- 1Research Unit, University Hospital Son Espases-Health Research Institute of the Balearic Islands (IdISBa), Palma, Spain
- 2Department of Microbiology, University Hospital Son Espases, Palma, Spain
- 3Centro de Investigación Biomédica en Red, Enfermedades Infecciosas (CIBERINFEC), Madrid, Spain
- 4Infectious Diseases Service, Hospital del Mar, Hospital del Mar Research Institute, Barcelona, Spain
- 5Universitat Pompeu Fabra (UPF), Universitat Autònoma de Barcelóna (UAB), Barcelona, Spain
The interplay between antibiotic resistance and bacterial fitness/virulence has attracted the interest of researchers for decades because of its therapeutic implications, since it is classically assumed that resistance usually entails certain biological costs. Reviews on this topic revise the published data from a general point of view, including studies based on clinical strains or in vitro-evolved mutants in which the resistance phenotype is seen as a final outcome, i.e., a combination of mechanisms. However, a review analyzing the resistance/fitness balance from the basic research perspective, compiling studies in which the different resistance pathways and respective biological costs are individually approached, was missing. Here we cover this gap, specifically focusing on Pseudomonas aeruginosa, a pathogen that stands out because of its extraordinary capacity for resistance development and for which a considerable number of recent and particular data on the interplay with fitness/virulence have been released. The revised information, split into horizontally-acquired vs. mutation-driven resistance, suggests a great complexity and even controversy in the resistance-fitness/virulence balance in the acute infection context, with results ranging from high costs linked to certain pathways to others that are seemingly cost-free or even cases of resistance mechanisms contributing to increased pathogenic capacities. The elusive mechanistic basis for some enigmatic data, knowledge gaps, and possibilities for therapeutic exploitation are discussed. The information gathered suggests that resistance-fitness/virulence interplay may be a source of potential antipseudomonal targets and thus, this review poses the elementary first step for the future development of these strategies harnessing certain resistance-associated biological burdens.
1. Introduction
The fitness (also known as biological success) of a bacterial species is its capacity to thrive within a given context defined by nutrient availability, physicochemical conditions, presence of antibiotics, etc. (Botelho et al., 2019). Evidently, when considering a pathogen microorganism, one feature intimately linked to fitness is virulence, since the greater the ability to infect an individual through virulence factors and strategies to evade immunity, the more chances it will have to survive and multiply (Beceiro et al., 2013). Therefore, although fitness has been classically quantified through growth rate and inter-bacterial competitiveness, these parameters also inseparably impact the pathogenic capacity of the bacterium: in general, the slower it grows inside the host, the less virulent it will be and vice versa, at least in acute infections (Andersson, 2006; Andersson and Hughes, 2010; Melnyk et al., 2015). Conversely, when considering chronic infections such as those typically appearing in the lungs of patients with cystic fibrosis, selection for slow growth and reduced virulence often seems to be the hallmark of successfully adapted strains and hence, the analysis of fitness is not that simple in this case (Høiby et al., 2010; Lorè et al., 2012; Malhotra et al., 2019). Therefore, in the present review we will focus on the interplay between resistance and fitness/virulence in the classic acute infection context, and appealing to a sensu lato conception, we will indistinctly refer to virulence or fitness because of their abovementioned close linkage although we know that, strictly speaking, they are not equivalent terms. Accordingly, the concepts of virulence attenuation and biological cost (or burden) will also be used as synonymous. Moreover, as shown below, not only growth but also other specific virulence-related parameters (motility, production of toxins, pigments and/or proteases, cytotoxicity, biofilm formation, etc.) (Gallant et al., 2005; Deptuła and Gospodarek, 2010; Stickland et al., 2010; Pérez-Gallego et al., 2016; Schroeder et al., 2017) are altered in association with certain resistance mechanisms, which supports our inclusive use of both fitness and virulence concepts.
Although there is a classic idea advocating that acquired antibiotic resistance often entails an associated biological cost, mostly in terms of reduced growth rate and competitiveness in assays mating susceptible vs. resistant strains, this assumption is far from being so straightforward in reality (Beceiro et al., 2013; Melnyk et al., 2015; Vogwill and MacLean, 2015; Melnyk et al., 2017). This notion is based on the fact that typical mutation-driven resistance mechanisms may have important impacts on relevant biological processes, which end up affecting bacterial growth and/or pathogenic behavior (Beceiro et al., 2013): (i) mutations in antibiotic targets that reduce the affinity of the drug but also impair the efficiency of the original protein; (ii) overexpression of β-lactamases entailing high energy costs because of the increased amount of protein produced. Additionally, the β–lactamase enzymatic activity itself and/or the mechanism of hyperproduction, potentially causing alterations in the peptidoglycan structure and/or its metabolism, could hinder cell viability; (iii) efflux pumps hyperexpression, which in parallel to antibiotics may excessively extrude other compounds needed for an effective pathogenic performance, such as quorum-sensing signals; (iv) porins loss, causing a reduced influx of the antibiotic but potentially also of other substances that could contribute to efficient growth. Additionally, certain porins (e.g., OprF) have been described to take part in specific pathogenesis-related events such as host cell adhesion, invasiveness, and biofilm formation; thus, these parameters would be altered if the porin is disrupted (Beceiro et al., 2013; Fernando and Kumar, 2013; Chevalier et al., 2017).
Meanwhile, regarding horizontally-acquired resistance, the fact of producing an external protein such as a β-lactamase in high amounts (or even large groups of other proteins codified in the same carrier mobile genetic element) may entail additional energy burdens and/or collateral effects on metabolism, which could impair growth (Beceiro et al., 2013; Barceló et al., 2022a). Interestingly, it has been proposed that the biological cost associated with horizontally-acquired resistance is overall lower than that of the mutation-driven mechanisms, that usually have greater impacts on bacterial biology through the abovementioned phenomena (Vogwill and MacLean, 2015).
Either way, following the aforementioned simplified conception of the interplay between resistance and fitness, susceptible strains would be expected to outcompete resistant ones if antibiotic selective pressure was eliminated, as has been experimentally demonstrated (Vogwill and MacLean, 2015). However, as will be shown throughout this review there is also a considerable number of examples in which the allegedly typical burden associated with resistance is not found at all, and even some cases of increased virulence linked to certain resistance pathways (Aoki et al., 2004; Gallant et al., 2005; Roux et al., 2015; Guillard et al., 2016; Jorth et al., 2017; Cervoni et al., 2021; Barceló et al., 2022a,b). The basis underlying these phenomena has been explained by different facts, i.e., (i) horizontally-acquired and mutation-driven resistance mechanisms that appear to be cost-free because of their intrinsic nature and features; (ii) genetic linkage or co-selection between resistance and other markers (e.g., presence of virulence factors encoded in the same plasmid harboring the resistance determinant); (iii) the selection of compensatory mutations that specifically reduce the initial burden associated with the particular resistance mechanism, and/or mutations that increase general fitness irrespectively of antibiotic resistance. In this last regard, the biological cost associated with certain resistance phenotypes in Pseudomonas aeruginosa has been shown to be alleviated in hypermutator backgrounds, likely due to an increased capacity to acquire cost-compensatory mutations compared to non-hypermutator strains (Perron et al., 2010; Cabot et al., 2014, 2016). All these circumstances would thus underlie the phenomenon of perpetuation of resistance mechanisms occurring even in the absence of antibiotic pressure (Andersson, 2006; Andersson and Hughes, 2010; Schulz Zur Wiesch et al., 2010; Melnyk et al., 2015). However, it has also been proposed that this outcome may be overestimated in laboratory conditions, in which case, compensatory adaptation would not be as effective in vivo, a circumstance that should be delved into for the development of therapies (MacLean and Vogwill, 2014; McLean et al., 2019; Hernando-Amado et al., 2022). Therefore, understanding the compensation mechanisms of resistance-associated fitness costs is a topic of great interest because of its potential therapeutic implications. In this sense, some papers on the topic using P. aeruginosa rifampicin resistance as a model revealed different typologies of compensatory mutations appearing in response to low vs. high costs associated with the resistant phenotype, as well as the existence of various epistatic interactions finally determining the associated burden depending on genetic background and external physicochemical conditions (Hall and MacLean, 2011; Qi et al., 2016; Vogwill et al., 2016; Gifford et al., 2016a,b; Souque et al., 2023). At any rate, an essential first step to fully understanding compensatory evolution (which by the way seems an insufficiently studied field at least in relevant pathogen species) is to completely dissect the resistance vs. fitness/virulence interplay from a basic and fundamental research perspective, the goal addressed in this review.
Altogether these facts suggest that the interplay between antibiotic resistance and fitness is a topic of extreme complexity in which many elements are involved. Thus, it has to be dissected by taking into account different factors, such as the specific resistance-causing pathway, scenario (in vitro vs. in vivo, laboratory conditions vs. clinical context), type of mechanistic basis (horizontally-acquired vs. mutation-driven), and, obviously, the bacterial species. A multitude of published studies in the field are available for different pathogens (Andersson, 2006; Andersson and Hughes, 2010; Beceiro et al., 2013; Melnyk et al., 2015, 2017; Guillard et al., 2016; Cepas and Soto, 2020), being an important part of the knowledge likely generalizable for a wide range of species. However, because of its outstanding importance as a human opportunistic pathogen, its multidrug-resistant nature, and the urgent need for the development of new therapeutic weapons (Bassetti et al., 2017; Reig et al., 2022), here we focus on the interplay between resistance and virulence in P. aeruginosa exclusively. We include classic works but also particular and/or recent results that have not been found in other species, and that therefore deserve an update. To make the analysis easier, the topic is split into two parts, respectively reviewing the basic research and fundamental knowledge about i) the impact of horizontally-acquired resistance on fitness/virulence; and ii) the burden associated with mutation-driven resistance mechanisms. An overview of the main findings on both subjects in P. aeruginosa is shown in Tables 1, 2, respectively.
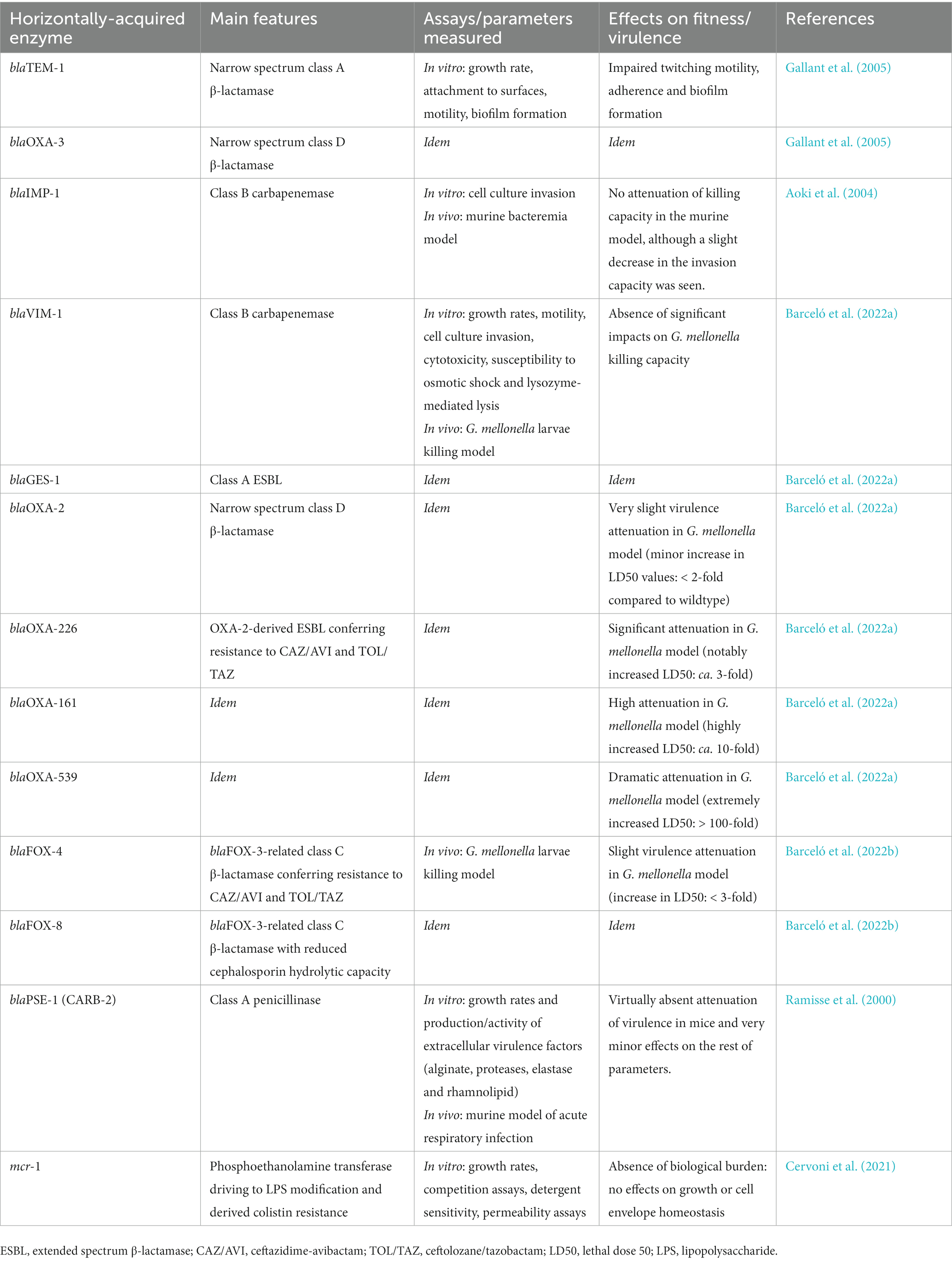
Table 1. Overview of the main basic research concerning the interplay between horizontally-acquired antibiotic resistance and fitness/virulence in P. aeruginosa.
