (Moore et al., 1968). Papiliotrema has been reported to be resistant to heavy metals (Nguyen et al., 2020). These biological functions possibly supply essential nutrients for the growth of cotton boll larvae or aid the host in detoxification processes. Different yeast species appeared in the gut of cotton boll larvae under different feeding conditions. This difference could be a result of wild larvae adapting to the constantly changing natural environment, including factors such as sunlight, temperature, moisture, and food availability. The larvae may require a greater presence of symbiotic yeasts with specific functions to help them withstand external adversities.
PCoA analysis revealed variations in the composition of gut yeast populations. Interestingly, we observed no significant difference in the structure of intestinal yeast microflora between the groups fed tomatoes (T) and pears (P) (Permonova: R2 = 0.275, p = 0.3). A daring hypothesis to elucidate this observation is that tomatoes and pears share certain similar nutrients, potentially amino acids or acids, fulfilling the growth requirements of cotton bollworm larvae (Shao et al., 2011). It is well-established that the composition of gut microbiota is influenced by both plant secondary metabolites and the nutritional needs of the host (Myers et al., 2006; Blanco et al., 2007; Muhammad et al., 2017). Insects’ host are influenced by many factors, including environmental conditions, dietary maturity, host volatiles, and so on, all of which indirectly affect the composition of the insect gut symbiotic microbial community. There is a close relationship between the three (insect, insects host and the insect gut symbiotic microbial community).
Controlling H. armigera in China poses significant challenges, with current methods relying on chemical pesticides and the cultivation of Genetically Modified (GM) crops. However, people are gradually realizing that these methods confer stronger resistance to insects. Transgenic pest control strategies involving the modification of microorganisms (Paratransgenesis) have been proposed. In this strategy, genetically engineered microorganisms colonize the insect gut and produce effector molecules that target and kill pests. For instance, research has integrated genes expressing lytic peptides into yeast, creating an eco-friendly bait system targeting termites. The engineered yeast spreads widely in the social insect population, and the expressed lytic peptides kill protozoa in the gut within 4 weeks, followed by termite mortality within 6 weeks (Sethi et al., 2014).
Another innovative strategy is RNA interference (RNAi), a reverse genetic approach with potential applications in pest control. Yeast, commonly found on the surface of many fruits and constituting a major part of the microbial communities in various insect species, makes it highly attractive to insects. Murphy et al. (2016) utilized genetically modified baker’s yeast to feed Drosophila suzukii, delivering species-specific dsRNA. After feeding on this “yeast biopesticide,” the survival rate of Drosophila larvae, as well as the mobility and reproductive capacity of the adults, was reduced (Murphy et al., 2016). There are also relevant experiments using fungi as feeding stimulants to increase insecticide ingestion. For example, the combination of sucrose with Saccharomyces cerevisiae or Aureobasidium pullulans can serve as ingestion stimulants, enhancing the efficacy of Cyantraniliprole and Spinosad (Knight et al., 2015). Yeast can also serve as an adjuvant for biopesticides. Yeast isolated from the gut of Cydia pomonella larvae can enhance the activity of Cydia pomonella granulovirus (CpGV), significantly increasing the mortality rate of newly hatched larvae (Knight and Witzgall, 2013). Fungi that are attractive to insects can be directly used for pest monitoring, forecasting, and lure-and-kill strategies. For instance, the yeast Candida utilis has been used as bait to trap adult vinegar flies (Daane and Johnson, 2010).
In conclusion, our research findings confirm that the gut yeast community of cotton bollworm larvae is influenced by the host’s diet. Variations in dominant yeast species occur with different host diets, and transitioning cotton bollworm larvae from artificial feed to apple, pear, and tomato diets induces changes in yeast population abundance. These alterations likely contribute to mutual adaptation with the host. Understanding the composition of the gut yeast population in cotton bollworms is crucial due to the physiological and ecological functions of symbiotic yeast. This knowledge lays the foundation for developing targeted pest control technologies based on symbiotic yeast (Berasategui et al., 2016).
Data availability statement
The datasets presented in this study can be found in NCBI SRA repository, accession number PRJNA946556 (https://www.ncbi.nlm.nih.gov/sra/PRJNA946556).
