- Collaborative Innovation Center of Sustainable Forestry in Southern China of Jiangsu Province, Nanjing Forestry University, Nanjing, China
Soil microorganisms play a crucial role in remediating contaminated soils in modern ecosystems. However, the potential of combining microorganisms with legumes to enhance the remediation of heavy metal-contaminated soils remains unexplored. To investigate this, we isolated and purified a highly efficient cadmium and lead-tolerant strain. Through soil-cultivated pot experiments with two leguminous plants (Robinia pseudoacacia L. and Sophora xanthantha), we studied the effects of applying this microbial agent on plant nutrient uptake of soil nutrients, heavy metal accumulation, and the dynamics of heavy metal content. Additionally, we examined the response characteristics of inter-root microbial and bacterial communities. The results demonstrated that microorganisms screened from heavy metal-contaminated soil environments exhibited strong survival and adaptability in heavy metal solutions. The use of the Serratia marcescens WZ14 strain-phytoremediation significantly increased the soil’s ammonium nitrogen (AN) and organic carbon (OC) contents compared to monoculture. In addition, the lead (Pb) and cadmium (Cd) contents of the soil significantly decreased after combined remediation than those of the soil before potting. However, the remediation effects on Pb- and Cd-contaminated soils differed between the two legumes following the Serratia marcescens WZ14 inoculation. The combined restoration altered the composition of the plant inter-rhizosphere bacterial community, with the increase in the relative abundance of both Proteobacteria and Firmicutes. Overall, the combined remediation using the tolerant strain WZ14 with legumes proved advantageous. It effectively reduced the heavy metal content of the soil, minimized the risk of heavy metal migration, and enhanced heavy metal uptake, accumulation, and translocation in the legumes of S. xanthantha and R. pseudoacacia. Additionally, it improved the adaptability and resistance of both legumes, leading to an overall improvement in the soil’s environmental quality. These studies can offer primary data and technical support for remediating and treating Cd and Pb in soils, as well as rehabilitating mining sites.
1. Introduction
The issue of heavy metal pollution in soil, driven by unnatural factors from rapid industrialization, is increasingly severe and requires urgent solutions. Soil, as the most abundant and diverse ecosystem on Earth, plays a crucial role in reflecting soil health and function through the dynamics of soil quality, surface vegetation, and microbial communities in complex environments (Jiang et al., 2016). Mine remediation using microorganisms has been vastly studied over recent decade for remediation and ecological systems restoration at various mine sites (Xiao et al., 2023).
The unnatural uptake of heavy metals during mining is the primary cause of soil heavy metal contamination (Rachelle et al., 2018; Wang et al., 2022). Heavy metals (HMs) contamination has led to severe environmental issues, including soil nutrient losses, sharp reductions in soil microbial diversity, and hindered plant growths (Liu et al., 2019; Gonalves et al., 2020; Shuaib et al., 2021). Unlike organic pollutants, heavy metal pollution is characterized by difficult degradation, hidden, long-term, and high toxicity, and it is difficult to achieve the intended effect of complete removal in the short term in the remediation of soil heavy metal pollution (Luo et al., 2019). As traditional remediation techniques like physical and chemical methods are increasingly limited in addressing soil heavy metal pollution, phytoremediation has gained significant attention as an alternative due to its ecological, economic, and sustainable advantages (Fatima et al., 2016; Saxena et al., 2019; Xiao L. et al., 2021), emerging as one of the most promising remediation approaches. In addition, plants play a vital role in improving soil quality and optimizing the soil microbial community (Schloter et al., 2018; Beiyuan et al., 2021). Various microorganisms living in the rhizosphere, can have beneficial effects on plant growth, and health and increase plant biomass production (Evlat et al., 2023). Therefore, investigating changes in soil nutrients and microbial community structure during phytoremediation is crucial for successful ecological restoration.
Most studies on phytoremediation for soil heavy metal remediation, especially on phytoextraction, have primarily focused on utilizing super-enriched plants (HMH) to extract soil heavy metals (Duan et al., 2020; Atikur-Rahman et al., 2022). Although HMH has demonstrated favorable remediation results, certain studies have presented its limitations, such as slow growth and shallow root systems, which hinder its ability to reach deeper soil layers and extract heavy metals to a treatable level (Słomka et al., 2012). In addition, Wood et al. (2016) observed that non-HMH species often extracted more heavy metals than HMH when measuring the net number of metals extracted per plant, with the number of extracted heavy metals closely correlated to plant biomass. Although HMH remains valuable in practical phytoremediation, non-HMH species with high biomass may represent a more suitable option for developing efficient phytoextractors in the future.
Leguminosae, with 172 genera, 1,485 species, and 153 varieties in China (Hei et al., 2019), are widely distributed throughout the country and hold significance in soil improvement and ecological restoration (Dary et al., 2010; Cai et al., 2015). Legumes have exhibited excellent tolerance and effectiveness in heavy metal remediation, with some species exhibiting remediation capabilities comparable to HMH due to their robust root biomass (Shi et al., 2012; Guo and Chi, 2017; Zeng, 2017). The advantages of legumes in this regard include: (i) their strong root biomass can produce abundant secretions that confer resistance to heavy metal pollution stress (Pereira et al., 2006), with the dissolution of insoluble heavy metals in the soil by the organic acids released from the roots, which can enhance plant uptake of soil heavy metals. (ii) Legume roots contain abundant rhizobia, effectively improving soil quality (Maynaud et al., 2013). These traits enable legumes to enhance, maintain, and develop stable soil systems. Therefore, employing legumes in phytoremediation holds significant potential for soil heavy metal remediation, contributing to the future refinement of plant species screening and phytoextraction in ecological restoration.
In order to improve phytoextraction efficiency, the inoculation of characteristic microorganisms into plant roots is a common strategy (Abhilash et al., 2012; Sessitsch et al., 2013). The identification and development of new, effective PGPR strains would be very efficient, providing several beneficial activities such as improved nutrient uptake, improved stress tolerance, enhanced plant growth, and resistance to fungal or bacterial pathogens (Xiao C. Q. et al., 2021). The phytoremediation coupled with Pb-resistant phosphate-solubilizing bacteria effectively improved the efficiency of Pb bioremediation. For example, The inoculation of soil with strain LA greatly promoted the growth of ryegrass and sonchus, increased the concentration of bioavailable P and Pb in plants, and decreased the bioavailability of Pb in the soil (Guo et al., 2021). In the “biotrophic bacteria-plant” mechanism, on the one hand, “bacteria” generally refers to inter-root biotrophic bacteria that can promote plant growth or improve soil quality, and the microorganisms promote plant growth through the production of iron carriers, phytohormones, organic acids and functional enzymes to enhance the remediation of heavy metals in the soil; On the other hand, some microorganisms themselves have the ability to dissolve and activate heavy metals, which reduces the content of heavy metals in the soil, increases the base of heavy metals that can be absorbed by the soil, and improves the possibility of plant uptake of heavy metals in the soil, so that the total amount of heavy metals in the soil is reduced to a harmless level (Ni et al., 2019). Several studies have demonstrated the microorganisms can convert Cr (VI) in soil into Cr (III) or directly adsorb it in their bodies by means of bioreduction and biosorption, while plants uptake and accumulate Cr in tissues, thus reducing the total Cr content in the soil (Wu et al., 2014). Li (2021) inoculated Variovorax paradoxus DE5 into Celosia argentea plants and found that DE5 was able to significantly promote the growth of Celosia argentea plants and enhance the uptake of soil cadmium by Celosia argentea. The results of the pot test showed that the application of Lactobacillus casei at 105 cfu·mL−1 reduced the pH of the soil, increased the soil enzyme activity, promoted the growth and development of cabbage mustard, and facilitated the remediation efficiency of cabbage mustard on Cd and Zn composite contaminated soil (Liu, 2022). It has been shown that the application of organic acid-secreting endophytic bacteria effectively increased the conversion of insoluble Pb into the effective state of Pb, increased the content of soil heavy metal Pb in the effective state, and significantly improved the enrichment efficiency of oilseed rape for soil heavy metal Pb (Ma Y. et al., 2022). Typically, the candidate microorganisms inoculated into plants originate from internal plant tissues or root secretions and function as “probiotics,” directly or indirectly influencing plant growth (Li et al., 2017). However, such microorganisms often exhibit weak resistance against external pollution stress (Zhang et al., 2011). Conversely, microorganisms exposed to and surviving in heavy metal-contaminated soil over extended periods may possess higher tolerance and resistance (Ibiang et al., 2020). Therefore, understanding the source of microorganisms, their impact on heavy metal morphology, and their probiotic effects on plants prove beneficial for soil heavy metal remediation efforts.
Our study addressed these limitations through a comprehensive approach. We first screened lead (Pb)- and cadmium (Cd)-tolerant microorganisms (resistant bacteria) for inoculum in experiments, using long-term heavy metal-contaminated soil as substrate. In addition, the heavy metal environment was simulated through indoor resistance growth experiments. Concurrently, outdoor pot experiments were conducted to investigate the impact of legumes (Sophora xanthantha and Robinia pseudoacacia L.) and resistant bacteria-legume combinations on the remediation of heavy metal-contaminated soil. The objective of this study was (a) to determine whether tolerant bacterial inoculum would enhance the effectiveness of legume-based remediation, (b) to examine whether tolerant bacterial inoculum would lead to plant improvement in soil physicochemical properties and reduction in heavy metal content, and (c) to investigate key species of contaminated soil bacterial communities using high-throughput sequencing techniques. These studies have provided essential data and technical support for the utilization of legumes in the remediation of Cd and Pb in soils and the rehabilitation of mining sites.
2. Materials and methods
2.1. Study area overview and experimental design
The lead-zinc-silver mine is situated in Zhijiadi, Gaojiazhuang Township, south of Lingqiu County, Shanxi Province (39.21°N, 114.12°E). The region experiences a temperate semi-arid continental climate, with an average annual temperature of 7°C and an average annual rainfall of 421 mm. The mine, commissioned in 2003, is expected to have a lifespan of 20 years or more. Long-term mining activities have led to long-term heavy metal pollution in the soil. In order to address this issue, soil samples were collected from three different functional types: mine center, mine wasteland, and tailings. These soils were used for screening heavy metal Pb and Cd tolerant microorganisms (Figure 1). The experiment involved soils contaminated with two heavy metal complexes, Pb and Cd. Sterilized soil was uniformly sprayed with a solution of 400 mg/L Pb2+ (Pb (NO3)2) and 60 mg/L Cd2+ (CdCl2). After thorough mixing, the soil was left for 15 days for potting experiments.
