- 1Department of Food Biotechnology, Instituto de la Grasa (CSIC), Campus Universitario Pablo de Olavide, Seville, Spain
- 2Universidad Internacional de Valencia, Comunidad Valencia, Spain
Lactiplantibacillus pentosus (Lbp. pentosus) is a species of lactic acid bacteria with a great relevance during the table olive fermentation process, with ability to form non-pathogenic biofilms on olive epidermis. The objective of this work is to deepen into the genetic mechanisms of adaptation of Lpb. pentosus LPG1 during Spanish-style green table olive fermentations, as well as to obtain a better understanding of the mechanisms of adherence of this species to the fruit surface. For this purpose, we have carried out a transcriptomic analysis of the differential gene expression of this bacterium during 60 days of fermentation in both brine and biofilms ecosystems. In brines, it was noticed that a total of 235 genes from Lpb. pentosus LPG1 were differentially expressed during course of fermentation and grouped into 9 clusters according to time-course analysis. Transport and metabolism of carbohydrates and amino acids, energy production, lactic acid and exopolysaccharide synthesis genes increased their expression in the planktonic cells during course of fermentation. On the other hand, expression of genes associated to stress response, bacteriocin synthesis and membrane protein decreased. A total of 127 genes showed significant differential expression between Lpb. pentosus LPG1 planktonic (brine) and sessile (biofilms) cells at the end of fermentation process (60 days). Among the 64 upregulated genes in biofilms, we found genes involved in adhesion (strA), exopolysaccharide production (ywqD, ywqE, and wbnH), cell shape and elongation (MreB), and well as prophage excision. Deeping into the genetic bases of beneficial biofilm formation by Lpb. pentosus strains with probiotic potential will help to turn this fermented vegetable into a carrier of beneficial microorganisms to the final consumers.
1. Introduction
Table olive are one of the most important fermented vegetables in the Mediterranean basin, with an annual worldwide production of 2.8 million tonnes in the 2021/2022 season (International Olive Council, 2023). Lactic acid bacteria (LAB) play a crucial role during the fermentation process of Spanish-style green table olives, the most relevant type of table olive elaboration with approximately 50% of the market. They produce lactic acid after the consumption of sugars, lowering the pH to safe values (<4.2) which prevent the development of undesirable microorganisms and provides product stability (Hurtado et al., 2012). The predominant LAB species involved during the fermentation of lye-treated olives are Lactiplantibacillus pentosus (Lbp. pentosus) and Lactiplantibacillus plantarum (Lbp. plantarum; Garrido-Fernandez et al., 1997; Hurtado et al., 2012). In fact, starter cultures containing these species have been developed to ensure a safer food fermentation process (Ruiz-Barba et al., 1994; Rodriguez-Gomez et al., 2014).
Previous studies have demonstrated the ability of LAB species to form non-pathogenic biofilms on olive epidermis including more than 107 CFU/g after concluding fermentation process, albeit they are also present in brine during course of fermentation (107–109 CFU/mL) as planktonic cells (Nychas et al., 2002; Lavermicocca et al., 2005; Arroyo-López et al., 2012; Domínguez-Manzano et al., 2012). There are important differences between the behavior of bacterial cells during its planktonic or sessile state, although both forms are responsible for carrying out the table olive fermentation process. In biofilm, the microorganism are embedded within an extracellular polymeric substance produced by themselves, creating a robust attachment to solid surfaces which confer a great resistance to environmental factors (Davey and O’toole, 2000; Remis et al., 2010). The mechanisms underlying the adhesion of LAB to make these beneficial biofilms are multifaceted, yet they invariably entail a harmonious interplay between the hydrophilic nature of bacterial cell and biotic or abiotic surfaces (Faten et al., 2016). Although essential roles in biofilm formation are played by microbial structures like fimbriae, flagella, and pili, it’s noteworthy that environmental factors, including pH, temperature, exposure duration, and ionic influences, also exert a considerable impact on adhesion processes (Wimpenny, 2009; Domínguez-Manzano et al., 2012; Jahid and Ha, 2012). Biofilms established on the olive surface typically encompass a spectrum of LAB species, prominently Lbp. pentosus and Lbp. plantarum, alongside diverse yeast species such as Wickerhamomyces anomalus and Saccharomyces cerevisiae, among others. On the contrary, planktonic cells are designed to colonize new niches, and they have a lower chance of survival to the adverse condition (low pH, high salt concentration, presence of antimicrobial compounds, etc.) usually found during table olive fermentations (Hurtado et al., 2012; Hernández-Jiménez et al., 2013).
Lbp. pentosus LPG1 (hereinafter referred to as LPG1) is a microorganism isolated from the biofilms formed during table olive fermentations (Benítez-Cabello et al., 2019). LPG1 has shown remarkable technological features such as esterase and phytase activity, production of lactic acid, bacteriocin production, etc., (Benítez-Cabello et al., 2019). A recent genomic analysis of the LPG1 strain has revealed various genes involved in adhesion, biofilm formation, bacteriocin production, degradation of carbohydrates, and metabolism of phenolic compounds, among these important technological features (López-García et al., 2023a). This microorganism has also shown important potential probiotic features, proving to be an anti-inflammatory agent, reduce cholesterol levels, inhibit foodborne pathogens, and adhere to Caco−2 cells (Benítez-Cabello et al., 2019, 2020). Moreover, LPG1 has recently shown the capacity to modulate the intestinal microbiota of healthy individuals (López-García et al., 2023b).
In a food market dominated mainly by dairy-based probiotic products, the demand for plant-based alternatives has been growing, particularly among vegetarians and individuals with lactose intolerance. In addition to LPG1, other Lactiplantibacillus strains isolated from vegetable fermentations have been identified as microorganisms with probiotic potential (Abriouel et al., 2017; Huang et al., 2018; Kim et al., 2020). Thereby, table olives can act as a carrier of beneficial bacteria to consumers. However, it is necessary to deepen in the study of the genes and processes that lead to the formation of beneficial biofilms by Lpb. pentosus on olive epidermis.
In this aspect, the use of NGS techniques such as RNA-seq could be very useful in understanding the mechanism involved in biofilm formation. This technique provides a detailed view of the genes expressed at a specific moment. Consequently, it enables the identification and quantification of gene expression levels, facilitating comparisons between planktonic (brine) and sessile (biofilms) populations. Thus, numerous RNA-seq studies have been conducted to investigate the genes responsible for producing the biofilm ecosystem in pathogenic microorganisms (Charlebois et al., 2016; De Celis et al., 2022). However, few transcriptomic studies have focused on Lpb. pentosus or Lpb. plantarum and the formation of these non-pathogenic biofilms (Alonso García et al., 2021; Zhu et al., 2022). Therefore, an assessment of the genes involved in the biofilm process is necessary, particularly within a plant matrix such as table olive fermentations.
The objective of this study was to evaluate the differential gene expression of LPG1 during table olive fermentation, identifying the genes involved in its adhesion to the olive surface through transcriptomic analysis, mapping against the annotated LPG1 genome.
2. Materials and methods
2.1. Olive processing
Manzanilla olives were processed according to the Spanish-style during 2021/2022 season in the pilot plant of Instituto de la Grasa (CSIC, Seville, Spain). The fruits were treated with a 2.0% NaOH solution until 2/3 of the pulp was reached. Then, the fruits were washed with tap water for 3 h and placed into 250 mL ISO glass containers (180 gr fruits +136 mL brine). An 11% (w/v) NaCl brine was initially added to cover the olives. Subsequently, the containers were closed and pasteurized at 80°C for 10 min to remove the initial microbial load, and finally stored at 4°C for 7 days until the pH and salt levels were kept constant at 8.1 and 4.9%, respectively. A total of 18 olive containers were used in the present study.
2.2. Experimental design
A commercial lyophile of LPG1 (Oleica, Seville, Spain) was reconstituted by triplicate in 0.1% of sterile peptone water (0.1%, w/v) at a concentration of 8 log10 CFU/mL. After waiting for 1 h, 5 mL were collected from each individual reconstitution process and referenced as AP. Then, 15 pasteurized olive containers were individually inoculated with this reconstituted LPG1 inoculum at a theoretical inoculum concentration of 5 log10 CFU/mL. After 1 h, 10 mL of brine were collected from 3 inoculated olive containers and marked as sample t0. Then, the rest of inoculated (n = 12) and non-inoculated control (n = 3) olive containers were stored in the laboratory during 60 days at 37°C. Brine and fruit samples were collected during fermentation with sampling references t1 = 3 days, t2 = 10 days, t3 = 25 days, and t4 = 60 days. The olive containers were discarded once they were analyzed. All analytical were carried out by triplicate on the individual olive containers. Figure 1 shows a scheme of the experimental design followed in this work.
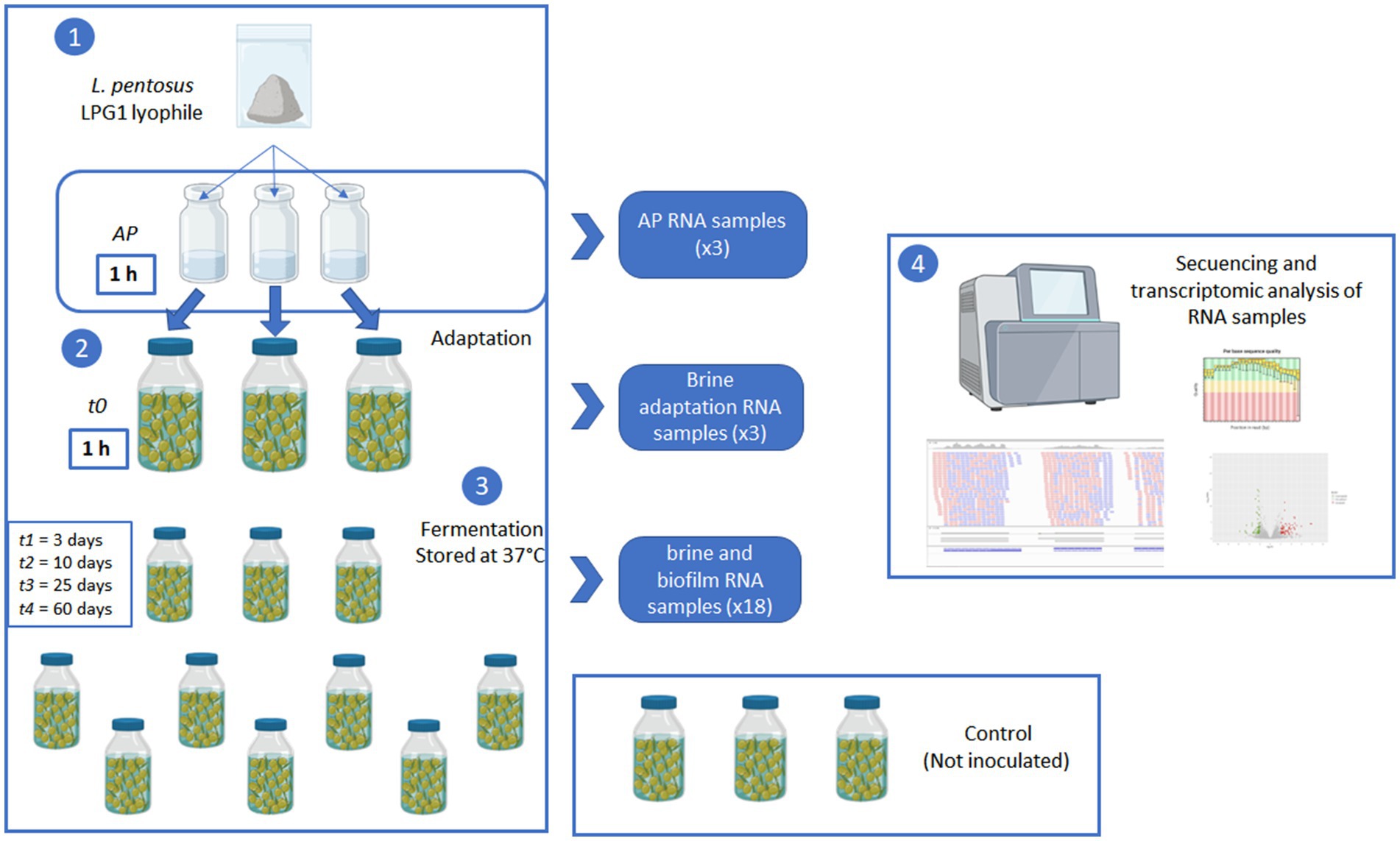
Figure 1. Scheme of the experimental design used in the transcriptomic analysis of LPG1 during Spanish-style green table olive fermentations.
