- School of Agriculture, Meiji University, Kawasaki, Kanagawa, Japan
Cyanobacteria serve as useful hosts in the production of substances to support a low-carbon society. Specifically, the unicellular cyanobacterium Synechocystis sp. PCC 6803 (Synechocystis 6803) can produce organic acids, such as acetate, lactate, and succinate, as well as hydrogen, under dark, anaerobic conditions. The efficient production of these compounds appears to be closely linked to the regulation of intracellular redox balance. Notably, alterations in intracellular redox balance have been believed to influence the production of organic acids and hydrogen. To achieve these alterations, genetic manipulations involved overexpressing malate dehydrogenase (MDH), knocking out D-lactate dehydrogenase (DDH), or knocking out acetate kinase (AK), which subsequently modified the quantities and ratios of organic acids and hydrogen under dark, anaerobic conditions. Furthermore, the mutants generated displayed changes in the oxidation of reducing powers and the nicotinamide adenine dinucleotide hydrogen (NADH)/NAD+ ratio when compared to the parental wild-type strain. These findings strongly suggest that intracellular redox balance, especially the NADH/NAD+ ratio, plays a pivotal role in the production of organic acids and hydrogen in Synechocystis 6803.
1 Introduction
In recent years, the issue of fossil fuel depletion and climate change has gained prominence due to global population growth (Stephens et al., 2010; Baicha et al., 2016). The use of fossil fuels for energy and as raw materials in the chemical industry releases greenhouse gasses such as carbon dioxide (CO2), making it a significant contributor to climate change, including phenomena like global warming and ocean acidification (Baicha et al., 2016). Studies predict that if the world’s energy demand relies solely on fossil fuels, these finite reserves will be exhausted between 2069 and 2088 (Stephens et al., 2010). The low-carbon technologies are essential for transitioning to a carbon-neutral society and economy (Yamane and Osanai, 2023). Cyanobacteria, photosynthetic bacteria capable of producing various metabolites from CO2, have attracted attention as ideal hosts for these challenges (Oliver et al., 2016; Katayama et al., 2018; Yamane and Osanai, 2023).
Synechocystis sp. PCC 6803, referred to as Synechocystis 6803 hereafter, is a non-nitrogen-fixing unicellular cyanobacterium. It is widely employed as a model organism in both basic and applied studies (Vijay et al., 2019) due to its whole genomic information (Kaneko et al., 1996), natural transformation capability (Yu et al., 2013), and resilience to cryopreservation. Synechocystis 6803 fixes carbon dioxide via oxygenic photosynthesis and stores glycogen when subjected to nitrogen starvation (Neumann et al., 2021). Under dark, anaerobic conditions, glycogen is metabolized via the glycogen catabolism pathway, leading to the excretion of organic acids, including acetate, lactate, and the four-carbon dicarboxylic acids, including succinate, fumarate, and malate (Stal and Moezelaar, 1997; Osanai et al., 2015; Hasunuma et al., 2016). Notably, succinate, fumarate, and malate are listed in the twelve most important building block chemicals in biorefinery, as recognized by the U.S. Department of Energy (Werpy and Petersen, 2004). These compounds find universal applications in the food, pharmaceutical, and industrial sectors (Chen and Nielsen, 2016; Dai et al., 2018; Shi et al., 2022). Additionally, Synechocystis 6803 exhibits the ability to produce hydrogen under dark, anaerobic conditions, along with organic acids (Stal and Moezelaar, 1997; Tamagnini et al., 2007). Hydrogen is considered a promising energy resource due to its remarkable energy yield per unit mass, which surpasses hydrocarbon fuels by a factor of 2.75, and its emission of zero greenhouse gasses (Levin et al., 2004; Kapdan and Kargi, 2006). As a result, the diverse range of fermentation metabolites produced by Synechocystis 6803 has the potential to substitute for petroleum-derived substances, contributing significantly to the realization of a carbon-neutral society.
Organic acids and hydrogen production by Synechocystis 6803, while promising, has not yet reached industrial-scale application (Dai et al., 2018; Liu et al., 2022; Opel et al., 2023). Under dark, anaerobic conditions, in Synechocystis 6803, acetate is produced by acetate kinase (ackA) (sll1299), lactate by D-lactate dehydrogenase (ddh) (slr1556), and four-carbon dicarboxylic acids by malate dehydrogenase (MDH) (encoded by citH) (sll0891), fumarase (fumC) (slr0018), and succinate dehydrogenase (sdhA, sdhB) (slr1233, sll0823, sll1625) (as illustrated in Figure 1). Acetate, lactate, and four-carbon dicarboxylic acids compete for carbon intermediates (phosphoenolpyruvate and pyruvate) between pathways (Osanai et al., 2015; Hasunuma et al., 2016; Iijima et al., 2021). Extensive research efforts have been undertaken to improve the production rate, yield, and titer of lactate and four-carbon dicarboxylic acids in terms of carbon intermediate competition. This involves the regulation of specific gene expression and the engineering of metabolic pathways (Khanna and Lindblad, 2015; Oliver et al., 2016; Kamshybayeva et al., 2022; Yamane and Osanai, 2023). Notably, when acetate kinase (AK) is lacking in the ΔackA strain, acetate production decreases while lactate and succinate production increases compared to the parental wild-type strain (Osanai et al., 2015). Conversely, overexpressing MDH, which catalyzes the conversion of oxaloacetate to malate, increases the production of four-carbon dicarboxylic acids while reducing acetate and lactate production (Iijima et al., 2021). On the other hand, in Δddh lacking lactate dehydrogenase (DDH), the production of succinate and lactate is lower than in the parental wild-type strain (Osanai et al., 2015). Additionally, in Δacs lacking acetyl-CoA synthetase (encoded by acs) (sll0542), which interconverts acetyl-CoA and acetate, the production of each organic acid decreases compared to the parental wild-type strain. However, the relative proportions of the organic acids remain similar to those in the parental wild-type strain (Osanai et al., 2015). Furthermore, the overexpression of phosphoenolpyruvate carboxylase (PEPC) (encoded by ppc) (sll0920), responsible for generating oxaloacetate from phosphoenolpyruvate (PEP) and bicarbonate ions, increases acetate and succinate production but does not significantly affect lactate production (Hasunuma et al., 2016). In addition to the regulation of the expression of genes related to organic acids metabolism, the pool size and turnover of metabolites have been analyzed by metabolomic analysis using a stable isotope such as 13C, and understanding of carbon source competition is steadily advancing (Hasunuma et al., 2018). However, it is important to note that while competition for carbon sources or intermediates plays an important role in organic acids production under dark, anaerobic conditions, it is also regulated by many other factors beyond carbon competition.
