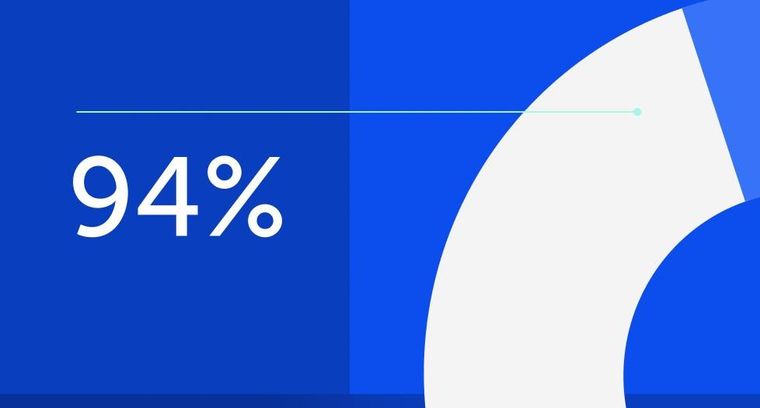
94% of researchers rate our articles as excellent or good
Learn more about the work of our research integrity team to safeguard the quality of each article we publish.
Find out more
ORIGINAL RESEARCH article
Front. Microbiol., 08 January 2024
Sec. Microbial Physiology and Metabolism
Volume 14 - 2023 | https://doi.org/10.3389/fmicb.2023.1321428
Microbiome engineering is an emerging research field that aims to design an artificial microbiome and modulate its function. In particular, subtractive modification of the microbiome allows us to create an artificial microbiome without the microorganism of interest and to evaluate its functions and interactions with other constituent bacteria. However, few techniques that can specifically remove only a single species from a large number of microorganisms and can be applied universally to a variety of microorganisms have been developed. Antisense peptide nucleic acid (PNA) is a potent designable antimicrobial agent that can be delivered into microbial cells by conjugating with a cell-penetrating peptide (CPP). Here, we tested the efficacy of the conjugate of CPP and PNA (CPP-PNA) as microbiome modifiers. The addition of CPP-PNA specifically inhibited the growth of Escherichia coli and Pseudomonas putida in an artificial bacterial consortium comprising E. coli, P. putida, Pseudomonas fluorescens, and Lactiplantibacillus plantarum. Moreover, the growth inhibition of P. putida promoted the growth of P. fluorescens and inhibited the growth of L. plantarum. These results indicate that CPP-PNA can be used not only for precise microbiome engineering but also for analyzing the growth relationships among constituent microorganisms in the microbiome.
A variety of microorganisms have been isolated from the natural environment over the past two centuries. The elucidation of their biological functions and industrial applications has long been a subject of research interest by microbiologists. However, in nature, microorganisms do not exist alone; rather, they are part of a huge ecosystem formed by interactions among microorganisms. These complex microbial systems are known as microbiomes and have a profound impact on the physiology of their host and the state of their habitat. For example, in the human gut, gut microbiome benefits host health by providing protection against pathogens (Chiu et al., 2017), immunomodulation (Zheng et al., 2020), and nutrient metabolism (Silva et al., 2020). Conversely, an imbalance in the gut microbial community is involved in the pathogenesis of many diseases such as nonalcoholic fatty liver disease (Wang et al., 2016), colorectal cancer (Sobhani et al., 2019), and type 2 diabetes (Pedersen et al., 2016). Therefore, understanding and controlling the functions of microbiomes is a new frontier in microbiology.
Microbiome engineering provides a possible solution to improve the imbalance in the microbial population and modulate the function of the microbiome. Microbiome engineering aims to manipulate the composition and function of microbes in the microbiome, and many strategies have been proposed to achieve this approach. The oral administration of probiotics can inhibit the growth of disease-associated bacteria and promote host health (Kerry et al., 2018). In addition to live microorganisms, several additives, including feed enzymes (Kiarie et al., 2013), signaling molecules (Vincent et al., 2022), and organic acids (Dai et al., 2021), have been used to promote or inhibit the growth of beneficial or harmful microorganisms. These additives commonly act on a wide range of microorganisms, making it difficult to precisely manipulate the microbiome community structure. In contrast, bacteriophages, bacterial viruses, have attracted attention as precise antimicrobials. The administration of lytic phages to gnotobiotic mice, which were colonized with defined commensal human gut bacteria, reduced the number of susceptible bacteria by one to two orders of magnitude (Hsu et al., 2019). In addition, the use of the engineered temperate phage expressing programmable nuclease-deactivated Cas9, dCas9, enabled gene modulation of its targeted strain, showing the possibility of strain-specific gene modulation in microbiomes (Hsu et al., 2020). These phage-based technologies assume that phages infecting their hosts are available; however, most microorganisms in microbiomes remain uncultured (Steen et al., 2019), limiting their application to microbiome engineering.
