- 1Laboratory of Biologically Active Compounds, Intercollegiate Faculty of Biotechnology of UG and MUG, University of Gdańsk, Gdańsk, Poland
- 2Bioimaging Laboratory, Faculty of Biology, University of Gdańsk, Gdańsk, Poland
- 3Laboratory of Plant Microbiology, Intercollegiate Faculty of Biotechnology of UG and MUG, University of Gdańsk, Gdańsk, Poland
- 4Laboratory of Mass Spectrometry-Core Facility Laboratories, Intercollegiate Faculty of Biotechnology of UG and MUG, University of Gdańsk, Gdańsk, Poland
- 5Laboratory of Molecular Biology, Intercollegiate Faculty of Biotechnology of UG and MUG, University of Gdańsk, Gdańsk, Poland
Tailocins are nanomolecular machines with bactericidal activity. They are produced by bacteria to contribute to fitness in mixed communities, and hence, they play a critical role in their ecology in a variety of habitats. Here, we characterized the new tailocin produced by Dickeya dadantii strain 3937, a well-characterized member of plant pathogenic Soft Rot Pectobacteriaceae (SRP). Tailocins induced in D. dadantii were ca. 166 nm long tubes surrounded by contractive sheaths with baseplates having tail fibers at one end. A 22-kb genomic cluster involved in their synthesis and having high homology to the cluster coding for the tail of the Peduovirus P2 was identified. The D. dadantii tailocins, termed dickeyocins P2D1 (phage P2-like dickeyocin 1), were resistant to inactivation by pH (3.5–12), temperature (4–50°C), and elevated osmolarity (NaCl concentration: 0.01–1 M). P2D1 could kill a variety of different Dickeya spp. but not any strain of Pectobacterium spp. tested and were not toxic to Caenorhabditis elegans.
Introduction
Under natural conditions, bacterial species inhabit shared environments, developing spatial and temporal interspecies associations and communities having complex networks of interactions (Little et al., 2008; Gorter et al., 2020). In such communities, a particular member needs to continuously compete for limited resources (i.e., scarce nutrients and limited space) with most other members of the community to gain a competitive edge (Bauer et al., 2018; Wagner, 2022). Given such challenging conditions, bacteria have evolved diverse strategies to successfully coexist with both closely and distantly related microbes (Granato et al., 2019). Such strategies, although employing a broad range of mechanisms, can be distinguished as either (1) indirect, exploitative competition that occurs through the consumption of resources and (2) direct, interference competition, where individual cells directly kill one another, limiting their lifespan (Ghoul and Mitri, 2016). Whereas exploitative competition depends primarily on the utilization of limited resources by a strain, thereby restricting it from the competitor, interference competition relies on producing various antimicrobial agents that aim to kill other cells (Hibbing et al., 2010). These antimicrobials include, but are not limited to, broad-spectrum antibiotics, toxins, contact-dependent inhibition, effectors transported via type VI secretion system (T6SS effectors), low molecular weight bacteriocins, and tailocins (Stubbendieck and Straight, 2016; Granato et al., 2019). A given bacterial cell may often use several such systems to gain fitness advantages in the environment (Hibbing et al., 2010). Among the systems bacteria exploit to fight competitive microbes, tailocins are now receiving increasing attention (Scholl, 2017; Patz et al., 2019).
Tailocins are syringe-like nanomolecular entities that are evolutionary and morphologically related to bacteriophage tails, type VI secretion systems, and extracellular contractile injection systems (Scholl, 2017). These particles, also known as high molecular weight bacteriocins or phage tail-like particles, are chromosomally encoded and ribosomally synthesized toxins that usually express a narrow killing range, interacting only with closely-related bacterial species that typically would occupy the same niche (Patz et al., 2019). These agents adsorp to the surface of susceptible cells, thereby puncturing the cell envelope, leading to depolymerization of the cell membrane and, ultimately, the death of the attacked cell (Ge et al., 2015).
Tailocins are classified into two distinct families: rigid and contractile (R-type) and noncontractile but flexible particles (F-type; Ghequire and De Mot, 2015). The R-type tailocins have features of tails of Peduovirus P2 or T-even bacteriophages infecting Escherichia coli. In contrast, the F-type tailocins resemble the flexible tails of bacteriophage lambda (λ). Although tailocins exhibit remarkable morphological similarity to bacteriophage tails of the viruses mentioned above, it is now believed that they have evolved independently from bacteriophages and should not be considered exclusively as domesticated prophages or phage remnants that bacteria harness for their advantage (Scholl, 2017).
The production of tailocins has been demonstrated both in Gram-negative and Gram-positive bacterial species. Producing strains include both human, animal, and plant pathogens and saprophytic bacteria residing in various environments (Morales-Soto et al., 2012; Liu et al., 2013; Ghequire and De Mot, 2014; Gebhart et al., 2015). Until recently, tailocins have been best characterized in Pseudomonas species (Michel-Briand and Baysse, 2002; Fischer et al., 2012). There are, however, reports of tailocins isolated from Clostridioides spp., Serratia spp., Xenorhabdus spp., Burkholderia spp., Kosakonia spp., Budvicia spp., Pragia spp., Pectobacterium spp. as well as from other bacteria (Smarda et al., 2005; Becker et al., 2022).
The omnipresence of tailocins in phylogenetically unrelated bacterial genera suggests that these particles are important for fitness in various habitats (Scholl, 2017). However, the ecological role of tailocins in the natural environment of the producing strains has received little attention, especially for plant-pathogenic bacteria residing in agricultural locations. This issue is important given the diversity of conditions such bacteria encounter in such natural settings. No comprehensive studies have addressed tailocins produced by Soft Rot Pectobacteriaceae (SRP) bacteria (Van Gijsegem et al., 2021), which, due to their complex lifestyle, have a variety of spatial and temporal interactions in varied environments (Perombelon, 2002; Charkowski, 2007, 2018).
Plant pathogenic SRP (consisting of Pectobacterium spp., Dickeya spp., and Musicola spp., formerly characterized as pectinolytic Erwinia spp.) are a useful model for studying the environmental role of tailocins. SRP bacteria are widespread in various ecological niches, including rain and surface water, natural and agricultural bulk and rhizosphere soils, sewage, the exterior and interior of host and non-host plants as well as the surface and interior of insects (Van Gijsegem et al., 2021). Because of the diverse environments in which SRP bacteria may be found, these pathogens may encounter various other bacteria with whom they must effectively compete.
This study aimed to assess the presence and activity of tailocins induced and isolated from pectinolytic Dickeya dadantii strain 3937 (Kotoujansky et al., 1982). This strain (formerly Erwinia chrysanthemi and Pectobacterium chrysanthemi; Samson et al., 2005) is a well-known necrotrophic plant pathogen that causes soft rot disease in a variety of crop, ornamental, and other nonfood plants worldwide, causing losses in agriculture (Kotoujansky, 1987). Strain 3937 has been widely used as a potent model system for research on the molecular biology and pathogenicity of bacteria belonging to Soft Rot Pectobacteriaceae for several decades (Reverchon and Nasser, 2013; Reverchon et al., 2016). While this strain continues to be the most studied strain of all Dickeya species its production of tailocins has not been previously described. Here, we characterize for the first time the tailocin produced by D. dadantii strain 3937.
Results
Dickeya dadantii 3937 produces tailocins
Cells of D. dadantii 3937 treated with mitomycin C produced syringe-like macromolecular structures resembling bacteriophage tails. Following convention, the tailocins produced by D. dadantii were named dickeyocins. Imaging using TEM and AFM revealed that these structures consist of a central rod-shaped core (tube) wrapped in a contractable sheath (Figure 1). Both the tube and the sheath were built of multiple protein subunits, with a clearly visible helical arrangement of the subunits in the sheath. The average length of the dickeyocins produced by strain 3937 was 166 ± 7 nm. When the sheath entirely covered the tube (in the extended, “loaded” form), the individual dickeyocin has a diameter of 23 ± 2 nm. Fibers were visible at the distal end of the sheath. When the sheath contracts, it revealed an internal tube of a length of 92 ± 7 nm with an attached spike at the distal end (Figure 1).
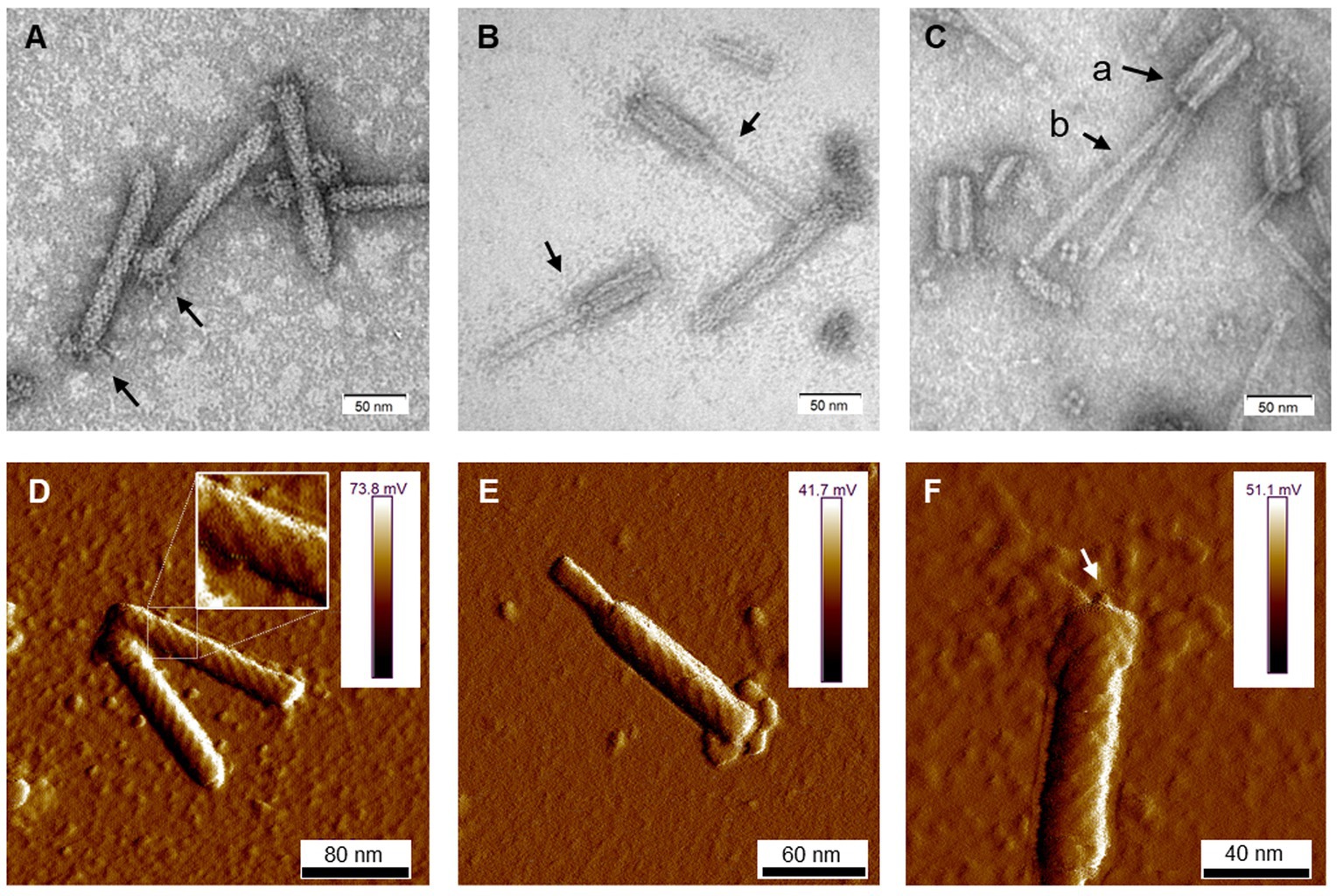
Figure 1. The morphology of tailocins from Dickeya dadantii 3937. Images were obtained using TEM (A–C) and AFM (D–F). The individual panels show: (A) particles in an extended (active) form. Arrows indicate the positioning of fibers; (B) contracted particles indicated by arrows; (C) tailocins disintegrated by heat treatment (50°C); Arrows indicate: (a) empty sheath with a hollow inner channel and (b) tube (core) separated from the sheath; (D) two extended particles. A fragment of the photo was magnified to display the helical arrangement of protein subunits in the sheath; (E) a contracted molecule in an AFM image; and (F) an extended particle with an arrow pointing at the presumed tube-attached spike.
