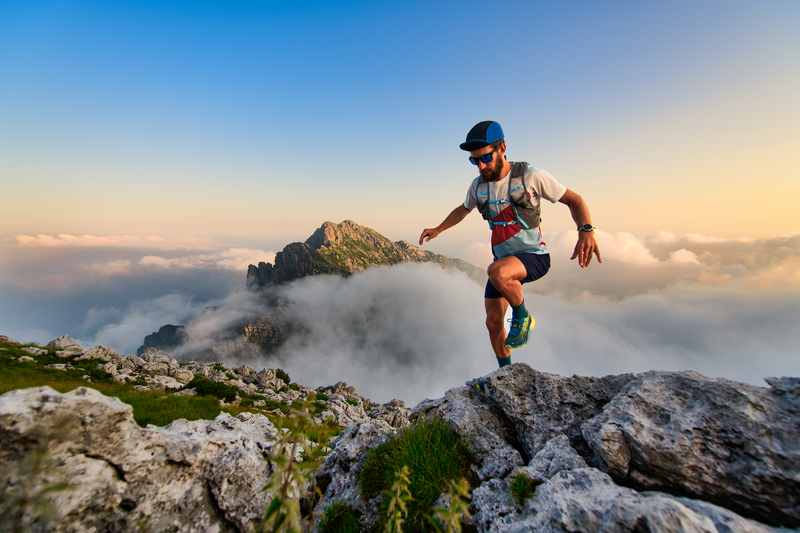
95% of researchers rate our articles as excellent or good
Learn more about the work of our research integrity team to safeguard the quality of each article we publish.
Find out more
MINI REVIEW article
Front. Microbiol. , 22 September 2023
Sec. Infectious Agents and Disease
Volume 14 - 2023 | https://doi.org/10.3389/fmicb.2023.1281303
This article is part of the Research Topic New Insights in the Microbe-Vector Interaction View all 8 articles
Q fever is a zoonotic disease caused by Coxiella burnetii, an obligatory intracellular bacterial pathogen. Like other intracellular pathogens, C. burnetii is able to survive and reproduce within host cells by manipulating host cellular processes. In particular, the relationship between C. burnetii infection and host autophagy, a cellular process involved in degradation and recycling, is of great interest due to its intricate nature. Studies have shown that autophagy can recognize and target intracellular pathogens such as Legionella and Salmonella for degradation, limiting their replication and promoting bacterial clearance. However, C. burnetii can actively manipulate the autophagic pathway to create an intracellular niche, known as the Coxiella-containing vacuole (CCV), where it can multiply and evade host immune responses. C. burnetii promotes the fusion of CCVs with lysosomes through mechanisms involving virulence factors such as Cig57 and CvpF. This review summarizes the latest findings on the dynamic interaction between host autophagy and C. burnetii infection, highlighting the complex strategies employed by both the bacterium and the host. A better understanding of these mechanisms could provide important insights into the development of novel therapeutic interventions and vaccine strategies against C. burnetii infections.
Coxiella burnetii, known as the causative agent of Q fever, is an exceptionally infectious, intracellular bacterium that is regarded as a potential bioterrorism agent due to its highly contagious nature (Shaw and Voth, 2019). This gram-negative bacterium was first identified in the 1930s and was later named after Dr. Frank Burnet and Dr. Cox who were the first to describe it (Minnick and Raghavan, 2011). C. burnetii infection in humans ranges from asymptomatic to acute or chronic Q fever. Asymptomatic cases are common, and the infection is often discovered incidentally through serological testing. Acute Q fever is characterized by sudden onset of flu-like symptoms such as high fever, headache, muscle aches, fatigue, and cough (Ullah et al., 2022). The diagnosis is confirmed by serological testing, culture, and PCR (Ullah et al., 2022). In severe cases, acute hepatitis, pneumonia, or endocarditis may occur (Ullah et al., 2022). Chronic Q fever develops in less than 5% of infected individuals, usually in those with pre-existing heart valve defects, vascular disorders, or immunosuppression (Espana et al., 2020). Chronic Q fever can cause endocarditis, hepatitis, osteomyelitis, or vascular infections and requires prolonged antibiotic therapy and sometimes surgery (Espana et al., 2020).