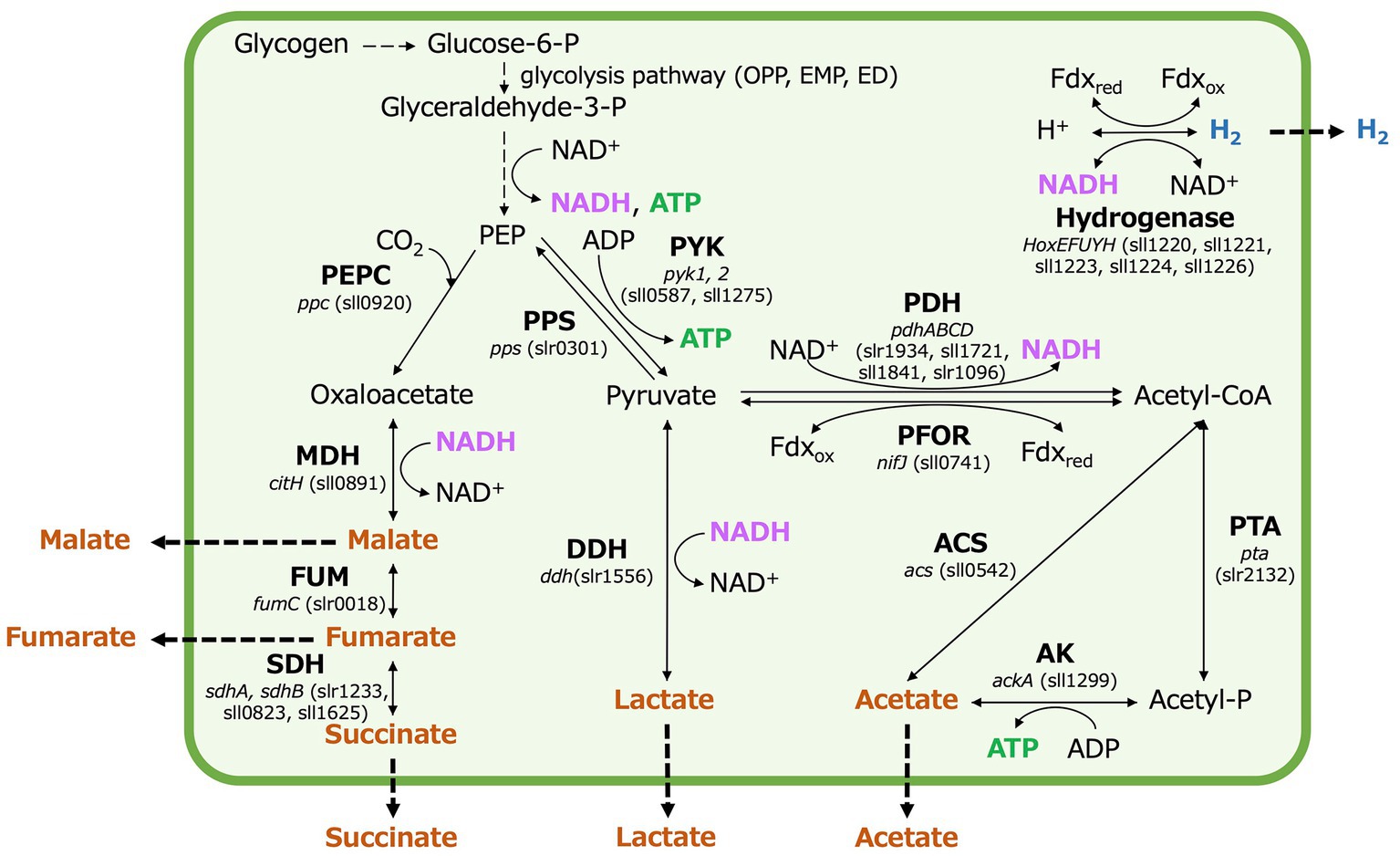
Figure 1. Production of fermentation metabolites in Synechocystis 6803 under dark, anaerobic conditions, with a focus on organic acid and hydrogen, nicotinamide adenine dinucleotide hydrogen (NADH), ferredoxin, and adenosine triphosphate (ATP) production. P designates phosphate. OPP; oxidative pentose phosphate, EMP; Embden–Meyerhof–Parnas, ED; the Entner–Doudoroff, PEP; phosphoenolpyruvate, PEPC; phosphoenolpyruvate carboxylase, PPS; phosphoenolpyruvate synthase, PYK; pyruvate kinase, MDH; malate dehydrogenase, FUM; fumarase, SDH; succinate dehydrogenase, DDH; D-lactate dehydrogenase, PDH; pyruvate dehydrogenase, PFOR; pyruvate: ferredoxin oxidoreductase, Fdxred; reduced ferredoxin, Fdxox; oxidized ferredoxin, ACS; acetyl-CoA synthetase, PTA; phosphotransacetylase, and AK; acetate kinase.
Hydrogen production by Synechocystis 6803 is catalyzed by a bidirectional hydrogenase (Carrieri et al., 2011), which can utilize NADH, reduced ferredoxin, and flavodoxin as substrates (Gutekunst et al., 2014). This hydrogenase plays a pivotal role in regulating the redox balance under dark, anaerobic conditions (Appel et al., 2000; Pinto et al., 2012). Additionally, when DDH and MDH in Synechocystis 6803 function as part of the fermentation pathway, they also utilize NADH (Angermayr et al., 2016; Takeya et al., 2018). Alterations in the expression of ddh, citH, and hoxH result in changes in NADH utilization, leading to either an increase or decrease in hydrogen production under dark, anaerobic conditions (Chongsuksantikul et al., 2015; Iijima et al., 2016, 2021). A flexible balance of ATP and NAD(P)H is crucial for metabolite production in Synechocystis 6803, whether in photoautotrophic or mixotrophic conditions (Kugler and Stensjö, 2023). In addition, lowering the adenylate energy charge has proven effective for succinate production under dark, anaerobic conditions in Synechocystis 6803 (Hasunuma et al., 2018). It is worth noting that the NADPH pool of Synechocystis 6803 is larger than the NADH pool (Takahashi et al., 2008), making the use of NADPH-dependent enzymes advantageous (Kugler and Stensjö, 2023). However, when transitioning to dark, anaerobic conditions, intracellular NADPH is entirely depleted (Osanai et al., 2015). The oxidative utilization of NADH by DDH, MDH, and hydrogenases is regulated at the gene expression level in response to environmental conditions, primarily due to differences in energy gain, substrate availability, and product formation within each fermentation pathway (Kariyazono and Osanai, 2023). Therefore, the intracellular redox balance of Synechocystis 6803 under dark, anaerobic conditions may be regulated differently compared to photoautotrophic and mixotrophic conditions. Such intracellular redox balance is expected to involve DDH, MDH, and hydrogenase, all of which use reducing power as substrates, as well as AK, which is crucial for ATP generation. Because of this complexity, there has been a notable absence of comprehensive discussions regarding carbon sources, the utilization of reducing power, intracellular ATP, or redox balance in metabolite production under dark anaerobic conditions. Furthermore, there is the technical difficulty of understanding all of the metabolites inside and outside the cell.
In this study, we conducted an analysis to explore the relationship between fermentation metabolite, organic acids and hydrogen production, and intracellular redox balance under dark, anaerobic conditions. We utilized various mutant strains of Synechocystis 6803 that had previously been genetically modified to increase organic acids and hydrogen production.
2 Materials and methods
2.1 Cyanobacterial strains and culture conditions
The glucose-tolerant (GT) strain of Synechocystis sp. PCC 6803, originally isolated by Williams (1988) and re-sequenced by Kanesaki et al. (2012), was cultivated in modified BG-11 medium, which consists of BG-110 liquid medium (Rippka, 1988), supplemented with 5 mM NH4Cl (buffered with 20 mM HEPES-KOH, pH 7.8). Several mutant strains, including Δddh (lacking lactate dehydrogenase), Δacs (lacking acetyl-CoA synthetase), ΔackA (lacking acetate kinase) (Osanai et al., 2015), CitHox, (overexpressing citH) (Iijima et al., 2021), ΔcitH (lacking malate dehydrogenase) were previously generated (Katayama et al., 2022). Cyanobacterial cultures were aerated with air containing 1% (v/v) CO2 and incubated at 30°C under continuous white light (~50–60 μmol photons m−2 s−1) at the 70 mL scale. The Δddh, Δacs, ΔackA, and ΔcitH strains were pre-cultivated in the presence of 10 μg/mL chloramphenicol, while the CitHox strain was pre-cultivated with 10 μg/mL kanamycin. Cell densities were assessed at a wavelength of 730 nm wavelength (OD730) using a Shimadzu UV-2700 spectrophotometer (Shimadzu, Kyoto, Japan).
2.2 Plasmid construction
The CitHox strain was constructed by the following procedure by Iijima et al. (2021). The nucleotides corresponding to open reading frame of Synechocystis 6803 citH (sll0891) with an N–terminal NdeI site and a C-terminal EcoRV site was synthesized at Eurofin Genomics Japan (Tokyo, Japan). The synthetic DNA was digested using NdeI and EcoRV; the fragment was cloned into the NdeI–HpaI sites of the pTKP2031 vector constructed by Satoh et al. (2001). The citH gene with the psbAII promoter (spanning −297 to +3 from the initiation codon of psbAII) was introduced into the neutral site within the open reading frame slr2031 with a kanamycin resistance in the Synechocystis 6803 genome by homologous recombination (Osanai et al., 2011). The plasmids were transformed into cells by natural transformation. Cell cultures (approximately 3 mL) were suspended to 100 μL, and 1 μL of plasmid solution (100 μg/mL) was added. The cell cultures were spread onto a mixed cellulose membrane on a BG-11 plate solidified with 1.5% agar and 2 mM sodium thiosulfate. After incubation at 30°C under continuous illumination (50 μmol photons m−2 s−1) overnight, the filter was transferred onto a BG-11 plate with 50 μg/mL kanamycin followed by incubation at 30°C under continuous illumination (50 μmol photons m−2 s−1) for 2–3 weeks. Colonies were re-streaked twice onto fresh BG-11 medium with kanamycin, and the strain overexpressing citH was designated as CitHox.