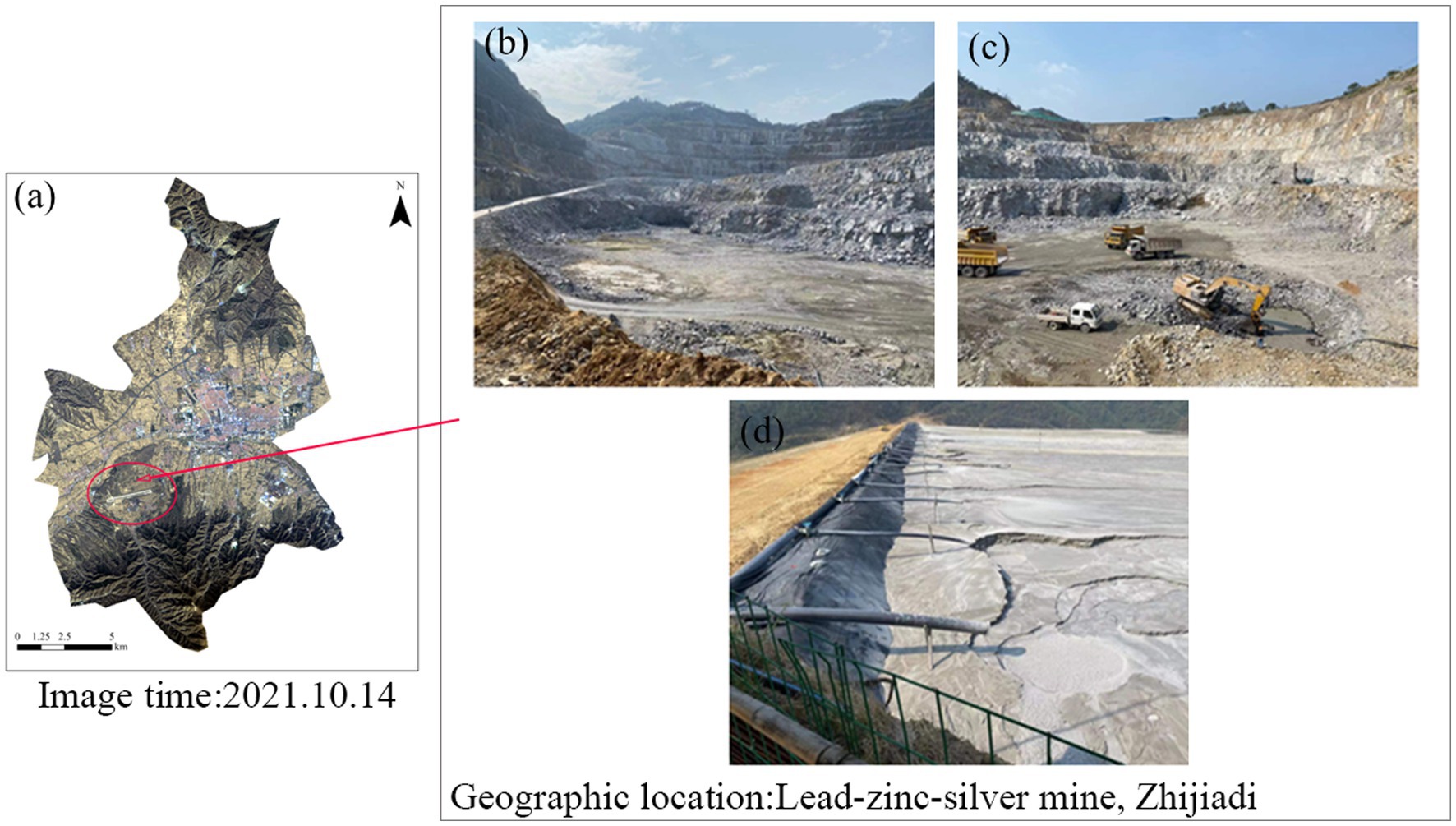
Figure 1. Collection of three different functional types of soils (a), including mine center soils (b), mine wasteland soils (c), and tailings soils (d).
2.1.1. Resilient growth experiment
Bacteria and fungi were isolated from soil samples using 10-fold serial dilutions plated onto heavy metal-free nutrient agar and potato dextrose agar solid media, respectively. The plates were then incubated for 3 days at 28°C. Once colonies covered two-thirds of the plates, colonies indicating different morphological colors were selected and purified. Subsequently, solid medium plates containing a Pb2+ concentration of 100 mg/L and a Cd2+ concentration of 40 mg/L were prepared. The purified strains were plated on these plates using the “trilinear” method. The screening of strains that grew well on plates containing heavy metals for resistance growth experiments was conducted to identify strains capable of thriving in environments contaminated with lead and cadmium while reducing unnecessary testing efforts.
The viability of the screened strains was quantitatively assessed through resistance growth experiments. Luria broth (LB) cultures with varying concentrations of Pb and Cd (suitable for bacterial and fungal colonization) were prepared. The Pb concentrations in the LB cultures were set at 400, 600, 800, 1,000, 1,500, and 2,000 mg/L, achieved by replacing pure water with different concentrations of Pb (NO3)2 solution. Similarly, the Cd2+ concentrations in LB cultures were maintained at 50, 100, 150, 200, 250, and 300 mg/L. Well-grown colonies (WZ13, WZ14, WG20, and S22) with a diameter of 1 mm were added to 500 mL conical flasks containing 400 mL LB culture medium with varying Pb and Cd concentrations. Control flasks without colonies were also included. The flasks were then incubated on a shaker (speed: 180 rpm, temperature: 25°C ± 0.3) for 2 days. During this period, absorbance values were measured at 12, 24, 36, and 48 h for each treatment, providing insights into the strain’s ability to survive in an environment contaminated with lead and cadmium (Fang et al., 2012).
2.1.2. Pot experiment
Based on the results of resistance growth and soil cultivation experiments, a promising resistant strain was selected for investigating the remediation effects of soil Pb and Cd through potting experiments. The seeds of the test plants were provided by the State-owned Qiaotou Forest Farm, Wengniute Banner, Chifeng City, Inner Mongolia. Seeds were soaked in pure water for 12 h (Zheng et al., 2021). After filtering the water and allowing the seeds to dry on the surface, the obtained strain was disinfected by immersing them in a 5% sodium hypochlorite solution for 10 min (Zhuang et al., 2021). After washing the seeds with pure water until they were odorless, 5 to 7 seeds were placed in seedling cups for 1 week. Seedlings of similar height and growth were carefully selected for transplantation into plastic pots (15 cm in diameter, 20 cm in height, containing 2.0 kg of soil mixture; Bao, 2000). Once the seedling roots stabilized, the prepared WZ14 inoculum was inoculated into the plant roots, creating a combination of tolerant bacteria-plant treatment. Seedlings inoculated with sterile culture served as the control treatment. Each treatment was replicated three times and consistently watered and randomized to maintain a standardized regimen. After 3 months, plant and soil samples were collected for analysis.
2.2. Sample collection and analysis
After a 3-month growth period, all plants and soil were harvested. Plant samples were collected by separating roots, stems, and leaves, which were then washed, dried, and crushed. Soil samples were obtained by shaking the inter-root soil attached to plant roots. The selected soil samples were divided into two parts and stored. One portion of the soil was stored in a 4°C ice box and brought back to the laboratory for further analysis. The second portion of the soil samples was air-dried to determine the physical and chemical properties of the soil.
The pH of the soil solution and the soil’s physicochemical properties were analyzed following Bao (2000) and Lou et al. (2019) standard method. Potentiometric measurements determined the pH, volumetric potassium dichromate analysis determined soil organic matter, and the elemental analyzer determined total soil nitrogen. The molybdenum-antimony anti-colorimetric method was employed for the determination of total soil phosphorus, while total soil potassium was measured using the NaOH alkali fusion-flame photometric method. Soil alkaline nitrogen was determined via the alkaline diffusion method, and effective phosphorus was analyzed using the molybdenum-antimony anti-colorimetric UV spectrophotometric method. Fast-acting potassium was determined by ammonium acetate extraction followed by flame analysis. The effective phosphorus content was quantified using molybdenum antimony anti-colorimetric UV spectrophotometry. The total amount of heavy metal elements in soil samples was analyzed through soil digestion using the electric hot plate digestion method. Additionally, the effective state content of soil heavy metals was extracted using the hydrochloric acid leaching method (HJ804-2016). Both analyses were conducted using an Optima 5300 DV inductively coupled plasma emission spectrometer test (ICP-AES, PerkinElmer, United States).
1. Measurement of total heavy metals in soil: Soil samples were air-dried and sifted through a 100-mesh sieve. Then, 0.1 g of the soil sample was put in a 25 mL PTFE beaker, lightly moistened with Milli-Qultrapure water and heated at a low temperature of 180°C with 5 mL of concentrated hydrochloric acid for 20 min (evaporated to about 3 mL) to eliminate sulfur compounds present in the sample. Subsequently, 3 mL of concentrated nitric acid, 3 mL of hydrofluoric acid, and 1 mL of perchloric acid were added. The beaker was then heated on a heating plate with a lid to a temperature of 280°C for approximately 1.5 h until a clear solid was produced. Afterward, 19 mL of ultrapure water and 1 mL each of concentrated hydrochloric acid and concentrated nitric acid (concentrated hydrochloric acid: concentrated nitric acid = 3:1) were added to the beaker. Then, the mixture was transferred to a 25 mL volumetric flask, and the volume was determined. Ultimate, taking 5 mL of the solution that has passed through a 0.45 μm aqueous filter membrane and place it in a 10 mL centrifuge tube. For testing.
2. Determination of the effective state content of soil heavy metals: The soil samples were air-dried and passed through a 100-mesh sieve, and 5 g of soil samples were placed in a 15 mL centrifuge tube. Then 0.1 mol-L−1 of dilute hydrochloric acid was added to the centrifuge tube, the lid was tightly closed, and the tube was placed on a shaker (speed: 200 rpm, temperature: 20°C ± 2°C) for 4 h to allow the reaction to complete, and the tube was removed from the shaker. Ultimate, after being left standing for 24 h, take 5 mL of the solution that has passed through a 0.45 μm aqueous filter membrane and transfer it to a 10 mL centrifuge tube for testing.
The method for determining heavy metals in different parts of the plant was identical to the one described above for total soil heavy metals.
2.3. Soil DNA extraction, PCR amplification, and high-throughput gene sequencing
The PowerSoil DNA Isolation Kit (MO BIO, CA, United States) was utilized to extract DNA from all soil samples. In order to minimize the impact of soil heterogeneity on test results and avoid biases from single DNA extraction or low DNA content in samples, each soil sample underwent multiple DNA extracts for subsequent analysis. The purity and concentration of the DNA were assessed using a NanoDrop-2000 spectrophotometer (Thermo-Scientific, DE, United States) and agarose gel electrophoresis (Li et al., 2020). For soil DNA amplification, specific primers 338F (5′-ACTCCTACGGGAGGCAGCAG-3′) and 806R (5′-GGACTACHVGGGTWTCTAAT-3′) targeting the V3–V4 region of bacterial 16S rRNA were employed (Ma et al., 2020). The PCR amplification steps were as follows: the predenaturation at 95°C for 3 min, followed by 35 cycles at 95°C for 30s, 55°C for 30 s, and 72°C for 45 s, were performed with a final extension at 72°C for 5 min. The total volume of the PCR reaction was 25 μL. After PCR amplification, the constructed libraries were analyzed through Qubit and qPCR before being sequenced on an Illumina Miseq system (Guangzhou Kidio Technology Services). The raw data obtained from sequencing was processed by trimming, filtering, and splicing to obtain valid data for subsequent analysis. The optimized sequences, showing >97% similarity, were clustered into operational taxonomic units (OTUs). The taxonomic identities of the bacteria were determined using RDP software and Silva schemes (Wang et al., 2007; Quast et al., 2012).