Research on C. burnetii has increased in recent years, mainly due to its biodefense potential and its unique features as an intracellular pathogen. C. burnetii has a small genome (2.0 Mb) that encodes for approximate 1,800 proteins, including type IV secretion system (T4SS), lipopolysaccharides, and other virulence factors (Beare et al., 2006; Abou Abdallah et al., 2022). It can survive and replicate within the phagolysosome of macrophages and modulate host cell pathways to avoid immune recognition and clearance. C. burnetii also has the ability to form a dormant spore-like form, known as a small cell variant, which has increased virulence and persistence in host tissues (Sandoz et al., 2014). Understanding the molecular mechanisms of C. burnetii pathogenesis and host immune responses is crucial for developing effective treatment and prevention strategies.
Coxiella burnetii is known to have a wide range of hosts, including mammals, birds, and arthropods, which serve as vectors for its transmission to human and animal populations (Celina and Cerny, 2022). Many different species of ticks, fleas, and lice have been identified as potential vectors for C. burnetii, and transmission also occurs through direct contact with infected animals or ingestion of contaminated food products (Celina and Cerny, 2022). Ticks are considered the primary vector host for C. burnetii, with the most common species being Dermacentor variabilis and Rhipicephalus sanguineus (Cabrera et al., 2022; Celina and Cerny, 2022). These ticks are known to feed on many different mammalian and avian hosts, and they can transmit C. burnetii to their hosts through their saliva. Fleas are another vector host for C. burnetii, with the most common species being Xenopsylla cheopis, which is known to transmit the bacterium to humans in regions where it is endemic (Eldin et al., 2017). Lice have also been identified as potential vector hosts for C. burnetii, although their role in transmission is not well understood (Eldin et al., 2017).
One of the key virulence factors of C. burnetii is the T4SS. This complex apparatus is used by the bacterium to deliver effector proteins directly into the host cell cytoplasm. The T4SS of C. burnetii plays a crucial role in host-pathogen interactions and is a major determinant of the bacterium’s ability to cause disease (Cordsmeier et al., 2019; Thomas et al., 2020). The T4SS of C. burnetii is composed of multiple components, including the T4SS core complex, the coupling proteins, and the effector proteins. The T4SS core complex is the scaffold that supports the entire system, while the coupling proteins provide a bridge between the core complex and the effector proteins (Cordsmeier et al., 2019). The effector proteins of C. burnetii, similar to those of Legionella species (Song et al., 2021, 2022; Fu J. et al., 2022), play a crucial role as key virulence factors by being transported into the host cell cytoplasm. Once inside, they strategically manipulate host cell functions to promote bacterial survival and replication (Huang et al., 2022; Zhang et al., 2022).
Autophagy is a highly regulated process that involves the formation of double-membraned vesicles called autophagosomes, which sequester intracellular components and deliver them to the lysosomes for degradation (Aman et al., 2021). This process is crucial for maintaining cellular homeostasis and plays a role in various physiological and pathological conditions, including bacterial infections (Yuk et al., 2012; Aman et al., 2021). Several mechanisms by which autophagy influences bacterial infections have been identified. One important aspect is the selective targeting of bacteria by autophagy. Upon infection, autophagy can recognize and engulf intracellular bacteria within autophagosomes, leading to their delivery to lysosomes for degradation (Sharma et al., 2018). This process restricts bacterial replication and spread, thereby limiting the severity of the infection. Examples of bacterial pathogens targeted by autophagy include Salmonella, Legionella, Mycobacterium tuberculosis, and Group A Streptococcus (Sharma et al., 2018; Thomas et al., 2020).
