- 1Navy Submarine Academy, Qingdao, China
- 2CAS Key Laboratory of Marine Environment of Corrosion and Bio-fouling, Institute of Oceanology, Chinese Academy of Sciences, Qingdao, China
A large amount of nuclear waste produced in the process of nuclear energy utilization has always been a key problem to be solved urgently for nuclear safety. At present, “deep geological disposal” is a feasible method and generally accepted by many countries. The oxygen content in the near field environment of the waste container will decrease to anaerobic conditions, and hydrogen will permeation into the internal materials of container for a long time. Hydrogen evolution corrosion may cause a risk of hydrogen embrittlement. The harm of hydrogen embrittlement in metal container is far more severe than predictable uniform corrosion. It is a research hotspot that the microorganisms impact on the corrosion behavior of container materials in the deep geological environment. Microbial corrosion in deep geological environments can be divided into two types: aerobic microbial corrosion and anaerobic microbial corrosion. There is a type of hydrogen consuming microorganism in the natural environment that uses the oxidation of hydrogen as the energy for its life activities. This provides a new approach for us to study reducing the hydrogen embrittlement sensitivity of nuclear waste container materials.
Introduction
Nuclear energy has been widely used in fields such as medicine, national defense, agriculture, and industry due to its advantages of low cost, high efficiency and no greenhouse gas generation, greatly improving human life. However, high-level nuclear waste is an inevitable waste generated during the process of nuclear energy utilization, with characteristics such as high radioactivity, long half-life and high toxicity. The various high radioactive elements pose great harm to the human body and the biosphere. For example, technetium (Tc) is a problematic fission product, as its long half-life, high fission rate and environmental mobility of high technetium salts make long-term disposal of nuclear waste more complex (Lukens and Saslow, 2017). At present, countries are vigorously developing nuclear power plants, and the rapid development of nuclear power will inevitably generate more high-level nuclear waste. For China, it is expected to produce 3,000 m2 high-level nuclear waste by 2030. Therefore, countries around the world have regarded efficient disposal of high-level nuclear waste as an important challenge for the development of the nuclear energy industry (Wang et al., 2004).
Regarding the disposal plan for high-level nuclear waste, researchers have proposed “deep seabed disposal,” “frozen disposal,” “hydraulic cage disposal,” “space disposal” and “deep geological disposal” (Arup, 1985; Milnes, 1985; Pang, 1989; Gao, 1995; Bradley, 1997; Shen, 2002). At present, the widely accepted solution in various countries is the deep geological disposal method, which means that high-level nuclear waste is disposed of in a suitable rock mass storage warehouse at a depth of about 500–1,000 meters from the surface, forming a “multi-barrier system” consisting of solidified high-level nuclear waste, metal container and buffer backfill materials, as well as surrounding rock and its geological environment, forming a natural barrier (SKB, 2001; Wang et al., 2005; Duquette et al., 2009). Metal container is particularly important as the first barrier for nuclear waste. Titanium and its alloys are one of the alternative materials for container due to their excellent corrosion resistance. Canada and Japan have used titanium and its alloys as alternative materials for container and have conducted extensive research (Tsujikawa and Kojima, 1990; He et al., 2002). Currently, the United States plans to use titanium droplet shields on nickel alloy containers at Yucca Mountain (Hua et al., 2005a,b, 2008; Mon, 2008; Rebak, 2009). Carbon steel has also been used as an alternative material for container in Japan, South Korea and Canada due to its advantages of simple processing and low cost (King and Padovani, 2011).