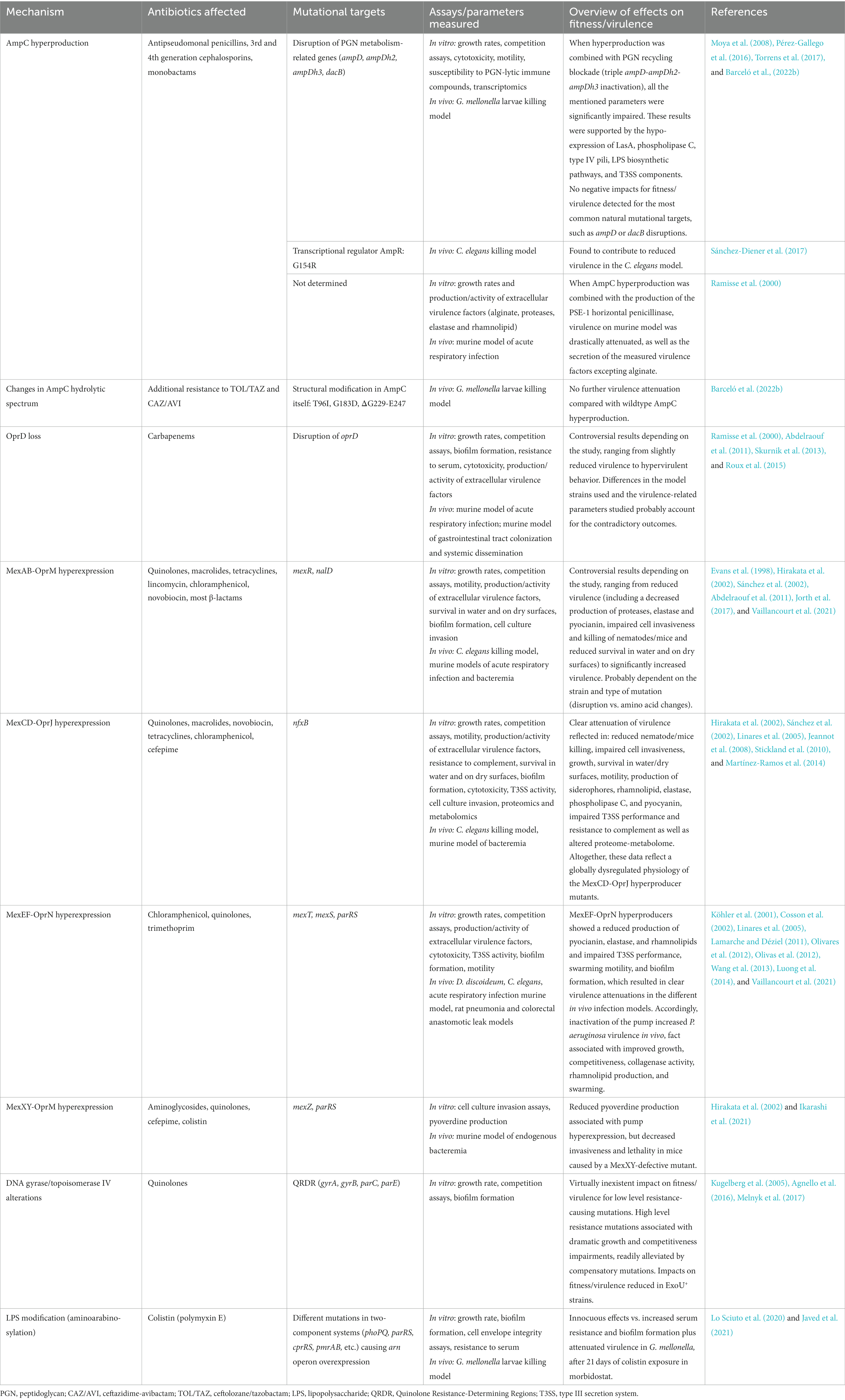
Table 2. Overview of the main basic research published data regarding the interplay between mutation-driven antibiotic resistance and fitness/virulence in P. aeruginosa.
2. Horizontally-acquired resistance
Virtually all the knowledge regarding the biological cost of horizontally-acquired resistance in P. aeruginosa deals with the incorporation of β-lactamases from any of Ambler’s classes, including extended spectrum β-lactamases (ESBLs) and carbapenemases (Yoon and Jeong, 2021). In P. aeruginosa these enzymes are usually encoded in class I integrons, which are the predominant platforms for acquisition of resistance markers and ulterior dissemination through transferable elements such as plasmids. Excellent reviews on the topic can be resourced for more information concerning these resistance-related vehicles and their mechanisms of horizontal transfer (Escudero et al., 2015; Zhao and Hu, 2015). Besides β-lactamases, it is usual that different aminoglycoside-modifying enzymes and even other less frequent determinants (e.g., fluoroquinolones and colistin resistance genes) are carried in integrons/plasmids (Chávez-Jacobo et al., 2018; Khan et al., 2020; Kocsis et al., 2021; Nitz et al., 2021), although little knowledge regarding their biological cost is available. An exception to this is a recent paper in which the burden of the colistin resistance-conferring gene mcr-1, more commonly found in Enterobacteriaceae, was assessed suggesting the worrisome absence of any associated fitness barrier for the spread of this determinant in P. aeruginosa (Cervoni et al., 2021; Table 1).
Returning to the balance between horizontal β-lactamase-dependent resistance and virulence in P. aeruginosa, the volume of published information is not as high as for other species (Marciano et al., 2007; Dubois et al., 2009; Cottell et al., 2012; Fernández A. et al., 2012; Cordeiro et al., 2014; Colquhoun et al., 2021, 2023; Rajer and Sandegren, 2022), but some interesting data are available. For instance, Gallant and colleagues showed that the production of the cloned enzymes TEM-1 and OXA-3 (an OXA-2-related variant) caused significant defects in twitching motility, adherence, and biofilm formation of P. aeruginosa without affecting its growth, which suggests a finely tuned pathogenic behavior modulation rather than a general loss of biological efficacy (Gallant et al., 2005). Meanwhile, the production of the penicillinase PSE-1 had no apparent effects on P. aeruginosa fitness/virulence according to Ramisse et al. (2000) (Table 1).
More recently, the influence of other acquired β-lactamases on P. aeruginosa biological success has been analyzed through different assays including the invertebrate Galleria mellonella infection model, revealing that the production of some widely disseminated enzymes such as the class B carbapenemase VIM-1, the class A ESBL GES-1, or the narrow spectrum OXA-2 caused virtually insignificant decreases in fitness/virulence (Barceló et al., 2022a; Table 1). In another recent study, the burden of producing two less frequent class C transferable enzymes related to FOX-3, namely FOX-4 (variant conferring resistance to ceftolozane/tazobactam and ceftazidime/avibactam) and FOX-8 (a derivative with reduced cephalosporin hydrolysis capacity) was approached, and a very minor impact on P. aeruginosa virulence was also demonstrated for both (Barceló et al., 2022b; Table 1). Returning to VIM-1, GES-1, and OXA-2 (Barceló et al., 2022a), the mentioned results were somehow to be expected, because if the biological burden associated to their expression were high, they would not be so usually detected in clinical samples (Oliver et al., 2015; Del Barrio-Tofiño et al., 2020; Kocsis et al., 2021). In this regard, some studies with different species have shown that plasmids harboring very diverse β-lactamases may carry other elements that balance their associated burden or even increase the pathogenic potential by codifying additional virulence factors (Harrison and Brockhurst, 2012; Carattoli, 2013; Tsang, 2017; Lee et al., 2020). However, this is not the case for the abovementioned VIM-1, GES-1, and OXA-2 in P. aeruginosa, since they were produced from multicopy vectors devoid of any extra virulence factor, demonstrating a very low burden of these enzymes by themselves (Barceló et al., 2022a). A similar result had previously been obtained with another cloned class B carbapenemase (IMP-1) that did not significantly alter P. aeruginosa virulence, supporting the wide dissemination of this metallo-β-lactamase in this and other species (Aoki et al., 2004, Pongchaikul and Mongkolsuk, 2022; Table 1). Interestingly, in the aforementioned work (Barceló et al., 2022a) it was shown that, in contrast to OXA-2, the production of its extended spectrum derivatives OXA-161, OXA-226, and especially OXA-539 entailed dramatic virulence attenuations. These were reflected in significant decreases in the G. mellonella larvae killing capacity, with lethal dose 50 values of the strains expressing the OXA-2 derivatives ca. several hundred-fold higher than those of the wild-type strain in some cases. Nevertheless, these phenotypes were seemingly not relying on the alteration of other fitness/virulence-related parameters such as growth rate, cytotoxicity, motility, or susceptibility to lysis (Barceló et al., 2022a; Table 1). In the light of these data, hypothesizing that the high biological cost may pose a barrier for a more usual selection/dissemination of certain enzymatic variants with increased hydrolytic capacity (in contrast with the originative enzymes), seems reasonable and likely applicable to at least some specific β-lactamase derivatives.
Although the exact mechanistic bases for the results exposed in these two previous paragraphs remain unclear, some explanations have been proposed. For instance, Gallant et al. hypothesized that β-lactamases from the A and D classes (TEM-1 and OXA-3), because of their relatedness to low molecular weight Penicillin Binding Proteins (PBPs), could be sequestering the peptidoglycan substrates of such enzymes in the periplasm. This fact would in turn alter the cell wall metabolism/architecture and, consequently, impair the abovementioned virulence-related parameters (Gallant et al., 2005). Interestingly, an intact enzymatic activity was shown to be necessary to cause the fitness cost associated with TEM-1 but not with OXA-3, suggesting a different mechanistic basis linked to each β-lactamase (Gallant et al., 2005). Closely related to this, the important virulence attenuation associated with the aforementioned ESBLs, OXA-161, OXA-226, and OXA-539 but not with their parental enzyme OXA-2, suggests certain involvement of the enzymes’ active sites, because if mutations change the hydrolytic spectrum of β-lactamases (from narrow to extended), why should they not be altering the effect on other substrates? Moreover, in this same work, it was shown that the higher the ceftazidime hydrolytic capacity of each variant (OXA539 > OXA-161 > OXA-226), the greater the virulence attenuation associated, reinforcing the idea of a starring role for the active site of the β–lactamases. In this sense, it was proposed that, given the common evolutionary origin of certain β-lactamases with endo/carboxy-peptidase PBPs, a residual peptidoglycan-degrading power could exist for the former, fact in which an alteration of cell wall metabolism could rely on to finally impair fitness/virulence (Chang et al., 1990; Bishop and Weiner, 1992; Damblon et al., 1995; Rhazi et al., 1999; Urbach et al., 2008; Fernández A. et al., 2012; Pratt, 2016; Barceló et al., 2022a,b). Obviously, this would be the case for the mentioned OXA-2-derived ESBLs but not for the originative enzyme, whose active site would remain unable to significantly cleave peptidoglycan. Whether the alleged residual activity of the mentioned ESBLs could affect the murein sacculus itself weakening its structure (Pérez-Gallego et al., 2016; Torrens et al., 2017; Barceló et al., 2022a,b) and/or potential peptidoglycan soluble fragments (muropeptides)-based signaling networks ultimately regulating pathogenic behavior (Folkesson et al., 2005; Escobar-Salom et al., 2023), remains to be elucidated.
Beyond these studies with specific enzymes, it is imperative to consider the typical event of association of P. aeruginosa high-risk clones (e.g., ST235, ST111, and ST175, among others) with different acquired β-lactamases, posing successful combinations of clonal lineages plus resistance determinants of epidemic dissemination. This fact clearly suggests that—despite having not been assessed in terms of their specific biological burdens—enzymes such as OXA-10, PER-1, NDM-1, KPC-2, VIM-2, and certain GES-type ESBLs/carbapenemases among many others, probably do not involve high costs for P. aeruginosa because if this were the case, their appearance would not be that frequent (Oliver et al., 2015; Del Barrio-Tofiño et al., 2020; Kocsis et al., 2021). On the other hand, it is not possible to fully rule out the well-known phenomenon of certain plasmids encoding features that alleviate the biological cost of β-lactamases contributing to this outcome (Harrison and Brockhurst, 2012; Carattoli, 2013; Tsang, 2017; Kottara et al., 2018; Pongchaikul and Mongkolsuk, 2022), although, to our knowledge, this fact has not been specifically demonstrated in P. aeruginosa. This contrasts with other species such as K. pneumoniae, in which the phenomenon seems to be frequent (Gomez-Simmonds and Uhlemann, 2017; Jia et al., 2022). Finally, although a very specific case of a natural plasmid harboring two widely detected β-lactamases (OXA-10 and VIM-2) entailing high biological costs when conjugated to P. aeruginosa has been recently described (Li et al., 2023), this does not seem to be a common occurrence at all and could probably be related to intrinsic features of the starring plasmid yet to be determined. Considering all these facts, the idea of a relatively low cost associated with most horizontal β-lactamases seems more than reasonable. The influence of the species in this outcome is obviously also important, being P. aeruginosa a very good carrier of enzymes in contrast to other pathogens for which the acquisition of specific horizontal β-lactamases seems to entail a significant cost, such as the particular case of Salmonella enterica serovar Typhimurium producing an Enterobacter cloacae-derived AmpC or a VIM-2 carbapenemase (Morosini et al., 2000; Cordeiro et al., 2014).
Returning to the molecular mechanisms that mediate the biological cost associated with certain horizontal β–lactamases, and taking into account that they may not be necessarily common to all enzymes, it would be very interesting to fully decipher them in order to better understand the interplay between their dependent resistance and fitness/virulence in P. aeruginosa. The knowledge gaps to be filled in this context are mostly related to the intrinsic burden of specific β-lactamases, being of great interest to confirm that globally disseminated enzymes are virtually cost-free unlike specific amino acid variants, that could be associated with great costs, fact determining their limited appearance. In this regard, particularly interesting would be the analysis of fitness costs associated with horizontal β-lactamase variants (mainly OXA-2, OXA-10, and KPC-2/−3 derivatives) that are progressively arising as novel resistance determinants pushed by the selective pressure exerted by recently introduced antipseudomonal drugs such as ceftolozane/tazobactam, ceftazidime/avibactam, and imipenem/relebactam, which have never been studied from the fitness/virulence impact perspective (Fraile-Ribot et al., 2018; Arca-Suárez et al., 2019, 2021; Faccone et al., 2022; Lasarte-Monterrubio et al., 2022; Tu et al., 2022; Yang et al., 2023). Finally, it would also be desirable to ascertain whether or not certain plasmid-encoded enzymes are linked to genes responsible for burden-alleviating mechanisms, which could therefore become interesting therapeutic objectives. Another topic not addressed to date in P. aeruginosa is to decipher whether the presence of the acquired β-lactamase gene in the original plasmid vs. its integration in the chromosome (an outcome happening in a considerable number of cases), could entail differential biological costs. All this knowledge yet to be developed in future could help unveil targets aimed at attenuating P. aeruginosa virulence and/or hinder the dissemination of certain β-lactamases, thereby aiding to curb the threat posed by this fearsome species.
3. Mutation-driven resistance
As will be seen in this section, information concerning the relatedness between mutation-driven mechanisms and fitness/virulence in P. aeruginosa (Table 2) is greater than for horizontally-acquired resistance. As mentioned above, although a general idea of decreased fitness/virulence associated with resistance can be obtained mostly from studies with collections of clinical strains (Mulet et al., 2013; Sun et al., 2013; Gómez-Zorrilla et al., 2016; Kaiser et al., 2017), when a deeper approach is performed one can see that several exceptions exist and many clarifications need to be done. Moreover, in this type of studies the resistance phenotype is treated as a whole, i.e., the result of a sum of features, and thus each particular pathway or even a set of different mechanisms for a given antibiotic are not addressed separately. In fact, it is usual, in these investigations, for considerable numbers of clinical strains to display high resistance levels [e.g., Multidrug-Resistant (MDR), Extensively Drug-Resistant (XDR) (Magiorakos et al., 2012)] derived from combining mutation-driven and horizontally-acquired mechanisms, making it very difficult to establish associations between specific resistances and consequences for fitness/virulence. Another confounding factor potentially influencing resistance-virulence balance is the clonal lineage of the strains since, evidently, depending on the initial pathogenic level of each (i.e., strong associations between certain sequence types and the presence of potent virulence factors such as the ExoU toxin), the resistance-associated burden could vary greatly (Hendrickson et al., 2001; Sánchez-Diener et al., 2017; Del Barrio-Tofiño et al., 2020; Recio et al., 2020).
