- 1Microbial Processes and Technology Division (MPTD), CSIR-National Institute for Interdisciplinary Science and Technology (NIIST), Thiruvananthapuram, India
- 2Central Laboratory of Agricultural and Food Products, FAFSEM, University of Debrecen, Debrecen, Hungary
- 3Department of Molecular Biotechnology and Microbiology, Institute of Biotechnology Faculty of Science and Technology, University of Debrecen, Debrecen, Hungary
Mycotoxins produced by Fusarium species are secondary metabolites with low molecular weight formed by filamentous fungi generally resistant to different environmental factors and, therefore, undergo slow degradation. Contamination by Fusarium mycotoxins in cereals and millets is the foremost quality challenge the food and feed industry faces across the globe. Several types of chemical preservatives are employed in the mitigation process of these mycotoxins, and they help in long-term storage; however, chemical preservatives can be used only to some extent, so the complete elimination of toxins from foods is still a herculean task. The growing demand for green-labeled food drives to evade the use of chemicals in the production processes is getting much demand. Thus, the biocontrol of food toxins is important in the developing food sector. Fusarium mycotoxins are world-spread contaminants naturally occurring in commodities, food, and feed. The major mycotoxins Fusarium species produce are deoxynivalenol, fumonisins, zearalenone, and T2/HT2 toxins. Lactic acid bacteria (LAB), generally regarded as safe (GRAS), is a well-explored bacterial community in food preparations and preservation for ages. Recent research suggests that LAB are the best choice for extenuating Fusarium mycotoxins. Apart from Fusarium mycotoxins, this review focuses on the latest studies on the mechanisms of how LAB effectively detoxify and remove these mycotoxins through their various bioactive molecules and background information of these molecules.
1 Introduction
Mycotoxin contamination is one of the primary concerns of all the necessary actions for securing food (Streit et al., 2013). It is predicted that over 25% of global cereal and roughly 20% of global plant production may be contaminated with mycotoxins, and a loss of 60 billion USD is reported due to the same (Johns et al., 2022). However, the level of contamination may vary substantially depending on the regional production and storage environment (Varsha et al., 2014; Bryła et al., 2018). The genera of fungi that can cause mycoses and mycotoxicosis in animals and people are Aspergillus, Penicillium, Fusarium, and Claviceps. Long-term mycotoxin exposure has been linked to nephrotoxic, hepatotoxic, immunotoxic, neurotoxic, teratogenic, estrogenic, hemorrhagic, and immunosuppressive effects (Cinar and Onbaşı, 2019). Diverse secondary metabolite mycotoxins like deoxynivalenol (DON), nivalenol (NIV), T-2 and HT-2 toxins, zearalenone (ZEA), fumonisins (FUMs) are produced by Fusarium species that are responsible for the production of Fusarium mycotoxins usually appear together in contaminated matrices. There are 127 mycotoxin combinations detected up to now, and DON+ZEA, aflatoxins+FUM, aflatoxins+ochratoxin, and FUM+ZEA toxin combinations are the most usual ones (BIOMIN Ltd.).
Various physical, chemical, and enzymatic possibilities exist to eliminate multiple fungal mycotoxins (Karlovsky et al., 2016). Biological methods are available that use microorganisms or their enzymes to mitigate mycotoxins. These strategies can be classified followed by their mode of action: (i) direct or indirect inhibition of the growth and mycotoxin production of Fusarium species through; (ii) adsorption of the molecules to polymer cell components, e.g., cell wall constituents; (iii) biotransformation of mycotoxins into non-toxic metabolites; and (iv) conjugation of mycotoxins to cell components.
The ability of bacteria cultures to inactivate and remove Fusarium mycotoxins has been discovered and studied for a long time. In recent years, in vitro screening helped identify more strains with this specific function, but their reports on food and feed are still limited. This is due to rigorous requests for biological methods applied to the food source of animals and humans. Since safety is a priority, bacteria, besides the detoxification potential, must not contain any unwanted bacterial toxins or allergens, which is why species that have been closely related to food production or are generally GRAS are more favored. In addition, in the case of the detoxification process, the products of the reaction must exert none or less toxicity than their parental molecules and be unable to convert back to the original mycotoxins.
Lactic acid bacteria have an extended history of usage as a natural bio-preservative in food and animal feed (Muhialdin et al., 2020; Petrova et al., 2022). LAB are Gram-positive, non-motile, and catalase-negative bacteria without endospore production, and as their name states excrete lactic acid as a primary product. Homo- and heterofermentative LAB increase the variability of primary metabolites in food and feed products. Diverse antimicrobial substances generated by LAB serve as food preservatives (Shi and Maktabdar, 2022). Additionally, some GRAS LAB strains are chosen as probiotics, which can improve health by immunomodulation and inhibition of internal adhesion of enteropathogenic bacteria (Masood et al., 2011; Arena et al., 2018), working against xenobiotics like mycotoxins (Abbès et al., 2016), or tumor cancer formation (Bell et al., 2022).
This review mainly emphasizes the contribution of the molecular background of LAB antifungal and detoxification potential against mycotoxins produced by Fusarium species.
2 Diversity of Fusaria
Most plant-pathogenic Fusarium spp. are members of four species complexes: F. fujikuroi species complex (FFSC), F. graminearum species complex (FGSC), F. solani species complex and F. oxysporum species complex (Aoki et al., 2014). Outside of these four groups, F. langsethiae, F. sporotrichioides, and F. poae are several other species that also receive attention (Munkvold, 2017). Infection of these molds results in a wide range of serious blights, wilts, rots, and cankers, broadly affecting many important crops. Fusarium Head Blight (FHB), the most agricultural concern related to Fusarium, has caused huge economic loss, specifically in wheat, barley, and maize production (Wegulo et al., 2015). It is generally induced by members of the F. graminearum complex, typically F. graminearum, F. avenaceum, and F. culmorum. Besides FHB, maize production also must face two serious Fusarium infection diseases, including Fusarium Ear Rot or “pink ear rot” and Gibberella Ear Rot. While F. proliferatum, F. verticillioides, and F. subglutinans, as members of FFSC, are causal agents of the former, the latter is mainly caused by a few prominent FGSC members: F. graminearum, F. culmorum, and to a lesser extent, F. avenaceum (Ferrigo et al., 2016). The infection is also unavoidable in other crops, additionally causing rot in different parts of the plants and seedling diseases (Broders et al., 2007; Abu Bakar et al., 2013).
Head Blight and other Fusarium-caused diseases are known to have a close relationship with the presence of mycotoxins. FHB main carriers, members of FGSC, are potent producers of type A and B trichothecenes (TCTs)—a toxin group that is especially compromised due to its common occurrence and prominent phyto- and myotoxicity, along with other minor pathogens associated with FHB such as F. poae, F. langsethiae and F. sporotrichioides (Munkvold, 2017). ZEA shares some same producers with type B TCTs, with the species of FGSC being the most relevant ones, thus, it frequently co-occurs along with several TCT toxins. Meanwhile, FFSC members, particularly F. verticillioides and F. proliferatum, are found to be the most important species for FUM production. They predominantly produce type B FUMs, the most frequent group detected, and food and feed. Several new Fusarium mycotoxins recently received safety concerns in studies due to their emerging occurrence in plants and crops, including beauvericin, moniliformin, and enniatins (Nazari et al., 2015; Jajić et al., 2019).
In general, moderately warm, and highly moist conditions in this period are favorable for the distribution of most Fusarium species. FGSC is frequently found in wheat and barley, with the highest prevalence of F. graminearum in many countries of Asia, America, and Europe (Van Der Lee et al., 2015). Its presence is likely observed in cool areas where the mean temperature is not higher than 15°C and is predicted to be distributed in most major rainfed wheat-growing regions of the world (Qu et al., 2007; Backhouse, 2014; Schöneberg et al., 2018). On the other hand, F. asiaticum is well adapted to areas where the warmest season has a high mean temperature (>22°C) and precipitation (>320 mm) (Backhouse, 2014). It is reported to prefer rice-growing regions or crops mainly grown in rotation with rice (Van Der Lee et al., 2015). Likewise, F. langsethiae, one of the main type A TCT producers, prefers similar conditions but seems slightly more sensitive to water activity than other Fusarium species, with no growth observed below 0.90 aw. Its optimal temperature is 25°C and aw > 0.98, representing 28–30% moisture content in grains at 15–25°C, which would be very wet conditions (Medina and Magan, 2010). Under climate change, the alteration in the dominant species and the general abundance in the Fusarium complex may also occur (Uhlig et al., 2013). The high occurrence of F. poae in wheat is relatively connected to warm and dry conditions during anthesis (Chrpová et al., 2016). F. poae and F. avenaceum tend to be more active in climatic conditions that are unfavorable for FHB causal agents like F. graminearum (Wiśniewska et al., 2014; Covarelli et al., 2015). Meanwhile, F. boothii might be sensitive to cold sites but can also be more competitive in warmer regions. It has outnumbered F. graminearum to become one of the main Fusarium pathogens in wheat in Southern Mexico and Kenya, where it is normally dominant in maize (Cerón-Bustamante et al., 2018). FUM-producing species like F. proliferatum and F. verticillioides can survive in a wide range of conditions and particularly the most optimal temperature is between 25 and 30°C. F. verticillioides additionally show considerably better performance at high temperatures and water stress than F. proliferatum (Marín et al., 2010).
3 Trichothecenes (TCTs)
Trichothecenes consist of a sesquiterpenoid structure, with or without macrocyclic esters or ester ether bridges between C-4 and C-15. The cytotoxicity of a TCT structure is due to the presence of 12, 13-epoxyalkylene groups and 9, 10 double bonds with different side-chain substitutions (McCormick et al., 2011). Type A toxins include T-2 [insariotoxin or 8-(3-methylbutyryloxy)diacetoxyscirpenol], HT-2, neosolaniol (ENNS), and diacetoxyscirpenol (DAS) (Figure 1). Type B toxins include deoxynivalenol (DON) and its derivatives, also nivalenol (NIV), together with the acetylated precursor of NIV [4-acetyl nivalenol, also termed fusarenon-X (FUX)] (Kimura et al., 2007).
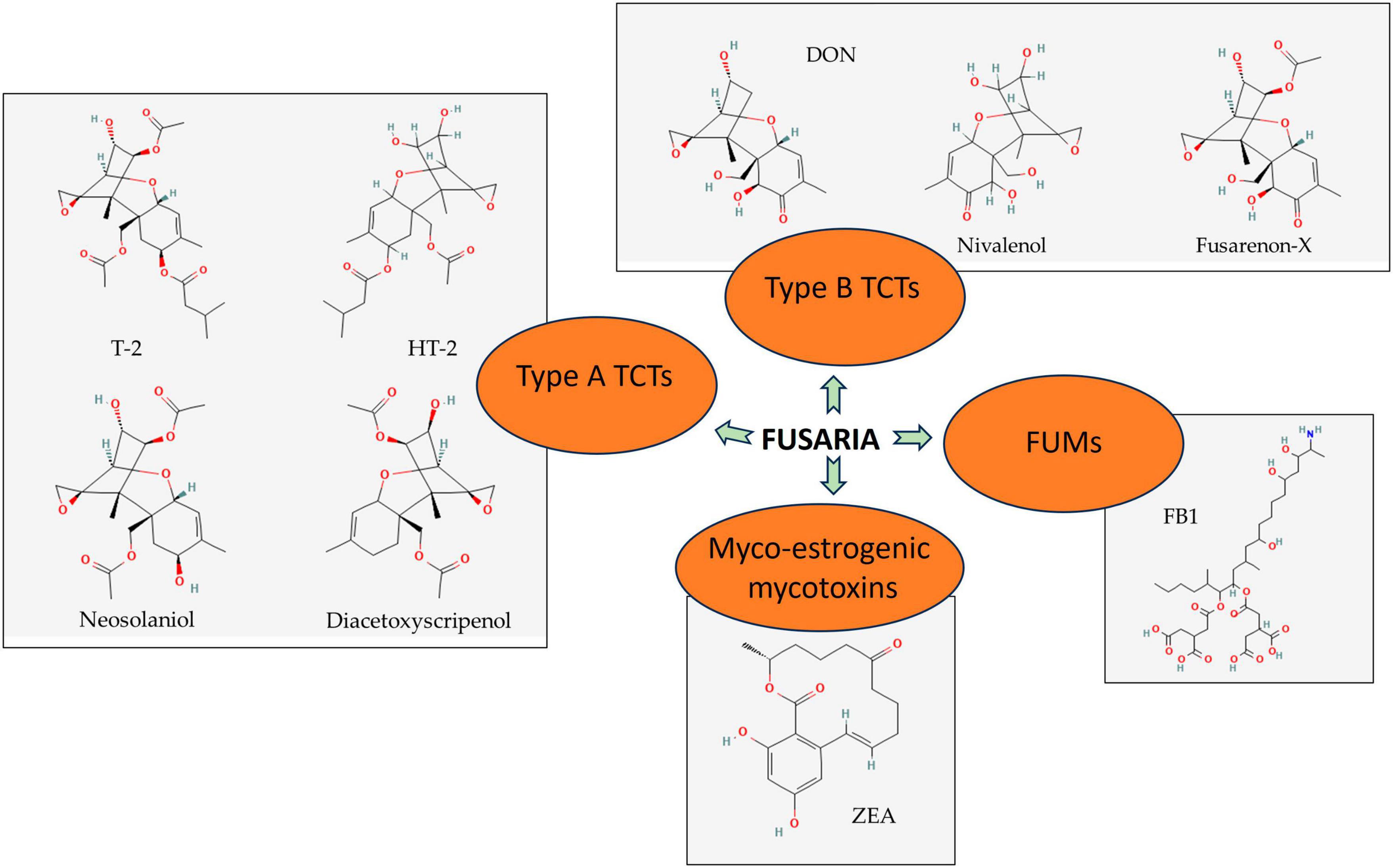
Figure 1. Significant secondary metabolites produced by Fusaria. Type A trichothecenes (TCTs), type B TCSs, myco-oestrogenic mycotoxins, and fumonisins (FUMs) are detected generally in agricultural commodities. Source: National Center for Biotechnology Information (2023). PubChem Compound Summary. Retrieved June 1, 2023.
