- 1College of Civil and Transportation Engineering, Shenzhen University, Shenzhen, China
- 2Institute for Advanced Study, Shenzhen University, Shenzhen, China
- 3Institute for Innovative Development of Food Industry, Shenzhen University, Shenzhen, China
Foodborne diseases are caused by food contaminated by pathogenic bacteria such as Escherichia coli, Salmonella, Staphylococcus aureus, Listeria monocytogenes, Campylobacter, and Clostridium, a critical threat to human health. As a novel antibacterial agent against foodborne pathogens, endolysins are peptidoglycan hydrolases encoded by bacteriophages that lyse bacterial cells by targeting their cell wall, notably in Gram-positive bacteria due to their naturally exposed peptidoglycan layer. These lytic enzymes have gained scientists’ interest in recent years due to their selectivity, mode of action, engineering potential, and lack of resistance mechanisms. The use of endolysins for food safety has undergone significant improvements, which are summarized and discussed in this review. Endolysins can remove bacterial biofilms of foodborne pathogens and their cell wall-binding domain can be employed as a tool for quick detection of foodborne pathogens. We explained the applications of endolysin for eliminating pathogenic bacteria in livestock and various food matrices, as well as the limitations and challenges in use as a dietary supplement. We also highlight the novel techniques of the development of engineering endolysin for targeting Gram-negative bacterial pathogens. In conclusion, endolysin is safe and effective against foodborne pathogens and has no adverse effect on human cells and beneficial microbiota. As a result, endolysin could be employed as a functional bio-preservative agent to improve food stability and safety and maintain the natural taste of food quality.
1. Introduction
Food-borne sickness and death are caused by bacterial pathogens such as Escherichia coli, Salmonella, Staphylococcus aureus, Listeria monocytogenes, Campylobacter, and Clostridium, which represent an increasing problem for both the food market and healthcare system on a global basis. According to the World Health Organization (WHO), an estimated six hundred million people become sick from consuming contaminated food, and approximately 420,000 die yearly from food-borne illnesses (Kulinkina et al., 2016). This has affected the global economy to US$110 billion (Kulinkina et al., 2016). Despite increased efforts to enhance hygiene practices and spread awareness, outbreaks of food-borne illnesses have not been diminished (Bintsis, 2017). Pathogenic bacteria can cause food-borne diseases and contamination at any step in the food storage and distribution chains, such as food production, transportation, handling, and packing. To sustain the safety of the food supply chain, effective interventions for foods and the food processing environment are required. Foodstuff processing companies use various methods, such as high-pressure processing (HPP) and radiation, to reduce bacterial load in food products. However, these methods have drawbacks. HPP can produce unpleasant sensory changes in some foods, as well as cause damage to the texture of fragile food. Irradiation could negatively impact the organoleptic properties of foods (Bajovic et al., 2012; Maherani et al., 2016; Wang C.-Y. et al., 2016). Different chemicals, including acetic acid, acetates, diacetates, lactates, dehydroacetic acid, chlorine, hypochlorite, peroxyacetic acid, sodium propionate, and acidified calcium sulfate, are used as antimicrobial agents in meat, dairy, and other food products. However these chemicals leave residues of harmful compounds in food, and their use in foods has low customer interest (Sohaib et al., 2016). Antibiotics are one of the livestock’s most common antimicrobial agents. Antibiotics are widely used in poultry to help stop disease outbreaks and treat illnesses, and they are also the most commonly used feed supplements to increase mammal growth (Mehdi et al., 2018). But antibiotics kill a broad range of bacterial strains, causing the disturbance of microbiota composition in food products, especially the substantial loss of beneficial bacteria (Endersen et al., 2014). In addition, this leads to the emergence of multidrug-resistant (MDR) bacteria, a critical threat to human health and the livestock industry (Boeckel et al., 2015; Ma et al., 2021).