There are other facts revealing the complexity of the topic: for instance, although in some studies several typical virulence-related parameters appear to be impaired in association with high-level resistance (pigment production, motility, lethality in animal models, susceptibility to serum, etc.), some other specific features such as biofilm formation seem to be improved (Mulet et al., 2013; Kaiser et al., 2017). Even some examples of controversial results exist, i.e., data showing XDR strains with increased in vitro competitiveness compared to more susceptible ones, demonstrating again the intricate nature of mutational resistance-fitness balance (Kaiser et al., 2017). In any case, since dissecting the knowledge related to wide collections of clinical strains is not a primary objective here, and there are already some reviews addressing the topic (Sawa et al., 2014; Oliver et al., 2015; Juan et al., 2017a), we will focus on basic research only. Moreover, despite fitting with the basic research concept, other studies with in vitro-evolved mutants also analyze the final resistance phenotype as a whole and in many cases, several mutations need to be accumulated in order to enable certain resistances (Cabot et al., 2016, 2018; Gomis-Font et al., 2020, 2022; Barceló et al., 2021), making it difficult to extract cause-effect relationships between specific targets and impacts on fitness and virulence. For these reasons, we will mostly approach here the cases of unequivocal interconnection between a specific resistance mechanism and a final impact on fitness/virulence in P. aeruginosa, always from a fundamental perspective (Table 2).
3.1. AmpC cephalosporinase
This class C β-lactamase is an inducible enzyme under the control of a LysR family transcriptional regulator, AmpR, which depending on which muropeptides coming from the degradation of the peptidoglycan it is bound to, acquires different conformations. Depending on the conformation, AmpR can exert a repressor (in regular conditions) or a transient promotor role of ampC expression (during the challenge with an inducer β-lactam) which enables P. aeruginosa natural resistance to aminopenicillins and 1st and 2nd generation cephalosporins (Juan et al., 2017b; Torrens et al., 2019a). Besides this regular inducible nature, AmpC stable hyperproduction probably poses the most relevant resistance mechanism in this species, affecting monobactams, antipseudomonal penicillins, and 3rd/4th generation cephalosporins, and can be achieved through the selection of mutations in different targets. For instance, mutational disruptions of the low mass PBP4 (dacB gene), the cytosolic amidase AmpD or the cytosolic muropeptide ligase Mpl, are highly prevalent in clinical strains (Del Barrio-Tofiño et al., 2017; López-Causapé et al., 2018; Torrens et al., 2022). These mutations end up altering the peptidoglycan metabolism and the derived pool of muropeptides binding to AmpR, constitutively enabling its activator role. In addition, certain specific amino acid changes in AmpR itself (e.g., D135N or G154R) have been shown to cause its constitutive activator conformation as well (Cabot et al., 2012; Caille et al., 2014; Juan et al., 2017b; Torrens et al., 2019a,b).
As expected, the repressed production of AmpC does not entail any biological cost for P. aeruginosa (Pérez-Gallego et al., 2016; Barceló et al., 2022b) and what is more, its deletion caused a decreased fitness in a murine model of infection (Roux et al., 2015). The mechanistic basis for this intriguing apparent contribution of AmpC to the basal virulence of P. aeruginosa remains to be investigated. Moreover, when Cabot and colleagues analyzed the capacity of P. aeruginosa to develop β-lactam resistance in mutants devoid of their capacity for AmpC hyperproduction (through the disruption of ampC or of ampG, encoding the permease that enables the entrance of the AmpR-activating muropeptides into the cytosol), although some complex resistance pathways were selected, their associated biological costs were dramatic (Cabot et al., 2018). These results indicate that AmpC hyperproduction is a very efficient and virtually cost-free resistance mechanism in this species which makes the selection of these alternative pathways highly unlikely (Cabot et al., 2018). In fact, most pathways driving to AmpC hyperproduction (at least dacB and ampD disruptions) have been shown to display no impacts on virulence (Moya et al., 2008; Pérez-Gallego et al., 2016; Torrens et al., 2019b; Barceló et al., 2022b), which is also supported by the high frequency of occurrence of these mechanisms in nature (Del Barrio-Tofiño et al., 2017). However, a previous work in which AmpC hyperproduction was combined with the presence of the acquired PSE-1 β-lactamase showed a dramatic reduction of virulence factors production and mortality in mice, being proposed a derived defect of intercellular signaling as possible explanation for the results (Ramisse et al., 2000; Table 2).
On the other hand, some amino acid changes in the AmpC sequence itself (e.g., T96I, G183D, or ΔG229-E247) combined with hyperproduction have been shown to cause resistance to ceftazidime/avibactam and ceftolozane/tazobactam, but in exchange for this increased spectrum of conferred resistance, there were virtually non-existent added burdens (Barceló et al., 2022b; Table 2). This circumstance poses a worrisome warning, since apparently there is no biological cost-related barrier to avoid the spread of this kind of mutations (Barceló et al., 2022b). Nevertheless, it had been previously shown that resistance to ceftolozane/tazobactam developed in vitro in non-hypermutator strains, was due to other combined mutations that determined global pleiotropic effects associated with a severe fitness cost (Cabot et al., 2014). Conversely, in the hypermutator background, P. aeruginosa resistance to ceftolozane/tazobactam was associated with the mentioned confluence of circumstances (hyperproduction and structural modification of AmpC), together with many other mutations determining a much lower biological cost compared to the non-mutator background, probably reflecting the increased likelihood of acquiring compensatory mutations (Cabot et al., 2014). This circumstance was later generally seen for other mutation-driven resistance mechanisms (ceftazidime, ciprofloxacin, and meropenem), although with an extraordinary variability in fitness costs depending on the mutants analyzed (Cabot et al., 2016). At any rate, although the mentioned AmpC structural variations (T96I, G183D, or ΔG229-E247) conferring resistance to ceftazidime/avibactam and ceftolozane/tazobactam have been characterized from the fitness cost perspective, many other derivatives involved in resistance to these and other new antipseudomonal drugs remain to be studied in this regard (Arca-Suárez et al., 2020; Fraile-Ribot et al., 2020; Gomis-Font et al., 2022; Ruedas-López et al., 2022; Gomis-Font et al., 2023).
Still related to the AmpC-associated biological cost in P. aeruginosa, but in an even more basic research context, certain particular combinations of features such as the triple disruption of AmpD-AmpDh2-AmpDh3 homologs (that entail AmpC de-repression as well as great peptidoglycan recycling alteration), or the over-expression of AmpC from a multicopy plasmid in a peptidoglycan recycling-defective strain (AmpG KO mutant) have been shown to entail drastic attenuations in terms of G. mellonella killing (Pérez-Gallego et al., 2016; Torrens et al., 2017). In these specific conditions, several parameters linked to this outcome were also affected, such as growth rate, motility, cytotoxicity, resistance to peptidoglycan-targeting immune proteins, and inter-bacterial competitiveness. These circumstances were supported by transcriptomics, which revealed a reduced expression of protease LasA, phospholipase C, type IV pili (involved in motility), lipopolysaccharide (LPS) biosynthetic pathways, or type III secretion system (T3SS) components (Pérez-Gallego et al., 2016, Torrens et al., 2017; Table 2). Although the mentioned combinations of factors do not occur in nature, it is interesting to understand the underlying mechanisms mediating the virulence attenuation, as this could provide information to unveil therapeutic targets. In this regard, it has been recently demonstrated that in order to obtain these attenuations, an intact enzymatic activity of AmpC is needed (Barceló et al., 2022b) as had been demonstrated for TEM-1 β-lactamase (Gallant et al., 2005), reinforcing the idea of a residual peptidoglycanase activity in P. aeruginosa AmpC that could be debilitating the cell wall. In fact, a reduction in the amount of peptidoglycan per cell, concordant with a potential partially degraded sacculus, actually occurred in the mentioned conditions, which could be responsible for an increased susceptibility to lysis mediated by osmotic changes and/or immune weapons and thus entailing reduced fitness/virulence (Pérez-Gallego et al., 2016, Torrens et al., 2017). Therefore, this explanation is in accordance with what is mentioned above for OXA-2 derivatives, i.e., AmpC potentially displaying a significant residual capacity to degrade peptidoglycan (Chang et al., 1990; Bishop and Weiner, 1992; Damblon et al., 1995; Rhazi et al., 1999; Urbach et al., 2008; Fernández A. et al., 2012; Pratt, 2016; Barceló et al., 2022a,b), either the sacculus itself or signal muropeptides participating in potential virulence-regulating networks proposed to exist in P. aeruginosa and other species (Folkesson et al., 2005; Escobar-Salom et al., 2023).
In this last regard, it is worth highlighting the role of the AmpC transcriptional regulator, AmpR, which apparently has an important repertoire of virulence-related genes under its control (Kong et al., 2005; Balasubramanian et al., 2011, 2012). This fact is in accordance with the proposed existence of networks of muropeptides acting as signals that, once bound to the appropriate transcriptional regulator (AmpR or others), could enable its conformational changes ultimately affecting the expression of certain genes and thus conditioning pathogenic behavior (Folkesson et al., 2005, Escobar-Salom et al., 2023). Therefore, if the pool of muropeptides is altered through a β-lactamase retaining certain peptidase activity, combined or not with another particular event such as the peptidoglycan recycling blockade, it is reasonable to think that the pathogenic response once these fragments bind to the regulator is also modulated (Folkesson et al., 2005; Barceló et al., 2022a,b; Escobar-Salom et al., 2023). Accordingly, it has been shown that certain specific amino acid changes in AmpR (e.g., G154R), responsible for a constitutive AmpC-activating conformation, also contribute to a decreased virulence of the bacterium, which could be related to a repressor role of this particular AmpR variant over certain pathogenesis-related genes (Table 2). However, this reduction does not seem to pose a significant barrier for the dissemination of the ST175 high-risk clone, in which this AmpR mutation is readily detected (Oliver et al., 2015; Sánchez-Diener et al., 2017).
3.2. OprD porin
OprD porin-inactivating mutations, a well-known carbapenem resistance pathway, have been found to strongly increase bacterial fitness/virulence in two studies (Skurnik et al., 2013; Roux et al., 2015), probably through different transcriptional and post-transcriptional changes motivated by the absence of this porin. However, in previous works quite the opposite result had been shown, i.e., OprD deletion was associated with a slight decrease in virulence in murine models (Ramisse et al., 2000, Abdelraouf et al., 2011; Table 2). Differences in the model strains used and the various fitness/virulence-related parameters analyzed in each work might well account for these discordant outcomes.
3.3. Efflux pumps
Besides AmpC, efflux pumps are probably the most well-known resistance mechanism of P. aeruginosa, with those within the Resistance-Nodulation-Division (RND) family as the most clinically relevant. These pumps are structured in three compounds: an inner membrane transporter, a periplasmic adaptor, and an outer membrane channel, usually encoded as operons. The existence of a connection between certain RND pumps and virulence is a well-known fact in Gram-negative bacteria (Fernando and Kumar, 2013; Rampioni et al., 2017), entailing an obvious interplay with resistance. Regardless, although up to 12 different RND efflux systems have been found in the PAO1 reference strain, the most relevant variants by far in terms of resistance conferred are MexAB-OprM, MexXY-OprM, MexCD-OprJ, and MexEF-OprN (Lorusso et al., 2022). Altogether, they affect virtually all antibiotic families and, similar to AmpC, often display a basal level of expression partially accounting for the intrinsic level of resistance to several drugs, which can be amplified through the selection of mutations that trigger their overexpression. The array of targets/mutations that determine different levels of hyperexpression of these pumps and consequent resistance phenotypes is wide and complex, but we will only approach the specific mechanisms that have been studied from the fitness/virulence point of view. As will be seen here, the topic of relatedness between efflux pumps and virulence, which is intimately linked to quorum sensing, is probably the one with the most published data within the field of resistance-fitness interplay in P. aeruginosa, revealing a great complexity that deserves delving into (Table 2).
The MexAB-OprM pump hyperexpression (classically known as nalB phenotype) has been related to mutations in different targets such as its repressors mexR, nalC, and nalD, and contributes to resistance to most antipseudomonal antibiotics, especially quinolones and β-lactams (Lorusso et al., 2022). More than two decades ago some studies started to characterize the potential costs associated with the hyperexpression of this pump, suggesting a significant burden for the strains showing this phenotype, with reduced production of pyocyanin, protease and elastase, a slightly impaired capacity to invade cell cultures as well as a significantly attenuated virulence in the nematode Caenorhabditis elegans and murine systemic infection models (Evans et al., 1998; Hirakata et al., 2002; Sánchez et al., 2002). The excessive extrusion, driven by the hyperexpressed pump, of some quorum-sensing autoinducers ultimately regulating the expression of virulence genes, was suggested as a possible explanation for these results (Evans et al., 1998; Sánchez et al., 2002). More recent works have also demonstrated a slight cost associated with MexAB-OprM hyperproduction (specifically mediated by mexR deletion) in mice, which was very significantly increased when combined with OprD inactivation (Abdelraouf et al., 2011). Moreover, hyperexpression of this efflux pump seems essential to provide resistance to the recently introduced cefepime/zidebactam combination in addition to other mutations affecting PBPs, altogether determining very high biological costs for P. aeruginosa (Barceló et al., 2021).
Conversely, other investigations showed that MexAB-OprM hyperproduction (achieved through mutations in mexR or nalD) was associated with increased virulence in murine models in a strain-dependent fashion: whereas PAO1 mutants displayed a hypervirulent behavior, the pump hyperproduction had no effect on PA14 virulence (Jorth et al., 2017; Vaillancourt et al., 2021). These data, added to those in other studies showing that the deletion/inhibition of mexAB and/or oprM resulted in dramatically reduced cell invasiveness and drastically impaired fitness/virulence in murine colonization/infection models (Hirakata et al., 2002, 2009; Aoki et al., 2004; Roux et al., 2015), suggest the existence of a very intricate connection between MexAB-OprM-dependent resistance and virulence. Some explanations for these certainly complex results can be provided, such as the suggested contribution of this pump to toxin and/or quorum-sensing signal secretion (Evans et al., 1998; Hirakata et al., 2002; Sánchez et al., 2002; Jorth et al., 2017), the unequal effects of the different types of mutations in mexR studied [protein disruption (Jorth et al., 2017) vs. specific amino acid changes (Sánchez et al., 2002)], or the intrinsic features of each P. aeruginosa strain used. Either way, all these facts warrant the need for more research to fully understand the influence of MexAB-OprM on the modulation of P. aeruginosa fitness/virulence.
The MexCD-OprJ efflux pump is controlled by the repressor NfxB and its regular expression has an apparent low impact on the basal phenotype of P. aeruginosa resistance (Lorusso et al., 2022). Several types of mutations in nfxB have been described as a cause of MexCD-OprJ increased expression, entailing clinically relevant levels of resistance mainly to quinolones and cefepime (Jeannot et al., 2008; Lorusso et al., 2022). Dealing with virulence interplays, deletion of this pump has no effects on P. aeruginosa lethality in a murine model (Hirakata et al., 2002), and in contrast with MexAB-OprM in which intricate results certainly do exist, all studies point in the same direction: overexpression of MexCD-OprJ is associated with significant impairments in fitness/virulence. These attenuations have been shown to be reflected in different features of the bacterium such as growth rate, motility, production of extracellular factors (rhamnolipid, elastase, phospholipase C, and pyocyanin), T3SS activation levels, and resistance to complement system (Linares et al., 2005; Jeannot et al., 2008; Stickland et al., 2010; Martínez-Ramos et al., 2014). The underlying basis for these results seems not to be linked to the effects of the NfxB regulator mutation per se, but rather to the inappropriate overexpression of a functional MexCD-OprJ efflux pump triggering pleiotropic changes that globally dysregulate the bacterial physiology (Stickland et al., 2010). Moreover, it has been also shown that, similarly to what has been explained for MexAB-OprM, hyperexpression of MexCD-OprJ caused an excessive extrusion of certain quorum-sensing-related signals (entailing a consequently insufficient intracellular concentration), finally impacting the expression of certain virulence factors controlled by this system (Alcalde-Rico et al., 2018). What is intriguing regarding all these facts is how the hyperproduction of this pump can be selected in clinical strains with a relatively high frequency (Lorusso et al., 2022) if the associated costs are apparently that high? A frequent appearance of compensatory mutations is likely the most logical explanation, but this possibility has not been experimentally ascertained yet.