Author contributions
MY: Data curation, Methodology, Writing – original draft. YaL: Formal analysis, Writing – review & editing. JJ: Software, Writing – review & editing. YoL: Resources, Writing – review & editing. YS: Funding acquisition, Methodology, Resources, Writing – review & editing.
Funding
The author(s) declare financial support was received for the research, authorship, and/or publication of this article. The work was funded by the National Natural Science Foundation of China (project number: 31860003).
Acknowledgments
We would like to thank all the reviewers who participated in the review, as well as Frontiers in Microbiology editor for providing English editing services during the preparation of this manuscript.
Conflict of interest
The authors declare that the research was conducted in the absence of any commercial or financial relationships that could be construed as a potential conflict of interest.
Publisher’s note
All claims expressed in this article are solely those of the authors and do not necessarily represent those of their affiliated organizations, or those of the publisher, the editors and the reviewers. Any product that may be evaluated in this article, or claim that may be made by its manufacturer, is not guaranteed or endorsed by the publisher.
Supplementary material
The Supplementary material for this article can be found online at: https://www.frontiersin.org/articles/10.3389/fmicb.2024.1287083/full#supplementary-material
References
Abdelgaffar, H., Tague, E. D., Castro Gonzalez, H. F., Campagna, S. R., and Jurat-Fuentes, J. L. (2019). Midgut metabolomic profiling of fall armyworm (Spodoptera frugiperda) with field-evolved resistance to cry 1F corn. Insect Biochem. Mol. Biol. 106, 1–9. doi: 10.1016/j.ibmb.2019.01.002
Ahmad, S., Cheema, H. M. N., Khan, A. A., Khan, R. S. A., and Ahmad, J. N. (2019). Resistance status of Helicoverpa armigera against Bt cotton in Pakistan. Transgenic Res. 28, 199–212. doi: 10.1007/s11248-019-00114-9
Anand, A. A., Vennison, S. J., Sankar, S. G., Prabhu, D. I., Vasan, P. T., Raghuraman, T., et al. (2010). Isolation and characterization of bacteria from the gut of Bombyx mori that degrade cellulose, xylan, pectin and starch and their impact on digestion. J. Insect Sci. 10:107. doi: 10.1673/031.010.10701
Ayayee, P. A., Larsen, T., and Sabree, Z. (2016). Symbiotic essential amino acids provisioning in the American cockroach, Periplaneta americana (Linnaeus) under various dietary conditions. PeerJ 4:e2046. doi: 10.7717/peerj.2046
Berasategui, A., Shukla, S., Salem, H., and Kaltenpoth, M. (2016). Potential applications of insect symbionts in biotechnology. Appl. Microbiol. Biotechnol. 100, 1567–1577. doi: 10.1007/s00253-015-7186-9
Bergauer, P., Fonteyne, P. A., Nolard, N., Schinner, F., and Margesin, R. (2005). Biodegradation of phenol and phenol-related compounds by psychrophilic and cold-tolerant alpine yeasts. Chemosphere 59, 909–918. doi: 10.1016/j.chemosphere.2004.11.011
Bing, X., Gerlach, J., Loeb, G., and Buchon, N. (2018). Nutrient-dependent impact of microbes on Drosophila suzukii development. MBio 9:2199. doi: 10.1128/mBio.02199-17
Blanco, C. A., Terán-Vargas, A. P. Jr., López, J. D. Jr., Kauffman, J. V., and Wei, X. (2007). Densities of Heliothis virescens and Helicoverpa zea (Lepidoptera: Noctuidae) in three plant hosts. Florida Entomologist 90, 742–750. doi: 10.1653/0015-4040(2007)90[742:DOHVAH]2.0.CO;2
Bolger, A. M., Lohse, M., and Usadel, B. (2014). Trimmomatic: a flexible trimmer for Illumina sequence data. Bioinformatics 30, 2114–2120. doi: 10.1093/bioinformatics/btu170
Capece, A., Fiore, C., Maraz, A., and Romano, P. (2005). Molecular and technological approaches to evaluate strain biodiversity in Hanseniaspora uvarum of wine origin. J. Appl. Microbiol. 98, 136–144. doi: 10.1111/j.1365-2672.2004.02434.x