2.3. Physic-chemical and microbiological analysis
At each sampling time, physic-chemical analysis were carried out following the methodology described by Garrido-Fernandez et al. (1997). The NaCl content (%), pH, and titratable acidity (expressed as g of lactic acid per 100 mL of brine) were determined in brine using an automatic titrator model Excellence (Mettler Toledo, Columbus, OH, United States). Samples of brines and fruits were also collected to determine LAB counts using specific MRS agar medium (Oxoid, Basingstoke, Hampshire, United Kingdom) according to methodology described by Benítez-Cabello et al. (2015). Briefly, fruits (10 g) were washed twice with a 0.9% sterile NaCl solution for removing non- and low-adherent cells, pitted, weighed in sterile conditions, and transferred into a stomacher bag containing 25 mL of sterile saline solution. Then, the fruits were homogenized for 3 min at 300 rpm in a stomacher model Seward 400 (Seward, United Kingdom). Decimal dilutions of brines or the stomacher bag liquid were spread onto culture media using a spiral platemaker model easySpiral Dilute (Interscience, Saint Nom la Brétèche, France). The counts were determined using an automatic image analysis system model Scan4000 (Interscience, SaintNom la Brétèche, France) and expressed as log10 CFU/mL (brine) or log10 CFU/g (biofilm), respectively.
2.4. RNA extraction, library preparation and sequencing
For each sampling time point (except t3), AP, brine, and biofilm samples were processed for RNA extraction. In the case of olives, prior to centrifugation, the cells adhered to the olive epidermis (non-pathogenic biofilms) were recovered using a stomacher model Seward 400 (Seward, United Kingdom) as described previously. Then, samples were centrifuged at 10.000 rpm during 10 min, and supernatants were carefully removed. In the case of brines, they were also centrifugated in the same conditions described above. Cell pellets were resuspended in 500 ul RNAlater solution and immediately stored at −80°C until further analysis. A total of 24 samples (AP-peptone water, t0-brine, t1-brine, t1-biofilm, t2-brine, t2-biofilm, t4-brine, and t4-biofilm) were selected for the RNA-seq experiment. Total RNA was extracted using the MasterPure Complete DNA and RNA Purification Kit (LGC Biosearch Technologies, United States) and subjected to DNAase treatment using Baseline Zero DNase (LGC Biosearch Technologies, United States). Once the RNA was extracted from each sample, its integrity was assessed with the Qubit™ RNA IQ kit, and its concentration was measured with the Qubit™ HS RNA kit. Libraries were prepared using the Illumina Stranded Total RNA Prep kit, quantified with the HS DNA Qubit™ kit (Q32851), and then loaded onto the NextSeq500 for sequencing. Sequencing generated 75-bp paired-end sequences. All raw RNA-Seq data have been deposited in ENA repository under PRJEB63117 accession number.
2.5. Transcriptomic analysis
Raw reads quality evaluation was performed using the fastp tool (Chen et al., 2018). The mapping of curated reads against the LPG1 genome (accession number PRJEB51357) was carried out using Hisat2 version 2.2.1 (Kim et al., 2015). Alignment parameters included the use of concordant pairs of reads only in the forward-reverse orientation, trimming of 0 bases from the 3′ and 5′ ends, skipping the first N reads or pairs in the input as 0, and applying a penalty equal to 1. The quality alignment was measured with Qualimap version 2.2.2d (Okonechnikov et al., 2016). Subsequently, the quantification of mapped transcript in the genome was performed with StrignTie version 2.2.1 (Pertea et al., 2015). The analysis of the differential gene expression was conducted using the Bioconductor package DESeq2 version 1.38.3 in the R statistical software program (Love et al., 2014). A principal component analysis (PCA) was carried out to obtain a broader approach to transcriptomic analysis. Subsequently, the focus was directed to each individual gene. Genes were considered differentially expressed when the Benjamini and Hochberg multiple correction method, commonly known as adjusted value of p or false discovery rate (FDR), was less than 0.05, and the Log2 fold change (Log2FC) was greater than 2 or less than −2. Moreover, Clusters of Orthologous Genes (COG) enrichment analysis was performed to compare the non-pathogenic biofilm and brine samples.
Due to the exclusion of the samples t1-biofilm and t2-biofilm (quality control failure), the analysis of differentially expressed genes over time was performed only with brine samples. The time-course analysis was conducted using Bioconductor package maSigPro version 1.70.0 (Conesa et al., 2006). This package fitted a regression model for each gene and returned a list of false discovery rate (FDR) corrected significant genes. An FDR < 0.05 was required to consider a gene as differentially expressed, and the default value 0.6 was used for the regression model R-squared cut-off value.
3. Results and discussion
3.1. Evolution of fermentation process
All inoculated olive containers followed the usual fermentation process described for this type of table olive elaboration (Garrido-Fernandez et al., 1997). Thereby, the average pH decreased from initial 8.1 to a value close to 4.3 after 10 days of fermentation, and finally reached a value of 4.0 from 25 days onward (Figure 2). The titratable acidity increased from 0.02% at the start of the experiment to final 1.03% due to the fermentation activity of LPG1. On the contrary, the salt levels were kept constant at 4.9% until the end of the experiment. The LPG1 population grew in both brine and biofilm ecosystems, reaching maximum levels in brines at 10 days of fermentation (9 log10 CFU/mL) and in non-pathogenic biofilms at 15 days of fermentation (8 log10 CFU/g). However, after this moment, the population decreased obtaining an average population of 5.8 log10 CFU/g in the mature biofilms at the end of fermentation (60 days), whereas the counts in brine had a higher decline up to 5 log10 CFU/mL (Figure 2). Thereby, planktonic LPG1 cells were less resistant to the adverse conditions present during table olive fermentations than those forming biofilms, as described previously by Hernández-Jiménez et al. (2013). In the non-inoculated olive containers, it was not noticed any development of LAB and the pH and salt concentration were kept constant during 60 days of fermentation at 5.64 and 5.1%, respectively. This was indicative that no lactic acid fermentation took place in the control olive containers.
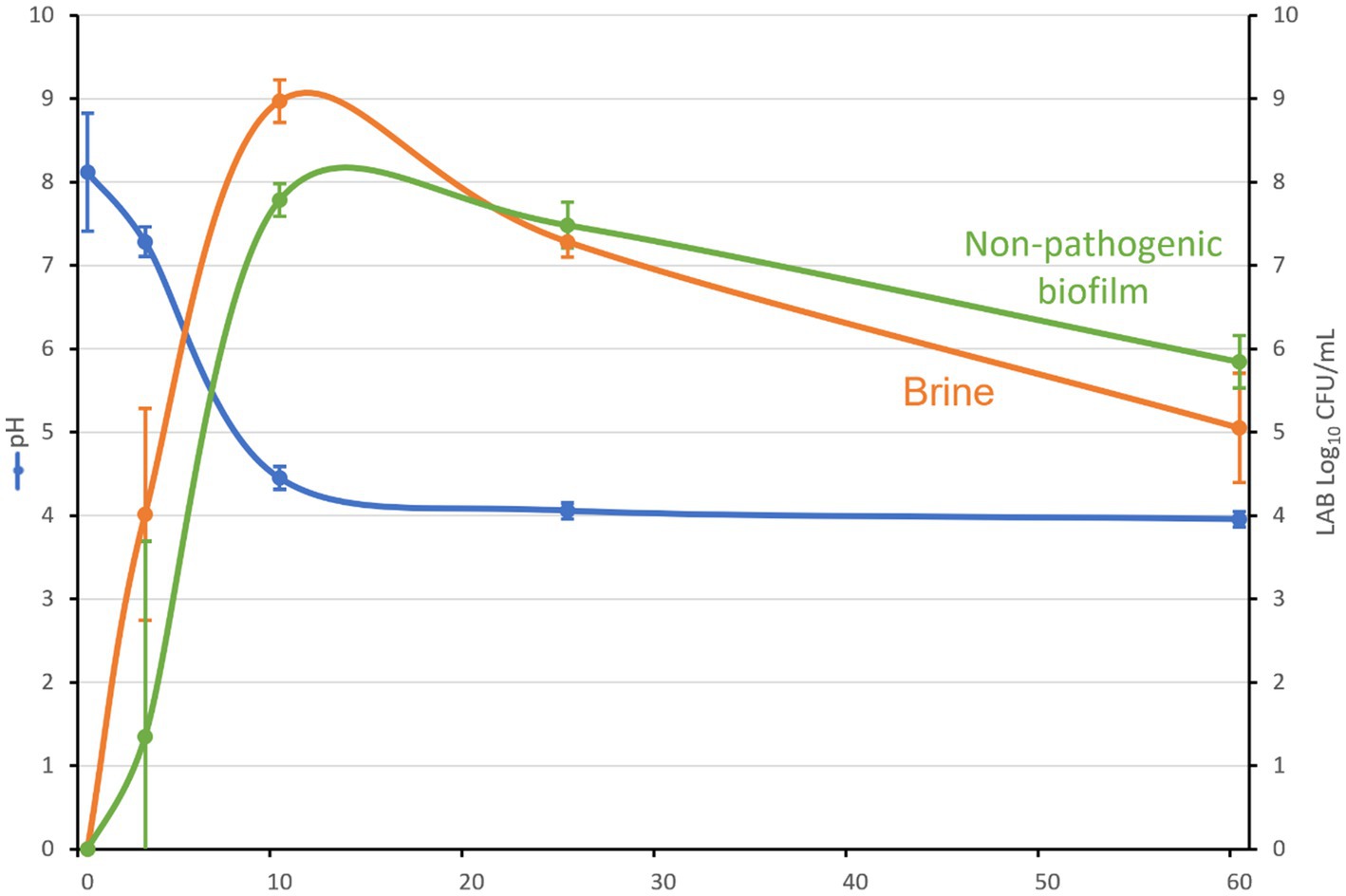
Figure 2. pH evolution, LAB counts in brine (log10 CFU/mL) and non-pathogenic biofilms (log10 CFU/g) during Spanish-style green table olive fermentation.
3.2. Overview of transcriptomic analysis
In this work, a total of 27 GB raw data were generated by the 24 samples during RNA-seq analysis. Table 1 shows the total raw reads obtained for each sample, the total number of cleaned reads, and the ratio of reads that passed the filter quality. Most of the samples achieved 10 million reads, with some cases exceeding 30 million reads per sample. Thereby, a total of over 400 million reads were obtained, with up to 375 million passing the quality filters. One replicate of t1-biofilm and two replicates of t2-biofilm lost a large number of reads during quality control, and for this reason, they were removed from the final analysis.