Basal expression of the MexEF-OprN system does not contribute to the intrinsic resistance of P. aeruginosa, but when hyperproduced it significantly increases quinolone resistance levels. Regulation of MexEF-OprN is more complex than the rest of pumps, with it being influenced by different elements such as MexT (a LysR-like transcriptional activator), the oxidoreductase MexS, and the ParRS two component system, among others (Wang et al., 2013; Lorusso et al., 2022). Hyperexpression of MexEF-OprN is often associated with a concomitant decreased expression of the porin OprD (and derived carbapenem resistance), which is based on the fact that MexT, besides regulating MexEF-OprN expression, also acts as an oprD repressor. Thus, amino acid changes driving to increased activity of MexT regarding both roles would explain this phenomenon (Lorusso et al., 2022). Since, as mentioned above, OprD loss seems to be related to increased fitness and virulence (Skurnik et al., 2013; Roux et al., 2015), it would be expectable that decreased expression of this porin appearing in parallel to MexEF-OprN hyperexpression would have similar effects, which could be partially alleviating the attenuation associated with the pump. However, this possibility has not been experimentally demonstrated yet.
Returning to MexEF-OprN itself, it was proposed more than two decades ago that hyperproduction of this pump involved an excessive extrusion of certain quorum-sensing signals that would finally cause a reduced production of pyocyanin, elastase, and rhamnolipids (essential for correct swarming motility), all under the control of the las and rhl systems of P. aeruginosa (Köhler et al., 2001). Decreased activity of T3SS was also seen to be associated with MexEF-OprN overexpression (Linares et al., 2005), which on the other hand was shown to cause several changes in the bacterial transcriptome, principally in terms of reduced expression of quorum-sensing regulated genes. These changes, which entailed significant virulence attenuations in different infection models (Dictyostelium discoideum, C. elegans, rodents), were found to be pump-dependent as opposed to MexT-dependent (Cosson et al., 2002; Lamarche and Déziel, 2011; Olivares et al., 2012). However, MexT was also reported to display a direct capacity of control over other virulence-related features such as the expression of components of T3SS in a MexEF-OprN-independent fashion, reinforcing the complexity of the topic (Tian et al., 2009). It was later shown that the biological burden associated with MexEF-OprN, and in fact with the hyperproduction of the rest of the pumps, is partially alleviated through a metabolic re-accommodation based on an increased expression of the anaerobic nitrate respiratory chain, a response envisaged as an interesting therapeutic target (Olivares et al., 2014; Olivares Pacheco et al., 2017). Finally, in accordance with the burden associated with hyperproduction, the contrary situation (i.e., deletion of the pump or, what is virtually the same, inactivation of MexT) has been shown to increase P. aeruginosa virulence in vivo, a feature at least partially based on a documented increase in collagenase activity, rhamnolipid production, and swarming motility (Olivas et al., 2012; Wang et al., 2013; Luong et al., 2014; Vaillancourt et al., 2021). However, the underlying mechanisms explaining these results remain to be investigated.
Finally, although MexXY-OprM is the typical pump, in some strains the MexXY operon contains a coding sequence for another outer membrane protein, OprA, which provides the MexXY-OprA variant. Regardless, this antimicrobial-inducible pump displays quite a wide array of affected antibiotics, among which aminoglycosides are probably the most efficiently extruded. Different mutations driving to the hyperproduction of this pump have been described, but few studies have addressed the associated biological costs (Lorusso et al., 2022). In this regard, it was shown two decades ago that a P. aeruginosa mutant defective in MexXY displayed partially decreased invasiveness and lethality in a murine model (Hirakata et al., 2002). Conversely, impaired pyoverdine production associated with MexXY-OprM hyperproduction has recently been reported (Ikarashi et al., 2021), suggesting that only an appropriate level of expression of this pump enables a regular pathogenic behavior. These facts once more evidence the complex interplay between efflux pumps and the modulation of virulence in P. aeruginosa.
Finally, although their impact on P. aeruginosa antibiotic resistance seems marginal, other efflux pumps have been studied from the fitness/virulence point of view. In this regard, the alteration of certain components of the MexGHI-OpmD pump resulted in a marked reduction in the production of specific quorum-sensing signals and caused the attenuation of virulence in rat and plant models (Aendekerk et al., 2005). Thus, this pump seems to be intimately related to quorum-sensing (Karatuna and Yagci, 2010), a general feature for all the aforementioned ones (perhaps with the only exception of MexXY), but it has also been linked to the delivery of a phenazine required for correct biofilm formation which would have obvious virulence-related implications (Sakhtah et al., 2016). On the other hand, inactivation of the MuxABC-OpmB system, which causes an increased level of carbenicillin resistance, also entailed significant impairments in motility and virulence in animal/plant models. However, the underlying mechanisms and biological relevance of this phenomenon have not been delved into (Yang et al., 2011).
3.4. DNA gyrase/topoisomerase IV
These two enzymes (each comprising two subunits: GyrA and GyrB, and ParC and ParE, respectively) are involved in the DNA replication process by modulating the level of its supercoiling through their nuclease/ligase activities. These enzymes are the target of quinolones, which form complexes with them, thus disabling their regular activity, thereby blocking DNA replication and finally promoting its fragmentation and derived cell death (Malik et al., 2006; Bruchmann et al., 2013). These type 2 topoisomerases pose a paradigm of mutation-driven resistance caused by alteration of the target and derived loss of affinity for the drug. Many amino acid changes in their sequences have been demonstrated to cause different levels of quinolone resistance in P. aeruginosa and other species, which justifies the denomination of certain positions within their sequences as Quinolone Resistance-Determining Regions (QRDR). Interestingly, not all the mutations in the QRDR have the same effect on the derived resistance level, and as could be expected, there is an accumulative effect in terms of the phenotype conferred when sequential changes in QRDR are selected over time (Bruchmann et al., 2013). In this regard, mutations in gyrA and parC are probably those most usually found in P. aeruginosa clinical strains (nearly universal in high-risk clones), and are usually combined with a concomitant hyperexpression of efflux pumps (Bruchmann et al., 2013; Del Barrio-Tofiño et al., 2017; Melnyk et al., 2017). In terms of biological costs associated with QRDR mutations in P. aeruginosa, there is a surprisingly low number of publications in the field of basic research (Table 2), with that of Kugelberg and colleagues as the pioneer work (Kugelberg et al., 2005). In this study, low level resistance was shown to be readily obtained in vitro through different single mutations in gyrA or gyrB, which in the vast majority of cases were cost-free. However, when selecting mutants in intermediate concentrations of norfloxacin (8 mg/L), some of them were associated with very important growth impairments. This was a general feature for all the selected high level resistant mutants, which always displayed two mutations: either in two topoisomerase genes or in one topoisomerase gene plus nfxB. The biological cost of these mutations was related to a partial loss of function of the mutated protein(s): a decreased supercoiling of DNA was appreciated in the strains with reduced fitness, which would in turn dampen DNA transcription/replication thereby reducing growth rates. When the appearance of compensatory mutations was studied, it was seen that, due to them, the supercoiling level was recovered in parallel to the regular growth rate (although a slight decrease in quinolone resistance level was also reported) (Kugelberg et al., 2005). In any case, what can be deduced from this study and from the epidemiological panorama of quinolone-resistant P. aeruginosa clinical strains nowadays (Oliver et al., 2015; Kocsis et al., 2021) is that, although some mutations may entail an important fitness cost, selection of compensatory mutations seems a frequent outcome that suggests that this type of resistance is not an unbearable handicap. In this regard, in a more recent work (Melnyk et al., 2017) it was shown that the appearance of cost-free quinolone-resistant mutants was much more likely under a discontinuous regime of ciprofloxacin compared to a constant one, and that the main driver for this output was the appearance of second-site compensatory mutations rather than the initial selection of cost-free alleles. However, in this study, virtually all the mutants obtained displayed combined mutations in nfxB plus gyrA or gyrB, making it difficult to draw mechanism-specific conclusions (Melnyk et al., 2017).
Finally, regardless of compensatory mutations, a fact that likely contributes to the success of quinolone-resistance in P. aeruginosa is the well-known association between the prevalence of mutations in QRDR and the presence of the T3SS-exported ExoU toxin (highly cytotoxic and whose codification is usually exclusive for the less virulence-conferring ExoS) (Wong-Beringer et al., 2008; Agnello and Wong-Beringer, 2012; Cho et al., 2014). This circumstance could be interpreted as an additional strategy to minimize the burden associated with quinolone resistance; that is to say, if the initial level of virulence of a strain is very high (like that of ExoU-positive strains), the burden associated with resistance mechanisms would have less impact than if the original virulence was lower. However, some recent data suggest that the presence of ExoU per se also determines direct changes in P. aeruginosa biology enabling a better regulation of DNA supercoiling, which alleviates the burden linked to topoisomerases mutations (Agnello et al., 2016). Therefore, from this last study one could deduce that not only can resistance mechanisms have a direct impact on fitness/virulence, but also the opposite: the presence of certain virulence factors, such as ExoU, could influence the dynamics of mutation-driven resistance acquisition by finely tuning the associated costs. This is a groundbreaking idea that should be delved into, since other unknown phenomena of virulence factors ultimately determining the fate of different mutational resistance pathways acquisition may exist, which could pose interesting interconnections to be exploited from the therapeutic point of view.
3.5. LPS modification
LPS modifications consisting of the addition of positively charged molecules are the major polymyxin resistance mechanism in P. aeruginosa, and can be achieved through different pathways driving to a reduction in the affinity of the drug. The aminoarabinosylation of LPS lipid A has been described as the primary colistin (polymyxin E) resistance mechanism in P. aeruginosa, and is usually associated with mutations in different two-component systems (e.g., PhoPQ, ParRS, CprRS, or PmrAB) leading to the overexpression of the arnBCADTEF operon, which encodes the enzymes that perform the addition of 4-amino-4-deoxy-l-arabinose to lipid A (Jeannot et al., 2017). Regarding the biological costs of this mechanism, it has been shown that the overexpression of this operon and the derived aminoarabinosylation have virtually innocuous effects for the fitness and virulence of P. aeruginosa (Lo Sciuto et al., 2020). In addition, in another work analyzing the collateral effects of resistant mutants obtained in a morbidostat device, although an increased serum resistance and biofilm formation capacity appeared in parallel to colistin resistance, virulence attenuation in G. mellonella model was also seen after 21 days of exposure to the drug, once more evidencing the intricate nature of resistance vs. fitness/virulence interplay (Dößelmann et al., 2017; Javed et al., 2021). Finally, another less known potential colistin resistance mechanism, namely the addition of phosphoethanolamine to lipid A through the chromosomal enzyme EptA (Nowicki et al., 2015; Freire et al., 2021), has also been approached regarding its potentially associated burden. In this sense, EptA hyperexpression has been shown to cause growth, competitiveness, and cell envelope defects that were not linked to phosphoethanolamine transferase activity per se (Cervoni et al., 2023). This fact could explain why other mechanisms based on aminoarabinosylation are those appearing in clinical strains and in vitro evolution experiments (Cervoni et al., 2023; Table 2).
3.6. Other mechanisms
Finally, some other specific acquired/intrinsic resistance mechanisms have been assessed in terms of their associated costs, and whereas fosfomycin resistance achieved through deletion of glpT (encoding for the glycerol-3-phosphate transporter that enables this drug uptake) caused no measurable fitness defects or even a hypervirulent response (Castañeda-García et al., 2009; Rodríguez-Rojas et al., 2010; Roux et al., 2015), the deletion of aph (encoding for an aminoglycoside phosphotransferase for high-level resistance to kanamycin) caused decreased virulence (Roux et al., 2015). Therefore, these data demonstrate once more that cases in which antimicrobial resistance mechanisms are devoid of costs or even contribute to pathogenesis are not exceptional in P. aeruginosa, and thus contradict the general notion accepting that resistance always entails a fitness cost.
4. Concluding remarks and future directions
As shown throughout this review, the topic of the balance between resistance and fitness/virulence in P. aeruginosa in the field of basic research applied to the context of acute infection has been significantly explored, providing interesting data revealing a great complexity. The published information is especially abundant in the case of mutation-driven mechanisms, and in this specific field but also in that of horizontally-acquired resistance, the diversity of data is astonishing, even with a considerable number of contradictory results. The information gathered here also evidences that there are still many knowledge gaps that need filling, for instance regarding most of the horizontally acquired β-lactamases and aminoglycoside-modifying enzymes, as well as specific mutation-driven mechanisms such as, among others, mutations in the PBP3 encoding gene (ftsI) or in elongation factor G (fusA1), triggering aztreonam and aminoglycoside resistance, respectively. These mechanisms, despite being reported relatively often in clinical strains, have been barely studied in terms of their associated costs (Jorth et al., 2017; Bolard et al., 2018; McLean et al., 2019).
Another field that has been barely approached is the balance between resistance and fitness/virulence not from an acute infection perspective as we have done, but in the context of chronic infection, in which the concept of fitness is much more complex. That is to say, selection for slow growth (which enables a better adaptation to nutrient scarcity and resistance to certain antibiotics) and reduced virulence (triggering a less effective activation of the immune system) is a typical outcome of P. aeruginosa in cystic fibrosis infections for instance (Høiby et al., 2010; Lorè et al., 2012; Malhotra et al., 2019), and therefore, the fitness burden assumed to be negative in the acute context, seems that could be somehow advantageous in the chronic one. In fact, there are very few investigations specifically assessing the antibiotic resistance burden applied to chronic infection (McCaughey et al., 2013; Rundell et al., 2020). Hence, research delving into this intricate field is warranted to better understand the complex adaptive needs that P. aeruginosa have to cope with in such a particular niche.
Returning to the acute context, gaining basic knowledge about resistance-fitness/virulence interplay is envisaged as an essential weapon to reveal therapeutic targets, especially if we consider that new antipseudomonal drugs have been introduced in the last few years (ceftolozane/tazobactam, imipenem/relebactam, ceftazidime/avibactam, and cefiderocol) and others (aztreonam/avibactam, cefepime/zidebactam, and cefepime/taniborbactam) are likely to be approved in the near future (Yahav et al., 2020). Therefore, we need to determine whether the resistance mechanisms selected against these new drugs could inevitably carry high biological costs, and consequently entail increased difficulties for mutation-driven compensation. Obviously, information regarding the fitness cost for these new drugs is still scarce (Gomis-Font et al., 2020; Barceló et al., 2021) and must be amplified since it may be useful for the development of anti-virulence and/or resistance-breaking strategies (Mark et al., 2011; Guillard et al., 2016; De Oliveira et al., 2020; Rezzoagli et al., 2020; Langendonk et al., 2021; Liao et al., 2022; Reig et al., 2022). Unfortunately, the analysis of the epidemiological picture of the resistance phenomenon in P. aeruginosa reveals a warning signal as is the clear association between certain mutation-driven/horizontal mechanisms and successful high-risk clones, indicating that the associated burden of classic antibiotic resistance is not a significant barrier to the appearance and dissemination of many mechanisms (Oliver et al., 2015; Del Barrio-Tofiño et al., 2020; Kocsis et al., 2021). This idea has to be considered as a real possibility also for the mentioned new antipseudomonal agents [e.g., AmpC/FOX variants causing ceftolozane-tazobactam resistance with very little burden, see above (Barceló et al., 2022b)], but still, a deeper analysis must be approached with the hope of finding new drugs (including combinations with classic ones) for which resistance mechanisms are necessarily associated with high fitness/virulence impairments. Finally, since it is a topic in which research is not yet especially prolific, a more profound analysis of P. aeruginosa compensatory evolution associated with the different resistance mechanisms (especially regarding the most recent drugs) is needed in order to identify the genes responsible for compensation mechanisms and determine whether they could be used as alternative therapeutic targets.