Because the target of their producers is greatly diverse, TCTs are found in most of the important economic cereals (e.g., wheat, oat, barley) and legumes (e.g., soybeans), and potatoes during the pre-harvest or post-harvest stage of improperly stored commodities (Delgado et al., 2010; Barros et al., 2011; Bernhoft et al., 2012; Mylona et al., 2012).
Important type B TCTs commonly concerned with food safety are DON and NIV. Their structures only differ in one hydroxyl group, thus sharing various toxicological features. F. graminearum and F. culmorum are the key fungal species that produce DON. DON is chemically described as 12, 13-epoxy-3α, 7α, 15-trihydroxytrichothec-9-en-8-one (Figure 1). DON acetylated form, particularly 3-acetyl and 15-acetyl-deoxynivalenol (3-ADON and 15-ADON), also catch attention owing to their likelihood of occurrence. These mycotoxins usually accompany the symptoms of FHB and other Fusaria-caused diseases in crops. They show a certain role in the aggressiveness of the pathogens during host invasion since they are one of the Fusarium virulent factors (Audenaert et al., 2013). In the case of oral ingestion, intestinal cells are one of the first targets for NIV and DON to conduct function disruption, with the greater acute induced impacts of NIV compared to DON (Ghareeb et al., 2015; Zingales et al., 2021).
Common TCTs of type A are T-2 and HT-2 (Figure 1), identified as secondary products of F. langsethiae, F. poae, and F. sporotrichioides. Diacetoxyscirpenol and neosolaniol (Figure 1) are also under concern, but they have limited incidence. NX toxins—novel TCTs in grains mainly produced by FGSC strains- are also listed in the type A group due to their similar structure (Chen et al., 2022). Despite the higher occurrence frequency and produced amount in food and feed of type B, its toxicity is not comparable to that of type A (Munkvold, 2017).
T-2 is considered one of the most acutely toxic TCTs. T-2 cannot be excreted intact, it is rapidly microbially metabolized in the intestine into HT-2—its major metabolite. However, unlike DOM-1, HT-2 retains nearly completely the toxicity of its precursor (Cope, 2018).
4 Myco-estrogenic mycotoxins—Zearalenone and its derivatives
Fusarium graminearum and F. cerealis are major Fusarium species that produce an estrogenic mycotoxin called zearalenone (ZEA) (Figure 1) that contaminates food grains globally. ZEA is 6-(10-hydroxy-6-oxo-trans-1-undeceny) β-resorcylic acid lactone, which has a molecular formula of C18H12O5. ZEA and its derivatives are the second most regular Fusarium mycotoxins that endanger human health through foods just after TCTs. ZEA has the same producers with type B TCTs, with FGSC being the most important one; thus, it frequently co-occurs along with DON and/or NIV on crops and grains. 17-beta estradiol (17β-estradiol) is a major reproductive hormone resembling the ZEA structure (Zielonka et al., 2014). Unlike TCTs, ZEA neither exhibits fatal acute toxicity toward animals nor is a virulence factor of its producers (Munkvold, 2017). Instead, due to the similarities to estrogenic hormones in chemical structure, it expresses some estrogenic features and primarily targets the reproductive system of animals. After ingestion, ZEA is absorbed and degraded into some of its metabolites, including major ones α-zearalenol (α-ZEA) and β-zearalenol (β-ZEA). Subsequently, they are transferred from the alimentary tract to the reproductive tract, competing with estrogens for binding to estrogen receptors (Zhang et al., 2018).
5 Fumonisins
Fumonisins are a group of mycotoxins with a long chain backbone structurally resembling sphingosine. FFSC members, particularly F. verticillioides, F. proliferatum, and F. nygamai, are found to be the main species responsible for FUM production. A series of 4 types of B fumonisins (FBs) named FB1 (Figure 1), FB2, FB3, and FB4 are the most prevalent FUM members detected in food and feed (Li et al., 2015). Among these, FB1 has the highest toxicity and frequency of occurrence, accounting for 70–80% of total FUMs production. Because of the similarities in structure, FB1 expresses its toxicity by disrupting the conversion of sphingosine and inactivating the enzyme ceramide synthase. The congestion leads to the accumulation of free sphingosine and its metabolites and the shortage of desired sphingolipids–important components in eukaryotic cell membrane biology and cellular signaling. In addition, FB1 attempts to imbalance the intracellular dynamic equilibrium between antioxidants and oxidants and regulate gene expression. These series of sabotage are underlying FB1’s immunotoxicity, reproductive toxicity, carcinogenicity, apoptosis, and autophagy initiation (Chen et al., 2021).
FB1 and FB2 are the most common FBs. One of the most representative characteristics of FB1 and FB2 is their long hydrocarbon chain, which contributes to their toxicity (Hussein, 2001). Contaminations by FBs commonly occur during pre-harvest or at the beginning of storage (Marín et al., 2013), and maize is the matrix in which almost all FB contaminations are produced (EFSA, 2014). Contamination levels can vary drastically between maize samples, especially between maize fractions intended for animal feed and raw maize (EFSA, 2014). Despite this high presence of FBs in maize samples, concentration levels do not increase during storage (Marín et al., 2013), which makes it easier to control them.
6 Biological control of Fusaria by lactic acid bacteria
Among multiple bacteria species taken into consideration, along with in vitro evaluations, LAB and Bacillus spp. are two groups of bacteria that are tested on food the most due to their advantages raising the possibility of application. Their role has been reported not to be limited to antimicrobial ability, certain strains could inhibit fungal mycotoxin productions or decrease mycotoxin concentrations of the matrices (Byrne et al., 2022; Nasrollahzadeh et al., 2022a; Raman et al., 2022; Steglińska et al., 2022; Hirozawa et al., 2023; Mateo et al., 2023; Vargas et al., 2023). Not to mention that they can be isolated from food, plants, or animal intestinal and have various applications in both food and non-food sectors. Bacterial cells typically adsorb mycotoxins, such as DON on their cell wall components, or can detoxify these pollutants using enzymes such as ZEA by zearalenone hydrolase. They can simply hinder the growth of molds by different metabolites, such as bacteriocins and acid generation (Soltani et al., 2021; Houssni et al., 2023; Mateo et al., 2023). Arena et al. (2018) claimed that a protonated form of lactic acid inhibits the pH gradient between the outside acidic environment and the inside alkaline cytosol, dissipating the membrane potential and killing cells. LAB metabolites reported that molecules such as hydrogen peroxide, acetate, butyrate, formic acid, succinate, propionate, valerate, caproic acid, and cyclic dipeptides have potent antifungal properties (Fazeli et al., 2004; Bergsma et al., 2022; Zapaśnik et al., 2022). An experiment on sourdough bread between six strains of Lactiplantibacillus plantarum showed that strain AR524 at 108 CFU ml–1 could remove the highest amount of DON (0.359 mg kg–1), and this process majorly occurred in the fermentation stage (Cao et al., 2021). Throughout the simultaneous in vitro experiments, neither significant differences were observed for DON reduction rate between viable and non-viable cells, nor DON metabolites were detected. This finding supported pre-existing speculation that the main removal mechanism is physical adsorption by cell wall peptidoglycan and polysaccharides rather than biodegradation. Biotreatment of malting wheat grains with a suspension of bacteria culture containing Pediococcus pentosaceus strains greatly affected the mycotoxin content of grains, especially TCT contamination (Juodeikiene et al., 2018; Peter et al., 2022). Instead of exhibiting a direct absorption ability, some strains act as probiotics and reduce the impairment caused by mycotoxins. For instance, Lacticaseibacillus rhamnosus RC007 limited intestinal toxicity of DON to pig jejunum explants model at a probiotic dose (García et al., 2018). Since Bacillus spp. and Lactiplantibacillus spp. are both Gram-positive bacteria with similar cell wall characteristics, ZEA could be absorbed on its surface (Chen et al., 2019; Salah-Abbès et al., 2021). Meanwhile, esterase activity was indicated in certain strains, which is hypothesized to be linked to their ZEA degradation ability by partially contributing to the cleavage of ZEA lactone structure (Chen et al., 2019). Zhao et al. (2015), in a study with the strain L. plantarum Lp22 showed a 45 % degradation of ZEA in liquid media through a slow and continuous process by 48 h of incubation.
Recently, more new bacteria strains have been screened in vitro, providing more potential options for novel food and feed additives. As previously mentioned, the toxicity of TCTs is mainly determined by the presence of an epoxy ring at C-12, 13 and a double bond at C-9, 10. Hence, biotransformation conducted by several bacteria strains is found to focus on these structures, making TCTs become less toxic agents (McCormick, 2013; Li et al., 2015).
The two main pathogenic fungal strains reported to be suppressed in vitro by P. pentosaceus are F. verticillioides and F. proliferatum (Achar and Sreenivasa, 2021). Antifungal activity in P. pentosaceus culture supernatant was observed near the end of the exponential growth phase and was pH-dependent. The antifungal metabolites produced demonstrated good thermal stability and resistance to proteolytic enzymes. In culture fractions, substances with molecular weights between 500 and 1400 Da were discovered to have antifungal properties. P. pentosaceus has been used frequently in the fermentation of various foods, has GRAS status, and could be a suitable biocontrol organism to improve the quality of ensilage (Magnusson et al., 2003).
6.1 Reuterin (3-HPA “complex”)
Reuterin is a non-proteinaceous, water-soluble antibacterial compound made by Limosilactobacillus reuteri, L. brevis, L. buchneri, L. collinoides, L. coryniformis (Magnusson et al., 2003). It has also been generated by other bacterial genera, such as Bacillus, Citrobacter, Clostridium, Enterobacter, and Klebsiella (Vimont et al., 2019). Under anaerobic conditions, it is produced from glycerol by starving cells (Vollenweider and Lacroix, 2004; Schmidt et al., 2018), and the active reuterin (Figure 2) is not chemically homogenous being a mixture of monomeric, hydrated monomeric, cyclic dimeric forms of 3-HPA (Asare et al., 2019) and acrolein (Engels et al., 2016).
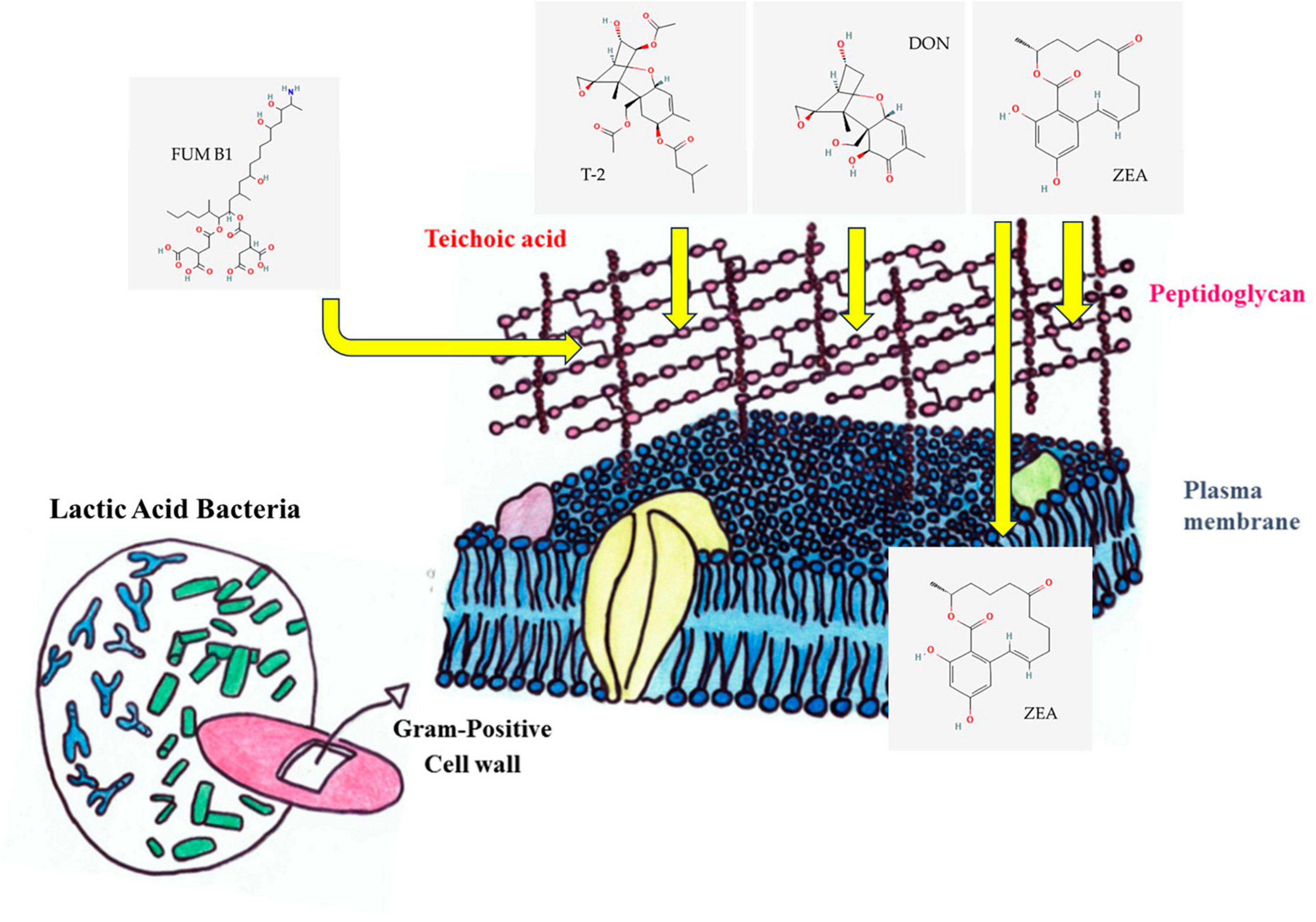
Figure 2. Process of mycotoxin binding. Zearalenone (ZEA) can stabilize hydrophobic and electrostatic interaction with cell surface proteins, adsorption to peptidoglycan, and association with intracellular proteins (Krol et al., 2018). The decrease in T2 and deoxynivalenol (DON) mycotoxins in the environment is also caused by the binding to the cell wall (El-Nezami et al., 2002b). Electrostatic effects and charges on fumonisins (FUMs) direct the toxins toward a unique binding site in the cell wall of LAB. The interaction between FB1 tricarballylic side chain and the peptidoglycan is also well known (Dawlal et al., 2019).