The container corrosion is closely related to the surrounding environment. The factors that affect container corrosion in deep geological environments mainly include oxygen content (partial interception of oxygen by the closed storage), temperature (decay heat release of nuclear waste), radiation (strong radioactivity of nuclear waste), microbial activity, etc. However, the near field environment of the container may change over time, for example, the oxygen content may decrease to anaerobic conditions. Winsley et al. (2011) believe that when the oxygen content around the container decreases to low oxygen concentration environmental conditions, hydrogen evolution corrosion dominates. Long term permeation of hydrogen into the interior of container materials may cause a risk of hydrogen embrittlement (HE). The good corrosion resistance of titanium and its alloys depends on the formation of a stable oxide film on their surface. If exposed to hydrogen environments, titanium is highly susceptible to hydrogen absorption. Dwivedi and Vishwakarma classified titanium as a material susceptible to HE (Dwivedi and Vishwakarma, 2018). Meanwhile, hydrogen will react with titanium and hydrides formed (Qiao et al., 2021). The titanium hydrides show brittleness. Research has shown that hydrogen ions can also be reduced and hydrogen can penetrate into carbon steel under aerobic corrosion conditions (Huang and Zhu, 2005; Tsuru et al., 2005). Therefore, hydrogen evolution corrosion will accompany the whole process of container corrosion. The harm of HE in metal container is far more severe than predictable uniform corrosion, so it is particularly important to reduce the HE sensitivity of metal container materials.
Microbial corrosion of container
The impact of microorganisms on the corrosion behavior of container materials in the deep geological environment is currently a research hotspot. Microbial corrosion refers to the life activities of microorganisms attached to the biofilm on the surface of a material, leading to or promoting the corrosion and destruction of the material. The attachment of biofilms and the presence of metabolites alter the anodic or cathodic reaction kinetics at the metal/solution interface, leading to accelerated corrosion of metal materials (Ornek et al., 2002).
Corrosive microorganisms are often participants in the iron sulfur cycle in the environment (Huang et al., 2017). According to the types and functions of bacteria, they can be divided into sulfate reducing bacteria (SRB), sulfur oxidizing bacteria (SOB), acid producing bacteria (APB), iron oxidizing bacteria (IOB), iron reducing bacteria (IRB), nitrate reducing bacteria (NRB) and slime producing bacteria (SFB).
There are currently several main mechanisms of microbial corrosion.
(1) Cathodic depolarization theory
The cathodic depolarization theory (von Wolzogen and van der Vlugt, 1934) suggests that a “hydrogen film” will adhere to the metal surface in anaerobic environments due to the cathodic hydrogen evolution reaction, ultimately hindering the metal corrosion. This obstruction is often referred to “polarization.” SRB can be adsorbed on metal surfaces and utilize the cathodic hydrogen on the metal surface through hydrogenase in the body to reduce local hydrogen partial pressure. This “depolarization” makes metal corrosion continue. The hydrogen consumption of SRB plays a role in cathodic depolarization. The relevant reactions are as follows:
Anode reaction
Hydrogen depolarization of hydrogenase
Corrosion products
Total reaction
(2) Metabolite corrosion mechanism
Microorganisms have metabolic diversity. Therefore, the metabolites of microorganisms also have diversity, including some metabolites that can promote corrosion. For example, the metabolite H2S of SRB can react quickly with metal iron to generate FeS (Li et al., 2014), accelerating corrosion. The sulfide metabolites of microorganisms can also react with Fe2+ generated during the corrosion process to form a loose iron sulfur (FeSx) composite layer, which has no protective effect on metals compared to a dense FeS protective film (Liu et al., 2009). In addition, the acidic metabolites of acid producing bacteria can reduce the pH value in the environment, which is prone to serious pore corrosion and pore leakage.
(3) Concentration cell mechanism
The growth and metabolism process of microorganisms can establish various concentration batteries on metal surfaces, such as oxygen concentration batteries, metal ion concentration batteries, activation and passivation batteries, etc. The presence of concentration batteries can cause local corrosion of metals. For example, that an oxygen concentration cell with a positive potential in the rich oxygen region as the cathode and a negative potential in the poor oxygen region as the anode is formed because of the differences in oxygen consumption among different bacterial colonies within the biofilm leads to accelerated corrosion in the poor oxygen region (Abdolahi et al., 2014).