Carvalho, M., Schwudke, D., Sampaio, J. L., Palm, W., Riezman, I., Dey, G., et al. (2010). Survival strategies of a sterol auxotroph. Development 137, 3675–3685. doi: 10.1242/dev.044560
Daane, K. M., and Johnson, M. W. (2010). Olive fruit fly: managing an ancient pest in modern times. Annu. Rev. Entomol. 55, 151–169. doi: 10.1146/annurev.ento.54.110807.090553
de Almeida, E. L. M., Ventorim, R. Z., de Moura Ferreira, M. A., and da Silveira, W. B. (2022). Papiliotrema laurentii: general features and biotechnological applications. Appl. Microbiol. Biotechnol. 106, 6963–6976. doi: 10.1007/s00253-022-12208-2
Dillon, R. J., and Dillon, V. M. (2004). The gut bacteria of insects: nonpathogenic interactions. Annu. Rev. Entomol. 49, 71–92. doi: 10.1146/annurev.ento.49.061802.123416
Douglas, A. E. (2007). Symbiotic microorganisms: untapped resources for insect pest control. Trends Biotechnol. 25, 338–342. doi: 10.1016/j.tibtech.2007.06.003
Douglas, A. E. (2018). The Drosophila model for microbiome research. Lab Anim. 47, 157–164. doi: 10.1038/s41684-018-0065-0
Dubovskiy, I. M., Grizanova, E. V., Whitten, M. M., Mukherjee, K., Greig, C., Alikina, T., et al. (2016). Immuno-physiological adaptations confer wax moth galleria mellonella resistance to Bacillus thuringiensis. Virulence 7, 860–870. doi: 10.1080/21505594.2016.1164367
Edgar, R. C., Haas, B. J., Clemente, J. C., Quince, C., and Knight, R. (2011). UCHIME improves sensitivity and speed of chimera detection. Bioinformatics 27, 2194–2200. doi: 10.1093/bioinformatics/btr381
Fitt, G. P. (1989). The ecology of Heliothis species in relation to agroecosystems. Annu. Rev. Entomol. 34, 17–53. doi: 10.1146/annurev.en.34.010189.000313
Fukatsu, T., and Hosokawa, T. (2002). Capsule-transmitted gut symbiotic bacterium of the Japanese common plataspid stinkbug, Megacopta punctatissima. Appl. Environ. Microbiol. 68, 389–396. doi: 10.1128/aem.68.1.389-396.2002
Godjevargova, T., and Gabrovska, K. (2003). Immobilization of urease onto chemically modified acrylonitrile copolymer membranes. J. Biotechnol. 103, 107–111. doi: 10.1016/s0168-1656(03)00107-x
Gong, Q., Cao, L. J., Sun, L. N., Chen, J. C., Gong, Y. J., Pu, D. Q., et al. (2020). Similar gut bacterial microbiota in two fruit-feeding moth pests collected from different host species and locations. Insects 11:840. doi: 10.3390/insects11120840
Gu, M., Xue, Z., Lv, S., Cai, Y., Zhang, L., and Gao, X. (2022). Corynebacterium sp. 2-TD mediated toxicity of 2-Tridecanone to Helicoverpa armigera. Toxins (Basel) 14:698. doi: 10.3390/toxins14100698
Gujar, N., Nikte, S. V., Joshi, R. S., and Joshi, M. (2021). Molecular characterization of the β2-like Octopamine receptor of Helicoverpa armigera. J. Membr. Biol. 254, 311–319. doi: 10.1007/s00232-021-00172-3
Gurung, K., Wertheim, B., and Falcao Salles, J. (2019). The microbiome of pest insects: it is not just bacteria. Entomol. Exp. Appl. 167, 156–170. doi: 10.1111/eea.12768
Harris, E. V., de Roode, J. C., and Gerardo, N. M. (2019). Diet-microbiome-disease: investigating diet's influence on infectious disease resistance through alteration of the gut microbiome. PLoS Pathog. 15:e1007891. doi: 10.1371/journal.ppat.1007891
Hong, Y. A., and Park, H. D. (2013). Role of non-Saccharomyces yeasts in Korean wines produced from Campbell early grapes: potential use of Hanseniaspora uvarum as a starter culture. Food Microbiol. 34, 207–214. doi: 10.1016/j.fm.2012.12.011
Horgan, F. G., Peñalver Cruz, A., Arida, A., Ferrater, J. B., and Bernal, C. C. (2021). Adaptation by the Brown Planthopper to resistant Rice: A test of female-derived virulence and the role of yeast-like symbionts. Insects 12:908. doi: 10.3390/insects12100908
James, C. (2013). Global status of commercialized biotech/GM crops: 2012. China Biotechnology 33, 1–8.