2.3 Dark and anaerobic incubation
Cells, initially cultured in 70 mL of modified BG-11 medium (started from OD730 = 0.4) for five days, were collected by centrifugation at 5800 × g for 2 min. Following the removal of the supernatant, the cells were concentrated in a 10 mL of a HEPES buffer (20 mM HEPES-KOH, pH 7.8) at OD730 = 20. This concentrated cell suspension was placed in a 20 mL GC-vial. The vials were flushed with N2 gas for 1 min using syringes to remove the oxygen and immediately sealed with butyl rubber and wrapped with aluminum foil. Subsequently, the vials were shaken at 30°C for three days. After this incubation period, the cell cultures underwent another round of centrifugation at 5800 × g for 2 min, following which the supernatant was filtered. A volume of 1 mL of the filtered supernatant was subjected to freeze-drying. The resulting dried supernatants were then utilized for the analysis of organic acids using high-performance liquid chromatography (HPLC).
2.4 Glycogen measurement
Equal volumes of cells (10 mL of cell culture with OD730 = 1.0) were collected through centrifugation (20,500 × g at 25°C for 1 min), with subsequent removal of the supernatant. The resulting cell pellets were then resuspended in 100 μL of 3.5% (w/v) sulfuric acid and subjected to incubation at 100°C for 80 min. Following another round of centrifugation (20,500 × g at 4°C for 1 min), 1.3 μL of the supernatant was combined with 200 μL of LabAssay Glucose reaction mixture (Fujifilm Wako Chemicals, Osaka, Japan) within a 96-well plate and incubated at 37°C for 15 min, and subsequently, the absorbance was measured at 595 nm using a Multiskan FC microplate reader (Thermo Scientific, MA, United States).
2.5 Measurement of excreted organic acids via high-performance liquid chromatography (HPLC)
The freeze-dried supernatants were reconstituted by dissolving in 100 μL of filtered 3 mM perchloric acid and analyzed by HPLC using an LC-2000Plus system (JASCO, Tokyo, Japan) equipped with two RSpak KC-811 columns (Showa Denko, Tokyo, Japan). Quantification of organic acids was achieved using a solution containing 0.2 mM bromothymol blue in 15 mM sodium phosphate buffer, with peak detection occurring at 445 nm. The column was maintained at a temperature of 60°C, and the flow rates were 0.7 mL/min for the 9 mM perchloric acid and 1.2 mL/min for the 0.2 mM bromothymol blue solution. To calibrate the measurements, standard powders of succinate, malate, fumarate, lactate, and acetate were employed, all of which were obtained from Fujifilm Wako Chemicals. Citrate powders were purchased from Nacalai Tesque, INC. (Kyoto, Japan).
2.6 Measurement of hydrogen evolution by gas chromatography-thermal conductivity detector (GC-TCD)
The cells were subjected to incubation under dark, anaerobic conditions, similar to the conditions used in the organic acids excretion experiment. The H2 gas that accumulated in the headspace of a GC-vial was quantified using a gas chromatograph (GC-2014 AT, Shimadzu, Kyoto, Japan). N2 was employed as the carrier gas, flowing at a flow rate of 10 mL/min.
2.7 Measurement of NADH and total NAD and NADH
Equal volumes of cells (1 mL of cell culture with OD730 = 50) were collected via centrifugation (300 × g at 25°C for 5 min) after the incubation period under dark, anaerobic conditions. The extraction and quantification of NAD+ from Synechocystis 6803 cells was carried out using the NAD/NADH Assay Kit-WST (Dojindo Laboratories, Kumamoto, Japan). The absorbance was measured at 450 nm using a Multiskan FC microplate reader (Thermo Scientific). To account for any background effects in visually colored samples, the absorbance before the reaction with the chromogenic dye mixture and the absorbance of the blank were considered.
2.8 Statistical analysis
The calculation of means and standard deviations, along with the determination of p-values, were conducted using Microsoft Excel 2019 MSO. All results were obtained through biologically independent replicates.
3 Result
3.1 Acetate kinase knockout increased the rate of glycogen consumption
Under continuous light and aerobic conditions, Synechocystis 6803 accumulates glycogen, utilizing it as a carbon and energy source under dark conditions (Stal and Moezelaar, 1997). After five days of light, aerobic cultivation, all strains, including GT, Δddh, Δacs, ΔackA, and CitHox, accounted similar glycogen levels for approximately 40–45% of cell dry weight (Figure 2). Subsequently, these cells were collected and concentrated in 10 mL of 20 mM HEPES buffer to OD730 = 20 before undergoing incubation under dark, anaerobic conditions for three days. Following this incubation, the glycogen levels in GT, Δddh, Δacs, and CitHox decreased by approximately 10%, and ΔackA decreased by approximately 20% compared to their initial glycogen levels before the dark, anaerobic incubation (Table 1).
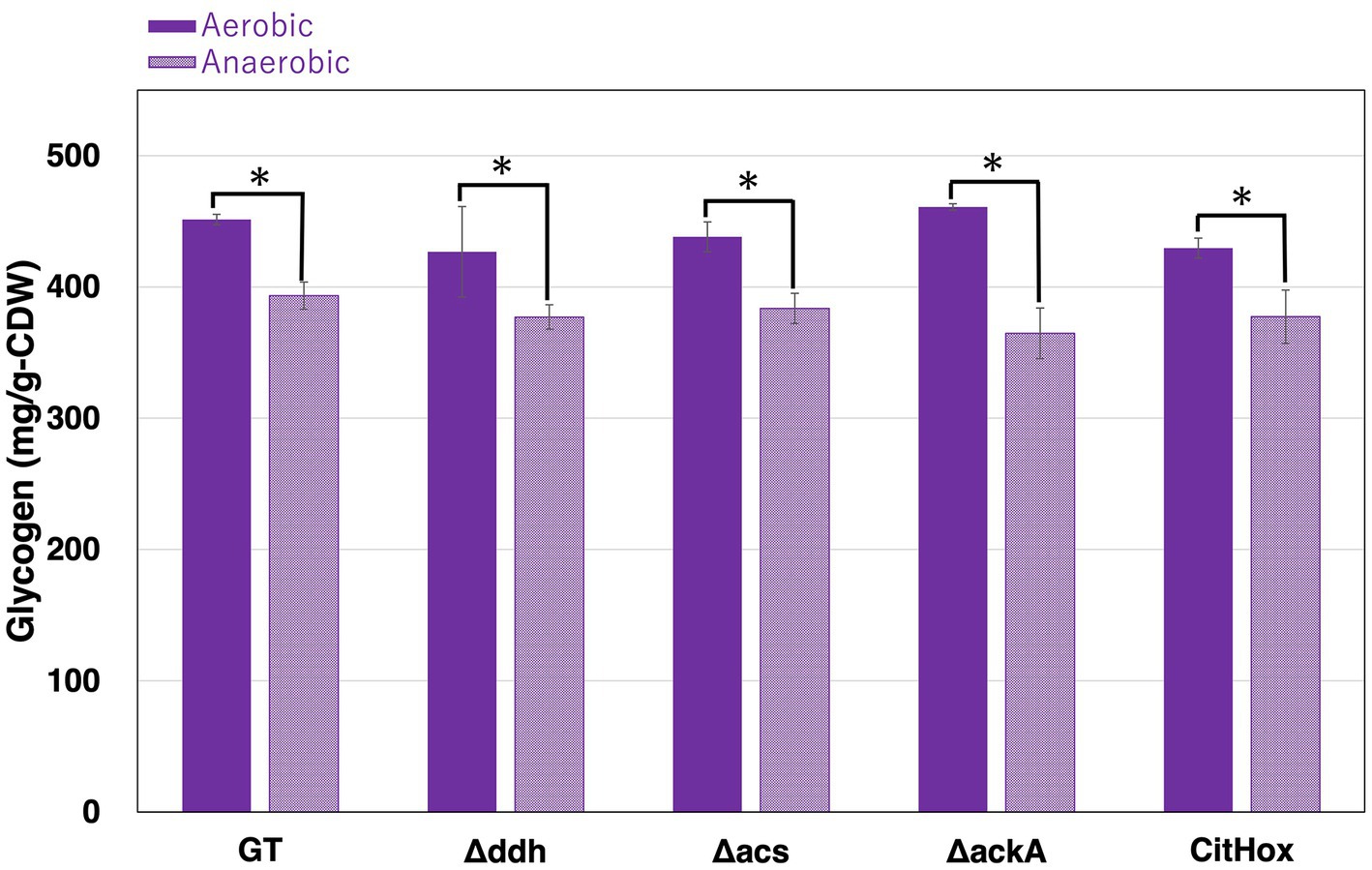
Figure 2. Changes in glycogen levels before and after dark, anaerobic conditions. Synechocystis 6803 GT, Δddh, Δacs, ΔackA, and CitHox were subjected to aerobic conditions with continuous illumination (50 μmol photons m−2 s−1) for five days. Following this, cells were incubated under dark, anaerobic conditions for three days. Glycogen levels were quantified using the LabAssay Glucose kit. The term “Anaerobic” indicates glycogen levels of the cells after three days of incubation under dark, anaerobic conditions. Data represents the means ± SD from four biologically independent experiments. Asterisks indicate statistically significant differences between glycogen levels before and after dark, anaerobic conditions for each strain (Student’s t-test; *p < 0.05).