For further experiments, the nucleotide sequence of WZ14 and the ITS sequence of the potting soil have been uploaded to the NCBI database under the accession numbers OR492361 and PRJNA1012100, respectively.
2.4. Data analysis
Data were compiled using Microsoft Excel 2019 software, and statistical analyses were conducted using one-way analysis of variance (ANOVA) with SPSS 20.0 (IBM, United States) for total soil heavy metals, effective soil heavy metal status, and soil physicochemical properties. Statistical significance was accepted at p < 0.05. To address soil microbial diversity and richness variations, Simpson and Shannon diversity indices, as well as Chao1 and ACE richness indices, were calculated using Mothur and analyzed for alpha diversity. The data were plotted using the Origin 2019 software, and the significance of differences was determined using the DUNCAN method (α = 0.05). Additionally, heat maps were generated to illustrate the correlation between soil physicochemical factors and soil heavy metals. All bioinformatics analyses were performed using the Omicsmart online analysis platform developed by Guangzhou Kidio Technology Services.
3. Results and analysis
3.1. Resistance growth and soil culture trials based on microbial screening
In this study, we isolated a total of 70 strains from the mine center, mine wasteland, and tailings. A Pb2+ concentration of 100 mg·kg−1 and a Cd2+ concentration of 40 mg·kg−1 were used as screening thresholds for microbial adaptation to heavy metal environments. Among the strains tested, 26 exhibited the ability to tolerate heavy metals. Following a qualitative evaluation, we selected four strains with excellent lead and cadmium tolerance, which were named WZ14, WZ13, S22, and WG20. These selected strains were further subjected to resistance growth experiments.
The four strains exhibited varying growth responses to cultures with different Pb2+ and Cd2+ concentrations (Figures 2, 3). Each strain displayed distinct sensitivity levels to the same heavy metal. Notably, WZ14 exhibited the most favorable adaptation to different Pb2+ concentrations without showing a peak in the growth curve for each concentration. It differed from other strains in cultures with different Pb2+ concentrations. WZ13 and S22 demonstrated weak acclimation in Cd2+ cultures, with both strains exhibiting growth of no more than 0.5 cfu·mL−1 in various Cd2+ concentrations, significantly lower than that of WZ14 and WG20. Comparatively, WZ14 displayed a higher growth curve than WG20 at the same Cd2+ concentration. In addition, its curve gradually shifted downward as Cd2+ concentration increased, suggesting the suitability of WZ14 for survival in a low-concentration Cd-contaminated environment. The results from the resistance growth experiment indicated that WZ14 exhibited robust growth and excellent resistance in both Cd- and Pb-contaminated environments, outperforming other strains.
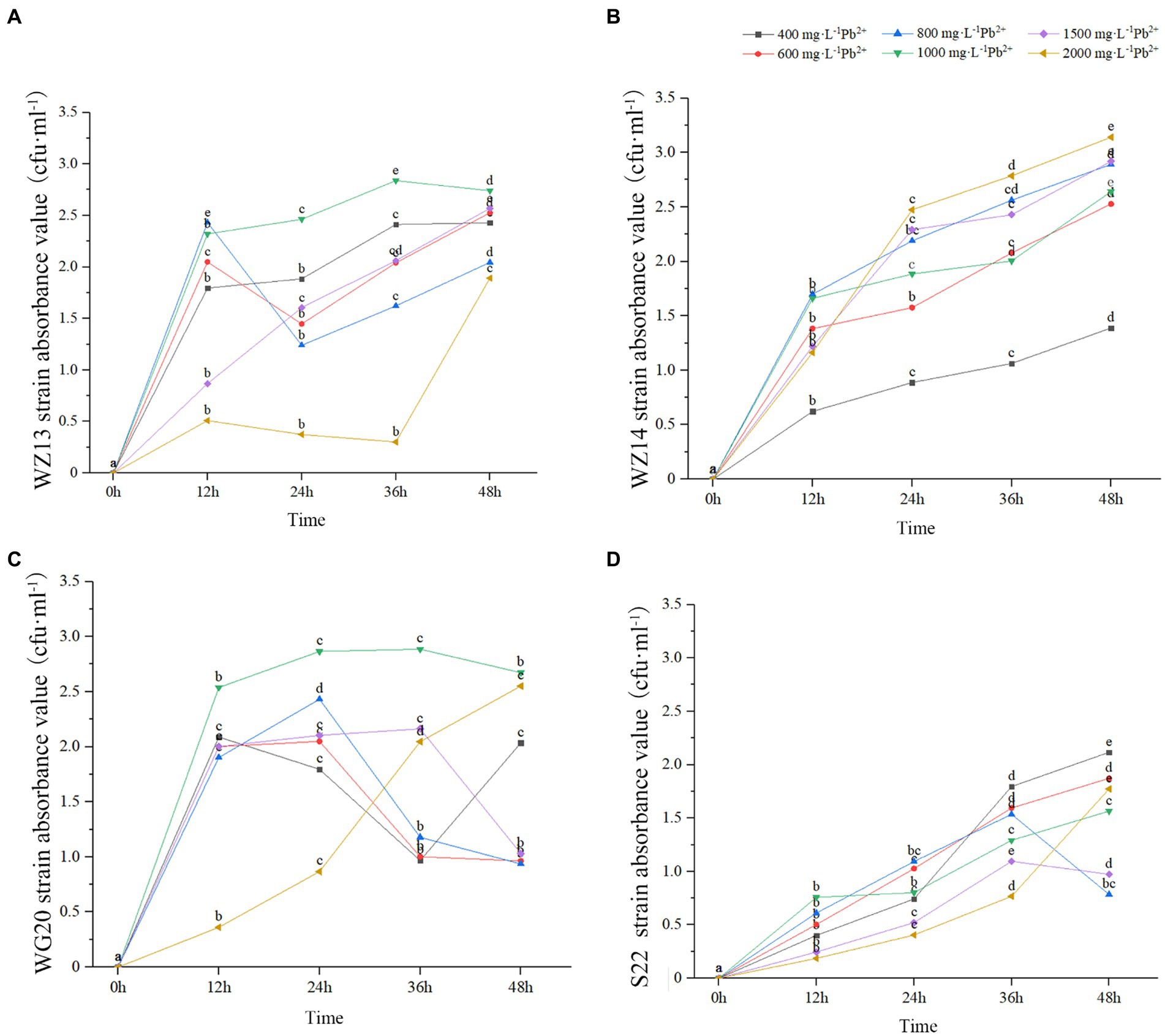
Figure 2. Growth of four strains in medium with different concentrations of Pb2+. Different lowercase letters indicate significant differences between concentrations (p < 0.05). WZ13 strain (A); WZ14 strain (B); WG20 strain (C); S22 strain (D).
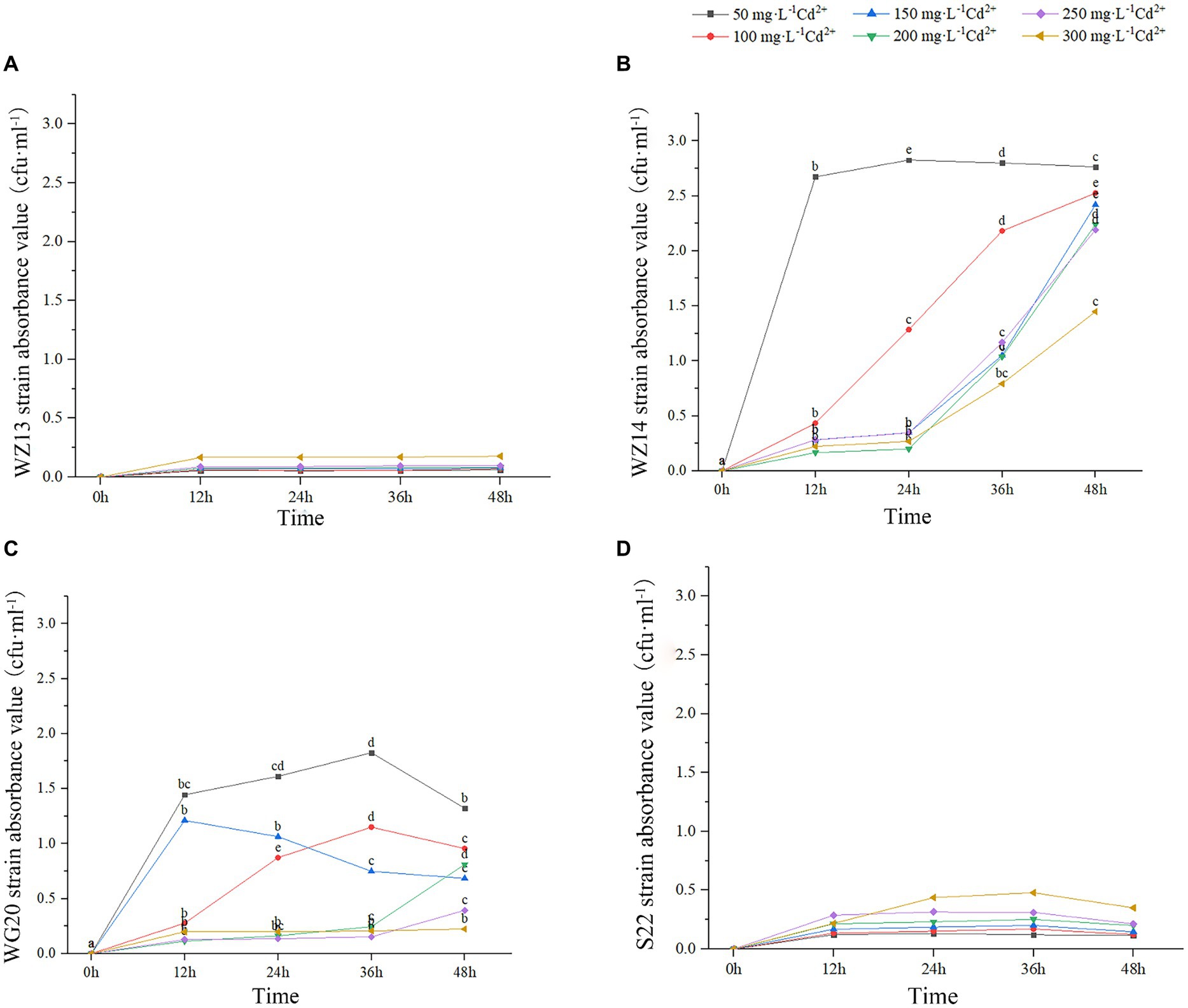
Figure 3. Growth of four strains in medium with different concentrations of Cd2+. Different lowercase letters indicate significant differences between concentrations (p < 0.05). WZ13 strain (A); WZ14 strain (B); WG20 strain (C); S22 strain (D).