The yield of tailocins purified from mitomycin-induced cells of D. dadantii 3937 equaled approx. 1011 particles mL−1 of culture. The particles could also be isolated from non-treated cells, indicating a low basal production level. However, induction with mitomycin C increased the yield approx. 10–100-fold. We employed three different methods to estimate the concentration of phage-tail-like particles in the tested preparations. The results were consistent for the independently obtained batches of purified dickeyocins (Supplementary Table S2). The average concentration from three independently obtained samples was 106 relative units (AU) mL−1, 1011 killing particles mL−1, and 1011 particles mL−1, according to the spot test, the Poisson distribution killing method, and the NanoSight measurements, respectively. Furthermore, comparing the results from the activity-based Poisson method and the direct particle count indicated that most phage-like particles purified from the cultures of D. dadantii strain 3937 were undamaged and in the extended (“loaded”) form. This was in line with the microscopic observations done with TEM and AFM.
Dickeyocins from Dickeya dadantii strain 3937 are phylogenetically related to the tail of Peduovirus P2
Proteins in the dickeyocins were separated by SDS-PAGE and sequenced. Eight cleary distinguishable bands were excised from the gel, and the digested peptides were analyzed by MS. The peptides could be readily mapped to six D. dadantii proteins with annotations implying their phage relationship: phage baseplate assembly protein (encoded in locus Dda3937_00029/DDA3937_RS12055), baseplate assembly protein J (Dda3937_00030/DDA3937_RS12060), tail fiber protein (Dda3937_04606/DDA3937_RS12070), putative side tail fiber protein (Dda3937_03808/DDA3937_RS12100), major sheath protein (Dda3937_03810/DDA3937_RS12110), and major tail tube protein (Dda3937_03811/DDA3937_RS12115; Figure 2). Genes encoding these six proteins were mapped to a single ca. 22-kb region in the genome of D. dadantii strain 3937 (GenBank accession number: NC_014500.1: genome location: 2,734,508–2,757,061; Figure 2C). This genomic region contained 28 genes, from which 16 genes encoded various bacteriophage structural proteins.
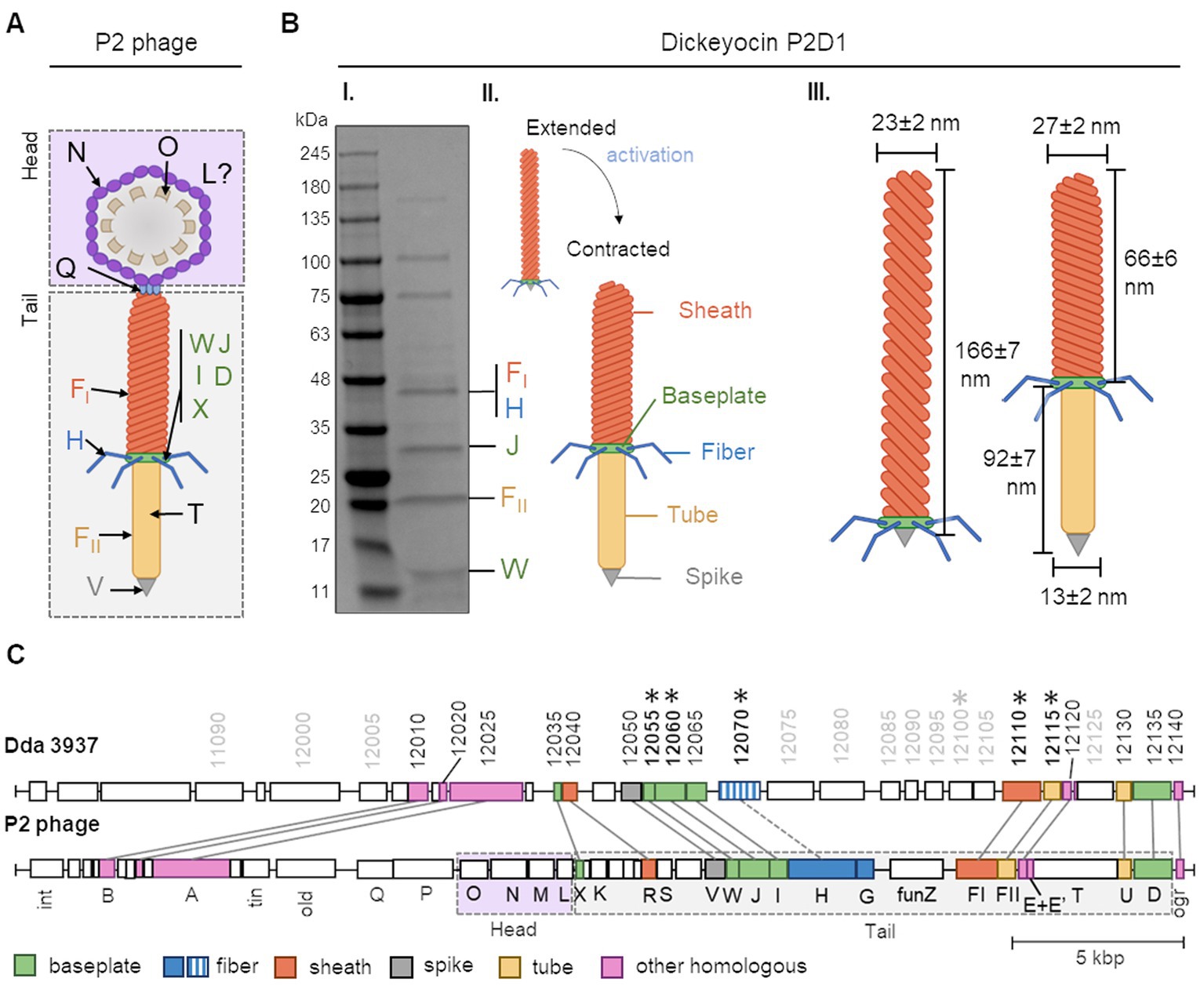
Figure 2. The building blocks of tailocins of Dickeya dadantii strain 3937 and their encoding genes in relation to those of the Peudovirus P2. Panel (A) shows a schematic representation of the structure of the P2 phage, together with the nomenclature of the building proteins. The graph was prepared based on Christie and Calendar (2016) (PMID: 27144088, https://pubmed.ncbi.nlm.nih.gov/27144088/). WJIDX—baseplate proteins, FI—sheath, H—fiber, FII—tube, V—spike, T—tape measure protein, Q—portal protein, N—major capsid protein, O—capsid scaffold, and L—head completion protein. Panel (B) shows: (I) an SDS-PAGE separation of proteins from mitomycin-induced cultures of D. dadantii 3937. Bands containing proteins homologous to those of the P2 phage are provided with the respective protein designations; (II) schematic representation of extended and contracted forms of dickeyocin P2D1; and (III) dimensions of extended and contracted forms of dickeyocin P2D1. Panel (C) shows the alignment between the complete genome sequence of the P2 phage (NC_001895; 33,593 bp) and a region of the same length in the genome of D. dadantii 3937 (NC_014500.1; range: 2,723,487–2,757,061). The numbering of genes in D. dadantii corresponds to the numbering of loci in the genome (locus tag prefix DDA3937_RS). Homologous proteins are marked with the same color.
The nucleotide sequence of the 22-kb putative dickeyocin-encoding fragment in D. dadantii strain 3937 showed the highest similarity to a prophage region in D. dadantii strain XJ12 [100% query coverage (qc), 99.35% identity]. Highly similar regions were also found in the genomes of several other strains of D. dadantii, as well as D. solani, D. dianthicola, and D. fangzhongdai (Supplementary Data S1). Strains of D. zeae showed lower similarity, with query coverage between 54 and 76% and identity of 83–84%. A much lower score was observed for the next best hit—Musicola paradisiaca (formerly Dickeya paradisiaca). Other non-Dickeya microorganisms with regions showing some degree of homology included Serratia sp. ATCC 39006 (Supplementary Data S1; with 10% query coverage and 81.45% identity).
Importantly, at the nucleotide level, the dickeyocin region of D. dadantii strain 3937 showed no significant similarity to the known carotovorocin Er cluster of P. carotovorum Er (Genbank accession: AB045036; Yamada et al., 2006). Moreover, the amino acid sequence of the sheath protein of tailocin from strain 3937 showed only a 34% identity to that of carotovorocin Er, with a query coverage of 83%.
The ca. 22-kb cluster encoding dickeyocin from D. dadantii strain 3937 was also surveyed against the collection of viral sequences (NCBI taxid: 10239), yielding only low similarity scores (7% query coverage, 78% identity for the best hit).
Furthermore, in an attempt to find undomesticated phages related to dickeyocin we searched the viral protein database, using three structural proteins of dickeyocin as queries: sheath protein (WP_013318223), tail tube protein (WP_013318224), and baseplate assembly protein J (WP_013318212). Based on this search, proteins in dickeyocin exhibited homology to proteins of Salmonella phages SW9 and PSP3, Erwinia phage Etg, Enterobacteria phage fiAA91-ss, Peduovirus P2, several Escherichia and Yersinia phages, as well as to multiple poorly-characterized phages of bacteria in class Caudoviricetes, derived from the human metagenome (Supplementary Data S1). The best-characterized phage having a high homology to dickeyocin was Peduovirus P2—a phage that infects Escherichia coli and other hosts, including Salmonella and Klebsiella (Bertani, 1951; Christie and Calendar, 2016). For the three investigated dickeyocin proteins, their amino acid identity toward their P2 homologs ranged from 70 to 79%, with 100% query coverage (Supplementary Data S2). Therefore, we used the Peduovirus P2 bacteriophage as a reference to assign functions to proteins in dickeyocin, as well as to investigate the genetic rearrangements in the dickeyocin cluster in relation to the fully-functional phage P2 (Figure 2C). The dickeyocin cluster in the genome of D. dadantii strain 3937 lacked sequences associated with the phage head (proteins O, M, N, L), as well as the tape measure protein. There was also a difference in the genetic content of the intergenic region of the tail, as well as low homology of proteins building the tail fibers (31% query coverage, 54% identity). Following the present naming convention, we named the newly characterized tailocins from D. dadantii 3937 dickeyocin P2D1 (P2-like Dickeyocin 1).
Dickeyocin P2D1 expresses bactericidal activity exclusively against members of soft rot Pectobacteriaceae
Fifty-two bacterial strains were surveyed for their sensitivity to tailocins induced from D. dadantii strain 3937. These included 41 strains of different species and subspecies of Dickeya and Pectobacterium, as well as six other bacterial strains belonging to the Enterobacteriaceae family, a single strain of Serratia marcescens (family Yersiniaceae), three Pseudomonas spp., and a single strain of Staphylococcus aureus representing Gram-positive bacteria (Supplementary Table S1). Bactericidal activity was observed against eight strains [D. dadantii subsp. dieffenbachie strain NCPPB 2976, D. dianthicola strains NCPPB 3534 and IPO 980, D. undicola CFBP 8650, D. zeae strains NCPPB 3532 and 3531, D. oryzae strain CSL RW192, and Musicola paradisiaca strain NCPPB 2511 (old name: Dickeya paradisiaca strain NCPPB 2511)], but not against any of the Pectobacterium spp. tested (Supplementary Table S1; Figure 3). Likewise, dickeyocin P2D1 was inactive against any of the non-SRP strains included in the screening assay: six Enterobacteriaceae strains (Citrobacter freundii ATCC 8090, Escherichia coli ATCC 8739, Escherichia coli ATCC 25922, Escherichia coli OP50, Klebsiella quasipneumoniae ATCC 700603, and Klebsiella aerogenes ATCC 51697), three Pseudomonas spp.: (Pseudomonas aeruginosa PA14, Pseudomonas aeruginosa PAO1, and Pseudomonas donghuensis P482), Serratia marcescens ATCC 14756 and S. aureus ATCC 25923 indicating their limited range of bactericidal activity (Figure 3).