It is interesting to note that the relationship between host autophagy and C. burnetii infection is quite distinct from that of other pathogens mentioned above. The Coxiella-containing vacuole (CCV) formed during C. burnetii infection accumulates markers commonly found in autophagosomes and lysosomes such as Rab7 (Beron et al., 2002), LC3 (Gutierrez et al., 2005), Beclin 1 (Vazquez and Colombo, 2010) and LAMP-1/2 (Schulze-Luehrmann et al., 2016). Research has indicated that the stimulation of host autophagy, whether through nutrients deprivation or the administration of rapamycin, results in a rise in C. burnetii replication, viability, and CCV size (Gutierrez et al., 2005), whereas inhibiting autophagy or preventing vacuole acidification impairs CCV development (Beron et al., 2002; Latomanski and Newton, 2018). Essential autophagy genes, such as TFEB/TFE3, autophagy-related (ATG) proteins, and STX17, are required for the homotypic fusion process, resulting in the formation of large CCVs (Mcdonough et al., 2013; Newton et al., 2020; Padmanabhan et al., 2020; Lau et al., 2022). Therefore, C. burnetii benefits from the autophagy process and facilitates autophagosome-lysosome fusion to establish its distinct parasitophorous niche for replication.
Given the critical role of autophagy in facilitating infection, it is unsurprising that C. burnetii has developed intricate mechanisms to exploit and manipulate the host’s autophagic machinery for its own benefit. Romano et al. (2007) reported that C. burnetii infection resulted in a postponement of the usual arrival of cathepsin D, a lysosomal protease, to the CCV, which was further extended by autophagy induction by starvation. Under normal circumstances, the cargo receptor p62/sequestosome 1 (SQSTM-1) and LC3 interact to facilitate the selection of cargo for degradation through autophagy, leading to the degradation of p62 and recycling of LC3 (Zhou et al., 2023). The levels of p62 and LC3 were increased in macrophages in response to C. burnetii infection, implying that the pathogen plays a role in stabilizing the proteins (Winchell et al., 2014, 2018; Larson et al., 2019). Furthermore, several genes involved in autophagy-related pathways, specifically membrane trafficking and lipid metabolism, were upregulated in C. burnetii Nine Mile phase II (NMII) infected cells, while they were downregulated in NMI-infected cells (Kumaresan et al., 2022).
The mounting evidence suggests that the functional type IV secretion system (T4SS) of C. burnetii is essential for manipulating host autophagy. LC3 and p62 were present in the wild-type CCVs but not in CCVs containing T4SS mutants of C. burnetii (Winchell et al., 2014). In addition, upon activation of the T4SS 24 h post-infection, there was a subsequent recruitment of autophagosomes (Winchell et al., 2014). TFEB and TFE3 were both activated upon C. burnetii infection, as indicated by their movement from the cytoplasm to the nucleus (Padmanabhan et al., 2020). The movement of the transcription factors was discovered to depend on the T4SS, as the nuclear movement of TFEB and TFE3 was reduced in a Dot/Icm mutant (Padmanabhan et al., 2020).
CvpB, also known as Cig2, is one of the key effectors delivered by the T4SS of C. burnetii. CpvB plays a crucial role in the modulation of host immune responses and the establishment of a favorable intracellular niche for C. burnetii replication. Studies have shown that CpvB is involved in multiple aspects of C. burnetii infection. One of its important functions is interfering with host autophagy. Newton, et al. identified mutants displaying a multi-vacuolar phenotype, all of which had a transposon insertion in the gene encoding the effector protein CvpB (Newton et al., 2014). In comparison to vacuoles containing wild-type C. burnetii, the vacuoles formed by the cvpB mutant lacked detectable amounts of the autophagosome protein LC3 (Newton et al., 2014). Interestingly, when host autophagy was disrupted during infection by wild-type C. burnetii, a similar multi-vacuolar phenotype was observed, resembling that of the cvpB mutant (Newton et al., 2014). CvpB, as well as CvpC, CvpD, and CvpE, label the CCV membrane and LAMP1-positive vesicles and deletion mutants for any of these substrates show impaired intracellular replication and CCV formation (Larson et al., 2015). Furthermore, CvpB is crucial for facilitating continuous fusion between the CCV and autophagosomes produced through selective autophagy (Kohler et al., 2016). This interaction leads to the formation of an autolysosomal vacuole (Kohler et al., 2016). These findings strongly suggest that the functional CvpB protein plays a vital role in the interaction between the CCV and host autophagosomes.