Beyond the field of drug development, complete insight into the topic in basic research terms could make it easier to decipher the intricate picture of resistance-virulence co-evolution in the clinical environment. In other words, it could help to understand how antibiotic resistance, depending on the specific mechanism(s), may tune the virulence of P. aeruginosa, thus enabling a prediction of the evolution of pathogenesis and consequences for the patient, which could be very useful in clinical practice (Geisinger and Isberg, 2017). For this purpose, other factors that should be considered, and that are addressed in other reviews are: type of infection, patient background, lineage of the P. aeruginosa strain and its arsenal of virulence factors, and the nature of antibiotic treatments received (Mulet et al., 2013; Peña et al., 2015; Sánchez-Diener et al., 2017; Juan et al., 2017a). If we manage to integrate all these parameters to fully understand the resistance-fitness balance in P. aeruginosa, we will hopefully be capable of predicting which treatments could be more likely to cause deleterious biological costs associated with resistance development, with obvious positive implications for the patients. Unfortunately, these goals still seem far off for the time being and in any case, in order to attain them it is necessary to fully understand the elementary bases that govern resistance-virulence interplay in P. aeruginosa, the field addressed in this review.
A final topic that has been barely studied in specific terms is the fitness cost potentially associated with the phenomenon of adaptive resistance in P. aeruginosa. Given the close relation of this type of transient resistance with stress responses, two-component systems and quorum sensing signaling (Gooderham and Hancock, 2009; Fernández L. et al., 2012; Stewart et al., 2015; Sandoval-Motta and Aldana, 2016; Moradali et al., 2017; Ducret et al., 2022), along with the well-known influence that most of these elements exert on virulence, a connection between adaptive resistance and the temporary modulation of pathogenic behavior is highly likely (Strempel et al., 2013). Thus, this is a virtually virgin topic that should be delved into with the goal of delineating therapeutic strategies to disable this type of inducible resistance and/or take advantage of the potential associated costs.
Altogether, the data reviewed here suggest that we need a holistic approach to understand all the edges of the interplay between resistance and fitness/virulence in P. aeruginosa, including basic but also clinical points of view. Opening/delving into new fields of research such as those mentioned in this manuscript is a must in order to defeat one of the greatest challenges for public health in the twenty-first century, as is P. aeruginosa antibiotic resistance.
Author contributions
EJ-L: Investigation, Writing – original draft. IB: Investigation, Writing – original draft. ME-S: Investigation, Writing – original draft. ME: Investigation, Writing – original draft. LZ: Conceptualization, Supervision, Validation, Writing – original draft. SG-Z: Conceptualization, Funding acquisition, Supervision, Validation, Writing – original draft. ES: Conceptualization, Supervision, Validation, Writing – original draft. AO: Funding acquisition, Supervision, Validation, Writing – review & editing. CJ: Conceptualization, Funding acquisition, Supervision, Validation, Writing – review & editing.
Funding
The author(s) declare financial support was received for the research, authorship, and/or publication of this article. This work was supported by the Balearic Islands Government grant FPI/2206/2019 and grants IJC2019-038836-I (Ministerio de Ciencia e Innovación, Spain), PI21/00509, PI21/00753, PI21/00017, FI19/00004 and CB21/13/00099 [Centro de Investigación Biomédica en Red-Enfermedades Infecciosas (CIBERINFEC-ISCIII)] from the Instituto de Salud Carlos III (Spain) co-financed by the European Regional Development Fund “A way to achieve Europe” and the contract CM22/00008 funded by Instituto de Salud Carlos III and cofunded by the European Union – NextGenerationEU.
Conflict of interest
The authors declare that the research was conducted in the absence of any commercial or financial relationships that could be construed as a potential conflict of interest.
Publisher’s note
All claims expressed in this article are solely those of the authors and do not necessarily represent those of their affiliated organizations, or those of the publisher, the editors and the reviewers. Any product that may be evaluated in this article, or claim that may be made by its manufacturer, is not guaranteed or endorsed by the publisher.
References
Abdelraouf, K., Kabbara, S., Ledesma, K. R., Poole, K., and Tam, V. H. (2011). Effect of multidrug resistance-conferring mutations on the fitness and virulence of Pseudomonas aeruginosa. J. Antimicrob. Chemother. 66, 1311–1317. doi: 10.1093/jac/dkr105
Aendekerk, S., Diggle, S. P., Song, Z., Høiby, N., Cornelis, P., Williams, P., et al. (2005). The MexGHI-OpmD multidrug efflux pump controls growth, antibiotic susceptibility and virulence in Pseudomonas aeruginosa via 4-quinolone-dependent cell-to-cell communication. Microbiology 151, 1113–1125. doi: 10.1099/mic.0.27631-0
Agnello, M., Finkel, S. E., and Wong-Beringer, A. (2016). Fitness cost of fluoroquinolone resistance in clinical isolates of Pseudomonas aeruginosa differs by type III secretion genotype. Front. Microbiol. 7:1591. doi: 10.3389/fmicb.2016.01591
Agnello, M., and Wong-Beringer, A. (2012). Differentiation in quinolone resistance by virulence genotype in Pseudomonas aeruginosa. PLoS One 7:e42973. doi: 10.1371/journal.pone.0042973
Alcalde-Rico, M., Olivares-Pacheco, J., Alvarez-Ortega, C., Cámara, M., and Martínez, J. L. (2018). Role of the multidrug resistance efflux pump MexCD-OprJ in the Pseudomonas aeruginosa quorum sensing response. Front. Microbiol. 9:2752. doi: 10.3389/fmicb.2018.02752
Andersson, D. I. (2006). The biological cost of mutational antibiotic resistance: any practical conclusions? Curr. Opin. Microbiol. 9, 461–465. doi: 10.1016/j.mib.2006.07.002
Andersson, D. I., and Hughes, D. (2010). Antibiotic resistance and its cost: is it possible to reverse resistance. Nat. Rev. Microbiol. 8, 260–271. doi: 10.1038/nrmicro2319
Aoki, S., Hirakata, Y., Kondoh, A., Gotoh, N., Yanagihara, K., Miyazaki, Y., et al. (2004). Virulence of metallo-beta-lactamase-producing Pseudomonas aeruginosa in vitro and in vivo. Antimicrob. Agents Chemother. 48, 1876–1878. doi: 10.1128/AAC.48.5.1876-1878.2004
Arca-Suárez, J., Fraile-Ribot, P., Vázquez-Ucha, J. C., Cabot, G., Martínez-Guitián, M., Lence, E., et al. (2019). Challenging antimicrobial susceptibility and evolution of resistance (OXA-681) during treatment of a long-term nosocomial infection caused by a Pseudomonas aeruginosa ST175 clone. Antimicrob. Agents Chemother. 63, e01110–e01119. doi: 10.1128/AAC.01110-19
Arca-Suárez, J., Lasarte-Monterrubio, C., Rodiño-Janeiro, B. K., Cabot, G., Vázquez-Ucha, J. C., Rodríguez-Iglesias, M., et al. (2021). Molecular mechanisms driving the in vivo development of OXA-10-mediated resistance to ceftolozane/tazobactam and ceftazidime/avibactam during treatment of XDR Pseudomonas aeruginosa infections. J. Antimicrob. Chemother. 76, 91–100. doi: 10.1093/jac/dkaa396
Arca-Suárez, J., Vázquez-Ucha, J. C., Fraile-Ribot, P. A., Lence, E., Cabot, G., Martínez-Guitián, M., et al. (2020). Molecular and biochemical insights into the in vivo evolution of AmpC-mediated resistance to ceftolozane/tazobactam during treatment of an MDR Pseudomonas aeruginosa infection. J. Antimicrob. Chemother. 75, 3209–3217. doi: 10.1093/jac/dkaa291
Balasubramanian, D., Kong, K. F., Jayawardena, S. R., Leal, S. M., Sautter, R. T., and Mathee, K. (2011). Co-regulation of β-lactam resistance, alginate production and quorum sensing in Pseudomonas aeruginosa. J. Med. Microbiol. 60, 147–156. doi: 10.1099/jmm.0.021600-0
Balasubramanian, D., Schneper, L., Merighi, M., Smith, R., Narasimhan, G., Lory, S., et al. (2012). The regulatory repertoire of Pseudomonas aeruginosa AmpC ß-lactamase regulator AmpR includes virulence genes. PLoS One 7:e34067. doi: 10.1371/journal.pone.0034067
Barceló, I., Cabot, G., Palwe, S., Joshi, P., Takalkar, S., Periasamy, H., et al. (2021). In vitro evolution of cefepime/zidebactam (WCK 5222) resistance in Pseudomonas aeruginosa: dynamics, mechanisms, fitness trade-off and impact on in vivo efficacy. J. Antimicrob. Chemother. 76, 2546–2557. doi: 10.1093/jac/dkab213
Barceló, I. M., Jordana-Lluch, E., Escobar-Salom, M., Torrens, G., Fraile-Ribot, P. A., Cabot, G., et al. (2022b). Role of enzymatic activity in the biological cost associated with the production of AmpC β-lactamases in Pseudomonas aeruginosa. Microbiol. Spectr. 10:e0270022. doi: 10.1128/spectrum.02700-22
Barceló, I. M., Torrens, G., Escobar-Salom, M., Jordana-Lluch, E., Capó-Bauzá, M. M., Ramón-Pallín, C., et al. (2022a). Impact of peptidoglycan recycling blockade and expression of horizontally acquired β-lactamases on Pseudomonas aeruginosa virulence. Microbiol. Spectr. 10:e0201921. doi: 10.1128/spectrum.02019-21
Bassetti, M., Poulakou, G., Ruppe, E., Bouza, E., Van Hal, S. J., and Brink, A. (2017). Antimicrobial resistance in the next 30 years, humankind, bugs and drugs: a visionary approach. Intensive Care Med. 43, 1464–1475. doi: 10.1007/s00134-017-4878-x
Beceiro, A., Tomás, M., and Bou, G. (2013). Antimicrobial resistance and virulence: a successful or deleterious association in the bacterial world? Clin. Microbiol. Rev. 26, 185–230. doi: 10.1128/CMR.00059-12
Bishop, R. E., and Weiner, J. H. (1992). Coordinate regulation of murein peptidase activity and AmpC beta-lactamase synthesis in Escherichia coli. FEBS Lett. 304, 103–108. doi: 10.1016/0014-5793(92)80598-B
Bolard, A., Plésiat, P., and Jeannot, K. (2018). Mutations in gene fusA1 as a novel mechanism of aminoglycoside resistance in clinical strains of Pseudomonas aeruginosa. Antimicrob. Agents Chemother. 62, e01835–e01817. doi: 10.1128/AAC.01835-17
Botelho, J., Grosso, F., and Peixe, L. (2019). Antibiotic resistance in Pseudomonas aeruginosa - mechanisms, epidemiology and evolution. Drug Resist. Updat. 44:100640. doi: 10.1016/j.drup.2019.07.002
Bruchmann, S., Dötsch, A., Nouri, B., Chaberny, I. F., and Häussler, S. (2013). Quantitative contributions of target alteration and decreased drug accumulation to Pseudomonas aeruginosa fluoroquinolone resistance. Antimicrob. Agents Chemother. 57, 1361–1368. doi: 10.1128/AAC.01581-12
Cabot, G., Bruchmann, S., Mulet, X., Zamorano, L., Moyà, B., Juan, C., et al. (2014). Pseudomonas aeruginosa ceftolozane-tazobactam resistance development requires multiple mutations leading to overexpression and structural modification of AmpC. Antimicrob. Agents Chemother. 58, 3091–3099. doi: 10.1128/AAC.02462-13
Cabot, G., Florit-Mendoza, L., Sánchez-Diener, I., Zamorano, L., and Oliver, A. (2018). Deciphering β-lactamase-independent β-lactam resistance evolution trajectories in Pseudomonas aeruginosa. J. Antimicrob. Chemother. 73, 3322–3331. doi: 10.1093/jac/dky364
Cabot, G., Ocampo-Sosa, A. A., Domínguez, M. A., Gago, J. F., Juan, C., Tubau, F., et al. (2012). Genetic markers of widespread extensively drug-resistant Pseudomonas aeruginosa high-risk clones. Antimicrob. Agents Chemother. 56, 6349–6357. doi: 10.1128/AAC.01388-12
Cabot, G., Zamorano, L., Moyà, B., Juan, C., Navas, A., Blázquez, J., et al. (2016). Evolution of Pseudomonas aeruginosa antimicrobial resistance and fitness under low and high mutation rates. Antimicrob. Agents Chemother. 60, 1767–1778. doi: 10.1128/AAC.02676-15
Caille, O., Zincke, D., Merighi, M., Balasubramanian, D., Kumari, H., Kong, K. F., et al. (2014). Structural and functional characterization of Pseudomonas aeruginosa global regulator AmpR. J. Bacteriol. 196, 3890–3902. doi: 10.1128/JB.01997-14
Carattoli, A. (2013). Plasmids and the spread of resistance. Int. J. Med. Microbiol. 303, 298–304. doi: 10.1016/j.ijmm.2013.02.001
Castañeda-García, A., Rodríguez-Rojas, A., Guelfo, J. R., and Blázquez, J. (2009). The glycerol-3-phosphate permease GlpT is the only fosfomycin transporter in Pseudomonas aeruginosa. J. Bacteriol. 191, 6968–6974. doi: 10.1128/JB.00748-09
Cepas, V., and Soto, S. M. (2020). Relationship between virulence and resistance among gram-negative bacteria. Antibiotics 9:719. doi: 10.3390/antibiotics9100719
Cervoni, M., Lo Sciuto, A., Bianchini, C., Mancone, C., and Imperi, F. (2021). Exogenous and endogenous phosphoethanolamine transferases differently affect colistin resistance and fitness in Pseudomonas aeruginosa. Front. Microbiol. 12:778968. doi: 10.3389/fmicb.2021.778968