Peptide nucleic acids (PNAs) have application potential as alternative, precise antimicrobials. PNA is a bio-mimic of DNA, and its nucleobases are attached to the N-(2-aminoethyl)-glycine backbone instead of the sugar-phosphate backbone (Good and Nielsen, 1998). This unnatural structure makes PNA resistant to proteinases and nucleases (Demidov et al., 1994). Considering that PNA can bind RNA and form PNA/RNA heteroduplexes, antisense PNA, which can bind to the mRNA of an essential gene, can inhibit the translation of mRNA and act as an antimicrobial. However, the application of PNA is hindered by its poor uptake by bacterial cells. To overcome this limitation, chemical conjugation of PNA to a variety of carrier compounds has been proposed. Równicki et al. (2017) synthesized a conjugate of vitamin B12 and PNA, which was successfully taken up by Escherichia coli and Salmonella Typhimurium cells via the vitamin B12 uptake pathway. Liu et al. (2023) also synthesized a conjugate of glucose polymer and PNA, and the conjugate was delivered into the E. coli and Staphylococcus aureus cells through the ABC sugar transporter pathway. Among these carriers, cell-penetrating peptides (CPP), which are short cationic peptides and facilitate the cellular uptake of biomolecules, has been used for the longest time and applied to many microorganisms. KFFKFFKFFK ((KFF)3K) is a well-known CPP that can permeate the cell membranes of a variety of microorganisms, including Gram-negative and Gram-positive bacteria (Hatamoto et al., 2009; Ghosal and Nielsen, 2012; Bai et al., 2012b; Mondhe et al., 2014). Good et al. (2001) synthesized a CPP and PNA conjugate (CPP-PNA) with a CPP sequence of (KFF)3K, and the PNA sequence was designed to bind to the mRNA encoding the acyl carrier protein (acpP), an essential protein for bacterial growth. The addition of CPP-PNA targeting acpP in E. coli successfully inhibited the growth of E. coli (Good et al., 2001). This CPP-PNA was further shown to inhibit the growth of the pathogenic E. coli strain (Popella et al., 2022). Likewise, a variety of antisense CPP-PNAs against clinically pathogenic bacteria were synthesized, and their antibacterial potency has been validated (Wojciechowska et al., 2020). Importantly, CPP-PNAs can be designed if the genome sequence of the target microorganism is available, and can be synthesized by solid-phase synthesis (Pipkorn et al., 2012). Therefore, CPP-PNA could be used to modify the microbiome regardless of whether the target microorganism is culturable. However, PNA-based antimicrobials have primarily been applied to purely cultured strains, and few studies have applied them to microbiome engineering.
In the present study, we demonstrated microbiome engineering using antisense CPP-PNA in an artificial bacterial consortium (Figure 1) consisting of E. coli, Pseudomonas putida, Pseudomonas fluorescens, and Lactiplantibacillus plantarum (formally known as Lactobacillus plantarum). The growth of E. coli or P. putida was selectively inhibited by adding CPP-PNAs to the essential genes in each species, thereby allowing only three bacterial species to grow. Consequently, the bacterial population of the target microorganisms in the consortium can be modified subtractively. Our results also suggested that this microbiome-modification technique could be applied to analyze the growth linkage among the bacteria in the bacterial community. This analysis was accomplished by evaluating how the growth inhibition of one microorganism alters the growth of others.
Figure 1. Subtractive modification of the microbiome using a conjugate of CPP and antisense PNA. CPP-PNA can nonspecifically permeate the bacterial membrane in the microbiome through the action of CPP, whereas the PNA region can specifically bind to the mRNA of essential genes of the target species, thereby inducing growth arrest of the target species. As a result, other microorganisms grow and the relative number of target species decrease.
The strains used in this study are listed in Table 1. E. coli MG 1655 was obtained from National Institute of Genetics (Shizuoka, Japan). P. fluorescens NBRC 15829 and P. putida NBRC 14164 were obtained from Biological Research Center, National Institute of Technology and Evaluation (NITE; Tokyo, Japan). L. plantarum NCIMB 8826 was obtained from National Collection of Industrial, Food and Marine Bacteria (NCIMB; Scotland, UK). The chloramphenicol-resistant strain of E. coli MG 1655 (E. coli CmR) and neomycin-resistant strain of P. putida NBRC 14164 (P. putida NeoR) were constructed as described in the Materials and Methods section of the Supplementary material. The resulting E. coli CmR and P. putida NeoR in addition to P. fluorescens and L. plantarum were used for growth-inhibition and microbiome-modification experiments using CPP-PNAs.
The sequences of CPP-PNAs used in this study are listed in Table 1. CPP-PNAs were synthesized by Panagene Inc. (Daejeon, South Korea) with a purity of 99.9% and their target sequences are shown in Table 2. CPP-EcPNA was designed to bind the −5 to +5 region of the mRNA of the acpP gene in E. coli, whereas CPP-PpPNA was designed to bind the +4 to +13 region of the mRNA of the ftsZ gene in P. putida. CPP with a (KFF)3K sequence was attached to the N-terminal of both PNAs via an ethylene glycol linker. The CPP-PNAs were obtained as dry pellets and dissolved in presterilized deionized water to give 50 μM. The samples were incubated at 90°C for 10 min immediately before use. To ensure the sequence specificity of CPP-EcPNA and CPP-PpPNA for the target region of the mRNA, off-target analysis was performed by comparing the target sequences of CPP-PNAs with the genome sequences of E. coli (NCBI RefSeq: NC_000913.3), P. fluorescens (NCBI RefSeq: NZ_BDAA00000000.1), P. putida (GenBank: AP013070.1), and L. plantarum (GenBank: AL935263.2).
Escherichia coli CmR, P. fluorescens, and P. putida NeoR were cultivated in Luria–Bertani (LB) medium at 30°C, whereas L. plantarum was cultured in 5 mL of MRS medium (Difco Laboratories, Detroit, MI, USA) at 30°C. Then, 50 μL aliquots of each culture were mixed with an equivalent volume of 30% (w/v) glycerol solution and stored at −80°C until use.
For the precultivation of E. coli, P. fluorescens, and P. putida, M9 minimal medium (glucose, 4 g/L; Na2HPO4, 6 g/L; K2HPO4, 3 g/L; NaCl, 0.5 g/L; NH4Cl, 1 g/L; MgSO4, 1 mM; CaCl2, 0.3 mM; thiamine∙HCl, 1 mg/L) was used, whereas MRS medium diluted 10 times was used for precultivation of L. plantarum. One portion of the glycerol stock was inoculated into a test tube containing 5 mL of the medium and cultivated at 30°C for 14 h. The cells were collected by centrifugation at 8,000 × g at 4°C for 3 min and washed twice with 2 mM PIPES-NaOH (pH = 6.8). Then, the cells were resuspended in the same buffer to a cell concentration of 1.0 × 107 CFU/mL.