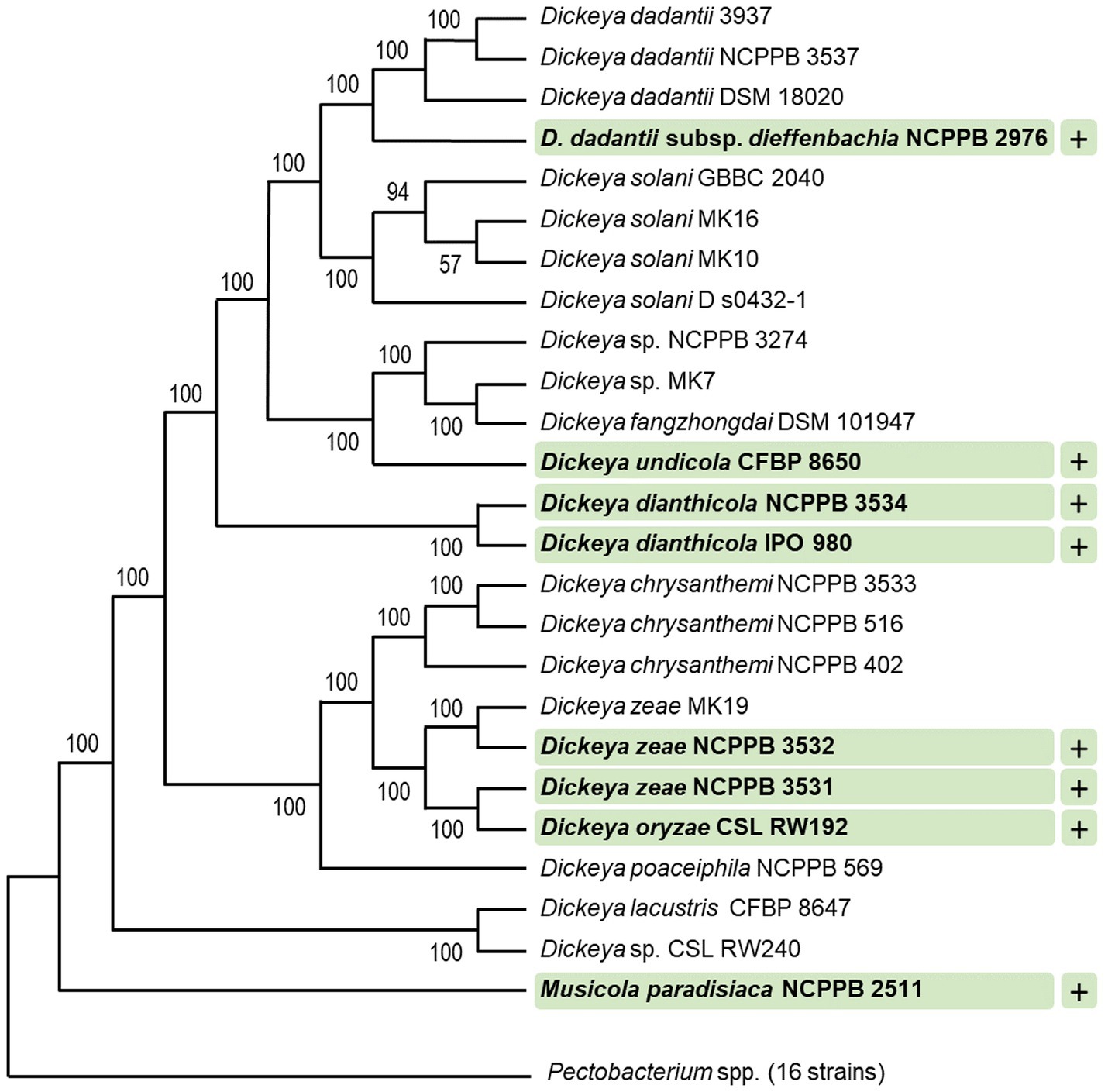
Figure 3. Target range of tailocins from Dickeya dadantii 3937. Strains susceptible to P2D1 dickeyocin were marked with a plus (+). A phylogenetic tree for 41 SRP strains was obtained using EDGAR 3.2 Fasttree 2.1 algorithm. The tree was built out of a core of 1,616 genes per genome (nucleotide sequences), 66,256 genes in total. The core had 1,560,951 bp per genome, 63,998,991 in total. The lengths of the branches do not reflect the phylogenetic distance. All analyzed Pectobacterium spp. were collapsed. This included 16 strains: Pectobacterium cacticida CFBP 3628, Pectobacterium fontis CFBP 8629, Pectobacterium betavasculorum CFBP 2122, Pectobacterium peruviense CFBP 5834, Pectobacterium atrosepticum SCRI1043, Pectobacterium atrosepticum NCPPB 549, Pectobacterium parmentieri CFBP 8475, Pectobacterium parmentieri SCC3193, Pectobacterium polonicum DPMP 315, Pectobacterium punjabense CFBP 8604, Pectobacterium actinidiae LMG 26003, Pectobacterium brasiliense LMG21371, Pectobacterium polaris NCPPB 4611, Pectobacterium versatile CFBP 6051, Pectobacterium carotovorum CFBP 2046, and Pectobacterium aroidearum NCPPB 929. The numbers at the tree branches represent Shimodaira-Hasegawa (SH) branch support values (Shimodaira, 2002).
P2D1 killing efficiency is bacterial species-dependent
We determined the kinetics of killing of eight susceptible strains treated with P2D1. The share of intact cells at two time points: 20 and 120 min post treatment is shown in Supplementary Figure S1. In all the cases, the killing of the susceptible cells was fast; in the first 20 min, the most killing effect was observed for D. dianthicola strain IPO 980 (ca. 60% reduction of cell numbers). In contrast, the slowest killing caused by dickeyocin P2D1 was observed for D. dianthicola strain NCPP 3534 (ca. 22% reduction of cell numbers). For the other six strains tested, on average, a 45–60% reduction in cell numbers was observed after this same time (20 min.). About 50–60% killing of all strains by dickeyocin P2D1 was seen after 120 min-incubation (Supplementary Figure S1).
P2D1 dickeyocin directly punctures the cell envelope of susceptible bacterial strains
We assessed the interaction of dickeyocin P2D1 on cells of the susceptible M. paradisiaca strain at the single-cell level using transmission electron microscopy (TEM). Images clearly showed that dickeyocin P2D1 adsorbed to the bacterial cell envelope (Figures 4A,B), followed by puncturing the outer cell membrane (resulting from the conformational change of dickeyocin P2D1 from an extended “loaded” to a contracted form; Figures 4C,D), creating a pore connecting the cytoplasm of the cell with the external environment and leading eventually to the death of the attacked cell.
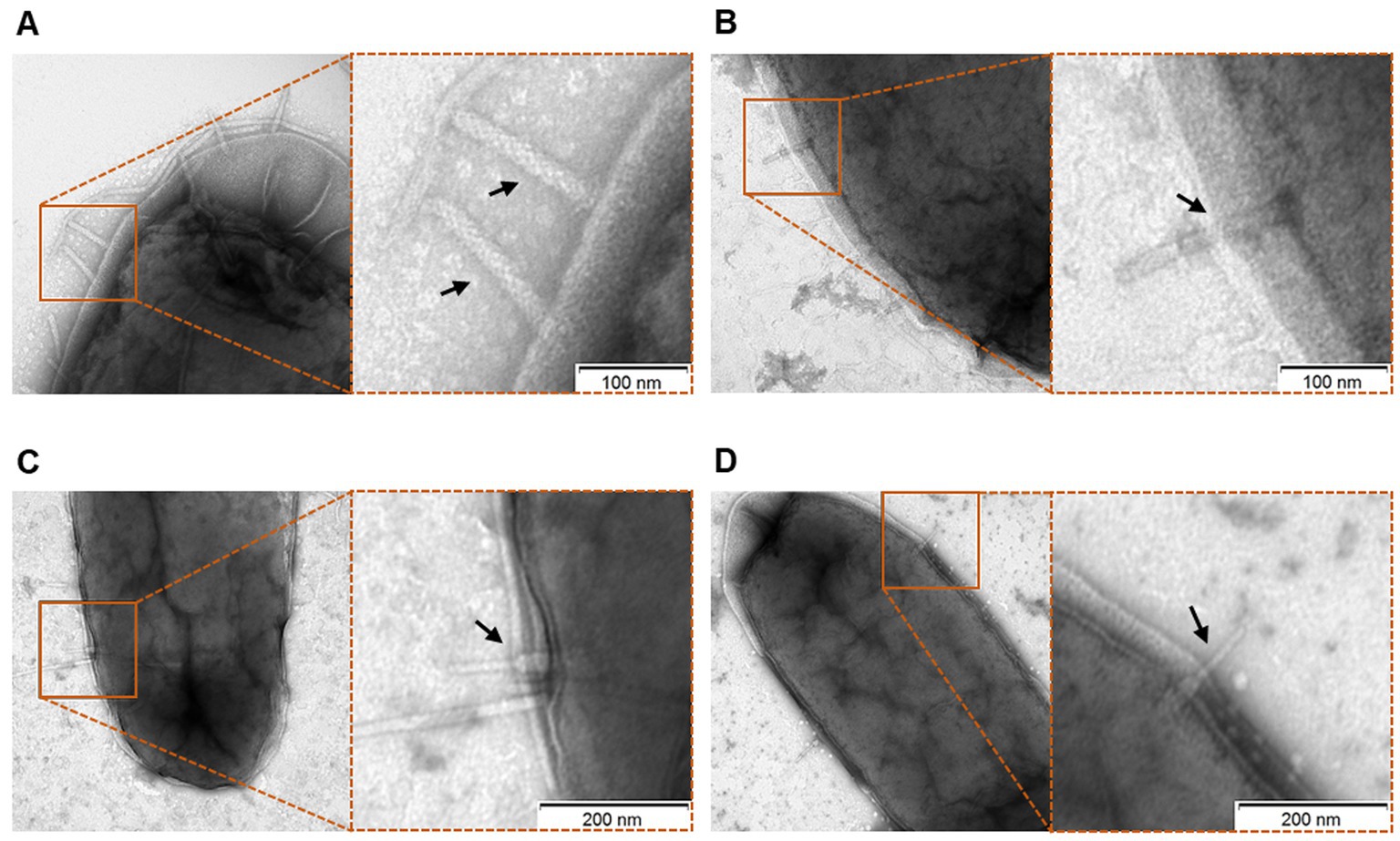
Figure 4. Interaction of P2D1 dickeyocins with the cells of a susceptible strain Musicola paradisiaca NCPPB strain 2511, evaluated using transmission electron microscopy (TEM). Bacterial cells of M. paradisiaca NCPPB 2511 from overnight culture in TSB were harvested and washed with PBS. Such prepared cells were incubated with PEG-purified dickeyocins (approximate concentration in bacterial suspension 106 particles mL−1) for 20 min, followed by obtaining images with TEM. For all panels, the TEM images on the right are the enlarged sections of the original micrographs on the left. Arrows indicate P2D1 attached to the surface of bacterial cells, either in extended (A,B) or contracted (C,D) form.
Dickeyocin P2D1 is able to bind to nonviable (dead) bacterial cells
A cell adsorption assay was employed to investigate whether the dickeyocin P2D1 can adsorb to nonviable (dead) and viable bacterial cells of the susceptible or resistant bacterial species. Dickeyocin P2D1 was incubated either with viable or chloramphenicol-killed cells of susceptible M. paradisiaca or with viable or antibiotic-killed cells of resistant D. dadantii for 40 min and the remaining dickeyocin P2D1 in the medium was measured. Incubation of dickeyocin P2D1 both with the dead and viable cells of M. paradisiaca resulted in the total loss of the tailocin activity, indicating that it bound equally efficiently to viable as well as nonviable cells of the susceptible bacterium. No adsorption of dickeyocin P2D1 to either dead or alive cells of the resistant D. dadantii cells was observed (Supplementary Figure S2).