Another study has reported that CpvB interacts with PI(3)P and phosphatidylserine (PS) on CCVs and early endosomes (Martinez et al., 2016). CvpB disrupts the activity of a phosphatidylinositol 5-kinase called PIKfyve, which plays a crucial role in regulating PI(3)P metabolism (Martinez et al., 2016). Within CCVs, CvpB’s association with PI(3)P derived from early endosomes and autophagy pathways, along with the inhibition of PIKfyve, enhances the association of the autophagosomal machinery with CCVs (Martinez et al., 2016). However, the molecular mechanisms by which CvpB prevents PIKfyve recruitment to PI(3)P-positive membranes remain unknown. Further research is required to elucidate the exact mechanisms underlying these observations and to fully understand the role of CvpB in manipulating PI(3)P metabolism, autophagy and CCV biogenesis.
CvpF belongs to the Cvp family of effectors, which are secreted by the Dot/Icm secretion system of C. burnetii. Upon screening a C. burnetii transposon mutant library, researchers found that mutations in the cvpF gene resulted in defects in intracellular replication and vacuole formation (Siadous et al., 2021). CvpF localizes to vesicles with autolysosomal characteristics, as well as CCVs. CvpF was found to interact specifically with the host protein RAB26, a small GTPase involved in regulating endosomal trafficking (Siadous et al., 2021). This interaction leads to the recruitment of MAP1LC3B/LC3B, a marker protein for autophagosomes, to the CCVs (Siadous et al., 2021). Importantly, studies using cvpF::Tn mutants showed that these mutants were highly attenuated and less virulent compared to wild-type C. burnetii in a mouse model of infection (Siadous et al., 2021). This highlights the significance of CvpF in C. burnetii’s ability to cause disease and establish infection within the host.
Another C. burnetii effector protein CpeB, which is encoded by the QpH1 plasmid, was found to localize on the membrane of the CCVs and interact with LC3 and LAMP1, respectively (Voth et al., 2011). This interaction promotes the accumulation of LC3-II, a lipidated form of LC3 that is associated with autophagosomal membranes (Fu M. et al., 2022). When the QpH1 plasmid was absent in C. burnetii strains, there was reduced LC3-II accumulation, smaller CCVs, and lower bacterial loads in host cells (Fu M. et al., 2022). Furthermore, expression of CpeB in the QpH1-deficient strain restored LC3-II accumulation, indicating that CpeB plays a significant role in promoting LC3-II accumulation (Fu M. et al., 2022). The study also found that CpeB targets a host protein called Rab11a to promote LC3-II accumulation (Fu M. et al., 2022). Rab11a is involved in regulating membrane trafficking pathways, including those associated with autophagy. When Rab11a was conditionally knocked out in mice and subsequently infected with C. burnetii, notable reductions in bacterial burdens and the development of milder lung lesions were observed (Fu M. et al., 2022), suggesting that Rab11a plays a role in C. burnetii infection and virulence.
Apart from the aforementioned proteins, such as CpeB, additional C. burnetii effector proteins like Cig57, CvpA, CvpC, and others are believed to contribute to the intricate interplay between C. burnetii infection and autophagy (Thomas et al., 2020).