The main cultivation was performed in a 1.5-mL PROKEEP low-binding microtube (Fukae-Kasei Co., Ltd., Hyogo, Japan) to evaluate the growth inhibitory effect of the CPP-PNAs on each of the four bacteria. Each of the four strains was inoculated into microtubes containing 200 μL of the medium as in the pre-culture at 1.0 × 105 CFU/mL, and each CPP-PNA was added at 0–10 μM. The microtubes were sealed with Parafilm M (Sigma-Aldrich Co. LLC, St. Louis, MO, USA) and cultivated at 30°C and 180 rpm. After 24 h of cultivation, the culture was serially diluted and 50 μL of dilutants were spotted on the agar media. In determining viable cell numbers of E. coli, P. fluorescens, and P. putida, LB media supplemented with 30 μg/mL of chloramphenicol, 50 μg/mL of streptomycin, and 50 μg/mL of neomycin were used, respectively. The MRS medium prepared at pH 6.0 was used for the cultivation of L. plantarum. E. coli and L. plantarum were cultivated at 37°C, whereas P. fluorescens and P. putida were cultivated at 30°C.
A cell suspension (1.0 × 107 CFU/mL) of E. coli CmR, P. fluorescens, P. putida NeoR, and L. plantarum was prepared as described above. The aforementioned strains were co-inoculated at 1.0 × 105 CFU/mL onto each microtube containing 200 μL of M9 medium to form artificial bacterial consortium. Then, CPP-EcPNA or CPP-PpPNA were added to the microtubes to inhibit the growth of their target bacteria, E. coli or P. putida, respectively. The bacterial consortium was cultivated at 180 rpm and 30°C, and the cultures were regularly harvested. Finally, the consortium was serially diluted, and 50 μL of dilutant was spotted on four different agar media as described above to selectively cultivate and count the colony number of each of the four strains.
The natural microbiome is composed of a mixture of Gram-negative and Gram-positive bacteria from a variety of genera and species. In this study, E. coli, P. putida, P. fluorescens, and L. plantarum were selected to construct the artificial bacterial consortium, and E. coli and P. putida were used as the target bacteria to induce species-selective growth inhibition. This model is suitable for elucidating whether PNA can be used for subtractive modification of microbiome, including bacteria across (1) species, (2) genera, and (3) Gram-negative and Gram-positive bacteria. In co-culture system, it is difficult to individually monitor the growth of the constituent microorganisms. From this point of view, this bacterial combination is useful as it allows to individually evaluate viable cell numbers using the corresponding selective media for each microorganism (see the Materials and methods section). P. fluorescens is naturally resistant to streptomycin, and L. plantarum grew in the MRS medium, with pH adjusted to 6.0 (Supplementary Table 1), whereas other bacteria hardly grew under these conditions. E. coli and P. putida are the representative hosts for genetic modification. Thus, the antibiotic-resistant derivatives, E. coli CmR and P. putida NeoR, were easily constructed (Supplementary materials and methods). Although P. fluorescens was resistant to chloramphenicol, it could not grow at 37°C. Therefore, E. coli could be selectively grown on LB medium supplemented with chloramphenicol at 37°C.
The antibacterial activity of PNA is largely affected by various factors, such as the target gene, localization of the target sequence, and PNA length (Goltermann et al., 2019). Therefore, we selected PNAs, which are already known to have antibacterial activity, to facilitate the proof-of-concept of microbiome engineering using CPP-PNAs. mRNAs of several essential genes were targeted by CPP-PNAs in E. coli such as acpP (Good et al., 2001), rpoD (Bai et al., 2012a), and murA (Mondhe et al., 2014). Among them, CPP-PNA, which targets mRNA of acpP (CPP-EcPNA) showed the lowest minimal inhibitory concentration (MIC) of 0.6 μM in E. coli (Wojciechowska et al., 2020). For the growth inhibition of pseudomonads, only Pseudomonas aeruginosa has been targeted by antimicrobial PNA because this strain causes infectious diseases. In P. aeruginosa PAO1, mRNAs of acpP and ftsZ genes were targeted by antisense CPP-PNAs, and both CPP-PNAs had the same MIC of 2.0 μM (Ghosal and Nielsen, 2012). Remarkably, the target sequence of ftsZ showed mismatches among species, with four different sequence variations among the six species (Supplementary Table 2). In contrast, the target sequence of acpP showed lower diversity, and four species had the same sequence as that of P. aeruginosa. Therefore, CPP-PNA, which targets ftsZ (CPP-PpPNA), was used in this study because of its ability to induce cell death in a species-selective manner, even in the presence of microorganisms belonging to the same genus.
As a CPP, a (KFF)3K synthetic peptide was selected and conjugated with PNA because of its ability to transport PNA to Gram-negative bacteria, such as E. coli, P. aeruginosa, and Klebsiella pneumoniae, as well as Gram-positive bacteria, such as Bacillus subtilis, S. aureus, and Corynebacterium efficiens (Hatamoto et al., 2009; Ghosal and Nielsen, 2012; Bai et al., 2012b; Mondhe et al., 2014).
In performing microbiome engineering using CPP-PNAs, off-target effects should be minimized. Thus, the potential off-target sequences of CPP-PNAs were analyzed. The comparison of the target sequences of CPP-EcPNA and CPP-PpPNA with the genomes of E. coli, P. putida, P. fluorescens, and L. plantarum revealed the presence of several identical sequences. For CPP-EcPNA, 11, 4, 3, and 4 off-targets were found in the genome of each of the four bacteria, whereas 1, 19, 13, and 4 sequences were found for CPP-PpPNA, respectively (Table 2). Dryselius et al. (2003) reported that the translation initiation region, including the ribosome-binding site and start codon, is sensitive to antisense PNA inhibition. Analysis of 2,458 bacterial genomes revealed that the commonly utilized ribosome-binding sequences have a length of 3–6 nt and were 5–10 nt away from the start codon (Omotajo et al., 2015). Moreover, the +4 to +6 region from the translation start site showed sensitivity to antisense PNA inhibition (Ghosal and Nielsen, 2012). Therefore, the PNA overlapping the −16 to +6 region will show a high antimicrobial effect. None of the off-target sequences was located in this region of any gene (Table 2). Thus, CPP-EcPNA and CPP-PpPNA are expected to show high specificity for their respective target sequences.