Production of dickeyocin P2D1 is not associated with T6SS
To determine whether dickeyocin P2D1 production in D. dadantii depends on the type VI secretion system (T6SS), dickeyocin P2D1 production was induced in D. dadantii mutant A5587 (Golanowska, 2015) that carries an insertional mutation in the tssK gene (type VI secretion system baseplate subunit TssK)—an essential subunit of the type VI secretion apparatus (Nguyen et al., 2017). The tailocins obtained from the D. dadantii T6SS mutant using standard mitomycin induction methods were morphologically indistinguishable and similarly abundant compared to dickeyocin P2D1 induced in the wild-type D. dadantii strain 3937 (Supplementary Figure S3). These results suggest that at least tssK gene, essential for the proper function of T6SS, does not affect dickeyocin P2D1 production, morphology, or release from the cell.
Activity of P2D1 dickeyocins is modulated by environmental conditions and enzyme treatments
Dickeyocin P2D1 was stable at temperatures between 4 and 42°C, showing no significant loss in activity following 24 h incubation at these temperatures. Temperatures above 50°C, however, led to a 32-fold loss in activity while incubation at 65 and 80°C (Figure 5A) led to a complete loss of activity. Likewise, a single freeze–thaw cycle negatively affected the stability of dickeyocin P2D1. Freezing at −20°C resulted in a 128-fold reduction in activity, while no bactericidal effect remained after freezing at −80°C (Figure 5A). We tested the stability of P2D1 under seven different pH conditions (2, 3.5, 5, 7, 9, 10.5, and 12). The particles remained active after 24 h incubation in pHs ranging from 3.5 to 12. However, their bactericidal properties were lost at pH 2 (Figure 5B). To determine whether dickeyocin P2D1 that was inactivated at low pH could be restored to an active (extended, “loaded”) form, we diluted the pH 2-treated, inactive dickeyocin P2D1 in PBS buffered to pH 12 to achieve a neutral pH environment. Dickeyocin P2D1 did not regain bactericidal activity at pH 7. Osmotic conditions generated by NaCl added to water at concentrations between 0.01 and 5 M had no adverse effect on the activity of dickeyocin P2D1 within 24 h when compared to controls (dickeyocin P2D1 in PBS containing 0.137 M NaCl and 0.0027 mM KCl). In contrast, incubation of dickeyocin P2D1 in deionized water led to a 4-fold loss in activity (Figure 5C). Dickeyocin P2D1 activity was significantly (p < 0.05) reduced by treatment with proteinase K and chloroform for 1 h (4-fold and 2-fold loss of activity, respectively). Complete loss of activity was seen after incubation in 1% SDS. In contrast, neither lysozyme nor DNase influenced dickeyocin P2D1 activity (Figure 5D).
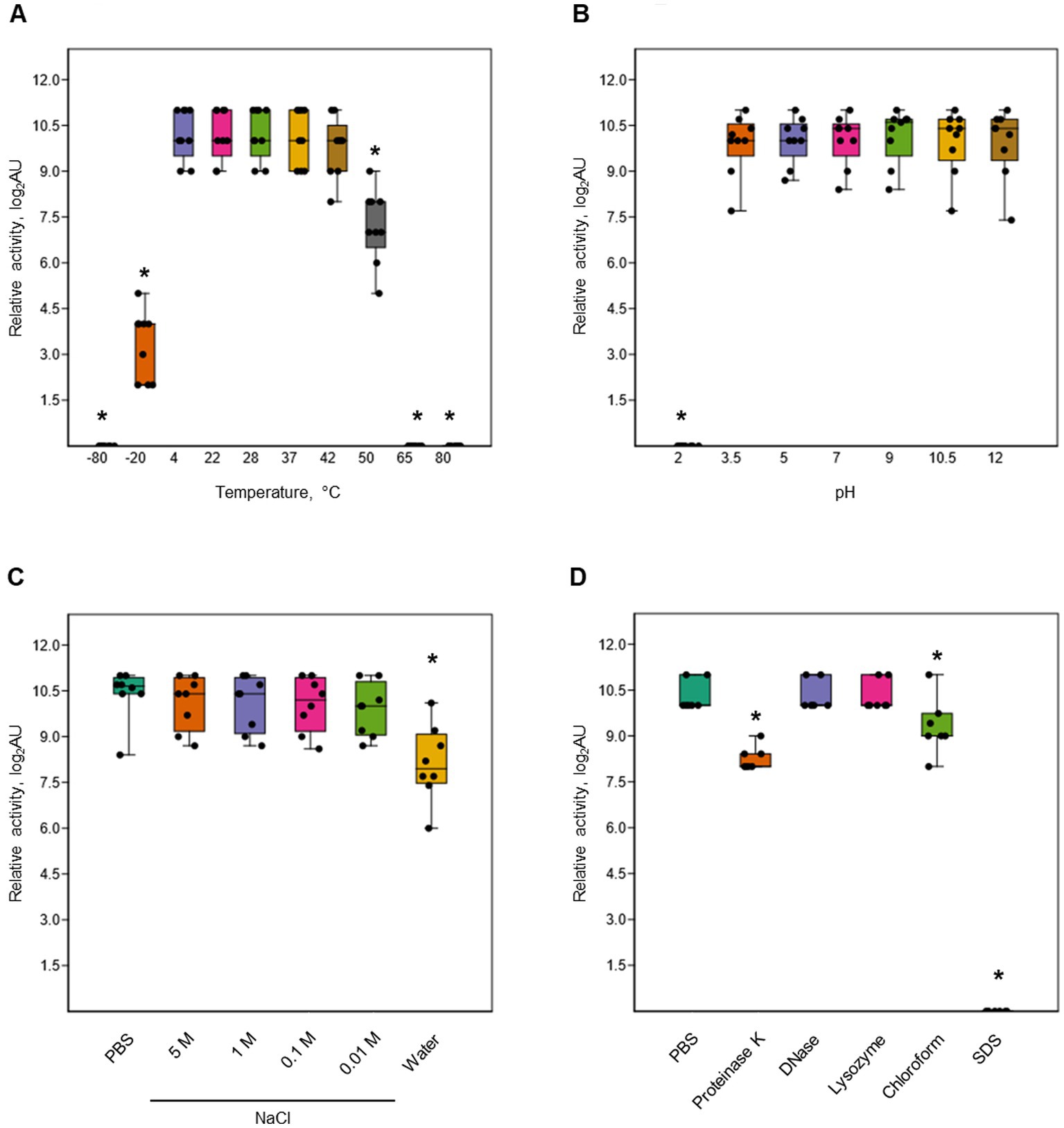
Figure 5. Stability of P2D1 dickeyocins from Dickeya dadantii strain 3937 upon different treatments. The graphs show the influence of temperature (A), pH (B), salinity (C), as well as enzymatic digestion (proteinase K, DNase, and lysozyme), chloroform treatment, and SDS detergent (D) on bactericidal activity of P2D1 against Musicola paradisiaca NCPPB 2511. For temperature, pH/osmolarity, and enzyme/detergent/organic solvent treatments, dickeyocins stored in PBS pH 7, were used as a control. The results are shown as a box plot; whiskers reflect the maximum and minimum, box sides reflect the first and third quartile, and the bars reflect medians. Points indicate particular measurements (n = 9). The asterisks (*) represent statistically significant differences (p < 0.05) between the treatment and the control using the Mann–Whitney test.
Dickeyocin P2D1 is nontoxic for Caenorhabditis elegans
No inhibition of the survival of C. elegans was seen after its exposure to high concentrations of purified dickeyocin P2D1 (Supplementary Figure S4). The average survival rate of C. elegans cultivated in the presence of P2D1 dickeyocins was ca. 98–99% and was not significantly statistically different from that of nematodes grown without dickeyocin P2D1. The survival of C. elegans was not dickeyocin P2D1-concentration-dependent, as similar survival rates were recorded for all dickeyocin concentrations tested (Supplementary Figure S4). Furthermore, dickeyocin P2D1 did not exhibit bactericidal activity against E. coli OP50—the food source for C. elegans.
Discussion
Our understanding of the mechanisms underlying bacterial competitive behaviors remains limited. Although numerous bacterial species are known to produce and exploit tailocins for their competitive advantage, little is known of how commonly such entities are employed by plant-pathogenic bacteria, including pectinolytic, necrotrophic SRP pathogens, to enable their environmental fitness (Scholl, 2017; Patz et al., 2019). This study revealed the presence of tailocins in one of the broadest characterized and economically important member of the Soft Rot Pectobacteriaceae family—strain D. dadantii 3937. We identified and characterized in detail a novel phage tail-like particle—dickeyocin P2D1, produced by this strain not only upon mitomycin C treatment but also constitutively in culture. Although tailocins were initially noted in a limited number of Dickeya spp. (strains of Erwinia chrysanthemi dissimilar from strain 3937) isolated from several crop and ornamental plants in the past (1960 and 1970s; Echandi, 1979), these particles were not characterized in detail, probably due to the lack of appropriate molecular techniques at that time.
Dickeyocin P2D1 has a typical morphology and expresses features similar to those of other R-type tailocins described so far for a large group of bacteria, including Escherichia coli (Mennigmann, 1965), Burkholderia cenocepacia (Yao et al., 2017), Pseudomonas aeruginosa (Scholl, 2017), Proteus vulgaris (Coetzee et al., 1968), and Yersinia enterocolitica (Strauch et al., 2001). Likewise, tailocins were also identified in Pectobacterium spp. (Kamimiya et al., 1977; Choi and Kim, 1988; Yamada et al., 2006)—plant pathogens closely related to Dickeya spp. The tailocin of SRP bacteria best characterized to date is carotovorocin Er isolated from P. carotovorum Er (Kamimiya et al., 1977; Nguyen et al., 2002). Interestingly, as shown in this study, despite the morphological similarities of dickeyocin P2D1 and carotovorocin Er and the rather close phylogenetic relationship and the lifestyle shared by the producer strains, these tailocins show little sequence homology. This suggests that dickeyocin P2D1 and carotovorocin Er originated from different phage ancestors and that the ability to produce tailocins was acquired several times and independently by various members of the SRP family.
The cluster encoding dickeyocin P2D1 exhibits high homology with that encoding the tail of phage Peduovirus P2, indicating that these two probably had a common ancestor. Phage P2 is a temperate bacteriophage commonly found in genomes of strains belonging to the Pseudomonadota phylum (Christie and Calendar, 2016). Its presence has been noted in at least 127 genera in 32 Pseudomonadota families (Casjens and Grose, 2016), indicating its extreme ubiquitousness in the bacterial world. Accordingly, R-type tailocins resembling the tail of phage P2 have been extensively studied and are now the best characterized to date (Scholl, 2017; Granato et al., 2019). To explore the occurrence of R-type tailocins in SRP, we searched available Dickeya spp. and Pectobacterium spp. genomes for regions carrying phage tail-like genes homologous to those encoding dickeyocin P2D1 and having organizational similarity. Our analysis revealed the presence of P2D1-like clusters in several Dickeya spp. strains, including D. solani, D. dianthicola, D. zeae, and D. fangzhongdai. The P2D1-like clusters were, however, absent in all Pectobacterium spp. genomes as well as in genomes of the other bacterial species unrelated to SRP analyzed in our study. The lack of the dickeyocin P2D1 cluster both in Pectobacterium spp. and in bacteria phylogenetically distant to SRP may indicate a strong and sustained phylogenetic association between dickeyocin P2D1and Dickeya spp. This is somewhat unexpected given that various Dickeya and Pectobacterium are often present in the same environment (e.g., soil, water, and plant surface; Van Gijsegem et al., 2021). Under such conditions, horizontal gene transfer would frequently occur between these phylogenetically related SRP species (Toth et al., 2021).