Jin, L., Zhang, H., Lu, Y., Yang, Y., Wu, K., Tabashnik, B. E., et al. (2015). Large-scale test of the natural refuge strategy for delaying insect resistance to transgenic Bt crops. Nat. Biotechnol. 33, 169–174. doi: 10.1038/nbt.3100
Johnston, P. R., and Rolff, J. (2015). Host and symbiont jointly control gut microbiota during complete metamorphosis. PLoS Pathog. 11:e1005246. doi: 10.1371/journal.ppat.1005246
Kaltenpoth, M., and Steiger, S. (2014). Unearthing carrion beetles' microbiome: characterization of bacterial and fungal hindgut communities across the Silphidae. Mol. Ecol. 23, 1251–1267. doi: 10.1111/mec.12469
Keidser, G., Dillon, H., Carter, L., and O'Brien, A. (2012). NAL-NL2 empirical adjustments. Trends Amplif. 16, 211–223. doi: 10.1177/1084713812468511
Kim, D. S., and Lee, J. H. (2002). Egg and larval survivorship of Carposina sasakii (Lepidoptera: Carposinidae) in apple and peach and their effects on adult population dynamics in orchards. Environ. Entomol. 31, 686–692. doi: 10.1603/0046-225X-31.4.686
Knight, A. L., Basoalto, E., and Witzgall, P. (2015). Improving the performance of the Granulosis virus of codling moth (Lepidoptera: Tortricidae) by adding the yeast Saccharomyces cerevisiae with sugar. Environ. Entomol. 44, 252–259. doi: 10.1093/ee/nvv008
Knight, A. L., and Witzgall, P. (2013). Combining mutualistic yeast and pathogenic virus--a novel method for codling moth control. J. Chem. Ecol. 39, 1019–1026. doi: 10.1007/s10886-013-0322-z
Kudo, R., Masuya, H., Endoh, R., Kikuchi, T., and Ikeda, H. (2019). Gut bacterial and fungal communities in ground-dwelling beetles are associated with host food habit and habitat. ISME J. 13, 676–685. doi: 10.1038/s41396-018-0298-3
Lauzon, C. R., Sjogren, R. E., and Prokopy, R. J. (2000). Enzymatic capabilities of Bacteria associated with apple maggot flies: A postulated role in attraction. J. Chem. Ecol. 26, 953–967. doi: 10.1023/A:1005460225664
Li, J., Hu, W., Huang, X., and Xu, Y. (2018). Investigation of yeast population diversity and dynamics in spontaneous fermentation of Vidal blanc icewine by traditional culture-dependent and high-throughput sequencing methods. Food Res. Int. 112, 66–77. doi: 10.1016/j.foodres.2018.06.011
Li, Y. M., Shivas, R. G., Li, B. J., and Cai, L. (2019). Diversity of Moesziomyces (Ustilaginales, Ustilaginomycotina) on Echinochloa and Leersia (Poaceae). Myco Keys 52, 1–16. doi: 10.3897/mycokeys.52.30461
Li, Q., Wang, D., Li, A., and Gu, J. (2021). Microbial lipids production from wastes by Metschnikowia pulcherrima: a review. Sheng Wu Gong Cheng Xue Bao 37, 2753–2764. doi: 10.13345/j.cjb.200599
Liu, Y. H., Shah, M. M. R., Song, Y., and Liu, T. X. (2020). Host plant affects symbiont abundance in Bemisia tabaci (Hemiptera: Aleyrodidae). Insects 11:501. doi: 10.3390/insects11080501
Liu, Y., Zou, Z., Hu, Z., Wang, W., and Xiong, J. (2019). Morphology and molecular analysis of Moesziomyces antarcticus isolated from the blood samples of a Chinese patient. Front. Microbiol. 10:254. doi: 10.3389/fmicb.2019.00254
Ludvigsen, J., Andersen, Å., Hjeljord, L., and Rudi, K. (2020). The honeybee gut Mycobiota cluster by season versus the microbiota which cluster by gut segment. Vet Sci 8:4. doi: 10.3390/vetsci8010004
Luo, M. H., Guo, X. R., Zhang, H. L., Wang, X. F., and Xiong, J. W. (1999). Study on variations of body colour and heredity of the oriental tobacco budworm (Heliothis assulta Guenee) and cotton bollworm (H. armigera Hubner). Oxford University Press.