3.2 Knockout of ddh, ackA, or overexpression of citH altered the amount of organic acids production
After five days of growth of GT, Δddh, Δacs, ΔackA, and CitHox under light, aerobic conditions, followed by three days of incubation under dark, anaerobic conditions, the excreted organic acids were quantified. The sum of four-carbon dicarboxylic acids (succinate, fumarate, and malate) differed among strains, with GT producing 0.35 ± 0.02 mM, ackA knockout yielding 0.69 ± 0.06 mM, and citH overexpression resulting in 0.92 ± 0.18 mM under the same conditions (Figure 3). In contrast, acs knockout decreased the sum of four-carbon dicarboxylic acids levels by 12%, while ddh knockout did not significantly affect these levels (Figure 3). Lactate levels also varied among strains, with GT producing 0.23 ± 0.01 mM, ackA knockout yielding 0.66 ± 0.05 mM, and citH overexpression resulting in 0.08 ± 0.03 mM (Figure 3). The ddh knockout led to undetectable lactate production, and acs knockout did not affect lactate levels (Figure 3). Regarding acetate production, GT produced 3.22 ± 0.25 mM, and ackA knockout produced 0.52 ± 0.09 mM (Figure 3). No significant changes in acetate levels were observed with citH overexpression, ddh or acs knockout (Figure 3). The sum of four-carbon dicarboxylic acids and lactate decreased by 41% for Δddh and increased by 136% for ΔackA and by 73% for CitHox compared to GT (Figure 3). Overall, the total organic acids production, encompassing the sum of four-carbon dicarboxylic acids, lactate, and acetate, decreased by 51% for ΔackA and increased by 25% for CitHox compared to GT (Figure 3).
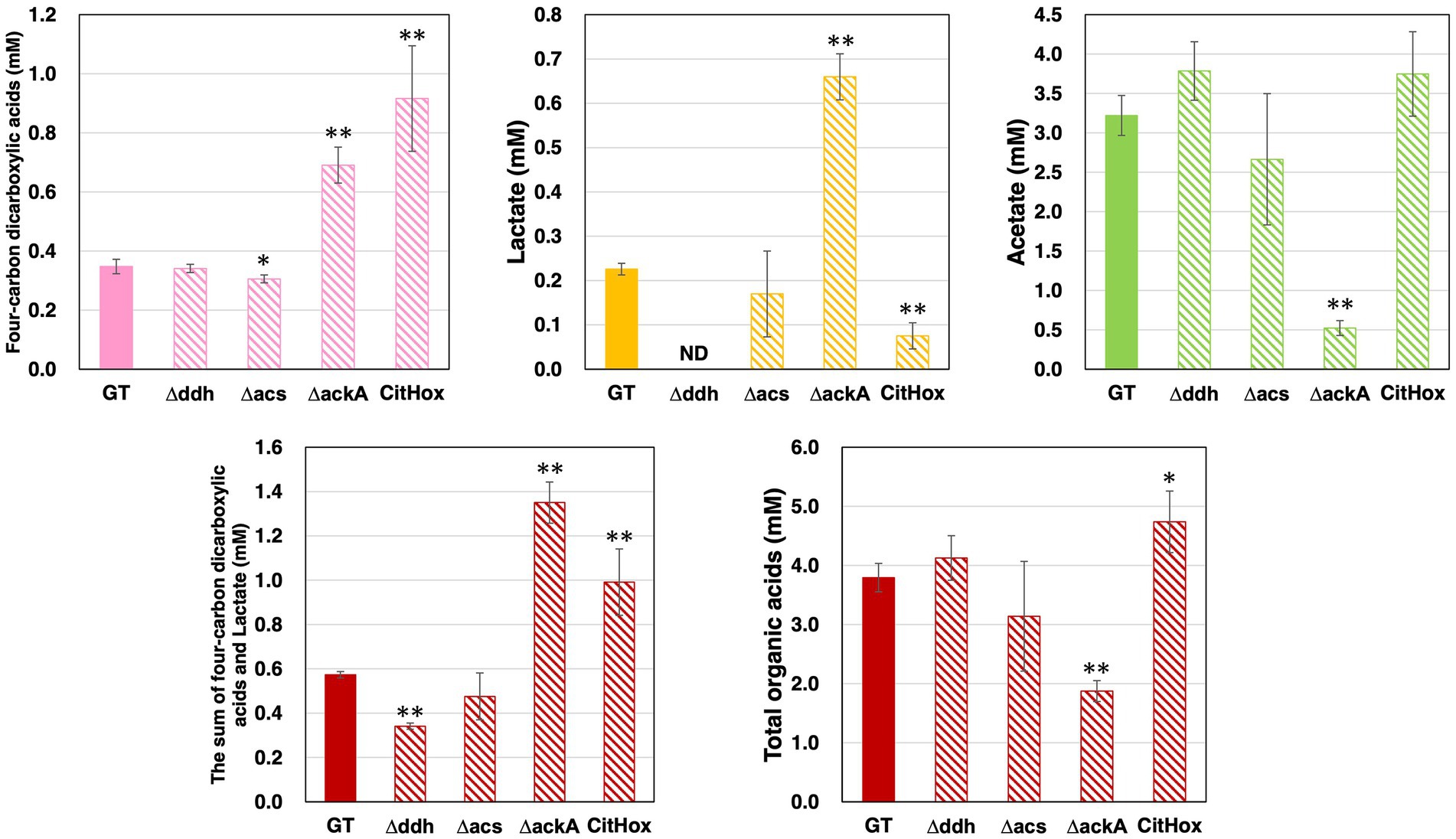
Figure 3. Quantification of four-carbon dicarboxylic acids, lactate, and acetate in Synechocystis 6803 GT, Δddh, Δacs, ΔackA, and CitHox under dark, anaerobic conditions. The levels of organic acids excreted from cells after three days of incubation under dark, anaerobic conditions were determined using high-performance liquid chromatography. Total organic acids represent the combined levels of four-carbon dicarboxylic acids, lactate, and acetate. Data is presented as means ± SD from three to four biologically independent experiments. Four-carbon dicarboxylic acids refer to the sum of succinate, fumarate, and malate. ND indicates undetectable levels. Asterisks indicate statistically significant differences between GT and the mutant strains (Student’s t-test; *p < 0.05, **p < 0.005).
3.3 Knockout or overexpression of genes related to organic acids production altered hydrogen production
Synechocystis 6803 produces hydrogen along with organic acids under dark anaerobic conditions (Stal and Moezelaar, 1997; Tamagnini et al., 2007). To examine the relationship between hydrogen and organic acid production, GT, Δddh, Δacs, ΔackA, CitHox, and ΔcitH were incubated under dark anaerobic conditions for 3 days, and hydrogen levels were quantified. GT produced hydrogen at a level of 27010 ± 3610 ppm, ackA knockout yielded 20463 ± 3228 ppm, and citH overexpression resulted in 11906 ± 2694 ppm under the same conditions (Figure 4). The ddh or acs knockout did not significantly affect hydrogen levels (Figure 4). In contrast, when citH was knocked out, hydrogen production increased by 137% to 36896 ± 2475 ppm compared to GT (Supplementary Figure S1a).