Genes of glycerol dehydratase are usually clustered in the pdu operon (Greppi et al., 2019) and it is sometimes also neighboring genes for cobalamin (vitamin B12) synthesis, e.g., L. reuteri CRL1089 and organized in cbi-cob operon (Morita et al., 2008; Greppi et al., 2019). A study of the complete genome sequence revealed a unique cluster of 58 genes for the biosynthesis of 3-HPA and cobalamin in the reuterin-producing L. reuteri JCM 1112T genome both characteristics are beneficial in a probiotic microbe in the intestines. These operons are arranged in the pdu-cbi-cob-hem gene cluster (Morita et al., 2008; Greppi et al., 2019).
Limosilactobacillus reuteri ATCC 55730 completely lost its 3-HPA-producing ability after five passages of the strains on the same medium under aerobic or anaerobic conditions (Kiňová Sepová and Bilková, 2013). Co-incubation of 3-HPA-producing L. reuteri strains with other bacteria like E. coli increased the amount of 3-HPA produced. E. coli-produced molecules can induce gene expression through quorum sensing (Schaefer et al., 2010) or it is induced by self-induction (Zamudio-Jaramillo et al., 2009).
3-HPA can function in a wide pH range and continue to function in the presence of different enzymes (El-Ziney et al., 1999). It has been mentioned as a potential antibacterial and antifungal agent against a wide range of microorganisms and used as a food preservative in the food industry (Ortiz-Rivera et al., 2017). 3-HPA has been studied for several of its inhibitory properties against a wide range of microorganisms and Fusaria (Rahimi-Kakolaki and Omidi, 2020). Gram-positive microorganisms were more resistant to 3-HPA than Gram-negative microorganisms and L. reuteri as a main producer itself has a moderate tolerance against 3-HPA (8.5 mM for L. reuteri ATCC 53608) (Ortiz-Rivera et al., 2017). Chung et al. (1989) measured 8.1 mM 3-HPA as a minimal inhibitory concentration MIC for Fusarium sambucinum. L. reuteri produced reuterin and showed higher activity against fungal spores of F. culmorum (L. reuteri R29; Schmidt et al., 2018). F. oxysporum (L. reuteri DSM 20016; Rodrigues et al., 2023).
The research on its antifungal action is limited; however, it was concluded that L. reuteri cells are stimulated to produce 3-HPA when are in direct interactions with other microorganisms (Chung et al., 1989). Reuterin is an electrophilic molecule and two possible, but essential pathways can lead to its cytotoxicity (i) by inducing reactive oxygen species (ROS) and (ii) by binding to thiols, modulating selective cysteine oxidation, and causing ribosomal biogenesis inhibition (Bell et al., 2022). Moreover, the reduced form of reuterin, 1,3-propanediol is a volatile compound that increases plant growth (Thomas et al., 2020).
6.2 Organic acids
Lactic acid bacteria create a variety of organic acids that are used in a variety of foods and beverages for their antibacterial and flavor-enhancing characteristics. The principal organic acids produced by LAB comprise lactic acid, propionic acid, acetic acid, hydroxyphenyl lactic acid, succinic acid, 3-phenylacetic acid, etc. (Perczak et al., 2018). By lowering the pH in the system, LAB prevent unwelcome microbial and fungal growth. However, most of the fungi prefer slightly acidic medium for their growth, but lower levels of pH attributed to these organic acids are detrimental. To create an acidic cytoplasm, lactic acid diffuses through the cell membrane in a hydrophobic state, breaks down inside the cell, and releases H+ ions. For growth and spore germination inhibition of F. oxysporum f. spp. radicis-cucumerinum all sorbic acid, propionic acid, acetic acid, butylamine, and formic acid had a strong effect (Tzatzarakis et al., 2000). Biological soil disinfestation also applies labile organic acids (acetic, butyric, isovaleric, propionic acids) that were proven to be effective against F. oxysporum f. spp. lycopersici, F. spinaciae, F. radicis-lycopersici, and Fusarium redolens (Huang et al., 2015). Lactic acid together with citric acid (5% solutions) also lowered the concentration of DON, 15Ac-DON, and NIV, while, citric acid and lactic acid treatments did not affect the concentration of some Fusarium mycotoxins, e.g., ZEA, FBs, and culmorin (Humer et al., 2016).
Phenyl lactic acid (PLA) (Figure 2) is a naturally occurring bioactive substance that exhibits broad-spectrum inhibitory activity against a variety of bacteria, fungi, molds, and yeast, including Enterococcus spp., Listeria monocytogenes, Salmonella spp., and Staphylococcus aureus (Abdul-Rahim et al., 2021; Wu et al., 2023). The synthesis of PLA in L. plantarum was regulated by LuxS/AI-2 quorum sensing mechanism (Chai et al., 2023). LAB like Lactobacillus, Weissella, and Leuconostoc typically produce PLA through the metabolism of phenylalanine. Phenylalanine is transaminated into phenyl pyruvic acid (PPA) in this process, which is then further reduced to PLA by the enzyme lactate dehydrogenase (Guan et al., 2019; Xu et al., 2021). PLA is well known for its potential antifungal activity and additionally, it can prevent asexual reproduction or the condition in fungi by the up-regulation of the pHiA gene (Ström et al., 2002; Jung et al., 2019; Xu et al., 2021). PLA is also responsible for the reduction in Fusarium growth and decrease of T-2 toxin concentration (Gerez et al., 2009; Kawtharani et al., 2020). The compound can disrupt cell membranes and interfere with mitochondrial energy metabolism (Ning et al., 2021; Zhao et al., 2023). Moreover, PLA does not kill LAB strains, instead, it inhibits their growth and is also harmful to associated pathogens, suggesting that the PLA is the best option for an antimicrobial agent (Guan et al., 2019; Abdul-Rahim et al., 2021) as an alternative to benzoic acid (Ning et al., 2021). As a result, PLA made by LAB increase food product shelf life, enhancing food safety and ultimately improving consumer health. Consequently, PLA has gained widespread acceptance in the food business as a natural preservative.
6.3 Cyclic dipeptides (CDP)
Cyclic dipeptides are secondary metabolites that have been identified from several bacterial species (Mishra et al., 2017), including L. plantarum, and Bacillus cereus subsp. thuringiensis, and Bacillus velezensis AR1 (Bayisa et al., 2021). Higher temperatures do not denature CDPs and are resistant to hydrolytic enzyme denaturation. Individual CDPs are bio-effectors; however, combined CDPs may be bioactive against various diseases. Additionally, the combination of CDPs may display cooperative antibacterial effects whether antibiotics are present or absent. As an antimicrobial activity, CDPs may prevent the synthesis of ergosterol, change the osmotic balance, make the mold membrane porous, reduce mycelial growth, and alter the nuclear DNA’s functional and structural characteristics (Kwak et al., 2014; Sellamani et al., 2016; Nasrollahzadeh et al., 2022b). Dal Bello et al. (2007) found that cyclo(L-Phe L-Pro) and cyclo(L-Leu-L-Pro) produced by L. plantarum FST1.7 have antifungal activity against F. culmorum and F. graminearum. Cyclo(L-Phe-L-Pro) and cyclo(L-Phe-trans-4-OH-L-Pro) peptides in synergy with PLA of L. plantarum MiLAB 393 were active against F. sporotrichioides and Aspergillus fumigatus molds, and Kluyveromyces marxianus yeast species and are involved in a quorum sensing mechanism (Ström et al., 2002). Cyclo(L-His-L-Pro), cyclo(L-Pro-L-Pro), cyclo(L-Met-L-Pro), cyclo(L-Leu-L-Pro), and cyclo(L-Try-L-Pro) produced by Lactobacillus amylovorus DSM 19280 (Ryan et al., 2011), while, cyclo(Gly-L-Leu) (Figure 3) produced by L. plantarum VTT E-78076 inhibited the growth of F. avenaceum in synergism with lactic acid (Niku-Paavola et al., 1999). L. lactis subsp. cremoris produced cyclo(L-Leu-L-Pro) and tetradecanoic acid with antifungal activity (Gajbhiye and Kapadnis, 2021).
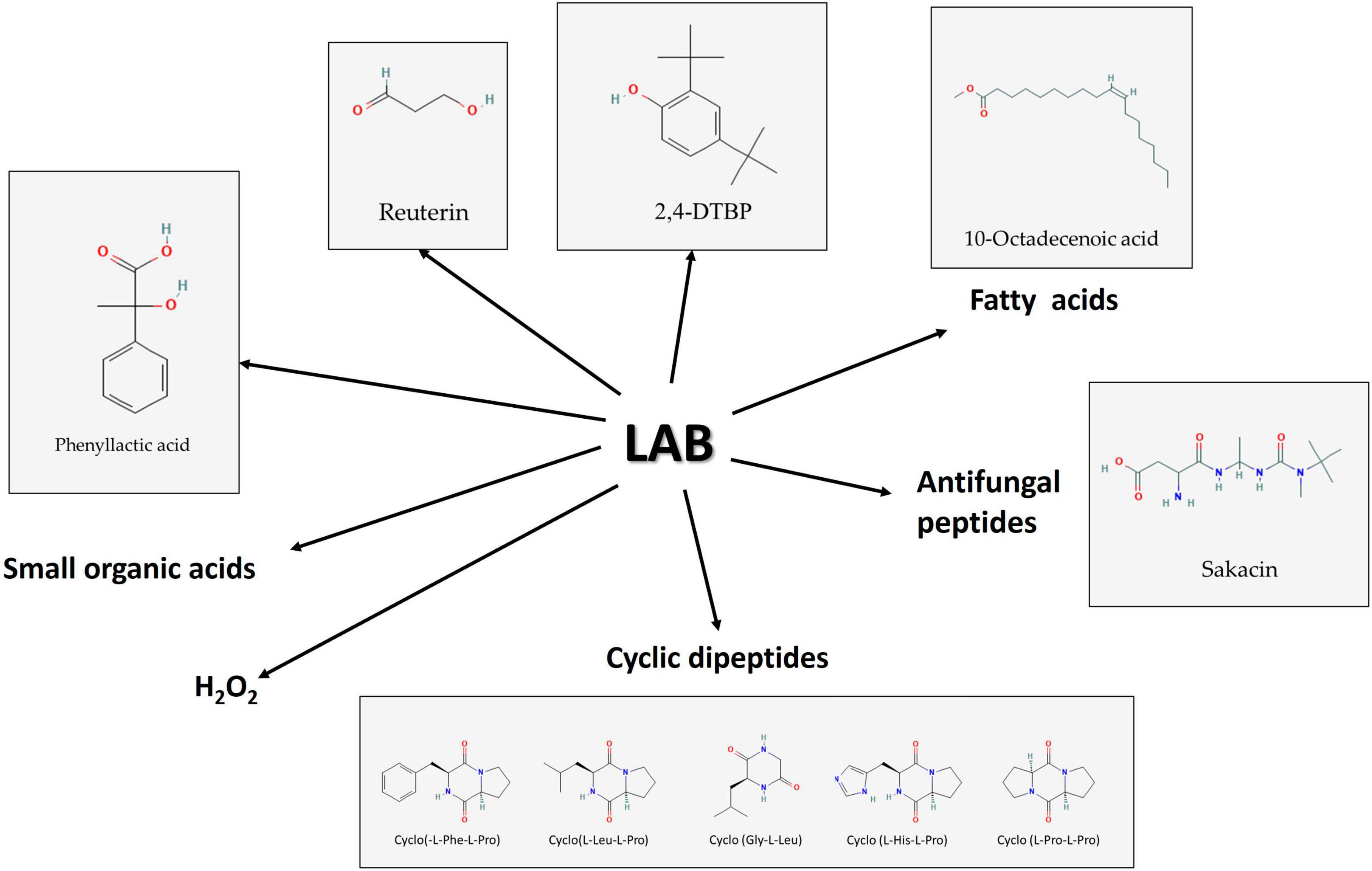
Figure 3. Some of the bioactive molecule groups produced by lactic acid bacteria (LAB) have antifungal effects against Fusaria. Source: National Center for Biotechnology Information (2023). PubChem Compound Summary. Retrieved June 1, 2023.
6.4 Antimicrobial peptides (AMP)
All living organisms produce AMPs, many of which are called antimicrobial peptides because of their relatively small size. Bacteria produce two types of AMPs: synthesized by ribosomes (bacteriocins) and AMPs that are not synthesized by ribosomes, without structural genes. LAB possess peptide transporters that act as a shuttle system to transport the peptides degraded by proteinases into the cell. Intracellular proteinases further cleave the ingested peptides into different amino acids (Zadravec et al., 2022). AMPs are polypeptide fragments with chains ranging in length from 20 to 50 amino acids that have particular antibacterial capabilities against various pathogens and are utilized as biocontrol agents in various industries.
The class of peptides known as antifungal peptides (AFPs) is responsible for the suppression of fungi, and it has been suggested that some LAB can produce AFPs (Chen et al., 2023). According to a study by Coda et al. (2011), a water-soluble antimicrobial peptide produced by a LAB strain can be utilized as a bio-preservative and can inhibit several fungi that are typically found in contaminated bread items. A similar investigation was carried out in vitro. The antimicrobial peptide called APT with 40.32 KDa from L. plantarum ALAC-4 was discovered to have strong antifungal activity by degrading cell wall and membrane in the pH range of 4-4.5, and the activity was lowered by the proteinase treatment (Chen et al., 2018). It was also found to decrease phosphorus demand and respiratory enzyme activity in Candida albicans (Wang et al., 2022).
Bacteriocins are categorized into several classes (Zendo, 2013; Cesa-Luna et al., 2021; Timothy et al., 2021) and LAB are known to produce class IIa bacteriocins which are described as small, heat-stable peptides. Several antifungal bacteriocins were identified along with some organic acids. The development of other microbes, such as bacteria, fungi, and even some viruses, can be prevented by these peptides, which are tiny, cationic molecules and are commonly utilized in fermented foods because they can dramatically reduce the growth of fungi that cause food spoilage and mycotoxin formation (Digaitiene et al., 2012; Garcha, 2018; Woraprayote et al., 2023; Figure 3).