(4) Direct or indirect electronic transmission
Some microorganisms can utilize cytochrome C proteins on the surface of cell membranes and biological nanowires to directly obtain electrons from metal surfaces (Dinh et al., 2004; Reguera et al., 2005), thereby accelerating corrosion. In addition, some microorganisms can efficiently indirectly transfer extracellular electrons through reversible redox active electron mediators, For example, the phenazine like substances of Pseudomonas and the flavin like substances secreted by Shewanella (Huang et al., 2017) perform efficient indirect extracellular electron transfer, thereby accelerating metal corrosion.
Overall, there are two types of microbial corrosion in the deep geological environment of nuclear waste container. One is that in the early stages of disposal, there is a high oxygen content in the deep geological environment and aerobic microbial corrosion occurs; One is that in the medium to long term of disposal, the oxygen content decreases to an anaerobic environment, resulting in anaerobic microbial corrosion. Figure 1 shows the variation of microbial corrosion types with oxygen concentration in deep geological environments.
Aerobic conditions will certainly prevail during the repository operating phase. Many aerobic bacteria produce metabolites which can be corrosive for metallic materials in general and steels in particular (Féron and Crusset, 2014). An estimation of corrosion due to ferro-oxidizing bacteria was made on a carbon steel (Pitonzo et al., 2004) exposed to synthetic solutions representative of the waters of Yucca Mountain site (same pH, same redox and same concentration of main ions). Electrochemical measurements showed decreasing corrosion rates with time. After 1 month of testing, the mean rate of corrosion is 58 mm/year (while corrosion rate of around 30 mm/year is found on carbon steel coupons immersed in the same synthetic aqueous solutions without bacteria). Shrestha et al. examined microbial corrosion of carbon steel in synthetic bentonite pore water inoculated with natural underground water containing microorganisms over a period of 780-days under sterile and anaerobic conditions (Shrestha et al., 2021). The results indicate that nitrate-reducing bacteria could represent a potential threat to waste canisters under nuclear repository conditions.
Hydrogen consuming microorganisms reduce hydrogen embrittlement sensitivity
Currently, most research is focused on the issue of microbial accelerated corrosion of nuclear waste container. However, there is little research on whether certain microorganisms exist in deep geological environments that can reduce the corrosion rate of container materials. There is a type of hydrogen consuming microorganism in the natural environment that uses hydrogen oxidation as the energy for its life activities. This provides a new approach for us to study reducing the HE sensitivity of nuclear waste container materials (Figure 2).
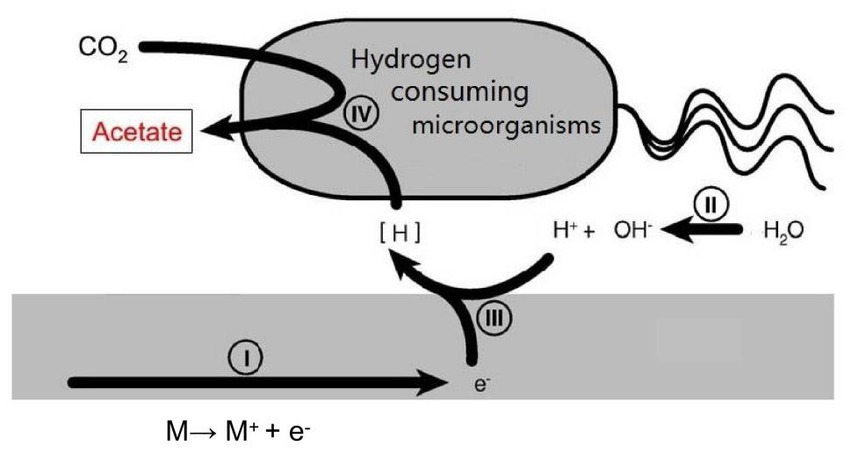
Figure 2. Schematic illustration of the mutual effects between hydrogen consuming organisms and metal surface.
