- 1Institute of Jeju Microbial Resources, BioPS Co., Ltd., Jeju, Republic of Korea
- 2Department of Biological Sciences and Biotechnology, Hannam University, Daejon, Republic of Korea
- 3BioPS Co., Ltd., Daejeon, Republic of Korea
The taxonomic relationships of 10 strains isolated from seaweeds collected from two beaches in Republic of Korea were studied by sequencing and analyses of 16S rRNA genes and whole genomes. For the construction of a more reliable and robust 16S rRNA gene phylogeny, the authentic and nearly complete 16S rRNA gene sequences of all the Microbacterium type strains were selected through pairwise comparison of the sequences contained in several public databases including the List of Prokaryotic names with Standing in Nomenclature (LPSN). The clustering of the ten study strains into five distinct groups was apparent in this single gene-based phylogenetic tree. In addition, the 16S rRNA gene sequences of a few type strains were shown to be incorrectly listed in LPSN. An overall phylogenomic clustering of the genus Microbacterium was performed with a total of 113 genomes by core genome analysis. As a result, nine major (≥ three type strains) and eight minor (two type strains) clusters were defined mostly at gene support index of 92 and mean intra-cluster OrthoANIu of >80.00%. All of the study strains were assigned to a Microbacterium liquefaciens clade and distributed further into four subclusters in the core genome-based phylogenetic tree. In vitro phenotypic assays for physiological, biochemical, and chemotaxonomic characteristics were also carried out with the ten study strains and seven closely related type strains. Comparison of the overall genomic relatedness indices (OGRI) including OrthoANIu and digital DNA–DNA hybridization supported that the study strains constituted four new species of the genus Microbacterium. In addition, some Microbacterium type strains were reclassified as members of preexisting species. Moreover, some of them were embedded in a new genus of the family Microbacteriaceae based on their distinct separation in the core genome-based phylogenetic tree and amino acid identity matrices. Based on the results here, four new species, namely, Microbacterium aurugineum sp. nov., Microbacterium croceum sp. nov., Microbacterium galbinum sp. nov., and Microbacterium sufflavum sp. nov., are described, along with the proposal of Paramicrobacterium gen. nov. containing five reclassified Microbacterium species from the “Microbacterium agarici clade”, with Paramicrobacterium agarici gen. nov., comb. nov. as the type species.
Introduction
The genus Microbacterium Orla-Jensen 1919 (Approved Lists, 1980) emend. Fidalgo et al. (2016) is the type genus of the family Microbacteriaceae Park et al., 1995 emend. Zhi et al. (2009) of the order Microbacteriales (Salam et al., 2020) and currently contains >130 species with validly published names (https://lpsn.dsmz.de/genus/microbacterium). The genus forms a monophyletic taxon in the phylogenetic trees of the family Microbacteriaceae based on the sequences of 16S rRNA genes (Evtushenko, 2012) and housekeeping genes (Richert et al., 2007) but is paraphyletic in whole-genome-based phylogeny (Nouioui et al., 2018). Members of the genus contain different types of peptidoglycan with D-ornithine, L-lysine, or diaminobutyric acid as the diamino acid of the cell wall peptidoglycan (Suzuki and Hamada, 2012; Fidalgo et al., 2016) and can be readily differentiated from other genera of the family Microbacteriaceae using a combination of morphological and chemotaxonomic features (Evtushenko, 2012) as well as genome-based phylogeny (Nouioui et al., 2018). The intra-genus phylogenomic relationships have been partly studied, for < 50 species, through the descriptions of new species (Dong et al., 2020; Bellassi et al., 2021; Tian et al., 2021; Xie et al., 2021) or in the whole-genome-based taxonomic classification of the phylum Actinobacteria (Nouioui et al., 2018). In the recent descriptions of two new species from larvae of insects (Lee and Kim, 2023), the genomes of 81 Microbacterium strains were included in a core genome-based phylogeny.
Members of the genus are widely distributed in diverse environments such as soil, sediment or activated sludge, clinical specimens, plants, freshwater, and seawater (Suzuki and Hamada, 2012). Some species have been isolated from animal feces (Anandham et al., 2011; Dong et al., 2020), plants (Suzuki and Hamada, 2012; Chen et al., 2020), and insects (Steinhaus, 1941; Lysenko, 1959; Heo et al., 2020; Lee and Kim, 2023). About 20% of Microbacterium species have been isolated from marine habitats: nine strains from sediments (Suzuki and Hamada, 2012; Yu et al., 2013; Zhang et al., 2014; Mawlankar et al., 2015; Yan et al., 2015; Xie et al., 2021), six from halophyte plants (Alves et al., 2014; Fidalgo et al., 2016; Li et al., 2018; Zhu et al., 2019, 2021), five from seawater (Suzuki and Hamada, 2012; Zhang et al., 2012; Xie et al., 2021), four from marine invertebrates (Kämpfer et al., 2011; Kim et al., 2011; Suzuki and Hamada, 2012; Kaur et al., 2016), and one from marine ascidian (Suzuki and Hamada, 2012). No Microbacterium species has been reported to date as isolated from seaweeds.
The aim of this study was to determine the taxonomic relationships of bacterial strains isolated from seaweeds using 16S rRNA gene sequencing and analysis. For an establishment of more reliable and robust 16S rRNA gene phylogeny, the authentic and nearly complete 16S rRNA gene sequences were selected through comparison of the sequences that have been deposited in several public databases for each Microbacterium type strain. Whole-genome sequences were obtained for the ten study strains, including Microbacterium aurantiacum KACC 20510T, Microbacterium luteolum KACC 14465T, Microbacterium kitamiense KACC 20514T, and Microbacterium imperiale KACC 11896T, and analyzed using a combination of the overall genomic relatedness indices (OGRI) and core genome analyses. For these, the 103 genome sequences available for the genus Microbacterium (96 type and seven non-type strains) were included in the construction of a core genome-based phylogenomic tree, and the overall phylogenomic clustering of the genus was performed. Moreover, all the Microbacterium type strains were compared to evaluate whether they are maintained as separate species using the OGRI including the OrthoANIu, digital DNA–DNA hybridization (dDDH), and amino acid identity (AAI).
Materials and methods
Bacterial isolation and maintenance
Ten bacterial strains were isolated from seaweeds collected from Gwakji Beach (33°27′02 N 126°18′14 E) and Samyang Beach (33°31'34“ N 126°35'11” E) in Jeju, Republic of Korea, in 2003 and 2004, respectively. For bacterial isolation, pieces of dried seaweeds were transferred directly onto WAT-SW agar plates (Lee, 2007). Colonies on the plates, incubated at 30°C for 14 days, were subcultured on TSA-SW medium [trypticase soy agar (TSA; Difco) in a mixture of 60% (v/v) natural seawater and 40% (v/v) distilled water]. The pure cultures were maintained in 20% glycerol suspensions supplemented with 60% natural sea water at −20°C and −80°C. The bacterial strains used in this study are listed in Table 1. The reference type strains for phenotypic analyses were obtained from the Korean Agricultural Culture collection (KACC; 11 strains) and German Collection of Microorganisms and Cell Cultures GmbH (DSM; one strain).
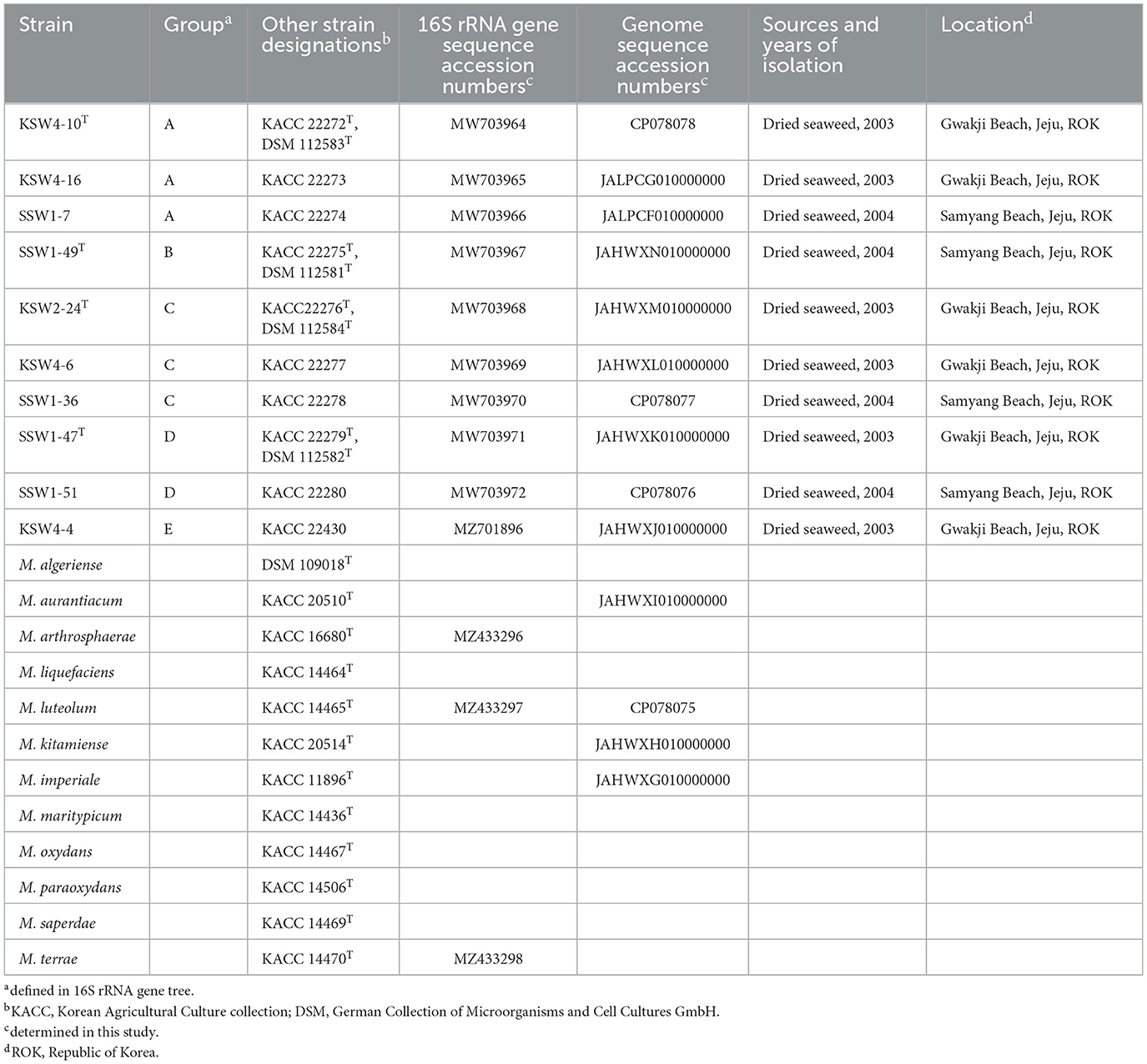
Table 1. List of bacterial isolates and the Microbacterium type strains used in this study, and the accession numbers of their 16S rRNA gene and genome sequences.
16S rRNA gene sequencing and analysis
For sequencing of 16S rRNA genes, the 10 study strains were grown on marine agar at 30°C for 3 days, and Microbacterium arthrosphaerae KACC 16680T, M. luteolum KACC 14465T, and Microbacterium terrae KACC 14470T were cultivated on trypticase soy agar (Difco) at 30°C for 3 days. Genomic DNA isolation was performed as described previously (Lee et al., 2020). The PCR amplification and sequencing of the 16S rRNA genes were performed using an ABI Prism 3730XL DNA analyzer with a BigDye Terminator kit v.3.1 (Invitrogen, USA) at the SolGent Company Limited (Deajon, Korea). Multiple alignments of the sequences were performed using CLUSTAL_X (Thompson et al., 1997). 16S rRNA gene-based phylogenetic analyses were performed using the neighbor-joining algorithm contained in the PHYLIP (v 3.68) for 1,329 nucleotide positions. Evolutionary distances were calculated using the Jukes–Cantor model (Jukes and Cantor, 1969). Bootstrap analysis (Felsenstein, 1985) was based on 1,000 replicates.
Quality check of 16S rRNA gene sequences
Many of Microbacterium type strains contain one or more 16S rRNA gene sequences in the NCBI database, one of which is also listed in the List of Prokaryotic names with Standing in Nomenclature (LPSN; https://lpsn.dsmz.de/genus/Microbacterium) or EzBioCloud server (http://www.ezbiocloud.net; Yoon et al., 2017a). To find authentic and near full-length sequences, the 16S rRNA gene sequences of each type strain were compared with one another and further with genome-derived sequences, if available. The alignment of the sequences was manually optimized according to the secondary structure of Escherichia coli 16S rRNA (Brosius et al., 1978). The 16S rRNA gene sequence similarity was manually calculated after the gaps or nucleotides present only in one sequence were removed using BioEdit ver 7.7.1 or determined using the EzBioCloud server (https://www.ezbiocloud.net/identify).
Genome sequencing and phylogenomic analysis
The whole genomes of the 10 study strains, together with M. aurantiacum KACC 20510T, M. luteolum KACC 14465T, M. kitamiense KACC 20514T, and M. imperiale KACC 11896T (Table 1), were sequenced using the Illumina HiSeq platform. Raw sequence reads were quality-filtered and de novo assembled using SPAdes (http://cab.spbu.ru/software/spades/). To check their authenticity, the genome-derived 16S rRNA gene sequences were compared with the corresponding ones determined by Sanger method. The CheckM (Parks et al., 2014) was used in checking the completeness and contamination of the genomes. For phylogenomic analysis, the genome sequences available for the Microbacterium type strains were retrieved from the NCBI database. For non-type strains, the only genome sequences that were confirmed for their identity were included in this study. The phylogenomic tree was reconstructed using the Up to date Bacterial Core Gene (UBCG) pipeline (https://www.ezbiocloud.net/tools/ubcg; Na et al., 2018) based on 92 bacterial core genes present as a single copy in all the genomes. The reliability of the tree branches was evaluated using gene support index (GSI).
OGRI analyses
As one of thresholds for species delineation, the OrthoANIu values were calculated using the ANI Calculator (https://www.ezbiocloud.net/tools/ani; Yoon et al., 2017b). The dDDH values were calculated using the Genome-to-Genome Distance Calculator with built-in settings (GGDC 2.1) (Meier-Kolthoff et al., 2013). The AAI values as a threshold for genus delineation were determined using the Kostas lab AAI calculator web server (http://enve-omics.ce.gatech.edu/aai/; Rodriguez and Konstantinidis, 2014).
Phenotypic characterization
A set of physiological and biochemical tests were carried out with the 10 study strains and seven closely related type strains (Microbacterium algeriense DSM 109018T, Microbacterium liquefaciens KACC 14464T, M. luteolum KACC 14465T, Microbacterium maritypicum KACC 14436T, Microbacterium oxydans KACC 14467T, Microbacterium paraoxydans KACC 14506T, and Microbacterium saperdae KACC 14469T) using traditional methods and commercial kits such as API systems (API 20NE, API ZYM, and AMI 50CH) as reported previously (Lee and Kim, 2023), the categories of which included acid production from substrates, enzyme activity, carbon source assimilation, pH and temperature ranges for growth, NaCl tolerance, esculin degradation, glucose fermentation, and indole production. The growth and cultural characteristics for the ten study strains also were checked using marine agar (MA; BD), nutrient agar (NA; BD), R2A agar (BD), and trypticase soy agar (TSA; BD). Cell morphology and motility, catalase and oxidase activities, and Gram staining were examined as described previously (Lee and Kim, 2023). The diamino acid of the cell wall peptidoglycan was determined by reverse-phase HPLC (Waters 2690) of the derivatized amino acid with AccQ-Fluor Reagent (Waters) according to the instructions of the manufacturer, with the purified cell walls (Lee, 2007). The N-glycolylated muramic acid in cell wall peptidoglycan was determined by a colorimetric method (Uchida and Aida, 1977). The preparation of isoprenoid quinones and polar lipids was performed by the integrated procedure of Minnikin et al. (1984). Analysis of isoprenoid quinones was performed using HPLC as described previously (Kroppenstedt, 1985). Analysis of polar lipids was performed by two-dimensional thin-layer chromatography on silica gel G60 plates (Merck) as previously described (Minnikin et al., 1977). For fatty acid analysis, the 10 study strains and seven closely related type strains were grown on trypticase soy agar (Difco) for 1–2 days at 30°C. The cellular fatty acids were extracted, methylated, and analyzed following the instructions of Microbial Identification System (Sherlock v.6.1) with TSBA6 of the MIDI database. The DNA G+C contents were determined using genome sequencing platform.