The antibacterial activities of CPP-EcPNA and CPP-PpPNA against the four bacterial species were evaluated. When E. coli was cultivated in the absence of CPP-PNAs, the number of viable cells increased from 1.00 × 105 CFU/mL to 8.60 × 106 CFU/mL after 24 h of cultivation (Figure 2A). On the other hand, no growth of E. coli was observed in the presence of 1 μM CPP-EcPNA and the number of viable cells after 24 h of cultivation was 9.27 × 104 CFU/mL (p = 0.044 in t-test). For the other bacterial species, EcPNA can be considered as a scrambled PNA with random PNA sequences. No significant decrease in the viable cell number was observed in the nontarget species, P. fluorescens and L. plantarum, even in the presence of 10 μM CPP-EcPNA (p = 0.36 and 0.079, respectively). However, CPP-EcPNA showed toxicity to P. putida. The addition of ≥2 μM CPP-EcPNA had a decisive effect on the growth of P. putida. In the presence of 2 μM CPP-EcPNA, the number of viable cells dropped from 1.87 × 107 CFU/mL to 2.97 × 105 CFU/mL (98.4% decrease; p = 0.018), whereas the addition of 1 μM CPP-EcPNA maintained the growth of P. putida at 52.4% (p = 0.0013).
Figure 2. Evaluation of the antibacterial activity of CPP-PNAs against four bacterial species. CPP-EcPNA (A) or CPP-PpPNA (B) was added at various concentrations to the culture of E. coli, P. putida, P. fluorescens, and L. plantarum. After cultivation at 30°C for 24 h, the viable cell numbers were counted. Dashed line indicates the initial cell concentration prior to cultivation and NA indicates that growth inhibitory effect was not assessed. Data bars represent mean ± standard deviation values of three independent experiments. The growth of each strain with the addition of CPP-PNAs was compared with that in nontreated condition. Asterisks and double-asterisks indicate p values are less than 0.05 and 0.01 in the t-test, respectively.
CPP-PpPNA showed a slightly higher specificity than CPP-EcPNA (Figure 2B). Although the addition of 4 μM CPP-PpPNA did not completely inhibited the growth of P. putida (4.79 × 105 CFU/mL; p = 0.018), the addition of 6 μM CPP-PpPNA was enough to inhibit the growth of P. putida. The number of viable cells decreased from 1.00 × 105 CFU/mL to 3.20 × 102 CFU/mL after 24 h of cultivation, which is significantly lower than the growth of the strain without adding CPP-PpPNA (1.87 × 107 CFU/mL; p = 0.019). At this concentration, no growth inhibition was observed in the nontarget species, P. fluorescens and L. plantarum. Because the +4 to +13 region of the ftsZ gene, which is the target sequence of PpPNA, differs by only one base pair between P. putida and P. fluorescens (Supplementary Table 2), PpPNA works as an one-mismatched PNA against P. fluorescens. This result indicates that one-mismatched PNA is not effective to target ftsZ of P. fluorescens at this concentration. On the other hand, E. coli showed a decrease in its growth, but 69.4% of growth was retained (p = 0.021).
It is well known that free PNA poorly penetrate bacterial cell membrane. The addition of free PNA (with the same sequence as in this experiment) at a high concentration of 32 μM did not inhibit the growth of E. coli MG1655 (Goltermann et al., 2022). In addition, the addition of 32 μM free PNA targeting RNA polymerase α-subunit (rpoA) of Listeria monocytogens also showed no growth inhibitory effect (Abushahba et al., 2016), though its CPP conjugates inhibited the growth of L. monocytogens at lower concentrations (1–2 μM). On the other hand, some CPPs are known to show cytotoxicity (Lee et al., 2021). To evaluate whether the growth inhibition of E. coli and P. putida by CPP-PNAs was not due to CPP-induced cytotoxicity, the effect of free CPP on the growth of E. coli and P. putida was investigated (Supplementary Figure 1). In the presence of 6 μM free CPP, the number of viable cells of E. coli and P. putida increased to 8.73 × 106 CFU/mL and 2.90 × 107 CFU/mL after 24 h of cultivation, respectively. These values are comparable to those of nontreated cells (1.65 × 107 CFU/mL and 2.15 × 107 CFU/mL, respectively) and no significant decrease was observed (p = 0.13 and 0.29, respectively). Therefore, we concluded that the growth inhibition of E. coli and P. putida was caused by the CPP-PNA conjugates, and 1 μM CPP-EcPNA and 6 μM CPP-PpPNA were used for the following microbiome modifications.
The artificial bacterial consortium was constructed by co-cultivating four bacterial species in M9 minimal medium at an initial cell concentrations of 105 CFU/mL of each. When the bacterial consortium was cultivated without adding CPP-PNAs, numbers of viable cell of E. coli, P. putida, P. fluorescens, and L. plantarum reached 1.57 × 108, 1.59 × 108, 1.71 × 107, and 1.83 × 105 CFU/mL after 34 h of cultivation, respectively (Figure 3A). The addition of 1 μM of CPP-EcPNA completely inhibited the growth of E. coli, and the viable cell number at 34 h was 2.38 × 104 CFU/mL (Figure 3B; p = 0.035). On the other hand, no significant change was observed in the growth of P. fluorescens and L. plantarum as the p values were 0.12 and 0.059 in t-test, respectively (Figure 4). Although slight growth inhibition was observed in the monoculture of P. putida (Figure 2A), no significant growth inhibition was observed with the addition of CPP-EcPNA in the coculture experiment (p = 0.069; Figure 4). Since CPP-EcPNA is taken up by the four bacterial species, the effective concentration of CPP-EcPNA for each of the four bacteria may be lower than that in monoculture experiments. As well as monoculture experiment, addition of higher concentration of CPP-EcPNA (2 μM) inhibited the growth of P. putida in microbiome modification (Supplementary Figure 2). About 95% decrease was observed in numbers of viable cell after 34 h of cultivation (8.13 × 106 CFU/mL; p = 0.011) compared to those without CPP-EcPNA (1.59 × 108 CFU/mL). Consequently, the growth of E. coli was successfully inhibited by adding 1 μM of CPP-EcPNA without perturbing the growth of nontarget bacteria, and the artificial bacterial consortium was subtractively modified.