Cervoni, M., Sposato, D., Lo Sciuto, A., and Imperi, F. (2023). Regulatory landscape of the Pseudomonas aeruginosa phosphoethanolamine transferase gene eptA in the context of colistin resistance. Antibiotics 12:200. doi: 10.3390/antibiotics12020200
Chang, Y. H., Labgold, M. R., and Richards, J. H. (1990). Altering enzymatic activity: recruitment of carboxypeptidase activity into an RTEM beta-lactamase/penicillin-binding protein 5 chimera. Proc. Natl. Acad. Sci. U. S. A. 87, 2823–2827. doi: 10.1073/pnas.87.7.2823
Chávez-Jacobo, V. M., Hernández-Ramírez, K. C., Romo-Rodríguez, P., Pérez-Gallardo, R. V., Campos-García, J., Gutiérrez-Corona, J. F., et al. (2018). CrpP is a novel ciprofloxacin-modifying enzyme encoded by the Pseudomonas aeruginosa pUM505 plasmid. Antimicrob. Agents Chemother. 62, e02629–e02617. doi: 10.1128/AAC.02629-17
Chevalier, S., Bouffartigues, E., Bodilis, J., Maillot, O., Lesouhaitier, O., Feuilloley, M. G. J., et al. (2017). Structure, function and regulation of Pseudomonas aeruginosa porins. FEMS Microbiol. Rev. 41, 698–722. doi: 10.1093/femsre/fux020
Cho, H. H., Kwon, K. C., Kim, S., and Koo, S. H. (2014). Correlation between virulence genotype and fluoroquinolone resistance in carbapenem-resistant Pseudomonas aeruginosa. Ann. Lab. Med. 34, 286–292. doi: 10.3343/alm.2014.34.4.286
Colquhoun, J. M., Farokhyfar, M., Anderson, A. C., Bethel, C. R., Bonomo, R. A., Clarke, A. J., et al. (2023). Collateral changes in cell physiology associated with ADC-7 β-lactamase expression in Acinetobacter baumannii. Microbiol Spectr. 19:e0464622. doi: 10.1128/spectrum.04646-22
Colquhoun, J. M., Farokhyfar, M., Hutcheson, A. R., Anderson, A., Bethel, C. R., Bonomo, R. A., et al. (2021). OXA-23 β-lactamase overexpression in Acinetobacter baumannii drives physiological changes resulting in new genetic vulnerabilities. MBio 12, e03137–e03121. doi: 10.1128/mBio.03137-21
Cordeiro, N. F., Chabalgoity, J. A., Yim, L., and Vignoli, R. (2014). Synthesis of metallo-β-lactamase VIM-2 is associated with a fitness reduction in Salmonella enterica Serovar typhimurium. Antimicrob. Agents Chemother. 58, 6528–6535. doi: 10.1128/AAC.02847-14
Cosson, P., Zulianello, L., Join-Lambert, O., Faurisson, F., Gebbie, L., Benghezal, M., et al. (2002). Pseudomonas aeruginosa virulence analyzed in a Dictyostelium discoideum host system. J. Bacteriol. 184, 3027–3033. doi: 10.1128/JB.184.11.3027-3033.2002
Cottell, J. L., Webber, M. A., and Piddock, L. J. (2012). Persistence of transferable extended-spectrum-β-lactamase resistance in the absence of antibiotic pressure. Antimicrob. Agents Chemother. 56, 4703–4706. doi: 10.1128/AAC.00848-12
Damblon, C., Zhao, G. H., Jamin, M., Ledent, P., Dubus, A., Vanhove, M., et al. (1995). Breakdown of the stereospecificity of DD-peptidases and beta-lactamases with thiolester substrates. Biochem. J. 309, 431–436. doi: 10.1042/bj3090431
De Oliveira, D. M. P., Forde, B. M., Kidd, T. J., Harris, P. N. A., Schembri, M. A., Beatson, S. A., et al. (2020). Antimicrobial resistance in ESKAPE pathogens. Clin. Microbiol. Rev. 33, e00181–e00119. doi: 10.1128/CMR.00181-19
Del Barrio-Tofiño, E., López-Causapé, C., Cabot, G., Rivera, A., Benito, N., Segura, C., et al. (2017). Genomics and susceptibility profiles of extensively drug-resistant Pseudomonas aeruginosa isolates from Spain. Antimicrob. Agents Chemother. 61, e01589–e01517. doi: 10.1128/AAC.01589-17
Del Barrio-Tofiño, E., López-Causapé, C., and Oliver, A. (2020). Pseudomonas aeruginosa epidemic high-risk clones and their association with horizontally-acquired β-lactamases: 2020 update. Int. J. Antimicrob. Agents 56:106196. doi: 10.1016/j.ijantimicag.2020.106196
Deptuła, A., and Gospodarek, E. (2010). Reduced expression of virulence factors in multidrug-resistant Pseudomonas aeruginosa strains. Arch. Microbiol. 192, 79–84. doi: 10.1007/s00203-009-0528-1
Dößelmann, B., Willmann, M., Steglich, M., Bunk, B., Nübel, U., Peter, S., et al. (2017). Rapid and consistent evolution of colistin resistance in extensively drug-resistant Pseudomonas aeruginosa during morbidostat culture. Antimicrob. Agents Chemother. 61, e00043–e00017. doi: 10.1128/AAC.00043-17
Dubois, D., Prasadarao, N. V., Mittal, R., Bret, L., Roujou-Gris, M., and Bonnet, R. (2009). CTX-M beta-lactamase production and virulence of Escherichia coli K1. Emerg. Infect. Dis. 15, 1988–1990. doi: 10.3201/eid1512.090928
Ducret, V., Perron, K., and Valentini, M. (2022). Role of two-component system networks in Pseudomonas aeruginosa pathogenesis. Adv. Exp. Med. Biol. 1386, 371–395. doi: 10.1007/978-3-031-08491-1_14
Escobar-Salom, M., Barceló, I. M., Jordana-Lluch, E., Torrens, G., Oliver, A., and Juan, C. (2023). Bacterial virulence regulation through soluble peptidoglycan fragments sensing and response: knowledge gaps and therapeutic potential. FEMS Microbiol. Rev. 47:fuad010. doi: 10.1093/femsre/fuad010
Escudero, J. A., Loot, C., Nivina, A., and Mazel, D. (2015). The integron: adaptation on demand. Microbiol. Spectr. 3:MDNA3-0019-2014. doi: 10.1128/microbiolspec.MDNA3-0019-2014
Evans, K., Passador, L., Srikumar, R., Tsang, E., Nezezon, J., and Poole, K. (1998). Influence of the MexAB-OprM multidrug efflux system on quorum sensing in Pseudomonas aeruginosa. J. Bacteriol. 180, 5443–5447. doi: 10.1128/JB.180.20.5443-5447.1998
Faccone, D., de Mendieta, J. M., Albornoz, E., Chavez, M., Genero, F., Echegorry, M., et al. (2022). Emergence of KPC-31, a KPC-3 variant associated with ceftazidime-avibactam resistance, in an extensively drug-resistant ST235 Pseudomonas aeruginosa clinical isolate. Antimicrob. Agents Chemother. 66:e0064822. doi: 10.1128/aac.00648-22
Fernández, L., Jenssen, H., Bains, M., Wiegand, I., Gooderham, W. J., and Hancock, R. E. (2012). The two-component system CprRS senses cationic peptides and triggers adaptive resistance in Pseudomonas aeruginosa independently of ParRS. Antimicrob. Agents Chemother. 56, 6212–6222. doi: 10.1128/AAC.01530-12
Fernández, A., Pérez, A., Ayala, J. A., Mallo, S., Rumbo-Feal, S., Tomas, M., et al. (2012). Expression of OXA-type and SFO-1 beta-lactamases induces changes in peptidoglycan composition and affects bacterial fitness. Antimicrob. Agents Chemother. 56, 1877–1884. doi: 10.1128/AAC.05402-11
Fernando, D. M., and Kumar, A. (2013). Resistance-nodulation-division multidrug efflux pumps in gram-negative bacteria: role in virulence. Antibiotics 2, 163–181. doi: 10.3390/antibiotics2010163
Folkesson, A., Eriksson, S., Andersson, M., Park, J. T., and Normark, S. (2005). Components of the peptidoglycan-recycling pathway modulate invasion and intracellular survival of Salmonella enterica serovar typhimurium. Cell. Microbiol. 7, 147–155. doi: 10.1111/j.1462-5822.2004.00443.x
Fraile-Ribot, P. A., Cabot, G., Mulet, X., Periañez, L., Martín-Pena, M. L., Juan, C., et al. (2018). Mechanisms leading to in vivo ceftolozane/tazobactam resistance development during the treatment of infections caused by MDR Pseudomonas aeruginosa. J. Antimicrob. Chemother. 73, 658–663. doi: 10.1093/jac/dkx424
Fraile-Ribot, P. A., Zamorano, L., Orellana, R., Del Barrio-Tofiño, E., Sánchez-Diener, I., Cortes-Lara, S., et al. (2020). Activity of imipenem-relebactam against a large collection of Pseudomonas aeruginosa clinical isolates and isogenic β-lactam-resistant mutants. Antimicrob. Agents Chemother. 64, e02165–e02119. doi: 10.1128/AAC.02165-19
Freire, C. M. A. D. S., Taunay-Rodrigues, A., Gonzatti, M. B., Fonseca, F. M. P., and Freire, J. E. D. C. (2021). New insights about the EptA protein and its correlation with the pmrC gene in polymyxin resistance in Pseudomonas aeruginosa. Curr. Res. Microb. Sci. 2:100042. doi: 10.1016/j.crmicr.2021.100042
Gallant, C. V., Daniels, C., Leung, J. M., Ghosh, A. S., Young, K. D., Kotra, L. P., et al. (2005). Common beta-lactamases inhibit bacterial biofilm formation. Mol. Microbiol. 58, 1012–1024. doi: 10.1111/j.1365-2958.2005.04892.x
Geisinger, E., and Isberg, R. R. (2017). Interplay between antibiotic resistance and virulence during disease promoted by multidrug-resistant Bacteria. J. Infect. Dis. 215, S9–S17. doi: 10.1093/infdis/jiw402
Gifford, D. R., Moss, E., and MacLean, R. C. (2016b). Environmental variation alters the fitness effects of rifampicin resistance mutations in Pseudomonas aeruginosa. Evolution 70, 725–730. doi: 10.1111/evo.12880
Gifford, D. R., Toll-Riera, M., and MacLean, R. C. (2016a). Epistatic interactions between ancestral genotype and beneficial mutations shape evolvability in Pseudomonas aeruginosa. Evolution 70, 1659–1666. doi: 10.1111/evo.12958
Gomez-Simmonds, A., and Uhlemann, A. C. (2017). Clinical implications of genomic adaptation and evolution of Carbapenem-resistant Klebsiella pneumoniae. J. Infect. Dis. 215, S18–S27. doi: 10.1093/infdis/jiw378
Gómez-Zorrilla, S., Juan, C., Cabot, G., Camoez, M., Tubau, F., Oliver, A., et al. (2016). Impact of multidrug resistance on the pathogenicity of Pseudomonas aeruginosa: in vitro and in vivo studies. Int. J. Antimicrob. Agents 47, 368–374. doi: 10.1016/j.ijantimicag.2016.02.010
Gomis-Font, M. A., Cabot, G., López-Argüello, S., Zamorano, L., Juan, C., Moyá, B., et al. (2022). Comparative analysis of in vitro dynamics and mechanisms of ceftolozane/tazobactam and imipenem/relebactam resistance development in Pseudomonas aeruginosa XDR high-risk clones. J. Antimicrob. Chemother. 77, 957–968. doi: 10.1093/jac/dkab496
Gomis-Font, M. A., Cabot, G., Sánchez-Diener, I., Fraile-Ribot, P. A., Juan, C., Moya, B., et al. (2020). In vitro dynamics and mechanisms of resistance development to imipenem and imipenem/relebactam in Pseudomonas aeruginosa. J. Antimicrob. Chemother. 75, 2508–2515. doi: 10.1093/jac/dkaa206
Gomis-Font, M. A., Sastre-Femenia, M. À., Taltavull, B., Cabot, G., and Oliver, A. (2023). In vitro dynamics and mechanisms of cefiderocol resistance development in wild-type, mutator and XDR Pseudomonas aeruginosa. J. Antimicrob. Chemother. 30:dkad172. doi: 10.1093/jac/dkad172
Gooderham, W. J., and Hancock, R. E. (2009). Regulation of virulence and antibiotic resistance by two-component regulatory systems in Pseudomonas aeruginosa. FEMS Microbiol. Rev. 33, 279–294. doi: 10.1111/j.1574-6976.2008.00135.x
Guillard, T., Pons, S., Roux, D., Pier, G. B., and Skurnik, D. (2016). Antibiotic resistance and virulence: understanding the link and its consequences for prophylaxis and therapy. Bioessays 38, 682–693. doi: 10.1002/bies.201500180
Hall, A. R., and MacLean, R. C. (2011). Epistasis buffers the fitness effects of rifampicin- resistance mutations in Pseudomonas aeruginosa. Evolution 65, 2370–2379. doi: 10.1111/j.1558-5646.2011.01302.x
Harrison, E., and Brockhurst, M. A. (2012). Plasmid-mediated horizontal gene transfer is a coevolutionary process. Trends Microbiol. 20, 262–267. doi: 10.1016/j.tim.2012.04.003
Hendrickson, E. L., Plotnikova, J., Mahajan-Miklos, S., Rahme, L. G., and Ausubel, F. M. (2001). Differential roles of the Pseudomonas aeruginosa PA14 rpoN gene in pathogenicity in plants, nematodes, insects, and mice. J. Bacteriol. 183, 7126–7134. doi: 10.1128/JB.183.24.7126-7134.2001
Hernando-Amado, S., Laborda, P., Valverde, J. R., and Martínez, J. L. (2022). Rapid decline of ceftazidime resistance in antibiotic-free and sublethal environments is contingent on genetic background. Mol. Biol. Evol. 39:msac049. doi: 10.1093/molbev/msac049
Hirakata, Y., Kondo, A., Hoshino, K., Yano, H., Arai, K., Hirotani, A., et al. (2009). Efflux pump inhibitors reduce the invasiveness of Pseudomonas aeruginosa. Int. J. Antimicrob. Agents 34, 343–346. doi: 10.1016/j.ijantimicag.2009.06.007
Hirakata, Y., Srikumar, R., Poole, K., Gotoh, N., Suematsu, T., Kohno, S., et al. (2002). Multidrug efflux systems play an important role in the invasiveness of Pseudomonas aeruginosa. J. Exp. Med. 196, 109–118. doi: 10.1084/jem.20020005
Høiby, N., Ciofu, O., and Bjarnsholt, T. (2010). Pseudomonas aeruginosa biofilms in cystic fibrosis. Future Microbiol. 5, 663–674. doi: 10.2217/fmb.10.125
Ikarashi, K., Kutsuna, R., Tomida, J., Kawamura, Y., and Morita, Y. (2021). Overexpression of the MexXY multidrug efflux system correlates with deficient pyoverdine production in Pseudomonas aeruginosa. Antibiotics 10:658. doi: 10.3390/antibiotics10060658
Javed, M., Jentzsch, B., Heinrich, M., Ueltzhoeffer, V., Peter, S., Schoppmeier, U., et al. (2021). Transcriptomic basis of serum resistance and virulence related traits in XDR P. aeruginosa evolved under antibiotic pressure in a morbidostat device. Front. Microbiol. 11:619542. doi: 10.3389/fmicb.2020.619542