Results
Identification of authentic 16S rRNA gene sequences
Before the 16S rRNA gene analysis, the sequences available for each Microbacterium type strain were retrieved from public databases such as the NCBI, LPSN, and EzBioCloud and checked by pairwise comparison. As a result, the 16S rRNA gene sequences of some type strains were found to be incorrectly listed in the LPSN, in contrast to their original descriptions (Yokota et al., 1993a; Takeuchi and Hatano, 1998b; Zlamala et al., 2002; Schippers et al., 2005; Kämpfer et al., 2011; Anand et al., 2012) (Supplementary Table S1).
Microbacterium aerolatum (Zlamala et al., 2002) is represented by two 16S rRNA sequences in the NCBI: AJ309929, in its original description and also listed in the LPSN, and MT760116 (M. aerolatum CCM 4955T). The former sequence shares sequence similarity of 99.71% (4 nt differences) with the genome-derived sequence (BJUW01000027) of M. aerolatum NBRC 103071T, while it showed sequence similarity of 98.58% (19 nt differences) with the MT760116 sequence, which is the same as the genome-derived sequence (BJML01000022) of Microbacterium testaceum NBRC 12675T. On the other hand, the genome-derived 16S rRNA gene sequence (BMCD01000006) of M. aerolatum CCM 4955T is the same as the genome-derived sequence (BJUW01000027) of M. aerolatum NBRC 103071T. These results indicate that the MT760116 (M. aerolatum CCM 4955T) is not suitable as a reference sequence of M. aerolatum.
Microbacterium amylolyticum N5T (Anand et al., 2012) is represented by two 16S rRNA sequences in the NCBI: HQ605925 in its original description and MT760185 (M. amylolyticum CCM 7881T, also listed in the LPSN). The former sequence shares 100% sequence identity with the genome-derived sequence (CP049253) of M. amylolyticum DSM 24221T, whereas it shows sequence similarity of 94.84% with the latter sequence (MT760185), which is the same as that (FN870023) given in the original description of M. arthrosphaerae CC-VM-YT (Kämpfer et al., 2011). These results show that the MT760185 (M. amylolyticum CCM 7881T) sequence listed in LPSN should not be used as a reference sequence of M. amylolyticum.
The 16S rRNA gene sequence (FN870023) of M. arthrosphaerae CC-VM-YT (Kämpfer et al., 2011), which is very short at the 3′-end, showed low sequence similarity (97.89%; 27 nt differences) with the MT760166 sequence (from M. arthrosphaerae CCM 7681T) listed in LPSN, sharing 100% identity with that (HE585693) given in the original description of Microbacterium murale I-Gi-001T (Kämpfer et al., 2012). The genome sequence of the type strain has not been determined yet. Therefore, we also determined the 16S rRNA gene sequence (MZ433296) of M. arthrosphaerae KACC 16680T, revealing that it was in agreement with the original one. These results show that the MT760166 (M. arthrosphaerae CCM 7681T) sequence listed in LPSN should not be used as a reference sequence of M. arthrosphaerae.
The original description of Microbacterium aurum H-5T did not contain information for its 16S rRNA gene sequence (Yokota et al., 1993a) but is currently represented by the four sequences of the type strain in the NCBI: AB007418 and D21340 (M. aurum IFO 15204T), Y17229 (M. aurum DSM 8600T, also listed in LPSN), and MW227637 (M. aurum KACC 15219T). Among them, the Y17229 shares sequence similarity of 99.72% (4 nt differences) with the genome-derived sequence (CP018762) of M. aurum KACC 15219T and was shown as the nearly complete 16S rRNA gene sequence of M. aurum. The other two sequences (AB007418 and D21340), which are short and incomplete at the 5′-end, revealed low sequence similarity (95.49% and 98.48%, respectively) with the genome-derived one (CP018762). Moreover, the fourth sequence (MW227637) also indicated low sequence similarity (98.67%; 19 nt differences) with the genome-derived one (CP018762), despite being determined from the same strain (KACC 15219T). These results show that the genome-derived 16S rRNA gene sequence is the more suitable reference sequence for M. aurum.
The original description of Microbacterium dextranolyticum (Yokota et al., 1993a) did not contain a 16S rRNA gene sequence. Currently, three 16S rRNA gene sequences are included in the NCBI: D21341 and AB007417 (M. dextranolyticum IFO 14592T) and Y17230 (M. dextranolyticum DSM 8607T, also listed in LPSN). The genome-derived 16S rRNA gene sequence (JAFBBR010000001) of M. dextranolyticum DSM 8607T shared sequence similarity of 99.72% (4 nt differences) with the Y17230, while it showed low sequence similarities with the AB007417 (99.51%; 7 nt differences) and D21341 (99.11%; 11 nt differences) sequences. These results show that genome-derived 16S rRNA gene sequence is the more suitable reference sequence for M. dextranolyticum.
The 16S rRNA gene sequence (HM222660) of Microbacterium hydrothermale 0704CP-2T (Zhang et al., 2014) revealed sequence similarity of 99.39% (9 nt differences) with the genome-derived one (JAODLQ010000012) recently available from the same type strain. This result suggests that the genome-derived 16S rRNA gene sequence is the better reference sequence for M. hydrothermale.
Microbacterium rhizosphaerae CHO1T (Cho and Lee, 2017) is currently represented by two 16S rRNA sequences in the NCBI: KP722591 from the original description (also listed in LPSN) and LT593972. The former sequence, which is unstable before position 190 (E. coli numbering), showed sequence similarity of 98.50% (21 nt differences) with the latter (LT593972). These results show that the LT593972 rather than the KP722591 is the more suitable reference sequence of M. rhizosphaerae.
The 16S rRNA gene sequence (MF084212) of Microbacterium suaedae YZYP 306T (Zhu et al., 2019) revealed sequence similarity of 99.21% (12 nt differences) with the genome-derived one (PNFD01000009) obtained from the same type strain, suggesting that the latter sequence is the more suitable reference one of M. suaedae.
The 16S rRNA gene sequence (X77445) of M. testaceum DSM 20166T (Takeuchi and Hatano, 1998b), which is also listed in LPSN, is the same as the genome-derived sequence (BJML01000022) of M. testaceum NBRC 12675T, while it revealed very low sequence similarity (94.80%) with the MT760091 sequence (M. testaceum CCM 2299T) which is the same as that (HQ605295) given in the original description of M. amylolyticum N5T (Anand et al., 2012). These results indicate that the MT760091 (M. testaceum CCM 2299T) is not suitable as a reference sequence of M. testaceum.
In addition, many type strains of Microbacterium species contained slightly inaccurate 16S rRNA gene sequences, which showed differences of one to seven nucleotides depending on the type strains, as compared with genome-derived ones (Supplementary Table S1). These results indicate that for unraveling the more coherent phylogenetic relationship of the genus Microbacterium using 16S rRNA gene phylogeny, it can be better to use genome-derived 16S rRNA gene sequences, if available.
16S rRNA gene phylogeny
A phylogenetic analysis was performed with the 16S rRNA gene sequences of the ten study strains (Table 1) and three Microbacterium type strains determined in this study and those of other 129 Microbacterium species. The 16S rRNA gene tree (Figure 1) showed that all the study strains were closely related to the type strains of seven Microbacterium species (M. algeriense, M. liquefaciens, M. maritypicum, M. luteolum M. oxydans, M. paraoxydans, and M. saperdae), most of which have been isolated from various habitats different from the marine environments selected in this study. The study strains were distributed into five groups: Group A contained strains KSW4-10T, KSW4-16, and SSW1-7 (with 100% sequence identity to one another) and was positioned between M. algeriense, isolated from oil production waters (Lenchi et al., 2020), and a clade including M. liquefaciens isolated from dairy products (Collins et al., 1983; Takeuchi and Hatano, 1998b), M. maritypicum from sea water and marine mud (ZoBell and Upham, 1944; Takeuchi and Hatano, 1998a), and M. oxydans from hospital materials (Chatelain and Second, 1966; Schumann et al., 1999); Group B consisted of only one isolate, SSW1-49T, and occupied a position between M. saperdae from dead larvae of an insect (Lysenko, 1959; Takeuchi and Hatano, 1998b) and M. algeriense; Group C encompassed strains KSW2-24T, KSW4-6, and SSW1-36 (intra-group sequence identity of 100%) and formed a distinct clade between M. luteolum from soil (Yokota et al., 1993b; Takeuchi and Hatano, 1998b) and M. saperdae; and Group D contained strains SSW1-47T and SSW1-51 (with 100% sequence identity and the same origin of isolation) and were closely related to Group E encompassing strain KSW4-4 and M. paraoxydans isolated from human blood (Laffineur et al., 2003), sharing 100% sequence identity to each other (Figure 1, Supplementary Table S2).
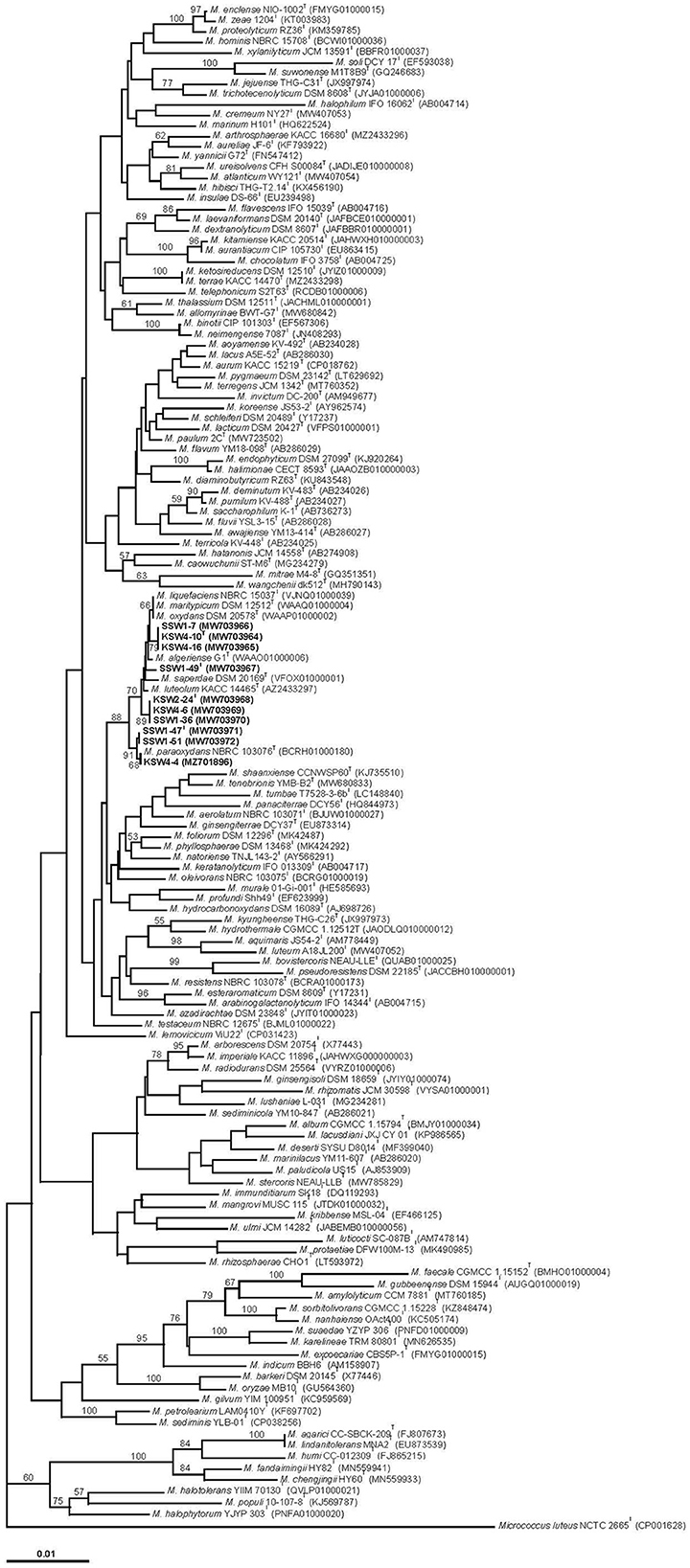
Figure 1. Neighbor-joining phylogenetic tree based on 16S rRNA gene sequences showing the relationships between the 10 study strains and 132 Microbacterium type strains. Distances were calculated with the Juke-Cantor model. The tree is based on 1,329 nt. Bootstrap support values >50% (1,000 resamplings) are shown at branches. Bar, 0.01 substitutions per nucleotide position.
Inter-group sequence similarities of the study strains ranged from 99.43% (8 nt differences) to 99.86% (2 nt differences), while the 16S rRNA gene sequence similarity values between the study strains and seven closely related type strains were 99.36% (9 nt differences)−100%. On the other hand, the above type strains showed sequence similarities between 99.38% (9 nt differences) and 100% to one another. Among them, the type strains of M. liquefaciens, M. maritypicum, and M. oxydans shared 100% sequence identity to one another and also showed high sequence similarity values (99.79–99.87%; 2–3 nt differences) with M. algeriense, M. luteolum, and M. saperdae (Supplementary Table S2). As visualized by very short lengths of branches in the 16S rRNA gene tree (Figure 1), these results indicate that the study strains and reference type strains have very close phylogenetic relationships with one another.
In addition, several pairs of Microbacterium species were also found to have very short lengths of branches in the 16S rRNA gene tree (Figure 1), with high 16S RNA gene sequence similarity (99.50–100%) for each pair. These species pairs are discussed in detail below.
UBCG phylogenomic analysis
The phylogenomic analysis was carried out based on 92 bacterial core gene sequences using the UBCG pipeline (Na et al., 2018), with the genomes of the ten study strains and 103 Microbacterium strains including 96 type and seven non-type strains. In the core genome-based phylogenetic tree (Figure 2), all the Microbacterium strains were resolved into nine major clusters (clusters I–IX including ≥three type strains), and eight minor clusters (clusters X–XVII with two type strains) were defined mostly at GSI of 92 and mean intra-cluster OrthoANIu of >80.00% (Table 2). The topologies of the nine major clusters were maintained irrespective of the addition/deletion of the related sequences and also supported in the core genome analyses reported previously (Dong et al., 2020; Bellassi et al., 2021; Tian et al., 2021). The origin of isolation for each type strain included in the clusters is given in Supplementary Table S3.