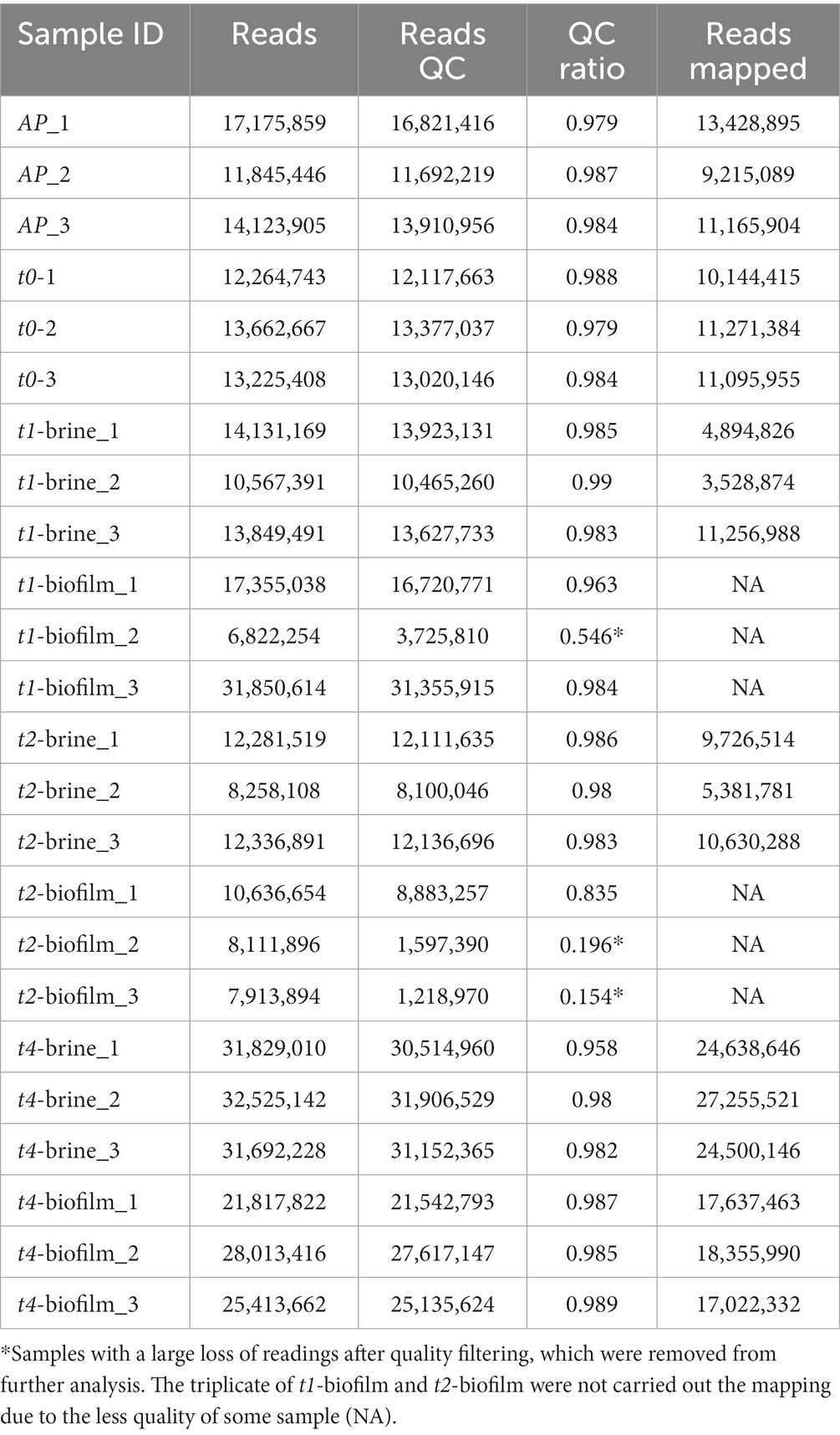
Table 1. Reference of samples and total number of reads generated during transcriptomic analysis of LPG1 [before and after quality control (QC) filtering], as well as total number of mapped reads against the LPG1reference genome.
The LPG1 annotated genome was used for mapping. The genome of LPG1 has a length of 3,700,533 bp distributed among one chromosome and two plasmids, which contain a total of 3,345 protein-coding genes and 89 non-coding sequences (73 tRNA and 16 rRNA genes), with a G + C content of 46.34% (López-García et al., 2023a). Regarding mapping quality, the mean coverage of the samples was more than 300%, and the mean mapping quality of the samples reached a value of 58, with a mean insert size of 154 bp.
PCA has proved to be a useful statistical tool in numerous RNA-seq studies to initially visualize the clustering of samples in the space. In addition, PCA can help to identify genes responsible for the observed deviations between samples (Wold et al., 1987; Curiel et al., 2017). The first two principal component of the PCA explained a total of the 80% variance of the samples (Figure 3). According to PCA, samples were grouped in 3 regions. The samples from AP, t0, and t1-brine were very similar among them, and they were grouped in the negative part of PC1. In contrast, t4-biofilm samples were located in the positive part of PC1 and PC2, while t2 and t4-brine samples were mainly located in the positive part of PC1 and negative part of PC2. Thus, this preliminary analysis clearly reported a differentially gene expression between non-pathogenic biofilm and brine samples at the end of fermentation (t4), and between brine samples from beginning (AP, t0, and t1) and middle-final points (t2 and t4) of fermentation, when low pHs and high titratable acidity levels were obtained. Similar results have been reported in previous studies, where different microbial responses were successfully grouped by means of PCA analysis (Curiel et al., 2017; Zhu et al., 2022).
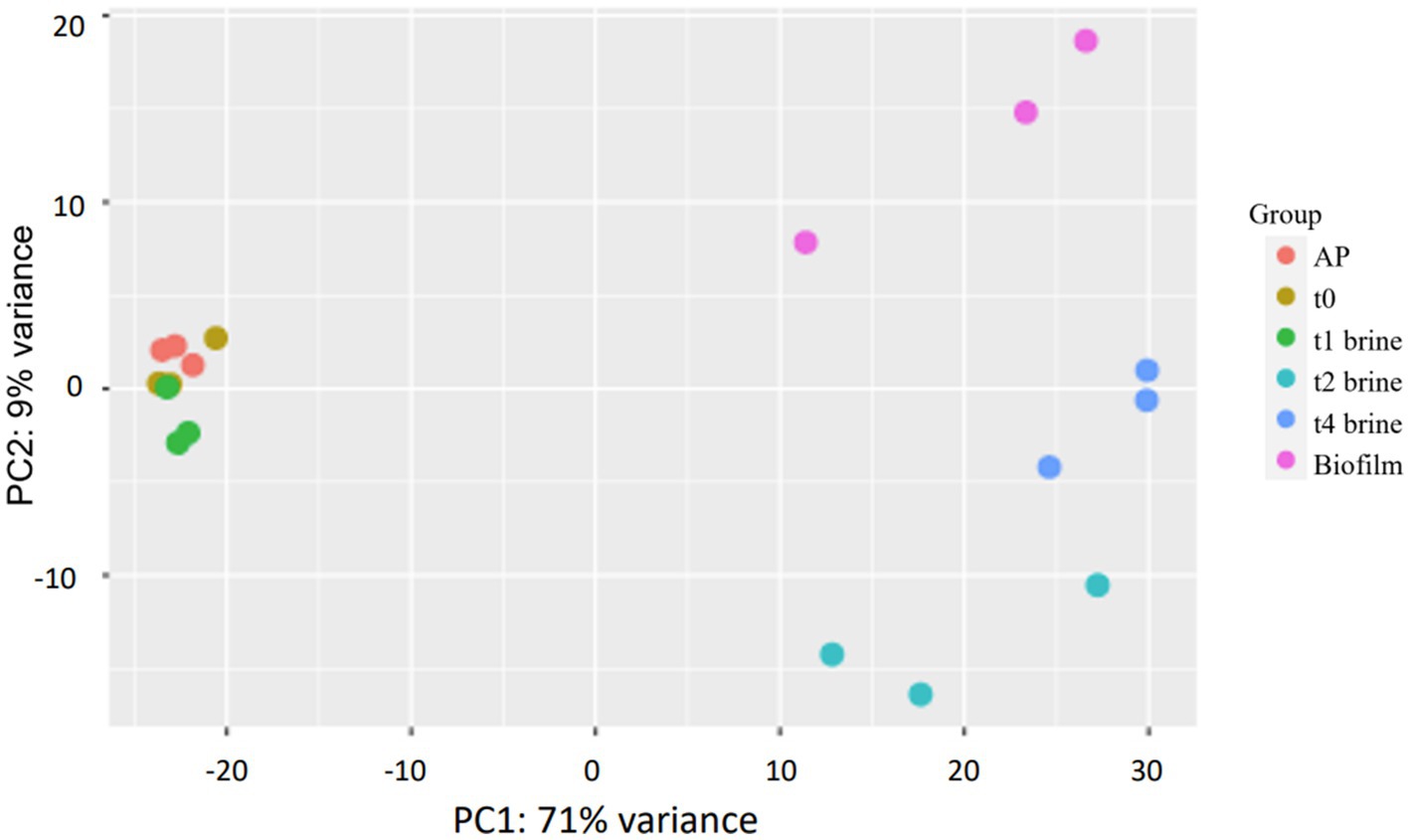
Figure 3. Principal component analysis (PCA) for the 21 samples analyzed which passed quality control. AP (lyophiles reconstituted in peptone water 0.1%), t0 (inoculated samples in brine collected after 1 h), t1-brine (brine sample at 3 days of fermentation), t2-brine (brine sample at 10 days of fermentation), t4-brine (brine sample at 60 days of fermentation), and Biofilm (non-pathogenic biofilm samples at t4-60 days of fermentation).
3.3. Short-term adaptation of LPG1 planktonic cells to olive brine
Microorganisms involved in the Spanish-style fermentation process must be capable of fermenting the carbohydrates present in the olive brine (mainly glucose, fructose, saccharose, and mannitol), tolerating a certain salt concentration (6–8% NaCl), growing in the presence of specific phenolic antimicrobial compounds (oleuropein, hydroxytirosol, tyrosol, etc.), and producing a significant amount of lactic acid (Garrido-Fernandez et al., 1997; Medina et al., 2010). As mentioned before, Lpb. pentosus and Lpb. plantarum are the most abundant LAB species in table olive fermentations (Hurtado et al., 2012). Both species have a remarkable ability to colonize diverse environments due to their enzymatic machinery encoded by relatively large genomes ranging from 3.2 to 4.2 Mb (O’Sullivan et al., 2009; Inglin et al., 2018). Thus, adaptation to the stressing conditions of olive brines is a crucial step for the successful fermentation carried out by Lpb. pentosus.
As described above, according to the PCA analysis, no great differences were observed between the samples from AP and t0-brine. In fact, a differential gene expression analysis yielded a total of 1 upregulated gene and 2 downregulated genes. Therefore, the time elapsed (only 1 h) between the AP and t0-brine samples was not adequate to observe differentially significant expressions. Thus, t1-brine sample (3 days) was chosen to observe the adaptation of LPG1 planktonic cells to olive brine. Figure 4 shows the enrichment of COG categories where the differentially expressed genes were classified. A total of 18 genes were upregulated in t1-brine compared to AP, while 50 genes were downregulated (Supplementary Table S1). At 3 days of fermentation, LPG1 genes involved in the formation of extracellular exopolysaccharide (EPS) were found upregulated. Moreover, the LOPJBOPB 01174 gene, encoding the polysaccharide polymerase protein located in an EPS production cluster, was upregulated. This membrane protein, containing up to 10 transmembrane domains, is responsible for polysaccharide polymerization (Deo et al., 2019). Additionally, the wbbI gene encoding beta-1,6-galactofuranosyltransferase was also found upregulated. The product of the wbbI gene is an enzyme responsible for adding a galactofuranose residue at a specific position of a specific substrate. This addition of the galactofuranose residue contributes to the formation and stability of the bacterial cell wall, as well as resistance to environmental factors (Wing et al., 2006). Therefore, in the early stages of fermentation, the LPG1 planktonic cells increased the expression of genes involved in cellular resilience. It has been described as cell surface modeling acts as an adaptive mechanism for different species in stressful or hostile environments (Martinez et al., 2020). On the other hand, genes involved in sugar transport, amino acid metabolism, and translation were also found upregulated. Although, differential expression of certain genes known to be essential for fermentation adaptation was not observed, numerous transcripts for these genes were detected (Perpetuini et al., 2016). All the genes classified as essential were found to be expressed to some extent. These genes include eno_1, rex, mroQ, degV_1, LOPJBOPB_01576, LOPJBOPB_01576, and pgi, which encode for the enolase protein, redox sensing transcriptional repressor Rex, membrane-embedded CAAX protease MroQ, DegV protein, integral membrane protein, transcription regulator TetR, and glucose 6-phosphate isomerase, respectively.