WZ14 exhibited the highest resistance and most effective reduction in heavy metal content than other three strains, leading to its selection for the subsequent tests.
3.2. Influence of applied microbial inoculants on nutrient uptake and accumulation of Pb and Cd by plants
Robinia pseudoacacia monoculture, WZ14-R. pseudoacacia, S. xanthantha monoculture, and WZ14-S. xanthantha significantly increased soil pH compared to the soil background (BJZ; Table 1). However, when WZ14 was applied to R. pseudoacacia and S. xanthantha, it slightly reduced the soil pH compared to plant monocultures. All four treatments significantly increased the organic matter content (OC) of the soil compared to BJZ. WZ14-R. pseudoacacia and WZ14-S. xanthantha showed a significant difference, with an increase of 14.36% and 18.29%, respectively, compared to no strain application. For soil alkaline nitrogen content, all treatments significantly reduced it compared to BJZ. Robinia pseudoacacia and S. xanthantha significantly increased soil alkaline nitrogen content by 79.66% and 61.04%, respectively, after applying the WZ14 strain compared to no strain application. All four treatments significantly reduced the soil’s effective phosphorus content compared to BJZ, with R. pseudoacacia having the lowest soil effective phosphorus content significantly after the application of the WZ14 strain. The strain-legume combinations significantly reduced soil fast-acting potassium content compared to plant monocultures with WZ14-R. pseudoacacia showing the most significant reduction. Regarding allotropic nutrients, compared to plant monocultures, both R. pseudoacacia and S. xanthantha showed increased soil allotropic nitrogen, phosphorus, and potassium content after applying the WZ14 strain, indicating a higher potential for allotropic nutrients to transform into effective nutrients that can be easily absorbed by plants. However, the application of WZ14 also caused irregular changes in soil effective nutrient content, influenced by both the decomposition of insoluble nutrients and the absorption capacity of surface plants.
After phytoremediation and combined remediation, the soil Pb and Cd contents were significantly lower than the soil heavy metal contents before potting (Figure 4; p < 0.05). However, the effectiveness of the two legumes in remediating Pb- and Cd-contaminated soil differed after the application of the WZ14 strain. Compared to the single plant treatments, the combined WZ14-R. pseudoacacia and WZ14-S. xanthantha combinations reduced total soil Pb by 13.33% and 19.45%, respectively, and reduced the effective state of soil Pb by 29.63% and 18.11%, respectively. In addition, the WZ14-R. pseudoacacia combination treatment significantly reduced the soil Cd total and Cd active state content by 20.37% and 90.72%, respectively, compared to the soil heavy metal content before potting (Figure 4; p < 0.05). The WZ14-S. xanthantha combination treatment significantly reduced the soil Cd total and Cd active state content by 55.72% and 82.71%, respectively. Regarding the reduction of soil Cd effective state, the plant monoculture treatments of R. pseudoacacia and S. xanthantha showed a reduction of 91.51% and 83.14% in soil Cd effective state, respectively, compared to the soil background values before potting. The WZ14-legume combinations were more effective than single-crop treatments in reducing soil Cd levels but were weaker than single-crop treatments in effectively remediating soil Cd status.
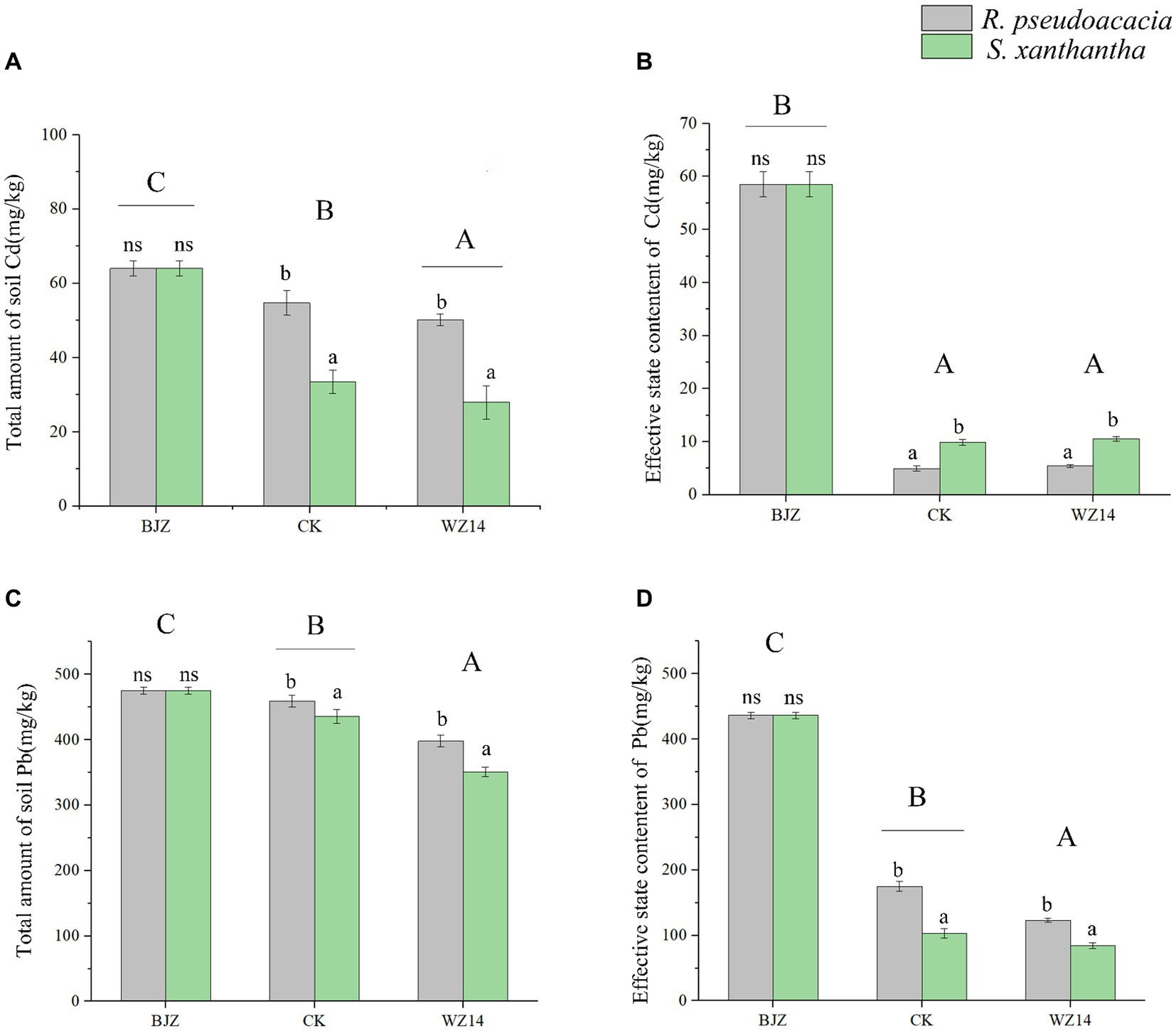
Figure 4. Effect of different treatments on total soil heavy metals Cd (A) and Pb (C) and effective state content of Cd (B) and Pb (D). BJZ, background value of soil before pot experiment; CK, no application of microbial agent; WZ14, the application of WZ14 microbial inoculum. Different lowercase letters indicate significant differences between different plants in the same treatment (p < 0.05). Different uppercase letters indicate significant differences between treatments (p < 0.05). ns: indicates no significant differences between different plants in the same treatment (p < 0.05).
The Pb and Cd contents in the roots of S. xanthantha were significantly higher than in the CK (plant monoculture treatment) and R. pseudoacacia treatments under bacterial addition. In contrast, the Pb content in the stem of the WZ14-R. pseudoacacia treatment was significantly higher than in other treatments (Figure 5, p < 0.05). For R. pseudoacacia plants, Pb content in roots, stems, and leaves was generally low, ranging from 12.37 to 26.07, 3.01 to 7.35, and 1.24 to 3.29 mg·kg−1, respectively. However, when combined with WZ14, the Pb content significantly increased in WZ14-R. pseudoacacia roots by 110.8% and in stems and leaves by 144.2% and 165.3%, respectively, compared to R. pseudoacacia monoculture (Figure 5, p < 0.05). In the case of Cd content, there was no significant difference in the stems of the two plants under the strain application treatment, and both Pb and Cd content in R. pseudoacacia roots were significantly lower than in S. xanthantha under monoculture (p < 0.05). Cd content was higher in S. xanthantha plants, and under the strain application treatment, Cd content in roots, stems, and leaves increased significantly by 130.79%, 88.64%, and 159.13%, respectively, compared to CK (p < 0.05). Pb content in S. xanthantha stems and leaves also increased significantly by 124.9% and 157.8%, respectively, compared to CK (p < 0.05). In comparing the uptake capacity of Pb and Cd, it was observed that the Pb and Cd contents in roots and leaves were significantly higher in the combined WZ14-S. xanthantha treatment than in the combined WZ14-R. pseudoacacia treatment. However, in stems, the Pb content was lower in the combined WZ14-S. xanthantha treatment than in WZ14-R. pseudoacacia, and there was no significant difference in the Cd content between the two (p > 0.05). This pattern was consistent with the single plant treatment, indicating that S. xanthantha (except for the stem) had a better capacity for soil Pb and Cd uptake than R. pseudoacacia (except for the stem).