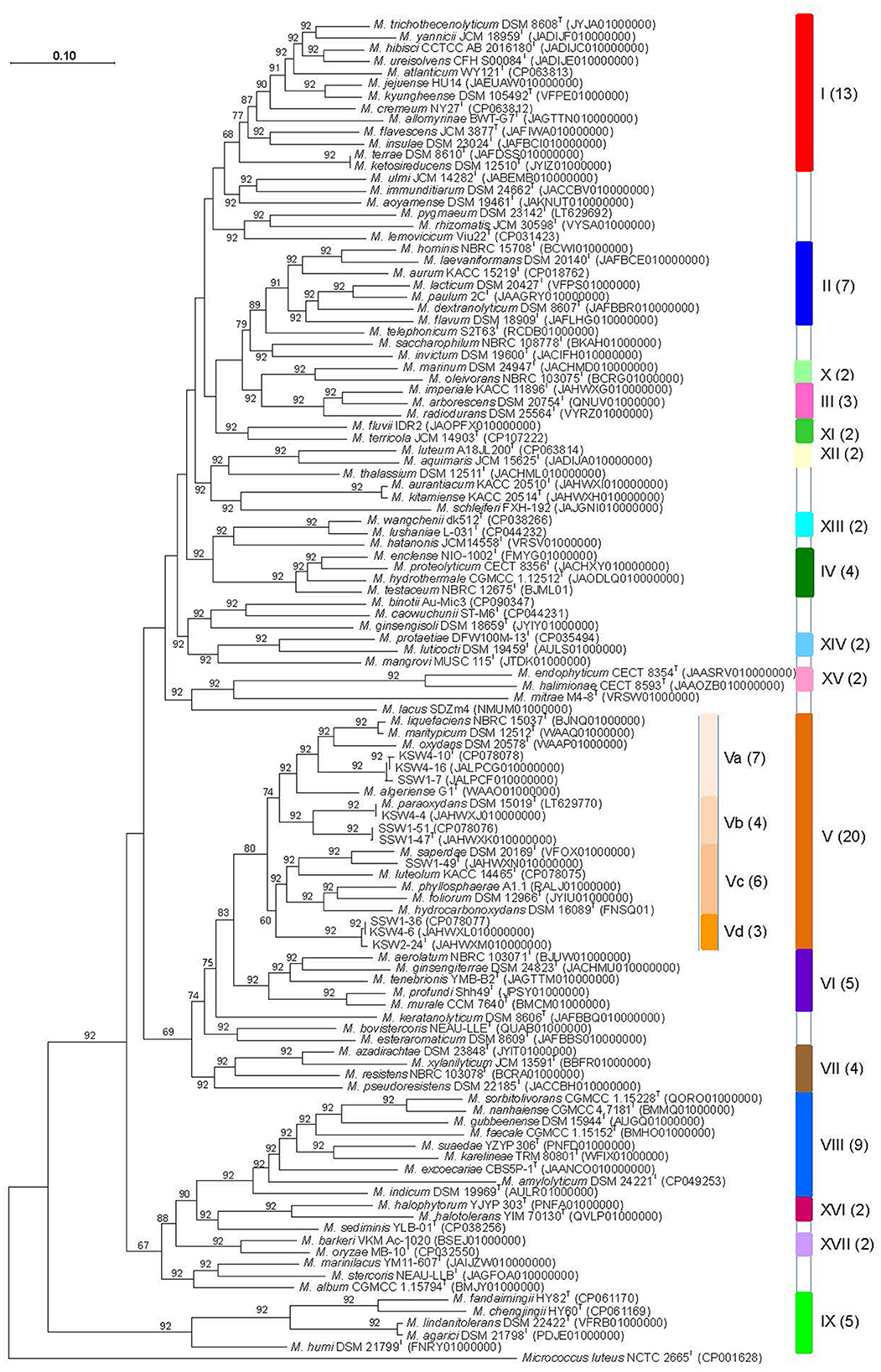
Figure 2. Core genome-based phylogenetic tree based on the genomes of the 10 study strains and 103 Microbacterium strains including 96 type and seven non-type strains. Colors on the right represent the clusters, most of which were defined at GSI of 92 and the mean intra-cluster OrthoANIu >80.00%. GSI values are given at branches.
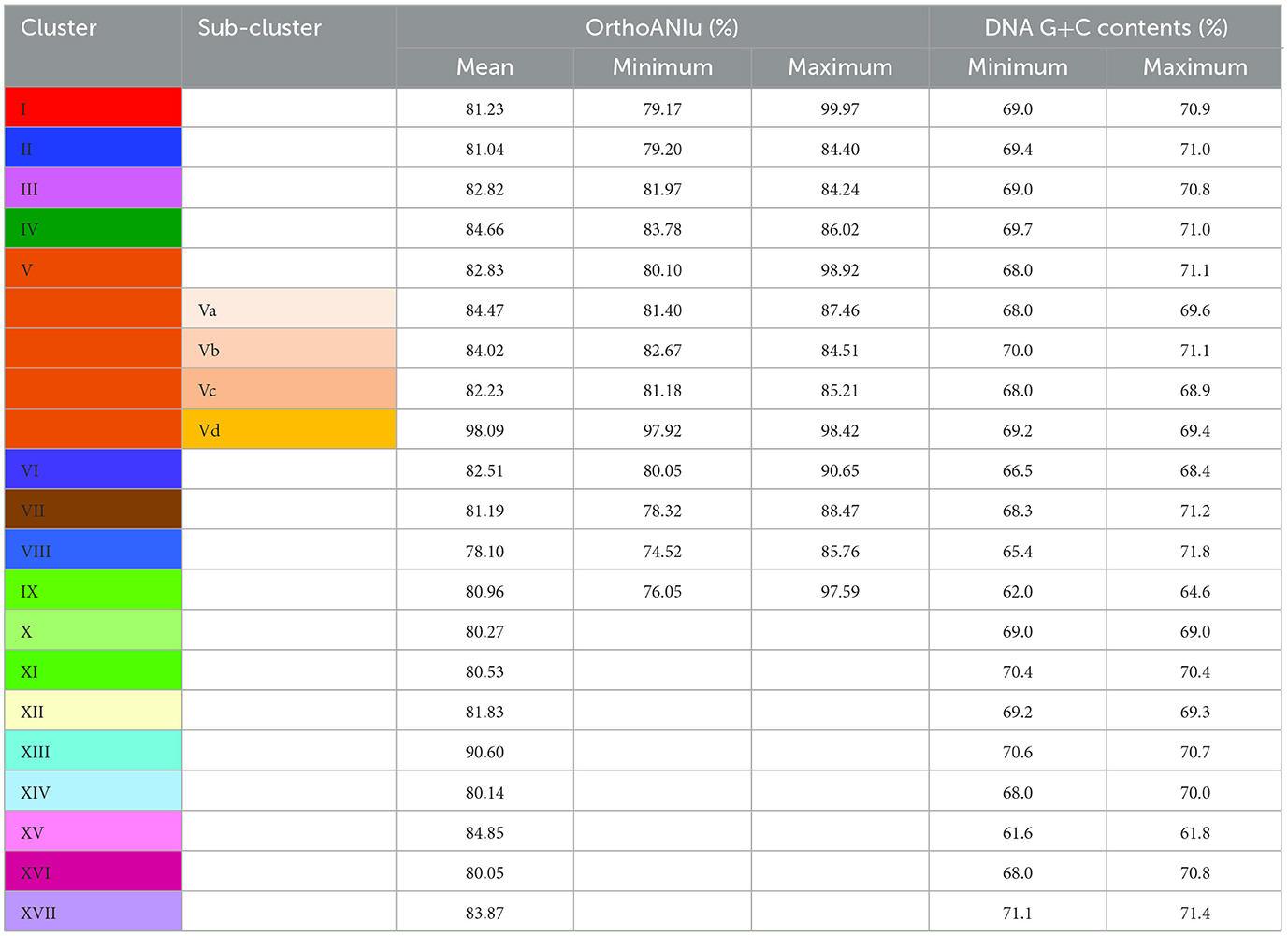
Table 2. Intra-cluster OrthoANIu values and DNA G+C contents of the nine major and eight minor clusters defined in genus Microbacterium.
Cluster I (Microbacterium trichothecenolyticum clade) consists of 13 species: Microbacterium allomyrinae (Lee and Kim, 2023), Microbacterium atlanticum and Microbacterium cremeum (Xie et al., 2021), Microbacterium flavescens (Collins et al., 1983; Takeuchi and Hatano, 1998b), Microbacterium hibisci (Yan et al., 2017), Microbacterium insulae (Yoon et al., 2009), Microbacterium jejuense (Kook et al., 2014), Microbacterium kyungheense (Kook et al., 2014), Microbacterium ketosireducens (Takeuchi and Hatano, 1998a), M. terrae and M. trichothecenolyticum (Yokota et al., 1993b; Takeuchi and Hatano, 1998b), Microbacterium ureisolvens (Cheng et al., 2019), and Microbacterium yannicii (Karojet et al., 2012). The cluster was defined relatively at low GSI (68) and showed intra-cluster OrthoANIu values of ≥79.17% (mean, 81.23%) and DNA G+C contents of 69.0–70.9% (Table 2). Most of the type strains also formed a coherent cluster in a previous core genome analysis (Xie et al., 2021), albeit that many of them formed well-separated sublines in the 16S rRNA gene trees (Dong et al., 2020; Lee and Kim, 2023; Figure 1). Among the species of which the genome sequences were not available yet, M. arthrosphaerae (Kämpfer et al., 2011) and Microbacterium aureliae were shown to be closely related to M. atlanticum, M. hibisci, M. ureisolvens, and M. yannicii in the 16S rRNA gene trees, albeit with low bootstrap support (Figure 1; Dong et al., 2020; Lee and Kim, 2023).
Cluster II (Microbacterium lacticum clade) contains seven species, namely, M. aurum (Yokota et al., 1993a), M. dextranolyticum (Yokota et al., 1993a), Microbacterium flavum (Kageyama et al., 2007a), Microbacterium hominis (Takeuchi and Hatano, 1998a), Microbacterium laevaniformans (Dias and Bhat, 1962; Collins et al., 1983), M. lacticum (Orla-Jensen, 1919; Approved Lists, 1980), and Microbacterium paulum (Bellassi et al., 2021), and revealed intra-cluster OrthoANIu values≥79.20% (mean, 81.04%) and DNA G+C contents of 69.4–71.0% (Table 2). Most of the type strains were also found as a single cluster in a previous core genome analysis (Bellassi et al., 2021), albeit that many of the strains formed well-separated sublines in the 16S rRNA gene trees (Dong et al., 2020; Lee and Kim, 2023; Figure 1).
Cluster III (Microbacterium imperiale clade) is composed of M. arborescens (Imai et al., 1984), M. imperiale (Collins et al., 1983), and Microbacterium radiodurans (Zhang et al., 2010), with intra-cluster OrthoANIu values of 81.97–84.24% (mean, 82.82%) and DNA G+C contents of 69.0–70.8% (Table 2), and formed a coherent cluster in the 16S rRNA gene trees, with high bootstrap support (Dong et al., 2020; Lee and Kim, 2023; Figure 1) and the previous analyses of core genomes (Dong et al., 2020; Bellassi et al., 2021; Tian et al., 2021).
Cluster IV (Microbacterium testaceum clade) consists of four species, namely, Microbacterium enclense (Mawlankar et al., 2015), M. hydrothermale (Zhang et al., 2014), Microbacterium proteolyticum (Alves et al., 2015), and M. testaceum (Takeuchi and Hatano, 1998b), the type strains of which shared intra-cluster OrthoANIu values of 83.78–86.02% (mean, 84.66%) and DNA G+C contents of 69.7–71.0% (Table 2). Most of them were also shown to be clustered together in the core genome-based phylogenetic trees reported previously (Dong et al., 2020; Tian et al., 2021; Xie et al., 2021). Although the genome sequence are not determined yet, Microbacterium zeae (Gao et al., 2017) was tightly associated with M. enclense and M. proteolyticum of this cluster in the 16S rRNA gene trees (Dong et al., 2020; Lee and Kim, 2023; Figure 1).
Cluster V (Microbacterium liquefaciens clade) is the largest and encompasses 10 Microbacterium species and the five groups of the study strains defined in 16S rRNA gene tree (Figure 1). This clade was defined at GSI of 80 and showed intra-cluster OrthoANIu values of ≥80.10% (mean, 82.83%) and DNA G+C contents of 68.0–71.1%, the strains of which were further assigned to four subclusters (Va–Vd) with support of 92 GSI. Subcluster Va contains the type strains of M. algeriense, M. liquefaciens, M. maritypicum, and M. oxydans together with three study strains, KSW4-10T, KSW4-16, and SSW1-7, having intra-subcluster OrthoANIu values of 81.40–87.46% (mean, 84.87%) and DNA G+C contents of 68.0–69.6%; subcluster Vb is composed of the type strain of M. paraoxydans and three study strains, KSW4-4, SSW1-47T, and SSW1-51, with intra-subcluster OrthoANIu values of 82.67–84.51% (mean, 84.02%) and DNA G+C contents of 70.0–71.1%; subcluster Vc encompasses the five type strains of M. luteolum, M. saperdae, M. foliorum, and M. phyllosphaerae (Behrendt et al., 2001), and M. hydrocarbonoxydans (Schippers et al., 2005), and one study strain, SSW1-49T, sharing intra-subcluster OrthoANIu values of 81.18–85.21% (mean, 82.23%) and DNA G+C contents of 68.0–68.9%; and subcluster Vd includes only the study strains, KSW2-24T, KSW4-6, and SSW1-36, with intra-subcluster OrthoANIu values of 97.92–98.42% (mean, 98.09%) and DNA G+C contents of 69.2–69.4% (Table 2). Among them, the six or seven type strains were associated together in the previous analyses of core genomes (Bellassi et al., 2021; Tian et al., 2021; Xie et al., 2021). In addition, most of the type strains were closely associated with one another in the 16S rRNA gene trees, while three species (M. foliorum and M. phyllosphaerae, and M. hydrocarbonoxydans) formed well-separated sublines from the other type strains (Dong et al., 2020; Lee and Kim, 2023; Figure 1).
Cluster VI (Microbacterium aerolatum clade) is composed of five species, namely, M. aerolatum (Zlamala et al., 2002), Microbacterium ginsengiterrae (Kim et al., 2010), M. murale (Kämpfer et al., 2012), Microbacterium profundi (Wu et al., 2008), and Microbacterium tenebrionis (Lee and Kim, 2023), and showed intra-cluster OrthoANIu values of 80.05–90.65% (mean, 82.51%) and DNA G+C contents of 66.5–68.4% (Table 2). The recently described Microbacterium ihumii (Yacouba et al., 2022) was also included in this cluster (data not shown). All the type strains also formed a single cluster in the previous analyses of core genomes (Lee and Kim, 2023) and whole genomes (Yacouba et al., 2022), although they were divided into two lineages in the 16S rRNA gene trees (Figure 1; Lee and Kim, 2023). Among the species of which the genome sequences were not determined yet, Microbacterium panaciterrae (Nguyen et al., 2015), Microbacterium shaanxiense (Peng et al., 2015), and Microbacterium tumbae (Nishijima et al., 2017) formed a coherent cluster with M. aerolatum, M. ginsengiterrae, and M. tenebrionis of this cluster in the 16S rRNA gene tree (Figure 1).
Cluster VII (Microbacterium resistens clade) consists of four species, namely, Microbacterium azadirachtae (Madhaiyan et al., 2010), Microbacterium pseudoresistens (Young et al., 2010), Microbacterium resistens (Behrendt et al., 2001), and Microbacterium xylanilyticum (Kim et al., 2005), the type strains of which showed intra-cluster OrthoANIu values of 78.32–88.47% (mean, 81.19%) and DNA G+C contents of 68.3–71.2% (Table 2). Members of this cluster were also associated together in the previous phylogenetic trees based on core genomes (Dong et al., 2020; Lee and Kim, 2023), albeit with the formation of four independent sublines in the 16S rRNA gene trees (Figure 1; Lee and Kim, 2023).
Cluster VIII (Microbacterium gubbeenense clade) was defined at GSI of 92 and contains nine type species, forming a tight cluster with high bootstrap support in the 16S rRNA gene trees (Dong et al., 2020; Lee and Kim, 2023; Figure 1): M. amylolyticum (Anand et al., 2012), Microbacterium excoecariae (Chen et al., 2020), Microbacterium faecale (Chen et al., 2016), M. gubbeenense (Brennan et al., 2001), Microbacterium indicum (Shivaji et al., 2007), Microbacterium karelineae (Zhu et al., 2021), Microbacterium nanhaiense (Yan et al., 2015), Microbacterium sorbitolivorans (Meng et al., 2016), and M. suaedae (Zhu et al., 2019). The intra-cluster OrthoANIu values and DNA G+C contents were 74.52–85.76% (mean, 78.10%) and 65.4–71.8%, respectively (Table 2). All the type strains formed a tight cluster with high bootstrap support in the 16S rRNA gene tree (Figure 1) and were also found as a single cluster in our previous core genome-based phylogenetic tree (Lee and Kim, 2023).
Cluster IX (Microbacterium agarici clade) forms the deepest branch within the genus Microbacterium and contains the five type strains of M. agarici and Microbacterium humi (Young et al., 2010), Microbacterium lindanitolerans (Lal et al., 2010), and Microbacterium chengjingii and Microbacterium fandaimingii (Zhou et al., 2021), being well separated from the other Microbacterium species with support of 92 GSI. This fact was also supported in both the previous core genome-based phylogenetic trees (Bellassi et al., 2021; Lee and Kim, 2023) and 16S rRNA gene trees (Figure 1; Lee and Kim, 2023). The intra-cluster OrthoANIu values were 76.05–97.59% (mean, 80.96%) and DNA G+C contents of 62.0–64.6% (Table 2).