The uses of these methods potentially reduce customers’ satisfaction and use. As a result, it is urgently necessary to develop novel and innovative techniques for controlling food-borne bacteria. The bacteriophage-derived endolysins, in particular, provide us with an excellent natural way of developing measures to prevent food-borne pathogens (Lee et al., 2022). Bacteriophages are essential biological self-reproducing organisms and are the specific killers of bacterial cells (Oyejobi et al., 2022; Mehmood Khan et al., 2023). Endolysins are bacteriophage-derived lytic proteins and have several benefits over other antibacterial compounds, such as a high degree of activity against antibiotic-resistant bacterial pathogens, rapid bacterial lysis, high specificity for the target bacterium, low resistance potential of bacterial-resistant mutants, and good efficacy in planktonic and biofilm cells (Abdelrahman et al., 2021; Gondil et al., 2021). Endolysins have gained recognition as antibacterial agents capable of being effectively used as natural preservatives in processing and packaging systems to limit pathogens and, ultimately, increase food safety. Endolysin treatment is a cutting-edge strategy for coping with the growing problem of antibiotic-resistant bacteria in the food industry (Chang, 2020). This review described the key features of endolysins, the synthetic engineering of endolysin, the applications of endolysins against food-borne bacteria and the current challenges and future perspectives on the development of endolysin for food safety.
1.1. Mechanism of action of the endolysin
There is essentially a three-step model system of bacterial host lysis. This model is made up of three proteins: endolysin, holin, and spanin. These proteins act on the bacterial outer membrane, inner membrane, and peptidoglycan, and can cause host lysis. In Gram-negative hosts, there are two different processes for peptidoglycan degradation: (1) Holin-endolysin and (2) Pinholin SAR endolysin. Degradation of the peptidoglycan is in the preceding stage when holin makes small pores in the host inner membrane; lysis is then initiated by releasing endolysin inside the periplasm and depleting the peptidoglycan. In the final phase, lysis begins when the pinholin may induce membrane depolarization, activating the secreted SAR endolysin. Previously, it was thought that degradation of the first two membrane barriers was sufficient for the lysis of Gram-negative hosts, but current research indicates that a third functional class of lysis proteins, spanins, is now believed to be essential for outer membrane disruption. Spanins get their name from the fact that they form a protein bridge that