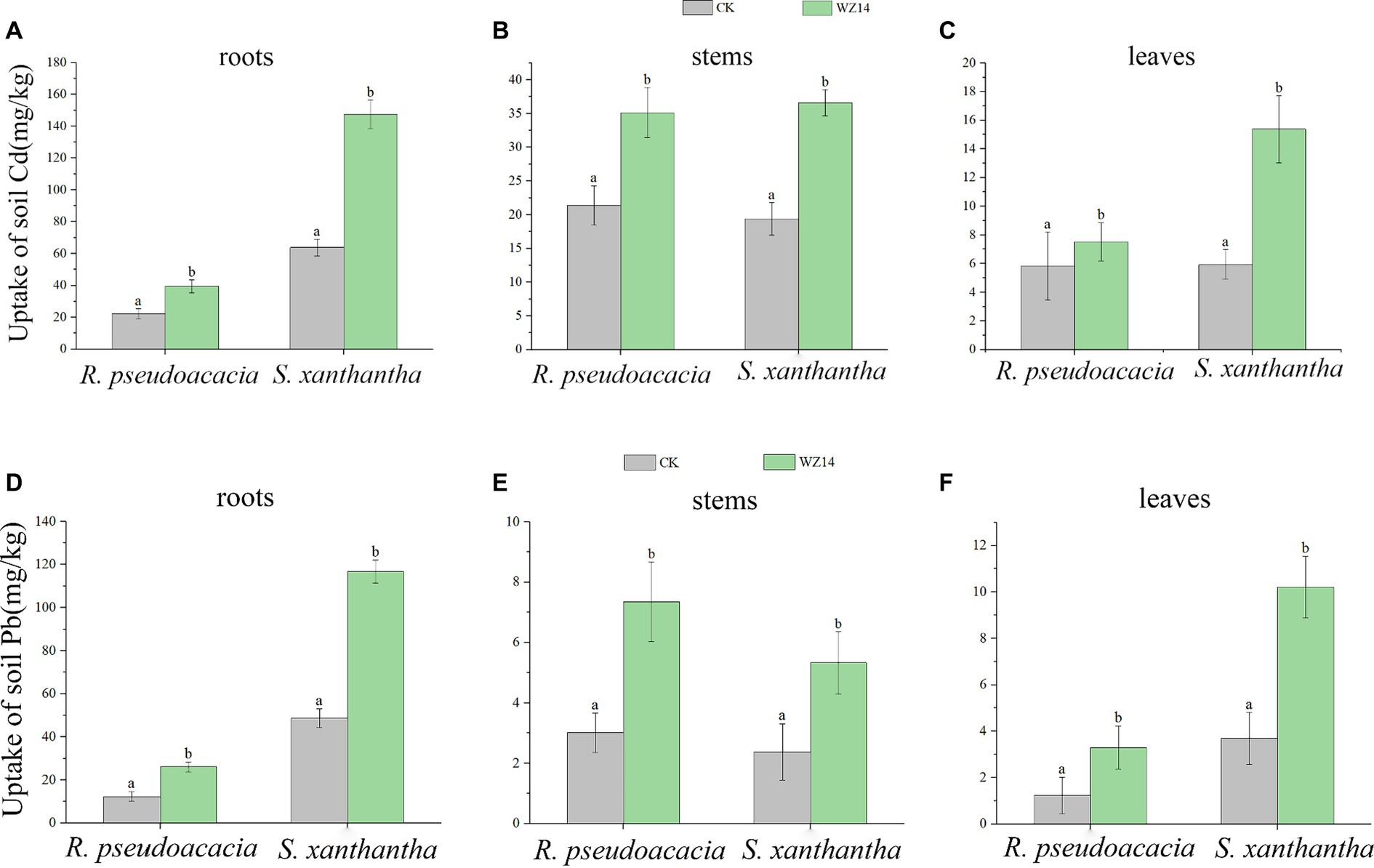
Figure 5. Uptake of soil heavy metals Cd (A–C) and Pb (D–F) by roots, stems, and leaves of Robinia pseudoacacia L. and Sophora japonica. Different lowercase letters indicate significant differences between treatments (p < 0.05).
3.3. Impact of the added bacterial agent on the inter-rhizosphere soil bacterial community
Overall, the addition of the WZ14 strain had varying effects on soil bacterial alpha diversity in R. pseudoacacia and S. xanthantha (Table 2). In R. pseudoacacia, the presence of the WZ14 strain increased the abundance and diversity of soil microbial communities compared to the plant treatment without the WZ14 strain. However, in S. xanthantha soil, the addition of the WZ14 strain significantly reduced the abundance of OTUs and the diversity of bacterial communities, with the Chao1 index and Shannon index decreasing by 9.86% and 6.45%, respectively, compared to S. xanthantha monoculture.
At the phylum level (Figure 6A), the dominant phyla of the bacterial community in the soil samples were Proteobacteria, Bacteroidetes, Patescibacteria, Chloroflexi, and Acidobacteria, each representing the relative abundance of more than 5% of the total soil bacterial community in the upper soil, accounting for 64.48% to 72.61%. After inoculation with WZ14, the relative abundances of Proteobacteria and Firmicutes in the soil of R. pseudoacacia and S. xanthantha significantly increased after inoculation with WZ14 compared to the treatment without microbial inoculants. Specifically, the relative abundance of Proteobacteria in the soil of R. pseudoacacia increased from 32.76% to 37.42% and in S. xanthantha from 20.92% to 29.78%, while Firmicutes increased from 2.60% to 5.35% in R. pseudoacacia and from 3.52% to 6.88% in S. xanthantha. Additionally, WZ14 inoculation significantly increased the relative abundance of Bacteroidetes from 10.28% to 17.91% (p < 0.05) and reduced the relative abundance of Patescibacteria from 13.51% to 8.01% in R. pseudoacacia and from 20.49% to 10.18% in S. xanthantha soils, respectively. These findings suggested that WZ14 inoculation may play a vital role in improving plant quality and remediating heavy metal contamination by increasing the abundance of dominant bacterial communities, particularly the relative abundance of Proteobacteria, Bacteroidetes, and Firmicutes.
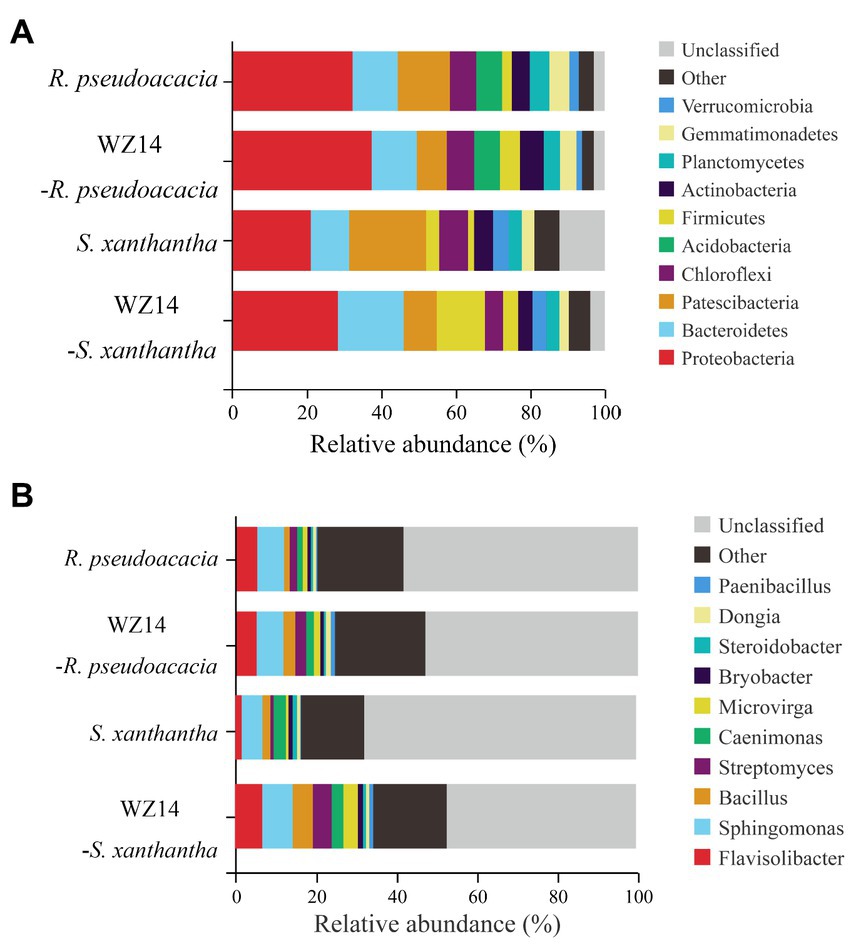
Figure 6. Effects of different treatments on the relative abundance of major phyla (A) and genera (B) of soil bacterial communities.
At the genus level (Figure 6B), the dominant genera in the soil samples were Flavisolibacter, Sphingomonas, Bacillus, Streptomyces, and Paenibacillus. The addition of WZ14 significantly increased the relative abundance of the dominant bacterial genera (total relative abundance of the five most abundant genera). Specifically, the relative abundances of Flavisolibacter, Sphingomonas, and Bacillus increased from 1.79%, 5.19% and 1.98% to 7.02%, 7.57%, and 5.03%, respectively, after the addition of WZ14. However, the relative abundance of Paenibacillus in the soil was significantly reduced. Similar trends were observed in the abundance of Bacillus and Streptomyces in R. pseudoacacia soils, with relative abundances increasing from 1.43% and 1.82% to 2.96% and 2.71%, respectively, after WZ14 application. However, the application of WZ14 did not significantly change the abundance of Sphingomonas and Flavisolibacter in R. pseudoacacia soils.
3.4. Correlation between inter-root bacterial communities and soil environmental factors
Spearman’s correlation analysis was used to clarify the relationship between soil bacterial communities and soil physicochemical factors in R. pseudoacacia and S. xanthantha after WZ14 inoculant application at the phylum and genus levels.
According to Spearman’s correlation heat map (Figure 7), Verrucomicrobia exhibited significant positive correlations with soil pH, AK, AP, and TN while showing a significant negative correlation with soil solution AN content. On the other hand, Firmicutes showed a significant negative correlation with soil pH and AK. Additionally, Bacteroidota showed significant positive correlations with OC and AN and negative correlations with soil pH, AP, AK, TN, and TK in S. xanthantha soils. Acidobacteriota, in the same soils, demonstrated significant negative correlations with OC and AN while positively correlating with AK. At the genus level, environmental factors appeared to mainly affect Bacillus, Steroidobacter, and Paenibacillus in R. pseudoacacia. For example, Bacillus showed significantly negative correlations with soil pH, AP, AK, and TN, but positive correlations with OC and AN. TP exhibited a significant positive correlation with Steroidobacter, and pH showed a negative correlation with Paenibacillus. Flavisolibacter in S. xanthantha soils showed highly significant negative correlations with AK and TK while exhibiting positive correlations with OC and AN. Microvirga showed significant negative correlations with AK and TK and positive correlations with OC and AN. Steroidobacter indicated significant negative correlations with pH, AK, TN, and TK and positive correlations with AN. This study identified that soil Pb content was significantly and positively correlated with Verrucomicrobia, but negatively correlated with Bacteroidota. The effective state of soil Cd was significantly and negatively correlated with Gemmatimonadetes, while the total soil Cd was significantly and negatively correlated with Bacteroidota.