Among the eight minor clusters (X–XVII) shown in Figure 2, Microbacterium aquimaris Kim et al. 2008–Microbacterium luteum Xie et al. 2021 (Cluster XII; 81.83% OrthoANIu), Microbacterium endophyticum Alves et al. 2014 –Microbacterium halimionae Alves et al. 2014 (Cluster XV; 84.85% OrthoANIu), Microbacterium protaetiae Heo et al. 2020–Microbacterium luticocti (Vaz-Moreira et al., 2008) (Cluster XIV; 80.14% OrthoANIu), Microbacterium halotolerans (Li et al., 2005)–Microbacterium halophytorum (Li et al., 2018) (Cluster XVI; 80.05% OrthoANIu), and Microbacterium barkeri Takeuchi and Hatano 1998b–Microbacterium oryzae (Kumari et al., 2013) (Cluster XVII; 83.37% OrthoANIu) pairs (Table 2) are also well supported by the 16S rRNA gene trees (Dong et al., 2020; Lee and Kim, 2023; Figure 1) and the previous analyses of core genomes (Dong et al., 2020; Bellassi et al., 2021; Tian et al., 2021; Xie et al., 2021; Lee and Kim, 2023). On the other hand, the remaining three minor clusters, namely, Microbacterium marinum Zhang et al. 2012–M. oleivorans Schippers et al. 2005 (Cluster X; 80.27% OrthoANIu), Microbacterium fluvii (Kageyama et al., 2007c)–Microbacterium terricola (Kageyama et al., 2007b) (Cluster XI; 80.53% OrthoANIu), and Microbacterium wangchenii Dong et al. 2020 –Microbacterium lushaniae Tian et al. 2021 pairs (Cluster XIII; 90.60% OrthoANIu), formed tight clades in the core genome-based phylogenetic tree (Figure 2, Table 2), but each type strain occupied independent positions in the 16S rRNA gene trees (Figure 1; Dong et al., 2020; Lee and Kim, 2023).
A further 27 Microbacterium strains constitute single-membered clusters, most of which showed low OrthoANIu values < 80.00% with members of the defined clusters above, albeit with support by high GSI in the core genome-based phylogenetic tree (Figure 2).
OGRI analyses
The OrthoANIu and dDDH values between the study strains and closely related type strains are given in Supplementary Table S3. The study strains which were defined as five groups in the 16S rRNA gene tree (Figure 1) were found to belong to the cluster V (M. liquefaciens clade) in the core genome-based phylogenomic tree (Figure 2). The intra-group OrthoANIu values of the groups A (KSW4-10T, KSW4-16, and SSW1-7), C (KSW2-24T, KSW4-6, and SSW1-36), and D (SSW1-47T and SSW1-51) were 97.74–98.89%, 97.92–98.42%, and 98.9%, respectively, while the inter-group OrthoANIu values were relatively low and ranged from 80.90 to 82.67% (Supplementary Table S4), revealing that the above three groups constituted independent taxa (Richter and Roselló-Móra, 2009). On the other hand, the strains of the group A showed high OrthoANIu values with the type strains of M. liquefaciens and M. maritypicum (85.31–85.64% and 85.64–85.90%, respectively). Strain SSW1-49T (group B) and the strains of the group C revealed high OrthoANIu values with the type strain of M. saperdae (89.40% and 80.80–81.95%, respectively), whereas the strains of the group D and KSW4-4 (group E) revealed high OrthoANIu values (84.40–84.48% and 98.9%, respectively) with the type strain of M. paraoxydans (Table 3). Concerning the ANI threshold (95–96%) for delineation of species (Richter and Roselló-Móra, 2009), these results support that the groups A–D represent four new species of the genus Microbacterium and strain KSW4-4 is a strain of M. paraoxydans, albeit with different origin of isolation.
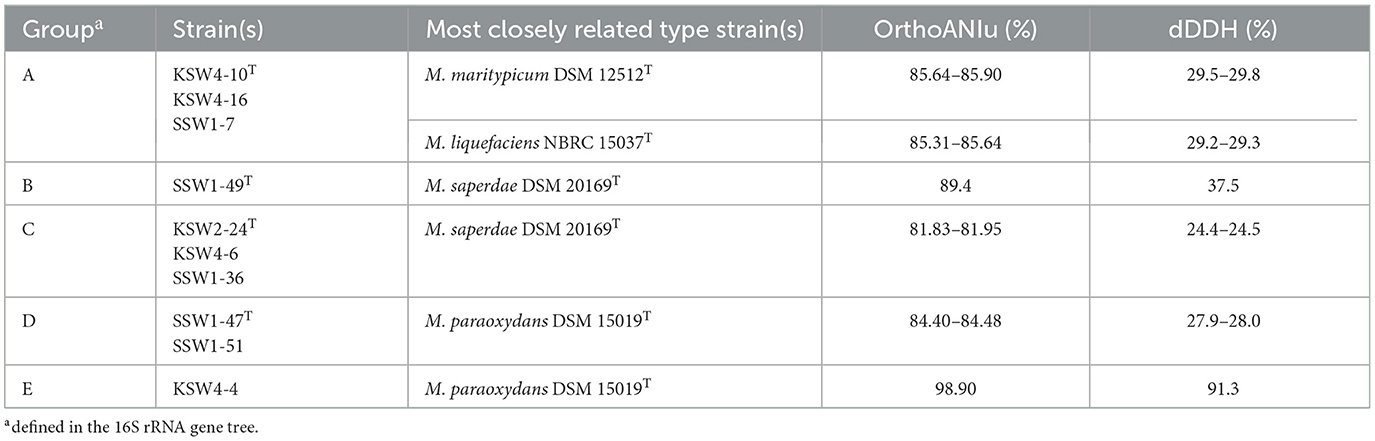
Table 3. OrthoANIu and dDDH values calculated between the 10 study strains and most closely related type strains.
The calculation of dDDH values also supported the results of ANI analysis. The intra-group dDDH values of the groups A, C, and D were 80.6–91.2%, 81.8–85.9%, and 90.9%, respectively, being higher than the threshold (70%) for prokaryotic species delineation (Wayne et al., 1987), while the inter-group dDDH values of the groups A–D were relatively low ( ≤ 25.0%) (Supplementary Table S4). On the other hand, all the study strains showed low dDDH values ( ≤ 37.5%) with the seven closely related type strains, with the exception that strain KSW4-4 shared a high dDDH (91.3%) with the type strain of M. paraoxydans (Table 3).
Phenotypic characteristics
The results for a total of 91 physiological and biochemical tests were compared for the study strains and seven closely related type strains. Among them, 44 phenotypic traits (48.4%) were positive or negative for all of the test strains (Supplementary Table S5). Differential phenotypic characteristics among the test strains contained 21 acid productions from substrates, seven carbon source assimilations, 14 enzyme activities, pH and temperature ranges for growth, and NaCl tolerance (Supplementary Table S6). The strains of the group A showed the largest intra-group variation (4.4%) and were variable in acid production from D-melezitose, D-melibiose, L-rhamnose, and ribose according to the strains. The strains of the groups C and D were variable in acid production from N-acetyl-glucosamine. In addition, gelatinase activity was also variable in the strains of the group C (Supplementary Table S6).
In some of the type strains tested in this study, there were shown to be the phenotypic traits that conflict with the previously described results (Collins et al., 1983; Takeuchi and Hatano, 1998a; Schumann et al., 1999; Laffineur et al., 2003; Lenchi et al., 2020). All the physiological and biochemical characters of the type strains were examined under the same conditions with the study strains except that acid production was recorded after 7-day incubation. M. paraoxydans KACC 14506T revealed the largest difference (12.1%) from the results of Laffineur et al. (2003) for up to 11 phenotypic traits (acid production from five substrates, four enzyme activities, and growth at 10°C and 42°C). M. maritypicum KACC 14436T showed the results (5.5%) conflicting with data of Takeuchi and Hatano (1998a) in one assimilation and four acid production tests. M. algeriense DSM 109018T (5.5%) differed from data of Lenchi et al. (2020) in growth tests at 10°C, 50°C, and pH 5, together with glucose fermentation and assimilation of L-arabinose, while M. liquefaciens KACC 14464T and M. oxydans KACC 14467T differed from the results reported previously (Collins et al., 1983; Schumann et al., 1999) only in one or two phenotypic traits (Supplementary Tables S5, S6), respectively.
The study strains contained D-ornithine as the diagnostic diamino acid and N-glycolylated mureins in their cell walls, a polar lipid profile including diphosphatidylglycerol, phosphatidylglycerol, and an unidentified glycolipid; and the fully unsaturated menaquinones with 10, 11, and 12 isoprene units (MK-10, MK-11, and MK-12, respectively). The type strains of their phylogenetically close neighbors showed the same patterns of diamino acid in the cell walls and polar lipids but did not contain MK-10 that was detected in the study strains (Supplementary Table S7). The cellular fatty acids of all the study strains and the seven reference type strains consisted mainly of iso- and anteiso-branched components (Supplementary Table S8). Most of the strains contained antiso-C15:0 (36.6–68.1%), antiso-C17:0 (13.7–33.7%), and iso-C16:0 (10.6–25.4%) as the major fatty acids (>10% of the total). The study strains of the group A and M. luteolum KACC 14465T also contained iso-C15:0 (13.6–15.8%), iso-C15:0 (12.1%), and anteiso-C15:1 A (10.6%), respectively, as additional major fatty acids, while M. liquefaciens KACC 14464T and M. maritypicum KACC 14436T contained small amounts of iso-C16:0 (9.4%) and antiso-C17:0 (8.1%), respectively, as compared with all the other strains (Supplementary Table S8).
Reclassification of Microbacterium species
Microbacterium ketosireducens Takeuchi and Hatano 1998 is a later heterotypic synonym of Microbacterium terrae (Yokota et al., 1993) Takeuchi and Hatano 1998
M. ketosireducens IFO 14548T (Takeuchi and Hatano, 1998a) isolated from soil is known to have an ability to reduce 2,5-diketo-D-gluconic acid to 2-keto-D-gluconic acid. In the same year, M. terrae (Takeuchi and Hatano, 1998b) was assigned to the redefined genus Microbacterium from Aureobacterium terrae (Yokota et al., 1993b) through the union of the genera Microbacterium and Aureobacterium based on 16S rRNA gene phylogeny. In the original description, M. ketosireducens IFO 14548T was shown to share in vitro DDH value of 47% with M. terrae IFO 15300T, but the 16S rRNA gene sequence similarity between both of the type strains was not compared.
In this study, M. ketosireducens IFO 14548T and M. terrae IFO 15300T were found to show 16S rRNA gene sequence similarity of 99.72% (4 nt differences) to each other, both of which sequences revealed 99.65% (5 nt differences) with their genome-derived ones, respectively (Supplementary Table S1), while the 16S rRNA gene sequence (MZ433298) of M. terrae KACC 14470T determined in this study shared 100% sequence identity with the genome-derived ones of M. terrae DSM 8610T (JAFDDS010000001) and M. ketosireducens DSM 12510T (JYIZ01000009). The OGRI analysis indicated that both of the type strains shared a dDDH value of 100% and an OrthoANIu of 99.97% to each other (Table 4), supporting the conclusion that Microbacterium ketosireducens Takeuchi and Hatano 1998 is a later heterotypic synonym of Microbacterium terrae (Yokota et al. 1993) Takeuchi and Hatano 1998 according to the page priority [Rule 24b (4) of the International Code of Nomenclature of Prokaryotes (ICNP; Oren et al., 2022)], for names validly published in the same year.
Microbacterium kitamiense Matsuyama et al. 1999 as a later heterotypic synonym of Microbacterium aurantiacum Takeuchi and Hatano 1998
M. kitamiense Kitami C2T (Matsuyama et al., 1999), a polysaccharide-producing bacterium isolated from the wastewater of a sugar-beet factory, was shown to be closely associated with the type strain of M. aurantiacum in the 16S rRNA gene tree (Figure 1). In that study, the in vitro DDH value between both of the type strains was 68%, with their DNA G+C contents of 69.2 mol% and 69.3 mol%, respectively, but 16S rRNA gene sequence similarity was not presented.
For the calculation of precise 16S rRNA gene sequence similarity and OGRI values, the genome sequences of M. kitamiense KACC 20514T and M. aurantiacum KACC 20510T were determined in this study. The genome-derived 16S rRNA gene sequence (JAHWXH010000003) of M. kitamiense KACC 20514T was the same as the corresponding sequence (AB013919) of M. kitamiense Kitami C2T (Supplementary Table S1), while the complete genome-derived 16S rRNA gene sequence of M. aurantiacum KACC 20510T was obtained through concatenation of the two partial ones present in different contigs of the genome (JAHWXI01000000), showing 100% sequence identity with sequence EU863415 of M. aurantiacum CIP 105730T determined by the Sanger method. Both of the type strains showed an OrthoANIu of 97.89% and a dDDH of 81.9% to each other (Table 4). Considering the thresholds for species delineation (Wayne et al., 1987; Richter and Roselló-Móra, 2009), Microbacterium kitamiense Matsuyama et al. 1999 should be considered as a later heterotypic synonym of Microbacterium aurantiacum Takeuchi and Hatano 1998.
Microbacterium maritypicum (ZoBell and Upham 1944) Takeuchi and Hatano 1998 is a later heterotypic synonym of Microbacterium liquefaciens (Collins et al. 1983) Takeuchi and Hatano 1998
M. maritypicum Takeuchi and Hatano 1998 was proposed by the reclassification of Flavobacterium maritypicum (ZoBell and Upham, 1944) based on phenotypic characteristics, together with in vitro DDH and 16S rRNA gene analysis, and shown to have in vitro DDH values of 13–27% to the close relatives (M. liquefaciens, M. luteolum, and M. saperdae), although there was no comparison of their 16S rRNA gene sequences. In the same year, M. liquefaciens (Takeuchi and Hatano, 1998b) was assigned to the redefined genus Microbacterium from Aureobacterium liquefaciens (Collins et al., 1983) through the union of the genera Microbacterium and Aureobacterium based on 16S rRNA gene phylogeny.
In this study, M. maritypicum belongs to cluster V (M. liquefaciens clade) in the core genome-based phylogenetic tree (Figure 2) and was closely related to six species (M. algeriense, M. liquefaciens, M. luteolum, M. oxydans, M. paraoxydans, and M. saperdae), as visualized by very short lengths of branches in the 16S rRNA gene tree (Figure 1). Comparison of all their genome-derived 16S rRNA gene sequences revealed that M. maritypicum DSM 12512T shares 100% sequence identity with the type strains of M. liquefaciens and M. oxydans and shows sequence similarity of 99.45–99.87% (2–8 nt differences) with the remaining type strains (Supplementary Table S2). The OGRI analysis showed that M. maritypicum DSM 12512T shares high OrthoANIu (98.30%) and dDDH (84.3%) values with M. liquefaciens NBRC 15037T (Table 4, Supplementary Table S4), while its OrthoANIu (82.19–87.18%) and dDDH ( ≤ 32.1%) values with the remaining type strains were relatively low (Supplementary Table S4). Based on the OGRI thresholds for species demarcation (Wayne et al., 1987; Richter and Roselló-Móra, 2009), these results support the conclusion that Microbacterium maritypicum (ZoBell and Upham, 1944) Takeuchi and Hatano 1998 is a later heterotypic synonym of Microbacterium liquefaciens (Collins et al., 1983) Takeuchi and Hatano 1998 according to the page priority [Rule 24b (4) of the ICNP], for names validly published in the same year.
Microbacterium species maintained or needed to be further verified as separate species
Several type strain pairs with high 16S rRNA gene sequence similarity (≥99.72%; up to 3 nt differences) were found in 16S rRNA gene analysis. The M. halimionae–M. endophyticum pair (Alves et al. 2014) share high sequence similarity (99.8%; 3 nt differences) but showed the OrthoANIu 84.85% of and dDDH of 28.0% to each other (Table 5). M. arborescens was described by Imai et al. (1984) only based on phenotypic differences without genotypic analysis. In this study, M. arborescens DSM 20754T shared 16S rRNA gene sequence similarity of 99.72% (3 nt differences) with M. imperiale KACC 11896T, but the OrthoANIu and dDDH values between both of the type strains were 84.24% and 27.2%, respectively (Table 5). In addition, M. oxydans DSM 20578T shared 100% sequence identity in 16S rRNA gene with the type strains of M. liquefaciens and M. maritypicum (Supplementary Table S2), but it showed an OrthoANIu of 87.46% and a dDDH of 32.7% with M. liquefaciens NBRC 15037T, followed by an OrthoANIu of 87.18% and a dDDH of 32.1% with M. maritypicum DSM 12512T (Table 5). These results of the OGRI analysis support their original descriptions of the above six species as separate taxa (Wayne et al., 1987; Richter and Roselló-Móra, 2009), except for M. maritypicum (see above).