6.5 Phenolic compounds
Phenolic compounds are chemicals found in plant systems and associated foods, comprising flavonoids, phenolic acids, and tannins. In comparison to other phenolic compounds, phenolic acids are more extensively investigated because of their diverse antibacterial and antioxidant potential. The hydroxybenzoic and hydroxycinnamic acids make up the two main types of phenolic acids based on their structural characteristics. Gallic, p-hydroxybenzoic, vanillic, protocatechuic, and syringic acids are among the most prominent hydroxybenzoic acids, whereas caffeic, p-coumaric, ferulic, and sinapic acids are important hydroxycinnamic acids (Martins et al., 2011). Phenolic acids and other organic acids are produced by a variety of microorganisms, and they are efficient food preservation agents against spoilage fungus and mycotoxigenic fungi. A study conducted by Peyer et al. (2016) using barley malt substrate fermented with L. plantarum FST1.7 and L. brevis R2Δ against F. culmorum was found to have significant inhibitory properties. Additionally, the combined action of phenolic acids and organic acids has been demonstrated to be cutting-edge food preservation methods in a variety of food firms. In a recent study, L. plantarum and P. pentosaceus, which were isolated from olive drupes, showed substantial antifungal activity against Penicillium nordicum, F. oxysporum, and Colletotrichum species. This was due to the presence of 14 different phenolic acids in the fermented broth, which shows that these cultures are effective against the fungi that can contaminate different products made from olives (Riolo et al., 2023). Using chromatographic methods and solvent extraction, the volatile chemical compound 2,4-di-tert-butyl phenol (2,4-DTBP) (Figure 3) was isolated from the cell-free supernatant of Lactococcus sp. The chemical was identified as having the molecular structure C14H22O using ESI-MS, 1H NMR, and FTIR research. It also demonstrated fungicidal efficacy against F. oxysporum (Varsha et al., 2014).
6.6 Phenazine compounds
Several bacterial species, including some LAB, have been found to produce phenazines, which are nitrogen-containing heterocyclic compounds with antifungal activity. There is limited information on the production of phenazine compounds by Firmicutes, although pyocyanin and phenazine-1-carboxamide (PCA) are examples of phenazine compounds produced by microorganisms (Jayaseelan et al., 2014). Electron microscopic analysis of PCA against Botrytis cinerea revealed mycelial withering with edge burrs, rupturing of vacuoles, and distorted structure of various organelles (Zhang et al., 2015). In a phenazine-1-carboxylic acid treatment of Pestalotiopsis kenyana phytopathogenic fungus, damage of cell membranes and mycelial formation, reduced mitochondrial membrane potential, and increased ROS levels were observed (Xun et al., 2023). A phenazine compound was obtained from Lactococcus spp. BSN307 using solvent extraction and chromatographic procedures (Varsha, 2015). It exhibited a detrimental impact on F. oxysporum and has considerable anti-oxidative and anti-cancer properties. More importantly, using molecules from bacteria with a GRAS status is an advantage when using food products directly (Varsha and Nampoothiri, 2016).
6.7 Other antifungal compounds
Due to their detergent properties, antifungal fatty acids can destroy the entire plasma membrane and cause the disintegration of the targeted cells by releasing proteins and intracellular electrolytes (Sjögren et al., 2003). From P. pentosaceus five active ingredients [9,12-octadecadienoic acid (fatty acid), 3-isobutyl-2,5-piperazine dione (antimicrobial compound), 9,12-octadecadienoic acid (Z, Z), 1-methyl ethyl ester (fatty acid ester), 9-octadecenoic acid (Z) (Figure 3), 2-hydroxy-1-(hydroxymethyl) ethyl ester (hydroxylated fatty acid ester), and pyrrolo[1,2-a]pyrazine-1,4-dione, hexahydro-7-hydroxy-phenylmethyl (a CDP)] were also detected in its antifungal fraction (Ebrahimi et al., 2020).
From the cell-free supernatant of L. plantarum MYS6, extracellular metabolites inhibited Fusarium growth and FUM production in feed. The major antifungal compounds produced by the isolate were methyl ester; palmitic acid, methyl ester; heptadecanoic acid, 16-methyl ester; stearic acid, and lauric acid besides the recently mentioned 10-octadecenoic acid (Deepthi et al., 2022).
7 Enzymatic and physical removal of Fusarium mycotoxins
As it was mentioned before, bioactive molecules produced by LAB are known to adopt strategies like adsorption, degradation, or detoxification of mycotoxins to inhibit the growth of Fusarium (Średnicka et al., 2021). Some LAB strains ensure the complete removal of mycotoxins through their binding to the cell wall (Vieco-Saiz et al., 2019; Nasrollahzadeh et al., 2022b). The enzymatic or metabolic machinery of LAB degrade or fine-tunes mycotoxins to non-toxins (Li et al., 2020; Nunes et al., 2021; Navale and Vamkudoth, 2022). The diverse composition of LAB cell walls including peptidoglycan, teichoic acids, polysaccharides, and other proteins provides an ambient platform for the adsorption of mycotoxins (Smaoui et al., 2022; Figure 4). The structural diversity of peptidoglycan cell walls and adsorption platforms helps to understand the mycotoxin binding phenomenon of the LAB (Franco et al., 2011; Sadiq et al., 2019). Four major mycotoxins FUM, ZEA, T-2, and DON interaction with cell wall components of LAB and their enzymatic degradation are discussed below.
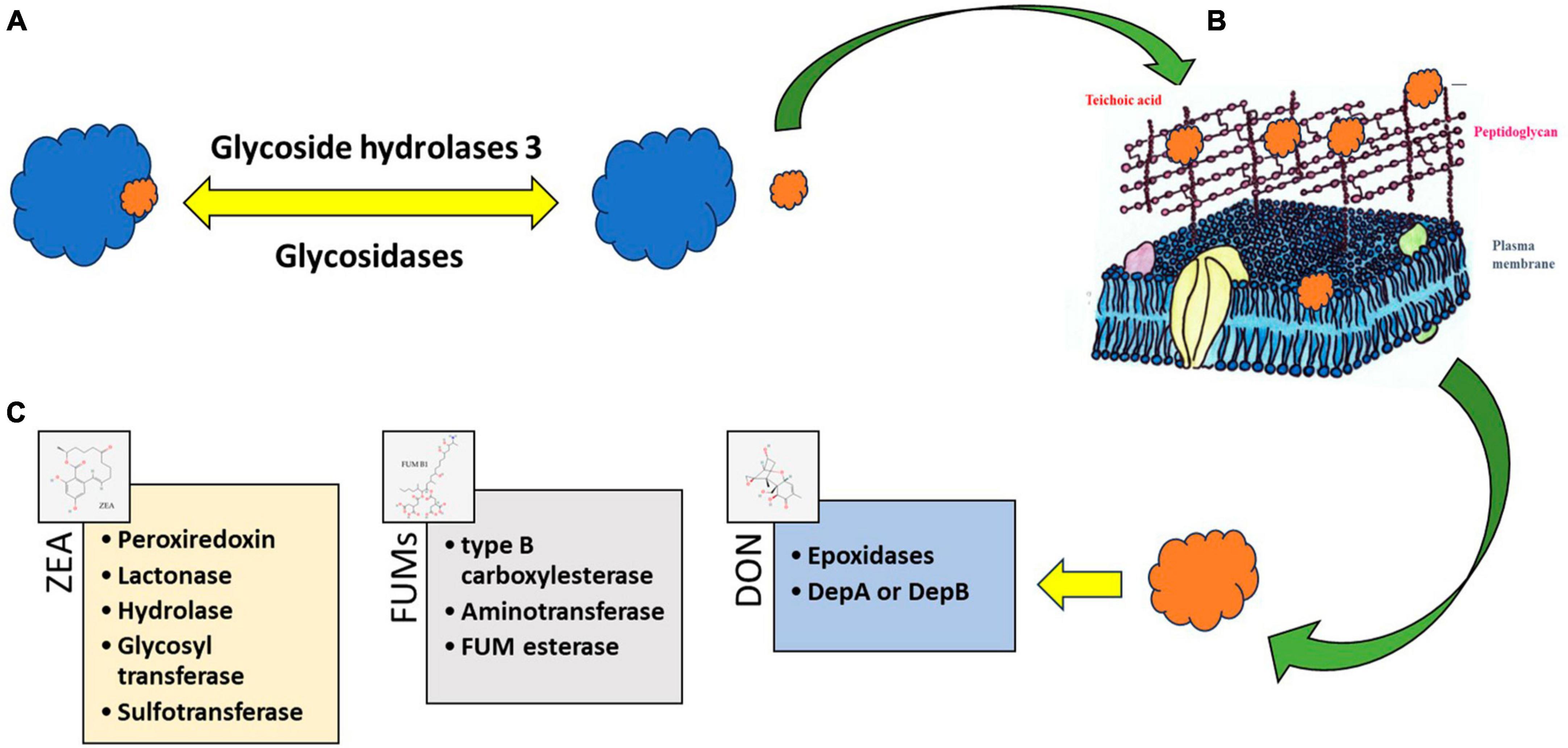
Figure 4. Processes consist of enzymatic modifications like (A) glycosidic linkages and their degradation—“freeing” of the plant-bound mycotoxins from a polymer protein or polysaccharide. The free mycotoxin can be adsorbed (B) to cell wall structures of lactic acid bacteria (LAB) or can be degraded (C) by enzymes. The degradation products become toxicologically inactivated or activated. Source: National Center for Biotechnology Information (2023). PubChem Compound Summary. Retrieved June 1, 2023.
7.1 Zearalenone (ZEA)
Glycoside hydrolase family 3, an enzyme class widely present in bifidobacteria and LAB (Michlmayr and Kneifel, 2014) can help to free the masked, glycosylated ZEA. Adsorption, electrostatic interaction, and ZEA association with inter-cell proteins can happen in ZEA-LAB interactions (Krol et al., 2018). Acid and heat-treated LAB strains, namely GG strain of L. rhamnosus and 705 strain, effectively removed α-zearalenol (α-ZOL) through adsorption. Heat-treated GG strain displayed 68% binding affinity toward ZOL and 70% affinity toward ZEA. Acid-treated strain, however, showed nearly equal binding efficiency toward both ZEA and ZOL, respectively. The control strain displayed only 50% binding efficiency toward ZOL and ZEA (El-Nezami et al., 2002a).
Bifidobacterium and L. lactis exhibited absorption levels at 88 and 90%,respectively (Krol et al., 2018). L. acidophilus CIP 76.13T and L. delbrueckii subsp. bulgaricus CIP 101027T cells removed up to 57% of ZEA (Ragoubi et al., 2021). For L. paracasei and L. lactis, the maximum sorption effectiveness was 53.3%, and 41.0%, respectively (Rogowska et al., 2019; Złoch et al., 2020).
Likewise, the cell wall of the GG strain of L. rhamnosus contains the polysaccharides that play a vital role in the binding of ZEA (Haskard et al., 2000). Polysaccharides of L. gasseri contain binding sites for pyrolysates of amino acids (El-Nezami et al., 2004).
Different microbial platforms have been explored for the identification and degradation of ZEA. Through genome engineering, lactonohydrolase from Rhinocladiella mackenziei CBS 650.93 (RmZHD) was expressed on L. reuteri CGMCC1.3264 surface (Liu et al., 2019) to gain a probiotic and mycotoxin degrading organism. Lactonohydrolase enzyme coded by the gene ZHD101 from Clonostachys rosea was expressed in Kluyveromyces lactis GG799 (Qiu et al., 2023) and Escherichia coli BL21 (Fu et al., 2021). Enzyme characterization clearly showed that the degradation of ZEA was maximum at pH 11 and a turnover number of 0.51 per second. Likewise, enzymes capable of degrading ZEA into multiple small fragments namely ZEA-1 and ZEA-2 were also identified. Peroxiredoxin, lactonase, zearalenone hydrolase, glycosyl transferase, and sulfotransferase were identified as enzymes responsible for ZEA degradation (Lyagin and Efremenko, 2019).
7.2 Trichothecenes (TCTs)
Type A TCTs, commonly known as type-2 mycotoxins, are the most toxic among the other TCTs in several foods and feeds. LAB are widely explored in the study of the mechanism of TCT in vitro, and most of the mechanism is the binding or attachment to the cell wall (Feinberg and McLaughlin, 2017). According to El-Nezami et al. (2002b), there are significant variations in LAB’ ability to attach TCT to cell walls and remove it from the fermented medium. The experiment conducted by using L. plantarum LP102 removed a 19.90 ± 1.70% of T-2 toxin after 24 h of incubation, also further studies revealed that the reduction of T2 toxin was due to the binding to the cell wall of the organism and not due to the bioconversion (Zou et al., 2012). Enzymes such as epoxidase have been reported in the destruction of the epoxy ring in TCTs and related mycotoxins (Boutigny et al., 2008; Awad et al., 2010). Several reports show the presence of the tri101 gene responsible for the enzyme that does the 3-O-acetylation reaction, which detoxifies trichothecenes via acetylation (Tokai et al., 2005; Manoharan et al., 2006; Garvey et al., 2008).
7.3 Deoxynivalenol (DON)
Natural detoxification is mainly carried out by the gastrointestinal microflora and the body metabolism initiating various biotransformation (e.g., de-epoxylation, acetylation, oxidization, etc.). Under the effects of intestinal microbiota, DON can be metabolized into its non-toxic de-epoxidized derivative, de epoxy-deoxynivalenol (DOM-1); hence, the stability and amount of microbiota in the host are quite important to the DON resistance. Polygastric species have a lower susceptibility to these toxins than monogastric animals due to their additional microflora before the colon (Maresca, 2013). In swine, DON quickly presents in their bloodstream due to the fast absorption and little biotransformation, making them very sensitive to DON (Ghareeb et al., 2015). Studies on animals implicated DON as a disruptor of reproductive performance in both genders (Yu et al., 2017).