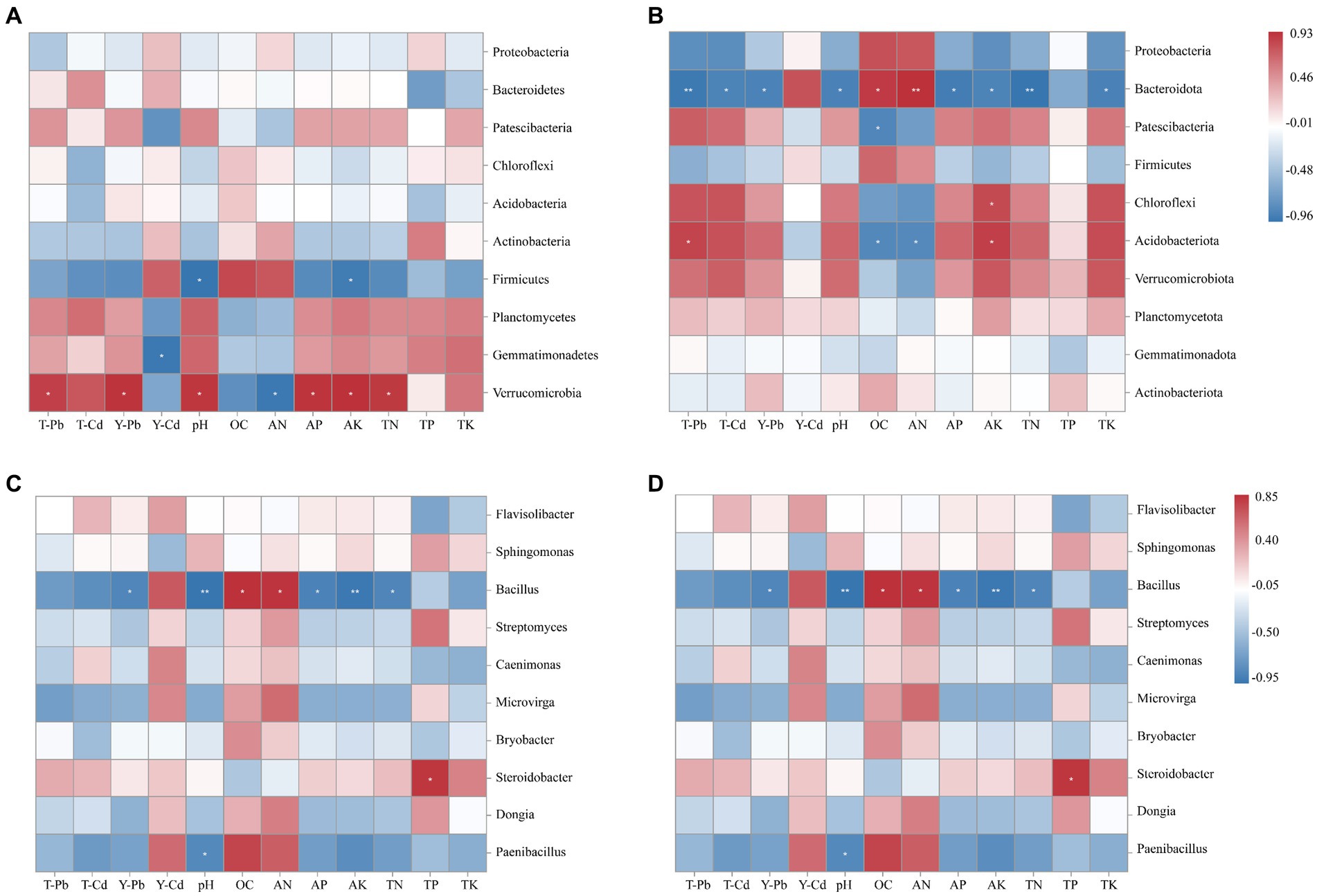
Figure 7. Spearman analysis between soil physicochemical properties in Robinia pseudoacacia (A,C) and Sophora xanthantha (B,D) and soil bacterial community at phylum and genus levels. Color represents R value; * indicates p < 0.05; ** indicates 0.05 ≤ p ≤ 0.01; *** indicates p < 0.01.
4. Discussion
4.1. Effect of soil physicochemical properties under combined remediation
Soil physicochemical properties are fundamental indicators used to characterize soil nutrients, which in turn determine the overall fertility level of the soil. The presence of volatile nutrients, susceptible to environmental influences, directly reflects soil quality (Glick, 2010). In this context, microorganisms act as essential “regulators” of geobiochemical processes, playing a vital role in maintaining soil vitality and ecological functions (Liu et al., 2023).
The total soil nutrient content, including total N and total P, and fast-acting nutrients demonstrated a decrease but without significant difference, consistent with the findings of Hussain et al. (2018). Soil nutrient loss is directly related to heavy metal contamination, with higher contamination levels leading to more significant loss of soil nitrogen, phosphorus, potassium, and fast-acting nutrients. Moreover, in this study, the observed changes in soil phosphorus content after applying mycorrhizal fungi can be attributed to the enhancement of plant nutrient uptake by these fungi. This, in turn, promoted plant growth and optimized the plant’s ability to remediate heavy metals through phosphorus solubilization (Table 1; Guo et al., 2013; Hu et al., 2019). Soil pH changes can directly affect the effective levels of soil nutrients, microbial activities, and the toxic effects of heavy metal ions. Under Cd and Pb contamination, both monoculture and combined remediation improved the soil organic carbon levels to some extent, with the combined system of inoculated bacteria and legumes showing the best results. However, propagating plants under heavy metal contamination reduced the alkaline-dissolved nitrogen levels of the soil. The inoculation of bacteria in plant monocultures inhibited the declining impact of plants on soil alkaline digestion of nitrogen. It also mitigated the decline in soil alkaline-dissolved nitrogen, consistent with the findings of Cui (2019) and Hussain et al. (2018). This may be attributed to the ability of inoculation treatment to enhance the accumulation of soil carbon and nitrogen nutrient to varying degrees, promote plant root growth, and improve the antagonistic ability of plants.
4.2. Effect of soil Pb and Cd uptake under combined remediation
Heavy metals in soil exist in various forms. The exchangeable form of heavy metals in soil is highly mobile and easily absorbed by plants, unlike the organic, carbonate, and ferro-manganese oxidation forms, which are less readily absorbed. Determination of the effective state content of heavy metals is an effective way to determine the extent of heavy metal contamination and to predict the impact of heavy metals on ecosystems (Zhou et al., 2017). Therefore, the primary objective of remediation is to reduce the amount of active heavy metals in the soil, which in turn affects their crop uptake (Wang et al., 2020). Numerous studies have demonstrated that the interaction between plant and microorganisms can enhance plant biomass and improve heavy metal tolerance, facilitating the absorption, fixation, and reduction of heavy metal concentrations in the soil. This process reduces the toxic effects of heavy metals (Khan et al., 2017; Fu et al., 2022; Zheng et al., 2022a). In this study, the combined treatment with WZ14 was more effective than individual plant treatments. The roots of R. pseudoacacia and S. xanthantha showed significantly higher levels of Pb and Cd than stems and leaves, which were the primary sites of soil Pb and Cd uptake. This difference was due to the limited translocation capacity of non-enriched plants, leading to the accumulation of Pb and Cd in their roots. These findings aligned with previous research (Sun and Mao, 2015).
Microorganisms in the soil play a crucial role in both the formation of soil humus and the mineralization of organic matter, the uptake and accumulation of soil Pb and Cd by R. pseudoacacia and S. xanthantha were strongly influenced by soil properties and fertility. In this study, the addition of WZ14 bacterial agent increased the OC content of soils occupied by R. pseudoacacia and S. xanthantha. Additionally, it enhanced the metabolic processes of inter-root microorganisms and their byproducts. These changes influenced the migration and release mechanisms of Pb and Cd, leading to reduced toxicity of heavy metals in the soil.
4.3. Correlation analysis of soil environmental factors and soil bacterial communities
Strong correlations exist between plant and soil microbial communities, with plant root secretions and residues influencing the function and structure of soil microbial communities (Bian et al., 2018). In this study, the addition of WZ14 bacterial agent had contrasting effects on soil bacterial communities of R. pseudoacacia and S. xanthantha. Although it enriched the richness and diversity of soil bacterial communities in R. pseudoacacia, it reduced the richness and diversity in S. xanthantha. These changes could be due to short-term responses of bacterial communities to environmental and pollution changes, reflecting their microenvironmental conditions (Yao et al., 2019). Although the exogenous soil microorganism WZ14 partially promoted the development of heavy metal-tolerant microorganisms, it also intensified competition among soil microorganisms. Consequently, less adaptable microorganisms struggled to cope with environmental changes and experienced a decline in abundance and diversity (Zhang et al., 2020).
The majority of bacterial communities in this study showed a significant negative correlation with soil physicochemical factors, possibly due to the ability to decompose and utilize soil nutrients for energy, either for themselves or plants (Yao et al., 2022). The results of this study revealed that Proteobacteria had the highest relative abundance in both monoculture and grafted-plant combination models, indicating the prevalence of Proteobacteria in heavy metal-contaminated soils and their tolerance to high levels of Cd and Pb contamination (Gupta et al., 2017). Proteobacteria emerged as the dominant phylum in the heavy metal-contaminated soil of the mining area and positively contributed to improving soil contamination status. However, its relative abundance was negatively correlated with the overall soil quality (Yu et al., 2020; Ma J. D. et al., 2022). In contrast, our study discovered the highest abundance of Proteobacteria in the soil of R. pseudoacacia and S. xanthantha when the heavy metal contamination level decreased after the application of the microbial inoculant. This discrepancy may arise from the specific focus of our experiment on a combined Pb and Cd contamination pattern, resulting in different responses of bacterial communities to Pb and Cd under heavy metal-contaminated conditions.
5. Conclusion
Microorganisms residing in heavy metal environments for extended periods have developed a notable tolerance and resistance to heavy metal ions. In order to address heavy metal contamination in soil, one of the effective approaches can be exploring heavy metal-resistant bacteria for their capacity to absorb and accumulate heavy metals (Zheng et al., 2022b). In this study, we successfully isolated and purified an efficient Cd- and Pb-tolerant strain from the contaminated soil of lead-zinc-silver mine in Zhijiadi, Shanxi Province. This strain exhibited robust acclimation in high Pb2+ concentration cultures (2,000 mg/L and 1,500 mg/L), with peak values ranging from 2.92 cfu·mL−1 to 3.14 cfu·mL−1. Additionally, it demonstrated strong viability and adaptability in Cd2+ cultures at varying concentration gradients, showing growth even at concentrations exceeding 100 mg/L, with growth ranging from 1.45 cfu·mL−1 to 2.77 cfu·mL−1. Both R. pseudoacacia and S. xanthantha are suitable for cultivation in heavy metal-contaminated soils, as they can absorb and translocate heavy metals, such as Pb and Cd, resulting in an improved soil microenvironment. Combined with crop-applied WZ14 inoculants, the remediation efficacy was enhanced, resulting in increased soil AN and OC levels and decreased total Pb and active heavy metals compared to monoculture. Furthermore, the application of WZ14 significantly facilitated the uptake of Cd by S. xanthantha, particularly in the roots, where the Cd uptake reached 147.44 mg/kg after microbial inoculant. This represented a substantial increase of 130.79% compared to monoculture, signifying the root’s significant role in the remediation of soil Cd contamination by S. xanthantha. The community structure of soil microorganisms in heavy metal-contaminated areas exhibited significant differences between planting and combined remediation treatments, resulting in notable changes in microbial abundance and diversity. The combination of R. pseudoacacia monoculture with WZ14-R. pseudoacacia proved to be particularly effective in enhancing microbial diversity, as the WZ14-R. pseudoacacia soils displayed the highest abundance and diversity of microorganisms compared to other treatments. In heavy metal-contaminated soils, the dominant bacterial phyla were identified as Proteobacteria, Bacteroidetes, Patescibacteria, Chloroflexi, and Acidobacteria. The inoculation with WZ14 significantly increased the relative abundance of Proteobacteria and Firmicutes. Soil microorganisms have been proven crucial in remediating heavy metal-contaminated soils, with combined mycorrhizal agents and plant remediation proving highly promising for reducing heavy metal content and enhancing the soil environment.