In conclusion, C. burnetii has a sophisticated set of tactics to subvert the host autophagic pathways, allowing it to survive and thrive within host cells. The bacterium’s ability to manipulate this innate cellular process and evade immune detection contributes to its pathogenesis in humans and animals. Looking forward, it is important to note that there are still gaps in our understanding of C. burnetii’s subversion of autophagy, particularly in identifying and characterizing autophagy-related effectors with explored biochemical activities. Future research should aim to fill these knowledge gaps by further investigating the specific virulence factors involved in C. burnetii’s manipulation of autophagy. This research may provide valuable insights into potential targets for antimicrobial interventions. Additionally, exploring strategies to modulate the host immune response to C. burnetii infections could enhance immune clearance of the bacterium. Ultimately, a comprehensive understanding of the host-pathogen interactions and the biochemical activities of autophagy-related effectors will be crucial for developing effective treatments and control measures to combat C. burnetii infections.
TW: Conceptualization, Writing – original draft, Writing – review & editing. CW: Conceptualization, Writing – original draft. CL: Conceptualization, Writing – review & editing. LS: Conceptualization, Funding acquisition, Writing – original draft, Writing – review & editing.
The author(s) declare financial support was received for the research, authorship, and/or publication of this article. This work was supported by National Natural Science Foundation of China (No. 32270185).
The authors declare that the research was conducted in the absence of any commercial or financial relationships that could be construed as a potential conflict of interest.
All claims expressed in this article are solely those of the authors and do not necessarily represent those of their affiliated organizations, or those of the publisher, the editors and the reviewers. Any product that may be evaluated in this article, or claim that may be made by its manufacturer, is not guaranteed or endorsed by the publisher.
The Supplementary material for this article can be found online at: https://www.frontiersin.org/articles/10.3389/fmicb.2023.1281303/full#supplementary-material
Abou Abdallah, R., Million, M., Delerce, J., Anani, H., Diop, A., Caputo, A., et al. (2022). Pangenomic analysis of Coxiella burnetii unveils new traits in genome architecture. Front. Microbiol. 13:1022356. doi: 10.3389/fmicb.2022.1022356
Aman, Y., Schmauck-Medina, T., Hansen, M., Morimoto, R. I., Simon, A. K., Bjedov, I., et al. (2021). Autophagy in healthy aging and disease. Nat Aging 1, 634–650. doi: 10.1038/s43587-021-00098-4
Beare, P. A., Samuel, J. E., Howe, D., Virtaneva, K., Porcella, S. F., and Heinzen, R. A. (2006). Genetic diversity of the Q fever agent, Coxiella burnetii, assessed by microarray-based whole-genome comparisons. J. Bacteriol. 188, 2309–2324. doi: 10.1128/JB.188.7.2309-2324.2006
Beron, W., Gutierrez, M. G., Rabinovitch, M., and Colombo, M. I. (2002). Coxiella burnetii localizes in a Rab7-labeled compartment with autophagic characteristics. Infect. Immun. 70, 5816–5821. doi: 10.1128/IAI.70.10.5816-5821.2002
Cabrera, R., Mendoza, W., Lopez-Mosquera, L., Cano, M. A., Ortiz, N., Campo, V., et al. (2022). Tick-borne-agents detection in patients with acute febrile syndrome and ticks from Magdalena Medio, Colombia. Pathogens 11:11. doi: 10.3390/pathogens11101090
Celina, S. S., and Cerny, J. (2022). Coxiella burnetii in ticks, livestock, pets and wildlife: a mini-review. Front Vet Sci 9:1068129. doi: 10.3389/fvets.2022.1068129
Cordsmeier, A., Wagner, N., Luhrmann, A., and Berens, C. (2019). Defying death - how Coxiella burnetii copes with intentional host cell suicide. Yale J. Biol. Med. 92, 619–628.