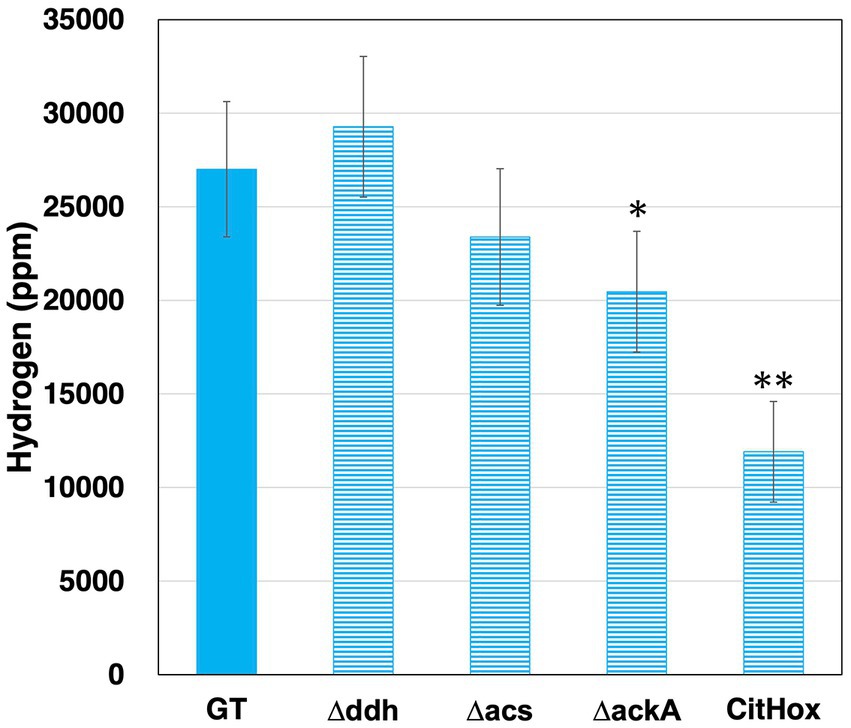
Figure 4. Quantification of hydrogen levels in Synechocystis 6803 GT, Δddh, Δacs, ΔackA, and CitHox cells under dark, anaerobic conditions. The concentration of hydrogen was determined using gas chromatography with a thermal conductivity detector. Data is presented as means ± SD from three to four biologically independent experiments. Asterisks indicate statistically significant differences between GT and the mutant strains (Student’s t-test; *p < 0.05, **p < 0.005).
3.4 Knockout or overexpression of genes related to organic acids production altered intracellular NADH/NAD+ ratios
In Δddh, ΔackA, CitHox, and ΔcitH, hydrogen production and/or the sum of four-carbon dicarboxylic acids and lactate altered. To determine whether changes in the utilization of pathways involving NADH oxidation resulted in differences the percentage of oxidized forms, intracellular NADH levels and the sum of NAD+ and NADH were measured for GT, Δddh, Δacs, ΔackA, CitHox, and ΔcitH. To determine the intracellular NAD+ levels, we subtracted the amount of NADH measured from the total sum of NAD+ and NADH. After subjecting the cells to three days of dark, anaerobic culture, we observed that the intracellular NADH/NAD+ ratio decreased to 0.39 ± 0.15 by the ackA knockout and to 0.70 ± 0.09 by citH overexpression, with GT under the same conditions being set at 1 (Figure 5). In contrast, the ddh or citH knockout did not lead to any significant alteration in the NADH/NAD+ ratio (Figures 5 and Supplementary Figure S1b).
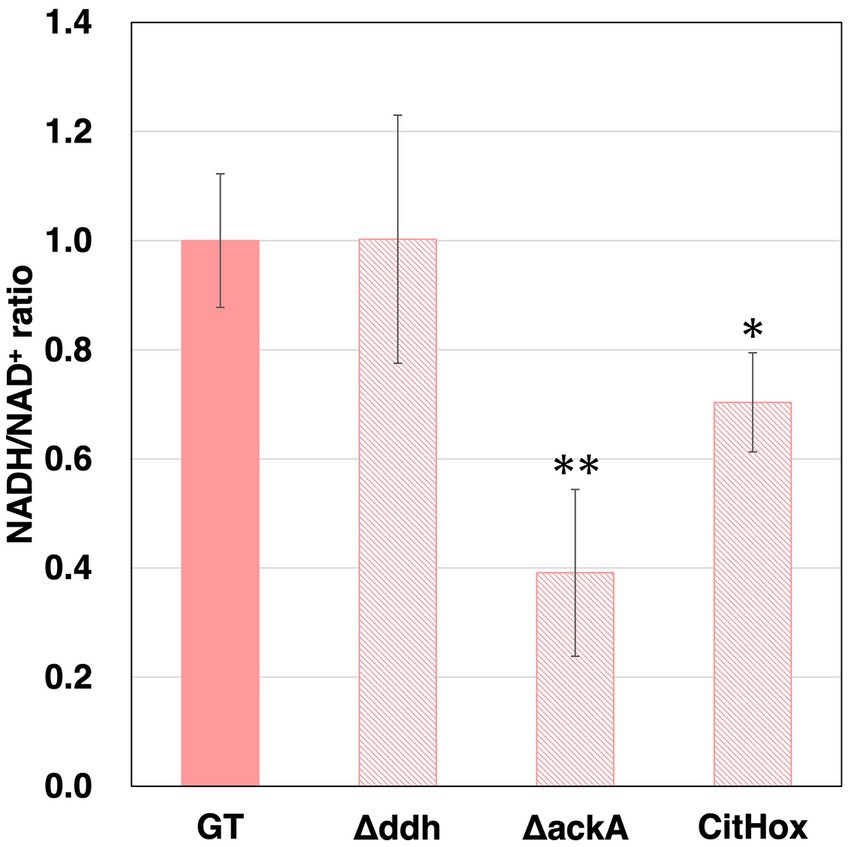
Figure 5. Intracellular NADH/NAD+ ratios of Synechocystis 6803 GT, Δddh, ΔackA, and CitHox cells under dark, anaerobic conditions. NADH and the total sum of NAD+ and NADH levels were quantified using the NAD/NADH Assay Kit-WST. The intracellular NADH/NAD+ ratios were calculated from the NADH levels and the total sum of NAD+ and NADH within the cells. The data are presented as relative values, with the values of GT set as 1 after three days of dark, anaerobic incubation. Data is presented as means from four biologically independent experiments. These results represent means from four biologically independent experiments. Asterisks indicate statistically significant differences in the ratios between GT and the mutant strain (Student’s t-test; *p < 0.05, **p < 0.005).
4 Discussion
In this study, we demonstrated the correlation between organic acid and hydrogen production and NADH/NAD+ ratios under dark, anaerobic conditions in Synechocystis 6803. Since PEP and pyruvate are competed as carbon intermediates in organic acid production, the perspective of carbon intermediate competition is important, and the regulation of the expression of genes related to organic acid metabolism and metabolomic analysis has been conducted (Osanai et al., 2015; Hasunuma et al., 2016; Hidese et al., 2020). NADH is essential for the catalytic reactions of MDH and DDH, which metabolize carbon intermediates to organic acids, however, there is a lack of references focusing on NADH, and this study focuses on NADH. Since NAD kinase (sll1415, slr0400), which catalyzes NAD+ to NADP+ conversion, plays an important role in Synechocystis 6803 under heterotrophic conditions (Ishikawa et al., 2016, 2021), NADPH may also act as an electron donor. However, NADH-dependent enzymes act in Synechocystis 6803 under dark, anaerobic conditions (Katayama et al., 2022), and hence, NADH is a major electron donor under dark, anaerobic conditions in this cyanobacterium.
Under dark, anaerobic conditions, the ackA knockout resulted in a 1.7-fold increase in glycogen consumption compared to GT, while the ddh or acs knockout or citH overexpression had no significant impact (Table 1). Also, the interruption of acetate production resulted in a 2.9- and 2.0-fold increase in lactate production and the sum of four-carbon dicarboxylic acids, respectively (Figure 3). Notably, acetate is a prominent fermentation metabolite in Synechocystis 6803 under these conditions (Osanai et al., 2015; Iijima et al., 2021), and its biosynthesis is believed to be an AK dependent pathway (Osanai et al., 2015). AK is also involved in ATP generation, and the absence of ackA leads to decreased intracellular ATP levels and alterations in carbon flow. The decrease of glycogen levels in the ackA knockout compared to GT suggest that an increased flow toward alternative ATP-generating pathways, such as pyruvate kinase (PYK), potentially accelerating the glucose catabolic pathway (Table 1). Indeed, the activation of PYK in Synechocystis 6803 is known to enhance sugar catabolic flux (Knowles and Plaxton, 2003). By the ackA knockout, the increased demand for ATP generation by PYK may be accompanied by an increased flow toward pyruvate. Increase in glucose catabolism is also accompanied by increased generation of NADH (Figure 2). Also, PEP is a substrate for PYK and a key branching point in organic acids synthesis (Osanai et al., 2015; Hasunuma et al., 2016; Iijima et al., 2021). The observed increase in lactate and four-carbon dicarboxylic acids by the ackA knockout compared to GT may be due to altered carbon source allocation and an increased supply of NADH as a substrate for MDH and DDH through glucose catabolic pathway, with the greater percentage increase in lactate potentially linked to ATP generation.