The ability of the Lactobacillus strains to inhibit F. graminearum and DON production has been reported. Nearly 51% of DON was eliminated by L. plantarum through cell wall binding (Cao et al., 2021). Similarly, a L. paracasei strain eliminated 41% of DON through an adsorption mechanism that involved cell wall components. The strain could bind 39% DON and nearly 33% of DON was removed in less than 20 h using a phosphate-buffered solution (Zhai et al., 2019). Nearly, 35% of DON was bound by the genera Streptococcus at a concentration of 5 × 108 CFU ml–1. L. acidophilus CIP 76.13T and L. delbrueckii subsp. bulgaricus CIP 101027T cells removed up to 30% of DON (Ragoubi et al., 2021).
Enzymes of LAB degrade DON to keto moiety attached DON (DepA) or DepB (Yao and Long, 2020). The Dep A and Dep B enzymes convert DON into DepA (keto form) and further convert it into DepB with less toxicity. The glycosidase enzyme generates the glycosidic acid form of DON to reduce its highly toxic nature. Hydrolysis of oxygen present at the eighth position or acetylation of a hydroxyl group at the third carbon position was reported as another method to reduce the toxicity of DON effectively.
7.4 Fumonisins (FUMs)
Peptidoglycans present in the cell wall of LAB are considered to be the central target of FUM. Medium pH was vital in removing fumonisin B1 (FB1) and fumonisin B2 (FB2) by LAB (Niderkorn et al., 2006, 2009). Nearly 80 to 100% of FB1 and FB2 were removed when the pH of the MRS broth was maintained at 4. At neutral pH, the removal efficacy for completely reduced.
Electrostatic effects and charges on FUM direct the toxins toward a unique binding site in the cell wall of LAB. An additional hydrogen bonding with the hydroxyl group (OH–) at the 5th carbon and 10th carbon position of FB1 is absent in FB2 or FB3. FB4 displays a different structure with no hydroxyl moieties. Hence, a cage-shaped module formed due to folding interaction between carboxylic acid side chains with backbone modulates the activity of FB1, FB2, and FB3 (Dawlal et al., 2019).
The B7 strain of L. plantarum degraded FB1 to nearly 52%, whereas the efficiency increased to 58% using the strain of L. pentosus (Zhao et al., 2016). Another report portrayed the distinct role of peptidoglycans of L. pentosus for augmented affinity toward FUM (Zhao et al., 2017). Mutants with defective peptidoglycan displayed decreased FB2 only by 20–25% (Niderkorn et al., 2006).
Fumonisins detoxification is mediated by a two-step enzymatic reaction involving de-esterification to produce hydrolyzed FUM followed by deamination. Type B carboxylesterase and aminotransferase were identified as two genes responsible for the degradation of FUMs. Recombinant expression of carboxylesterase and transferase were proved to efficiently catalyze the production of hydrolyzed FB1 and deaminated FB1 in the presence of pyruvic acid (McCormick, 2013). FumD product fumonisin esterase effectively detoxified FB by hydrolysis and removal of the tricarballylic acid groups and was commercialized as FUMzyme® (Alberts et al., 2019, 2021).
8 Conclusion
The use of LAB as a biocontrol agent against phytopathogenic fungi presents both challenges and opportunities for the management and storage of food grains and other agricultural products. Food products free of mycotoxins are a major concern in food quality standards. Hence, LAB promise a prominent biological approach in the mitigation of Fusarium mycotoxins from food and feedstock, and an appropriate selection and use of LAB strains can be one of the most impacting tools in the control of toxigenic Fusarium spp. and their mycotoxins in food. Several bioactive molecules produced by LAB efficiently eliminate Fusarium mycotoxin production up to a great extent. Thus, the studies related to the identification and quantification of potent bioactive molecules from LAB contribute greatly to the quality of food grains and this review provides more insight into this based on the latest literature.
Author contributions
SK: Writing – original draft. KN: Conceptualization, Supervision, Writing – original draft, Writing – review and editing. AS: Writing – original draft. NL: Writing – original draft. PB: Writing – original draft. IP: Conceptualization, Supervision, Writing – review and editing. TP: Conceptualization, Writing – review and editing.
Funding
The author(s) declare financial support was received for the research, authorship, and/or publication of this article. The authors gratefully acknowledge the Department of Science Technology (DST), India for the financial support for the Indo-Hungary International project [DST/INT/Hun/P-27/2020(G) Dated 9.7.2021]. The project, no. 2019-2.1.13-TÉT_IN-2020-00056 has been implemented with the support provided by the National Research, Development and Innovation Fund of Hungary, financed under the 2019-2.1.13-TÉT_IN funding scheme. Project no. TKP2021-NKTA-32 has been implemented with the support provided by the Ministry of Culture and Innovation of Hungary from the National Research, Development and Innovation Fund, financed under the TKP2021-NKTA funding scheme.
Conflict of interest
The authors declare that the research was conducted in the absence of any commercial or financial relationships that could be construed as a potential conflict of interest.
The author(s) declared that they were an editorial board member of Frontiers, at the time of submission. This had no impact on the peer review process and the final decision.
Publisher’s note
All claims expressed in this article are solely those of the authors and do not necessarily represent those of their affiliated organizations, or those of the publisher, the editors and the reviewers. Any product that may be evaluated in this article, or claim that may be made by its manufacturer, is not guaranteed or endorsed by the publisher.
References
Abbès, S., Ben Salah-Abbes, J., Jebali, R., Younes, R. B., and Oueslati, R. (2016). Interaction of aflatoxin B1 and fumonisin B1 in mice causes immunotoxicity and oxidative stress: possible protective role using lactic acid bacteria. J. Immunotoxicol. 13, 46–54. doi: 10.3109/1547691X.2014.997905
Abdul-Rahim, O., Wu, Q., Price, T. K., Pistone, G., Diebel, K., Bugni, T. S., et al. (2021). Phenyl-lactic acid is an active ingredient in bactericidal supernatants of L. crispatus. J. Bacteriol. 203:e0036021. doi: 10.1128/JB.00360-21
Abu Bakar, A. I., Nur Ain Izzati, M. Z., and Umi Kalsom, Y. (2013). Diversity of Fusarium species associated with post-harvest fruit rot disease of tomato. Sains Malaysiana 42, 911–920.
Achar, P. N., and Sreenivasa, M. Y. (2021). Current perspectives of biocontrol agents for management of Fusarium verticillioides and its fumonisin in cereals—A review. J. Fungi 7:776. doi: 10.3390/jof7090776
Alberts, J. F., Davids, I., Moll, W. D., Schatzmayr, G., Burger, H. M., Shephard, G. S., et al. (2021). Enzymatic detoxification of the fumonisin mycotoxins during dry milling of maize. Food Control 123:107726. doi: 10.1016/j.foodcont.2020.107726
Alberts, J., Schatzmayr, G., Moll, W.-D., Davids, I., Rheeder, J., Burger, H.-M., et al. (2019). Detoxification of the fumonisin mycotoxins in maize: an enzymatic approach. Toxins 11:523. doi: 10.3390/Toxins11090523
Aoki, T., O’Donnell, K., and Geiser, D. M. (2014). Systematics of key phytopathogenic Fusarium species: current status and future challenges. J. Gen. Plant Pathol. 80, 189–201. doi: 10.1007/S10327-014-0509-3
Arena, M. P., Capozzi, V., Russo, P., Drider, D., Spano, G., and Fiocco, D. (2018). Immunobiosis and probiosis: antimicrobial activity of lactic acid bacteria with a focus on their antiviral and antifungal properties. Appl. Microbiol. Biotechnol. 102, 9949–9958. doi: 10.1007/s00253-018-9403-9
Asare, P. T., Zurfluh, K., Greppi, A., Lynch, D., Schwab, C., Stephan, R., et al. (2019). Reuterin demonstrates potent antimicrobial activity against a broad panel of human and poultry meat Campylobacter spp. isolates. Microorganisms 8:78. doi: 10.3390/microorganisms8010078
Audenaert, K., Vanheule, A., Höfte, M., and Haesaert, G. (2013). Deoxynivalenol: a major player in the multifaceted response of Fusarium to its environment. Toxins 6, 1–19. doi: 10.3390/Toxins6010001
Awad, W. A., Ghareeb, K., Böhm, J., and Zentek, J. (2010). Decontamination and detoxification strategies for the Fusarium mycotoxin deoxynivalenol in animal feed and the effectiveness of microbial biodegradation. Food Addit. Contam. 27, 510–520. doi: 10.1080/19440040903571747
Backhouse, D. (2014). Global distribution of Fusarium graminearum, F. asiaticum and F. boothii from wheat in relation to climate. Eur. J. Plant Pathol. 139, 161–173. doi: 10.1007/S10658-013-0374-5
Barros, G. G., Oviedo, M. S., Ramirez, M. L., and Ramirez, S. N. (2011). “Safety aspects in soybean food and feed chains: fungal and mycotoxins contamination,” in Soybean–biochemistry, chemistry and physiology, ed. N. Tzi-Bun (Rijeka: InTech Open), 7–20.
Bayisa, R. A., Cho, J. Y., and Kim, K. Y. (2021). Purification and identification of a new antifungal dipeptide from Bacillus velezensis AR1 culture supernatant. Pest Manag. Sci. 77, 775–779. doi: 10.1002/ps.6078
Bell, H. N., Rebernick, R. J., Goyert, J., Singhal, R., Kuljanin, M., Kerk, S. A., et al. (2022). Reuterin in the healthy gut microbiome suppresses colorectal cancer growth through altering redox balance. Cancer Cell 40, 185–200. doi: 10.1016/j.ccell.2021.12.001
Bergsma, S., Euverink, G. J. W., Charalampogiannis, N., Poulios, E., Janssens, T. K., and Achinas, S. (2022). Biotechnological and medical aspects of lactic acid bacteria used for plant protection: a comprehensive review. BioTech 11:40. doi: 10.3390/biotech11030040
Bernhoft, A., Torp, M., Clasen, P. E., Løes, A. K., and Kristoffersen, A. B. (2012). Influence of agronomic and climatic factors on Fusarium infestation and mycotoxin contamination of cereals in Norway. Food Addit. Contam. Part A 29, 1129–1140. doi: 10.1080/19440049.2012.672476
Boutigny, A. L., Richard-Forget, F., and Barreau, C. (2008). Natural mechanisms for cereal resistance to the accumulation of Fusarium trichothecenes. Eur. J. Plant Pathol. 121, 411–423. doi: 10.1007/s10658-007-9266-x
Broders, K. D., Lipps, P. E., Paul, P. A., and Dorrance, A. E. (2007). Evaluation of Fusarium graminearum associated with corn and soybean seed and seedling disease in Ohio. Plant Dis. 91, 1155–1160. doi: 10.1094/PDIS-91-9-1155
Bryła, M., Waśkiewicz, A., Ksieniewicz-Woźniak, E., Szymczyk, K., and Jędrzejczak, R. (2018). Modified Fusarium mycotoxins in cereals and their products—Metabolism, occurrence, and toxicity: an updated review. Molecules 23:963. doi: 10.3390/molecules23040963
Byrne, M. B., Thapa, G., Doohan, F. M., and Burke, J. I. (2022). Lactic acid bacteria as potential biocontrol agents for Fusarium head blight disease of spring barley. Front. Microbiol. 13:912632. doi: 10.3389/fmicb.2022.912632
Cao, H., Meng, D., Zhang, W., Ye, T., Yuan, M., Yu, J., et al. (2021). Growth inhibition of Fusarium graminearum and deoxynivalenol detoxification by lactic acid bacteria and their application in sourdough bread. Int. J. Food Sci. Technol. 56, 2304–2314. doi: 10.1111/ijfs.14852
Cerón-Bustamante, M., Ward, T. J., Kelly, A., Vaughan, M. M., McCormick, S. P., Cowger, C., et al. (2018). Regional differences in the composition of Fusarium head blight pathogens and mycotoxins associated with wheat in Mexico. Int. J. Food Microbiol. 273, 11–19. doi: 10.1016/j.ijfoodmicro.2018.03.003
Cesa-Luna, C., Alatorre-Cruz, J. M., Carreno-Lopez, R., Quintero-Hernandez, V., and Baez, A. (2021). Emerging applications of bacteriocins as antimicrobials, anticancer drugs, and modulators of the gastrointestinal microbiota. Pol. J. Microbiol. 70, 143–159. doi: 10.33073/pjm-2021-020
Chai, Y., Ma, Q., Nong, X., Mu, X., and Huang, A. (2023). Dissecting LuxS/AI-2 quorum sensing system-mediated phenyllactic acid production mechanisms of Lactiplantibacillus plantarum L3. Food Res. Int. 166:112582. doi: 10.1016/j.foodres.2023.112582
Chen, H., Yan, X., Du, G., Guo, Q., Shi, Y., Chang, J., et al. (2023). Recent developments in antifungal lactic acid bacteria: application, screening methods, separation, purification of antifungal compounds and antifungal mechanisms. Crit. Rev. Food Sci. Nutr. 63, 2544–2558. doi: 10.1080/10408398.2021.1977610
Chen, J., Wei, Z., Wang, Y., Long, M., Wu, W., and Kuca, K. (2021). Fumonisin B1: mechanisms of toxicity and biological detoxification progress in animals. Food Chem. Toxicol. 149:111977. doi: 10.1016/j.fct.2021.111977
Chen, L., Yang, J., Wang, H., Yang, X., Zhang, C., Zhao, Z., et al. (2022). NX toxins: new threat posed by Fusarium graminearum species complex. Trends Food Sci. Technol. 119, 179–191. doi: 10.1016/J.TIFS.2021.11.027
Chen, S.-W., Wang, H.-T., Shih, W.-Y., Ciou, Y.-A., Chang, Y.-Y., Ananda, L., et al. (2019). Application of zearalenone (ZEN)-detoxifying Bacillus in animal feed decontamination through fermentation. Toxins 11:330. doi: 10.3390/toxins11060330
Chen, Z., Li, X., and Gao, H. (2018). Production of proteinaceous antifungal substances from Lactobacillus plantarum ALAC-4 isolated from Inner Mongolian traditional fermented dairy food. Int. J. Dairy Technol. 71, 223–229. doi: 10.1111/1471-0307.12426
Chrpová, J., Šíp, V., Sumíková, T., Salava, J., Palicová, J., Štočková, L., et al. (2016). Occurrence of Fusarium species and mycotoxins in wheat grain collected in the Czech Republic. World Mycotoxin J. 9, 317–327. doi: 10.3920/WMJ2015.1917
Chung, T. C., Axelsson, L., Lindgren, S. E., and Dobrogosz, W. J. (1989). In vitro studies on reuterin synthesis by Lactobacillus reuteri. Microb. Ecology Health Dis. 2, 137–144. doi: 10.3109/08910608909140211
Cinar, A., and Onbaşı, E. (2019). “Mycotoxins: the hidden danger in foods,” in Mycotoxins and food safety, ed. S. Sabuncuoglu (Boston, MA: IntechOpen), 1–21. doi: 10.5772/intechopen.77743
Coda, R., Cassone, A., Rizzello, C. G., Nionelli, L., Cardinali, G., and Gobbetti, M. (2011). Antifungal activity of Wickerhamomyces anomalus and Lactobacillus plantarum during sourdough fermentation: identification of novel compounds and long-term effect during storage of wheat bread. Appl. Environ. Microbiol. 77, 3484–3492. doi: 10.1128/AEM.02669-10
Cope, R. B. (2018). “Trichothecenes,” in Veterinary toxicology, 3rd Edn, ed. R. C. Gupta (Amsterdam: Elsevier), 1043–1053.