Collet et al. (2005) studied the effect of hydrogen consuming microorganisms on the metabolism of Clostridium thermophilum. Experiments have shown that hydrogen consuming microorganisms such as Methanothermophyter thermophilus and Moorella thermophilica increase acetic acid production by three times. Thermophilic Lactobacillus not only produces acetic acid but also by-products such as hydrogen, carbon dioxide, and lactic acid when metabolizing lactose. Thermophilic autotrophic methane bacteria play an important role as effective hydrogen removal agents, which can significantly reduce the partial pressure of hydrogen in the system and convert hydrogen into methane. Moorella thermautotrophica converts hydrogen, lactic acid, and carbon dioxide into acetic acid. Hoehler et al. (2001) involved a hydrogen consuming microbial methane producing archaea when studying the apparent minimum free energy required by microorganisms in hypoxic marine sediments. Homoacetic acid bacteria are also hydrogen consuming microorganisms, and Kotsyurbenko et al. (2001) studied their competitive behavior with methanogenic archaea for hydrogen in anaerobic environments. Lin et al. (2014) suggests that basalt aquifers on the seafloor can support hydrogen driven ecosystems. Feng et al. (2014) and Zhao (2012) conducted an experimental research on the enhancement of methane production during anaerobic digestion of sludge by adding 0 valent iron. The reason for the enhancement was that hydrogen consuming bacteria utilized hydrogen produced by iron corrosion. Mori et al. (2010) isolated 26 microbial species from petroleum facilities. The hydrogen consuming microorganisms do not have a strong promoting effect on the corrosion of iron, while the other non hydrogen consuming microorganisms cause severe corrosion of iron. These are the only literature found on hydrogen consuming microbial corrosion. Nybo et al. (2015) reviewed the use of chemotrophic autotrophic microorganisms to immobilize greenhouse gasses in the production of fuels and chemical products, which also require hydrogen consumption. There are also literature (Giblin et al., 2000; Libert et al., 2011) on the research of microbial engineering using hydrogen consuming microorganisms. It can be seen that hydrogen consuming microorganisms are widely present in nature. If the hydrogen consumption effect of these hydrogen consuming microorganisms can be utilized to consume the hydrogen generated during natural corrosion processes and reduce the diffusion of hydrogen into the metal interior, the HE possibility in metal materials can be reduced. Although microorganisms do not have a significant impact on the corrosion behavior of titanium and its alloys, if hydrogen consuming microorganisms in deep geological environments can consume excess hydrogen atoms on their surfaces, this will reduce the combination hydride of hydrogen and titanium, which is the main cause of HE in titanium and its alloys.
Conclusion
In summary, the cathodic reaction of corrosion has shifted from oxygen absorption corrosion to hydrogen evolution corrosion due to the continuous consumption of oxygen, so there is a possibility of long-term HE in metal container during nuclear waste disposal. Unpredictable HE often has a more severe impact on nuclear waste container materials than predictable uniform corrosion. Hydrogen consuming microorganisms exist in deep geological environments, which use hydrogen oxidation as their energy for life activities. If these hydrogen consuming microorganisms can consume the hydrogen atoms generated during the natural corrosion process of the container material, thereby preventing hydrogen from permeation into the interior of the container material, greatly reducing the possibility of HE. However, most current research has focused on microbial accelerated corrosion rate of container materials in deep geological environments, only considering the adverse factors of microorganisms. However, the presence of hydrogen consuming microorganisms provides a new approach for studying the reduction of HE sensitivity for nuclear waste container materials.
Author contributions
QZ: Writing – original draft. YJ: Funding acquisition, Supervision, Writing – review & editing. XZ: Supervision, Writing – review & editing. JD: Investigation, Supervision, Writing – review & editing. LC: Writing – review & editing. YX: Writing – review & editing.
Funding
The author(s) declare financial support was received for the research, authorship, and/or publication of this article. This work was financially supported by the Natural Science Foundation of Shandong Province under grant no. ZR2022QE131.
Conflict of interest
The authors declare that the research was conducted in the absence of any commercial or financial relationships that could be construed as a potential conflict of interest.