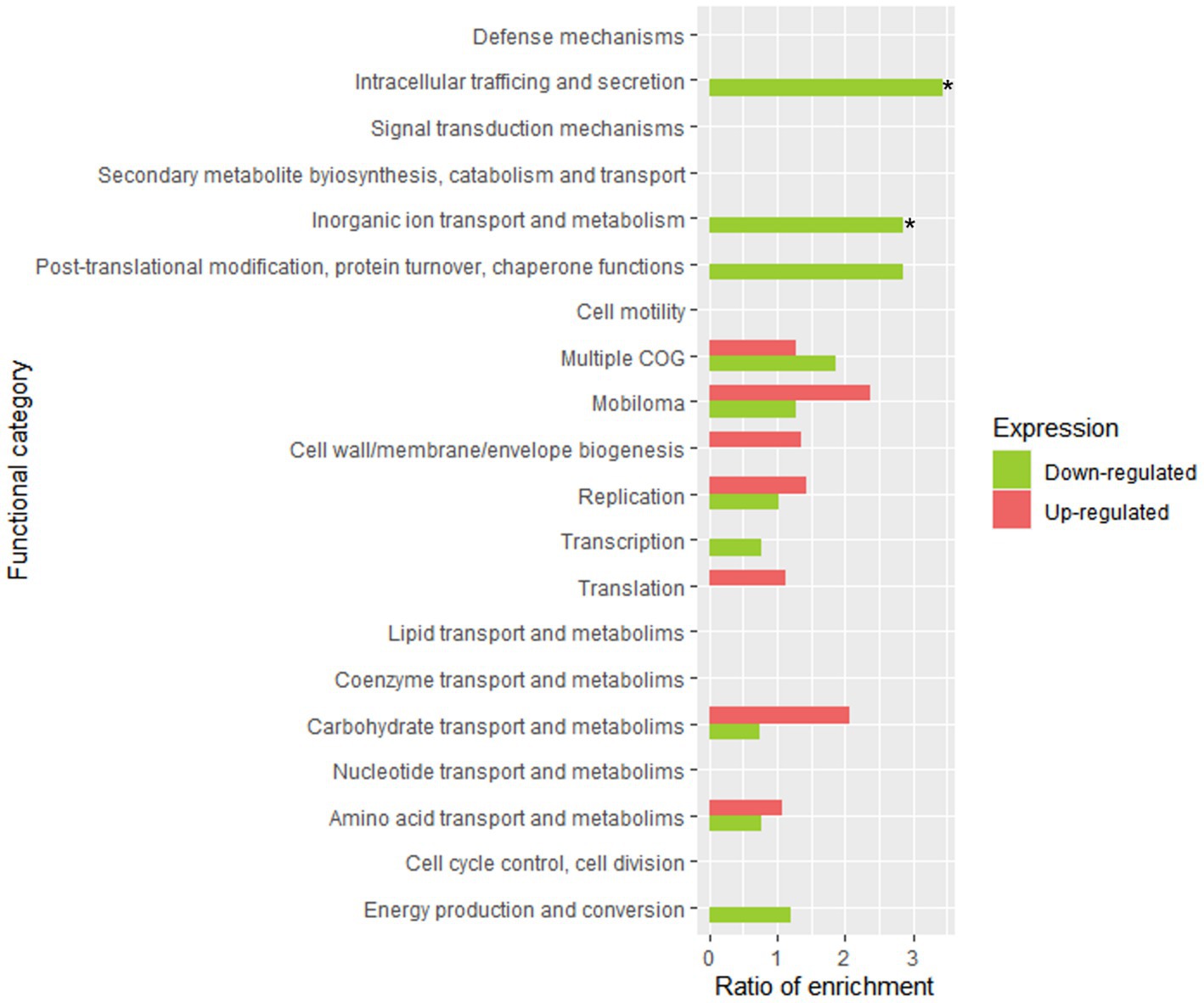
Figure 4. Functional enrichment analysis of clustered genes orthologous (COG) in LPG1 genes in adaptation to brine. The enrichment ratio was calculated as the percentage of genes within a specific functional category in the upregulated or downregulated RNA-seq dataset, relative to the percentage of genes assigned to that functional category in LPG1 genome. * Significant COG categories enrichment amongst genes.
In contrast, the response to oxidative stress was decreased at 3 days of fermentation, as the genes msrB (peptide methionine sulfoxide reductase MrsB), garB_2 (glutathione amide reductase), and perR (peroxide operon regulator) were downregulated. These gene products are related to the response to oxidative stress. Glutathione amide reductase helps to maintain the redox balance in cells by participating in the regeneration of reduced glutathione, which is an important antioxidant molecule involved in detoxification processes and protection against oxidative stress (Pophaly et al., 2012). MrsB protein reduces methionine sulfoxide residues (oxidized methionine) in proteins, restoring their functionality (Grimaud et al., 2001). Finally, the protein encoded by perR gene acts as a transcriptional repressor that controls the expression of genes involved in the response to oxidative stress (Mongkolsuk and Helmann, 2002). Thereby, all these downregulated proteins prevent the accumulation of oxidative compound in the cell and contribute to cellular redox homeostasis. Downregulation of these genes may be due to the low oxidative stress and the cell repressing this expression to conserve energy. Similarly, genes encoding proteins involved in resistance to thermic, oxidative, osmotic, or toxic stress, such as LOPJBOPB 03141 (belongs to the small heat shock protein (HSP20) family), spxA (regulatory protein Spx), gla (glycerol facilitator-aquaporin gla), and LOPJBOPB 03204 (belongs to the dps family), were found to be downregulated. The results obtained show that after 3 days of fermentation, the cellular stress of the LPG1 strain is lower than after its reconstitution in peptone water, as numerous stress-responsive genes were found to be downregulated, which is indicative that the bacteria is already well adapted to the olive fermentation conditions.
3.4. LPG1 planktonic cell gene expression during fermentation
Throughout 60 days of fermentation, a total of 235 genes from LPG1 planktonic cells were significantly differentially expressed in brine (Supplementary Table S2). These genes were grouped into 9 clusters according to time-course analysis (Figure 5).
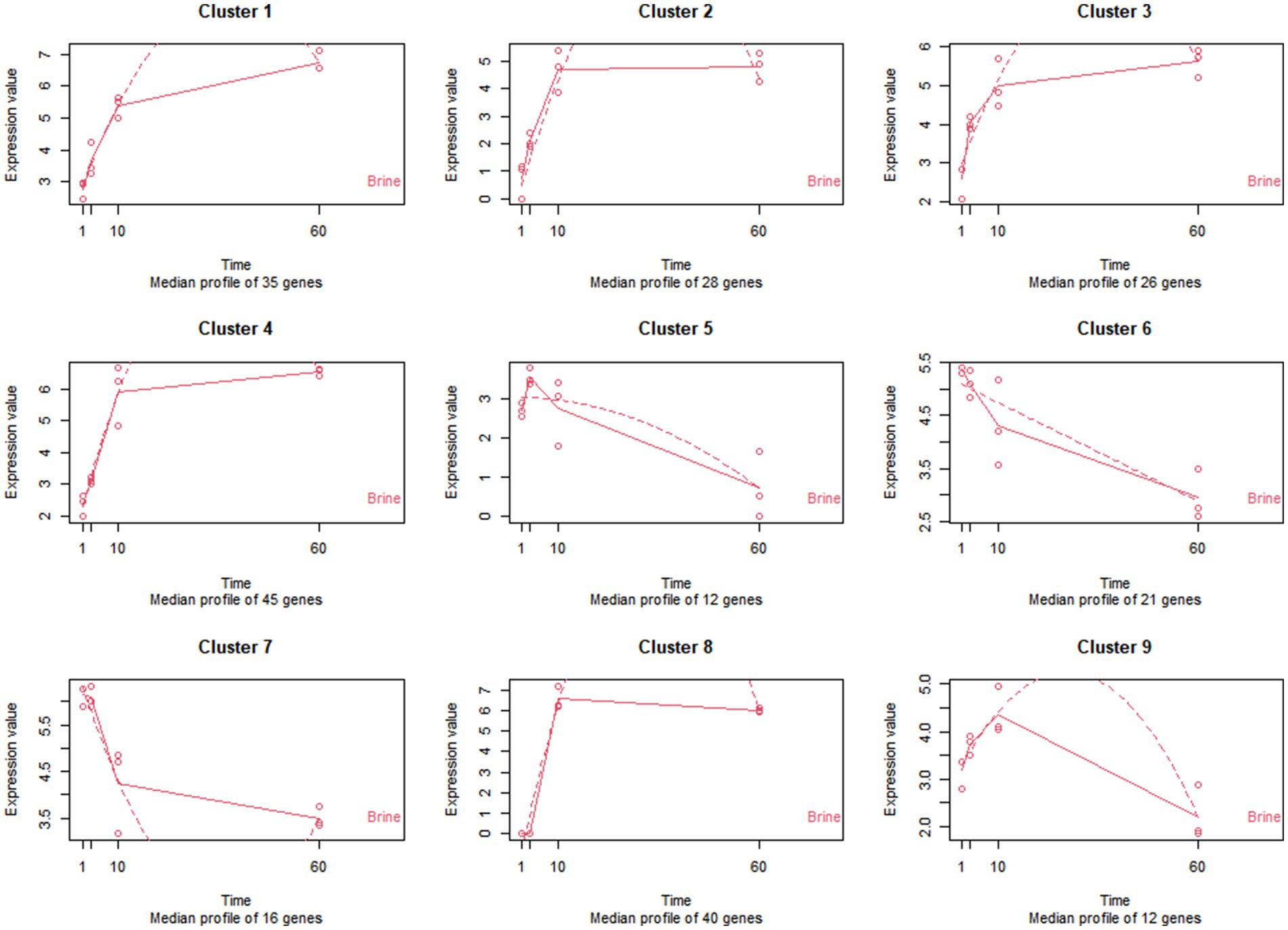
Figure 5. Patterns of expression of the 9 clusters of genes identified in the time-course analysis of LPG1 strain in brine. The expression values are expressed in TPM (transcripts per million). The solid line represents the actual values, and the dashed line represents the regression curve.
Genes grouped in clusters 1 to 4 had an increase in expression during 60 days of fermentation. Transport and metabolism of carbohydrates and amino acids, energy production, lactic acid and EPS synthesis were the prominent functions observed in gene clusters 1 to 4. The exponential growth of the LPG1 population in brine could be mainly due to the utilization of a wide variety of sugars present in the brine, such as sucrose, glucose, fructose, and mannitol, and the ability of the LPG1 strain to hydrolyze them. According to the time-course analysis, cluster 4 exhibited higher gene expression than the other clusters at 10 days. In cluster 1 and 4, genes encoding key enzymes were found for sucrose hydrolysis, such as oligo-1,6-glucosidase and sucrose-6-phosphate hydrolase, respectively. Similar results have been observed in the carbohydrate metabolism of Lpb. pentosus during adaptation in olive oil (Alonso García et al., 2021). Additionally, gene encoding the enzyme mannitol-1-phosphate 5-dehydrogenase responsible for mannitol hydrolysis was also found in this cluster. Furthermore, the gene encoding mannitol-specific phosphotransferase enzyme IIA component, involved in mannitol transport into the cell, was present in cluster 4. Also present in this cluster was the gene encoding the fructokinase enzyme responsible for the phosphorylation of fructose for subsequent utilization in glycolysis. On the other hand, cluster 1 showed higher expression than cluster 4 at the end of fermentation. Within cluster 1, several genes involved in glucose metabolism were found, including gdh encoding glucose 1-dehydrogenase, which catalyzes the oxidation of glucose to glucose-6-phosphate. This glucose-6-phosphate can be subsequently utilized for energy production through glycolysis. Additionally, the glcU gene (glucose uptake protein), responsible for transporting glucose across the membrane, was also present in cluster 1. Several genes involved in carbohydrate metabolism were found in these 4 clusters, more specifically in galactose metabolism, such as genes implicated in synthesis (galT), transport (lacS, agaC, and gatC) and degradation (lacL and lacM). The presence of galactose, which is a sugar not commonly associated with the fermentation of table olives, suggests that LPG1 strain could has the ability to produce this carbohydrate. In fact, within these four clusters, various genes were found to be differentially expressed over time, indicating their involvement in carbohydrate biosynthesis and extracellular translocation. Specifically, genes such as glgE (Alpha-1,4-glucan:maltose-1-phosphate maltosyltransferase), fba (Fructose-bisphosphate aldolase), LOPJBOPB_01767, galT (galactose-1-phosphate uridylyltransferase), and ppsA (phosphoenolpyruvate synthase) were implicated in anabolic processes related to carbohydrate metabolism. Conversely, LOPJBOPB_02006 (oligosaccharide flippase family protein) gene was associated with the transport of carbohydrates to the extracellular space, contributing to the formation of the EPS. EPS, composed of carbohydrates, proteins, nucleic acid, and lipid, primarily acts as a protective barrier against external agents. Moreover, EPS plays a critical role in biofilm formation and maturation (Dertli et al., 2015). However, under nutrient-limited conditions, bacteria can use as fermentable substrate the EPS previously produced (Rios-Covian et al., 2016). It is noteworthy that lactic acid production carried out by ldhD (D-lactate dehydrogenase) gene included in cluster 2 is essential for lowering pH during fermentation.