In addition, high 16S rRNA gene sequence similarity (> 99.79%; 3 nt differences) was found for two other species pairs, M. deminutum–M. pumilum (Kageyama et al., 2006) and M. neimengense (Gao et al., 2013)–M. biotini (Clermont et al., 2009) (Table 5), as revealed by very short lengths of branches in 16S rRNA gene phylogeny (Figure 1), but their genome sequences are not available yet. Considering that in vitro DDH assays for some Microbacterium type strains were inaccurate (Takeuchi and Hatano, 1998a; Matsuyama et al., 1999), the genome sequencing and analysis of these four species are further required.
Transfer of five Microbacterium species to a new genus
The five type strains of cluster IX (M. agarici clade) that form the deepest branch in the phylogenomic tree (Figure 2) are shown to be well separated from the other Microbacterium species, which is also supported in 16S rRNA gene trees (Dong et al., 2020; Bellassi et al., 2021; Tian et al., 2021; Lee and Kim, 2023; Figure 1). To determine the generic assignment of these species (M. agarici, M. chengjingii, M. fandaimingii, M. humi, and M. lindanitolerans), AAI values were determined between the five type strains of these species and all the other Microbacterium strains. As a result, the intra-cluster AAI values of cluster IX were ≥72.64% (mean, 80.95%), while the inter-cluster AAI values with other members of the genus Microbacterium were ≤ 59.18% (mean, 57.27%) (Table 6, Supplementary Table S9), being notably lower than the thresholds (74–76% or 68%) proposed for genus delineation (Wirth and Whitman, 2018; Nicholson et al., 2020; Zheng et al., 2020). These results support the conclusion that the five type strains of cluster IX should be transferred to a new genus of the family Microbacteriaceae separated from the genus Microbacterium, for which the name Paramicrobacterium gen. nov. is proposed. Among these species, M. agarici (Young et al., 2010) and M. lindanitolerans (Lal et al., 2010) were not compared to each other for their 16S rRNA gene sequences because they were published in the same year. In this study, both of the type strains were shown to share 16S rRNA gene sequence similarity of 100%, an OrthoANIu of 97.59 %, and a dDDH of 78.6% to each other (Table 4), supporting the conclusion that Microbacterium lindanitolerans Lal et al. 2010 is a later heterotypic synonym of M. agarici Young et al. 2010 according to the page priority [Rule 24b (4) of the ICNP], for names validly published in the same year.
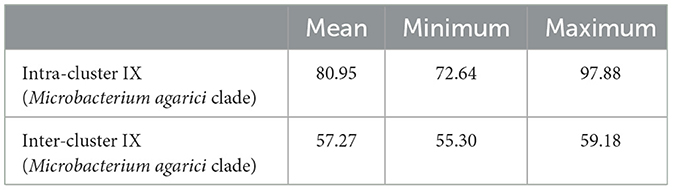
Table 6. Intra- and inter-cluster AAI values (%) of cluster IX (Microbacterium agarici clade) in the genus Microbacterium.
Discussion
The 16S rRNA gene has traditionally been used as a good molecular marker for taxonomic studies, albeit with the limitation in resolving phylogenetic relationships at the species level (Carro et al., 2018; Na et al., 2018). In this study, before performing 16S rRNA gene-based phylogenetic analysis, the 16S rRNA gene sequences available for each type strain of all Microbacterium species were retrieved from public databases, such as the LPSN, EzBioCloud, and NCBI, and compared for checking their authenticity and completeness. As a result, it was shown that many of the 16S rRNA gene sequences were slightly inaccurate when compared with genome-derived sequences, while some of them were incorrectly reported as the reference sequence of the type strains in LPSN. The sequences of M. hydrothermale 0704CP-2T (HM222660), M. rhizosphaerae CHO1T (KP72259), and M. suaedae YZYP 306T (MF084212) listed in their original descriptions and LPSN, together with those of M. aerolatum CCM 4955T (MT760116) and M. dextranolyticum IFO 14592T (D21341 and AB007417) contained in NCBI, revealed sequence similarities of 98.50–99.51% (7–21 nt differences) compared with the original or corresponding genome-derived ones. Considering that some Microbacterium type strains, albeit with revealing very short branch lengths in the 16S rRNA gene tree, are maintained as independent taxa at the species level, it is evident that the use of the above six sequences in the 16S rRNA gene phylogeny can make it very difficult to unravel their intrinsic phylogenetic relationships. Moreover, the 16S rRNA gene sequences of M. amylolyticum CCM 4955T (MT760185) and M. arthrosphaerae CCM 7681T (MT760166) listed in LPSN and M. testaceum CCM 2299T (MT760091) contained in NCBI were found to be the same with those given in the original descriptions of the type strains of M. arthrosphaerae, M. murale, and M. amylolyticum, respectively. These results indicate that the MT760185 and MT760166 were incorrectly listed as the reference sequences of M. amylolyticum and M. arthrosphaerae, respectively, in LPSN.
The 10 strains isolated from seaweeds from two beaches (Table 1), together with M. arthrosphaerae KACC 16680T, M. luteolum KACC 14465T, and M. terrae KACC 14470T, were subjected to 16S rRNA gene sequencing to identify their closely related neighbors. The 16S rRNA gene analysis with near full-length sequences showed that the strains from this study can be divided into five groups with 100% intra-group sequence identity. Very close phylogenetic relationships were found among the study strains and between the study strains and reference type strains of M. algeriense, M. liquefaciens, M. luteolum, M. maritypicum, M. oxydans, M. paraoxydans, and M. saperdae, as given by the very short lengths of the tree branches and high 16S RNA gene sequence similarity (99.36–100%; 0–9 nt differences). Among the type strains, M. liquefaciens NBRC 15037T, M. maritypicum DSM 12512T, and M. oxydans DSM 20578T share 100% identity in 16S rRNA gene sequence to one another.
In this study, we report the overall phylogenomic clustering of the genus Microbacterium by core genome analysis, with a total of the 113 genomes, including the ten study strains and 103 reference Microbacterium strains. In general, core genome-based phylogenetic trees are known to be consistent with those using whole-genome data (Riesco et al., 2018) and can additionally provide bootstrap support by means of GSI values. The core genome-based phylogenetic tree reveals that nine major, eight minor, and 27 single-membered clusters can be defined, most of which were established at GSI of 92 and mean intra-cluster OrthoANIu values of >80.00%. Considering that the genome sequences of 28 Microbacterium type strains are not still available yet, the presence of many single-membered clusters suggests that the genus Microbacterium is still under-speciated. Only three of the major clusters were recognized in their entirety in the 16S rRNA gene trees: the clusters III (M. imperiale clade), VIII (M. gubbeenense clade), and IX (M. agarici clade), although M. amylolyticum of cluster VIII showed significantly lower OrthoANIu values (75.40–75.78%) and DNA G+C content (65.4%) than the mean intra-cluster OrthoANIu value (78.10%) and DNA G+C content (68.9%). The five species of cluster IX consistently formed the deepest branches in both the core genome- and 16S rRNA gene-based phylogenetic trees. Moreover, they formed a distinct cluster at a position located remotely from members of the genus Microbacterium in the whole-genome-based phylogenetic tree of the class Actinobacteria (Nouioui et al., 2018). This overall phylogenomic clustering of the genus Microbacterium can provide a framework for the future descriptions of new taxa. Despite the recent increase of available genome sequences, the overall phylogenomic analysis based on whole- or core genome sequences for the genus Microbacterium was not performed to date. In the recent descriptions of new species (Dong et al., 2020; Bellassi et al., 2021; Tian et al., 2021; Xie et al., 2021), core genome-based phylogenetic analyses have been partially performed with the 26–49 type strains of the genus Microbacterium. Among the Microbacterium strains included in the previous phylogenomic analyses (Dong et al., 2020; Tian et al., 2021), the genomes of “Microbacterium barkeri” 2011-R4 (AKVP01000000), “Microbacterium chocolatum” SIT 101 (CP015810), “M. kitamiense” Sa12 (PGGU01000000), and “Microbacterium paludicola” CC3 (CP018134) were not those of the type strains of the corresponding species in that the genome-derived 16S rRNA gene sequences were considerably different from those of the type strains determined by Sanger method, indicating that they should not be used as reference genomes.
The OGRI represent any measurements indicating how similar are two genome sequences (Chun and Rainey, 2014). Among them, ANI and dDDH have been widely used for prokaryotic species demarcation. The ANI threshold (95–96%) proposed for species delineation has been found to be correlated with the in vitro DDH threshold of 70% (Goris et al., 2007; Richter and Roselló-Móra, 2009). It is now evident that the dDDH calculated through comparisons of whole-genome sequences is more unbiased for discriminating between closely related strains than the values determined by error-prone and labor-extensive DDH experiments (Rosselló-Móra et al., 2011; Meier-Kolthoff et al., 2013). In this study, the dDDH values calculated from the genomes of some type strain pairs were found to be very higher than those determined by in vitro DDH experiments in previous studies (Takeuchi and Hatano, 1998a; Matsuyama et al., 1999). The results of the OGRI analysis support that the strains of the four groups (A–D) represent four new species of the genus Microbacterium.
The dDDH values estimated from the genomes of six pairs of the closely related type strains showed that three fell above the 70% threshold (Wayne et al., 1987), indicating that M. ketosireducens (Takeuchi and Hatano 1998a), M. kitamiense (Matsuyama et al. 1999), and M. maritypicum (Takeuchi and Hatano 1998a) are later heterotypic synonyms of preexisting species, while the others fell below the 70% threshold (Wayne et al., 1987), supporting that M. halimionae and M. endophyticum (Alves et al. 2015), M. imperiale (Collins et al., 1983) and M. arborescens (Imai et al., 1984), and M. oxydans (Schumann et al. 1999) and M. liquefaciens (Takeuchi and Hatano 1998b) are appropriately classified as distinct species.
Furthermore, AAI values have been used to define the genus boundary in several families (Wirth and Whitman, 2018; Nicholson et al., 2020; Zheng et al., 2020), although a standardized threshold has not been established. In this study, members of cluster IX (M. agarici clade) showed significantly lower inter-cluster AAI values with other members of the genus Microbacterium than the thresholds proposed for genus delineation. The results of AAI analysis, together with distinctness in all the phylogenetic trees (this study, Nouioui et al., 2018), support the conclusion that five members of this cluster, namely, M. agarici, M. chengjingii, M. fandaimingii, M. humi, and M. lindanitolerans, should be transferred to a new genus of the family Microbacteriaceae.
The kind and/or isomer of the diamino acids of the cell wall peptidoglycan is of taxonomically significant value in distinguishing actinomycete genera, in particular within the Microbacteriaceae (Evtushenko, 2012). Members of the genus Microbacterium contain L-lysine, D-ornithine, or diaminobutyric acid as the diagnostic diamino acid in their cell walls, depending on the strain (Suzuki and Hamada, 2012; Fidalgo et al., 2016). Only one diamino acid is found in most of the phylogenomic clusters defined in this study, but two diamino acids are present in the cell walls in some of the clusters (Suzuki and Hamada, 2012): M. radiodurans (D-ornithine; Zhang et al., 2010) and the other two species (L-lysine) of cluster III (M. imperiale clade); M. enclense (L-lysine; Mawlankar et al., 2015) and the other three species (D-ornithine) of cluster IV (M. testaceum clade); and M. gubbeenense (L-lysine) and the other eight species (D-ornithine) of cluster VIII (M. gubbeenense clade). The study strains contain D-ornithine in their cell walls, which is consistent with the type strains of cluster V (M. liquefaciens clade). Menaquinones with completely unsaturated (non-hydrogenated) side chains are found in members of the family Microbacteriaceae (Evtushenko, 2012). Members of the genus Microbacterium are known to contain completely unsaturated menaquinones with 10 to 14 isoprene units (Suzuki and Hamada, 2012). The study strains were shown to have MK-10 or MK-11 and MK-12 depending on the strain, similar to the seven type strains of cluster V (M. liquefaciens clade), except for the presence of MK-10. Other chemotaxonomic properties of the study strains, such as cell wall acyl type, polar lipid profiles, and major fatty acids, were typical for members of the genus Microbacterium (Suzuki and Hamada, 2012).
For the physiological and biochemical features tested in this study, the strains of groups A, C, and D showed intra-group variability of 4.4%, 2.2%, and 1.1%, respectively. In addition, some of the reference type strains gave results (1.1–12.1%) conflicting with the previously reported data, with the largest difference in M. paraoxydans. These results reflect that the use of the phenotypic traits should be applied at the strain level (Riesco et al., 2018) because of limited value in differentiating species (Amaral et al., 2014; Sutcliffe, 2015).
Concluding remarks
This study was designed to provide a more stable and reliable framework for the classification of the genus Microbacterium through phylogenetic analyses based on the 16S rRNA gene and genome sequences. For these, the 16S rRNA gene sequences of all Microbacterium type strains were compared with one another and further with the corresponding genome-derived ones if available, and the phylogenetic tree was constructed with authentic and nearly complete 16S rRNA gene sequences.
Second, the overall phylogenomic clustering of the genus Microbacterium was performed by core genome analysis for providing a working guideline for description of new taxa in future. Third, OGRI calculations, such as OrthoANIu and dDDH, were applied to the 10 study strains and Microbacterium type strains for the delineation of species. Moreover, the AAI values as genus boundary were compared between the Microbacterium type strains. In addition, the phenotypic markers such as chemotaxonomic, physiological, and biochemical traits were examined for characterization of the study strains and closely related type strains. The results of this study support the conclusion that the 10 study strains constitute members of four new Microbacterium species and that several Microbacterium species names are later heterotypic synonyms of earlier species names. In addition, five Microbacterium species from the “Microbacterium agarici clade” should be assigned to a new genus of the family Microbacteriaceae.
Taxonomic consequences: new taxa
Description of Paramicrobacterium gen. nov.
Paramicrobacterium (Gr. pref. para-, beside; N.L. neut. n. Microbacterium, a genus name; N.L. neut. n. Paramicrobacterium resembling the genus Microbacterium).
Cells are Gram-stain-positive, oxidase-positive and catalase-positive, and rod-shaped. The peptidoglycan is of type B2 with D-ornithine as diamino acid. The major menaquinone is MK-11. The presence of MK-10 or MK-12 is variable depending on species. The predominant polar lipids are diphosphatidylglycerol, phosphatidylglycerol, and an unidentified glycolipid. The major fatty acids are anteiso-C15:0, anteiso-C17:0, and iso-C16:0. The presence of iso-C15:0 as the main component is variable depending on species. The genomic DNA G+C contents are 62.0–67.3%. The type species is Paramicrobacterium agarici. The genus belongs to the family Microbacteriaceae.
Description of Paramicrobacterium agarici comb. nov.
Paramicrobacterium agarici (N.L. gen. masc. n. agarici, of Agaricus, the generic name of the mushroom Agaricus blazei from where the type strain was isolated).
Basonym: Microbacterium agarici Young et al. 2010
The description is as given by Young et al. (2010) and Nouioui et al. (2018). The type strain is CC-SBCK-209T (= DSM 21798T = CCM 7686T). “Microbacterium lindanitolerans” MNA2 (= DSM 22422 = CCM 7585) is a different strain of this species.
Description of Paramicrobacterium humi comb. nov.
Paramicrobacterium humi (hu'mi. L. gen. n. humi of earth, soil, of soil, the source of the type strain).
Basonym: Microbacterium humi Young et al. 2010
The description is as given by Young et al. (2010) and Nouioui et al. (2018). The type strain is CC-12309T (= DSM 21799T = CCM 7687T).
Description of Paramicrobacterium chengjingii comb. nov.
Paramicrobacterium chengjingii (cheng.jing'i.i. N.L. gen. masc. n. chengjingii, of Professor Jing Cheng, a famous medical biophysics expert, for his important achievements and innovations in the research of biochips).