Eldin, C., Melenotte, C., Mediannikov, O., Ghigo, E., Million, M., Edouard, S., et al. (2017). From Q fever to Coxiella burnetii infection: a paradigm change. Clin. Microbiol. Rev. 30, 115–190. doi: 10.1128/CMR.00045-16
Espana, P. P., Uranga, A., Cilloniz, C., and Torres, A. (2020). Q Fever (Coxiella Burnetii). Semin. Respir. Crit. Care Med. 41, 509–521. doi: 10.1055/s-0040-1710594
Fu, M., Zhang, J., Zhao, M., Zhang, S., Dai, L., Ouyang, X., et al. (2022). Coxiella burnetii plasmid effector B promotes LC3-II accumulation and contributes to bacterial virulence in a SCID mouse model. Infect. Immun. 90:e0001622. doi: 10.1128/iai.00016-22
Fu, J., Zhou, M., Gritsenko, M. A., Nakayasu, E. S., Song, L., and Luo, Z. Q. (2022). Legionella pneumophila modulates host energy metabolism by ADP-ribosylation of ADP/ATP translocases. elife 11:e73611. doi: 10.7554/eLife.73611
Gutierrez, M. G., Vazquez, C. L., Munafo, D. B., Zoppino, F. C., Beron, W., Rabinovitch, M., et al. (2005). Autophagy induction favours the generation and maturation of the Coxiella-replicative vacuoles. Cell. Microbiol. 7, 981–993. doi: 10.1111/j.1462-5822.2005.00527.x
Huang, D., Luo, J., Ouyang, X., and Song, L. (2022). Subversion of host cell signaling: the arsenal of Rickettsial species. Front. Cell. Infect. Microbiol. 12:995933. doi: 10.3389/fcimb.2022.995933
Kohler, L. J., Reed Sh, C., Sarraf, S. A., Arteaga, D. D., Newton, H. J., and Roy, C. R. (2016). Effector protein Cig2 decreases host tolerance of infection by directing constitutive fusion of autophagosomes with the Coxiella-containing vacuole. MBio 7:e01127-16. doi: 10.1128/mBio.01127-16
Kumaresan, V., Wang, J., Zhang, W., Zhang, Y., Xu, D., and Zhang, G. (2022). Coxiella burnetii virulent phase I and Avirulent phase II variants differentially manipulate autophagy pathway in neutrophils. Infect. Immun. 90:e0053421. doi: 10.1128/iai.00534-21
Larson, C. L., Beare, P. A., Voth, D. E., Howe, D., Cockrell, D. C., Bastidas, R. J., et al. (2015). Coxiella burnetii effector proteins that localize to the parasitophorous vacuole membrane promote intracellular replication. Infect. Immun. 83, 661–670. doi: 10.1128/IAI.02763-14
Larson, C. L., Sandoz, K. M., Cockrell, D. C., and Heinzen, R. A. (2019). Noncanonical inhibition of mTORC1 by Coxiella burnetii promotes replication within a phagolysosome-like vacuole. MBio 10:e02816-18. doi: 10.1128/mBio.02816-18
Latomanski, E. A., and Newton, H. J. (2018). Interaction between autophagic vesicles and the Coxiella-containing vacuole requires CLTC (clathrin heavy chain). Autophagy 14, 1710–1725. doi: 10.1080/15548627.2018.1483806
Lau, N., Thomas, D. R., Lee, Y. W., Knodler, L. A., and Newton, H. J. (2022). Perturbation of ATG16L1 function impairs the biogenesis of Salmonella and Coxiella replication vacuoles. Mol. Microbiol. 117, 235–251. doi: 10.1111/mmi.14858
Martinez, E., Allombert, J., Cantet, F., Lakhani, A., Yandrapalli, N., Neyret, A., et al. (2016). Coxiella burnetii effector CvpB modulates phosphoinositide metabolism for optimal vacuole development. Proc. Natl. Acad. Sci. U. S. A. 113, E3260–E3269. doi: 10.1073/pnas.1522811113