Figure 3. Subtractive modification of the artificial bacterial consortium consisting of four bacterial species. E. coli (green circles), P. putida (blue triangles), P. fluorescens (orange squares), and L. plantarum (pink diamonds) were co-inoculated to M9 medium at 1.0 × 105 CFU/mL of each. The growth of four bacteria without CPP-PNAs (A), with CPP-EcPNA (B), and with CPP-PpPNA (C) was compared. Data points represent mean ± standard deviation of three independent experiments.
Figure 4. Comparison of the final CFU after modification of the artificial bacterial consortium. E. coli (green bars), P. putida (blue bars), P. fluorescens (orange bars), and L. plantarum (pink bars) were co-inoculated to M9 medium and were co-cultivated at 30°C for 34 h without CPP-PNAs (solid bars), with CPP-EcPNA (vertical bars), and with CPP-PpPNA (horizontal bars). Data bars represent the mean ± standard deviation values in three independent experiments. Asterisks indicate p values are less than 0.05 in the t-test. For the results showing statistical significance, the difference of viable cell counts to the nontreated condition was shown in the figure.
Similarly, the growth inhibition of P. putida was induced by adding 6 μM of CPP-PpPNA, and the viable cell numbers of P. putida once decreased to undetectable level (< 20 CFU/mL) after 10 h of cultivation. Then, it increased to 8.93 × 104 CFU/mL after 34 h of cultivation (Figure 3C). To confirm whether this growth recovery was due to the development of PNA-resistant mutant, four colonies were randomly selected from the colonies formed after 34 h of cultivation, and their resistance to CPP-PpPNA was evaluated. As well as the parental strain, the viable cell counts of all four strains were under the detection limit (20 CFU/mL) after 10 h of cultivation in the presence of 6 μM of CPP-PpPNA (Supplementary Figure 3). Therefore, growth restoration of P. putida does not seem to be due to the acquisition of PNA resistance. Although the mechanism is unknown at this time, it may be that P. putida gradually degrades CPP-PpPNA or CPP-PpPNA was incorporated by other bacteria grown normally, allowing it to grow in the later stages of cultivation. Interestingly, an increase and decrease in the viable cell numbers of P. fluorescens and L. plantarum were also observed (6.65 × 107 and 9.87 × 103 CFU/mL, respectively), with statistical significance (p = 0.023 and 0.025, respectively, Figure 4). In determining whether the changes in the growth of P. fluorescens and L. plantarum were due to the addition of CPP-PpPNA or not, E. coli, P. fluorescens, and L. plantarum were co-cultivated without adding CPP-PpPNA (Supplementary Figure 4A). These three bacteria showed almost the same growth profiles as those of the four bacterial co-culture systems with the addition of CPP-PpPNA (p = 0.42, 0.30 and 0.20, respectively; Supplementary Figure 4B). This result implies that the changes in the growth of nontarget bacteria were not induced by CPP-PpPNA, indicating that P. putida inhibited the growth of P. fluorescens and stimulated the growth of L. plantarum in the four bacterial co-culture systems. This hypothesis was verified by comparing the growth profiles of P. fluorescens and L. plantarum between the monoculture and co-culture with P. putida. The result revealed that P. putida inhibited the growth of P. fluorescens and stimulated the growth of L. plantarum (Figure 5). These results suggested that CPP-PNA is beneficial not only for microbiome engineering but also for revealing growth linkages among microorganisms that make up the microbiome.
Figure 5. Comparison of bacterial growth with and without P. putida. P. fluorescens (A) and L. plantarum (B) were cultivated without (open symbols) and with (closed symbols) P. putida in M9 medium. Data points represent the mean ± standard deviation values in three independent experiments. Asterisk and double asterisk indicate that the p values are less than 0.05 and 0.01 in the t-test, respectively.
To date, CPP-PNAs have been used as an alternative to antibiotics and have focused on inhibiting the growth of pathogenic bacteria (Kulyté et al., 2005; Ghosal and Nielsen, 2012; Bai et al., 2012a) including their antibiotic-resistant mutants such as methicillin-resistant S. aureus (Bai et al., 2012b). Most of these studies have examined the target genes and targeting position in the mRNA using PNA, which exhibits high antisense effects, and few reports have examined their effects on the growth of microorganisms other than the target microorganism (Mondhe et al., 2014). In contrast, this study aimed to apply CPP-PNA as a tool for precise microbiome engineering in a subtractive manner. Thus, the selectivity of CPP-PNAs for the target bacteria should be considered. Our results demonstrated that CPP-EcPNA and CPP-PpPNA selectively inhibited the growth of their target bacteria, E. coli and P. putida, in a four-species mixed culture (Figure 3). To the best of our knowledge such species-specific growth inhibition using antisense PNA has only been reported by Mondhe et al. (2014). In their study, the growth of K. pneumoniae and S. Typhimurium was selectively inhibited by the addition of CPP-PNA targeting each of them in a three-species mixed culture, including Bacillus subtilis. Consequently, CPP-PNA was shown to be used for subtractive modification of the microbiome, including the bacteria across genera, and Gram-negative and Gram-positive bacteria. Our result that CPP-PpPNA selectively inhibited the growth of P. putida in the presence of P. fluorescens (Figure 3) indicates that CPP-PNA can exert selectivity, even at the species level, by selecting the appropriate PNA sequence.