On the contrary, genes of cluster 5 showed a pattern of increase their expression up to 3 days and then decrease until the end of fermentation. Within this cluster, dps gene encoding a DNA protection during starvation protein stands out. The function of this protein is to protect and preserve DNA integrity during adverse conditions. Therefore, when LPG1 was under unfavorable conditions the expression of the dps gene increased and when the pH started to decrease in brine the expression of the dps gene decreased. Previous studies have shown the rapid degradation of the protein encoded by the dps gene during the exponential growth phase (Stephani et al., 2003). In addition, the lexA gene coding for the LexA repressor maintained the expression of cluster 5. This repressor is responsible for inhibiting the SOS response network that activates in response to DNA damage. However, LexA repressor also affects the expression of genes involved in other functions. In previous transcriptomic studies, the lexA gene was found to be downregulated during biofilm formation process (Philips et al., 2017).
Genes of clusters 6 and 7 displayed a decrease expression over time. The primary functions associated with genes of clusters 6 and 7 were stress response, bacteriocin synthesis and membrane protein expression. Up to two annotated bacteriocin immunity genes were downregulated over time as shown the cluster 6, and a bacteriocin gene called LOPJBOPB 00365 was located into plantaricin bacteriocin cluster. Previous studies of bacteriocin production modeling reported an increase in bacteriocin production during exponential cell growth. Furthermore, after 50 h of cultivation, bacteriocin production decreased, which is consistent with the behavior observed for LPG1 in brine (Zhou et al., 2015). On the other hand, perR gene which encodes to peroxide operon regulator was also included in cluster 6. When LPG1 faces oxidative stress, such as the onset of fermentation, the peroxide operon increased its expression. Thus, as fermentation progresses, the oxidative stress decreases and consequently the expression of perR. Most of the genes included in cluster 7 were assigned to hypothetical proteins, after a homology search, the proteins encoded by these genes were identified as cell surface proteins. Cell surface remodeling occurs through the plasticity of the cell wall, and the expression of various surface proteins is a common phenomenon throughout the cellular life, primarily influenced by environmental conditions and the specific niche (Martinez et al., 2020).
Finally, genes from clusters 8 and 9 increased their expression up to 10 days and subsequently experienced a decrease throughout the fermentation process, with cluster 8 showing only a slight decrease. Genes involved in the transport and metabolism of amino acids, as well as those related to transcriptional regulation and membrane protein expression, were classified within these clusters.
3.5. Comparison of gene expression between sessile and planktonic LPG1 cells at the end of fermentation
During the fermentation of table olives, LAB exhibit progressively a strong adherence to the olive skin, forming a non-pathogenic biofilm on the fruit. This holds great importance given the probiotic potential presented by this specific strain of Lpb. pentosus (Benítez-Cabello et al., 2019, 2020; López-García et al., 2023b). Therefore, LPG1 needs to express genes encoding surface adhesion proteins, as observed in previous studies on diverse Lpb. plantarum and Lpb. pentosus strains (Ao et al., 2020; Sun et al., 2020). A total of 127 genes showed significant differential expression between non-pathogenic biofilms (sessile) and brines (planktonic cells) at the end of fermentation process (t4–60 days), with 64 genes upregulated and 63 genes downregulated, using the aforementioned threshold (Supplementary Table S3). Surprisingly, none of these genes were related to the quorum sensing phenomena described previously for diverse LAB species, with no changes in the levels of expression of histidine protein kinases or luxS genes (Man and Xiang, 2021).
Figure 6 displays the statistical significance (− Log10 FDR) and fold change (Log2 FC) of the analyzed genes. The number of significantly differentially expressed genes was lower than in other transcriptomic studies of Lpb. plantarum and Lpb. pentosus (Ao et al., 2020; Sun et al., 2020; Alonso García et al., 2021). However, this was likely due to the highly restrictive thresholds used in the present study, as the decision was made to consider genes that were four times more/less expressed (Log2FC = |2|). This approach allowed for a more confident assumption of differential expression. Enrichment analysis of COG categories was conducted to compare the enriched functional categories in the non-pathogenic biofilm and brine of LPG1 populations (Figure 7). Up to 4 COG categories were significantly enriched in non-pathogenic biofilms. The categories of cell motility, secondary metabolite biosynthesis, catabolism/transport, and cell cycle control/division were significantly enriched due to the upregulated genes in these categories. Conversely, the amino acid transport and metabolism category was significantly enriched due to the downregulated genes within this category.
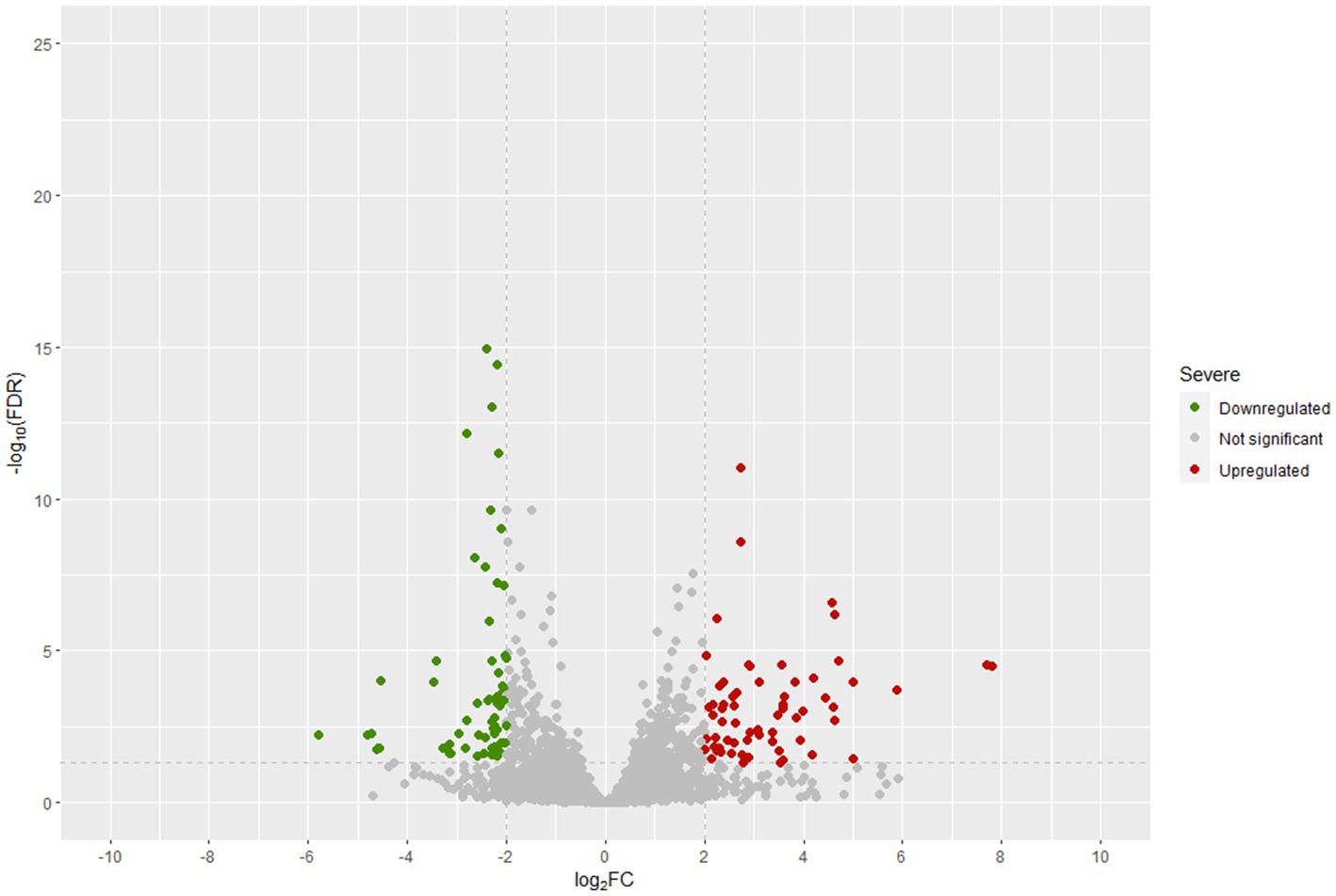
Figure 6. Differentially expressed genes (DEGs) in t4-biofilm samples compared to t4-brines samples at 60 days of fermentation.
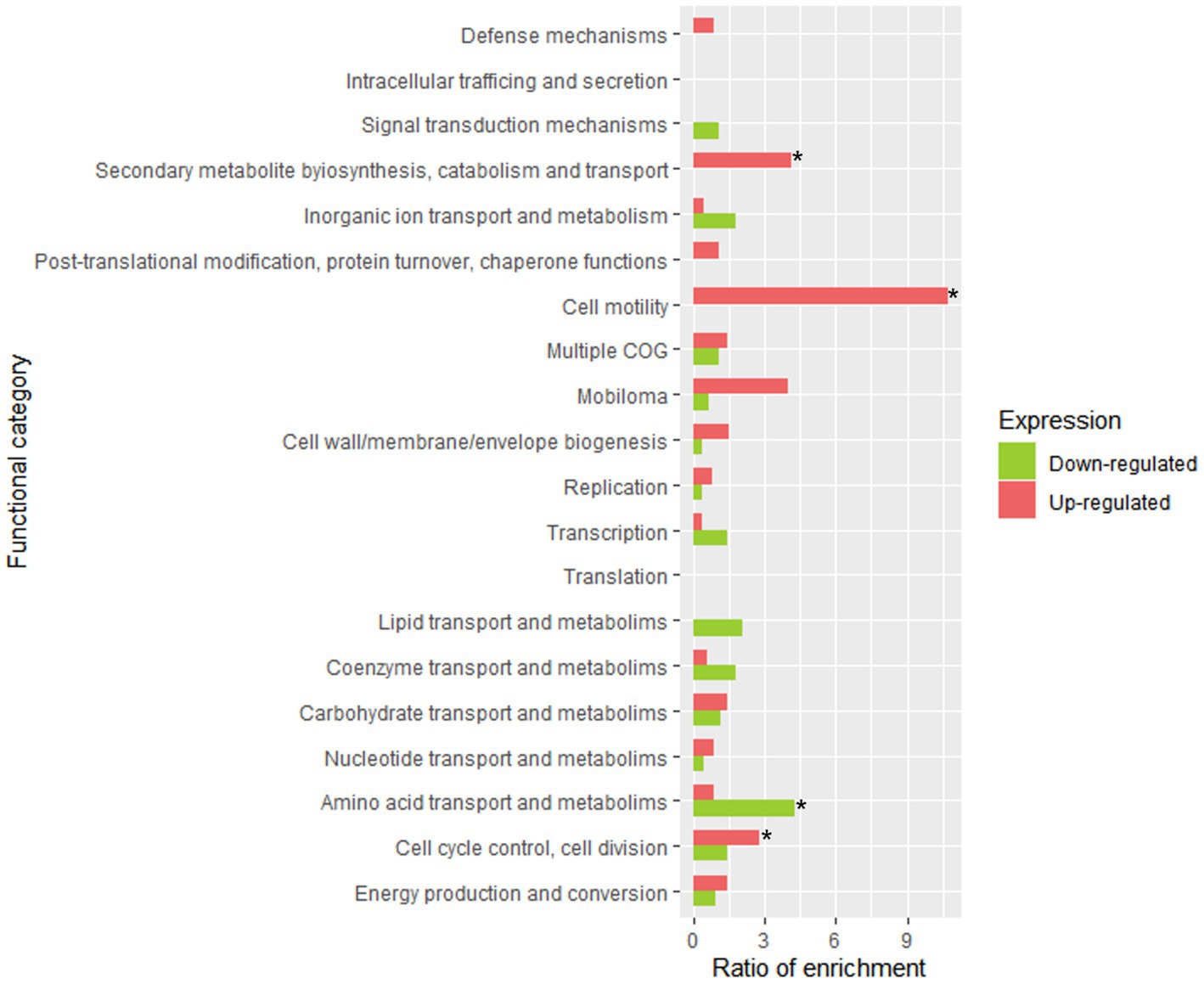
Figure 7. Functional enrichment analysis of clustered genes orthologous (COG) in LPG1 non-pathogenic biofilm (t4-biofilm) at 60 days of fermentation. The enrichment ratio was calculated as the percentage of genes within a specific functional category in the upregulated or downregulated RNA-seq dataset, relative to the percentage of genes assigned to that functional category in LPG1 genome. * Significant COG categories enrichment amongst genes.