Publisher’s note
All claims expressed in this article are solely those of the authors and do not necessarily represent those of their affiliated organizations, or those of the publisher, the editors and the reviewers. Any product that may be evaluated in this article, or claim that may be made by its manufacturer, is not guaranteed or endorsed by the publisher.
References
Abdolahi, A., Hamzah, E., Ibrahim, Z., and Hashim, S. (2014). Microbially influenced corrosion of steels by Pseudomonas aeruginosa. Corros. Rev. 32, 129–141. doi: 10.1515/corrrev-2013-0047
Arup, O. (1985). Ocean disposal of radioactive waste by penetrator emplacement. Graham & Trotman Ltd.. London
Bradley, D. J. (1997). Behind the nuclear curtain: radioactive waste management in the former soviet union. Phys. Today 51:63. doi: 10.1063/1.882248
Collet, C., Gaudard, O., Péringer, P., and Schwitzguébel, J. P. (2005). Acetate production from lactose by Clostridium thermolacticum and hydrogen-scavenging microorganisms in continuous culture—effect of hydrogen partial pressure. J. Biotechnol. 118, 328–338. doi: 10.1016/j.jbiotec.2005.05.011
Dinh, H. T., Kuever, J., Mußmann, M., Hassel, A. W., Stratmann, M., and Widdel, F. (2004). Iron corrosion by novel anaerobic microorganisms. Nature 427, 829–832. doi: 10.1038/nature02321
Duquette, D. J., Latanision, R. M., di Bella, C. A. W., and Kirstein, B. E. (2009). Corrosion issues related to disposal of high-level nuclear waste in the yucca mountain repository-peer reviewer’s perspective. Corrosion 65, 272–280. doi: 10.5006/1.3319133
Dwivedi, S. K., and Vishwakarma, M. (2018). Hydrogen embrittlement in different materials: a review. Int. J. Hydrog. Energy 43, 21603–21616. doi: 10.1016/j.ijhydene.2018.09.201
Feng, Y., Zhang, Y., Quan, X., and Chen, S. (2014). Enhanced anaerobic digestion of waste activated sludge digestion by the addition of zero valent iron. Water Res. 52, 242–250. doi: 10.1016/j.watres.2013.10.072
Féron, D., and Crusset, D. (2014). Microbial induced corrosion in French concept of nuclear waste underground disposal. Corros. Eng. Sci. 49, 540–547. doi: 10.1179/1743278214Y.0000000193
Giblin, T. L., Herman, D. C., and Frankenberger, W. T. (2000). Removal of Perchiorate from ground water by hydrogen-utilizing Bacteria. J. Environ. Qual. 29, 1057–1062. doi: 10.2134/jeq2000.00472425002900040004x
He, X., Noël, J. J., and Shoesmith, D. W. (2002). Temperature dependence of crevice corrosion initiation on titanium grade-2. J. Electrochem. Soc. 149, B440–B449. doi: 10.1149/1.1499501
Hoehler, T. M., Alperin, M. J., Albert, D. B., and Martens, C. S. (2001). Apparent minimum free energy requirements for methanogenic Archaea and sulfate-reducing bacteria in an anoxic marine sediment. FEMS Microbiol. Ecol. 38, 33–41. doi: 10.1111/j.1574-6941.2001.tb00879.x
Hua, F., De, G. C., and Gordon, G. M., (2005a). Hydrogen induced cracking of titanium alloys in environments anticipated in Yucca Mountain nuclear waste repository. Houston, Texas: Paper presented at the corrosion.
Hua, F., Mon, K., De, G., Gordon, G., and Andresen, P. L. (2008). The potential for the SCC of titanium alloys under repository-relevant environments for US nuclear waste. JOM 60, 66–72. doi: 10.1007/s11837-008-0010-6
Hua, F., Mon, K., Pasupathi, P., Gordon, G., and Shoesmith, D. (2005b). A review of corrosion of titanium grade 7 and other titanium alloys in nuclear waste repository environments. Corrosion 61, 987–1003. doi: 10.5006/1.3280899
Huang, Y., Liu, S. J., and Jiang, C. Y. (2017). Microbiologically influenced corrosion and mechanisms. J. Microbiol. 44, 1699–1713.