The restricted occurrence of genes encoding dickeyocin P2D1 in the genomes of Dickeya spp. aligns with its narrow bactericidal activity. In our study, dickeyocin P2D1 exclusively targeted Dickeya species as well as Musicola paradisiaca (former Dickeya paradisiaca) but not any other bacteria tested. It is noteworthy, however, that these Dickeya strains differed somewhat in the susceptibility to dickeyocin P2D1 while these differences were unrelated to the phylogenetical distance between them. This is in line with other studies (Chopra et al., 2015; Fagundes et al., 2016; Principe et al., 2018) that showed that the host range of pyocins, tailocins produced by Pseudomonas spp., is usually restricted to kin strains (Patz et al., 2019). Likewise the tailocins of Escherichia coli (Tantoso et al., 2022) and Yersinia enterocolitica (Strauch et al., 2001) also killed only related taxa. Contrarily, some recent reports have revealed tailocins that target different species of the same genus and/or even different genera. For example, Burkholderia cenocepacia tailocins were active against other Burkholderia species (Yao et al., 2017) and those of P. fluorescens were active against Xanthomonas vesicatoria (Principe et al., 2018). The quite narrow target range of dickeyocin P2D1 is somewhat surprising given that multiple species of SRP bacteria are often found together in the same infected plant (Perombelon, 1988; Pérombelon, 1992; Ge et al., 2021). In such a setting, these various species would be expected to experience intense competition and would be expected to benefit from a promiscuous tailocin (Shyntum et al., 2019). It thus appears that in such mixed infections, Dickeya spp. and Pectobacterium spp. compete using mechanisms unrelated to phage-tail-like particles, as reported previously (Ma et al., 2007; Czajkowski et al., 2013), but this conjecture requires more experimental support. Similarly, the presumed bacterial receptor for P2D1 remains unidentified. A number of tailocins that engage with Pseudomonas spp. bacteria rely on lipopolysaccharide (LPS) components as their receptors (Scholl, 2017). It is plausible to consider that P2D1 may also engage with the bacterial LPS of susceptible hosts, but further research is required to investigate this interaction on a molecular level.
Our study revealed that dickeyocin P2D1 is produced even without induction with mitomycin C, although such induction boosts the tailocin production 10 to 100-fold. To precisely estimate the concentration of P2D1 particles, we used a well-established Poisson distribution killing method (Kageyama et al., 1964; Williams et al., 2008) as well as a new approach we developed. The Poisson distribution killing method is an indirect enumeration method as it employs the number of survivors in tailocin-treated bacterial suspensions. Advantage of indirect methods is that they provide the number of active (“loaded”) tailocin particles in a sample. In this study, we have complemented the indirect counting approach by direct enumeration of tailocin particles with NanoSight NS300. Direct particle counts avoid the need for culturing and, when combined with killing-based methods, enables an estimation of the fraction of active tailocins in preparation. This appears to be a novel application of NanoSight to evaluate the quality and the active fraction of tailocin preparations.
One of the immediate applications of dickeyocin P2D1 would be in controlling SRP infections in crops (Becker et al., 2022). A major practical limitation of tailocins as therapeutic agents is their narrow bactericidal range. This can, however, be at least partially overcome by using cocktails of tailocins having different bactericidal ranges, similarly as in the use of bacteriophages (Abedon et al., 2021). Despite this limitation, tailocins have shown to be effective antibacterial agents in various applications (Scholl and Martin, 2008; Behrens et al., 2017). They have several benefits compared to other biological control agents evaluated to date (Becker et al., 2022). One of the biggest advantages of tailocins is their incapacity to proliferate on-site after application. Likewise, tailocins themselves cannot spread via transduction or transformation mechanisms because only the protein products and not the encoding nucleic acids are employed. The high target selectivity of tailocins also prevents disruption of other, often beneficial bacteria present in the same niche. Likewise, since dickeyocin P2D1 was nontoxic to C. elegans, a model eukaryotic organism, such tailocins are unlikely to impact other eukaryotic organisms present in soil and/or on plants (Balciunas et al., 2013). Additional studies are needed to fully explore the potential of dickeyocin P2D1 to achieve plant disease control, such as targeting production, the long-term effectiveness, and consistency of control under field conditions, including stability, formulation, and eco-toxicological risks. Studies of the in planta expression of dickeyocin P2D1 should provide great insight into the interactions of Dickeya species with each other and with other bacteria under natural and agricultural conditions.
Materials and methods
Bacterial strains and culture conditions
All bacterial strains included in this study are listed in Supplementary Table S1. Bacteria were routinely propagated in liquid Trypticase Soy Broth (TSB, Oxoid) or on solid Trypticase Soy Agar (TSA, Oxoid) at 28°C for 24 h. Liquid cultures were agitated during incubation (120 rpm). When required, bacterial cultures were supplemented with kanamycin (Sigma-Aldrich) to a final concentration of 50 μg mL−1.
Induction, purification, and concentration of tailocins from Dickeya dadantii 3937 culture
Dickeya dadantii strain 3937 was grown overnight (ca. 16 h) in TSB at 28°C with shaking (120 rpm). The cultures were then rejuvenated by diluting them 1: 40 in 250 mL of fresh TSB medium. The diluted culture grew for 2.5 h under the same conditions. Such prepared 3937 cultures were then supplemented with mitomycin C (Abcam, Poland) to a final concentration of 0.1 μg mL−1 to induce the production of tailocins. Following mitomycin C treatment, the cultures were incubated for another 24 h at 28°C with shaking (120 rpm). Bacterial cells were then removed by centrifugation (10 min, 8,000 RCF), and the supernatant containing putative particles was collected and filtered through a sterile 0.2 μm PES (polyether sulfone) membrane filter using the Nalgene Rapid-Flow Sterile Disposable Filter Units (Thermo Fisher Scientific). Finally, to precipitate tailocins, PEG-8000 (Promega) was added to the filtrate to a final concentration of 10%, and the sample was incubated at 4°C on a magnetic stirrer for the next 16–24 h. The tailocins were collected by centrifugation (1 h, 16,000 RCF, 4°C) and resuspended in 5 mL of Phosphate Buffered Saline (PBS, pH 7.2, Sigma-Aldrich). The resulting pellet was resuspended in 1/50 of the initial volume of the initial sample. The purified particles were stored at 4°C for future use.
Initial qualitative screen of tailocins for the bactericidal activity
The activity of the purified and concentrated particles was initially tested qualitatively on a limited panel of bacterial strains (=17 strains, Supplementary Table S1) using a spot test assay as described before (Hockett and Baltrus, 2017; Yao et al., 2017).
Microscopic imaging
Tailocins were imaged using both transmission electron microscopy (TEM) and atomic force microscopy (AFM), as described earlier (Vacheron et al., 2021). TEM analyses were done at the Laboratory of Electron Microscopy (Faculty of Biology, University of Gdansk, Poland). For TEM analysis, particles obtained as described above were adsorbed onto carbon-coated grids (GF Microsystems), stained with 1.5% uranyl acetate (Sigma-Aldrich), and directly visualized with an electron microscope (Tecnai Spirit BioTWIN, FEI) using a previously described protocol (Bartnik et al., 2022). At least 10 images were taken for each preparation to estimate the diameters of the particles. For the AFM analysis, purified and PEG-concentrated particles were used directly without further preparations. AFM imaging was conducted in air mode using the Bioscope Resolve microscope (Bruker), in ScanAsyst (Peak Force Tapping) mode, employing the SCANASYST-AIR probes (f0 7.0 kHz, diameter < 12 nm, k: 0.4 N/m) as described earlier (Sokolova et al., 2023). Similarly, as described for TEM analysis, for AFM, at least 10 images were taken for each preparation to estimate the diameters of the particles.
Determination of the concentration of tailocins
To estimate the concentration of tailocins, three independent methods were applied.
Direct particle count with NanoSight NS300
NanoSight NS300 instrument (Malvern Panalytical), equipped with an sCMOS camera and a Blue488 laser, was used to directly assess the concentration and size distribution of particles obtained after induction and purification, as described above. The tailocin samples were diluted 1,000 times in sterile PBS buffer pH 7.2 (Sigma-Aldrich) to achieve the optimal concentration for observation. The camera gain was set to 14, the number of captures per sample equaled 5, each lasting 60 s, and the detection threshold was set to 5 as suggested by the manufacturer. Measurements were conducted at room temperature (ca. 22–23°C). Three biological replicates were used to determine the concentration and size distribution of the obtained tailocins, and the results were averaged for further analysis.
Semiquantitative estimation by a spot test
Samples interrogated for tailocins were serially 2-fold diluted in PBS pH 7.2 (Sigma-Aldrich; Yao et al., 2017). Two μL of each dilution were spotted onto TSA plates overlayed with 15 mL of soft top agar [Nutrient Broth (NB, Oxoid) with 7 g L−1 agar]. Before pouring, the soft top agar was cooled to 45°C and inoculated with 250 μL of an overnight culture of a tailocin indicator strain (susceptible strain M. paradisiaca). Plates were incubated overnight at 28°C. The highest dilutions of particles capable of cell lysis, visible as plaques (halos) in the bacterial lawn in soft top agar, were determined. Each tailocin dilution was tested in triplicates and the entire experiment was repeated two times with the same setup. The reciprocal of the highest dilution causing a visible plaque was defined as the value of the relative activity in arbitrary units (=1 AU).
Poisson distribution killing method
Tailocins were also quantified using the Poisson distribution killing method based on the protocol described by Yao et al. (2017) and initially introduced by Kageyama et al. (1964) and Williams et al. (2008). This method is based on the number of bactericidal events, determined from the number of bacterial survivors in a population with a known number of initial viable cells (Yao et al., 2017). To determine this, 10 μL of undiluted and 10-fold diluted samples of tailocins were added to 100 μL of an overnight TSB (Oxoid) culture of a susceptible strain Musicola paradisiaca NCPPB 2511 (108 CFU mL−1) and incubated for 40 min at 28°C with shaking (120 rpm). As a negative control, PBS pH 7.2 was used instead of the tailocins suspension. Each combination was tested in triplicates. After incubation, the suspensions were serially diluted up to 10−7 in PBS and plated in triplicate on TSA plates. The colonies that emerged following overnight incubation at 28°C were enumerated to calculate the bacterial survival ratio (S) in the treated samples compared to the negative control. S was calculated as the number of viable bacteria in a sample incubated with tailocins divided by the number of viable bacteria in the negative control. The number of lethal events per bacterial cell (m) was calculated as m = −ln(S). The total number of active killing particles per milliliter (based on the assumption that tailocins adsorption to bacterial cells in each sample was quantitative within the first 40 min incubation period) was calculated by multiplying m by the initial number of bacterial cells per milliliter (CFU mL−1).
Target range of the tailocins isolated from Dickeya dadantii 3937
Overnight cultures of the investigated strains were prepared in 1 mL aliquots of TSB in 2 mL microcentrifuge tubes (Eppendorf) which were incubated horizontally with shaking (120 rpm) at 28°C for 16–24 h. The overnight cultures were used as an inoculum in a spot test carried out on 48-well plates (Greiner). Briefly, 10 μL of the inoculum was transferred to each well of the plate and mixed with 500 μL of liquified soft top agar [Nutrient Broth, NB, (Oxoid) with 7 g L−1 agar], precooled to 45°C in a water bath. Plates were gently stirred (20 rpm) to ensure an even distribution of bacterial cells in the inoculated wells. After the agar had solidified, plates were left to dry for 10 min. in a laminar flow hood. 2 μL of 10-fold diluted tailocins (approximately 1010 particles mL−1) purified from mitomycin-induced cultures of D. dadantii 3937 were spotted on the surface of the inoculated soft-top agar in the wells of the multitier plate. Inoculated plates were incubated for 24 h at 28°C and then inspected for the presence of a growth inhibition. A spot of lack of growth was interpreted as the susceptibility of the given strain to the tailocins (positive reaction). 2 μL of sterile PBS was spotted on a lawn of a susceptible strain as a negative control. The susceptibility of each bacterial strain was tested in triplicate, and the entire experiment was repeated twice.
Determination of the bacterial killing rate
The rapidity by which tailocins killed bacteria was measured in 96-well plates (Nest) using an Epoch 2 microplate reader (BioTek). 25 μL of a suspension containing tailocins (approximately 1011 particles mL−1) in PBS pH 7.2 were added to 100 μL of 5 McF (approximately 108 CFU mL−1) bacterial suspension in PBS. An overnight culture of a tested strain in TSB were harvested by centrifugation (5 min, 8,000 RCF). The OD600 of the PBS suspensions was measured each minute for 2 h. The plate was incubated at 28°C with shaking. The susceptible strain M. paradisiaca strain NCPPB 2511 was used as a positive control, and sterile PBS pH 7.2 without tailocins was used as a negative control. The log-transformed values of OD600 at each time point were normalized to the log-transformed starting OD600 and regressed against time. The regression coefficient [∆Log10(OD600)min−1] was calculated for each of the obtained curves. The killing proportion was estimated at two representative time points (20 and 120 min) as the average % of the initial OD600 of the selected bacterial culture compared to controls (n = 10).