In Synechocystis 6803 under dark, anaerobic conditions, the acetate production pathway was found to affect NADH oxidation (Figure 5). Decreased hydrogen production due to the ackA knockout indicates decreased consumption of NADH or reduced ferredoxin by hydrogenases (Figure 4). Increased four-carbon dicarboxylic acids and lactate production and decreased NADH/NAD+ ratios by the ackA knockout are due to increased NADH oxidation by MDH and DDH (Figures 3, 5), explaining the decreased hydrogen production in terms of NADH competition. A decrease in acetate was also observed by the ackA knockout (Figure 3). Pyruvate dehydrogenase (PDH) and pyruvate:ferredoxin oxidoreductase (PFOR), which catalyze pyruvate decarboxylation, produce NADH or reduced ferredoxin, respectively and contribute to providing reducing power (Figure 6) (Tittmann, 2009). The decrease in acetate suggests a decrease in flow to the acetate production pathway through PDH and PFOR and a decrease in the supply of reducing power to hydrogenase, reinforcing the reason for the decrease in hydrogen production.
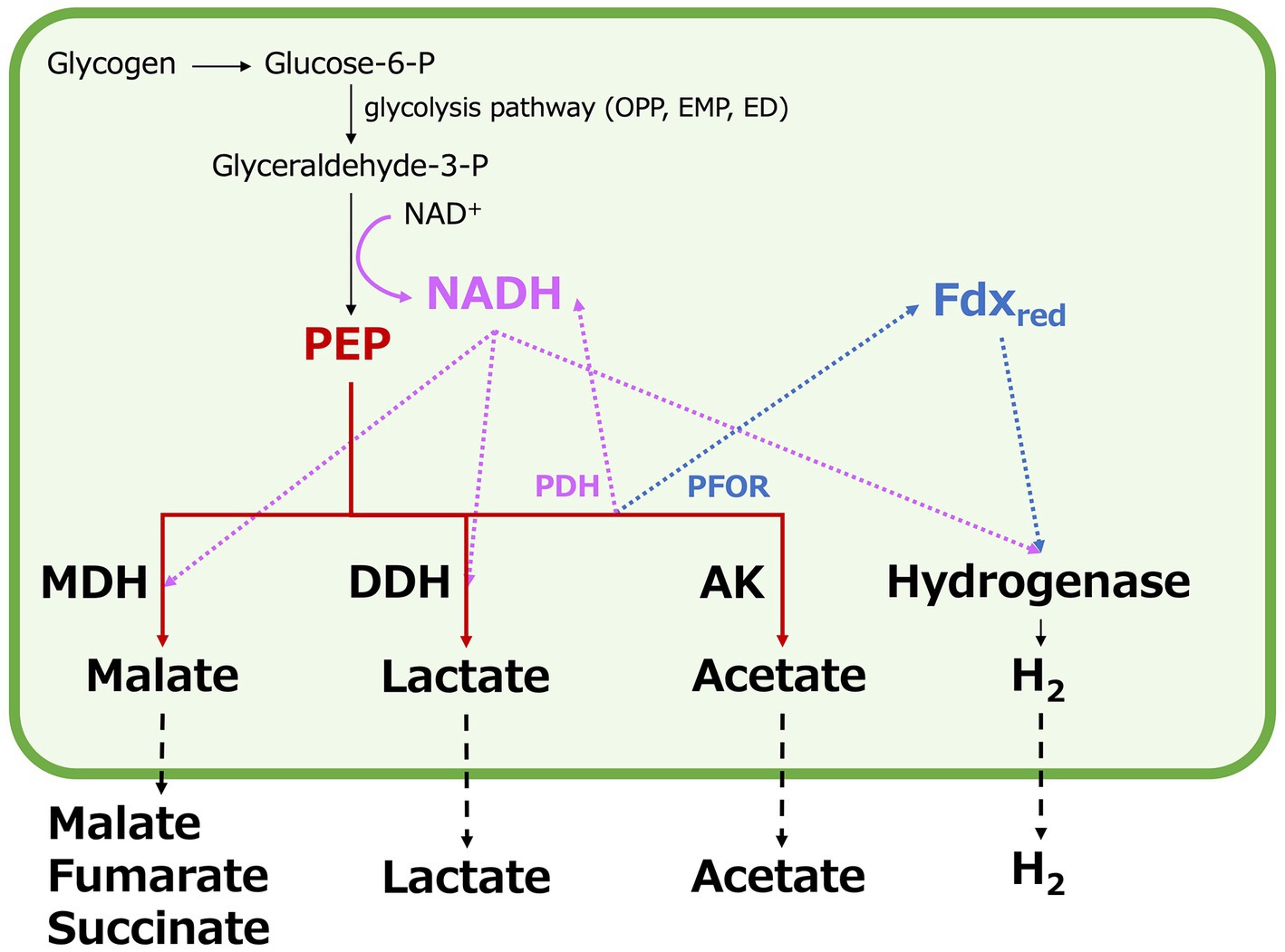
Figure 6. Schematic model of competition for carbon intermediates and reducing power by organic acids and hydrogen production in Synechocystis 6803 under dark, anaerobic conditions. P designates phosphate. OPP; oxidative pentose phosphate, EMP; Embden–Meyerhof–Parnas, ED; the Entner–Doudoroff, PEP; phosphoenolpyruvate, MDH; malate dehydrogenase, DDH; D-lactate dehydrogenase, PDH; pyruvate dehydrogenase, PFOR; pyruvate: ferredoxin oxidoreductase, Fdxred; reduced ferredoxin, and AK; acetate kinase.
The low NADH/NAD+ ratio by citH overexpression suggests increased NADH oxidation by MDH (Figure 5). Compared to GT, overexpression of citH resulted in a 2.6-, 0.3-, and 0.4-fold increase in four-carbon dicarboxylic acids, lactate, and hydrogen production, respectively (Figures 3, 4). The increase in four-carbon dicarboxylic acids due to citH overexpression is consistent with previous results (Iijima et al., 2021). The decrease in lactate may result from competition for NADH and the carbon source between MDH and DDH, while the decrease in hydrogen may result from competition for NADH between MDH and hydrogenase (Figure 6). The overall increase in total organic acids production by 25% by the citH overexpression compared to GT (Figure 4) may be attributed to a concurrent increase in carbon dioxide assimilation by phosphoenolpyruvate carboxylase (PEPC), an enzyme acting just before MDH.
The ddh knockout did not lead to an increase in the production of four-carbon dicarboxylic acids and hydrogen (Figures 3, 4). Also, acetate production, which supplies reducing power, did not increase by ddh knockout, and the NADH/NAD+ ratio was maintained at the same level as in GT (Figures 3, 5), suggesting that the ddh knockout has a limited effect on the production of fermentation products with oxidation of reducing power by MDH or hydrogenase. Meanwhile, DDH has the capacity to utilize oxaloacetate as a substrate, albeit with only approximately 20% of the activity for pyruvate (Ito et al., 2017). This suggests a potential involvement of DDH in succinate production. Thus, it is possible the production of four-carbon dicarboxylic acids by MDH was not increased in appearance by the ddh knockout.
The citH knockout resulted in a 1.4-fold increase in hydrogen production compared to GT (Supplementary Figure S1a). Notably, the catalytic efficiency (kcat/Km) for NADH analyzed at close pH and temperature differs between MDH and DDH, with MDH exhibiting a higher value of 1512 ± 274 s−1 mM−1 (pH 7.8, 30°C) compared to DDH’s 94.33 ± 7.83 s−1 mM−1 (pH 7.5, 30°C) (Ito et al., 2017; Katayama et al., 2022). This observation highlights that MDH is more efficient in catalyzing NADH oxidation than DDH, particularly in the presence of sufficient NADH. The results from our study support the notion that MDH plays a more significant role in NADH oxidation compared to DDH, in line with biochemical analysis.