Basonym: Microbacterium chengjingii Zhou et al. 2021
The description is as given by Zhou et al. (2021) with following changes. The genome size of the type strain is approximately 3.62 Mbp, and its genomic G+C content is 62.0%. The type strain is HY60T (= CGMCC 1.17468T = GDMCC 1.1951T = KACC 22102T).
Description of Paramicrobacterium fandaimingii comb. nov.
Paramicrobacterium fandaimingii (fan.dai.ming'i.i. N.L. gen. masc. n. fandaimingii, of Professor Daiming Fan, a well-known gastroenterologist, for his excellent research in clinical and basic research of digestive diseases and integrated medical theory).
Basonym: Microbacterium fandaimingii Zhou et al. 2021
The description is as given by Zhou et al. (2021) with following changes. The genome size of the type strain is approximately 3.62 Mbp, and its genomic G+C content is 63.3%. The type strain is HY82T (= CGMCC 1.17469T = GDMCC 1.1949T = KACC 22101T).
Description of Microbacterium aurugineum sp. nov.
Microbacterium aurugineum (au.ru.gi'ne.um. L. neut. adj. aurugineum yellowish).
Cells are Gram-stain-positive, strictly aerobic, and short rods (0.6–0.9 x 1.1–1.7 μm). Non-motile. Catalase-positive and oxidase-negative. Cells grow well on MA, R2A, and TSA and moderately on NA. No diffusible pigments are produced. Colonies are yellow in color and reach 0.5–1.0 mm in diameter after incubation on MA for 3 days at 30°C. The temperature and pH for growth are 10–40°C (optimum, 30°C) and pH 5.0–9.0 (optimum, pH 7.0). Salt tolerance for growth is 0–7% (w/v) NaCl (optimum, 1%). Nitrate is not reduced to nitrite. Esculin degradation and gelatin hydrolysis are observed. Glucose fermentation and activities of arginine dihydrolase and urease are absent. Cells are positive for assimilation of N-acetylglucosamine L-arabinose, gluconate, D-glucose, malate, D-maltose, D-mannitol, D-mannose, and phenylacetate (weak). Activities of N-acetyl-β-glucosamidase, acid phosphatase, alkaline phosphatase, esterase (C4), esterase lipase (C8), α-fucosidase, β-galactosidase, α-glucosidase, β-glucosidase, β-glucuronidase (weak), lipase (C14), leucine arylamidase, α-mannosidase, naphthol-AS-BI-phosphohydrolase, and valine arylamidase are present. Acid is produced from D-arabinose, L-arabinose, arbutin, D-cellobiose, D-fructose, D-galactose, gentibiose, gluconate, D-glucose, glycerol, D-maltose, D-mannitol, D-mannose, salicin, sucrose, D-trehalose, D-turanose, and D-xylose. Acid production from D-melezitose, methyl-α-D-glucoside, L-rhamnose, and D-ribose is variable depending on the strains. The diamino acid in the cell-wall peptidoglycan is D-ornithine. The major menaquinone is MK-10, with small amounts of MK-11 and MK-12. The polar lipids contain diphosphatidylglycerol, phosphatidylglycerol, and an unidentified glycolipid. The major cellular fatty acids (>10% of the total) are anteiso-C15:0, anteiso-C17:0, iso-C15:0, and iso-C16:0.
The type strain KSW4-10T (= KACC 22272T = DSM 112583T) was isolated from dried seaweed in Gwakji Beach in Jeju, Republic of Korea. The genome size of the type strain is approximately 3.63 Mbp, and its genomic G+C content is 68.2%. Additional strains, KSW4-16 (= KACC 22273) and SSW1-7 (= KACC 22274), were isolated from dried seaweeds collected from Gwakji and Samyang Beaches in Jeju, Republic of Korea, respectively.
Description of Microbacterium croceum sp. nov.
Microbacterium croceum (cro.ce'um. L. neut. adj. croceum, saffron-colored, yellow, golden, referring to the pale-yellow color of colonies).
Cells are Gram-stain-positive, strictly aerobic, and short rods (0.4–0.6 x 0.7–1.4 μm). Non-motile. Catalase-positive and oxidase-negative. Cells grow well on MA, R2A, and TSA and moderately on NA. No diffusible pigments are produced. Colonies are yellow in color and reach 1.0 mm in diameter after incubation on MA for 3 days at 30°C. The temperature and pH for growth are 10–37°C (optimum, 30°C) and pH 4.0–9.0 (optimum, pH 7.0). Salt tolerance for growth is 0–6% (w/v) NaCl (optimum, 1%). Nitrate is not reduced to nitrite. Esculin degradation is observed. Glucose fermentation, gelatin hydrolysis, and activities of arginine dihydrolase and urease are absent. Cells are positive for assimilation of L-arabinose, D-glucose, gluconate, malate, D-maltose, D-mannitol, D-mannose, and phenylacetate (weak). Activities of N-acetyl-β-glucosamidase, acid phosphatase, alkaline phosphatase, α-chymotrypsin, cystine arylamidase, esterase (C4), esterase lipase (C8), α-fucosidase (weak), α-galactosidase, β-galactosidase, α-glucosidase, β-glucosidase, β-glucuronidase (weak), lipase (C14), leucine arylamidase, α-mannosidase (weak), naphthol-AS-BI-phosphohydrolase, trypsin, and valine arylamidase are present. Acid is produced from D-arabinose, L-arabinose, D-cellobiose, D-fructose, L-fucose, D-galactose, gentibiose, gluconate, D-glucose, glycerol, D-lyxose, D-maltose, D-mannitol, D-mannose, D-melezitose, methyl-α-D-glucoside, L-rhamnose, D-ribose, salicin, sucrose, D-trehalose, D-turanose, and D-xylose. The diamino acid in the cell-wall peptidoglycan is D-ornithine. The major menaquinone is MK-10, with small amount of MK-11. The polar lipids contain diphosphatidylglycerol, phosphatidylglycerol, an unidentified glycolipid, and an unidentified lipid. The major cellular fatty acids (>10% of the total) are anteiso-C15:0, anteiso-C17:0, and iso-C16:0.
The type strain SSW1-49T (= KACC 22275T = DSM 112581T) was isolated from dried seaweed in Samyang Beach in Jeju, Republic of Korea. The genome size is approximately 3.68 Mbp, and its genomic G+C content is 68.4%.
Description of Microbacterium galbinum sp. nov.
Microbacterium galbinum (gal.bi'num. L. neut. adj. galbinum greenish yellow).
Cells are Gram-stain-positive, strictly aerobic, and short rods (0.4–0.7 x 1.4–1.6 μm). Non-motile. Catalase-positive and oxidase-negative. Cells grow well on MA, R2A, and TSA and moderately on NA. No diffusible pigments are produced. Colonies are greenish yellow in color and reach 0.5–1.0 mm in diameter after incubation on MA for 3 days at 30°C. The temperature and pH for growth are 10–37°C (optimum, 30°C) and pH 4.0–9.0 (optimum, pH 7.0). Salt tolerance for growth is 0–6% (w/v) NaCl (optimum, 1% NaCl). Nitrate is not reduced to nitrite. Esculin degradation is observed. Glucose fermentation and activities of arginine dihydrolase and urease are absent. Cells are positive for assimilation of L-arabinose, gluconate, D-glucose, malate, D-maltose, D-mannitol, and D-mannose. Activities of N-acetyl-β-glucosamidase, acid phosphatase, alkaline phosphatase, cystine arylamidase (weak), esterase (C4), esterase lipase (C8), α-galactosidase, β-galactosidase, α-glucosidase, β-glucosidase, lipase (C14), leucine arylamidase, α-mannosidase, naphthol-AS-BI-phosphohydrolase, trypsin, and valine arylamidase are present. Acid is produced from D-arabinose, L-arabinose, D-cellobiose, D-fructose, L-fucose, D-galactose, gentibiose, gluconate, D-glucose, glycerol, D-lyxose, D-maltose, D-mannitol, D-mannose, D-melezitose, D-melibiose, methyl-α-D-glucoside, salicin, sucrose, D-trehalose, D-turanose, and D-xylose. Gelatin hydrolysis and acid production from N-acetylglucosamine are variable depending on the strains. The diamino acid in the cell wall peptidoglycan is D-ornithine. The major menaquinone is MK-10, with small amount of MK-11. The polar lipids contain diphosphatidylglycerol, phosphatidylglycerol, and an unidentified glycolipid. The major cellular fatty acids (>10% of the total) are anteiso-C15:0, anteiso-C17:0, and iso-C16:0.
The type strain KSW2-24T (= KACC 22276T = DSM 112584T) was isolated from dried seaweed in Gwakji Beach in Jeju, Republic of Korea. The genome size of the type strain is approximately 3.57 Mbp, and its genomic G+C content is 69.2%. Additional strains, KSW4-6 (= KACC 22277) and SSW1-36 (= KACC 22278), were isolated from dried seaweeds collected from Gwakji and Samyang Beaches in Jeju, Republic of Korea, respectively.
Description of Microbacterium sufflavum sp. nov.
Microbacterium sufflavum (suf.fla'vum. L. neut. adj. sufflavum, light yellow).
Cells are Gram-stain-positive, strictly aerobic, and short rods (0.7–0.9 x 1.3–2.0 μm). Non-motile. Catalase-positive and oxidase-negative. Cells grow well on MA, R2A, and TSA and moderately on NA. No diffusible pigments are produced. Colonies are yellow in color and reach 0.5–1.2 mm in diameter after incubation on MA for 3 days at 30°C. The temperature and pH for growth are 10–45°C (optimum, 30°C) and pH 4.0–9.0 (optimum, pH 7.0). Salt tolerance for growth is 0–7% (w/v) NaCl (optimum, 1% NaCl). Nitrate is not reduced to nitrite. Esculin degradation is observed. Glucose fermentation, gelatin hydrolysis, and activities of arginine dihydrolase and urease are absent. Cells are positive for assimilation of N-acetylglucosamine, gluconate, D-glucose, malate D-maltose, D-mannitol, and D-mannose. Activities of N-acetyl-β-glucosamidase, acid phosphatase, alkaline phosphatase, esterase (C4), esterase lipase (C8) (weak), α-fucosidase, α-glucosidase, β-glucosidase, lipase (C14), leucine arylamidase, α-mannosidase, naphthol-AS-BI-phosphohydrolase, and valine arylamidase are present. Acid is produced from D-arabinose, D-cellobiose, D-fructose, D-galactose, gentibiose, gluconate, D-glucose, glycerol, D-lyxose, D-maltose, D-mannitol, D-mannose, D-melezitose, methyl-α-D-glucoside, L-rhamnose, sucrose, D-trehalose, D-turanose, and D-xylitol. Acid produced from N-acetylglucosamine is variable depending on the strains. The diamino acid in the cell wall peptidoglycan is D-ornithine. The major menaquinone is MK-10. The polar lipids contain diphosphatidylglycerol, phosphatidylglycerol, and an unidentified glycolipid. The major cellular fatty acids (>10% of the total) are anteiso-C15:0, anteiso-C17:0, and iso-C16:0.
The type strain SSW1-47T (= KACC 22279T = DSM 112582T) was isolated from seaweed in Samyang Beach in Jeju, Republic of Korea. The genome size of the type strain is approximately 3.63 Mbp, and its genomic G+C content is 71.0%. An additional strain, SSW1-51 (= KACC 22280), was isolated from the same source.
Data availability statement
The datasets presented in this study can be found in online repositories. The names of the repository/repositories and accession number(s) can be found below: https://www.ncbi.nlm.nih.gov/genbank/, MW703964-MW703972, MZ701896, MZ433296-MZ433298, CP078075-CP078078, JAWXG000000000-JAWXN000000000, JALPCF000000000-JALPCG000000000.
Author contributions
SL: Data curation, Formal analysis, Investigation, Methodology, Writing – original draft, Writing – review & editing. HY: Data curation, Formal analysis, Investigation, Methodology, Software, Writing – review & editing. IK: Writing – review & editing, Project administration, Supervision.
Funding
The author(s) declare financial support was received for the research, authorship, and/or publication of this article. This work was performed by financial support of BioPS CO., Ltd.
Acknowledgments
The authors are grateful to Hanna Choi and Dr. Ji-Seon Kim for preparation and analysis of cellular fatty acids and Professor Aharon Oren (The Hebrew University of Jerusalem, Israel) for help in preparing species names.
Conflict of interest
SL was employed by IK.
The remaining authors declare that the research was conducted in the absence of any commercial or financial relationships that could be construed as a potential conflict of interest.
Publisher's note
All claims expressed in this article are solely those of the authors and do not necessarily represent those of their affiliated organizations, or those of the publisher, the editors and the reviewers. Any product that may be evaluated in this article, or claim that may be made by its manufacturer, is not guaranteed or endorsed by the publisher.
Supplementary material
The Supplementary Material for this article can be found online at: https://www.frontiersin.org/articles/10.3389/fmicb.2023.1299950/full#supplementary-material
References
Alves, A., Correia, A., Igual, J. M., and Trujillo, M. E. (2014). Microbacterium endophyticum sp. nov. and Microbacterium halimionae sp. nov., endophytes isolated from the salt-marsh plant Halimione portulacoides and emended description of the genus Microbacterium. Syst. Appl. Microbiol. 37, 474–479. doi: 10.1016/j.syapm.2014.08.004
Alves, A., Riesco, R., Correia, A., and Trujillo, M. E. (2015). Microbacterium proteolyticum sp. nov. isolated from roots of Halimione portulacoides. Int. J. Syst. Evol. Microbiol. 65, 1794–1798. doi: 10.1099/ijs.0.000177
Amaral, G. R. S., Dias, G. M., Wellington-Oguri, M., Chimetto, L., Campeão, M. E., Thompson, F. L., et al. (2014). Genotype to phenotype: identification of diagnostic vibrio phenotypes using whole genome sequences. Int. J. Syst. Evol. Microbiol. 64, 357–365. doi: 10.1099/ijs.0.057927-0
Anand, S., Bala, K., Saxena, A., Schumann, P., and Lal, R. (2012). Microbacterium amylolyticum sp. nov., isolated from soil from an industrial waste site. Int. J. Syst. Evol. Microbiol. 62, 2114–2120. doi: 10.1099/ijs.0.034439-0
Anandham, R., Tamura, T., Hamada, M., Weon, H. Y., Kim, S. J., Kim, Y. S., et al. (2011). Microbacterium suwonense sp. nov., isolated from cow dung. J. Microbiol. 49, 852–856. doi: 10.1007/s12275-011-1036-y
Behrendt, U., Ulrich, A., and Schumann, P. (2001). Description of Microbacterium foliorum sp. nov. and Microbacterium phyllosphaerae sp. nov., isolated from the phyllosphere of grasses and the surface litter after mulching the sward, and reclassification of Aureobacterium resistens (Funke et al. 1998) as Microbacterium resistens comb. nov. Int. J. Syst. Evol. Microbiol. 51, 1267–1276. doi: 10.1099/00207713-51-4-1267
Bellassi, P., Fontana, A., Callegari, M. L., Cappa, F., and Morelli, L. (2021). Microbacterium paulum sp. nov., isolated from microfiltered milk. Int. J. Syst. Evol. Microbiol. 71, 5119. doi: 10.1099/ijsem.0.005119
Brennan, N., Brown, R., Goodfellow, M., Ward, A. C., Beresford, T. P., Vancanneyt, M., et al. (2001). Microbacterium gubbeenense sp. nov., from the surface of a smear-ripened cheese. Int. J. Syst. Evol. Microbiol. 51, 1969–1976. doi: 10.1099/00207713-51-6-1969
Brosius, J., Palmer, M. L., Kennedy, P. J., and Noller, H. F. (1978). Complete nucleotide sequence of a 16S ribosomal RNA gene from Escherichia coli. Proc.Natl. Acad. Sci. USA. 75, 4801–4805. doi: 10.1073/pnas.75.10.4801
Carro, L., Nouioui, I., Sangal, V., Meier-Kolthoff, J. P., Trujillo, M. E., Montero-Calasanz, M. D. C., et al. (2018). Genome-based classification of micromonosporae with a focus on their biotechnological and ecological potential. Sci. Rep. 8, 525. doi: 10.1038/s41598-017-17392-0
Chatelain, R., and Second, L. (1966). Taxonomie numérique de quelques Brevibacterium. Annales de l'Institut Pasteur (Paris) 111, 630–644.