In addition, PNA-based microbiome engineering could be used to analyze the growth relationships among microorganisms in the microbiome. Microbiome engineering creates the microbiome with the desired functions by artificially modifying the composition and function of microorganisms in the microbiome. However, this is only one aspect of microbiome engineering. Removing a particular microorganism from the microbiome is nothing more than creating a microbiome in which that microorganism is absent. Comparing the behavior and function of the microbiome before and after modification would determine the role of the removed microorganisms and their interactions with other microorganisms in the original microbiome. Our results showed that addition of CPP-PNA to the microbial consortium did not completely eliminate the target species, but reduced the number of the cells from the initial level of 1.00 × 105 CFU/mL (Figure 3). On the other hand, the number of cells of other species increases, allowing the population of the target species to be lowered over time. As a result, the contribution of the target species to the microbial consortium can be minimized. The growth rate is an easy change to detect, and the subtractive modification of microbiome in four bacterial systems successfully reveals that E. coli did not interfere with the growth of other microorganisms, but P. putida inhibited the growth of P. fluorescens and enhanced the growth of L. plantarum (Figure 4). As observed in our study, L. plantarum can hardly grow in M9 medium, which contains no amino acids and vitamins except for thiamine, without co-cultivating other bacteria (Mizuno et al., 2017), because L. plantarum needs a variety of nutrients such as vitamins and amino acids (Wegkamp et al., 2010). Accordingly, P. putida must support the growth of L. plantarum by providing nutrients. Understanding such a commensalism, as well as other interactions such as mutualism, cooperation, and competition, is necessary to understand the dynamics of the microbiome.
Compared to our top-down approach, several studies have employed a bottom-up approach to analyze the interactions of the constituent microorganisms in the microbiome. Venturelli et al. (2018) selected 12 prevalent human-associated intestinal species and analyzed the growth linkages for 66 combinations of two species. From these datasets, a predictive computational model for the dynamics of the microbial community was developed. Considering that interactions between two species are often modulated by a third species, Bairey et al. (2016) constructed a model that accounted for high-order interactions. The difficulty in applying such techniques to predict the dynamics of “real” microbiome may depend on the large species that comprise the microbiome. It is estimated that at least 160 bacterial species present in the microbiome of each individual (Qin et al., 2010), and experimental assessment of the growth linkages of each microorganism will require a great deal of time and effort. Moreover, the bottom-up approach assumes the use of isolated microorganisms and is not applicable to approximately 70% of the species that have not yet been cultured (Almeida et al., 2021). In contrast, PNA-based subtractive microbiome modification (top-down approach) has the potential to target all bacteria in the microbiota if genome sequences are available. Furthermore, this approach will allow us to examine the impact of one microbial species on the growth of all other species at once by inhibiting the growth of one microbial species.
A key obstacle in using CPP-PNAs to modify the actual microbiome is increasing microbial specificity. In monoculture experiments using four different microorganisms, CPP-EcPNA and CPP-PpPNA showed nonspecific growth inhibition against P. putida and E. coli, respectively, and inhibition became more pronounced as the concentration of CPP-PNAs increased (Figure 2). Because of this nonspecific growth inhibition, we were unable to add enough CPP-PNAs to completely abolish the target bacteria (Figure 3). In this study, PNAs with 10 nucleobases were used, and there are 1,048,576 variations of the 10 nucleobases (= 410). Given that the bacterial genome size is several Mbp, this PNA variation will not provide sufficient specificity. Therefore, increasing the length of the PNA will increase specificity; however, Goltermann et al. (2019) reported that increasing the length of the PNA decreases the membrane permeation efficiency of the CPP, thereby weakening the antisense effect. One of the advantages of CPPs is their sequence diversity. The CPPs composed of natural amino acids alone can produce 20n (n = CPP length) of sequences. Lee et al. (2021) have constructed CPP library including nearly 100 fluorescently labeled CPPs and evaluated their cellular uptake and cytotoxicity. As a result, they succeeded to obtain CPPs suitable for delivery of bioactive cargo into E. coli. Finding a CPP with a higher membrane permeation efficiency will allow us to introduce a longer PNA to microorganisms and to modify microbiomes with higher selectivity.
In conclusion, this study demonstrated that CPP-PNAs can selectively inhibit the growth of target microorganisms at the species level, and the model microbiome consisting of the four bacterial species was precisely modified in a subtractive way. This precise microbiome-modification technique will be used to create a model microbiome to prove its function or as a tool for creating synthetic ecosystems with desired functionality. We also demonstrated that PNA-based microbiome engineering could be used to analyze growth relationships among microorganisms in microbiome. Microbial growth linkages provide an opportunity to unravel interactions among microorganisms. By combining various approaches such as metabolomic analysis, it is expected to reveal what interactions are at work between microorganisms and what ecological forces are involved in the assembly and stability of the microbiome.
The original contributions presented in the study are included in the article/Supplementary material, further inquiries can be directed to the corresponding author.
TH: Investigation, Methodology, Writing – review & editing. YS: Investigation, Methodology, Writing – review & editing. HI: Writing – review & editing. KH: Funding acquisition, Supervision, Writing – review & editing. KO: Conceptualization, Formal analysis, Funding acquisition, Investigation, Methodology, Project administration, Writing – original draft.
The author(s) declare financial support was received for the research, authorship, and/or publication of this article. This research was partly funded by the Japan Society for the Promotion of Sciences (JSPS), KAKENHI Grant (22H04886 and 22K04840 for KO and 20K21275 for KH). This work was also supported in part by JST FOREST Program (Grant Number JPMJFR2202, Japan for KO).
The authors declare that the research was conducted in the absence of any commercial or financial relationships that could be construed as a potential conflict of interest.
All claims expressed in this article are solely those of the authors and do not necessarily represent those of their affiliated organizations, or those of the publisher, the editors and the reviewers. Any product that may be evaluated in this article, or claim that may be made by its manufacturer, is not guaranteed or endorsed by the publisher.