Our study has demonstrated that the intracellular redox balance of Synechocystis 6803, particularly the NADH/NAD+ ratio, correlates with the production of organic acids and hydrogen under dark, anaerobic conditions. Specifically, if the NADH/NAD+ ratio value of the mutant strain is lower than that of GT, production of four-carbon dicarboxylic acids and lactate is likely to occur, while hydrogen production is unfavorable. This finding provides a novel perspective on organic acids and hydrogen production under dark, anaerobic conditions by Synechocystis 6803. In estimating the amount of extracellular metabolites, the measurement of the NADH/NAD+ ratio was useful and decreased the number of parameters to be measured. However, it remains elucidated whether the changes in NADH/NAD+ ratio is caused by the altered production of fermentation products or the changes in NADH/NAD+ ratio altered the production of fermentation products. Further experiments are needed to clarify these correlations and causal relationships.
Data availability statement
The original contributions presented in the study are included in the article/Supplementary material, further inquiries can be directed to the corresponding author.
Author contributions
MA: Data curation, Formal analysis, Investigation, Writing – original draft. TO: Data curation, Funding acquisition, Writing – original draft.
Funding
The author(s) declare financial support was received for the research, authorship, and/or publication of this article. This research received support from the Japan Science and Technology Agency through JST-ALCA (grant number JPMJAL1306), JST-GteX (grant number JPMJGX23B0) and the Asahi Glass Foundation.
Conflict of interest
The authors declare that the research was conducted in the absence of any commercial or financial relationships that could be construed as a potential conflict of interest.
The author(s) declared that they were an editorial board member of Frontiers, at the time of submission. This had no impact on the peer review process and the final decision.
Publisher’s note
All claims expressed in this article are solely those of the authors and do not necessarily represent those of their affiliated organizations, or those of the publisher, the editors and the reviewers. Any product that may be evaluated in this article, or claim that may be made by its manufacturer, is not guaranteed or endorsed by the publisher.
Supplementary material
The Supplementary material for this article can be found online at: https://www.frontiersin.org/articles/10.3389/fmicb.2023.1332449/full#supplementary-material
References
Angermayr, S. A., van der Woude, A. D., Correddu, D., Kern, R., Hagemann, M., and Hellingwerf, K. J. (2016). Chirality matters: synthesis and consumption of the d-enantiomer of lactic acid by Synechocystis sp. strain PCC6803. Appl. Environ. Microbiol. 82, 1295–1304. doi: 10.1128/AEM.03379-15
Appel, J., Phunpruch, S., Steinmüller, K., and Schulz, R. (2000). The bidirectional hydrogenase of Synechocystis sp. PCC 6803 works as an electron valve during photosynthesis. Arch. Microbiol. 173, 333–338. doi: 10.1007/s002030000139
Baicha, Z., Salar-García, M. J., Ortiz-Martínez, V. M., Hernández-Fernández, F. J., De Los Ríos, A. P., Labjar, N., et al. (2016). A critical review on microalgae as an alternative source for bioenergy production: a promising low cost substrate for microbial fuel cells. Fuel Process. Technol. 154, 104–116. doi: 10.1016/j.fuproc.2016.08.017
Carrieri, D., Wawrousek, K., Eckert, C., Yu, J., and Maness, P. C. (2011). The role of the bidirectional hydrogenase in cyanobacteria. Bioresour. Technol. 102, 8368–8377. doi: 10.1016/j.biortech.2011.03.103
Chen, Y., and Nielsen, J. (2016). Biobased organic acids production by metabolically engineered microorganisms. Curr. Opin. Biotechnol. 37, 165–172. doi: 10.1016/j.copbio.2015.11.004
Chongsuksantikul, A., Asami, K., Yoshikawa, S., and Ohtaguchi, K. (2015). Dark anaerobic hydrogen production in the mutants of Synechocystis sp. strain PCC6803-GT defective in lactate dehydrogenase activity and/or alcohol dehydrogenase activity, incubated in buffer solutions with or without glucose. Int J Biol 7, 33–45. doi: 10.5539/ijb.v7n1p33
Dai, Z., Zhou, H., Zhang, S., Gu, H., Yang, Q., Zhang, W., et al. (2018). Current advance in biological production of malic acid using wild type and metabolic engineered strains. Bioresour. Technol. 258, 345–353. doi: 10.1016/j.biortech.2018.03.001
Gutekunst, K., Chen, X., Schreiber, K., Kaspar, U., Makam, S., and Appel, J. (2014). The bidirectional NiFe-hydrogenase in Synechocystis sp. PCC 6803 is reduced by flavodoxin and ferredoxin and is essential under mixotrophic, nitrate-limiting conditions. J. Biol. Chem. 289, 1930–1937. doi: 10.1074/jbc.M113.526376
Hasunuma, T., Matsuda, M., Kato, Y., Vavricka, C. J., and Kondo, A. (2018). Temperature enhanced succinate production concurrent with increased central metabolism turnover in the cyanobacterium Synechocystis sp. PCC 6803. Metab. Eng. 48, 109–120. doi: 10.1016/j.ymben.2018.05.013
Hasunuma, T., Matsuda, M., and Kondo, A. (2016). Improved sugar-free succinate production by Synechocystis sp. PCC 6803 following identification of the limiting steps in glycogen catabolism. Metab Eng Commun 3, 130–141. doi: 10.1016/j.meteno.2016.04.003
Hidese, R., Matsuda, M., Osanai, T., Hasunuma, T., and Kondo, A. (2020). Malic enzyme facilitates D-lactate production through increased pyruvate supply during anoxic dark fermentation in Synechocystis sp. PCC 6803. ACS Synth. Biol. 9, 260–268. doi: 10.1021/acssynbio.9b00281
Iijima, H., Shirai, T., Okamoto, M., Pinto, F., Tamagnini, P., Hasunuma, T., et al. (2016). Metabolomics-based analysis revealing the alteration of primary carbon metabolism by the genetic manipulation of a hydrogenase HoxH in Synechocystis sp. PCC 6803. Algal Res. 18, 305–313. doi: 10.1016/j.algal.2016.06.026
Iijima, H., Watanabe, A., Sukigara, H., Iwazumi, K., Shirai, T., Kondo, A., et al. (2021). Four-carbon dicarboxylic acid production through the reductive branch of the open cyanobacterial tricarboxylic acid cycle in Synechocystis sp. PCC 6803. Metab. Eng. 65, 88–98. doi: 10.1016/j.ymben.2021.03.007
Ishikawa, Y., Cassan, C., Kadeer, A., Yuasa, K., Sato, N., Sonoike, K., et al. (2021). The NAD kinase Slr0400 functions as a growth repressor in Synechocystis sp. PCC 6803. Plant Cell Physiol. 62, 668–677. doi: 10.1093/pcp/pcab023
Ishikawa, Y., Miyagi, A., Haishima, Y., Ishikawa, T., Nagano, M., Yamaguchi, M., et al. (2016). Metabolomic analysis of NAD kinase-deficient mutants of the cyanobacterium Synechocystis sp. PCC 6803. J. Plant Physiol. 205, 105–112. doi: 10.1016/j.jplph.2016.09.002
Ito, S., Takeya, M., and Osanai, T. (2017). Substrate specificity and allosteric regulation of a d-lactate dehydrogenase from a unicellular cyanobacterium are altered by an amino acid substitution. Sci. Rep. 7:15052. doi: 10.1038/s41598-017-15341-5
Kamshybayeva, G. K., Kossalbayev, B. D., Sadvakasova, A. K., Zayadan, B. K., Bozieva, A. M., Dunikov, D., et al. (2022). Strategies and economic feasibilities in cyanobacterial hydrogen production. Int. J. Hydrog. Energy 47, 29661–29684. doi: 10.1016/j.ijhydene.2022.06.277
Kaneko, T., Sato, S., Kotani, H., Tanaka, A., Asamizu, E., Nakamura, Y., et al. (1996). Sequence analysis of the genome of the unicellular cyanobacterium Synechocystis sp. strain PCC6803. II. Sequence determination of the entire genome and assignment of potential protein-coding regions. DNA Res. 3, 109–136. doi: 10.1093/dnares/3.3.109
Kanesaki, Y., Shiwa, Y., Tajima, N., Suzuki, M., Watanabe, S., Sato, N., et al. (2012). Identification of substrain-specific mutations by massively parallel whole-genome resequencing of Synechocystis sp. PCC 6803. DNA Res. 19, 67–79. doi: 10.1093/dnares/dsr042
Kapdan, I. K., and Kargi, F. (2006). Bio-hydrogen production from waste materials. Enzym. Microb. Technol. 38, 569–582. doi: 10.1016/j.enzmictec.2005.09.015
Kariyazono, R., and Osanai, T. (2023). CyAbrB2 in Synechocystis sp. PCC6803 is a cyanobacterial nucleoid-associated protein controlling the expression of hydrogenase under the fermentative condition bioRxiv.