connects both membranes. Most phages create a two-component spanin complex, which is made up of an outer membrane lipoprotein (o-spanin) and an inner membrane protein (i-spanin) containing a coiled-coil periplasmic domain. With the N-terminal lipoprotein signal and a C-terminal transmembrane domain, some phages have a novel sort of spanin that spans the periplasm as a single molecule. Furthermore, the meshwork of the peptidoglycan inhibits spanin action, thus linking the spanin step to the first two processes mediated by holin and endolysin. These proteins can cause the bacterial host cell to lysis (Cahill and Young, 2019; Abdelrahman et al., 2021). Figure 1 illustrates the mechanism of action of endolysin.
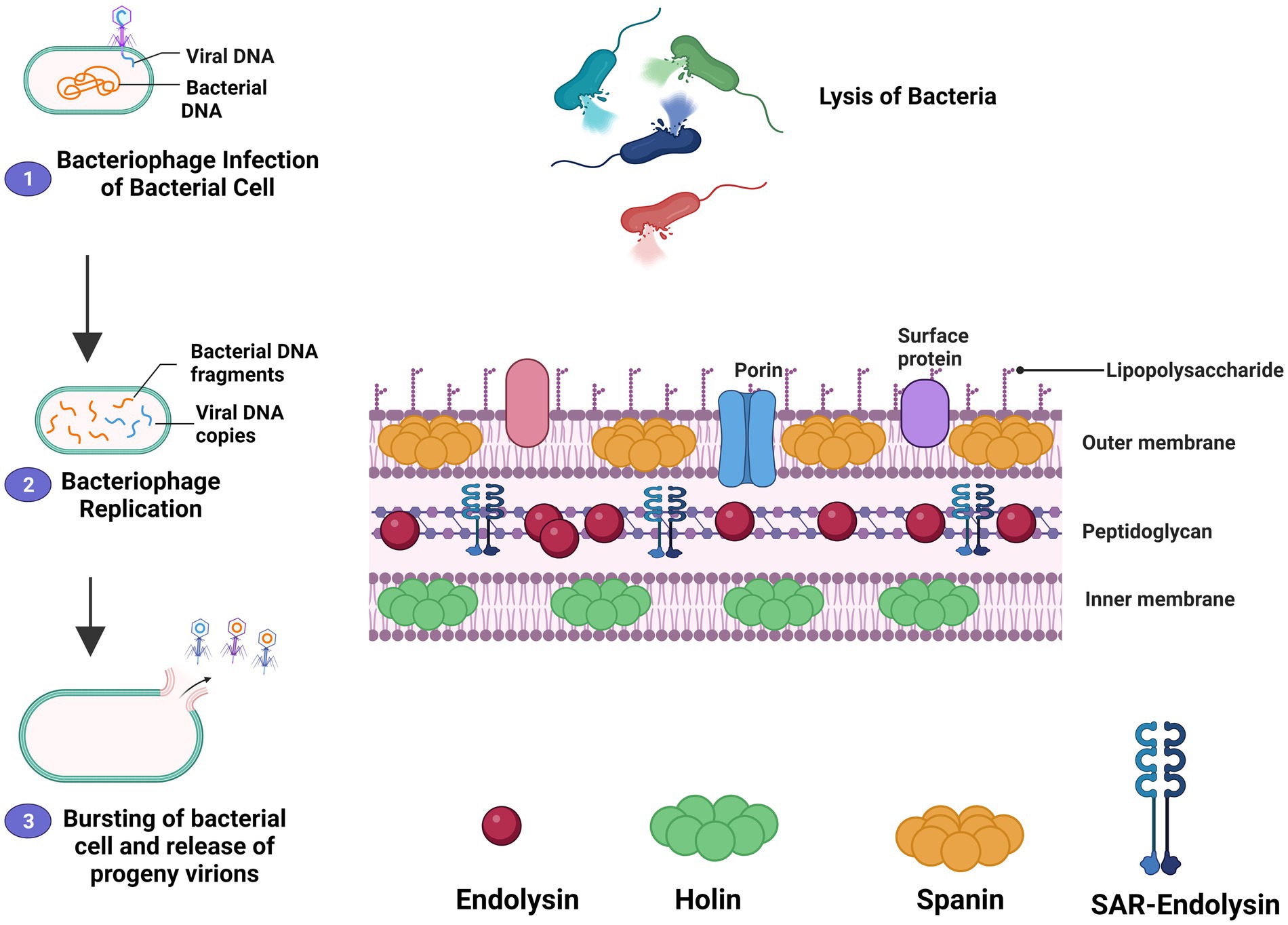
Figure 1. The mechanism of action of the lytic cycle of bacteriophage and bacteriophage-derived endolysin is described schematically. The figure shows how the holin-endolysin reaches and penetrates the Peptidoglycan layer and lysis the bacteria. The mechanism shows that the bacteriophage recognizes and attaches to bacterial-specific cell receptors through specific tail fiber protein in the first step. After attachment, the bacteriophage inserts its genetic material into the bacteria through the tail then it forms a progeny phage. The holin makes a channel for the entry and delivery of endolysin to the bacterial Peptidoglycan layer. In the last step, bacterial cell degradation occurs through endolysin.
1.2. Structure of endolysin
The majority of endolysins have a modular structure, whereas endolysins acting on Gram-negative bacteria have a basic globular structure (Oliveira et al., 2018). Modular endolysins are differentiated by the presence of one or two (multi-domain) N-terminal enzymatically active domain (EAD) linked to a C-terminal cell wall-binding domain (CBD) by a short, flexible linker region. Phages infecting Gram-positive bacteria and mycobacteria both possess such a structure (Oliveira et al., 2018; Gondil et al., 2021). The N-terminal EAD of modular endolysins cleaves specific peptidoglycan bonds in the host bacterium’s murein layer, whereas the C-terminal CBD detects and attaches to various epitopes in the cell wall for proper binding of the EAD’s catalytic effects (Matamp and Bhat, 2019). The bacteriophage-derived endolysins of Gram-negative hosts can be organized in different ways, but the majority of Gram-negative endolysins contain a basic globular EAD domain without a CBD (Briers et al., 2007). The new investigation additionally found Gram-negative endolysins with globular structures, with one or two CBDs at the N-terminus and the EAD module at the end of the C-terminal region (Briers et al., 2007).