Magoč, T., and Salzberg, S. L. (2011). FLASH: fast length adjustment of short reads to improve genome assemblies. Bioinformatics 27, 2957–2963. doi: 10.1093/bioinformatics/btr507
Majumder, R., Sutcliffe, B., Taylor, P. W., and Chapman, T. A. (2020). Fruit host-dependent fungal communities in the microbiome of wild Queensland fruit fly larvae. Sci. Rep. 10:16550. doi: 10.1038/s41598-020-73649-1
Malassigné, S., Minard, G., Vallon, L., Martin, E., Valiente Moro, C., and Luis, P. (2021). Diversity and functions of yeast communities associated with insects. Microorganisms 9:1552. doi: 10.3390/microorganisms9081552
Martemyanov, V. V., Belousova, I. A., Pavlushin, S. V., Dubovskiy, I. M., Ershov, N. I., Alikina, T. Y., et al. (2016). Phenological asynchrony between host plant and gypsy moth reduces insect gut microbiota and susceptibility to Bacillus thuringiensis. Ecol. Evol. 6, 7298–7310. doi: 10.1002/ece3.2460
Martin, M. M. (1979). Biochemical implications of insect mycophagy. Biol. Rev. 54, 1–21. doi: 10.1111/j.1469-185X.1979.tb00865.x
Mehta, V., Nayyar, C., Gulati, N., Singla, N., and Chandar, J. (2021). A comprehensive review of Trichosporon spp.: an invasive and emerging fungus. Cureus 13:e17345. doi: 10.7759/cureus.17345
Miller, K. K., and Alper, H. S. (2019). Yarrowia lipolytica: more than an oleaginous workhorse. Appl. Microbiol. Biotechnol. 103, 9251–9262. doi: 10.1007/s00253-019-10200-x
Moore, K., Rao, P. V., and Towers, G. H. (1968). Degradation of phenylalanine and tyrosine by Sporobolomyces roseus. Biochem. J. 106, 507–514. doi: 10.1042/bj1060507
Moran, N. A., Ochman, H., and Hammer, T. J. (2019). Evolutionary and ecological consequences of gut microbial communities. Annu. Rev. Ecol. Evol. Syst. 50, 451–475. doi: 10.1146/annurev-ecolsys-110617-062453
Muhammad, A., Fang, Y., Hou, Y., and Shi, Z. (2017). The gut Entomotype of red Palm weevil Rhynchophorus ferrugineus Olivier (Coleoptera: Dryophthoridae) and their effect on host nutrition metabolism. Front. Microbiol. 8:2291. doi: 10.3389/fmicb.2017.02291
Murphy, K. A., Tabuloc, C. A., Cervantes, K. R., and Chiu, J. C. (2016). Ingestion of genetically modified yeast symbiont reduces fitness of an insect pest via RNA interference. Sci. Rep. 6:22587. doi: 10.1038/srep22587
Myers, C. T., Hull, L. A., and Krawczyk, G. (2006). Early-season host plant fruit impacts on reproductive parameters of the oriental fruit moth (Lepidoptera: Tortricidae). J. Entomol. Sci. 41, 65–74. doi: 10.18474/0749-8004-41.1.65
Nguyen, K. C. T., Nguyen, P. V., and Truong, H. T. H. (2020). Heavy metal tolerance of novel Papiliotrema yeast isolated from Vietnamese Mangosteen. Mycobiology 48, 296–303. doi: 10.1080/12298093.2020.1767020
Paramasiva, I., Sharma, H. C., and Krishnayya, P. V. (2014). Antibiotics influence the toxicity of the delta endotoxins of Bacillus thuringiensis towards the cotton bollworm, Helicoverpa armigera. BMC Microbiol 14:200. doi: 10.1186/1471-2180-14-200
Park, R., Dzialo, M. C., Spaepen, S., Nsabimana, D., Gielens, K., Devriese, H., et al. (2019). Microbial communities of the house fly Musca domestica vary with geographical location and habitat. Microbiome 7:147. doi: 10.1186/s40168-019-0748-9