Analysis of the tailocins with SDS-PAGE and ESI LC–MS/MS
Proteins within tailocins were separated using a 4–20% sodium dodecyl sulfate-polyacrylamide gradient gel (Mini-PROTEAN TGX Stain-Free Precast, Bio-Rad Hercules, United States) electrophoresis (SDS-PAGE) using previous methods (Sambrook et al., 1989). Protein bands were excised from the gel using a sterile scalpel, and the excised gel pieces were placed in separate 1.5 mL Eppendorf tubes for amino acid sequencing. In-gel digestion was performed according to a standard protocol consisting of gel de-coloration and removal of Coomassie staining, reduction/alkylation with dithiothreitol (DTT), and iodoacetamide (IAA), respectively (Golebiowski et al., 2022). First, digestion was carried out overnight with trypsin (Promega Mass Spectrometry Grade Gold) at 37°C. The tryptic peptides were then eluted from the gel with sequential washing of gel pieces with 50 mM ammonium bicarbonate buffer, 5% formic acid in 50% acetonitrile, and 100% acetonitrile (Goldman et al., 2019). All samples were then concentrated (SpeedVac), and the final cleanup was carried out using the StageTips method on the C18 phase to a 50% acetonitrile solution with 1% acetic acid (Schmidt and Sinz, 2017). After concentrating the samples to 30 mL using the SpeedVac, fragmentation mass spectra were recorded for analysis. ESI LC–MS/MS analysis was performed on Triple Tof 5600+ mass spectrometer with DuoSpray Ion Source (AB SCIEX, Framingham, MA, United States) connected to the Eksigent microLC (Ekspert MicroLC 200 PLus System, Eksigent, Redwood City, CA, United States) equipped with the ChromXP C18CL column (3 μm, 120 Å, 150 mm × 0.5 mm). The microLC−MS/MS system was controlled by the AB SCIEX Analyst TF 1.6 software. Chromatographic separation was carried out for 30 min in a gradient program: (1) 0–1 min—20% solvent B, (2) 1–26 min—20 − 60% solvent B, (3) 26–28 min—98% solvent B, and (4) 29–30 min—20% solvent B, where solvent A was 0.1% formic acid in water and solvent B 0.1% formic acid in acetonitrile. The identification of proteins present in the examined gel bands was carried out based on the obtained fragmentation spectra using the ProteinPilot software (v 4.5) or Peaks Studio and the appropriate protein database (UniProt Dickeya dadantii 15.02.2023, unreviewed) with an automated false discovery rate (1% FDR).
Bioinformatic analyses
Prediction of prophage regions in the genome of D. dadantii 3937 (Genbank accession: NC_014500.1) was conducted using PHASTER (Arndt et al., 2016). The tailocin cluster and individual proteins it encodes were analyzed against NCBI databases using BLASTn and BLASTp (Altschul et al., 1990). The topology of the tailocin cluster in D. dadantii 3937 was investigated using BioCyc (Karp et al., 2019). Phylogenomic analysis of the Soft Rot Pectobacteriaceae (SRP) genomes was based on core genome sequences and was performed using EDGAR ver. 3.0 (Dieckmann et al., 2021) accessed via https://edgar3.computational.bio.uni-giessen.de.
Stability of tailocins
Tailocins were incubated for 24 h at several different temperatures (−80, −20, 4, 22, 28, 37, 42, 50, 65, and 80°C), pH values (2, 5, 3.5, 7, 9, 10.5, and 12), and NaCl concentrations (0.01, 0.1, 1, and 5 M), after which their bacterial growth inhibitory activity was assessed by a spot test using M. paradisiaca as described above. All tested samples had a total volume of 200 μL, and the initial concentration of PEG-purified tailocin of approximately 1010 particles mL−1. To obtain the test samples, tailocins were diluted by mixing a volume of 20 μL of PEG-purified samples with 180 μL of either PBS (temperature stability), PBS with pH modified by addition of either HCl or NaOH to the desired values (pH stability), or in water containing different concentrations of NaCl. The experiments were repeated three times, each with three technical replications per tested condition.
Effect of enzyme/detergent/organic solvent treatment on the activity of tailocins
Tailocins were incubated for 1 h at 37°C with the following enzymes: proteinase K (Sigma-Aldrich, final concentration: 0.5 mg mL−1), DNase (Sigma-Aldrich, final concentration: 10 U mL−1), or lysozyme (Sigma-Aldrich, final concentration: 0.5 mg mL−1), and at room temperature (22°C) with sodium dodecyl sulfate (SDS, Sigma Aldrich, final concentration: 1%), or chloroform (POCH, 50% v/v). The remaining activity of treated tailocins was then assessed in a spot test as described above. The processed samples had a total volume of 200 μL, and a tailocin concentration of approximately 1010 particles mL−1. The samples were prepared by mixing 20 μL of PEG-purified samples (about 1011 particles mL−1) with the appropriate volume of the factor stock to a final volume of 200 μL with PBS. The exception were the chloroform-treated samples where 200 μL of sample were mixed on a shaker with 200 μL of chloroform. Prior to testing, the chloroform-treated sample was centrifuged (5 min at 4000 RCF), and the aqueous phase was removed for assay. The experiments were repeated three times, each with three technical repetitions per tested condition.
Binding of tailocins to nonviable bacterial cells
Overnight bacterial cultures of M. paradisiaca NCPPB 2511 and D. dadantii strain 3937 grown in TSB were washed twice with PBS buffer and then killed by incubation with 5 mg mL−1 chloramphenicol (Sigma-Aldrich) for 60 min, with shaking (120 rpm, 28°C; Gonzalez and Kunka, 1987). The killing of the cells after 1 h was confirmed by plating 4 aliquots (10 μL) of treated culture on TSA plates, incubating at 28°C for 24 h, and verifying last of bacterial growth. After killing, the nonviable bacterial cells were again washed three times with PBS to remove the remaining antibiotic. The PEG-purified tailocin samples were then added to suspension of viable and nonviable susceptible and nonsusceptible bacteria to a final concentration of approximately 1010 particles mL−1 and incubated for 40 min with shaking at 120 rpm at 28°C. After incubation, samples were filtered through a 0.2 μm PES (polyether sulfone) membrane filter, and the remaining tailocins was assessed by a spot test using M. paradisiaca. The experiments were repeated three times, each with three technical replication per tested condition.
Testing the influence of pH on the bactericidal activity of tailocins
The PEG-purified tailocins (approximately 1011 particles mL−1) were diluted 10-fold in PBS buffered to pH 2, 7, and 12 and incubated for 24 h at room temperature. The pH of each sample was then adjusted to neutral pH (pH 7) using an equal volume of the buffered PBS. The solutions were then incubated for 4 h at room temperature and tested for growth inhibitory activity using a spot test in three replicates as described above.
Caenorhabditis elegans toxicity assay
Sensitivity of Caenorhabditis elegans to tailocins produced by D. dadantii was tested as described before (Kirienko et al., 2014) with slight modifications. Briefly, wild-type Bristol N2 strain of C. elegans nematode obtained from the Caenorhabditis Genetic Center (CGC, University of Minnesota, Minneapolis, United States) was maintained as described before (Stiernagle, 2006; Krzyzanowska et al., 2019) on Nematode Growth Medium (NGM) plates with Escherichia coli strain OP50 as a food source. The toxicity of the tailocins was tested on nematode cultures synchronized as described earlier (Porta-de-la-Riva et al., 2012). Briefly, nematode eggs were harvested from cultures treated with a mixture of 5 M NaOH and 5.25% NaOCl (1, 3, v:v)—a treatment that eliminates adult worms. Recovered eggs were hatched in an S-complete medium, and the nematodes were grown to the L4 stage using E. coli OP50 as a food source. The synchronized cultures were then transferred into wells of a 96-well plate. The worms were counted under the microscope (Leica MZ10f stereomicroscope, Leica) and ca. 30 worms placed in each well supplemented with PEG-purified and ultracentrifugation concentrated (1 h, 26,000 RCF, 4°C) tailocin in an S-complete medium at a concentration of 1012 particles mL−1. Nematode cultures grown without tailocins were used as control. The plates were incubated for 24 h in the dark at 25°C. The survival of nematodes in tailocin-supplemented cultures was compared to the control. The experiment was repeated twice with the same setup, and the results were averaged for analysis.
Statistical analysis
All statistical tests were conducted using either Past 4.13 software (Hammer et al., 2001) or Microsoft Office Excel.1 The Shapiro–Wilk (Shapiro and Wilk, 1965) and F-tests (Shen and Faraway, 2004) were used to test for normality and variance equality of data, respectively. For pairwise testing, the t-test (Semenick, 1990) was applied for data having a normal distribution and equal variances, the Welch test (Welch, 1947) was used for samples with a normal distribution but unequal variances, while the U Mann–Whitney test (Mann and Whitney, 1947) was applied for data that were not normally distributed. One-way ANOVA (Ross and Willson, 2017) was used to compare more than two data groups. Levene’s test (Schultz, 1985) was used to test the homogeneity of variance, and the normality of the residuals was conducted using the Shapiro–Wilk test. Welch’s one-way ANOVA, followed by the Games-Howell post hoc test (Mégevand, 2022), was used for the data groups with non-homogeneous variance and normally distributed residuals. For groups with a non-homogeneous variance and without normally distributed residuals Kruscal-Wallis’s one-way ANOVA (McKight and Najab, 2010) followed by Dunn’s post hoc test (Ruxton and Beauchamp, 2008) was applied.
Data availability statement
The datasets presented in this study can be found in online repositories. The names of the repository/repositories and accession number(s) can be found in the article/Supplementary material.
Author contributions
MB: Conceptualization, Investigation, Methodology, Visualization, Writing – original draft, Writing – review & editing. DK: Conceptualization, Investigation, Supervision, Visualization, Writing – original draft, Writing – review & editing. MN: Investigation, Methodology, Visualization, Writing – original draft. MS: Investigation, Methodology, Writing – original draft. MR: Investigation, Methodology, Writing – original draft. PC: Methodology, Writing – original draft. KW: Investigation, Methodology, Writing – original draft. RC: Conceptualization, Data curation, Funding acquisition, Resources, Supervision, Writing – original draft, Writing – review & editing.
Funding
The author(s) declare financial support was received for the research, authorship, and/or publication of this article. This research was financially supported by the National Science Center, Poland (Narodowe Centrum Nauki, Polska) via a research grant SONATA BIS 10 (2020/38/E/NZ9/00007) to RC.
Acknowledgments
The authors would like to express their gratitude to Nicole Hugouvieux-Cotte-Pattat [Laboratory of Microbiology Adaptation and Pathogenesis (UMR 5240) CNRS, Université Lyon 1 & INSA de Lyon, France] for providing strain A5587 (D. dadantii strain 3937 tssK::uidA kanR) for this study, Alfonso Jaramillo and Cristina Ramos [De novo Synthetic Biology Lab, Institute for Integrative Systems Biology (I2SysBio-CSIC)], for providing valuable information on the P2 phage, Jochen Blom (Justus Liebig University, Giessen, Germany) for helpful discussion on phylogenomic analysis, and Steven E. Lindow (University of California-Berkeley, Berkeley, CA, United States) for his comments on the manuscript and his editorial work.
Conflict of interest
The authors declare that the research was conducted in the absence of any commercial or financial relationships that could be construed as a potential conflict of interest.
The reviewer AJ-K declared a shared affiliation with the authors to the handling editor at the time of review.
The author(s) declared that they were an editorial board member of Frontiers, at the time of submission. This had no impact on the peer review process and the final decision.
Publisher’s note
All claims expressed in this article are solely those of the authors and do not necessarily represent those of their affiliated organizations, or those of the publisher, the editors and the reviewers. Any product that may be evaluated in this article, or claim that may be made by its manufacturer, is not guaranteed or endorsed by the publisher.