Chen, M. S., Li, F., Yan, X. R., and Tuo, L. (2020). Microbacterium excoecariae sp. nov., a novel endophytic actinobacterium isolated from bark of Excoecaria agallocha Linn. Int. J. Syst. Evol. Microbiol. 70, 6235–6239. doi: 10.1099/ijsem.0.004523
Chen, X., Li, G. D., Li, Q. Y., Xu, F. J., Jiang, C. L., Han, L., et al. (2016). Microbacterium faecale sp. nov., isolated from the faeces of Columba livia. Int. J. Syst. Evol. Microbiol. 66, 4445–4450. doi: 10.1099/ijsem.0.001372
Cheng, L. J., Ming, H., Zhao, Z. L., Ji, W. L., Zhang, L. Y., Li, L. Y., et al. (2019). Microbacterium ureisolvens sp. nov., isolated from a Yellow River sample. Int. J. Syst. Evol. Microbiol.69, 560–566. doi: 10.1099/ijsem.0.003203
Cho, S. J., and Lee, S. S. (2017). Microbacterium rhizosphaerae sp. nov., isolated from a Ginseng field, South Korea. Antonie van Leeuwenhoek 110, 11–18. doi: 10.1007/s10482-016-0768-4
Chun, J., and Rainey, F. A. (2014). Integrating genomics into the taxonomy and systematics of the Bacteria and Archaea. Int. J. Syst. Evol. Microbiol. 64, 316–324. doi: 10.1099/ijs.0.054171-0
Clermont, D., Diard, S., Bouchier, C., Vivier, C., Bimet, F., Motreff, L., et al. (2009). Microbacterium binotii sp. nov., isolated from human blood. Int. J. Syst. Evol. Microbiol. 59, 1016–1022. doi: 10.1099/ijs.0.003160-0
Collins, M. D., Jones, D., Keddie, R. M., Kroppenstedt, R. M., and Schleifer, K. H. (1983). Classification of some coryneform bacteria in a new genus Aureobacterium. Syst. Appl. Microbiol. 4, 236–252. doi: 10.1016/S0723-2020(83)80053-8
Dias, F., and Bhat, J. V. (1962). A new levan producing bacterium, Corynebacterium laevaniformans nov. spec. Antonie van Leeuwenhoek 28, 63–72. doi: 10.1007/BF02538722
Dong, K., Yang, J., Lu, S., Pu, J., Lai, X.-H., Jin, D., et al. (2020). Microbacterium wangchenii sp. nov., isolated from faeces of Tibetan gazelles (Procapra picticaudata) on the Qinghai-Tibet Plateau. Int. J. Syst. Evol. Microbiol. 70, 1307–1314. doi: 10.1099/ijsem.0.003912
Evtushenko, L. I. (2012). “Family XI. Microbacteriaceae Park, Suzuki, Yim, Lee, Kim, Yoon, Kim, Kho, Goodfellow and Komagata 1995, 418VP (Effective publication: Park, Suzuki, Yim, Lee, Kim, Yoon, Kim, Kho, Goodfellow and Komagata 1993, 312.) emend. Rainey, Ward-Rainey and Stackebrandt 1997, 485,” in Bergy's Mannual Systematic Bacteriology, Vol. 5, The Actinobacteria, eds M. Goodfellow, P. Kämpfer, H.-J. Busse, M.E. Trujillo, K. Suzuki, W. Ludwig, et al. (New York, NY: Springer), 807–813.
Felsenstein, J. (1985). Confidence limits on phylogenies, an approach using the bootstrap. Evolution 39, 783–791. doi: 10.2307/2408678
Fidalgo, C., Riesco, R., Henriques, I., Trujillo, M. E., and Alves, A. (2016). Microbacterium diaminobutyricum sp. nov., isolated from Halimione portulacoides, which contains diaminobutyric acid in its cell wall, and emended description of the genus Microbacterium. Int. J. Syst. Evol. Microbiol. 66, 4492–4500. doi: 10.1099/ijsem.0.001379
Gao, J. L., Sun, P., Wang, X. M., Lv, F. Y., and Sun, J. G. (2017). Microbacterium zeae sp. nov., an endophytic bacterium isolated from maize stem. Antonie Van Leeuwenhoek 110, 697–704. doi: 10.1007/s10482-017-0837-3
Gao, M., Wang, M., Zhang, Y. C., Zou, X. L., Xie, L. Q., Hu, H. Y., et al. (2013). Microbacterium neimengense sp. nov., isolated from the rhizosphere of maize. Int. J. Syst. Evol. Microbiol. 63, 236–240. doi: 10.1099/ijs.0.038166-0
Goris, J., Konstantinidis, K. T., Klappenbach, J. A., Coenye, T., Vandamme, P., and Tiedje, J. M. (2007). DNA–DNA hybridization values and their relationship to whole-genome sequence similarities. Int. J. Syst. Evol. Microbiol. 57, 81–91. doi: 10.1099/ijs.0.64483-0
Heo, J., Cho, H., Kim, M. A., Hamada, M., Tamura, T., Saitou, S., et al. (2020). Microbacterium protaetiae sp. nov., isolated from gut of larva of Protaetia brevitarsis seulensis. Int. J. Syst. Evol. Microbiol. 70, 2226–2232. doi: 10.1099/ijsem.0.003967
Imai, K., Takeuchi, M., and Banno, I. (1984). Reclassification of “Flavobacterium arborescens” (Frankland and Frankland) Bergey et al. in the genus Microbacterium (Orla-Jensen) Collins et al., as Microbacterium arborescens comb. nov., nom. rev. Curr. Microbiol. 11, 281–284. doi: 10.1007/BF01567386
Jukes, T. H., and Cantor, C. R. (1969). “Evolution of protein molecules,” in Mammalian Protein Metabolism, ed. H. N. Munro. (New York, NY: Academic Press), 21–132.
Kageyama, A., Matsuo, Y., Kasai, H., Shizuri, Y., Omura, S., and Takahashi, Y. (2007c). Microbacterium awajiense sp. nov., Microbacterium fluvii sp. nov. and Microbacterium pygmaeum sp. nov. Actinomycetologica 22, 1–5. doi: 10.3209/saj.SAJ220101
Kageyama, A., Takahashi, Y., Matsuo, Y., Adachi, K., Kasai, H., Shizuri, Y., et al. (2007a). Microbacterium flavum sp. nov. and Microbacterium lacus sp. nov., isolated from marine environments. Actinomycetologica 21, 53–58. doi: 10.3209/saj.SAJ210201
Kageyama, A., Takahashi, Y., and Omura, S. (2006). Microbacterium deminutum sp. nov., Microbacterium pumilum sp. nov. and Microbacterium aoyamense sp. nov. Int. J. Syst. Evol. Microbiol. 56, 2113–2117. doi: 10.1099/ijs.0.64236-0
Kageyama, A., Takahashi, Y., and Omura, S. (2007b). Microbacterium terricolae sp. nov., isolated from soil in Japan. J Gen. Appl. Microbiol. 53, 1–5. doi: 10.2323/jgam.53.1
Kämpfer, P., Rekha, P. D., Schumann, P., Arun, A. B., Young, C.-C., Chen, W.-M., et al. (2011). Microbacterium arthrosphaerae sp. nov., isolated from the faeces of the pill millipede Arthrosphaera magna Attems. Int. J. Syst. Evol. Microbiol., 61, 1334–1337. doi: 10.1099/ijs.0.026401-0
Kämpfer, P., Schäfer, J., Lodders, N., and Martin, K. (2012). Microbacterium murale sp. nov., isolated from an indoor wall. Int. J. Syst. Evol. Microbiol. 62, 2669–2673. doi: 10.1099/ijs.0.037705-0
Karojet, S., Kunz, S., and van Dongen, J. T. (2012). Microbacterium yannicii sp. nov., isolated from Arabidopsis thaliana roots. Int. J. Syst. Evol. Microbiol. 62, 822–826. doi: 10.1099/ijs.0.026955-0
Kaur, G., Mual, P., Kumar, N., Verma, A., Kumar, A., Krishnamurthi, S., et al. (2016). Microbacterium aureliae sp. nov., a novel actinobacterium isolated from Aurelia aurita, the moon jellyfish. Int. J. Syst. Evol. Microbiol. 66, 4665–4670. doi: 10.1099/ijsem.0.001407
Kim, K. K., Park, H. Y., Park, W., Kim, I. S., and Lee, S. T. (2005). Microbacterium xylanilyticum sp. nov., a xylan-degrading bacterium isolated from a biofilm. Int. J. Syst. Evol. Microbiol. 55, 2075–2079. doi: 10.1099/ijs.0.63706-0
Kim, Y. J., Kim, M. K., Bui, T. P., Kim, H. B., Srinivasan, S., and Yang, D. C. (2010). Microbacterium ginsengiterrae sp. nov., a beta-glucosidase-producing bacterium isolated from soil of a ginseng field. Int. J. Syst. Evol. Microbiol. 60, 2808–2812. doi: 10.1099/ijs.0.015784-0
Kim, Y. J., Roh, S. W., Jung, M. J., Kim, M. S., Park, E. J., and Bae, J. W. (2011). Microbacterium mitrae sp. nov., isolated from salted turban shell. Int. J. Syst. Evol. Microbiol. 61, 399–403. doi: 10.1099/ijs.0.021519-0
Kook, M., Son, H. M., and Yi, T. H. (2014). Microbacterium kyungheense sp. nov. and Microbacterium jejuense sp. nov., isolated from salty soil. Int. J. Syst. Evol. Microbiol. 64, 2267–2273. doi: 10.1099/ijs.0.054973-0
Kroppenstedt, R. M. (1985). “Fatty acid and menaquinone analysis of actinomycetes and related organisms,” in Chemical Methods in Bacterial Systematics, eds. M. Goodfellow, D. E. Minnikin. (London: Academic Press), 173–199.
Kumari, P., Bandyopadhyay, S., and Das, S. K. (2013). Microbacterium oryzae sp. nov., an actinobacterium isolated from rice field soil. Int. J. Syst. Evol. Microbiol. 63, 2442–2449. doi: 10.1099/ijs.0.046870-0
Laffineur, K., Avesani, V., Cornu, G., Charlier, J., Janssens, M., Wauters, G., et al. (2003). Bacteremia due to a novel Microbacterium species in a patient with leukemia and description of Microbacterium paraoxydans sp. nov. J. Clin. Microbiol. 41, 2242–2246. doi: 10.1128/JCM.41.5.2242-2246.2003
Lal, D., Gupta, S. K., Schumann, P., and Lal, R. (2010). Microbacterium lindanitolerans sp. nov., isolated from hexachlorocyclohexane-contaminated soil. Int. J. Syst. Evol. Microbiol. 60, 2634–2638. doi: 10.1099/ijs.0.017699-0
Lee, S. D. (2007). Labedella gwakjiensis gen. nov., sp. nov., a novel actinomycete of the family Microbacteriaceae. Int. J. Syst. Evol. Microbiol. 57, 2498–2502. doi: 10.1099/ijs.0.64591-0
Lee, S. D., and Kim, I. S. (2023). Microbacterium tenebrionis sp. nov. and Microbacterium allomyrinae sp. nov., isolated from larvae of Tenebrio molitor L. and Allomyrina dichotoma, respectively. Int. J. Syst. Evol. Microbiol. 73, 5729. doi: 10.1099/ijsem.0.005729
Lee, S. D., Kim, I. S, Verbarg, S., and Joung, Y. (2020). Antrihabitans stalactiti gen. nov., sp. nov., a new member of the family Nocardiaceae isolated from a cave. Int. J. Syst. Evol. Microbiol. 70, 5503–5511. doi: 10.1099/ijsem.0.004444
Lenchi, N., Anzil, A., Servais, P., Kebbouche-Gana, S., Gana, M. L., and Lliros, M. (2020). Microbacterium algeriense sp. nov., a novel actinobacterium isolated from Algerian oil production waters. Int. J. Syst. Evol. Microbiol. 70, 6044–6051. doi: 10.1099/ijsem.0.004434
Li, W. J., Chen, H. H., Kim, C. J., Park, D. J., Tang, S. K., Lee, J. C., et al. (2005). Microbacterium halotolerans sp. nov., isolated from a saline soil in the west of China. Int. J. Syst. Evol. Microbiol. 55, 67–70. doi: 10.1099/ijs.0.63239-0
Li, Y. R., Zhu, Z. N., Li, Y. Q., Xiao, M., Han, M. X., Wadaan, M. A. M., et al. (2018). Microbacterium halophytorum sp. nov., a novel endophytic actinobacterium isolated from halophytes. Int. J. Syst. Evol. Microbiol. 68, 3928–3934. doi: 10.1099/ijsem.0.003092
Lysenko, O. (1959). The occurrence of species of the genus Brevibacterium in insects. J. insect Path. 1, 34–42.