Table 2 summarized the genes included in the four significantly enriched COG categories. Among the upregulated genes in sessile cells, several genes involved in adhesion were identified based on the literature. The strA gene encoding sortase A protein, which has been associated with adhesion to epithelial cells, exhibited significant upregulation in the non-pathogenic biofilm samples, indicating its key role in adhesion to the surface of fruit during the fermentation process by LPG1 strain (Lalioui et al., 2005). Additionally, genes involved in non-pathogenic biofilm formation were detected, including three upregulated genes belonging to an EPS production cluster: ywqD (tyrosine-protein kinase), ywqE (tyrosine-protein phosphatase), and wbnH (O-antigen biosynthesis glycosyltransferase). EPS plays a fundamental role in the formation, stability, and functionality of the biofilm, and a decrease in EPS production resulted in reduced biofilm formation in Pseudomonas aeruginosa (De Celis et al., 2022). On the other hand, the lrgB gene, encoding the Antiholin-like protein LrgB, is part of the well-known LrgAB system into lrg operon. The lrgA and lrgB genes are annotated in the database as a regulator and effector of murein hydrolase, respectively. Previous studies have shown that mutations in the LrgAB system affect various cellular functions, including autolysis, biofilm formation, and response to oxidative stress (Ahn et al., 2010). Streptococcus mutans lrgB mutants exhibited decreased biofilm-forming capacity and increased autolysis compared to the wild-type strain (Rice et al., 2017). However, Bacillus cereus and Staphylococcus aureus lrgB mutants showed increased biofilm formation compared to the wild-type strain. The release of extracellular genomic DNA (eDNA) through cell lysis by lrgB mutant strains promoted biofilm development (Mann et al., 2009; Zhang et al., 2020). Thus, the overexpression of lrgB in LPG1 may act by promoting autolysis and enhancing the stability and cohesion of the biofilm through eDNA.
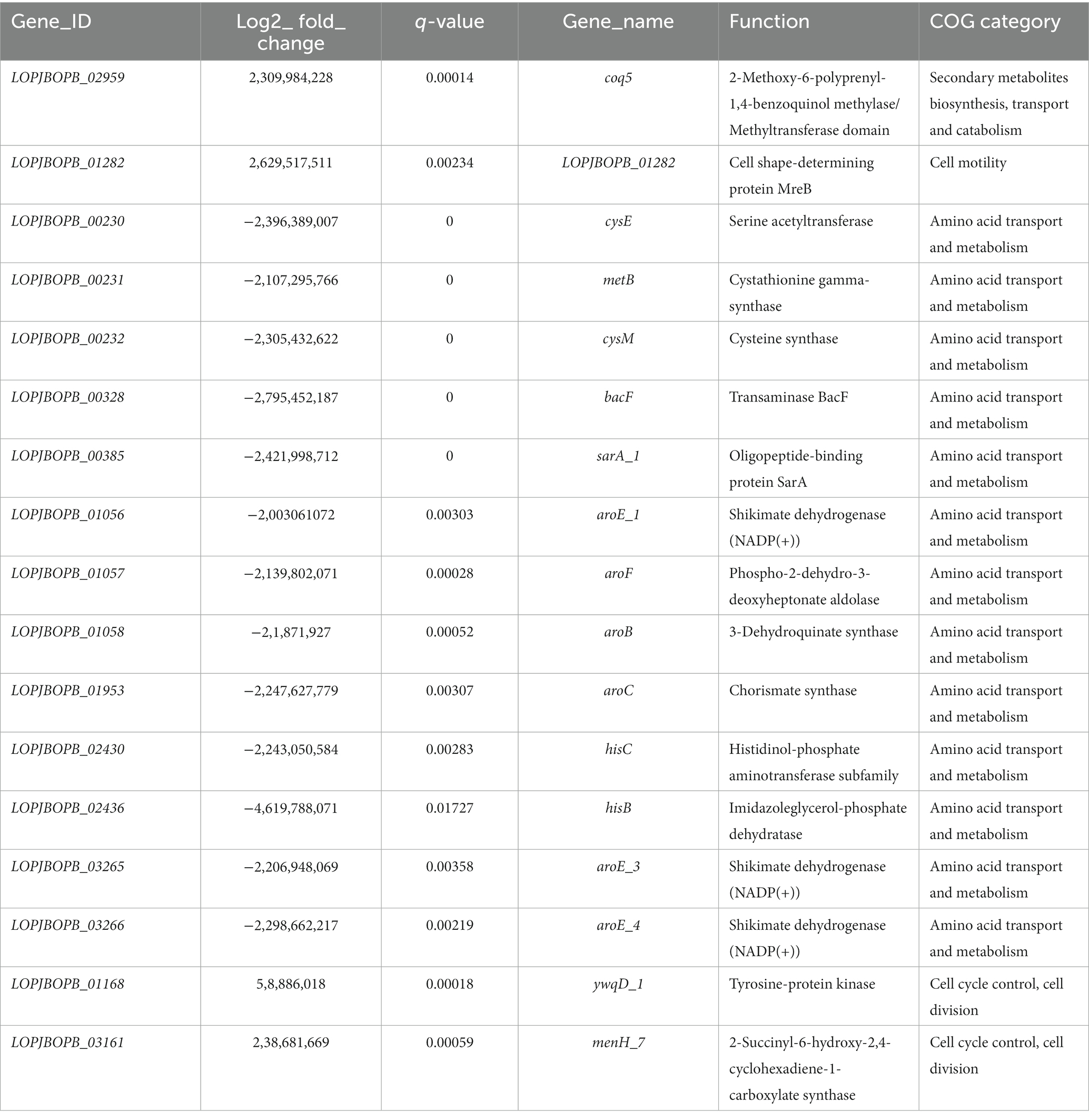
Table 2. Summary of the genes included in the four significantly enriched COG categories obtained during differential gene expression between sessile and planktonic LPG1 cells at the end of fermentation (60 days).
In other way, the upregulated gene associated with the enrichment of the motility COG category was LOPJBOPB_01282, encoding the cell shape-determining protein MreB. The MreB protein acts by regulating cell wall synthesis and elongation, thereby influencing cell shape. However, MreB protein has also been implicated in chromosomal segregation and cell polarity (Shih and Rothfield, 2006). Inhibition or loss-of-function mutations in the MreB protein result in cells adopting a spherical shape. Rhodobacter sphaeroides and Pseudomonas aeruginosa with inhibited MreB protein exhibited reduced ability to form biofilms. The authors suggested that cell shape affects biofilm formation by reducing the available surface for bacterial adhesion (Lin et al., 2015; Bonez et al., 2017). However, MreB protein also plays a role in oxidative stress response, as observed in Vibrio parahemolyticus and Latilactobacillus sakei (Chiu et al., 2008; Chiaramonte et al., 2010). Thus, overexpression of the mreB gene in LPG1 in mature non-pathogenic biofilms can have consequences on the structure and functionality of the biofilm. Finally, it is worth noting the overexpression of 21 genes belonging to a prophage out of a total of 58 genes comprising it. Considering the proportion of upregulated genes in the prophage relative to the total number of upregulated genes in the genome, the overexpression of prophage was found to be statistically significant. A prophage is the life form of a bacteriophage (bacterial virus) during its lysogenic cycle, where the phage incorporates into the bacterial genome (Moineau, 2013). Genes encoding major and minor capsid proteins, tail protein, head and tail connector protein, small and large subunits of the terminase were found to be upregulated in the biofilm. Previous RNA-seq studies have revealed that Vibrio parahaemolyticus, after the removal of prophage, exhibited a reduced ability to form biofilms due to a change in the cell surface hydrophobicity (Xu et al., 2022). However, few studies have been conducted on prophage induction within the biofilm context. Most studies have focuses on prophage release after bacterial death, where the liberated prophage can infect other bacteria. Therefore, further studies are needed to elucidate the role of prophages in the non-pathogenic biofilm formation and stability of Lpb. pentosus.
On the contrary, the genes downregulated in the non-pathogenic biofilm respect to brines were associated in a large number with amino acid transport and metabolism, as well as transcriptional regulations. Genes such as cysE, metB, and cysM were found to be downregulated and are clustered together. This gene cluster is involved in the synthesis of cysteine and methionine, which are essential amino acids for protein synthesis and cellular function. The proximity of these genes within the bacterial genome suggests coordinated regulation of their expression (Wüthrich et al., 2018).
Genes including aroE_1, aroE_3, aroE_4, aroF, aroB, LOPJBOPB_01952, and aroC, which are involved in the metabolism of shikimic acid were downregulated. The shikimate pathway plays a crucial role in the synthesis of aromatic amino acids such as phenylalanine, tyrosine, and tryptophan, as well as vitamins, folic acid, and other metabolites (Panina et al., 2003). Furthermore, genes hisC and hisB, responsible for histidine synthesis, were also downregulated. The downregulated genes in non-pathogenic biofilm involved in amino acid metabolism and transport has been previously reported (De Angelis et al., 2015; Liu et al., 2022). Zhao et al. (2021) speculated that the downregulated genes involved to amino acid synthesis pathways could be due to the presence of high levels of NaCl.
In contrast to bacterial cells within the non-pathogenic biofilm, planktonic cells in brines exhibited an overexpression of the lrgA_1 gene, encoding the Antiholin-like protein LrgA. This gene was predicted to encode the LrgA protein included in the aforementioned LrgAB system. As seen, the LrgAB system is involved in various cellular functions. As LrgB protein, LrgA protein is engaged with autolysis activity and biofilm formation. In this case, the LrgA protein encodes the regulator of murein hydrolase. Previous studies with S. mutans lrgA mutants have shown increased autolysis compared to lrgB mutants. Additionally, the product of the lrgB gene has been suggested to play a more significant role in stress tolerance compared to the lrgA gene (Ahn et al., 2010). Therefore, the lrgA gene does not appear to be essential for non-pathogenic biofilm formation by LPG1 strain.