Supplementary material
The Supplementary material for this article can be found online at: https://www.frontiersin.org/articles/10.3389/fmicb.2023.1307349/full#supplementary-material
Footnotes
References
Abedon, S. T., Danis-Wlodarczyk, K. M., and Wozniak, D. J. (2021). Phage cocktail development for bacteriophage therapy: toward improving Spectrum of activity breadth and depth. Pharmaceuticals (Basel) 14, 1–25. doi: 10.3390/ph14101019
Altschul, S. F., Gish, W., Miller, W., Myers, E. W., and Lipman, D. J. (1990). Basic local alignment search tool. J. Mol. Biol. 215, 403–410. doi: 10.1016/S0022-2836(05)80360-2
Arndt, D., Grant, J. R., Marcu, A., Sajed, T., Pon, A., Liang, Y., et al. (2016). PHASTER: a better, faster version of the PHAST phage search tool. Nucleic Acids Res. 44, W16–W21. doi: 10.1093/nar/gkw387
Balciunas, E. M., Martinez, F. A. C., Todorov, S. D., De Melo Franco, B. D. G., Converti, A., and De Souza Oliveira, R. P. (2013). Novel biotechnological applications of bacteriocins: a review. Food Control 32, 134–142. doi: 10.1016/j.foodcont.2012.11.025
Bartnik, P., Lewtak, K., Fiolka, M., Czaplewska, P., Narajczyk, M., and Czajkowski, R. (2022). Resistance of Dickeya solani strain IPO 2222 to lytic bacteriophage PhiD5 results in fitness tradeoffs for the bacterium during infection. Sci. Rep. 12:10725. doi: 10.1038/s41598-022-14956-7
Bauer, M. A., Kainz, K., Carmona-Gutierrez, D., and Madeo, F. (2018). Microbial wars: competition in ecological niches and within the microbiome. Microb. Cell 5, 215–219. doi: 10.15698/mic2018.05.628
Becker, Y., Patz, S., Werner, S., Hoppe, B., Feltgen, S., Berger, B., et al. (2022). Bacteria producing contractile phage tail-like particles (CPTPs) are promising alternatives to conventional pesticides. J. Kult. 74, 85–93. doi: 10.5073/JfK.2022.03-04.06
Behrens, H. M., Six, A., Walker, D., and Kleanthous, C. (2017). The therapeutic potential of bacteriocins as protein antibiotics. Emerg. Top Life Sci. 1, 65–74. doi: 10.1042/ETLS20160016
Bertani, G. (1951). Studies on lysogenesis. I. The mode of phage liberation by lysogenic Escherichia coli. J. Bacteriol. 62, 293–300. doi: 10.1128/jb.62.3.293-300.1951
Casjens, S. R., and Grose, J. H. (2016). Contributions of P2-and P22-like prophages to understanding the enormous diversity and abundance of tailed bacteriophages. Virology 496, 255–276. doi: 10.1016/j.virol.2016.05.022
Charkowski, A. O. (2007). “The soft rot Erwinia,” in Plant-Associated Bacteria. ed. S. S. Gnanamanickam (Dordrecht: Springer).
Charkowski, A. O. (2018). The changing face of bacterial soft-rot diseases. Annu. Rev. Phytopathol. 56, 269–288. doi: 10.1146/annurev-phyto-080417-045906
Choi, J. K., and Kim, I. O. (1988). Properties of carotovoricin H, a bacteriocin from Erwinia carotovora subsp. carotovora. Plant Pathol. J. 4, 33–39.
Chopra, L., Singh, G., Kumar Jena, K., and Sahoo, D. K. (2015). Sonorensin: a new bacteriocin with potential of an anti-biofilm agent and a food biopreservative. Sci. Rep. 5:13412. doi: 10.1038/srep13412
Christie, G. E., and Calendar, R. (2016). Bacteriophage P2. Bacteriophage 6:e1145782. doi: 10.1080/21597081.2016.1145782
Coetzee, H. L., De Klerk, H. C., Coetzee, J. N., and Smit, J. A. (1968). Bacteriophage-tail-like particles associated with intra-species killing of Proteus vulgaris. J. Gen. Virol. 2, 29–36. doi: 10.1099/0022-1317-2-1-29
Czajkowski, R., De Boer, W. J., Van Der Zouwen, P. S., Kastelein, P., Jafra, S., De Haan, E. G., et al. (2013). Virulence of ‘Dickeya solani’ Anddickeya dianthicolabiovar-1 and-7 strains on potato (Solanum tuberosum). Plant Pathol. 62, 597–610. doi: 10.1111/j.1365-3059.2012.02664.x
Dieckmann, M. A., Beyvers, S., Nkouamedjo-Fankep, R. C., Hanel, P. H. G., Jelonek, L., Blom, J., et al. (2021). EDGAR3.0: comparative genomics and phylogenomics on a scalable infrastructure. Nucleic Acids Res. 49, W185–W192. doi: 10.1093/nar/gkab341
Echandi, E. (1979). Production, properties, and morphology of Bacteriocins from Erwinia chrysanthemi. Phytopathology 69, 1204–1207. doi: 10.1094/Phyto-69-1204
Fagundes, P. C., Farias, F. M., Santos, O. C. S., De Oliveira, N. E. M., Da Paz, J. A. S., Ceotto-Vigoder, H., et al. (2016). The antimicrobial peptide aureocin A53 as an alternative agent for biopreservation of dairy products. J. Appl. Microbiol. 121, 435–444. doi: 10.1111/jam.13189
Fischer, S., Godino, A., Quesada, J. M., Cordero, P., Jofre, E., Mori, G., et al. (2012). Characterization of a phage-like pyocin from the plant growth-promoting rhizobacterium Pseudomonas fluorescens SF4c. Microbiology 158, 1493–1503. doi: 10.1099/mic.0.056002-0
Ge, T., Ekbataniamiri, F., Johnson, S. B., Larkin, R. P., and Hao, J. (2021). Interaction between Dickeya dianthicola and Pectobacterium parmentieri in potato infection under field conditions. Microorganisms 9:316. doi: 10.3390/microorganisms9020316
Ge, P., Scholl, D., Leiman, P. G., Yu, X., Miller, J. F., and Zhou, Z. H. (2015). Atomic structures of a bactericidal contractile nanotube in its pre-and postcontraction states. Nat. Struct. Mol. Biol. 22, 377–382. doi: 10.1038/nsmb.2995
Gebhart, D., Lok, S., Clare, S., Tomas, M., Stares, M., Scholl, D., et al. (2015). A modified R-type Bacteriocin specifically targeting Clostridium difficile prevents colonization of mice without affecting gut microbiota diversity. MBio 6:6. doi: 10.1128/mbio.02368-02314
Ghequire, M. G. K., and De Mot, R. (2014). Ribosomally encoded antibacterial proteins and peptides from Pseudomonas. FEMS Microbiol. Rev. 38, 523–568. doi: 10.1111/1574-6976.12079
Ghequire, M. G. K., and De Mot, R. (2015). The Tailocin tale: peeling off phage tails. Trends Microbiol. 23, 587–590. doi: 10.1016/j.tim.2015.07.011
Ghoul, M., and Mitri, S. (2016). The ecology and evolution of microbial competition. Trends Microbiol. 24, 833–845. doi: 10.1016/j.tim.2016.06.011
Golanowska, M. (2015). Characterization of Dickeya solani strains and identification of bacterial and plant signals involved in the induction of virulence. Doctor of philosophy, CNRS, Université Lyon 1 & INSA de Lyon, France, University of Gdansk, Gdansk, Poland.
Goldman, A. R., Beer, L. A., Tang, H. Y., Hembach, P., Zayas-Bazan, D., and Speicher, D. W. (2019). Proteome analysis using gel-LC-MS/MS. Curr. Protoc. Protein Sci. 96:e93. doi: 10.1002/cpps.93
Golebiowski, A., Pomastowski, P., Rafinska, K., Zuvela, P., Wong, M. W., Pryshchepa, O., et al. (2022). Functionalization of alpha-Lactalbumin by zinc ions. ACS Omega 7, 38459–38474. doi: 10.1021/acsomega.2c03674
Gonzalez, C. F., and Kunka, B. S. (1987). Plasmid-associated Bacteriocin production and sucrose fermentation in Pediococcus acidilactici. Appl. Environ. Microbiol. 53, 2534–2538. doi: 10.1128/aem.53.10.2534-2538.1987
Gorter, F. A., Manhart, M., and Ackermann, M. (2020). Understanding the evolution of interspecies interactions in microbial communities. Philos. Trans. R. Soc. Lond. Ser. B Biol. Sci. 375:20190256. doi: 10.1098/rstb.2019.0256
Granato, E. T., Meiller-Legrand, T. A., and Foster, K. R. (2019). The evolution and ecology of bacterial warfare. Curr. Biol. 29, R521–R537. doi: 10.1016/j.cub.2019.04.024
Hammer, Ø., Harper, D. A. T., and Ryan, P. D. (2001). PAST: paleontological statistics software package for education and data analysis. Palaeontol. Electron. 4:9.
Hibbing, M. E., Fuqua, C., Parsek, M. R., and Peterson, S. B. (2010). Bacterial competition: surviving and thriving in the microbial jungle. Nat. Rev. Microbiol. 8, 15–25. doi: 10.1038/nrmicro2259
Hockett, K. L., and Baltrus, D. A. (2017). Use of the soft-agar overlay technique to screen for bacterially produced inhibitory compounds. J. Vis. Exp. (119), e55064. doi: 10.3791/55064
Kageyama, M., Ikeda, K., and Egami, F. (1964). Studies of a Pyocin. Iii. Biological properties of the Pyocin. J. Biochem. 55, 59–64. doi: 10.1093/oxfordjournals.jbchem.a127841
Kamimiya, S., Izaki, K., and Takahashi, H. (1977). Bacteriocins in Erwinia-Aroideae with tail like structure of bacteriophages. Agric. Biol. Chem. 41, 911–912.
Karp, P. D., Billington, R., Caspi, R., Fulcher, C. A., Latendresse, M., Kothari, A., et al. (2019). The bio Cyc collection of microbial genomes and metabolic pathways. Brief. Bioinform. 20, 1085–1093. doi: 10.1093/bib/bbx085
Kirienko, N. V., Cezairliyan, B. O., Ausubel, F. M., and Powell, J. R. (2014). “Pseudomonas aeruginosa PA14 pathogenesis in Caenorhabditis elegans” in Pseudomonas methods and protocols. eds. A. Filloux and J.-L. Ramos (New York, NY: Springer New York), 653–669.
Kotoujansky, A. (1987). Molecular genetics of pathogenesis by soft-rot Erwinias. Annu. Rev. Phytopathol. 25, 405–430. doi: 10.1146/annurev.py.25.090187.002201
Kotoujansky, A., Lemattre, M., and Boistard, P. (1982). Utilization of a thermosensitive episome bearing transposon TN10 to isolate Hfr donor strains of Erwinia carotovora subsp. chrysanthemi. J. Bacteriol. 150, 122–131. doi: 10.1128/jb.150.1.122-131.1982
Krzyzanowska, D. M., Maciag, T., Siwinska, J., Krychowiak, M., Jafra, S., and Czajkowski, R. (2019). Compatible mixture of bacterial antagonists developed to protect potato tubers from soft rot caused by Pectobacterium spp. and Dickeya spp. Plant Dis. 103, 1374–1382. doi: 10.1094/PDIS-10-18-1866-RE
Little, A. E., Robinson, C. J., Peterson, S. B., Raffa, K. F., and Handelsman, J. (2008). Rules of engagement: interspecies interactions that regulate microbial communities. Annu. Rev. Microbiol. 62, 375–401. doi: 10.1146/annurev.micro.030608.101423
Liu, J., Chen, P., Zheng, C., and Huang, Y. P. (2013). Characterization of maltocin P28, a novel phage tail-like bacteriocin from Stenotrophomonas maltophilia. Appl. Environ. Microbiol. 79, 5593–5600. doi: 10.1128/AEM.01648-13
Ma, B., Hibbing, M. E., Kim, H. S., Reedy, R. M., Yedidia, I., Breuer, J., et al. (2007). Host range and molecular phylogenies of the soft rot enterobacterial genera pectobacterium and dickeya. Phytopathology 97, 1150–1163. doi: 10.1094/PHYTO-97-9-1150
Mann, H. B., and Whitney, D. R. (1947). On a test of whether one of two random variables is stochastically larger than the other. Ann. Math. Stat. 18, 50–60. doi: 10.1214/aoms/1177730491
Mckight, P. E., and Najab, J. (2010). “Kruskal-wallis test” in The Corsini Encyclopedia of Psychology. eds. I. B. Weiner and W. Edward Craighead (Wiley) 1.