Péter, G., Mounier, J., Garnier, L., Soós, D., and Dlauchy, D. (2019). Cutaneotrichosporon suis sp. nov., a lipolytic yeast species from food and food-related environment. Int. J. Syst. Evol. Microbiol. 69, 2367–2371. doi: 10.1099/ijsem.0.003485
Ranjith, M. T., Chellappan, M., Harish, E. R., Girija, D., and Nazeem, P. A. (2016). Bacterial communities associated with the gut of tomato fruit borer, Helicoverpa armigera (Hübner) (Lepidoptera: Noctuidae) based on Illumina next-generation sequencing. J. Asia Pac. Entomol. 19, 333–340. doi: 10.1016/j.aspen.2016.03.007
Schloss, P. D., Westcott, S. L., Ryabin, T., Hall, J. R., Hartmann, M., Hollister, E. B., et al. (2009). Introducing mothur: open-source, platform-independent, community-supported software for describing and comparing microbial communities. Appl. Environ. Microbiol. 75, 7537–7541. doi: 10.1128/aem.01541-09
Scott, M. P. (1998). The ecology and behavior of burying beetles. Annu. Rev. Entomol. 43, 595–618. doi: 10.1146/annurev.ento.43.1.595
Sethi, A., Delatte, J., Foil, L., and Husseneder, C. (2014). Protozoacidal Trojan-horse: use of a ligand-lytic peptide for selective destruction of symbiotic protozoa within termite guts. PLoS One 9:e106199. doi: 10.1371/journal.pone.0106199
Shao, Y., Spiteller, D., Tang, X., Ping, L., Colesie, C., Münchberg, U., et al. (2011). Crystallization of α- and β-carotene in the foregut of Spodoptera larvae feeding on a toxic food plant. Insect Biochem. Mol. Biol. 41, 273–281. doi: 10.1016/j.ibmb.2011.01.004
Shapira, M. (2016). Gut microbiotas and host evolution: scaling up Symbiosis. Trends Ecol. Evol. 31, 539–549. doi: 10.1016/j.tree.2016.03.006
Shelton, A. M., Zhao, J. Z., and Roush, R. T. (2002). Economic, ecological, food safety, and social consequences of the deployment of bt transgenic plants. Annu. Rev. Entomol. 47, 845–881. doi: 10.1146/annurev.ento.47.091201.145309
Shin, S. C., Kim, S. H., You, H., Kim, B., Kim, A. C., Lee, K. A., et al. (2011). Drosophila microbiome modulates host developmental and metabolic homeostasis via insulin signaling. Science 334, 670–674. doi: 10.1126/science.1212782
Streletskii, R. A., Kachalkin, A. V., Glushakova, A. M., Yurkov, A. M., and Demin, V. V. (2019). Yeasts producing zeatin. PeerJ 7:e6474. doi: 10.7717/peerj.6474
Su, W., Liu, J., Bai, P., Ma, B., and Liu, W. (2019). Pathogenic fungi-induced susceptibility is mitigated by mutual Lactobacillus plantarum in the Drosophila melanogaster model. BMC Microbiol. 19:302. doi: 10.1186/s12866-019-1686-1
Takatsuka, J., and Kunimi, Y. (2000). Intestinal bacteria affect growth of Bacillus thuringiensis in larvae of the oriental tea tortrix, Homona magnanima Diakonoff (Lepidoptera: tortricidae). J. Invertebr. Pathol. 76, 222–226. doi: 10.1006/jipa.2000.4973
Tsafack, N. M., Brevault, P., Soti, T., Deconchat, V., and Ouin, M. (2013). Effects of landscape context and agricultural practices on the abundance of cotton bollworm Helicoverpa armigera in cotton fields: a case study in northern Benin. Int. J. Pest Manag. 59, 294–302. doi: 10.1080/09670874.2013.852270
Vega, F. (2005). “The role of yeasts as insect endosymbionts” in Insect-fungal associations: Ecology and evolution. Oxford University Press. (https://www.sciencedirect.com/science/article/abs/pii/S0168160509003742).