4. Conclusion
To our knowledge, this is the first work on the global gene expression of a Lpb. pentosus strain during table olive fermentations. LPG1 showed a differential gene expression in brine during course fermentation, but also, between planktonic and sessile cells at the end of fermentation process. Deeping into the genetic bases of non-pathogenic biofilm formation by Lpb. pentosus strains with probiotic potential will help to turn this fermented vegetable into a carrier of beneficial microorganisms to the final consumers as an alternative to dairy products.
Data availability statement
The datasets presented in this study can be found in online repositories. The names of the repository/repositories and accession number(s) can be found at: https://www.ebi.ac.uk/ena, PRJEB63117.
Author contributions
EL-G: Formal analysis, Methodology, Writing – original draft, Writing – review & editing, Data curation. AB-C: Formal analysis, Methodology, Writing – original draft, Writing – review & editing. JT: Formal analysis, Supervision, Writing – review & editing. FA-L: Conceptualization, Funding acquisition, Project administration, Supervision, Writing – original draft, Writing – review & editing.
Funding
The author(s) declare financial support was received for the research, authorship, and/or publication of this article. This research was funded through the TOBE project (RTI2018-100883-B-I00, MCIU/AEI/FEDER, UE).
Acknowledgments
EL-G thanks the Spanish Ministry of Science and Innovation for his FPI contract (PRE2019-087812), while AB-C thanks Junta de Andalucia for his postdoctoral contract PAIDI2020-00162.
Conflict of interest
The authors declare that the research was conducted in the absence of any commercial or financial relationships that could be construed as a potential conflict of interest.
Publisher’s note
All claims expressed in this article are solely those of the authors and do not necessarily represent those of their affiliated organizations, or those of the publisher, the editors and the reviewers. Any product that may be evaluated in this article, or claim that may be made by its manufacturer, is not guaranteed or endorsed by the publisher.
Supplementary material
The Supplementary material for this article can be found online at: https://www.frontiersin.org/articles/10.3389/fmicb.2023.1264341/full#supplementary-material
References
Abriouel, H., Montoro, B. P., Casado Muñoz, M. D. C., Knapp, C. W., Gálvez, A., and Benomar, N. (2017). In silico genomic insights into aspects of food safety and defense mechanisms of a potentially probiotic Lactobacillus pentosus MP-10 isolated from brines of naturally fermented Aloreña green table olives. PLoS One 12, e0176801–e0176821. doi: 10.1371/journal.pone.0176801
Ahn, S. J., Rice, K. C., Oleas, J., Bayles, K. W., and Burne, R. A. (2010). The Streptococcus mutans Cid and Lrg systems modulate virulence traits in response to multiple environmental signals. Microbiology 156, 3136–3147. doi: 10.1099/mic.0.039586-0
Alonso García, E., de la Fuente Ordoñez, J. J., Lavilla Lerma, L., Estudillo-Martínez, M. D., Castillo-Gutiérrez, S., Benomar, N., et al. (2021). Transcriptomic profile and probiotic properties of Lactiplantibacillus pentosus pre-adapted to edible oils. Front. Microbiol. 12, 1–15. doi: 10.3389/fmicb.2021.747043
Ao, X., Zhao, J., Yan, J., Liu, S., and Zhao, K. (2020). Comparative transcriptomic analysis of Lactiplantibacillus plantarum RS66CD biofilm in high-salt conditions and planktonic cells. PeerJ 8, e9639–e9620. doi: 10.7717/peerj.9639
Arroyo-López, F. N., Bautista-Gallego, J., Domínguez-Manzano, J., Romero-Gil, V., Rodriguez-Gómez, F., García-García, P., et al. (2012). Formation of lactic acid bacteria-yeasts communities on the olive surface during Spanish-style Manzanilla fermentations. Food Microbiol. 32, 295–301. doi: 10.1016/j.fm.2012.07.003
Benítez-Cabello, A., Calero-Delgado, B., Rodríguez-Gómez, F., Garrido-Fernández, A., Jiménez-Díaz, R., and Arroyo-López, F. N. (2019). Biodiversity and multifunctional features of lactic acid bacteria isolated from table olive biofilms. Front. Microbiol. 10, 1–13. doi: 10.3389/fmicb.2019.00836
Benítez-Cabello, A., Romero-Gil, V., Rodríguez-Gómez, F., Garrido-Fernández, A., Jiménez-Díaz, R., and Arroyo-López, F. N. (2015). Evaluation and identification of poly-microbial biofilms on natural green Gordal table olives. Antonie van Leeuwenhoek. Int. J. Gen. Mol. Microbiol. 108, 597–610. doi: 10.1007/s10482-015-0515-2
Benítez-Cabello, A., Torres-Maravilla, E., Bermúdez-Humarán, L., Langella, P., Martín, R., Jiménez-Díaz, R., et al. (2020). Probiotic properties of Lactobacillus strains isolated from table olive biofilms. Probiotics Antimicrob. Proteins 12, 1071–1082. doi: 10.1007/s12602-019-09604-y
Bonez, P. C., Rossi, G. G., Bandeira, J. R., Ramos, A. P., Mizdal, C. R., Agertt, V. A., et al. (2017). Anti-biofilm activity of A22 ((S-3,4-dichlorobenzyl) isothiourea hydrochloride) against Pseudomonas aeruginosa: influence on biofilm formation, motility and bioadhesion. Microb. Pathog. 111, 6–13. doi: 10.1016/j.micpath.2017.08.008
Charlebois, A., Jacques, M., and Archambault, M. (2016). Comparative transcriptomic analysis of Clostridium perfringens biofilms and planktonic cells. Avian Pathol. 45, 593–601. doi: 10.1080/03079457.2016.1189512
Chen, S., Zhou, Y., Chen, Y., and Gu, J. (2018). Fastp: an ultra-fast all-in-one FASTQ preprocessor. Bioinformatics 34, i884–i890. doi: 10.1093/bioinformatics/bty560
Chiaramonte, F., Anglade, P., Baraige, F., Gratadoux, J. J., Langella, P., Champomier-Vergés, M. C., et al. (2010). Analysis of Lactobacillus sakei mutants selected after adaptation to the gastrointestinal tracts of axenic mice. Appl. Environ. Microbiol. 76, 2932–2939. doi: 10.1128/AEM.02451-09
Chiu, S. W., Chen, S. Y., and Wong, H. C. (2008). Localization and expression of MreB in Vibrio parahaemolyticus under different stresses. Appl. Environ. Microbiol. 74, 7016–7022. doi: 10.1128/AEM.01020-08
Conesa, A., Nueda, M. J., Ferrer, A., and Talón, M. (2006). maSigPro: a method to identify significantly differential expression profiles in time-course microarray experiments. Bioinformatics 22, 1096–1102. doi: 10.1093/bioinformatics/btl056
Curiel, J. A., Morales, P., Gonzalez, R., and Tronchoni, J. (2017). Different non-Saccharomyces yeast species stimulate nutrient consumption in S. cerevisiae mixed cultures. Front. Microbiol. 8, 1–9. doi: 10.3389/fmicb.2017.02121
Davey, M. E., and O’toole, G. A. (2000). Microbial biofilms: from ecology to molecular genetics. Microbiol. Mol. Biol. Rev. 64, 847–867. doi: 10.1128/mmbr.64.4.847-867.2000
De Angelis, M., Siragusa, S., Campanella, D., Di Cagno, R., and Gobbetti, M. (2015). Comparative proteomic analysis of biofilm and planktonic cells of Lactobacillus plantarum DB200. Proteomics 15, 2244–2257. doi: 10.1002/pmic.201400363
De Celis, M., Belda, I., Marquina, D., and Santos, A. (2022). Phenotypic and transcriptional study of the antimicrobial activity of silver and zinc oxide nanoparticles on a wastewater biofilm-forming Pseudomonas aeruginosa strain. Sci. Total Environ. 826:153915. doi: 10.1016/j.scitotenv.2022.153915
Deo, D., Davray, D., and Kulkarni, R. (2019). A diverse repertoire of exopolysaccharide biosynthesis gene clusters in Lactobacillus revealed by comparative analysis in 106 sequenced genomes. Microorganisms 7:444. doi: 10.3390/microorganisms7100444
Dertli, E., Mayer, M. J., and Narbad, A. (2015). Impact of the exopolysaccharide layer on biofilms, adhesion and resistance to stress in Lactobacillus johnsonii FI9785. BMC Microbiol. 15:8. doi: 10.1186/s12866-015-0347-2
Domínguez-Manzano, J., Olmo-Ruiz, C., Bautista-Gallego, J., Arroyo-López, F. N., Garrido-Fernández, A., and Jiménez-Díaz, R. (2012). Biofilm formation on abiotic and biotic surfaces during Spanish style green table olive fermentation. Int. J. Food Microbiol. 157, 230–238. doi: 10.1016/j.ijfoodmicro.2012.05.011
Faten, K., Hamida, K., Soumya, E. A., Saad, I. S. K., Hasna, M., Hassan, L., et al. (2016). Lactobacillus plantarum: effect of a protective biofilm on the surface of olives during storage. Brazilian J. Microbiol. 47, 202–209. doi: 10.1016/j.bjm.2015.11.028
Garrido-Fernandez, A., Fernandez-Diez, M. J., and Adams, M. R. (1997). Table Olives: Production and Processing. London, UK: Chapman & Hall.
Grimaud, R., Ezraty, B., Mitchell, J. K., Lafitte, D., Briand, C., Derrick, P. J., et al. (2001). Repair of oxidized proteins: identification of a new methionine sulfoxide reductase. J. Biol. Chem. 276, 48915–48920. doi: 10.1074/jbc.M105509200
Hernández-Jiménez, E., Campo, E., Toledano, V., Vallejo-Cremades, M. T., Muñoz, A., Largo, C., et al. (2013). Biofilm vs. planktonic bacterial mode of growth: which do human macrophages prefer? Biochem. Biophyc. Res. Commun. 441, 947–952. doi: 10.1016/j.bbrc.2013.11.012
Huang, M. L., Huang, J. Y., Kao, C. Y., and Fang, T. J. (2018). Complete genome sequence of Lactobacillus pentosus SLC13, isolated from mustard pickles, a potential probiotic strain with antimicrobial activity against foodborne pathogenic microorganisms. Gut Pathog. 10, 1–6. doi: 10.1186/s13099-018-0228-y
Hurtado, A., Reguant, C., Bordons, A., and Rozès, N. (2012). Lactic acid bacteria from fermented table olives. Food Microbiol. 31, 1–8. doi: 10.1016/j.fm.2012.01.006
Inglin, R. C., Meile, L., and Stevens, M. J. A. (2018). Clustering of Pan- and Core-genome of Lactobacillus provides novel evolutionary insights for differentiation. BMC Genomics 19, 284–215. doi: 10.1186/s12864-018-4601-5
International Olive Council . (2023). Economic Affairs & Promotion Unit - International Olive Council. Available at: (https://www.internationaloliveoil.org/what-we-do/economic-affairs-promotion-unit/#figures%0Ahttps://www.internationaloliveoil.org/what-we-do/economic-affairs-promotion-unit/#figures%0Ahttps://www.internationaloliveoil.org/what-we-do/economic-affairs-promoti).