Data availability statement
The datasets presented in this study can be found in online repositories. The names of the repository/repositories and accession number(s) can be found at: NCBI—OR492361, PRJNA1012100.
Author contributions
KZ: Conceptualization, Data curation, Writing – original draft. ZL: Investigation, Software, Writing – review & editing. CL: Formal Analysis, Methodology, Supervision, Writing – review & editing. JL: Methodology, Supervision, Writing – review & editing. JZ: Funding acquisition, Project administration, Resources, Writing – review & editing.
Funding
The author(s) declare financial support was received for the research, authorship, and/or publication of this article. This work was funded by the National Key R&D Program of China (2017YFC0505500 and 2019JSJG247).
Conflict of interest
The authors declare that the research was conducted in the absence of any commercial or financial relationships that could be construed as a potential conflict of interest.
Publisher’s note
All claims expressed in this article are solely those of the authors and do not necessarily represent those of their affiliated organizations, or those of the publisher, the editors and the reviewers. Any product that may be evaluated in this article, or claim that may be made by its manufacturer, is not guaranteed or endorsed by the publisher.
References
Abhilash, P. C., Powell, J. R., Singh, H. B., and Singh, B. K. (2012). Plant-microbe interactions: novel applications for exploitation in multipurpose remediation technologies. Trends Biotechnol. 30, 416–420. doi: 10.1016/j.tibtech.2012.04.004
Atikur-Rahman, M., Hossain, A., Riazul-Islam, M., Azim, A., Gaber, A., and Aftab, T. (2022). Metals and metalloids stress in plants: microorganisms and phytoremediation based mitigation strategies. Metals Metalloids Soil Plant Water Syst. 2022, 445–484. doi: 10.1016/B978-0-323-91675-2.00009-3
Beiyuan, J., Fang, L., Chen, H., Li, M., Liu, D., Li, M., et al. (2021). Nitrogen of EDDS enhanced removal of potentially toxic elements and attenuated their oxidative stress in a phytoextraction process. Environ. Pollut. 268:115719. doi: 10.1016/j.envpol.2020.115719
Bian, F. Y., Zhong, Z. K., and Zhang, X. P. (2018). Remediation effect of moso bamboo and companion Sedum on heavy metal-contaminated soil and the effect on microbial community. For Sci. 54, 106–116.
Cai, B. Y., Wang, L. Y., Hu, W., Jie, W. G., and Ling, H. G. (2015). Analysis of community structure of root rot pathogenic Fungi in seedling stage of soybean continuous cropping. Chin Agri Sci Bull. 31, 92–98.
Cui, T. T. (2019). Effect of inoculation of Bacillus coli in legume and gram intercropping systems on plant heavy metal uptake characteristics. Master Thesis, Northwest Agriculture and Forestry University. 1–64.
Dary, M., Chamber-Perez, M. A., Palomares, A. J., and Pajuelo, E. (2010). “In situ” phytostabilisation of heavy metal polluted soils using Lupinus luteus inoculated with metal resistant plant-growth promoting rhizobacteria. J. Hazard. Mater. 177, 323–330. doi: 10.1016/j.jhazmat.2009.12.035
Duan, G. L., Cui, H. L., Yang, Y. P., Yi, X. Y., Zhu, D., and Zhu, Y. G. (2020). Interactions among soil biota and their applications in synergistic bioremediation of heavy-metal contaminated soils. Chin. J. Biotechnol. 36, 455–470. doi: 10.13345/j.cjb.190598
Evlat, H., Toker, S. K., and Koçyiğit, A. (2023). Screening for agroactive and bioactive metabolites production by actinobacteria isolated from rhizospheric soils. Biologia 78, 187–200. doi: 10.1007/S11756-022-01226-0
Fang, F., Peng, Z. D., Guo, Z. M., Ma, X., Sun, L., and Jiang, H. (2012). Study on seed hardness characteristic and germination promoting of Robinia pseudoacacia seeds. J Central South Univ Forestry Technol. 33, 72–76. doi: 10.14067/j.cnki.1673-923x.2013.07.016
Fatima, K., Imran, A., Amin, I., and Afzal, M. (2016). Plant species affect colonization patterns and metabolic activity of associated endophytes during phytoremediation of crude oil-contaminated soil. Environ. Sci. Pollut. Res. 23, 6188–6196. doi: 10.1007/s11356-015-5845-0
Fu, Y., Wang, Y., Qiu, Y. L., Huang, X. Q., and Bu, S. W. (2022). Research Progress of toxic effects and mechanism in heavy metal Coba Stresson plant. Advances in the study of toxic effects and mechanisms of heavy metal cobalt stress on plants. Environ Sci Manage. 47, 65–70.
Glick, R. B. (2010). Using soil bacteria to facilitate phytoremediation. Biotechnol. Adv. 28, 367–374. doi: 10.1016/j.biotechadv.2010.02.001
Gonalves, A. C., Schwantes, D., Sousa, R., Zimmermann, J., Guimaraes, V. F., and Campagnolo, M. A. (2020). Phytoremediation capacity, growth and physiological responses of Crambe abyssinica Hochst on soil contaminated with cd and Pb. J. Environ. Manage. 262:110342. doi: 10.1016/j.jenvman.2020.110342
Guo, J. K., and Chi, J. (2017). 2014. Effect of cd-tolerant plant growth-promoting rhizobium on plant growth and cd uptake by Lolium multiflorum, lam. And Glycine max, (L.) Merr. In cd-contaminated soil. Plant and Soil 375, 205–214. doi: 10.1007/s11104-013-1952-1
Guo, S. Y., Xiao, C. Q., Zhou, N., and Chi, R. A. (2021). Speciation, toxicity, microbial remediation and phytoremediation of soil chromium contamination. Environ. Chem. Lett. 19, 1413–1431. doi: 10.1007/s10311-020-01114-6
Guo, W., Zhao, R., Zhao, W., Fu, R., Guo, J., Bi, N., et al. (2013). Effects of arbuscular mycorrhizal Fungi on maize (Zea mays L.) and Sorghum (Sorghum bicolor L. Moench) grown in rare earth elements of mine tailings. Appl. Soil Ecol. 72, 85–92. doi: 10.1016/j.apsoil.2013.06.001
Gupta, A., Dutta, A., Sarkar, J., Paul, D., Panigrahi, M. K., and Sar, P. (2017). Metagenomic exploration of microbial community in mine tailings of Malanjkhand copper project, India. Genomics Data. 12, 11–13. doi: 10.1016/j.gdata.2017.02.004
Hei, Z. W., Xiang, H. M., Zhang, J. E., and Liang, K. M. (2019). Advances in legumes-based remediation of heavy metals contaminated soil. Ecol Sci. 38, 218–224. doi: 10.14108/j.cnki.1008-8873.2019.03.027
Hu, Z. H., Zhuo, F., Jing, S. H., Li, X., Yan, T. X., Lei, L. L., et al. (2019). Combined application of arbuscular mycorrhizal Fungi and steel slag improves plant growth and reduces cd, Pb accumulation in Zea mays. Int. J. Phytoremediation 21, 857–865. doi: 10.1080/15226514.2019.1577355
Hussain, F., Hussain, I., Khan, A. H. A., Muhammad, Y. S., Iqbal, M., Soja, G., et al. (2018). Combined application of biochar, compost, and bacterial consortia with Italian ryegrass enhanced phytoremediation of petroleum hydrocarbon contaminated soil. Environ. Exp. Bot. 153, 80–88. doi: 10.1016/j.envexpbot.2018.05.012
Ibiang, S. R., Sakamoto, K., and Kuwahara, N. (2020). Performance of tomato and lettuce to arbuscular mycorrhizal fungi and Penicillium pinophilum EU0013 inoculation varies with soil, culture media of inoculum, and fungal consortium composition. Rhizosphere. 16:100246. doi: 10.1016/j.rhisph.2020.100246
Jiang, Y. Y., Zheng, Y., Tang, L., Jing, X., Jie, Z., and Ke-xin, Z. (2016). Rhizosphere biological processes of legume-cereal intercropping systems: a review. J Agri Resourc Environ. 33, 407–415. doi: 10.13254/j.jare.2016.0121
Khan, A. R., Waqas, M., Ullah, I., Khan, A. L., Khan, M. A., Lee, I. J., et al. (2017). Culturable endophytic fungal diversity in the cadmium hyperaccumulator Solanum nigrum L. and their role in enhancing phytoremediation. Environ. Exp. Bot. 135, 126–135. doi: 10.1016/j.envexpbot.2016.03.005
Li, Y. M. (2021). Study on bacteria assisted phytoremediation of Cd and Zn contaminated soils by Brassica juncea, coss. North West Agriculture and Forestry University, 1–69. doi: 10.27409/d.cnki.gxbnu.2021.001671
Li, C., Kong, X. X., Yu, Y. B., Zou, X., and Luo, H. (2020). Analysis of microflora diversity in crab paste based on high-throughput sequencing. Food Sci. 41:134. doi: 10.7506/spkx1002-6630-20180920-233
Li, H. Y., Xiong, Z., Li, X. Y., and Chu, L. (2017). Research on plant-microbe combined remediation of heavy metal contaminated soil. J Kunming Univ Sci Technol 42, 81–88. doi: 10.16112/j.cnki.53-1223/n.2017.03.013
Liu, C. J. (2022). Effects and mechanisms of endophyte-enhanced remediation of cadmium-contaminated soil by commercial land[D]. University of Science and Technology Beijing. Beijing.
Liu, S. L., Ali, S., Yang, R. J., Tao, J. J., and Ren, B. (2019). A newly discovered cd-hyperaccumulator Lantana camara. J. Hazard. Mater. 371, 233–242. doi: 10.1016/j.jhazmat.2019.03.016
Liu, Z. X., Zhuang, J. Y., Liu, C., Zheng, K., and Chen, L. (2023). Bacterial community analysis and ecological function characteristics of soil with different contamination levels in Datong Pb-Zn tailings. Environ. Sci., 44: 4191–4200. doi: 10.13227/j.hjkx.202209160
Lou, J., Liu, M., Gu, J., Liu, Q., Zhao, L., Ma, Y., et al. (2019). Metagenomic sequencing reveals microbial gene catalogue of phosphinothricin-utilized soils in South China. Gene 711:143942. doi: 10.1016/j.gene.2019.143942
Luo, Y. H., Sun, Y. Y., and Wang, N. (2019). Research progress of biochar remediation of soil heavy metal pollution. Western Dev. 4, 28–35.