Jeannot, K., Bolard, A., and Plésiat, P. (2017). Resistance to polymyxins in gram-negative organisms. Int. J. Antimicrob. Agents 49, 526–535. doi: 10.1016/j.ijantimicag.2016.11.029
Jeannot, K., Elsen, S., Köhler, T., Attree, I., van Delden, C., and Plésiat, P. (2008). Resistance and virulence of Pseudomonas aeruginosa clinical strains overproducing the MexCD-OprJ efflux pump. Antimicrob. Agents Chemother. 52, 2455–2462. doi: 10.1128/AAC.01107-07
Jia, X., Zhu, Y., Jia, P., Liu, X., Yu, W., Li, X., et al. (2022). Emergence of a superplasmid coharboring hypervirulence and multidrug resistance genes in Klebsiella pneumoniae poses new challenges to public health. Microbiol. Spectr. 10:e0263422. doi: 10.1128/spectrum.02634-22
Jorth, P., McLean, K., Ratjen, A., Secor, P. R., Bautista, G. E., Ravishankar, S., et al. (2017). Evolved aztreonam resistance is multifactorial and can produce hypervirulence in Pseudomonas aeruginosa. MBio 8:e00517. doi: 10.1128/mBio.00517-17
Juan, C., Peña, C., and Oliver, A. (2017a). Host and pathogen biomarkers for severe Pseudomonas aeruginosa infections. J. Infect. Dis. 215, S44–S51. doi: 10.1093/infdis/jiw299
Juan, C., Torrens, G., González-Nicolau, M., and Oliver, A. (2017b). Diversity and regulation of intrinsic β-lactamases from non-fermenting and other gram-negative opportunistic pathogens. FEMS Microbiol. Rev. 41, 781–815. doi: 10.1093/femsre/fux043
Kaiser, S. J., Mutters, N. T., DeRosa, A., Ewers, C., Frank, U., and Günther, F. (2017). Determinants for persistence of Pseudomonas aeruginosa in hospitals: interplay between resistance, virulence and biofilm formation. Eur. J. Clin. Microbiol. Infect. Dis. 36, 243–253. doi: 10.1007/s10096-016-2792-8
Karatuna, O., and Yagci, A. (2010). Analysis of quorum sensing-dependent virulence factor production and its relationship with antimicrobial susceptibility in Pseudomonas aeruginosa respiratory isolates. Clin. Microbiol. Infect. 16, 1770–1775. doi: 10.1111/j.1469-0691.2010.03177.x
Khan, M., Summers, S., Rice, S. A., Stapleton, F., Willcox, M. D. P., and Subedi, D. (2020). Acquired fluoroquinolone resistance genes in corneal isolates of Pseudomonas aeruginosa. Infect. Genet. Evol. 85:104574. doi: 10.1016/j.meegid.2020.104574
Kocsis, B., Gulyás, D., and Szabó, D. (2021). Diversity and distribution of resistance markers in Pseudomonas aeruginosa international high-risk clones. Microorganisms 9:359. doi: 10.3390/microorganisms9020359
Köhler, T., van Delden, C., Curty, L. K., Hamzehpour, M. M., and Pechere, J. C. (2001). Overexpression of the MexEF-OprN multidrug efflux system affects cell-to-cell signaling in Pseudomonas aeruginosa. J. Bacteriol. 183, 5213–5222. doi: 10.1128/JB.183.18.5213-5222.2001
Kong, K. F., Jayawardena, S. R., Indulkar, S. D., Del Puerto, A., Koh, C. L., Hoiby, N., et al. (2005). Pseudomonas aeruginosa AmpR is a global transcriptional factor that regulates expression of AmpC and PoxB beta-lactamases, proteases, quorum sensing, and other virulence factors. Antimicrob. Agents Chemother. 49, 4567–4575. doi: 10.1128/AAC.49.11.4567-4575.2005
Kottara, A., Hall, J. P. J., Harrison, E., and Brockhurst, M. A. (2018). Variable plasmid fitness effects and mobile genetic element dynamics across Pseudomonas species. FEMS Microbiol. Ecol. 94:fix172. doi: 10.1093/femsec/fix172
Kugelberg, E., Löfmark, S., Wretlind, B., and Andersson, D. I. (2005). Reduction of the fitness burden of quinolone resistance in Pseudomonas aeruginosa. J. Antimicrob. Chemother. 55, 22–30. doi: 10.1093/jac/dkh505
Lamarche, M. G., and Déziel, E. (2011). MexEF-OprN efflux pump exports the Pseudomonas quinolone signal (PQS) precursor HHQ (4-hydroxy-2-heptylquinoline). PLoS One 6:e24310. doi: 10.1371/journal.pone.0024310
Langendonk, R. F., Neill, D. R., and Fothergill, J. L. (2021). The building blocks of antimicrobial resistance in Pseudomonas aeruginosa: implications for current resistance-breaking therapies. Front. Cell. Infect. Microbiol. 11:665759. doi: 10.3389/fcimb.2021.665759
Lasarte-Monterrubio, C., Fraile-Ribot, P. A., Vázquez-Ucha, J. C., Cabot, G., Guijarro-Sánchez, P., Alonso-García, I., et al. (2022). Activity of cefiderocol, imipenem/relebactam, cefepime/taniborbactam and cefepime/zidebactam against ceftolozane/tazobactam- and ceftazidime/avibactam-resistant Pseudomonas aeruginosa. J. Antimicrob. Chemother. 77, 2809–2815. doi: 10.1093/jac/dkac241
Lee, H., Shin, J., Chung, Y. J., Park, M., Kang, K. J., Baek, J. Y., et al. (2020). Co-introduction of plasmids harbouring the carbapenemase genes, blaNDM-1 and blaOXA-232, increases fitness and virulence of bacterial host. J. Biomed. Sci. 27:8. doi: 10.1186/s12929-019-0603-0
Li, Q., Chen, Q., Liang, S., Wang, W., Zhang, B., Martín-Rodríguez, A. J., et al. (2023). Coexistence of tmexCD3-toprJ1b tigecycline resistance genes with two novel blaVIM-2-carrying and blaOXA-10-carrying transposons in a Pseudomononas asiatica plasmid. Front. Cell. Infect. Microbiol. 13:1130333. doi: 10.3389/fcimb.2023.1130333
Liao, C., Huang, X., Wang, Q., Yao, D., and Lu, W. (2022). Virulence factors of Pseudomonas aeruginosa and Antivirulence strategies to combat its drug resistance. Front. Cell. Infect. Microbiol. 12:926758. doi: 10.3389/fcimb.2022.926758
Linares, J. F., López, J. A., Camafeita, E., Albar, J. P., Rojo, F., and Martinez, J. L. J. (2005). Overexpression of the multidrug efflux pumps MexCD-OprJ and MexEF-OprN is associated with a reduction of type III secretion in Pseudomonas aeruginosa. J. Bacteriol. 187, 1384–1391. doi: 10.1128/JB.187.4.1384-1391.2005
Lo Sciuto, A., Cervoni, M., Stefanelli, R., Mancone, C., and Imperi, F. (2020). Effect of lipid A aminoarabinosylation on Pseudomonas aeruginosa colistin resistance and fitness. Int. J. Antimicrob. Agents 55:105957. doi: 10.1016/j.ijantimicag.2020.105957
López-Causapé, C., Cabot, G., Del Barrio-Tofiño, E., and Oliver, A. (2018). The versatile mutational resistome of Pseudomonas aeruginosa. Front. Microbiol. 9:685. doi: 10.3389/fmicb.2018.00685
Lorè, N. I., Cigana, C., De Fino, I., Riva, C., Juhas, M., Schwager, S., et al. (2012). Cystic fibrosis-niche adaptation of Pseudomonas aeruginosa reduces virulence in multiple infection hosts. PLoS One 7:e35648. doi: 10.1371/journal.pone.0035648
Lorusso, A. B., Carrara, J. A., Barroso, C. D. N., Tuon, F. F., and Faoro, H. (2022). Role of efflux pumps on antimicrobial resistance in Pseudomonas aeruginosa. Int. J. Mol. Sci. 23:15779. doi: 10.3390/ijms232415779
Luong, P. M., Shogan, B. D., Zaborin, A., Belogortseva, N., Shrout, J. D., Zaborina, O., et al. (2014). Emergence of the P2 phenotype in Pseudomonas aeruginosa PAO1 strains involves various mutations in mexT or mexF. J. Bacteriol. 196, 504–513. doi: 10.1128/JB.01050-13
MacLean, R. C., and Vogwill, T. (2014). Limits to compensatory adaptation and the persistence of antibiotic resistance in pathogenic bacteria. Evol. Med. Public Health 2015, 4–12. doi: 10.1093/emph/eou032
Magiorakos, A. P., Srinivasan, A., Carey, R. B., Carmeli, Y., Falagas, M. E., Giske, C. G., et al. (2012). Multidrug-resistant, extensively drug-resistant and pandrug-resistant bacteria: an international expert proposal for interim standard definitions for acquired resistance. Clin. Microbiol. Infect. 18, 268–281. doi: 10.1111/j.1469-0691.2011.03570.x
Malhotra, S., Hayes, D. Jr., and Wozniak, D. J. (2019). Cystic fibrosis and Pseudomonas aeruginosa: the host-microbe Interface. Clin. Microbiol. Rev. 32, e00138–e00118. doi: 10.1128/CMR.00138-18
Malik, M., Zhao, X., and Drlica, K. (2006). Lethal fragmentation of bacterial chromosomes mediated by DNA gyrase and quinolones. Mol. Microbiol. 61, 810–825. doi: 10.1111/j.1365-2958.2006.05275.x
Marciano, D. C., Karkouti, O. Y., and Palzkill, T. (2007). A fitness cost associated with the antibiotic resistance enzyme SME-1 beta-lactamase. Genetics 176, 2381–2392. doi: 10.1534/genetics.106.069443
Mark, B. L., Vocadlo, D. J., and Oliver, A. (2011). Providing β-lactams a helping hand: targeting the AmpC β-lactamase induction pathway. Future Microbiol. 6, 1415–1427. doi: 10.2217/fmb.11.128
Martínez-Ramos, I., Mulet, X., Moyá, B., Barbier, M., Oliver, A., and Albertí, S. (2014). Overexpression of MexCD-OprJ reduces Pseudomonas aeruginosa virulence by increasing its susceptibility to complement-mediated killing. Antimicrob. Agents Chemother. 58, 2426–2429. doi: 10.1128/AAC.02012-13
McCaughey, G., Diamond, P., Elborn, J. S., McKevitt, M., and Tunney, M. M. (2013). Resistance development of cystic fibrosis respiratory pathogens when exposed to fosfomycin and tobramycin alone and in combination under aerobic and anaerobic conditions. PLoS One 8:e69763. doi: 10.1371/journal.pone.0069763
McLean, K., Lee, D., Holmes, E. A., Penewit, K., Waalkes, A., Ren, M., et al. (2019). Genomic analysis identifies novel Pseudomonas aeruginosa resistance genes under selection during inhaled aztreonam therapy in vivo. Antimicrob. Agents Chemother. 63, e00866–e00819. doi: 10.1128/AAC.00866-19
Melnyk, A. H., McCloskey, N., Hinz, A. J., Dettman, J., and Kassen, R. (2017). Evolution of cost-free resistance under fluctuating drug selection in Pseudomonas aeruginosa. mSphere 2, e00158–e00117. doi: 10.1128/mSphere.00158-17
Melnyk, A. H., Wong, A., and Kassen, R. (2015). The fitness costs of antibiotic resistance mutations. Evol. Appl. 8, 273–283. doi: 10.1111/eva.12196
Moradali, M. F., Ghods, S., and Rehm, B. H. (2017). Pseudomonas aeruginosa lifestyle: a paradigm for adaptation, survival, and persistence. Front. Cell. Infect. Microbiol. 7:39. doi: 10.3389/fcimb.2017.00039
Morosini, M. I., Ayala, J. A., Baquero, F., Martínez, J. L., and Blázquez, J. (2000). Biological cost of AmpC production for Salmonella enterica serotype typhimurium. Antimicrob. Agents Chemother. 44, 3137–3143. doi: 10.1128/AAC.44.11.3137-3143.2000
Moya, B., Juan, C., Albertí, S., Pérez, J. L., and Oliver, A. (2008). Benefit of having multiple ampD genes for acquiring beta-lactam resistance without losing fitness and virulence in Pseudomonas aeruginosa. Antimicrob. Agents Chemother. 52, 3694–3700. doi: 10.1128/AAC.00172-08
Mulet, X., Cabot, G., Ocampo-Sosa, A. A., Domínguez, M. A., Zamorano, L., Juan, C., et al. (2013). Biological markers of Pseudomonas aeruginosa epidemic high-risk clones. Antimicrob. Agents Chemother. 57, 5527–5535. doi: 10.1128/AAC.01481-13
Nitz, F., de Melo, B. O., da Silva, L. C. N., de Souza, M. A., Marques, S. G., Monteiro-Neto, V., et al. (2021). Molecular detection of drug-resistance genes of blaOXA-23-blaOXA-51 and mcr-1 in clinical isolates of Pseudomonas aeruginosa. Microorganisms 9:786. doi: 10.3390/microorganisms9040786
Nowicki, E. M., O'Brien, J. P., Brodbelt, J. S., and Trent, M. S. (2015). Extracellular zinc induces phosphoethanolamine addition to Pseudomonas aeruginosa lipid A via the ColRS two-component system. Mol. Microbiol. 97, 166–178. doi: 10.1111/mmi.13018
Olivares, J., Alvarez-Ortega, C., Linares, J. F., Rojo, F., Köhler, T., and Martínez, J. L. (2012). Overproduction of the multidrug efflux pump MexEF-OprN does not impair Pseudomonas aeruginosa fitness in competition tests, but produces specific changes in bacterial regulatory networks. Environ. Microbiol. 14, 1968–1981. doi: 10.1111/j.1462-2920.2012.02727.x
Olivares, J., Álvarez-Ortega, C., and Martinez, J. L. (2014). Metabolic compensation of fitness costs associated with overexpression of the multidrug efflux pump MexEF-OprN in Pseudomonas aeruginosa. Antimicrob. Agents Chemother. 58, 3904–3913. doi: 10.1128/AAC.00121-14
Olivares Pacheco, J., Alvarez-Ortega, C., Alcalde Rico, M., and Martínez, J. L. (2017). Metabolic compensation of fitness costs is a general outcome for antibiotic-resistant Pseudomonas aeruginosa mutants overexpressing efflux pumps. MBio 8, e00500–e00517. doi: 10.1128/mBio.00500-17
Olivas, A. D., Shogan, B. D., Valuckaite, V., Zaborin, A., Belogortseva, N., Musch, M., et al. (2012). Intestinal tissues induce an SNP mutation in Pseudomonas aeruginosa that enhances its virulence: possible role in anastomotic leak. PLoS One 7:e44326. doi: 10.1371/journal.pone.0044326
Oliver, A., Mulet, X., López-Causapé, C., and Juan, C. (2015). The increasing threat of Pseudomonas aeruginosa high-risk clones. Drug Resist. Updat. 21–22, 41–59. doi: 10.1016/j.drup.2015.08.002
Peña, C., Cabot, G., Gómez-Zorrilla, S., Zamorano, L., Ocampo-Sosa, A., Murillas, J., et al. (2015). Influence of virulence genotype and resistance profile in the mortality of Pseudomonas aeruginosa bloodstream infections. Clin. Infect. Dis. 60, 539–548. doi: 10.1093/cid/ciu866
Pérez-Gallego, M., Torrens, G., Castillo-Vera, J., Moya, B., Zamorano, L., Cabot, G., et al. (2016). Impact of AmpC derepression on fitness and virulence: the mechanism or the pathway? MBio 7, e01783–e01716. doi: 10.1128/mBio.01783-16