Viana, F., Gil, J. V., Vallés, S., and Manzanares, P. (2009). Increasing the levels of 2-phenylethyl acetate in wine through the use of a mixed culture of Hanseniaspora osmophila and Saccharomyces cerevisiae. Int. J. Food Microbiol. 135, 68–74. doi: 10.1016/j.ijfoodmicro.2009.07.025
Vilanova, C., Baixeras, J., Latorre, A., and Porcar, M. (2016). The generalist inside the specialist: gut bacterial communities of two insect species feeding on toxic plants are dominated by Enterococcus sp. Front. Microbiol. 7:1005. doi: 10.3389/fmicb.2016.01005
Vogel, H., Shukla, S. P., Engl, T., Weiss, B., Fischer, R., Steiger, S., et al. (2017). The digestive and defensive basis of carcass utilization by the burying beetle and its microbiota. Nat. Commun. 8:15186. doi: 10.1038/ncomms15186
Wang, X., Sun, S., Yang, X., Cheng, J., Wei, H., Li, Z., et al. (2020). Variability of gut microbiota across the life cycle of Grapholita molesta (Lepidoptera: Tortricidae). Front. Microbiol. 11:1366. doi: 10.3389/fmicb.2020.01366
Yang, F. Y., Saqib, H. S. A., Chen, J. H., Ruan, Q. Q., Vasseur, L., He, W. Y., et al. (2020). Differential profiles of gut microbiota and metabolites associated with host shift of Plutella xylostella. Int. J. Mol. Sci. 21:6283. doi: 10.3390/ijms21176283
Yong, H. S., Song, S. L., Chua, K. O., and Lim, P. E. (2017). High diversity of bacterial communities in developmental stages of Bactrocera carambolae (Insecta: Tephritidae) revealed by Illumina MiSeq sequencing of 16S rRNA gene. Curr. Microbiol. 74, 1076–1082. doi: 10.1007/s00284-017-1287-x
Yuan, X., Zhang, X., Liu, X., Dong, Y., Yan, Z., Lv, D., et al. (2021). Comparison of gut bacterial communities of Grapholita molesta (Lepidoptera: Tortricidae) reared on different host plants. Int. J. Mol. Sci. 22:6843. doi: 10.3390/ijms22136843
Zhang, Z., Jiao, S., Li, X., and Li, M. (2018). Bacterial and fungal gut communities of Agrilus mali at different developmental stages and fed different diets. Sci. Rep. 8:15634. doi: 10.1038/s41598-018-34127-x
Zhang, H., Wang, Y., Chen, S., Zhao, Z., Feng, J., Zhang, Z., et al. (2018). Water bacterial and fungal community compositions associated with Urban Lakes, Xi'an, China. Int. J. Environ. Res. Public Health 15:469. doi: 10.3390/ijerph15030469
Zhao, M. (2021). The biodiversity of Helicoverpa armigera symbiotic BA cteria and its regulatory effects on the defense response in cotton. Wuhan: Huazhong Agricultural University.
Zheng, S., Liu, W., Luo, J., Wang, L., Zhu, X., Gao, X., et al. (2022). Helicoverpa armigera herbivory negatively impacts Aphis gossypii populations via inducible metabolic changes. Pest Manag. Sci. 78, 2357–2369. doi: 10.1002/ps.6865
Keywords: Helicoverpa armigera, gut yeast microbiota, culture-independent yeast, host diet, high-throughput sequencing
Citation: Yu M, Li Y, Ji J, Lei Y and Sun Y (2024) Gut yeast diversity of Helicoverpa armigera (Lepidoptera: Noctuidae) under different dietary conditions. Front. Microbiol. 15:1287083. doi: 10.3389/fmicb.2024.1287083
Edited by:
Takema Fukatsu, National Institute of Advanced Industrial Science and Technology (AIST), JapanReviewed by:
Marina S. Ascunce, Agricultural Research Service (USDA), United StatesYunqiu Yang, Anhui Agricultural University, China
Waqas Wakil, University of Agriculture, Faisalabad, Pakistan
Copyright © 2024 Yu, Li, Ji, Lei and Sun. This is an open-access article distributed under the terms of the Creative Commons Attribution License (CC BY). The use, distribution or reproduction in other forums is permitted, provided the original author(s) and the copyright owner(s) are credited and that the original publication in this journal is cited, in accordance with accepted academic practice. No use, distribution or reproduction is permitted which does not comply with these terms.
*Correspondence: Yonghui Lei, NDk3OTc2QHFxLmNvbQ==; Yanfei Sun, ODE3MTEzMDhAcXEuY29t