Mennigmann, H. (1965). Electronmicroscopy of the anti-bacterial agent produced by Escherichia coli 15. Microbiology 41, 151–154.
Michel-Briand, Y., and Baysse, C. (2002). The pyocins of Pseudomonas aeruginosa. Biochimie 84, 499–510. doi: 10.1016/S0300-9084(02)01422-0
Morales-Soto, N., Gaudriault, S., Ogier, J.-C., Thappeta, K. R. V., and Forst, S. (2012). Comparative analysis of P2-type remnant prophage loci in Xenorhabdus bovienii and Xenorhabdus nematophila required for xenorhabdicin production. FEMS Microbiol. Lett. 333, 69–76. doi: 10.1111/j.1574-6968.2012.02600.x
Nguyen, H. A., Kaneko, J., and Kamio, Y. (2002). Temperature-dependent production of carotovoricin Er and pectin lyase in phytopathogenic Erwinia carotovora subsp. carotovora Er. Biosci. Biotechnol. Biochem. 66, 444–447. doi: 10.1271/bbb.66.444
Nguyen, V. S., Logger, L., Spinelli, S., Legrand, P., Huyen Pham, T. T., Nhung Trinh, T. T., et al. (2017). Type VI secretion TssK baseplate protein exhibits structural similarity with phage receptor-binding proteins and evolved to bind the membrane complex. Nat. Microbiol. 2:17103. doi: 10.1038/nmicrobiol.2017.103
Patz, S., Becker, Y., Richert-Poggeler, K. R., Berger, B., Ruppel, S., Huson, D. H., et al. (2019). Phage tail-like particles are versatile bacterial nanomachines—a mini-review. J. Adv. Res. 19, 75–84. doi: 10.1016/j.jare.2019.04.003
Perombelon, M. C. M. (1988). “Ecology of Erwinias causing stem and tuber diseases” in Bacterial disease of potato: Report of the planning conference on bacterial diseases of potato).
Pérombelon, M. C. M. (1992). Potato blackleg: epidemiology, host-pathogen interaction and control. Neth. J. Plant Pathol. 98, 135–146. doi: 10.1007/BF01974480
Perombelon, M. C. M. (2002). Potato diseases caused by soft rot erwinias: an overview of pathogenesis. Plant Pathol. 51, 1–12. doi: 10.1046/j.0032-0862.2001.Shorttitle.doc.x
Porta-De-La-Riva, M., Fontrodona, L., Villanueva, A., and Ceron, J. (2012). Basic Caenorhabditis elegans methods: synchronization and observation. J. Vis. Exp. 64:e4019. doi: 10.3791/4019-v
Principe, A., Fernandez, M., Torasso, M., Godino, A., and Fischer, S. (2018). Effectiveness of tailocins produced by Pseudomonas fluorescens SF4c in controlling the bacterial-spot disease in tomatoes caused by Xanthomonas vesicatoria. Microbiol. Res. 212-213, 94–102. doi: 10.1016/j.micres.2018.05.010
Reverchon, S., Muskhelisvili, G., and Nasser, W. (2016). “Chapter three-virulence program of a bacterial plant pathogen: the Dickeya model” in Progress in Molecular Biology and Translational Science. ed. D. B. Teplow (San Francisco: Academic Press), 51–92.
Reverchon, S., and Nasser, W. (2013). Dickeya ecology, environment sensing and regulation of virulence programme. Environ. Microbiol. Rep. 5, 622–636. doi: 10.1111/1758-2229.12073
Ross, A., and Willson, V. L. (2017). “One-way ANOVA” in Basic and Advanced Statistical Tests (SensePublishers), 21–24.
Ruxton, G. D., and Beauchamp, G. (2008). Time for some a priori thinking about post hoc testing. Behav. Ecol. 19, 690–693. doi: 10.1093/beheco/arn020
Sambrook, J., Fritsch, E. F., and Maniatis, T. (1989). Molecular Cloning: A Laboratory Manual. Cold Spring Harbor, NY, USA: Cold Spring Harbor Laboratory Press.
Samson, R., Legendre, J. B., Christen, R., Fischer-Le Saux, M., Achouak, W., and Gardan, L. (2005). Transfer of Pectobacterium chrysanthemi (Burkholder et al. 1953) Brenner et al. 1973 and Brenneria paradisiaca to the genus Dickeya gen. Nov. as Dickeya chrysanthemi comb. nov. and Dickeya paradisiaca comb. nov. and delineation of four novel species, Dickeya dadantii sp. nov., Dickeya dianthicola sp. nov., Dickeya dieffenbachiae sp. nov. and Dickeya zeae sp. nov. Int. J. Syst. Evol. Microbiol. 55, 1415–1427. doi: 10.1099/ijs.0.02791-0
Schmidt, R., and Sinz, A. (2017). Improved single-step enrichment methods of cross-linked products for protein structure analysis and protein interaction mapping. Anal. Bioanal. Chem. 409, 2393–2400. doi: 10.1007/s00216-017-0185-1
Scholl, D. (2017). Phage tail–like bacteriocins. Annu. Rev. Virol. 4, 453–467. doi: 10.1146/annurev-virology-101416-041632
Scholl, D., and Martin, D. W. Jr. (2008). Antibacterial efficacy of R-type pyocins towards Pseudomonas aeruginosa in a murine peritonitis model. Antimicrob. Agents Chemother. 52, 1647–1652. doi: 10.1128/AAC.01479-07
Schultz, B. B. (1985). Levene’s test for relative variation. Syst. Zool. 34, 449–456. doi: 10.2307/2413207
Shapiro, S. S., and Wilk, M. B. (1965). An analysis of variance test for normality (complete samples). Biometrika 52, 591–611. doi: 10.1093/biomet/52.3-4.591
Shen, Q., and Faraway, J. (2004). An F test for linear models with functional responses. Stat. Sin. 14, 1239–1257.
Shimodaira, H. (2002). An approximately unbiased test of phylogenetic tree selection. Syst. Biol. 51, 492–508. doi: 10.1080/10635150290069913
Shyntum, D. Y., Nkomo, N. P., Shingange, N. L., Gricia, A. R., Bellieny-Rabelo, D., and Moleleki, L. N. (2019). The impact of type VI secretion system, Bacteriocins and antibiotics on bacterial competition of Pectobacterium carotovorum subsp. brasiliense and the regulation of Carbapenem biosynthesis by Iron and the ferric-uptake regulator. Front. Microbiol. 10:2379. doi: 10.3389/fmicb.2019.02379
Smarda, J., and Benada, O. (2005). Phage tail-like (high-molecular-weight) bacteriocins of Budvicia aquatica and Pragia fontium (Enterobacteriaceae). Appl. Environ. Microbiol. 71, 8970–8973. doi: 10.1128/AEM.71.12.8970-8973.2005
Sokolova, D., Smolarska, A., Bartnik, P., Rabalski, L., Kosinski, M., Narajczyk, M., et al. (2023). Spontaneous mutations in hlyD and tuf genes result in resistance of Dickeya solani IPO 2222 to phage varphiD5 but cause decreased bacterial fitness and virulence in planta. Sci. Rep. 13:7534. doi: 10.1038/s41598-023-34803-7
Stiernagle, T. (2006). Maintenance of C. elegans. Available at: https://www.ncbi.nlm.nih.gov/books/NBK19649/
Strauch, E., Kaspar, H., Schaudinn, C., Dersch, P., Madela, K., Gewinner, C., et al. (2001). Characterization of enterocoliticin, a phage tail-like bacteriocin, and its effect on pathogenic Yersinia enterocolitica strains. Appl. Environ. Microbiol. 67, 5634–5642. doi: 10.1128/AEM.67.12.5634-5642.2001
Stubbendieck, R. M., and Straight, P. D. (2016). Multifaceted interfaces of bacterial competition. J. Bacteriol. 198, 2145–2155. doi: 10.1128/JB.00275-16
Tantoso, E., Eisenhaber, B., Kirsch, M., Shitov, V., Zhao, Z. Y., and Eisenhaber, F. (2022). To kill or to be killed: pangenome analysis of Escherichia coli strains reveals a tailocin specific for pandemic ST131. BMC Biol. 20, 1–26. doi: 10.1186/s12915-022-01347-7
Toth, I. K., Barny, M.-A., Czajkowski, R., Elphinstone, J. G., Li, X., Pédron, J., et al. (2021). “Pectobacterium and Dickeya: taxonomy and evolution” in Plant Diseases Caused by Dickeya and Pectobacterium Species. (eds.) F. GijsegemVan, J. M. WolfVan Der, and I. K. Toth (Cham: Springer International Publishing), 13–37
Vacheron, J., Heiman, C. M., and Keel, C. (2021). Live cell dynamics of production, explosive release and killing activity of phage tail-like weapons for Pseudomonas kin exclusion. Commun. Biol. 4:87. doi: 10.1038/s42003-020-01581-1
Van Gijsegem, F., Van Der Wolf, J. M., and Toth, I. K. (2021). Plant Diseases Caused by Dickeya and Pectobacterium Species. Springer Nature Switzerland.
Wagner, A. (2022). Competition for nutrients increases invasion resistance during assembly of microbial communities. Mol. Ecol. 31, 4188–4203. doi: 10.1111/mec.16565
Welch, B. L. (1947). The generalisation of student’s problems when several different population variances are involved. Biometrika 34, 28–35.
Williams, S. R., Gebhart, D., Martin, D. W., and Scholl, D. (2008). Retargeting R-type pyocins to generate novel bactericidal protein complexes. Appl. Environ. Microbiol. 74, 3868–3876. doi: 10.1128/AEM.00141-08
Yamada, K., Hirota, M., Niimi, Y., Nguyen, H. A., Takahara, Y., Kamio, Y., et al. (2006). Nucleotide sequences and organization of the genes for carotovoricin (Ctv) from Erwinia carotovora indicate that Ctv evolved from the same ancestor as Salmonella typhi prophage. Biosci. Biotechnol. Biochem. 70, 2236–2247. doi: 10.1271/bbb.60177
Keywords: bacteriophage, phage tail, tailocin, Erwinia chrysanthemi, bacteriocin, bacteria-bacteria interactions, Caenorhabditis elegans
Citation: Borowicz M, Krzyżanowska DM, Narajczyk M, Sobolewska M, Rajewska M, Czaplewska P, Węgrzyn K and Czajkowski R (2023) Soft rot pathogen Dickeya dadantii 3937 produces tailocins resembling the tails of Peduovirus P2. Front. Microbiol. 14:1307349. doi: 10.3389/fmicb.2023.1307349
Edited by:
Alicja Wegrzyn, Polish Academy of Sciences, PolandReviewed by:
Bartłomiej Grygorcewicz, Pomeranian Medical University, PolandRavikumar Patel, Connecticut Agricultural Experiment Station, United States
Agata Jurczak-Kurek, University of Gdansk, Poland
Copyright © 2023 Borowicz, Krzyżanowska, Narajczyk, Sobolewska, Rajewska, Czaplewska, Węgrzyn and Czajkowski. This is an open-access article distributed under the terms of the Creative Commons Attribution License (CC BY). The use, distribution or reproduction in other forums is permitted, provided the original author(s) and the copyright owner(s) are credited and that the original publication in this journal is cited, in accordance with accepted academic practice. No use, distribution or reproduction is permitted which does not comply with these terms.
*Correspondence: Robert Czajkowski, cm9iZXJ0LmN6YWprb3dza2lAdWcuZWR1LnBs