Perron, G. G., Hall, A. R., and Buckling, A. (2010). Hypermutability and compensatory adaptation in antibiotic-resistant bacteria. Am. Nat. 176, 303–311. doi: 10.1086/655217
Pongchaikul, P., and Mongkolsuk, P. (2022). Comprehensive analysis of imipenemase (IMP)-type metallo-β-lactamase: a global distribution threatening asia. Antibiotics 11:236. doi: 10.3390/antibiotics11020236
Pratt, R. F. (2016). β-Lactamases: why and how. J. Med. Chem. 59, 8207–8220. doi: 10.1021/acs.jmedchem.6b00448
Qi, Q., Toll-Riera, M., Heilbron, K., Preston, G. M., and MacLean, R. C. (2016). The genomic basis of adaptation to the fitness cost of rifampicin resistance in Pseudomonas aeruginosa. Proc. Biol. Sci. 283:20152452. doi: 10.1098/rspb.2015.2452
Rajer, F., and Sandegren, L. (2022). The role of antibiotic resistance genes in the fitness cost of multiresistance plasmids. MBio 13:e0355221. doi: 10.1128/mbio.03552-21
Ramisse, F., van Delden, C., Gidenne, S., Cavallo, J., and Hernandez, E. (2000). Decreased virulence of a strain of Pseudomonas aeruginosa O12 overexpressing a chromosomal type 1 beta-lactamase could be due to reduced expression of cell-to-cell signaling dependent virulence factors. FEMS Immunol. Med. Microbiol. 28, 241–245. doi: 10.1111/j.1574-695X.2000.tb01483.x
Rampioni, G., Pillai, C. R., Longo, F., Bondì, R., Baldelli, V., Messina, M., et al. (2017). Effect of efflux pump inhibition on Pseudomonas aeruginosa transcriptome and virulence. Sci. Rep. 7:11392. doi: 10.1038/s41598-017-11892-9
Recio, R., Sánchez-Diener, I., Viedma, E., Meléndez-Carmona, M. Á., Villa, J., Orellana, M. Á., et al. (2020). Pathogenic characteristics of Pseudomonas aeruginosa bacteraemia isolates in a high-endemicity setting for ST175 and ST235 high-risk clones. Eur. J. Clin. Microbiol. Infect. Dis. 39, 671–678. doi: 10.1007/s10096-019-03780-z
Reig, S., Le Gouellec, A., and Bleves, S. (2022). What is new in the anti-Pseudomonas aeruginosa clinical development pipeline since the 2017 WHO alert? Front. Cell. Infect. Microbiol. 12:909731. doi: 10.3389/fcimb.2022.909731
Rezzoagli, C., Archetti, M., Mignot, I., Baumgartner, M., and Kümmerli, R. (2020). Combining antibiotics with antivirulence compounds can have synergistic effects and reverse selection for antibiotic resistance in Pseudomonas aeruginosa. PLoS Biol. 8:e3000805. doi: 10.1371/journal.pbio.3000805
Rhazi, N., Galleni, M., Page, M. I., and Frère, J. M. (1999). Peptidase activity of beta-lactamases. Biochem. J. 341, 409–413. doi: 10.1042/bj3410409
Rodríguez-Rojas, A., Maciá, M. D., Couce, A., Gómez, C., Castañeda-García, A., Oliver, A., et al. (2010). Assessing the emergence of resistance: the absence of biological cost in vivo may compromise fosfomycin treatments for P. aeruginosa infections. PLoS One. 2010 5:e10193. doi: 10.1371/journal.pone.0010193
Roux, D., Danilchanka, O., Guillard, T., Cattoir, V., Aschard, H., Fu, Y., et al. (2015). Fitness cost of antibiotic susceptibility during bacterial infection. Sci. Transl. Med. 7:297ra114. doi: 10.1126/scitranslmed.aab1621
Ruedas-López, A., Alonso-García, I., Lasarte-Monterrubio, C., Guijarro-Sánchez, P., Gato, E., Vázquez-Ucha, J. C., et al. (2022). Selection of AmpC β-lactamase variants and Metallo-β-lactamases leading to Ceftolozane/Tazobactam and ceftazidime/avibactam resistance during treatment of MDR/XDR Pseudomonas aeruginosa infections. Antimicrob. Agents Chemother. 66:e0206721. doi: 10.1128/AAC.02067-21
Rundell, E. A., Commodore, N., Goodman, A. L., and Kazmierczak, B. I. (2020). A screen for antibiotic resistance determinants reveals a fitness cost of the flagellum in Pseudomonas aeruginosa. J. Bacteriol. 202, e00682–e00619. doi: 10.1128/JB.00682-19
Sakhtah, H., Koyama, L., Zhang, Y., Morales, D. K., Fields, B. L., Price-Whelan, A., et al. (2016). The Pseudomonas aeruginosa efflux pump MexGHI-OpmD transports a natural phenazine that controls gene expression and biofilm development. Proc. Natl. Acad. Sci. U. S. A. 113, E3538–E3547. doi: 10.1073/pnas.1600424113
Sánchez, P., Linares, J. F., Ruiz-Díez, B., Campanario, E., Navas, A., Baquero, F., et al. (2002). Fitness of in vitro selected Pseudomonas aeruginosa nalB and nfxB multidrug resistant mutants. J. Antimicrob. Chemother. 50, 657–664. doi: 10.1093/jac/dkf185
Sánchez-Diener, I., Zamorano, L., López-Causapé, C., Cabot, G., Mulet, X., Peña, C., et al. (2017). Interplay among resistance profiles, high-risk clones, and virulence in the Caenorhabditis elegans Pseudomonas aeruginosa infection model. Antimicrob. Agents Chemother. 61, e01586–e01517. doi: 10.1128/AAC.01586-17
Sandoval-Motta, S., and Aldana, M. (2016). Adaptive resistance to antibiotics in bacteria: a systems biology perspective. Wiley Interdiscip. Rev. Syst. Biol. Med. 8, 253–267. doi: 10.1002/wsbm.1335
Sawa, T., Shimizu, M., Moriyama, K., and Wiener-Kronish, J. P. (2014). Association between Pseudomonas aeruginosa type III secretion, antibiotic resistance, and clinical outcome: a review. Crit. Care 18:668. doi: 10.1186/s13054-014-0668-9
Schroeder, M., Brooks, B. D., and Brooks, A. E. (2017). The complex relationship between virulence and antibiotic resistance. Genes (Basel) 8:39. doi: 10.3390/genes8010039
Schulz Zur Wiesch, P., Engelstädter, J., and Bonhoeffer, S. (2010). Compensation of fitness costs and reversibility of antibiotic resistance mutations. Antimicrob. Agents Chemother. 54, 2085–2095. doi: 10.1128/AAC.01460-09
Skurnik, D., Roux, D., Cattoir, V., Danilchanka, O., Lu, X., Yoder-Himes, D. R., et al. (2013). Enhanced in vivo fitness of carbapenem-resistant oprD mutants of Pseudomonas aeruginosa revealed through high-throughput sequencing. Proc. Natl. Acad. Sci. U. S. A. 110, 20747–20752. doi: 10.1073/pnas.1221552110
Souque, C., Escudero, J. A., and MacLean, R. C. (2023). Off-target integron activity leads to rapid plasmid compensatory evolution in response to antibiotic selection pressure. MBio 14:e0253722. doi: 10.1128/mbio.02537-22
Stewart, P. S., Franklin, M. J., Williamson, K. S., Folsom, J. P., Boegli, L., and James, G. A. (2015). Contribution of stress responses to antibiotic tolerance in Pseudomonas aeruginosa biofilms. Antimicrob. Agents Chemother. 59, 3838–3847. doi: 10.1128/AAC.00433-15
Stickland, H. G., Davenport, P. W., Lilley, K. S., Griffin, J. L., and Welch, M. (2010). Mutation of nfxB causes global changes in the physiology and metabolism of Pseudomonas aeruginosa. J. Proteome Res. 9, 2957–2967. doi: 10.1021/pr9011415
Strempel, N., Neidig, A., Nusser, M., Geffers, R., Vieillard, J., Lesouhaitier, O., et al. (2013). Human host defense peptide LL-37 stimulates virulence factor production and adaptive resistance in Pseudomonas aeruginosa. PLoS One 8:e82240. doi: 10.1371/journal.pone.0082240
Sun, Z., Jiao, X., Peng, Q., Jiang, F., Huang, Y., Zhang, J., et al. (2013). Antibiotic resistance in Pseudomonas aeruginosa is associated with decreased fitness. Cell. Physiol. Biochem. 31, 347–354. doi: 10.1159/000343372
Tian, Z. X., Fargier, E., Mac Aogáin, M., Adams, C., Wang, Y. P., and O’gara, F. (2009). Transcriptome profiling defines a novel regulon modulated by the LysR-type transcriptional regulator MexT in Pseudomonas aeruginosa. Nucleic Acids Res. 37, 7546–7559. doi: 10.1093/nar/gkp828
Torrens, G., Hernández, S. B., Ayala, J. A., Moya, B., Juan, C., Cava, F., et al. (2019a). Regulation of AmpC-driven β-lactam resistance in Pseudomonas aeruginosa: different pathways, different signaling. mSystems 4:e00524-19. doi: 10.1128/mSystems.00524-19
Torrens, G., Pérez-Gallego, M., Moya, B., Munar-Bestard, M., Zamorano, L., Cabot, G., et al. (2017). Targeting the permeability barrier and peptidoglycan recycling pathways to disarm Pseudomonas aeruginosa against the innate immune system. PLoS One 12:e0181932. doi: 10.1371/journal.pone.0181932
Torrens, G., Sánchez-Diener, I., Jordana-Lluch, E., Barceló, I. M., Zamorano, L., Juan, C., et al. (2019b). In vivo validation of peptidoglycan recycling as a target to disable AmpC-mediated resistance and reduce virulence enhancing the Cell-Wall-targeting immunity. J. Infect. Dis. 220, 1729–1737. doi: 10.1093/infdis/jiz377
Torrens, G., van der Schalk, T. E., Cortes-Lara, S., Timbermont, L., Del Barrio-Tofiño, E., Xavier, B. B., et al. (2022). Susceptibility profiles and resistance genomics of Pseudomonas aeruginosa isolates from European ICUs participating in the ASPIRE-ICU trial. J. Antimicrob. Chemother. 77, 1862–1872. doi: 10.1093/jac/dkac122
Tsang, J. (2017). Bacterial plasmid addiction systems and their implications for antibiotic drug development. Postdoc J. 5, 3–9. doi: 10.14304/SURYA.JPR.V5N5.2
Tu, Y., Wang, D., Zhu, Y., Li, J., Jiang, Y., Wu, W., et al. (2022). Emergence of a KPC-90 variant that confers resistance to ceftazidime-avibactam in an ST463 carbapenem-resistant Pseudomonas aeruginosa strain. Microbiol. Spectr. 10:e0186921. doi: 10.1128/spectrum.01869-21
Urbach, C., Fastrez, J., and Soumillion, P. (2008). A new family of cyanobacterial penicillin-binding proteins. A missing link in the evolution of class A beta-lactamases. J. Biol. Chem. 283, 32516–32526. doi: 10.1074/jbc.M805375200
Vaillancourt, M., Limsuwannarot, S. P., Bresee, C., Poopalarajah, R., and Jorth, P. (2021). Pseudomonas aeruginosa mexR and mexEF antibiotic efflux pump variants exhibit increased virulence. Antibiotics 10:1164. doi: 10.3390/antibiotics10101164
Vogwill, T., Kojadinovic, M., and MacLean, R. C. (2016). Epistasis between antibiotic resistance mutations and genetic background shape the fitness effect of resistance across species of Pseudomonas. Proc. Biol. Sci. 283:20160151. doi: 10.1098/rspb.2016.0151
Vogwill, T., and MacLean, R. C. (2015). The genetic basis of the fitness costs of antimicrobial resistance: a meta-analysis approach. Evol. Appl. 8, 284–295. doi: 10.1111/eva.12202
Wang, D., Seeve, C., Pierson, L. S. 3rd., and Pierson, E. A. (2013). Transcriptome profiling reveals links between ParS/ParR, MexEF-OprN, and quorum sensing in the regulation of adaptation and virulence in Pseudomonas aeruginosa. BMC Genomics 14:618. doi: 10.1186/1471-2164-14-618
Wong-Beringer, A., Wiener-Kronish, J., Lynch, S., and Flanagan, J. (2008). Comparison of type III secretion system virulence among fluoroquinolone-susceptible and -resistant clinical isolates of Pseudomonas aeruginosa. Clin. Microbiol. Infect. 14, 330–336. doi: 10.1111/j.1469-0691.2007.01939.x
Yahav, D., Giske, C. G., Grāmatniece, A., Abodakpi, H., Tam, V. H., and Leibovici, L. (2020). New β-lactam-β-lactamase inhibitor combinations. Clin. Microbiol. Rev. 34, e00115–e00120. doi: 10.1128/CMR.00115-20
Yang, L., Chen, L., Shen, L., Surette, M., and Duan, K. (2011). Inactivation of MuxABC-OpmB transporter system in Pseudomonas aeruginosa leads to increased ampicillin and carbenicillin resistance and decreased virulence. J. Microbiol. 49, 107–114. doi: 10.1007/s12275-011-0186-2
Yang, Q., Li, Y., Fang, L., Lei, T., Cai, H., Hua, X., et al. (2023). A novel KPC-113 variant conferring carbapenem and ceftazidime-avibactam resistance in a multidrug-resistant Pseudomonas aeruginosa isolate. Clin. Microbiol. Infect. 29, 387.e7–e14. doi: 10.1016/j.cmi.2022.10.013
Yoon, E. J., and Jeong, S. H. (2021). Mobile Carbapenemase Genes in Pseudomonas aeruginosa. Front. Microbiol. 12:614058. doi: 10.3389/fmicb.2021.614058
Keywords: Pseudomonas aeruginosa, antibiotic resistance, horizontal β-lactamases, AmpC, efflux pumps, fitness, virulence, biological cost
Citation: Jordana-Lluch E, Barceló IM, Escobar-Salom M, Estévez MA, Zamorano L, Gómez-Zorrilla S, Sendra E, Oliver A and Juan C (2023) The balance between antibiotic resistance and fitness/virulence in Pseudomonas aeruginosa: an update on basic knowledge and fundamental research. Front. Microbiol. 14:1270999. doi: 10.3389/fmicb.2023.1270999
Edited by:
Magdalena Popowska, University of Warsaw, PolandReviewed by:
Yujie Hu, China National Center for Food Safety Risk Assessment, ChinaHua Zhou, Zhejiang University, China
Copyright © 2023 Jordana-Lluch, Barceló, Escobar-Salom, Estévez, Zamorano, Gómez-Zorrilla, Sendra, Oliver and Juan. This is an open-access article distributed under the terms of the Creative Commons Attribution License (CC BY). The use, distribution or reproduction in other forums is permitted, provided the original author(s) and the copyright owner(s) are credited and that the original publication in this journal is cited, in accordance with accepted academic practice. No use, distribution or reproduction is permitted which does not comply with these terms.
*Correspondence: Elena Jordana-Lluch, ZWxlbmEuam9yZGFuYUBzc2liLmVz; Carlos Juan, Y2FybG9zLmp1YW5Ac3NpYi5lcw==
†These authors have contributed equally to this work