The Supplementary material for this article can be found online at: https://www.frontiersin.org/articles/10.3389/fmicb.2023.1321428/full#supplementary-material
Abushahba, M. F. N., Mohammad, H., Thangamani, S., Hussein, A. A. A., and Seleem, M. N. (2016). Impact of different cell penetrating peptides on the efficacy of antisense therapeutics for targeting intracellular pathogens. Sci. Rep. 6:20832. doi: 10.1038/srep20832
Almeida, A., Nayfach, S., Boland, M., Strozzi, F., Beracochea, M., Shi, Z. J., et al. (2021). A unified catalog of 204,938 reference genomes from the human gut microbiome. Nat. Biotechnol. 39, 105–114. doi: 10.1038/s41587-020-0603-3
Bai, H., Sang, G., You, Y., Xue, X., Zhou, Y., Hou, Z., et al. (2012b). Targeting RNA polymerase primary σ70 as a therapeutic strategy against methicillin-resistant Staphylococcus aureus by antisense peptide nucleic acid. PLoS One 7:e29886. doi: 10.1371/journal.pone.0029886
Bai, H., You, Y., Yan, H., Meng, J., Xue, X., Hou, Z., et al. (2012a). Antisense inhibition of gene expression and growth in gram-negative bacteria by cell-penetrating peptide conjugates of peptide nucleic acids targeted to rpoD gene. Biomaterials 33, 659–667. doi: 10.1016/j.biomaterials.2011.09.075
Bairey, E., Kelsic, E., and Kishony, R. (2016). High-order species interactions shape ecosystem diversity. Nat. Commun. 7:12285. doi: 10.1038/ncomms12285
Chiu, L., Bazin, T., Truchetet, M.-E., Schaeverbeke, T., Delhaes, L., and Pradeu, T. (2017). Protective microbiota: from localized to long-reaching co-immunity. Front. Immunol. 8:1678. doi: 10.3389/fimmu.2017.01678
Dai, D., Qiu, K., Zhang, H.-J., Wu, S.-G., Han, Y.-M., Wu, Y.-Y., et al. (2021). Organic acids as alternatives for antibiotic growth promoters alter the intestinal structure and microbiota and improve the growth performance in broilers. Front. Microbiol. 11:618144. doi: 10.3389/fmicb.2020.618144
Demidov, V. V., Potaman, V. N., Frank-Kamenetskil, M. D., Egholm, M., Buchard, O., Sönnichsen, S. H., et al. (1994). Stability of peptide nucleic acids in human serum and cellular extracts. Biochem. Pharmacol. 48, 1310–1313. doi: 10.1016/0006-2952(94)90171-6
Dryselius, R., Aswasti, S. K., Rajarao, G. K., Nielsen, P. E., and Good, L. (2003). The translation start codon region is sensitive to antisense PNA inhibition in Escherichia coli. Oligonucleotides 13, 427–433. doi: 10.1089/154545703322860753
Ghosal, A., and Nielsen, P. E. (2012). Potent antibacterial antisense peptide-peptide nucleic acid conjugates against Pseudomonas aeruginosa. Nucleic Acid Ther. 22, 323–334. doi: 10.1089/nat.2012.0370
Goltermann, L., Yavari, N., Zhang, M., Ghosal, A., and Nielsen, P. E. (2019). PNA length restriction of antibacterial activity of peptide-PNA conjugates in Escherichia coli through effects of the inner membrane. Front. Microbiol. 10:1032. doi: 10.3389/fmicb.2019.01032
Goltermann, L., Zhang, M., Ebbensgaard, A. E., Fiodorovaite, M., Yavari, N., Løbner-Olesen, A., et al. (2022). Effects of LPS composition in Escherichia coli on antibacterial activity and bacterial uptake of antisense peptide-PNA conjugates. Front. Microbiol. 13:877377. doi: 10.3389/fmicb.2022.877377
Good, L., Awasthi, S. K., Dryselius, R., Larsson, O., and Nielsen, P. E. (2001). Bactericidal antisense effects of peptide-PNA conjugates. Nat. Biotechnol. 19, 360–364. doi: 10.1038/86753
Good, L., and Nielsen, P. E. (1998). Antisense inhibition of gene expression in bacteria by PNA targeted to mRNA. Nat. Biotechnol. 16, 355–358. doi: 10.1038/nbt0498-355
Hatamoto, M., Nakai, K., Ohashi, A., and Imachi, H. (2009). Sequence-specific bacterial growth inhibition by peptide nucleic acid targeted to the mRNA binding site of 16S rRNA. Appl. Microbiol. Biotechnol. 84, 1161–1168. doi: 10.1007/s00253-009-2099-0
Hsu, B. B., Gibson, T. E., Yeliseyev, V., Liu, Q., Lyon, L., Bry, L., et al. (2019). Dynamic modulation of the gut microbiota and metabolome by bcteriophages in a mouse model. Cell Host Microb 25, 803–814.e5. doi: 10.1016/j.chom.2019.05.001
Hsu, B. B., Plant, I. N., Lyon, L., Anastassacos, F. M., Way, J. C., and Silver, P. A. (2020). In situ reprogramming of gut bacteria by oral delivery. Nat. Commun. 11:5030. doi: 10.1038/s41467-020-18614-2
Kerry, R. G., Patra, J. K., Gouda, S., Park, Y., Shin, H.-S., and Das, G. (2018). Benefaction of probiotics for human health: a review. J. Food Drug Anal. 26, 927–939. doi: 10.1016/j.jfda.2018.01.002
Kiarie, E., Romero, L. F., and Nyachoti, C. M. (2013). The role of added feed enzymes in promoting gut health in swine and poultry. Neutr. Res. Rev. 26, 71–88. doi: 10.1017/S0954422413000048
Kulyté, A., Nekhotiaeva, N., Awasthi, S. K., and Good, L. (2005). Inhibition of Mycobacterium smegmatis gene expression and growth using antisense peptide nucleic acids. J. Mol. Microbiol. Biotechnol. 9, 101–109. doi: 10.1159/000088840
Lee, H.-M., Ren, J., Tran, K. M., Jeon, B.-M., Park, W.-U., Kim, H., et al. (2021). Identification of efficient prokaryotic cell-penetrating peptides with applications in bacterial biotechnology. Commun. Biol. 4:205. doi: 10.1038/s42003-021-01726-w
Liu, M., Chu, B., Sun, R., Ding, J., Ye, H., Yang, Y., et al. (2023). Antisense oligonucleotides selectively enter human-derived antibiotic-resistant bacteria through bacterial-specific ATP-binding cassette sugar transporter. Adv. Mater. 35:2300477. doi: 10.1002/adma.202300477