1.3. Classification and characterization of endolysin
Endolysins are classified according to their location of cleavage position. The categories of these enzymes are lysozymes (N-acetylmuramidases), glycosidases (N-acetyl--d-glucosamidases), N-acetylmuramoyl-l-alanine amidases, and L-alanoyl-d-glutamate endopeptidases (Fenton et al., 2010; Abdelrahman et al., 2021). Phage-encoded cell wall hydrolases are a general word that could be used to describe these enzymes. Generally, endolysins consist of one of these four N-terminals linked to a different cell wall-binding domain (Murray et al., 2021).
1.3.1. Glycosidases
Glycosidases break the −1,4 glycosidic linkages that connect the peptidoglycan layer’s changing polymeric structures of N-acetylmuramic acids (MurNAc) and N-acetylglucosamines (GlcNAc). N-acetyl--D-muramidases, which split bonds within MurNAc and GlcNAc; N-acetyl--D-glucosidases, which split bonds within GlcNAc and MurNAc residues (Matamp and Bhat, 2019). Transglycosylases, like the other two glycosidases, cleave −1,4 bonds within MurNAc and GlcNAc, but they are additionally involved in an intramolecular process that leads to the formation of a 1,6-anhydro ring at the MurNAc residue (Rodríguez-Rubio et al., 2016). Bacteria are killed by lysozymes (N-acetylmuramidases) through specific hydrolysis. N-acetyl-β-d-glucosamidases, also known as glycosidases, regulate the hydrolysis of the glycosidic bond. The NAG (N-acetylglucosamine) and NAM (N-acetylmuramic acid) monomers of peptidoglycan polymers are linked together by β-1,4 glycosidic bonds. By hydrolyzing these linkages, lysozyme acts to disrupt the peptidoglycan cell wall’s structural integrity. This causes the turgor pressure to become unbalanced, which leads to the lysis of the bacterial cell (Ragland and Criss, 2017).
1.3.2. Amidases
Peptidoglycan amidases, also known as N-acetylmuramoyl-L-alanine amidases, act by breaking the amide bond that splits the glycan strand from the stem peptide situated between the L-alanine and N-acetylmuramic acid residues (Abdelrahman et al., 2021).
1.3.3. Endopeptidases
Endopeptidases are enzymes that break the bonds between two amino acids in the stem peptide. The peptide made up of the L-alanoyl-d-glutamate link is the target of interpeptide bridge-specific endopeptidases and L-alanoyl-d-glutamate endopeptidases. Bond cleavage can occur within an interpeptide bridge or between stem peptides (Rodríguez-Rubio et al., 2016).
2. The significant features of endolysin
2.1. Broad lytic spectrum
Endolysin is mainly active in Gram-positive bacteria that lack a protective outer cell membrane. The existence of an outer membrane in Gram-negative bacteria limits but does not entirely prevent the function of endolysin (Murray et al., 2021). Endolysin often has a broader range of specificity than phages, and their specificity is typically at the genus or species level. Endolysins’ selectivity for bacterial targets is due to their cell wall-binding domains, which detect and bind receptors independently to a specific substrate within the target cell wall. Due to this reason, Gram-negative lysins have a wider range of targets, while Gram-positive targeted lysins often have a narrower host range (Ajuebor et al., 2016). Endolysins with broad-spectrum activity against Gram-positive bacteria have been reported. A pneumococcal bacteriophage-derived lytic endolysin Pal can eradicate 15 prevalent pneumococcal serotypes, even those with a high level of penicillin resistance (Loeffler et al., 2001). The endolysin phi11 shows potent antimicrobial activities against various Staphylococcus strains including S. aurues, S. epidermidis, S. hyicus, S. simulans, S. xylosus and S. wraneri (Donovan et al., 2006). In many other studies, the endolysins’ efficacy against Gram-positive bacteria has been reported including ClyR, ClyJ, ClyV, ClyC, SAL200, LysK, and Cpl-1 (Yang et al., 2015a; Seijsing et al., 2018; Bae et al., 2019; Gondil et al., 2020a; Huang et al., 2020; Yang H. et al., 2020; Li X. et al., 2021). Some of the examples of endolysin showed broad-spectrum activity against Gram-negative bacteria. The endolysins LysAB54, LysP53 and LysPA26 showed antibacterial activity against multidrug-resistant Gram-negative bacteria such as A. baumannii, Pseudomonas aeruginosa, Klebsiella pneumoniae, and Escherichia coli (Guo et al., 2017; Khan et al., 2021; Li C. et al., 2021). The reported endolysin Abtn-4 and LysSS has a wide range of bactericidal activity against a wide range of Gram-positive and Gram-negative bacteria, including E. coli, A. abauminii, P. aeruginosa, K. pneumoniae, Salmonella spp. and S. aureus (Kim et al., 2020; Yuan et al., 2021).