Katayama, N., Iijima, H., and Osanai, T. (2018). Production of bioplastic compounds by genetically manipulated and metabolic engineered cyanobacteria. Adv. Exp. Med. Biol. 1080, 155–169. doi: 10.1007/978-981-13-0854-3_7
Katayama, N., Iwazumi, K., Suzuki, H., Osanai, T., and Ito, S. (2022). Malic enzyme, not malate dehydrogenase, mainly oxidizes malate that originates from the tricarboxylic acid cycle in cyanobacteria. MBio 13:e0218722. doi: 10.1128/mbio.02187-22
Khanna, N., and Lindblad, P. (2015). Cyanobacterial hydrogenases and hydrogen metabolism revisited: recent progress and future prospects. Int. J. Mol. Sci. 16, 10537–10561. doi: 10.3390/ijms160510537
Knowles, V. L., and Plaxton, W. C. (2003). From genome to enzyme: analysis of key glycolytic and oxidative pentose-phosphate pathway enzymes in the cyanobacterium Synechocystis sp. PCC 6803. Plant Cell Physiol. 44, 758–763. doi: 10.1093/pcp/pcg086
Kugler, A., and Stensjö, K. (2023). Optimal energy and redox metabolism in the cyanobacterium Synechocystis sp. PCC 6803. NPJ Syst Biol Appl 9:47. doi: 10.1038/s41540-023-00307-3
Levin, D. B., Pitt, L., and Love, M. (2004). Biohydrogen production: prospects and limitations to practical application. Int. J. Hydrog. Energy 29, 173–185. doi: 10.1016/S0360-3199(03)00094-6
Liu, X., Zhao, G., Sun, S., Fan, C., Feng, X., and Xiong, P. (2022). Biosynthetic pathway and metabolic engineering of succinic acid. Front. Bioeng. Biotechnol. 10:843887. doi: 10.3389/fbioe.2022.843887
Neumann, N., Doello, S., and Forchhammer, K. (2021). Recovery of unicellular cyanobacteria from nitrogen chlorosis: a model for resuscitation of dormant bacteria. Microb Physiol 31, 78–87. doi: 10.1159/000515742
Oliver, N. J., Rabinovitch-Deere, C. A., Carroll, A. L., Nozzi, N. E., Case, A. E., and Atsumi, S. (2016). Cyanobacterial metabolic engineering for biofuel and chemical production. Curr. Opin. Chem. Biol. 35, 43–50. doi: 10.1016/j.cbpa.2016.08.023
Opel, F., Itzenhäuser, M. A., Wehner, I., Lupacchini, S., Lauterbach, L., Lenz, O., et al. (2023). Toward a synthetic hydrogen sensor in cyanobacteria: functional production of an oxygen-tolerant regulatory hydrogenase in Synechocystis sp. PCC 6803. Front. Microbiol. 14:1122078. doi: 10.3389/fmicb.2023.1122078
Osanai, T., Oikawa, A., Azuma, M., Tanaka, K., Saito, K., Hirai, M. Y., et al. (2011). Genetic engineering of group 2 sigma factor SigE widely activates expressions of sugar catabolic genes in Synechocystis species PCC 6803. J. Biol. Chem. 286, 30962–30971. doi: 10.1074/jbc.M111.231183
Osanai, T., Shirai, T., Iijima, H., Nakaya, Y., Okamoto, M., Kondo, A., et al. (2015). Genetic manipulation of a metabolic enzyme and a transcriptional regulator increasing succinate excretion from unicellular cyanobacterium. Front. Microbiol. 6:1064. doi: 10.3389/fmicb.2015.01064
Pinto, F., van Elburg, K. A., Pacheco, C. C., Lopo, M., Noirel, J., Montagud, A., et al. (2012). Construction of a chassis for hydrogen production: physiological and molecular characterization of a Synechocystis sp. PCC 6803 mutant lacking a functional bidirectional hydrogenase. Microbiology (Reading) 158, 448–464. doi: 10.1099/mic.0.052282-0
Rippka, R. (1988). Isolation and purification of cyanobacteria. Methods Enzymol. 167, 3–27. doi: 10.1016/0076-6879(88)67004-2
Satoh, S., Ikeuchi, M., Mimuro, M., and Tanaka, A. (2001). Chlorophyll b expressed in Cyanobacteria functions as a light-harvesting antenna in photosystem I through flexibility of the proteins. J. Biol. Chem. 276, 4293–4297. doi: 10.1074/jbc.M008238200
Shi, Y., Pu, D., Zhou, X., and Zhang, Y. (2022). Recent progress in the study of taste characteristics and the nutrition and health properties of organic acids in foods. Foods 11:3408. doi: 10.3390/foods11213408
Stal, L. J., and Moezelaar, R. (1997). Fermentation in cyanobacteria. FEMS Microbiol. Rev. 21, 179–211. doi: 10.1016/S0168-6445(97)00056-9
Stephens, E., Ross, I. L., Mussgnug, J. H., Wagner, L. D., Borowitzka, M. A., Posten, C., et al. (2010). Future prospects of microalgal biofuel production systems. Trends Plant Sci. 15, 554–564. doi: 10.1016/j.tplants.2010.06.003
Takahashi, H., Uchimiya, H., and Hihara, Y. (2008). Difference in metabolite levels between photoautotrophic and photomixotrophic cultures of Synechocystis sp. PCC 6803 examined by capillary electrophoresis electrospray ionization mass spectrometry. J. Exp. Bot. 59, 3009–3018. doi: 10.1093/jxb/ern157
Takeya, M., Ito, S., Sukigara, H., and Osanai, T. (2018). Purification and characterisation of malate dehydrogenase from Synechocystis sp. PCC 6803: biochemical barrier of the oxidative tricarboxylic acid cycle. Front. Plant Sci. 9:947. doi: 10.3389/fpls.2018.00947
Tamagnini, P., Leitão, E., Oliveira, P., Ferreira, D., Pinto, F., Harris, D. J., et al. (2007). Cyanobacterial hydrogenases: diversity, regulation and applications. FEMS Microbiol. Rev. 31, 692–720. doi: 10.1111/j.1574-6976.2007.00085.x
Tittmann, K. (2009). Reaction mechanisms of thiamin diphosphate enzymes: redox reactions. FEBS J. 276, 2454–2468. doi: 10.1111/j.1742-4658.2009.06966.x
Vijay, D., Akhtar, M. K., and Hess, W. R. (2019). Genetic and metabolic advances in the engineering of cyanobacteria. Curr. Opin. Biotechnol. 59, 150–156. doi: 10.1016/j.copbio.2019.05.012
Werpy, T., and Petersen, G. (2004). Top value added chemicals from biomass 1. Results of screening for potential candidates from sugars and synthesis gas. U.S. Department of Energy, 1–76.
Williams, J. G. K. (1988). Construction of specific mutations in photosystem II photosynthetic reaction center by genetic engineering methods in Synechocystis 6803. Methods Enzymol. 167, 766–778. doi: 10.1016/0076-6879(88)67088-1
Yamane, M., and Osanai, T. (2023). Nondiazotrophic cyanobacteria metabolic engineering for succinate and lactate production. Algal Res. 71:103088. doi: 10.1016/j.algal.2023.103088
Keywords: Cyanobacteria, fermentation, hydrogen, organic acids, succinate, lactate, acetate
Citation: Akiyama M and Osanai T (2024) Regulation of organic acid and hydrogen production by NADH/NAD+ ratio in Synechocystis sp. PCC 6803. Front. Microbiol. 14:1332449. doi: 10.3389/fmicb.2023.1332449
Edited by:
Weiwen Zhang, Tianjin University, ChinaCopyright © 2024 Akiyama and Osanai. This is an open-access article distributed under the terms of the Creative Commons Attribution License (CC BY). The use, distribution or reproduction in other forums is permitted, provided the original author(s) and the copyright owner(s) are credited and that the original publication in this journal is cited, in accordance with accepted academic practice. No use, distribution or reproduction is permitted which does not comply with these terms.
*Correspondence: Takashi Osanai, dG9zYW5haUBtZWlqaS5hYy5qcA==