Madhaiyan, M., Poonguzhali, S., Lee, J. S., Lee, K. C., Saravanan, V. S., and Santhanakrishnan, P. (2010). Microbacterium azadirachtae sp. nov., a plant-growth-promoting actinobacterium isolated from the rhizoplane of neem seedlings. Int. J. Syst. Evol. Microbiol. 60, 1687–1692. doi: 10.1099/ijs.0.015800-0
Matsuyama, H., Kawasaki, K., Yumoto, I., and Shida, O. (1999). Microbacterium kitamiense sp. nov., a new polysaccharide-producing bacterium isolated from the wastewater of a sugar-beet factory. Int. J. Syst. Bacteriol. 49, 1353–1357. doi: 10.1099/00207713-49-4-1353
Mawlankar, R. R., Mual, P., Sonalkar, V. V., Thorat, M. N., Verma, A., Srinivasan, K., et al. (2015). Microbacterium enclense sp. nov., isolated from sediment sample. Int. J. Syst. Evol. Microbiol. 65, 2064–2070. doi: 10.1099/ijs.0.000221
Meier-Kolthoff, J. P., Auch, A. F., Klenk, H.-P., and Göker, M. (2013). Genome sequence-based species delimitation with confidence intervals and improved distance functions. BMC Bioinformat. 14, 60. doi: 10.1186/1471-2105-14-60
Meng, Y. C., Liu, H. C., Yang, L. L., Kang, Y. Q., Zhou, Y. G., and Cai, M. (2016). Microbacterium sorbitolivorans sp. nov., a novel member of Microbacteriaceae isolated from fermentation bed in pigpen. Int. J. Syst. Evol. Microbiol. 66, 5556–5561. doi: 10.1099/ijsem.0.001556
Minnikin, D. E., O'Donnell, A. G., Goodfellow, M., Alderson, G., Athalye, M., Schaal, A., et al. (1984). An integrated procedure for the extraction of bacterial isoprenoid quinones and polar lipids. J. Microbiol. Methods 2, 233–241. doi: 10.1016/0167-7012(84)90018-6
Minnikin, D. E., Patel, P. V., Alshamaony, L., and Goodfellow, M. (1977). Polar lipid composition in the classification of Nocardia and related bacteria. Int. J. Syst. Bacteriol. 27, 104–107. doi: 10.1099/00207713-27-2-104
Na, S. I., Kim, Y. O., Yoon, S. H., Ha, S. M., Baek, I., and Chun, J. (2018). UBCG: Up-to-date bacterial core gene set and pipeline for phylogenomic tree reconstruction. J. Microbiol. 56, 280–285. doi: 10.1007/s12275-018-8014-6
Nguyen, N. L., Kim, Y. J., Hoang, V. A., Min, J. W., Hwang, K. H., and Yang, D. C. (2015). Microbacterium panaciterrae sp. nov., isolated from the rhizosphere of ginseng. Int. J. Syst. Evol. Microbiol. 65, 927–933. doi: 10.1099/ijs.0.000041
Nicholson, A. C., Gulvik, C. A., Whitney, A. M., Bell, M. E., Holmes, B., Steigerwalt, A. G., et al. (2020). Division of the genus Chryseobacterium: Observation of discontinuities in amino acid identity values, a possible consequence of major extinction events, guides transfer of nine species to the genus Epilithonimonas, eleven species to the genus Kaistella, and three species to the genus Halpernia gen. nov., with description of Kaistella daneshvariae sp. nov. and Epilithonimonas vandammei sp. nov. derived from clinical specimens. Int. J. Syst. Evol. Microbiol. 70, 4432–4450. doi: 10.1099/ijsem.0.003935
Nishijima, M., Tazato, N., Handa, Y., Umekawa, N., Kigawa, R., Sano, C., et al. (2017). Microbacterium tumbae sp. nov., an actinobacterium isolated from the stone chamber of ancient tumulus. Int. J. Syst. Evol. Microbiol. 67, 1777–1783. doi: 10.1099/ijsem.0.001863
Nouioui, I., Carro, L., Garcia-Lopez, M., Meier-Kolthoff, J. P., Woyke, T., Kyrpides, N. C., et al. (2018). Genome-based taxonomic classification of the phylum Actinobacteria. Front. Microbiol. 9, 2007. doi: 10.3389/fmicb.2018.02007
Oren, A., Arahal, D. R., Göker, M., Moore, E. R. B., Rossello-Mora, R., and Sutcliffe, I. C. (2022). International code of nomenclature of prokaryotes. prokaryotic code (2022 Revision). Int. J. Syst. Evol. Microbiol. 73, 005585. doi: 10.1099/ijsem.0.005585
Parks, D. H., Imelfort, M., Skennerton, C. T., Hugenholtz, P., and Tyson, G. W. (2014). Assessing the quality of microbial genomes recovered from isolates, single cells, and metagenomes. Genome Res. 25, 1043–1055. doi: 10.1101/gr.186072.114
Peng, S., Dongying, L., Bingxin, Y., Mingjun, L., and Gehong, W. (2015). Microbacterium shaanxiense sp. nov., isolated from the nodule surface of soybean. Int. J. Syst. Evol. Microbiol. 65, 1437–1443. doi: 10.1099/ijs.0.000116
Richert, K., Brambilla, E., and Stackebrandt, E. (2007). The phylogenetic significance of peptidoglycan types: molecular analysis of the genera Microbacterium and Aureobacterium based upon sequence comparison of gyrB, rpoB, recA and ppk and 16S rRNA genes. Syst. Appl. Microbiol. 30, 102–108. doi: 10.1016/j.syapm.2006.04.001
Richter, M., and Roselló-Móra, R. (2009). Shifting the genomic gold standard for the prokaryotic species definition. Proc. Natl. Acad. Sci. USA. 106, 12196–19131. doi: 10.1073/pnas.0906412106
Riesco, R., Carro, L., Román-Ponce, B., Prieto, C., Blom, J., Klenk, H.-P., et al. (2018). Defining the species Micromonospora saelicesensis and Micromonospora noduli under the framework of genomics. Front Microbiol. 25, 1360. doi: 10.3389/fmicb.2018.01360
Rodriguez, R. L. M., and Konstantinidis, K. T. (2014). Bypassing cultivation to identify bacterial species. Microbe 9, 111–118. doi: 10.1128/microbe.9.111.1
Rosselló-Móra, R., Urdiain, M., and Lopez-Lopez, A. (2011). DNA-DNA hybridrization. Methods Microbiol. 38, 325–347. doi: 10.1016/B978-0-12-387730-7.00015-2
Salam, N., Jiao, J.-Y., Zhang, X.-T., and Li, W.-J. (2020). Update on the classification of higher ranks in the phylum Actinobacteria. Int. J. Syst. Evol. Microbiol. 70, 1331–1355. doi: 10.1099/ijsem.0.003920
Schippers, A., Bosecker, K., Spröer, C., and Schumann, P. (2005). Microbacterium oleivorans sp. nov. and Microbacterium hydrocarbonoxydans sp. nov., novel crude-oil-degrading Gram-positive bacteria. Int. J. Syst. Evol. Microbiol. 55, 655–660. doi: 10.1099/ijs.0.63305-0
Schumann, P., Rainey, F. A., Burghardt, J., Stackebrandt, E., and Weiss, N. (1999). Reclassification of Brevibacterium oxydans (Chatelain and Second 1966) as Microbacterium oxydans comb. nov. Int. J. Syst. Bacteriol. 49, 175–177. doi: 10.1099/00207713-49-1-175
Shivaji, S., Bhadra, B., Rao, R. S., Chaturvedi, P., Pindi, P. K., and Raghukumar, C. (2007). Microbacterium indicum sp. nov., isolated from a deep-sea sediment sample from the Chagos Trench, Indian Ocean. Int. J. Syst. Evol. Microbiol. 57, 1819–1822. doi: 10.1099/ijs.0.64782-0
Steinhaus, E. (1941). A study of the bacteria associated with thirty species of insects. J. Bacteriol. 42, 757–790. doi: 10.1128/jb.42.6.757-790.1941
Sutcliffe, I. C. (2015). Challenging the anthropocentric emphasis on phenotypic testing in prokaryotic species descriptions: rip it up and start again. Front. Genet. 6, 218. doi: 10.3389/fgene.2015.00218
Suzuki, K., and Hamada, M. (2012). “Genus I. Microbacterium Orla-Jensen 1919, 179AL emend. Takeuchi and Hatano 1998, 744VP,” in Bergy's Mannual Systematic Bacteriology, Vol. 5, The Actinobacteria, eds. M. Goodfellow, P. Kämpfer, H.-J. Busse, M.E. Trujillo, K. Suzuki, W. Ludwig, et al. (New York, NY: Springer), 814–855.
Takeuchi, M., and Hatano, K. (1998a). Proposal of six new species in the genus Microbacterium and transfer of Flavobacterium marinotypicum ZoBell and Upham to the genus Microbacterium as Microbacterium maritypicum comb. nov. Int. J. Syst. Bacteriol. 48, 973–982. doi: 10.1099/00207713-48-3-973
Takeuchi, M., and Hatano, K. (1998b). Union of the genera Microbacterium Orla-Jensen and Aureobacterium Collins et al. in a redefined genus Microbacterium. Int. J. Syst. Bacteriol. 48, 739–747. doi: 10.1099/00207713-48-3-739
Thompson, J. D., Gibson, T. J., Plewniak, F., Jeanmougin, F., and Higgins, D. G. (1997). The ClustalX windows interface: flexible strategies for multiple sequence alignment aided by quality analysis tools. Nucleic Acids Res. 24, 4876–4882. doi: 10.1093/nar/25.24.4876
Tian, Z., Yang, J., Lai, X. H., Pu, J., Jin, D., Luo, X., et al. (2021). Microbacterium caowuchunii sp. nov. and Microbacterium lushaniae sp. nov., isolated from plateau pika (Ochotona curzoniae) on the Qinghai-Tibet Plateau of PR China. Int. J. Syst. Evol. Microbiol. 71, 4662. doi: 10.1099/ijsem.0.004662
Uchida, K., and Aida, K. (1977). Acyl type of bacterial cell walls: its simple identification by a colorimetric method. J. Gen. Appl. Microbiol. 23, 249–260. doi: 10.2323/jgam.23.249
Vaz-Moreira, I., Lopes, A. R., Falsen, E., Schumann, P., Nunes, O. C., and Manaia, C. M. (2008). Microbacterium luticocti sp. nov., isolated from sewage sludge compost. Int. J. Syst. Evol. Microbiol. 58, 1700–1704. doi: 10.1099/ijs.0.65494-0
Wayne, L. G., Brenner, D. J., Colwell, R. R., Grimont, P. A. D., Kandler, O., et al. (1987). International Committee on Systematic Bacteriology. Report of the ad hoc committee on reconciliation of approaches to bacterial systematics. Int. J. Syst. Bacteriol. 37, 463–464. doi: 10.1099/00207713-37-4-463
Wirth, J. S., and Whitman, W. B. (2018). Phylogenomic analyses of a clade within the roseobacter group suggest taxonomic reassignments of species of the genera Aestuariivita, Citreicella, Loktanella, Nautella, Pelagibaca, Ruegeria, Thalassobius, Thiobacimonas and Tropicibacter, and the proposal of six novel genera. Int. J. Syst. Evol. Microbiol. 68, 2393–2411. doi: 10.1099/ijsem.0.002833
Wu, Y. H., Wu, M., Wang, C. S., Wang, X. G., Yang, J. Y., Oren, A., et al. (2008). Microbacterium profundi sp. nov., isolated from deep-sea sediment of polymetallic nodule environments. Int. J. Syst. Evol. Microbiol. 58, 2930–2934. doi: 10.1099/ijs.0.2008/000455-0
Xie, F., Niu, S., Lin, X., Pei, S., Jiang, L., Tian, Y., et al. (2021). Description of Microbacterium luteum sp. nov., Microbacterium cremeum sp. nov., and Microbacterium atlanticum sp. nov., three novel C50 carotenoid producing bacteria. J. Microbiol. 59, 886–897. doi: 10.1007/s12275-021-1186-5
Yacouba, A., Sissoko, S., Tchoupou Saha, O. F., Haddad, G., Dubourg, G., Gouriet, F., et al. (2022). Description of Acinetobacter ihumii sp. nov., Microbacterium ihumii sp. nov., and Gulosibacter massiliensis sp. nov., three new bacteria isolated from human blood. FEMS Microbiol. Lett. 369:1–8. doi: 10.1093/femsle/fnac038
Yan, L., Wang, J., Chen, Z., Guan, Y., and Li, J. (2015). Microbacterium nanhaiense sp. nov., an actinobacterium isolated from sea sediment. Int. J. Syst. Evol. Microbiol. 65, 3697–3702. doi: 10.1099/ijsem.0.000480
Yan, Z. F., Lin, P., Won, K. H., Yang, J. E., Li, C. T., Kook, M., et al. (2017). Microbacterium hibisci sp. nov., isolated from rhizosphere of mugunghwa (Hibiscus syriacus L.). Int. J. Syst. Evol. Microbiol. 67, 3564–3569. doi: 10.1099/ijsem.0.002167
Yokota, A., Takeuchi, M., Sakane, T., and Weiss, N. (1993b). Proposal of six new species in the genus Aureobacterium and transfer of Flavobacterium esteraromaticum Omelianski to the genus Aureobacterium as Aureobacterium esteraromaticum comb. nov. Int. J. Syst. Bacteriol. 43, 555–564. doi: 10.1099/00207713-43-3-555
Yokota, A., Takeuchi, M., and Weiss, N. (1993a). Proposal of two new species in the genus Microbacterium, Microbacterium dextranolyticum sp. nov. and Microbacterium aurum sp. nov. Int. J. Syst. Bacteriol. 43, 549–554. doi: 10.1099/00207713-43-3-549
Yoon, J. H., Schumann, P., Kang, S. J., Lee, C. S., Lee, S. Y., and Oh, T. K. (2009). Microbacterium insulae sp. nov., isolated from soil. Int. J. Syst. Evol. Microbiol. 59, 1738–1742. doi: 10.1099/ijs.0.007591-0
Yoon, S. H., Ha, S.-M., Kwon, S., Lim, J., Kim, Y., et al. (2017a). Introducing EzBioCloud, A taxonomically united database of 16S rRNA and whole genome assemblies. Int. J. Syst. Evol. Microbiol. 67, 1613–1617. doi: 10.1099/ijsem.0.001755
Yoon, S. H., Ha, S.-M., Lim, J., Kwon, S., and Chun, J. (2017b). A large-scale evaluation of algorithms to calculate average nucleotide identity. Antonie van Leeuwenhoek 110, 1281–1286. doi: 10.1007/s10482-017-0844-4
Young, C.-C., Busse, H.-J., Langer, S., Chu, J.-N., Schumann, P., Arun, A. B., et al. (2010). Microbacterium agarici sp. nov., Microbacterium humi sp. nov. and Microbacterium pseudoresistens sp. nov., isolated from the base of the mushroom Agaricus blazei. Int. J. Syst. Evol. Microbiol. 60, 854–860. doi: 10.1099/ijs.0.014092-0
Yu, L., Lai, Q., Yi, Z., Zhang, L., Huang, Y., Gu, L., et al. (2013). Microbacterium sediminis sp. nov., a psychrotolerant, thermotolerant, halotolerant and alkalitolerant actinomycete isolated from deep-sea sediment. Int. J. Syst. Evol. Microbiol. 63, 25–30. doi: 10.1099/ijs.0.029652-0
Zhang, L., Xi, L., Ruan, J., and Huang, Y. (2012). Microbacterium marinum sp. nov., isolated from deep-sea water. Syst. Appl. Microbiol. 35, 81–85. doi: 10.1016/j.syapm.2011.11.004
Zhang, W., Zhu, H. H., Yuan, M., Yao, Q., Tang, R., Lin, M., et al. (2010). Microbacterium radiodurans sp. nov., a UV radiation-resistant bacterium isolated from soil. Int. J. Syst. Evol. Microbiol. 60, 2665–2670. doi: 10.1099/ijs.0.017400-0
Zhang, Y., Ren, H., and Zhang, G. (2014). Microbacterium hydrothermale sp. nov., an actinobacterium isolated from hydrothermal sediment. Int. J. Syst. Evol. Microbiol. 64, 3508–3512. doi: 10.1099/ijs.0.061697-0
Zheng, J., Wittouck, S., Salvetti, E., Franz, C. M. A. P., Harris, H. M. B., Mattarelli, P., et al. (2020). A taxonomic note on the genus Lactobacillus: Description of 23 novel genera, emended description of the genus Lactobacillus Beijerinck 1901, and union of Lactobacillaceae and Leuconostocaceae. Int. J. Syst. Evol. Microbiol. 70, 2782–2858. doi: 10.1099/ijsem.0.004107
Zhi, X. Y., Li, W. J., and Stackebrandt, E. (2009). An update of the structure and 16S rRNA gene sequence-based definition of higher ranks of the class Actinobacteria, with the proposal of two new suborders and four new families and emended descriptions of the existing higher taxa. Int. J. Syst. Evol. Microbiol. 59, 589–608. doi: 10.1099/ijs.0.65780-0
Zhou, J., Huang, Y., Yang, J., Lai, X. H., Jin, D., Lu, S., et al. (2021). Microbacterium chengjingii sp. nov. and Microbacterium fandaimingii sp. nov., isolated from bat faeces of Hipposideros and Rousettus species. Int. J. Syst. Evol. Microbiol. 71, 4858. doi: 10.1099/ijsem.0.004858
Zhu, Q. H., Yang, C. L., Luo, X. X., Zhang, L. L., and Xia, Z. F. (2021). Microbacterium karelineae sp. nov. isolated from a halophyte plant in the Taklamakan desert. Int. J. Syst. Evol. Microbiol. 71, 4629. doi: 10.1099/ijsem.0.004629
Zhu, Z. N., Li, Y. R., Li, Y. Q., Xiao, M., Han, M. X., Wadaan, M. A. M., et al. (2019). Microbacterium suaedae sp. nov., isolated from Suaeda aralocaspica. Int. J. Syst. Evol. Microbiol. 69, 411–416. doi: 10.1099/ijsem.0.003162
Zlamala, C., Schumann, P., Kämpfer, P., Valens, M., Rosselló-Mora, R., Lubitz, W., et al. (2002). Microbacterium aerolatum sp. nov., isolated from the air in the 'Virgilkapelle' in Vienna. Int. J. Syst. Evol. Microbiol. 52, 1229–1234. doi: 10.1099/00207713-52-4-1229
Keywords: Microbacterium, genome sequencing, core genome analysis, seaweeds, four new species, Microbacteriaceae, Paramicrobacterium gen. nov., overall phylogenomic clustering
Citation: Lee SD, Yang HL and Kim IS (2023) Four new Microbacterium species isolated from seaweeds and reclassification of five Microbacterium species with a proposal of Paramicrobacterium gen. nov. under a genome-based framework of the genus Microbacterium. Front. Microbiol. 14:1299950. doi: 10.3389/fmicb.2023.1299950
Received: 23 September 2023; Accepted: 15 November 2023;
Published: 18 December 2023.
Edited by:
Iain Sutcliffe, Northumbria University, United KingdomReviewed by:
Imen Nouioui, German Collection of Microorganisms and Cell Cultures GmbH (DSMZ), GermanyKenichiro Suzuki, Tokyo University of Agriculture, Japan
Copyright © 2023 Lee, Yang and Kim. This is an open-access article distributed under the terms of the Creative Commons Attribution License (CC BY). The use, distribution or reproduction in other forums is permitted, provided the original author(s) and the copyright owner(s) are credited and that the original publication in this journal is cited, in accordance with accepted academic practice. No use, distribution or reproduction is permitted which does not comply with these terms.
*Correspondence: Soon Dong Lee, c2QubGVlQGJpb3BzLmNvLmty
†These authors have contributed equally to this work