Huang, Y. L., and Zhu, Y. Y. (2005). Hydrogen ion reduction in the process of iron rusting. Corros. Sci. 47, 1545–1554. doi: 10.1016/j.corsci.2004.07.044
King, F., and Padovani, C. (2011). Review of the corrosion performance of selected canister materials for disposal of UK HLW and/or spent fuel. Corros. Eng. Sci. Techn. 46, 82–90. doi: 10.1179/1743278211Y.0000000005
Kotsyurbenko, O. R., Glagolev, M. V., Nozhevnikova, A. N., and Conrad, R. (2001). Competition between homoacetogenic bacteria and methanogenic archaea for hydrogen at low temperature. FEMS Microbiol. Ecol. 38, 153–159. doi: 10.1111/j.1574-6941.2001.tb00893.x
Li, D. P., Zhang, L., Yang, J. W., Lu, M. X., Ding, J. H., and Liu, M. L. (2014). Effect of H2S concentration on the corrosion behavior of pipeline steel under the coexistence of H2S and CO2. Int. J. Miner. Metall. 21, 388–394. doi: 10.1007/s12613-014-0920-y
Libert, M., Bildstein, O., Esnault, L., Jullien, M., and Sellier, R. (2011). Molecular hydrogen: an abundant energy source for bacterial activity in nuclear waste repositories. Phys. Chem. Earth 36, 1616–1623. doi: 10.1016/j.pce.2011.10.010
Lin, H. T., Cowen, J. P., Olson, E. J., Lilley, M. D., Jungbluth, S. P., Wilson, S. T., et al. (2014). Dissolved hydrogen and methane in the oceanic basaltic biosphere. Earth Planet. Sci. Lett. 405, 62–73. doi: 10.1016/j.epsl.2014.07.037
Liu, T., Liu, H., Hu, Y., Zhou, L., and Zheng, B. (2009). Growth characteristics of thermophile sulfate-reducing bacteria and its effect on carbon steel. Mater. Corros. 60, 218–224. doi: 10.1002/maco.200805063
Lukens, W. W., and Saslow, S. A. (2017). Aqueous synthesis of technetium-doped titanium dioxide by direct oxidation of titanium powder, a precursor for ceramic nuclear waste forms. Chem. Mater. 29, 10369–10376. doi: 10.1021/acs.chemmater.7b03567
Mon, K. G. (2008). “Potential SCC initiation and propagation in titanium alloys under U.S. nuclear waste repository environmental conditions.” 17th International Corrosion Congress. Las Vegas, Nevada.
Mori, K., Tsurumaru, H., and Harayama, S. (2010). Iron corrosion activity of anaerobic hydrogen-consuming microorganisms isolated from oil facilities. J. Biosci. Bioeng. 110, 426–430. doi: 10.1016/j.jbiosc.2010.04.012
Nybo, S. E., Khan, N. E., Woolston, B. M., and Curtis, W. R. (2015). Metabolic engineering in chemolithoautotrophic hosts for the production of fuels and chemicals. Metab. Eng. 30, 105–120. doi: 10.1016/j.ymben.2015.04.008
Ornek, D., Wood, T. K., Hsu, C., Sun, Z., and Mansfeld, F. (2002). Pitting corrosion control of aluminum 2024 using protective biofilms that secrete corrosion inhibitors. Corrosion 58, 761–767. doi: 10.5006/1.3277659
Pang, W. K. (1989). Introduction of nuclear waste treatment method. Geol. Sci. Technol. Inf. 1, 143–148.