Jahid, I. K., and Ha, S. (2012). Do a review of microbial biofilms of produce: future challenge to food safety. Food Sci. Biotechnol. 21, 299–316. doi: 10.1007/s10068-012-0041-1
Kim, H., Kim, J. S., Kim, Y. G., Jeong, Y., Kim, J. E., Paek, N. S., et al. (2020). Antioxidant and probiotic properties of lactobacilli and Bifidobacteria of human origins. Biotechnol. Bioprocess Eng. 25, 421–430. doi: 10.1007/s12257-020-0147-x
Kim, D., Langmead, B., and Salzberg, S. L. (2015). HISAT: a fast spliced aligner with low memory requirements. Nat. Methods 12, 357–360. doi: 10.1038/nmeth.3317
Lalioui, L., Pellegrini, E., Dramsi, S., Baptista, M., Bourgeois, N., Doucet-Populaire, F., et al. (2005). The SrtA sortase of Streptococcus agalactiae is required for cell wall anchoring of proteins containing the LPXTG motif, for adhesion to epithelial cells, and for colonization of the mouse intestine. Infect. Immun. 73, 3342–3350. doi: 10.1128/IAI.73.6.3342-3350.2005
Lavermicocca, P., Valerio, F., Lonigro, S. L., De Angelis, M., Morelli, L., Callegari, M. L., et al. (2005). Study of adhesion and survival of lactobacilli and bifidobacteria on table olives with the aim of formulating a new probiotic food. Appl. Environ. Microbiol. 71, 4233–4240. doi: 10.1128/AEM.71.8.4233-4240.2005
Lin, T. Y., Santos, T. M. A., Kontur, W. S., Donohue, T. J., and Weibel, D. B. (2015). A cardiolipin-deficient mutant of Rhodobacter sphaeroides has an altered cell shape and is impaired in biofilm formation. J. Bacteriol. 197, 3446–3455. doi: 10.1128/JB.00420-15
Liu, B., Li, Y., and Zhang, L. (2022). Analysis and visualization of spatial transcriptomic data. Front. Genet. 12, 1–15. doi: 10.3389/fgene.2021.785290
López-García, E., Benítez-Cabello, A., Arenas-de Larriva, A. P., Gutierrez-Mariscal, F. M., Pérez-Martínez, P., Yubero-Serrano, E. M., et al. (2023b). Oral intake of Lactiplantibacillus pentosus LPG1 produces a beneficial regulation of gut microbiota in healthy persons: a randomised, placebo-controlled, single-blind trial. Nutrients 15:1931. doi: 10.3390/nu15081931
López-García, E., Benítez-Cabello, A., Ramiro-García, J., Ladero, V., and Arroyo-López, F. N. (2023a). In silico evidence of the multifunctional features of Lactiplantibacillus pentosus LPG1, a natural fermenting agent isolated from table olive biofilms. Foods 12:938. doi: 10.3390/foods12050938
Love, M. I., Huber, W., and Anders, S. (2014). Moderated estimation of fold change and dispersion for RNA-seq data with DESeq2. Genome Biol. 15, 550–521. doi: 10.1186/s13059-014-0550-8
Mann, E. E., Rice, K. C., Boles, B. R., Endres, J. L., and Ranjit, D. (2009). Modulation of eDNA Release and Degradation Affects staphylococcus aureus Biofilm Maturation. PLoS ONE 4:e5822. doi: 10.1371/journal.pone.0005822
Man, L. L., and Xiang, D. J. (2021). LuxS-mediated quorum sensing system in Lactobacillus plantarum NMD-17 from koumiss: induction of plantaricin MX in co-cultivation with certain lactic acid bacteria. Folia Microbial (Praha.). 66, 855–871. doi: 10.1007/s12223-021-00890-0
Martinez, B., Rodriguez, A., Kulakauskas, S., and Chapot-Chartier, M. P. (2020). Cell wall homeostasis in lactic acid bacteria: threats and defences. FEMS Microbiol. Rev. 44, 538–564. doi: 10.1093/femsre/fuaa021
Medina, E., Gori, C., Servili, M., de Castro, A., Romero, C., and Brenes, M. (2010). Main variables affecting the lactic acid fermentation of table olives. Int. J. Food Sci. Technol. 45, 1291–1296. doi: 10.1111/j.1365-2621.2010.02274.x
Moineau, S. B. (2013). In Brenner’s Encyclopedia of Genetics. 2nd Edn. (Cambridge, MA, USA: Academic Press), 280–283.
Mongkolsuk, S., and Helmann, J. D. (2002). Regulation of inducible peroxide stress responses. Mol. Microbiol. 45, 9–15. doi: 10.1046/j.1365-2958.2002.03015.x
Nychas, G. J. E., Panagou, E. Z., Parker, M. L., Waldron, K. W., and Tassou, C. C. (2002). Microbial colonization of naturally black olives during fermentation and associated biochemical activities in the cover brine. Lett. Appl. Microbiol. 34, 173–177. doi: 10.1046/j.1472-765x.2002.01077.x
O’Sullivan, O., O’Callaghan, J., Sangrador-Vegas, A., McAuliffe, O., Slattery, L., Kaleta, P., et al. (2009). Comparative genomics of lactic acid bacteria reveals a niche-specific gene set. BMC Microbiol. 9, 1–9. doi: 10.1186/1471-2180-9-50
Okonechnikov, K., Conesa, A., and García-Alcalde, F. (2016). Qualimap 2: advanced multi-sample quality control for high-throughput sequencing data. Bioinformatics 32, 292–294. doi: 10.1093/bioinformatics/btv566
Panina, E. M., Vitreschak, A. G., Mironov, A. A., and Gelfand, M. S. (2003). Regulation of biosynthesis and transport of aromatic amino acids in low-GC gram-positive bacteria. FEMS Microbiol. Lett. 222, 211–220. doi: 10.1016/S0378-1097(03)00303-3
Perpetuini, G., Pham-Hoang, B. N., Scornec, H., Tofalo, R., Schirone, M., Suzzi, G., et al. (2016). In Lactobacillus pentosus, the olive brine adaptation genes are required for biofilm formation. Int. J. Food Microbiol. 216, 104–109. doi: 10.1016/j.ijfoodmicro.2015.10.002
Pertea, M., Pertea, G. M., Antonescu, C. M., Chang, T. C., Mendell, J. T., and Salzberg, S. L. (2015). StringTie enables improved reconstruction of a transcriptome from RNA-seq reads. Nat. Biotechnol. 33, 290–295. doi: 10.1038/nbt.3122
Philips, J., Rabaey, K., Lovley, D. R., and Vargas, M. (2017). Biofilm formation by Clostridium ljungdahlii is induced by sodium chloride stress: experimental evaluation and transcriptome analysis. PLoS One 12, 1–25. doi: 10.1371/journal.pone.0170406
Pophaly, S. D., Singh, R., Pophaly, S. D., Kaushik, J. K., and Tomar, S. K. (2012). Current status and emerging role of glutathione in food grade lactic acid bacteria. Microb. Cell Factories 11, 1–14. doi: 10.1186/1475-2859-11-114
Remis, J. P., Costerton, J. W., and Auer, M. (2010). Biofilms: structures that may facilitate cell-cell interactions. ISME J. 4, 1085–1087. doi: 10.1038/ismej.2010.105
Rice, K. C., Turner, M. E., Carney, O. V., Gu, T., and Ahn, S.-J. (2017). Modification of the Streptococcus mutans transcriptome by LrgAB and environmental stressors. Microb. Genomics 3:e000104. doi: 10.1099/mgen.0.000104
Rios-Covian, D., Cuesta, I., Alvarez-Buylla, J. R., Ruas-Madiedo, P., Gueimonde, M., and De Los Reyes-Gavilán, C. G. (2016). Bacteroides fragilis metabolises exopolysaccharides produced by bifidobacteria. BMC Microbiol. 16:150. doi: 10.1186/s12866-016-0773-9
Rodriguez-Gomez, F., Romero-Gil, V., Bautista-Gallego, J., García-García, P., Garrido-Fernández, A., and Arroyo-Lopez, F. N. (2014). Production of potential probiotic Spanish-style green table olives at pilot plant scale using multifunctional starters. Food Microbiol. 44, 278–287. doi: 10.1016/j.fm.2014.03.023
Ruiz-Barba, J. L., Cathcart, D. P., Warner, P. J., and Jimenez-Diaz, R. (1994). Use of Lactobacillus plantarum LPCO10, a bacteriocin producer, as a starter culture in Spanish-style green olive fermentations. Appl. Environ. Microbiol. 60, 2059–2064. doi: 10.1128/aem.60.6.2059-2064.1994
Shih, Y.-L., and Rothfield, L. (2006). The bacterial cytoskeleton. Microbiol. Mol. Biol. Rev. 70, 729–754. doi: 10.1128/mmbr.00017-06
Stephani, K., Weichart, D., and Hengge, R. (2003). Dynamic control of Dps protein levels by ClpXP and ClpAP proteases in Escherichia coli. Mol. Microbiol. 49, 1605–1614. doi: 10.1046/j.1365-2958.2003.03644.x
Sun, L., Zhang, Y., Guo, X., Zhang, L., Zhang, W., Man, C., et al. (2020). Characterization and transcriptomic basis of biofilm formation by Lactobacillus plantarum J26 isolated from traditional fermented dairy products. LWT 125:109333. doi: 10.1016/j.lwt.2020.109333
Wimpenny, J. (2009). Microbial metropolis. Adv. Microb. Physiol. 56, 29–84. doi: 10.1016/S0065-2911(09)05602-1
Wing, C., Errey, J. C., Mukhopadhyay, B., Blanchard, J. S., and Field, R. A. (2006). Expression and initial characterization of WbbI, a putative d-Galf:α-d-Glc β-1,6-galactofuranosyltransferase from Escherichia coli K-12. Org. Biomol. Chem. 4, 3945–3950. doi: 10.1039/b609455d
Wold, S., Esbensen, K., and Geladi, P. (1987). Principal component analysis. Chemom. Intell. Lab. Syst. 2, 37–52. doi: 10.1016/0169-7439(87)80084-9
Wüthrich, D., Wenzel, C., Bavan, T., Bruggmann, R., Berthoud, H., and Irmler, S. (2018). Transcriptional regulation of cysteine and methionine metabolism in Lactobacillus paracasei FAM18149. Front. Microbiol. 9, 1–11. doi: 10.3389/fmicb.2018.01261
Xu, Y., Yang, L., Wang, Y., Zhu, Z., Yan, J., Qin, S., et al. (2022). Prophage-encoded gene VpaChn25_0734 amplifies ecological persistence of Vibrio parahaemolyticus CHN25. Curr. Genet. 68, 267–287. doi: 10.1007/s00294-022-01229-z
Zhang, J., Meng, L., Zhang, Y., Sang, L., Liu, Q., Zhao, L., et al. (2020). GapB is involved in biofilm formation dependent on LrgAB but not the SinI/R system in Bacillus cereus 0-9. Front. Microbiol. 11:591926. doi: 10.3389/fmicb.2020.591926
Zhao, L., Zhao, P., Shang, J., and Meng, X. (2021). Transcriptome analysis of the molecular mechanism of Bacteriocin synthesis in Lactobacillus plantarum KLDS1.0391 under nanoparticle NaCl stress. J. Biomed. Nanotechnol. 17, 369–381. doi: 10.1166/jbn.2021.3035
Zhou, K., Zeng, Y. T., Han, X. F., and Liu, S. (2015). Liang modelling growth and Bacteriocin production by Lactobacillus plantarum BC-25 in response to temperature and pH in batch fermentation. Appl. Biochem. Biotechnol. 176, 1627–1637. doi: 10.1007/s12010-015-1666-3
Keywords: Lactobacillus pentosus , vegetable fermentation, RNA-seq, biofilm, time-course analysis
Citation: López-García E, Benítez-Cabello A, Tronchoni J and Arroyo-López FN (2023) Understanding the transcriptomic response of Lactiplantibacillus pentosus LPG1 during Spanish-style green table olive fermentations. Front. Microbiol. 14:1264341. doi: 10.3389/fmicb.2023.1264341
Edited by:
Christian Magni, CONICET Instituto de Biología Molecular y Celular de Rosario (IBR), ArgentinaReviewed by:
Do-Won Jeong, Dongduk Women's University, Republic of KoreaCristian Botta, University of Turin, Italy
Copyright © 2023 López-García, Benítez-Cabello, Tronchoni and Arroyo-López. This is an open-access article distributed under the terms of the Creative Commons Attribution License (CC BY). The use, distribution or reproduction in other forums is permitted, provided the original author(s) and the copyright owner(s) are credited and that the original publication in this journal is cited, in accordance with accepted academic practice. No use, distribution or reproduction is permitted which does not comply with these terms.
*Correspondence: Elio López-García, ZWxvcGV6QGlnLmNzaWMuZXM=