Covarelli, L., Beccari, G., Prodi, A., Generotti, S., Etruschi, F., Juan, C., et al. (2015). Fusarium species, chemotype characterisation and trichothecene contamination of durum and soft wheat in an area of central Italy. J. Sci. Food Agric. 95, 540–551. doi: 10.1002/jsfa.6772
Dal Bello, F., Clarke, C. I., Ryan, L. A. M., Ulmer, H., Schober, T. J., Ström, K., et al. (2007). Improvement of the quality and shelf life of wheat bread by fermentation with the antifungal strain Lactobacillus plantarum FST 1.7. J. Cereal Sci. 45, 309–318. doi: 10.1016/j.jcs.2006.09.004
Dawlal, P., Brabet, C., Thantsha, M. S., and Buys, E. M. (2019). Visualisation and quantification of fumonisins bound by lactic acid bacteria isolates from traditional African maize-based fermented cereals, ogi and mahewu. Food Addit. Contam. Part A 36, 296–307. doi: 10.1080/19440049.2018.1562234
Deepthi, B. V., Deepa, N., Vanitha, P. R., and Sreenivasa, M. Y. (2022). Stress responses on the growth and mycotoxin biosynthesis of Fusarium proliferatum associated with stored poultry feeds. Appl. Food Res. 2:100091. doi: 10.1016/j.afres.2022.100091
Delgado, J. A., Schwarz, P. B., Gillespie, J., Rivera-Varas, V. V., and Secor, G. A. (2010). Trichothecene mycotoxins associated with potato dry rot caused by Fusarium graminearum. Phytopathology 100, 290–296. doi: 10.1094/PHYTO-100-3-0290
Digaitiene, A., Hansen, ÅS., Juodeikiene, G., Eidukonyte, D., and Josephsen, J. (2012). Lactic acid bacteria isolated from rye sourdoughs produce bacteriocin-like inhibitory substances active against Bacillus subtilis and fungi. J. Appl. Microbiol. 112, 732–742. doi: 10.1111/j.1365-2672.2012.05249.x
Ebrahimi, M., Sadeghi, A., and Mortazavi, S. A. (2020). The use of cyclic dipeptide producing LAB with potent anti-aflatoxigenic capability to improve techno-functional properties of clean-label bread. Ann. Microbiol. 70, 1–12. doi: 10.1186/s13213-020-01571-y
EFSA Panel on Contaminants in the Food Chain (Contam) (2014). Scientific opinion on the risks for human and animal health related to the presence of modified forms of certain mycotoxins in food and feed. EFSA J. 12:3916. doi: 10.2903/j.efsa.2014.3916
El-Nezami, H. S., Polychronaki, N., Salminen, S., and Mykkänen, H. (2002a). Binding rather than metabolism may explain the interaction of two food-grade Lactobacillus strains with zearalenone and its derivative α-zearalenol. Appl. Environ. Microbiol. 68, 3545–3549. doi: 10.1128/AEM.68.7.3545-3549.2002
El-Nezami, H. S., Chrevatidis, A., Auriola, S., Salminen, S., and Mykkänen, H. (2002b). Removal of common Fusarium toxins in vitro by strains of Lactobacillus and Propionibacterium. Food Addit. Contam. 19, 680–686. doi: 10.1080/02652030210134236
El-Nezami, H., Polychronaki, N., Lee, Y. K., Haskard, C., Juvonen, R., Salminen, S., et al. (2004). Chemical moieties and interactions involved in the binding of zearalenone to the surface of Lactobacillus rhamnosus strains GG. J. Agric. Food Chem. 52, 4577–4581. doi: 10.1021/jf049924m
El-Ziney, M. G., Van Den Tempel, T., Debevere, J., and Jakobsen, M. (1999). Application of reuterin produced by Lactobacillus reuteri 12002 for meat decontamination and preservation. J. Food Prot. 62, 257–261. doi: 10.4315/0362-028x-62.3.257
Engels, C., Schwab, C., Zhang, J., Stevens, M. J., Bieri, C., Ebert, M. O., et al. (2016). Acrolein contributes strongly to antimicrobial and heterocyclic amine transformation activities of reuterin. Sci. Rep. 6:36246. doi: 10.1038/srep36246
Fazeli, M. R., Shahverdi, A. R., Sedaghat, B., Jamalifar, H., and Samadi, N. (2004). Sourdough-isolated Lactobacillus fermentum as a potent anti-mould preservative of a traditional Iranian bread. Eur. Food Res. Technol. 218, 554–556. doi: 10.1007/s00217-004-0898-1
Feinberg, B., and McLaughlin, C. S. (2017). “Biochemical mechanism of action of trichothecene mycotoxins,” in Trichothecene mycotoxicosis: pathophysiologic effects, ed. V. R. Beasley (Boca Raton, FL: CRC Press), 27–36.
Ferrigo, D., Raiola, A., and Causin, R. (2016). Fusarium toxins in cereals: occurrence, legislation, factors promoting the appearance and their management. Molecules 21:627. doi: 10.3390/molecules21050627
Franco, T. S., Garcia, S., Hirooka, E. Y., Ono, Y. S., and Dos Santos, J. S. (2011). Lactic acid bacteria in the inhibition of Fusarium graminearum and deoxynivalenol detoxification. J. Appl. Microbiol. 111, 739–748. doi: 10.1111/j.1365-2672.2011.05074.x
Fu, X., Xu, M., Li, T., Li, Y., Zhang, H., and Zhang, C. (2021). The improved expression and stability of zearalenone lactonohydrolase from Escherichia coli BL21 (DE3). Appl. Biochem. Microbiol. 57, 79–85. doi: 10.1134/S0003683821010075
Gajbhiye, M., and Kapadnis, B. (2021). Lactococcus lactis subsp. cremoris of plant origin produces antifungal cyclo(Leu-Pro) and tetradecanoic acid. Indian J. Microbiol. 61, 74–80. doi: 10.1007/s12088-020-00917-z
Garcha, S. (2018). Control of food spoilage molds using Lactobacillus bacteriocins. J. Pure Appl. Microbiol. 12, 1365–1373. doi: 10.22207/JPAM.12.3.39
García, G. R., Payros, D., Pinton, P., Dogi, C. A., Laffitte, J., Neves, M., et al. (2018). Intestinal toxicity of deoxynivalenol is limited by Lactobacillus rhamnosus RC007 in pig jejunum explants. Arch. Toxicol. 92, 983–993. doi: 10.1007/s00204-017-2083-x
Garvey, G. S., McCormick, S. P., and Rayment, I. (2008). Structural and functional characterization of the TRI101 trichothecene 3-O-acetyltransferase from Fusarium sporotrichioides and Fusarium graminearum: kinetic insights to combating Fusarium head blight. J. Biol. Chem. 283, 1660–1669. doi: 10.1074/jbc.M705752200
Gerez, C. L., Torino, M. I., Rollán, G., and de Valdez, G. F. (2009). Prevention of bread mould spoilage by using lactic acid bacteria with antifungal properties. Food Control 20, 144–148. doi: 10.1016/j.foodcont.2008.03.005
Ghareeb, K., Awad, W. A., Böhm, J., and Zebeli, Q. (2015). Impacts of the feed contaminant deoxynivalenol on the intestine of monogastric animals: poultry and swine. J. Appl. Toxicol. 35, 327–337. doi: 10.1002/jat.3083
Greppi, A., Asare, P. T., Schwab, C., Zemp, N., Stephan, R., and Lacroix, C. (2019). Isolation and comparative genomic analysis of reuterin-producing Lactobacillus reuteri from poultry gastrointestinal tract. bioRxiv [preprint]. doi: 10.1101/793299
Guan, J., Han, C., Guan, Y., Zhang, S., Yun, J., and Yao, S. (2019). Optimizational production of phenyllactic acid by a Lactobacillus buchneri strain via uniform design with overlay sampling methodology. Chin. J. Chem. Eng. 27, 418–425. doi: 10.1016/j.cjche.2018.04.005
Haskard, C., Binnion, C., and Ahokas, J. (2000). Factors affecting the sequestration of aflatoxin by Lactobacillus rhamnosus strain GG. Chem. Biol. Interact. 128, 39–49. doi: 10.1016/S0009-2797(00)00186-1
Hirozawa, M. T., Ono, M. A., de Souza Suguiura, I. M., Garcia, S., Bordini, J. G., Amador, I. R., et al. (2023). Limosilactobacillus reuteri as sustainable biological control agent against toxigenic Fusarium verticillioides. Braz. J. Microbiol. 54, 2219–2226. doi: 10.1007/s42770-023-01081-4
Houssni, I. E., Khedid, K., Zahidi, A., and Hassikou, R. (2023). The inhibitory effects of lactic acid bacteria isolated from sourdough on the mycotoxigenic fungi growth and mycotoxins from wheat bread. Biocatal. Agric. Biotechnol. 50:102702. doi: 10.1016/j.bcab.2023.102702
Huang, X., Wen, T., Zhang, J., Meng, L., Zhu, T., and Cai, Z. (2015). Toxic organic acids produced in biological soil disinfestation mainly caused the suppression of Fusarium oxysporum f. sp. cubense. Biocontrol 60, 113–124. doi: 10.1007/s10526-014-9623-6
Humer, E., Lucke, A., Harder, H., Metzler-Zebeli, B. U., Böhm, J., and Zebeli, Q. (2016). Effects of citric and lactic acid on the reduction of deoxynivalenol and its derivatives in feeds. Toxins 8:285. doi: 10.3390/TOXINS8100285
Hussein, H. (2001). Toxicity, metabolism, and impact of mycotoxins on humans and animals. Toxicology 167, 101–134. doi: 10.1016/S0300-483X(01)00471-1
Jajić, I., Dudaš, T., Krstović, S., Krska, R., Sulyok, M., Bagi, F., et al. (2019). Emerging Fusarium mycotoxins fusaproliferin, beauvericin, enniatins, and moniliformin in Serbian maize. Toxins 11:357. doi: 10.3390/toxins11060357
Jayaseelan, S., Ramaswamy, D., and Dharmaraj, S. (2014). Pyocyanin: production, applications, challenges and new insights. World J. Microbiol. Biotechnol. 30, 1159–1168. doi: 10.1007/s11274-013-1552-5
Johns, L. E., Bebber, D. P., Gurr, S. J., and Brown, N. A. (2022). Emerging health threat and cost of Fusarium mycotoxins in European wheat. Nat. Food 3, 1014–1019. doi: 10.1038/s43016-022-00655-z
Jung, S., Hwang, H., and Lee, J. H. (2019). Effect of lactic acid bacteria on phenyllactic acid production in kimchi. Food Control 106:106701. doi: 10.1016/j.foodcont.2019.06.027
Juodeikiene, G., Bartkiene, E., Cernauskas, D., Cizeikiene, D., Zadeike, D., Lele, V., et al. (2018). Antifungal activity of lactic acid bacteria and their application for Fusarium mycotoxin reduction in malting wheat grains. LWT 89, 307–314. doi: 10.1016/j.lwt.2017.10.061
Karlovsky, P., Suman, M., Berthiller, F., De Meester, J., Eisenbrand, G., Perrin, I., et al. (2016). Impact of food processing and detoxification treatments on mycotoxin contamination. Mycotoxin Res. 32, 179–205. doi: 10.1007/s12550-016-0257-7
Kawtharani, H., Snini, S. P., Heang, S., Bouajila, J., Taillandier, P., Mathieu, F., et al. (2020). Phenyllactic acid produced by Geotrichum candidum reduces Fusarium sporotrichioides and F. langsethiae growth and T-2 toxin concentration. Toxins 12:209. doi: 10.3390/toxins12040209
Kimura, M., Tokai, T., Takahashi-Ando, N., Ohsato, S., and Fujimura, M. (2007). Molecular and genetic studies of Fusarium trichothecene biosynthesis: pathways, genes, and evolution. Biosci. Biotechnol. Biochem. 71, 2105–2123. doi: 10.1271/bbb.70183
Kiňová Sepová, H., and Bilková, A. (2013). Isolation and identification of new lactobacilli from goatling stomach and investigation of reuterin production in Lactobacillus reuteri strains. Folia Microbiol. 58, 33–38. doi: 10.1007/s12223-012-0166-x
Krol, A., Pomastowski, P., Rafińska, K., Railean-Plugaru, V., Walczak, J., and Buszewski, B. (2018). Microbiology neutralization of zearalenone using Lactococcus lactis and Bifidobacterium sp. Anal. Bioanal. Chem. 410, 943–952. doi: 10.1007/s00216-017-0555-8
Kwak, M. K., Liu, R., Kim, M. K., Moon, D., Kim, A. H., Song, S. H., et al. (2014). Cyclic dipeptides from lactic acid bacteria inhibit the proliferation of pathogenic fungi. J. Microbiol. 52, 64–70. doi: 10.1007/s12275-014-3520-7
Li, F., Jiang, D., Zheng, F., Chen, J., and Li, W. (2015). Fumonisins B1, B2 and B3 in corn products, wheat flour and corn oil marketed in Shandong province of China. Food Addit. Contam. Part B Surveill. 8, 169–174. doi: 10.1080/19393210.2015.1028480
Li, P., Su, R., Yin, R., Lai, D., Wang, M., Liu, Y., et al. (2020). Detoxification of mycotoxins through biotransformation. Toxins 12:121. doi: 10.3390/toxins12020121
Liu, F., Malaphan, W., Xing, F., and Yu, B. (2019). Biodetoxification of fungal mycotoxins zearalenone by engineered probiotic bacterium Lactobacillus reuteri with surface-displayed lactonohydrolase. Appl. Microbiol. Biotechnol. 103, 8813–8824. doi: 10.1007/s00253-019-10153-1
Lyagin, I., and Efremenko, E. (2019). Enzymes for detoxification of various mycotoxins: origins and mechanisms of catalytic action. Molecules 24:2362. doi: 10.3390/molecules24132362