Mizuno, K., Mizuno, M., Yamauchi, M., Takemura, A. J., Romero, V. M., and Morikawa, K. (2017). Adjacent-possible ecological niche: growth of lactobacillus species co-cultured with Escherichia coli in a synthetic minimal medium. Sci. Rep. 7:12880. doi: 10.1038/s41598-017-12894-3
Mondhe, M., Chessher, A., Goh, S., Good, L., and Stach, J. E. M. (2014). Species-selective killing of bacteria by antimicrobial peptide-PNAs. PLoS One 9:e89082. doi: 10.1371/journal.pone.0089082
Omotajo, D., Tate, T., Cho, H., and Choudhary, M. (2015). Distribution and diversity of ribosome binding sites in prokaryotic genomes. BMC Genomics 16:604. doi: 10.1186/s12864-015-1808-6
Pedersen, H. K., Gudmundsdottir, V., Nielsen, H. B., Hyotylainen, T., Nielsen, T., Jensen, B. A. H., et al. (2016). Human gut microbes impact host serum metabolome and insulin sensitivity. Nature 535, 376–381. doi: 10.1038/nature18646
Pipkorn, R., Wiessler, M., Waldeck, W., Hennrich, U., Nokihara, K., Beining, M., et al. (2012). Improved synthesis strategy for peptide nucleic acids (PNA) appropriate for cell-specific fluorescence imaging. Int. J. Med. Sci. 9, 1–10. doi: 10.7150/ijms.9.1
Popella, L., Jung, J., Do, P. T., Hayward, R. J., Barquist, L., and Vogel, J. (2022). Comprehensive analysis of PNA-based antisense antibiotics targeting various essential genes in uropathogenic Escherichia coli. Nucleic Acids Res. 50, 6435–6452. doi: 10.1093/nar/gkac362
Qin, J., Li, R., Raes, J., Arumugam, M., Burgdorf, K. S., Manichanh, C., et al. (2010). A human gut microbial gene catalogue established by metagenomic sequencing. Nature 464, 59–65. doi: 10.1038/nature08821
Równicki, M., Wojciechowska, M., Wierzba, A. J., Czarnecki, J., Bartosik, D., Gryko, D., et al. (2017). Vitamin B12 as a carrier of peptide nucleic acid (PNA) into bacterial cells. Sci. Rep. 7:7644. doi: 10.1038/s41598-017-08032-8
Silva, Y. P., Bernardi, A., and Frozza, R. L. (2020). The role of short-chain fatty acids from gut microbiota in gut-brain communication. Front. Endocrinol. 11:25. doi: 10.3389/fendo.2020.00025
Sobhani, I., Bergsten, E., Couffin, S., Amiot, A., Nebbad, B., Barau, C., et al. (2019). Colorectal cancer-associated microbiota contributes to oncogenic epigenetic signatures. Proc. Natl. Acad. Sci. U. S. A. 116, 24285–24295. doi: 10.1073/pnas.1912129116
Steen, A. D., Crits-Christoph, A., Carini, P., DeAngelis, K. M., Fierer, N., Lloyd, K. G., et al. (2019). High proportions of bacteria and archaea across most biomes remain uncultured. ISME J. 13, 3126–3130. doi: 10.1038/s41396-019-0484-y
Venturelli, O. S., Carr, A. V., Fisher, G., Hsu, R. H., Lau, R., Bowen, B. P., et al. (2018). Deciphering microbial interactions in synthetic human gut microbiome communities. Mol. Syst. Biol. 14:e8157. doi: 10.15252/msb.20178157
Vincent, S. A., Ebertz, A., Spanu, P. D., and Devlin, P. F. (2022). Salicylic acid-mediated disturbance increases bacterial diversity in the phyllosphere but is overcome by a dominant core community. Front. Microbiol. 13:809940. doi: 10.3389/fmicb.2022.809940
Wang, B., Jiang, X., Cao, M., Ge, J., Bao, Q., Tang, L., et al. (2016). Altered fecal microbiota correlates with liver biochemistry in nonobese patients with non-alcoholic fatty liver disease. Sci. Rep. 6:32002. doi: 10.1038/srep32002
Wegkamp, A., Teusink, B., de Vos, W. M., and Smid, E. J. (2010). Development of a minimal growth medium for Lactobacillus plantarum. Lett. Appl. Microbiol. 50, 57–64. doi: 10.1111/j.1472-765X.2009.02752.x
Wojciechowska, M., Równicki, M., Mieczkowski, A., Miszkiewicz, J., and Trylska, J. (2020). Antibacterial peptide nucleic acids—facts and perspectives. Molecules 25:559. doi: 10.3390/molecules25030559
Keywords: peptide nucleic acid, cell penetrating peptide, microbiome engineering, antisense effect, growth linkage analysis
Citation: Hizume T, Sato Y, Iwaki H, Honda K and Okano K (2024) Subtractive modification of bacterial consortium using antisense peptide nucleic acids. Front. Microbiol. 14:1321428. doi: 10.3389/fmicb.2023.1321428
Received: 17 October 2023; Accepted: 26 December 2023;
Published: 08 January 2024.
Edited by:
Nityananda Chowdhury, Medical University of South Carolina, United StatesReviewed by:
Nadia Andrea Andreani, Max Planck Institute for Evolutionary Biology, GermanyCopyright © 2024 Hizume, Sato, Iwaki, Honda and Okano. This is an open-access article distributed under the terms of the Creative Commons Attribution License (CC BY). The use, distribution or reproduction in other forums is permitted, provided the original author(s) and the copyright owner(s) are credited and that the original publication in this journal is cited, in accordance with accepted academic practice. No use, distribution or reproduction is permitted which does not comply with these terms.
*Correspondence: Kenji Okano, b2thbm8ua0BrYW5zYWktdS5hYy5qcA==
Disclaimer: All claims expressed in this article are solely those of the authors and do not necessarily represent those of their affiliated organizations, or those of the publisher, the editors and the reviewers. Any product that may be evaluated in this article or claim that may be made by its manufacturer is not guaranteed or endorsed by the publisher.
Research integrity at Frontiers
Learn more about the work of our research integrity team to safeguard the quality of each article we publish.