Ma, Y., Wang, Y., Shi, X. J., Chen, X. P., and Li, Z. L. (2022). Mechanism and application of plant growth-promoting Bacteria in heavy metal bioremediation. Environ. Sci. 43, 4911–4922. doi: 10.13227/j.hjkx.202112007
Ma, H., Wei, M., Wang, Z., Hou, S., Li, X., and Xu, H. (2020). Bioremediation of cadmium polluted soil using a novelcadmium immobilizing plant growth promotion strain Bacillus sp. TZ5 loaded on biochar. J. Hazard. Mater. 388:122065. doi: 10.1016/j.jhazmat.2020.122065
Ma, J., Dong, W. X., Zhu, Y. Y., Xiao, D., and Chen, F. (2022). Microbial community characteristics of reclaimed soils in the eastern plain and their assembly processes. Environ. Sci. 43, 3844–3853. doi: 10.13227/j.hjkx.202110018
Maynaud, G., Brunel, B., Mornico, D., Durot, M., Severac, D., Dubois, E., et al. (2013). Genome-wide transcriptional responses of two metal-tolerant symbiotic Mesorhizobium isolates to zinc and cadmium exposure. BMC Genomics 14, 2–24. doi: 10.1186/1471-2164-14-292
Ni, X., Li, Y. Q., Bai, S., and Ye, Z. Q. (2019). Study on the remediation effect of activator combined with willow on heavy metal cd contaminated soil[J]. J. Soil Water Conserv. 33, 365–371. doi: 10.13870/j.cnki.stbcxb.2019.03.053
Pereira, S. I. A., Lima, A. I. G., and Figueira, A. E. M. D. A. (2006). Screening possible mechanisms mediating cadmium resistance in Rhizobium leguminosarum bv. Viciae isolated from contaminated Portuguese soils. Microb. Ecol. 52, 176–186. doi: 10.1007/s00248-006-9057-5
Quast, C., Pruesse, E., Yilmaz, P., Gerken, J., Schweer, T., Yarza, P., et al. (2012). The silva ribosomal rna gene database project: improved data processing and web-based tools. Nucleic Acids Res. 41, D590–D596. doi: 10.1093/nar/gks1219
Rachelle, E. B., Wyatt, H., and Maria, F. C. (2018). Variation in microbial community structure correlates with heavy-metal contamination in soils decades after mining ceased. Soil Biol. Biochem. 126, 57–63. doi: 10.1016/j.soilbio.2018.08.011
Saxena, G., Purchase, D., Mulla, S. I., Saratale, G. D., and Bharagava, R. N. (2019). Phytoremediation of heavy metal-contaminated sites: eco-environmental concerns, field studies, sustainability issues, and future prospects. Rev. Environ. Contam. Toxicol. 249, 71–131. doi: 10.1007/398_2019_24
Schloter, M., Nannipieri, P., Sørensen, S. J., and Elsas, J. D. V. (2018). Microbial indicators for soil quality. Biol. Fertil. Soils 54, 1–10. doi: 10.1007/s00374-017-1248-3
Sessitsch, A., Kuffner, M., Kidd, P., Vangronsveld, J., Wenzel, W. W., Fallmann, K., et al. (2013). The role of plant-associated bacteria in the mobilization and phytoextraction of trace elements in contaminated soils. Soil Biol. Biochem. 60, 182–194. doi: 10.1016/j.soilbio.2013.01.012
Shi, X., Chen, Y. T., Wang, S. F., and Li, J. C. (2012). Pb, Zn accumulation and nutrient uptake of 15 plant species grown in abandoned mine tailings. Environ. Sci. 33, 2021–2027. doi: 10.13227/j.hjkx.2012.06.006
Shuaib, M., Azam, N., Bahadur, S., Romman, M., Qian, Y., and Chang, X. X. (2021). Variation and succession of microbial communities under the conditions of persistent heavy metal and their survival mechanism. Microb. Pathog. 150:104713. doi: 10.1016/j.micpath.2020.104713
Słomka, A., Jedrzejczyk-Korycinska, M., Rostanski, A., Karcz, J., Kawalec, P., and Kuta, E. (2012). Heavy metals in soil affect reproductive processes more than morphological characters in Viola tricolor. Environ. Exp. Bot. 75, 204–211. doi: 10.1016/j.envexpbot.2011.07.003
Sun, Q. W., and Mao, Q. (2015). Analysis of microbial contribution to soil fertility. Agri Technol Serv 32:96.
Wang, Q., Duan, C. J., Xu, C. Y., and Geng, Z. C. (2022). Efficient removal of cd (II) by phosphate-modified biochar’s derived from apple tree branches: processes, mechanisms, and application. Sci. Total Environ. 819:152876. doi: 10.1016/j.scitotenv.2021.152876
Wang, Q., Garrity, G. M., Tiedje, J. M., and Cole, J. R. (2007). Naive bayesian classifier for rapid as signment of rRNA sequences into the new bacterial taxonomy. Appl. Environ. Microbiol. 73, 5261–5267. doi: 10.1128/AEM.00062-07
Wang, Y. T., Wang, Z. Y., Liu, T. T., Liu, Y., and Zhang, D.. (2020). Effect of passivators on the remediation effect of cadmium-contaminated soil and the physiological effect of green vegetables. Environ. Chem. 39, 2395–2403. doi: 10.7524/j.issn.0254-6108.2020032505
Wood, J. L., Tang, C. X., and Franks, A. E. (2016). Microbial associated plant growth and heavy metal accumulation to improve phytoextraction of contaminated soils. Soil Biol. Biochem. 103:131. doi: 10.1016/j.soilbio.2016.08.021
Wu, B. Q., Liu, S. J., Zhang, M., Chen, F. M., Liu, L. H., Yang, X. L., et al. (2014). Effects of inoculation with cadmium-tolerant bacteria on the uptake and accumulation of cadmium in soil by Celosia argentea. J. Ecol. 33, 3409–3415. doi: 10.13292/j.1000-4890.2014.0307
Xiao, L., Bi, Y., Du, S., Wang, Y., Guo, C., and Christie, P. (2021). Response of ecological stoichiometry and stoichiometric homeostasis in the plant-litter-soil system to re-vegetation type in arid mining subsidence areas. J. Arid Environ. 184:104298. doi: 10.1016/j.jaridenv.2020.104298
Xiao, C. Q., Guo, S. Y., Wang, Q., and Chi, R. A. (2021). Enhanced reduction of lead bioavailability in phosphate mining wasteland soil by a phosphate-solubilizing strain of Pseudomonas sp., LA, coupled with ryegrass (Lolium perenne L.) and sonchus (Sonchus oleraceus L.). Environ. Pollut. 274:116572. doi: 10.1016/j.envpol.2021.116572
Xiao, C. Q., Zheng, C. L., Zhang, Y. F., He, H., and Ilyas, S. (2023). Editorial: application of microbial technology in ecological remediation of mines. Front. Microbiol. 14:1136851. doi: 10.3389/fmicb.2023.1136851
Yao, J., Bao, L. N., Jiang, Y., and Zhu, S. G. (2022). Combined plant-lead-tolerant microbial remediation of lead-contaminated soil. J Shandong Univ Sci Technol. 36, 7–13.
Yao, X. D., Li, X. G., Ding, C. F., Hang, Z. M., and Wang, X. X. (2019). Microzone distribution characteristics of soil microbial community with peanut cropping system, monocropping or rotation. Soil J. 56, 975–985. doi: 10.13367/j.cnki.sdgc.2022.04.009
Yu, F. M., Yao, Y. W., Xie, D. Y., Wang, X. R., Lin, J. M., Liu, Y., et al. (2020). Structural characteristics of soil microbial communities in six land use types in the siding mining area. China Environ. Sci. 40, 2262–2269. doi: 10.19674/j.cnki.issn1000-6923.2020.0259
Zeng, C. Y. (2017). Study on resistance mechanism and remediation potential of three woody leguminous plants to copper tailings ore. MA thesis of Jiangxi University of Finance and Economics.
Zhang, Y., He, L., Chen, Z., Wang, Q. Y., Qian, M., and Sheng, X. F. (2011). Characterization of ACC deaminase-producing endophytic bacteria isolated from copper-tolerant plants and their potential in promoting the growth and copper accumulation of Brassica napus. Chemosphere 83, 57–62. doi: 10.1016/j.chemosphere.2011.01.041
Zhang, J. F., Wang, C., Xu, W. W., Huang, X. R., and Zhang, Z. D. (2020). Characteristics of soil nutrient and bacterial community changes in northern China larch plantations in different generations. J Beijing Forestry Univ. 42, 36–45.
Zheng, Y. T., Li, Y. Z., Guo, S. Y., Yu, J. X., Chi, R. A., and Xiao, C. Q. (2022a). Enhanced phytoremediation of hexavalent chromium contamination in phosphate mining wasteland by a phosphate solubilizing bacterium. Biorem. J., 15, 1–12. doi: 10.1080/10889868.2022.2136135
Zheng, Y. T., Yu, S. Y., Li, Y. Z., Pen, J., Yu, J. X., Ruan, C., et al. (2022b). Efficient bioimmobilization of cadmium contamination in phosphate mining wastelands by the phosphate solubilizing fungus Penicillium oxalicum ZP6. Biochem. Eng. J. 187:108667. doi: 10.1016/j.bej.2022.108667
Zheng, Y. R., Zhao, J. C., Gong, S. F., and Wang, J. G. (2021). Effects of NaHCO3 and Na2CO3 stress on seed germination, seedling growth, and physiological indexes of Puccinelia chinampoensis and Elymus dahuricus. J Univ Chin Acad Sci. 38, 228–239.
Zhou, W. H., Zhang, J. J., Zou, M. M., Du, X. L., Zhang, Y., Yang, Y., et al. (2017). The detection and monitoring of available heavy metal content in soil: a review. Chin J Ecol Agri. 25, 605–615. doi: 10.13930/j.cnki.cjea.160904
Keywords: co-remediation, soil contamination, high-throughput sequencing, bacterial community structure, legume
Citation: Zheng K, Liu Z, Liu C, Liu J and Zhuang J (2023) Enhancing remediation potential of heavy metal contaminated soils through synergistic application of microbial inoculants and legumes. Front. Microbiol. 14:1272591. doi: 10.3389/fmicb.2023.1272591
Edited by:
Saurabh Kumar, ICAR-Research Complex for Eastern Region, IndiaReviewed by:
Chunqiao Xiao, Wuhan Institute of Technology, ChinaArtur Banach, The John Paul II Catholic University of Lublin, Poland
Copyright © 2023 Zheng, Liu, Liu, Liu and Zhuang. This is an open-access article distributed under the terms of the Creative Commons Attribution License (CC BY). The use, distribution or reproduction in other forums is permitted, provided the original author(s) and the copyright owner(s) are credited and that the original publication in this journal is cited, in accordance with accepted academic practice. No use, distribution or reproduction is permitted which does not comply with these terms.
*Correspondence: Jiayao Zhuang, bmx6amlheWFvQG5qZnUuZWR1LmNu