Magnusson, J., Ström, K., Roos, S., Sjögren, J., and Schnürer, J. (2003). Broad and complex antifungal activity among environmental isolates of lactic acid bacteria. FEMS Microbiol. Lett. 219, 129–135. doi: 10.1016/S0378-1097(02)01207-7
Manoharan, M., Dahleen, L. S., Hohn, T. M., Neate, S. M., Yu, X. H., Alexander, N. J., et al. (2006). Expression of 3-OH trichothecene acetyltransferase in barley (Hordeum vulgare L.) and effects on deoxynivalenol. Plant Sci. 171, 699–706. doi: 10.1016/j.plantsci.2006.07.004
Maresca, M. (2013). From the gut to the brain: journey and pathophysiological effects of the food-associated trichothecene mycotoxin deoxynivalenol. Toxins 5, 784–820. doi: 10.3390/toxins5040784
Marín, P., Magan, N., Vázquez, C., and González-Jaén, M. T. (2010). Differential effect of environmental conditions on the growth and regulation of the fumonisin biosynthetic gene FUM1 in the maize pathogens and fumonisin producers Fusarium verticillioides and Fusarium proliferatum. FEMS Microbiol. Ecol. 73, 303–313. doi: 10.1111/j.1574-6941.2010.00894.x
Marín, S., Ramos, A. J., Cano-Sancho, G., and Sanchis, V. (2013). Mycotoxins: Occurrence, toxicology, and exposure assessment. Food Chem. Toxicol. 60, 218–237. doi: 10.1016/j.fct.2013.07.047
Martins, S., Mussatto, S. I., Martínez-Avila, G., Montañez-Saenz, J., Aguilar, C. N., and Teixeira, J. A. (2011). Bioactive phenolic compounds: production and extraction by solid-state fermentation. A review. Biotechnol. Adv. 29, 365–373. doi: 10.1016/j.biotechadv.2011.01.008
Masood, M. I., Qadir, M. I., Shirazi, J. H., and Khan, I. U. (2011). Beneficial effects of lactic acid bacteria on human beings. Crit. Rev. Microbiol. 37, 91–98. doi: 10.3109/1040841X.2010.536522
Mateo, E. M., Tarazona, A., Aznar, R., and Mateo, F. (2023). Exploring the impact of lactic acid bacteria on the biocontrol of toxigenic Fusarium spp. and their main mycotoxins. Int. J. Food Microbiol. 387:110054. doi: 10.1016/j.ijfoodmicro.2022.110054
McCormick, S. P. (2013). Microbial detoxification of mycotoxins. J. Chem. Ecol. 39, 907–918. doi: 10.1007/s10886-013-0321-0
McCormick, S. P., Stanley, A. M., Stover, N. A., and Alexander, N. J. (2011). Trichothecenes: from simple to complex mycotoxins. Toxins 3, 802–814. doi: 10.3390/toxins3070802
Medina, A., and Magan, N. (2010). Comparisons of water activity and temperature impacts on growth of Fusarium langsethiae strains from northern Europe on oat-based media. Int. J. Food Microbiol. 142, 365–369. doi: 10.1016/j.ijfoodmicro.2010.07.021
Michlmayr, H., and Kneifel, W. (2014). β-Glucosidase activities of lactic acid bacteria: mechanisms, impact on fermented food and human health. FEMS Microbiol. Lett. 352, 1–10. doi: 10.1016/j.fct.2016.11.003
Mishra, A. K., Choi, J., Choi, S. J., and Baek, K. H. (2017). Cyclodipeptides: an overview of their biosynthesis and biological activity. Molecules 22:1796. doi: 10.3390/molecules22101796
Morita, H., Toh, H., Fukuda, S., Horikawa, H., Oshima, K., Suzuki, T., et al. (2008). Comparative genome analysis of Lactobacillus reuteri and Lactobacillus fermentum reveals a genomic island for reuterin and cobalamin production. DNA Res. 15, 151–161. doi: 10.1093/dnares/dsn009
Muhialdin, B. J., Saari, N., and Meor Hussin, A. S. (2020). Review on the biological detoxification of mycotoxins using lactic acid bacteria to enhance the sustainability of food supply. Molecules 25:2655. doi: 10.3390/molecules25112655
Munkvold, G. P. (2017). “Fusarium species and their associated mycotoxins,” in Mycotoxigenic fungi. Methods in molecular biology, Vol. 1542, eds A. Moretti and A. Susca (New York, NY: Humana Press). doi: 10.1007/978-1-4939-6707-0_4
Mylona, K., Sulyok, M., and Magan, N. (2012). Relationship between environmental factors, dry matter loss and mycotoxin levels in stored wheat and maize infected with Fusarium species. Food Addit. Contam. Part A 29, 1118–1128. doi: 10.1080/19440049.2012.672340
Nasrollahzadeh, A., Mokhtari, S., Khomeiri, M., and Saris, P. E. (2022a). Antifungal preservation of food by lactic acid bacteria. Foods 11:395. doi: 10.3390/foods11030395
Nasrollahzadeh, A., Mokhtari, S., Khomeiri, M., and Saris, P. (2022b). Mycotoxin detoxification of food by lactic acid bacteria. Int. J. Food Contam. 9, 1–9. doi: 10.1186/s40550-021-00087-w
National Center for Biotechnology Information (2023). PubChem compound summary. Bethesda, MD: National Center for Biotechnology Information.
Navale, V. D., and Vamkudoth, K. (2022). Toxicity and preventive approaches of Fusarium derived mycotoxins using lactic acid bacteria: state of the art. Biotechnol. Lett. 44, 1111–1126.
Nazari, F., Sulyok, M., Kobarfard, F., Yazdanpanah, H., and Krska, R. (2015). Evaluation of emerging Fusarium mycotoxins beauvericin, enniatins, fusaproliferin and moniliformin in domestic rice in Iran. Iran. J. Pharm. Res. IJPR 14, 505–512.
Niderkorn, V., Boudra, H., and Morgavi, D. P. (2006). Binding of Fusarium mycotoxins by fermentative bacteria in vitro. J. Appl. Microbiol. 101, 849–856. doi: 10.1111/j.1365-2672.2006.02958.x
Niderkorn, V., Morgavi, D. P., Aboab, B., Lemaire, M., and Boudra, H. (2009). Cell wall component and mycotoxin moieties involved in the binding of fumonisin B1 and B2 by lactic acid bacteria. J. Appl. Microbiol. 106, 977–985. doi: 10.1111/j.1365-2672.2008.04065.x
Niku-Paavola, M. L., Laitila, A., Mattila-Sandholm, T., and Haikara, A. (1999). New types of antimicrobial compounds produced by Lactobacillus plantarum. J. Appl. Microbiol. 86, 29–35. doi: 10.1046/j.1365-2672.1999.00632.x
Ning, Y., Fu, Y., Hou, L., Ma, M., Wang, Z., Li, X., et al. (2021). iTRAQ-based quantitative proteomic analysis of synergistic antibacterial mechanism of phenyllactic acid and lactic acid against Bacillus cereus. Food Res. Int. 139:109562. doi: 10.1016/j.foodres.2020.109562
Nunes, V. M., Moosavi, M., Khaneghah, A. M., and Oliveira, C. A. (2021). Innovative modifications in food processing to reduce the levels of mycotoxins. Curr. Opin. Food Sci. 38, 155–161.
Ortiz-Rivera, Y., Sánchez-Vega, R., Gutiérrez-Méndez, N., León-Félix, J., Acosta-Muñiz, C., and Sepulveda, D. R. (2017). Production of reuterin in a fermented milk product by Lactobacillus reuteri: inhibition of pathogens, spoilage microorganisms, and lactic acid bacteria. J. Dairy Sci. 100, 4258–4268. doi: 10.3168/jds.2016-11534
Perczak, A., Goliński, P., Bryła, M., and Waśkiewicz, A. (2018). The efficiency of lactic acid bacteria against pathogenic fungi and mycotoxins. Arh. Hig. Rada Toksikol. 69, 32–44. doi: 10.2478/aiht-2018-69-3051
Peter, S. B., Qiao, Z., Godspower, H. N., Ajeje, S. B., Xu, M., Zhang, X., et al. (2022). Biotechnological innovations and therapeutic application of Pediococcus and lactic acid bacteria: the next-generation microorganism. Front. Bioeng. Biotechnol. 9:802031. doi: 10.3389/fbioe.2021.802031
Petrova, P., Arsov, A., Tsvetanova, F., Parvanova-Mancheva, T., Vasileva, E., Tsigoriyna, L., et al. (2022). The complex role of lactic acid bacteria in food detoxification. Nutrients 14:2038. doi: 10.3390/nu14102038
Peyer, L. C., Zannini, E., and Arendt, E. K. (2016). Lactic acid bacteria as sensory biomodulators for fermented cereal-based beverages. Trends Food Sci. Technol. 54, 17–25. doi: 10.1016/j.tifs.2016.05.009
Qiu, Y., Xu, H., Ji, Q., Xu, R., Zhu, M., Dang, Y., et al. (2023). Mutation, food-grade expression, and characterization of a lactonase for zearalenone degradation. Appl. Microbiol. Biotechnol. 107, 5107–5118. doi: 10.1007/s00253-023-12638-6
Qu, B., Li, H. P., Zhang, J. B., Xu, Y. B., Huang, T., Wu, A. B., et al. (2007). Geographic distribution and genetic diversity of Fusarium graminearum and F. asiaticum on wheat spikes throughout China. Plant Pathol. 57, 15–24. doi: 10.1111/j.1365-3059.2007.01711
Ragoubi, C., Quintieri, L., Greco, D., Mehrez, A., Maatouk, I., D’ascanio, V., et al. (2021). Mycotoxin removal by Lactobacillus spp. and their application in animal liquid feed. Toxins 13:185. doi: 10.3390/TOXINS13030185
Rahimi-Kakolaki, M., and Omidi, A. (2020). Effect of cell-free supernatant of Lactobacillus reuteri on the growth rate of toxigenic Fusarium oxysporum in vitro. New Findings Vet. Microbiol. 3, 11–20. doi: 10.22034/nfvm.2020.114951
Raman, J., Kim, J. S., Choi, K. R., Eun, H., Yang, D., Ko, Y. J., et al. (2022). Application of lactic acid bacteria (LAB) in sustainable agriculture: advantages and limitations. Int. J. Mol. Sci. 23:7784. doi: 10.3390/ijms23147784
Riolo, M., Luz, C., Santilli, E., Meca, G., and Cacciola, S. O. (2023). Antifungal activity of selected lactic acid bacteria from olive drupes. Food Biosci. 52:102422. doi: 10.1016/j.fbio.2023.102422
Rodrigues, F. J., Cedran, M. F., Bicas, J. L., and Sato, H. H. (2023). Inhibitory effect of reuterin-producing Limosilactobacillus reuteri and edible alginate-konjac gum film against foodborne pathogens and spoilage microorganisms. Food Biosci. 52:102443. doi: 10.1016/j.fbio.2023.102443
Rogowska, A., Pomastowski, P., Walczak, J., Railean-Plugaru, V., Rudnicka, J., and Buszewski, B. (2019). Investigation of zearalenone adsorption and biotransformation by microorganisms cultured under cellular stress conditions. Toxins 11:463. doi: 10.3390/toxins11080463
Ryan, L. A., Zannini, E., Dal Bello, F., Pawlowska, A., Koehler, P., and Arendt, E. K. (2011). Lactobacillus amylovorus DSM 19280 as a novel food-grade antifungal agent for bakery products. Int. J. Food Microbiol. 146, 276–283. doi: 10.1016/j.ijfoodmicro.2011.02.036
Sadiq, F. A., Yan, B., Tian, F., Zhao, J., Zhang, H., and Chen, W. (2019). Lactic acid bacteria as antifungal and anti-mycotoxigenic agents: a comprehensive review. Compr. Rev. Food Sci. Food Saf. 18, 1403–1436. doi: 10.1111/1541-4337.12481
Salah-Abbès, J. B., Mannai, M., Belgacem, H., Zinedine, A., and Abbès, S. (2021). Efficacy of lactic acid bacteria supplementation against Fusarium graminearum growth in vitro and inhibition of zearalenone causing inflammation and oxidative stress in vivo. Toxicon 202, 115–122. doi: 10.1016/j.toxicon.2021.09.010
Schaefer, L., Auchtung, T. A., Hermans, K. E., Whitehead, D., Borhan, B., and Britton, R. A. (2010). The antimicrobial compound reuterin (3-hydroxypropionaldehyde) induces oxidative stress via interaction with thiol groups. Microbiology 156:1589. doi: 10.1099/mic.0.035642-0
Schmidt, M., Lynch, K. M., Zannini, E., and Arendt, E. K. (2018). Fundamental study on the improvement of the antifungal activity of Lactobacillus reuteri R29 through increased production of phenyllactic acid and reuterin. Food Control 88, 139–148. doi: 10.1016/j.foodcont.2017.11.041
Schöneberg, T., Musa, T., Forrer, H. R., Mascher, F., Bucheli, T. D., Bertossa, M., et al. (2018). Infection conditions of Fusarium graminearum in barley are variety specific and different from those in wheat. Eur. J. Plant Pathol. 151, 975–989. doi: 10.1007/s10658-018-1434-7
Sellamani, M., Kalagatur, N. K., Siddaiah, C., Mudili, V., Krishna, K., Natarajan, G., et al. (2016). Antifungal and zearalenone inhibitory activity of Pediococcus pentosaceus isolated from dairy products on Fusarium graminearum. Front. Microbiol. 7:890. doi: 10.3389/fmicb.2016.00890
Shi, C., and Maktabdar, M. (2022). Lactic acid bacteria as biopreservation against spoilage molds in dairy products–A review. Front. Microbiol. 12:819684. doi: 10.3389/fmicb.2021.819684