Mcdonough, J. A., Newton, H. J., Klum, S., Swiss, R., Agaisse, H., and Roy, C. R. (2013). Host pathways important for Coxiella burnetii infection revealed by genome-wide RNA interference screening. MBio 4, e00606–e00612. doi: 10.1128/mBio.00606-12
Minnick, M. F., and Raghavan, R. (2011). Genetics of Coxiella burnetii: on the path of specialization. Future Microbiol. 6, 1297–1314. doi: 10.2217/fmb.11.116
Newton, H. J., Kohler, L. J., Mcdonough, J. A., Temoche-Diaz, M., Crabill, E., Hartland, E. L., et al. (2014). A screen of Coxiella burnetii mutants reveals important roles for dot/Icm effectors and host autophagy in vacuole biogenesis. PLoS Pathog. 10:e1004286. doi: 10.1371/journal.ppat.1004286
Newton, P., Thomas, D. R., Reed, S. C. O., Lau, N., Xu, B., Ong, S. Y., et al. (2020). Lysosomal degradation products induce Coxiella burnetii virulence. Proc. Natl. Acad. Sci. U. S. A. 117, 6801–6810. doi: 10.1073/pnas.1921344117
Padmanabhan, B., Fielden, L. F., Hachani, A., Newton, P., Thomas, D. R., Cho, H. J., et al. (2020). Biogenesis of the spacious Coxiella-containing vacuole depends on host transcription factors TFEB and TFE3. Infect. Immun. 88:e00534-19. doi: 10.1128/IAI.00534-19
Romano, P. S., Gutierrez, M. G., Beron, W., Rabinovitch, M., and Colombo, M. I. (2007). The autophagic pathway is actively modulated by phase II Coxiella burnetii to efficiently replicate in the host cell. Cell. Microbiol. 9, 891–909. doi: 10.1111/j.1462-5822.2006.00838.x
Sandoz, K. M., Sturdevant, D. E., Hansen, B., and Heinzen, R. A. (2014). Developmental transitions of Coxiella burnetii grown in axenic media. J. Microbiol. Methods 96, 104–110. doi: 10.1016/j.mimet.2013.11.010
Schulze-Luehrmann, J., Eckart, R. A., Olke, M., Saftig, P., Liebler-Tenorio, E., and Luhrmann, A. (2016). LAMP proteins account for the maturation delay during the establishment of the Coxiella burnetii-containing vacuole. Cell. Microbiol. 18, 181–194. doi: 10.1111/cmi.12494
Sharma, V., Verma, S., Seranova, E., Sarkar, S., and Kumar, D. (2018). Selective autophagy and Xenophagy in infection and disease. Front. Cell Dev. Biol. 6:147. doi: 10.3389/fcell.2018.00147
Shaw, E. I., and Voth, D. E. (2019). Coxiella burnetii: a pathogenic intracellular Acidophile. Microbiology (Reading) 165, 1–3. doi: 10.1099/mic.0.000707
Siadous, F. A., Cantet, F., Van Schaik, E., Burette, M., Allombert, J., Lakhani, A., et al. (2021). Coxiella effector protein CvpF subverts RAB26-dependent autophagy to promote vacuole biogenesis and virulence. Autophagy 17, 706–722. doi: 10.1080/15548627.2020.1728098
Song, L., Luo, J., Wang, H., Huang, D., Tan, Y., Liu, Y., et al. (2022). Legionella pneumophila regulates host cell motility by targeting Phldb2 with a 14-3-3zeta-dependent protease effector. elife 11:e73220. doi: 10.7554/eLife.73220
Song, L., Xie, Y., Li, C., Wang, L., He, C., Zhang, Y., et al. (2021). TheLegionellaEffector SdjA is a bifunctional enzyme that distinctly regulates phosphoribosyl ubiquitination. M bio 12:e0231621. doi: 10.1128/mBio.02316-21
Thomas, D. R., Newton, P., Lau, N., and Newton, H. J. (2020). Interfering with autophagy: the opposing strategies deployed by Legionella pneumophila and Coxiella burnetii effector proteins. Front. Cell. Infect. Microbiol. 10:599762. doi: 10.3389/fcimb.2020.599762