Pitonzo, B. J., Castro, P., Amy, P. S., Southam, G., Jones, D. A., and Ringelberg, D. (2004). Microbiologically influenced corrosion capability of bacteria isolated from Yucca mountain. Corrosion 60, 64–74. doi: 10.5006/1.3299233
Qiao, Y., Xu, D., Wang, S., Ma, Y., Chen, J., Wang, Y., et al. (2021). Effect of hydrogen charging on microstructural evolution and corrosion behavior of Ti-4Al-2V-1Mo-1Fe alloy. J. Mater. Sci. Technol. 60, 168–176. doi: 10.1016/j.jmst.2020.06.010
Rebak, R. B. (2009). Corrosion testing of nickel and titanium alloys for nuclear waste disposition. Corrosion 65, 252–271. doi: 10.5006/1.3319132
Reguera, G., McCarthy, K. D., Mehta, T., Nicoll, J. S., Tuominen, M. T., and Lovley, D. R. (2005). Extracellular electron transfer via microbial nanowires. Nature 435, 1098–1101. doi: 10.1038/nature03661
Shrestha, R., Černoušek, T., Stoulil, J., Kovářová, H., Sihelská, K., Špánek, R., et al. (2021). Anaerobic microbial corrosion of carbon steel under conditions relevant for deep geological repository of nuclear waste. Sci. Total Environ. 800:149539. doi: 10.1016/j.scitotenv.2021.149539
Tsujikawa, S., and Kojima, Y. (1990). Repassivation method to predict long term integrity of low alloy titanium for nuclear waste package. Mater. Res. Soc. symp. proc. 212, 261–268. doi: 10.1557/PROC-212-261
Tsuru, T., Huang, Y. L., Ali, M. R., and Nishikata, A. (2005). Hydrogen entry into steel during atmospheric corrosion process. Corros. Sci. 47, 2431–2440. doi: 10.1016/j.corsci.2004.10.006
von Wolzogen, K. C. A. H., and van der Vlugt, I. S. (1934). The graphitization of cast iron as an electrobiochemical process in anaerobic soils. Water 1934, 147–165.
Wang, J., Fan, X. H., and Xu, G. G.. (2004). Ten years progress of geological disposal of high level radioactive waste in China. Beijing: Atomic Energy Press.
Wang, J., Xu, G. Q., and Fang, H. L. (2005). Geological disposal of high level radioactive waste in China: Progress during 1985-2004. World Nucl. Geosci. 22, 5–16.
Winsley, R. J., Smart, N. R., Rance, A. P., Fennell, P. A. H., Reddy, B., and Kursten, B. (2011). Further studies on the effect of irradiation on the corrosion of carbon steel in alkaline media. Corros. Eng. Sci. Techn. 46, 111–116. doi: 10.1179/1743278210Y.0000000010
Keywords: microbial corrosion, hydrogen embrittlement, hydrogen consuming microorganisms, deep geological disposal, nuclear waste
Citation: Zhang Q, Jiang Y, Zhao X, Duan J, Chen L and Xu Y (2023) A new research proposal to prevent hydrogen embrittlement for nuclear waste container by bacteria-a mini review. Front. Microbiol. 14:1304703. doi: 10.3389/fmicb.2023.1304703
Edited by:
Yizhi Sheng, China University of Geosciences, ChinaReviewed by:
Long Hao, Corrosion and Protection Center of Materials, Institute of Metal Research, Chinese Academy of Sciences, ChinaQiao Yanxin, Jiangsu University of Science and Technology, China
Copyright © 2023 Zhang, Jiang, Zhao, Duan, Chen and Xu. This is an open-access article distributed under the terms of the Creative Commons Attribution License (CC BY). The use, distribution or reproduction in other forums is permitted, provided the original author(s) and the copyright owner(s) are credited and that the original publication in this journal is cited, in accordance with accepted academic practice. No use, distribution or reproduction is permitted which does not comply with these terms.
*Correspondence: Yishan Jiang, anlzMTMwQDEyNi5jb20=; Xin Zhao, MTMxNTYyMzk1NzFAMTYzLmNvbQ==