Sjögren, J., Magnusson, J., Broberg, A., Schnürer, J., and Kenne, L. (2003). Antifungal 3-hydroxy fatty acids from Lactobacillus plantarum MiLAB 14. Appl. Environ. Microbiol. 69, 7554–7557. doi: 10.1128/AEM.69.12.7554-7557.2003
Smaoui, S., Agriopoulou, S., D’Amore, T., Tavares, L., and Mousavi Khaneghah, A. (2022). The control of Fusarium growth and decontamination of produced mycotoxins by lactic acid bacteria. Crit. Rev. Food Sci. Nutr. 62, 1–28. doi: 10.1080/10408398.2022.2087594
Soltani, S., Hammami, R., Cotter, P. D., Rebuffat, S., Said, L. B., Gaudreau, H., et al. (2021). Bacteriocins as a new generation of antimicrobials: toxicity aspects and regulations. FEMS Microbiol. Rev. 45:fuaa039. doi: 10.1093/femsre/fuaa039
Średnicka, P., Juszczuk-Kubiak, E., Wójcicki, M., Akimowicz, M., and Roszko, M. (2021). Probiotics as a biological detoxification tool of food chemical contamination: a review. Food Chem. Toxicol. 153:112306. doi: 10.1016/j.fct.2021.112306
Steglińska, A., Kołtuniak, A., Motyl, I., Berłowska, J., Czyżowska, A., Cieciura-Włoch, W., et al. (2022). Lactic acid bacteria as biocontrol agents against potato (Solanum tuberosum L.) pathogens. Appl. Sci. 12:7763. doi: 10.3390/app12157763
Streit, E., Naehrer, K., Rodrigues, I., and Schatzmayr, G. (2013). Mycotoxin occurrence in feed and feed raw materials worldwide: long-term analysis with special focus on Europe and Asia. J. Sci. Food Agric. 93, 2892–2899. doi: 10.1002/jsfa.6225
Ström, K., Sjögren, J., Broberg, A., and Schnürer, J. (2002). Lactobacillus plantarum MiLAB 393 produces the antifungal cyclic dipeptides cyclo (L-Phe-L-Pro) and cyclo (L-Phe-trans-4-OH-L-Pro) and 3-phenyllactic acid. Appl. Environ. Microbiol. 68, 4322–4327. doi: 10.1128/AEM.68.9.4322-4327.2002
Thomas, G., Withall, D., and Birkett, M. (2020). Harnessing microbial volatiles to replace pesticides and fertilizers. Microb. Biotechnol. 13, 1366–1376. doi: 10.1111/1751-7915.13645
Timothy, B., Iliyasu, A. H., and Anvikar, A. R. (2021). Bacteriocins of lactic acid bacteria and their industrial application. Curr. Top. Lact. Acid Bact. Probiotics 7, 1–13. doi: 10.35732/ctlabp.2021.7.1.1
Tokai, T., Fujimura, M., Inoue, H., Aoki, T., Ohta, K., Shibata, T., et al. (2005). Concordant evolution of trichothecene 3-O-acetyltransferase and an rDNA species phylogeny of trichothecene-producing and non-producing fusaria and other ascomycetous fungi. Microbiology 151, 509–519. doi: 10.1099/mic.0.27435-0
Tzatzarakis, M., Tsatsakis, A. M., Liakou, A., and Vakalounakis, D. J. (2000). Effect of common food preservatives on mycelial growth and spore germination of Fusarium oxysporum. J. Environ. Sci. Health Part B 35, 527–537. doi: 10.1080/03601230009373288
Uhlig, S., Eriksen, G. S., Hofgaard, I. S., Krska, R., Beltrán, E., and Sulyok, M. (2013). Faces of a changing climate: semi-quantitative multi-mycotoxin analysis of grain grown in exceptional climatic conditions in Norway. Toxins 5, 1682–1697. doi: 10.3390/toxins5101682
Van Der Lee, T., Zhang, H., Van Diepeningen, A., and Waalwijk, C. (2015). Biogeography of Fusarium graminearum species complex and chemotypes: a review. Food Addit. Contam. Part A 32, 453–460. doi: 10.1080/19440049.2014.984244
Vargas, C., Jiménez, H. R., Almario, C. G., and Almario, A. G. (2023). Effect of lactic acid bacteria on the control of Fusarium oxysporum and Ralstonia solanacearum on singly infected and co-infected tomato plants. J. Appl. Microbiol. 134:lxac053. doi: 10.1093/jambio/lxac053
Varsha, K. K. (2015). Isolation and characterization of antifungal compounds from lactic acid bacteria. Doctoral dissertation. Thiruvananthapuram: National Institute for Interdisciplinary Science and Technology (CSIR).
Varsha, K. K., and Nampoothiri, K. M. (2016). Appraisal of lactic acid bacteria as protective cultures. Food Control 69, 61–64. doi: 10.1016/j.foodcont.2016.04.032
Varsha, K. K., Priya, S., Devendra, L., and Nampoothiri, K. M. (2014). Control of spoilage fungi by protective lactic acid bacteria displaying probiotic properties. Appl. Biochem. Biotechnol. 172, 3402–3413. doi: 10.1007/s12010-014-0779-4
Vieco-Saiz, N., Belguesmia, Y., Raspoet, R., Auclair, E., Gancel, F., Kempf, I., et al. (2019). Benefits and inputs from lactic acid bacteria and their bacteriocins as alternatives to antibiotic growth promoters during food-animal production. Front. Microbiol. 10:57. doi: 10.3389/fmicb.2019.00057
Vimont, A., Fernandez, B., Ahmed, G., Fortin, H. P., and Fliss, I. (2019). Quantitative antifungal activity of reuterin against food isolates of yeasts and moulds and its potential application in yogurt. Int. J. Food Microbiol. 289, 182–188. doi: 10.1016/j.ijfoodmicro.2018.09.005
Vollenweider, S., and Lacroix, C. (2004). 3-Hydroxypropionaldehyde: applications and perspectives of biotechnological production. Appl. Microbiol. Biotechnol. 64, 16–27. doi: 10.1007/s00253-003-1497-y
Wang, S., Zhang, Y., Sun, Z., and Chen, Z. (2022). Properties and mechanism of the antimicrobial peptide APT produced by Lactobacillus ALAC-4. LWT 165:113713. doi: 10.1016/j.lwt.2022.113713
Wegulo, S. N., Baenziger, P. S., Hernandez Nopsa, J., Bockus, W. W., and Hallen-Adams, H. (2015). Management of Fusarium head blight of wheat and barley. Crop Prot. 73, 100–107. doi: 10.1016/J.CROPRO.2015.02.025
Wiśniewska, H., Stępień, Ł, Waśkiewicz, A., Beszterda, M., Góral, T., and Belter, J. (2014). Toxigenic Fusarium species infecting wheat heads in Poland. Cent. Eur. J. Biol. 9, 163–172. doi: 10.2478/s11535-013-0262-1
Woraprayote, W., Janyaphisan, T., Adunphatcharaphon, S., Sonhom, N., Showpanish, K., Rumjuankiat, K., et al. (2023). Bacteriocinogenic lactic acid bacteria from Thai fermented foods: potential food applications. Food Biosci. 52:102385. doi: 10.1016/j.fbio.2023.102385
Wu, H., Guang, C., Zhang, W., and Mu, W. (2023). Recent development of phenyllactic acid: physicochemical properties, biotechnological production strategies and applications. Crit. Rev. Biotechnol. 43, 293–308. doi: 10.1080/07388551.2021.2010645
Xu, J. J., Sun, J. Z., Si, K. L., and Guo, C. F. (2021). 3-Phenyllactic acid production by Lactobacillus crustorum strains isolated from naturally fermented vegetables. LWT 149:111780. doi: 10.1016/j.lwt.2021.111780
Xun, W., Gong, B., Liu, X., Yang, X., Zhou, X., and Jin, L. (2023). Antifungal mechanism of phenazine-1-carboxylic acid against Pestalotiopsis kenyana. Int. J. Mol. Sci. 24:11274. doi: 10.3390/ijms241411274
Yao, Y., and Long, M. (2020). The biological detoxification of deoxynivalenol: a review. Food Chem. Toxicol. 145:111649. doi: 10.1016/j.fct.2020.111649
Yu, M., Chen, L., Peng, Z., Nüssler, A. K., Wu, Q., Liu, L., et al. (2017). Mechanism of deoxynivalenol effects on the reproductive system and fetus malformation: current status and future challenges. Toxicol. In Vitro 41, 150–158. doi: 10.1016/J.TIV.2017.02.011
Zadravec, M., Markov, K., Lešić, T., Frece, J., Petrović, D., and Pleadin, J. (2022). Biocontrol methods in avoidance and downsizing of mycotoxin contamination of food crops. Processes 10:655. doi: 10.3390/pr10040655
Zamudio-Jaramillo, M. A., Hernandez-Mendoza, A., Robles, V. J., Mendoza-Garcia, P. G., Espinosa-de-los-Monteros, J. J., and Garcia, H. S. (2009). Reuterin production by Lactobacillus reuteri NRRL B-14171 immobilized in alginate. J. Chem. Technol. Biotechnol. 84, 100–105. doi: 10.1002/jctb.2012
Zapaśnik, A., Sokołowska, B., and Bryła, M. (2022). Role of lactic acid bacteria in food preservation and safety. Foods 11:1283. doi: 10.3390/foods11091283
Zendo, T. (2013). Screening and characterization of novel bacteriocins from lactic acid bacteria. Biosci. Biotechnol. Biochem. 77, 893–899. doi: 10.1271/bbb.130014
Zhai, Y., Hu, S., Zhong, L., Lu, Z., Bie, X., Zhao, H., et al. (2019). Characterization of deoxynivalenol detoxification by Lactobacillus paracasei LHZ-1 isolated from yogurt. J. Food Prot. 82, 1292–1299. doi: 10.4315/0362-028X.JFP-18-581
Zhang, G. L., Feng, Y. L., Song, J. L., and Zhou, X. S. (2018). Zearalenone: a mycotoxin with different toxic effect in domestic and laboratory animals’ granulosa cells. Front. Genet. 9:667. doi: 10.3389/fgene.2018.00667
Zhang, Y., Wang, C., Su, P., and Liao, X. (2015). Control effect and possible mechanism of the natural compound phenazine-1-carboxamide against Botrytis cinerea. PLoS One 10:e0140380. doi: 10.1371/journal.pone.0140380
Zhao, L., Jin, H., Lan, J., Zhang, R., Ren, H., Zhang, X., et al. (2015). Detoxification of zearalenone by three strains of Lactobacillus plantarum from fermented food in vitro. Food Control 54, 158–164. doi: 10.1016/j.foodcont.2015.02.003
Zhao, C., Penttinen, P., Zhang, L., Dong, L., Zhang, F., Li, Z., et al. (2023). Mechanism of inhibiting the growth and Aflatoxin B1 biosynthesis of Aspergillus flavus by phenyllactic acid. Toxins 15:370. doi: 10.3390/toxins15060370
Zhao, H., Vegi, A., and Wolf-Hall, C. (2017). Screening of lactic acid bacteria for anti-Fusarium activity and optimization of incubation conditions. J. Food Prot. 80, 1648–1656. doi: 10.4315/0362-028X.JFP-17-100
Zhao, H., Wang, X., Zhang, J., Zhang, J., and Zhang, B. (2016). The mechanism of Lactobacillus strains for their ability to remove fumonisins B1 and B2. Food Chem. Toxicol. 97, 40–46. doi: 10.1016/j.fct.2016.08.028
Zielonka, Ł, Gajęcka, M., Rozicka, A., Dąbrowski, M., Żmudzki, J., and Gajęcki, M. (2014). The effect of environmental mycotoxins on selected ovarian tissue fragments of multiparous female wild boars at the beginning of astronomical winter. Toxicon 89, 26–31. doi: 10.1016/j.toxicon.2014.06.021
Zingales, V., Fernández-Franzón, M., and Ruiz, M. J. (2021). Occurrence, mitigation and in vitro cytotoxicity of nivalenol, a type B trichothecene mycotoxin–Updates from the last decade (2010–2020). Food Chem. Toxicol. 152:112182. doi: 10.1016/j.fct.2021.112182
Złoch, M., Rogowska, A., Pomastowski, P., Railean-Plugaru, V., Walczak-Skierska, J., Rudnicka, J., et al. (2020). Use of Lactobacillus paracasei strain for zearalenone binding and metabolization. Toxicon 181, 9–18. doi: 10.1016/j.toxicon.2020.03.011
Keywords: lactic acid bacteria, Fusarium, mycotoxin, antagonism, biocontrol bioactive, biocontrol, mycotoxins
Citation: Krishnan SV, Nampoothiri KM, Suresh A, Linh NT, Balakumaran PA, Pócsi I and Pusztahelyi T (2024) Fusarium biocontrol: antagonism and mycotoxin elimination by lactic acid bacteria. Front. Microbiol. 14:1260166. doi: 10.3389/fmicb.2023.1260166
Received: 17 July 2023; Accepted: 20 November 2023;
Published: 03 January 2024.
Edited by:
Xuan Zhang, Hunan Academy of Forestry, ChinaReviewed by:
M. Y. Sreenivasa, University of Mysore, IndiaFuguo Xing, Chinese Academy of Agricultural Sciences, China
Copyright © 2024 Krishnan, Nampoothiri, Suresh, Linh, Balakumaran, Pócsi and Pusztahelyi. This is an open-access article distributed under the terms of the Creative Commons Attribution License (CC BY). The use, distribution or reproduction in other forums is permitted, provided the original author(s) and the copyright owner(s) are credited and that the original publication in this journal is cited, in accordance with accepted academic practice. No use, distribution or reproduction is permitted which does not comply with these terms.
*Correspondence: Tünde Pusztahelyi, cHVzenRhaGVseWlAYWdyLnVuaWRlYi5odQ==