Ullah, Q., Jamil, T., Saqib, M., Iqbal, M., and Neubauer, H. (2022). Q fever-a neglected zoonosis. Microorganisms 10:1530. doi: 10.3390/microorganisms10081530
Vazquez, C. L., and Colombo, M. I. (2010). Coxiella burnetii modulates Beclin 1 and Bcl-2, preventing host cell apoptosis to generate a persistent bacterial infection. Cell Death Differ. 17, 421–438. doi: 10.1038/cdd.2009.129
Voth, D. E., Beare, P. A., Howe, D., Sharma, U. M., Samoilis, G., Cockrell, D. C., et al. (2011). The Coxiella burnetii cryptic plasmid is enriched in genes encoding type IV secretion system substrates. J. Bacteriol. 193, 1493–1503. doi: 10.1128/JB.01359-10
Winchell, C. G., Dragan, A. L., Brann, K. R., Onyilagha, F. I., Kurten, R. C., and Voth, D. E. (2018). Coxiella burnetii subverts p62/Sequestosome 1 and activates Nrf2 signaling in human macrophages. Infect. Immun. 86:e00608-17. doi: 10.1128/IAI.00608-17
Winchell, C. G., Graham, J. G., Kurten, R. C., and Voth, D. E. (2014). Coxiella burnetii type IV secretion-dependent recruitment of macrophage autophagosomes. Infect. Immun. 82, 2229–2238. doi: 10.1128/IAI.01236-13
Yuk, J. M., Yoshimori, T., and Jo, E. K. (2012). Autophagy and bacterial infectious diseases. Exp. Mol. Med. 44, 99–108. doi: 10.3858/emm.2012.44.2.032
Zhang, Y., Fu, J., Liu, S., Wang, L., Qiu, J., Van Schaik, E. J., et al. (2022). Coxiella burnetii inhibits host immunity by a protein phosphatase adapted from glycolysis. Proc. Natl. Acad. Sci. U. S. A. 119:e2110877119. doi: 10.1073/pnas.2110877119
Keywords: Q fever, type IV secretion system, effector, autophagosome, lysosome
Citation: Wang T, Wang C, Li C and Song L (2023) The intricate dance: host autophagy and Coxiella burnetii infection. Front. Microbiol. 14:1281303. doi: 10.3389/fmicb.2023.1281303
Received: 22 August 2023; Accepted: 11 September 2023;
Published: 22 September 2023.
Edited by:
Yong Qi, Huadong Research Institute for Medicine and Biotechniques, ChinaReviewed by:
Jun Jiao, Beijing Institute of Microbiology and Epidemiology, ChinaCopyright © 2023 Wang, Wang, Li and Song. This is an open-access article distributed under the terms of the Creative Commons Attribution License (CC BY). The use, distribution or reproduction in other forums is permitted, provided the original author(s) and the copyright owner(s) are credited and that the original publication in this journal is cited, in accordance with accepted academic practice. No use, distribution or reproduction is permitted which does not comply with these terms.
*Correspondence: Chang Li, Y2hhbmdsaUBqbHUuZWR1LmNu; Lei Song, bHNvbmdAamx1LmVkdS5jbg==
†These authors have contributed equally to this work
Disclaimer: All claims expressed in this article are solely those of the authors and do not necessarily represent those of their affiliated organizations, or those of the publisher, the editors and the reviewers. Any product that may be evaluated in this article or claim that may be made by its manufacturer is not guaranteed or endorsed by the publisher.
Research integrity at Frontiers
Learn more about the work of our research integrity team to safeguard the quality of each article we publish.