2.2. Less resistance
Endolysins are regarded as persistent therapeutic agents in terms of bacterial resistance development. Their specificity for a bacterial species or genus gives them several advantages over broad-spectrum antibiotics. The co-evolution of phages with their host bacterium has brought numerous benefits, including conserved binding ability and activity, as well as a rare possibility of mutation in binding sites, making resistance to endolysins a remarkable finding. Because the majority of antimicrobial resistance mechanisms are within the cell, the extracellular application of endolysins and the presence of target peptidoglycan on the cell’s outer surface prevent the development of resistance to endolysin (Gondil et al., 2020b). The repeated exposure of Streptococcus pneumoniae to endolysin Pal at a low concentration shows no resistance (Loeffler et al., 2001). Experiments were performed and attempted to bring resistance in Bacillus spp. against endolysin PlyG and methicillin-resistant S. aureus against endolysin ClyS. Still, no resistance occurred in both bacteria against their corresponding endolysins, while antibiotic resistance occurred in these bacterial strains using the same methodology (Schuch et al., 2002; Pastagia et al., 2011). There is no published report on the resistance of bacteria to endolysin.
2.3. No harmful impact on the normal microbiota
To protect its host from attacking pathogens, the normal microbiota develop a physical barrier (Cash et al., 2006). Any disruption in the natural microbiome can lead to significant disease (Wang et al., 2017). Antibiotics facilitate human health and life span; yet, broad-spectrum antibiotics disturb the existing microbiota, resulting in dysbiosis and adverse effects (Keeney et al., 2014). Endolysins, compared to typical broad-spectrum antibiotics, can target selected bacterial species with minimal adverse effects on the surrounding microbiome, and the absence of bacterial resistance mechanisms has also been reported as a benefit of the use of endolysin. Because of these characteristics, endolysins have been allocated to a new class of antimicrobial agents known as enzybiotics (Murray et al., 2021). PlyV12, a bacteriophage-derived endolysin showed a strong lytic activity against the vancomycin-resistant strains of Enterococcus faecalis and E. faecium. Endolysin causes less disruption of the normal microbiota because they do not transfer resistance genes or bacterial toxins that harm the colonizing bacteria of mucous membranes (Rahimzadeh et al., 2018). They can stimulate the immune system without neutralizing or inhibiting antibacterial action. As a result, they can be used to treat systemic infections (Fischetti, 2008). Imanishi et al. (2019) investigated the therapeutic potential of a recombinant S25-3 against S. aureus. They found out, firstly, that the recombinant endolysin S25-3 was effective against S. aureus. Secondly, in an experimental mouse model of impetigo, topical administration of recombinant S25-3 endolysin reduced the number of intraepidermal Staphylococci. Thirdly, in the same mice, treatment with recombinant S25-3 endolysin boosted the diversity of the skin microbiota. The Pal in-vivo analysis showed that microbial species commonly present in the human oropharynx were not affected by the Pal endolysin (Loeffler et al., 2001). These findings showed that endolysin has no adverse effect on the human microbiota.
2.4. Safety
Bacteriophages are part of the human microbiome. Hence bacteriophage-derived endolysin is unlikely to harm human health (Navarro and Muniesa, 2017; Kirsch et al., 2021). Endolysins can surpass the immune system due to their fast action and specific receptors of the CBD on its target host (Jado et al., 2003). Still, their eventual acceptance as antimicrobial agents for the general public heavily depends on their immunogenicity, how the body’s immune system will respond to them, and their safety assessment on human health (Abdelrahman et al., 2021). In this regard, several studies show endolysins’ safety assessment. The safety and toxicity of two pneumococcal endolysins, Pal and Cpl-1 were investigated in human macrophages and pharyngeal cells. Similarly, no physical or behavioral changes were observed in mice injected with 15 mg/kg of each endolysin. IgG levels in mice exposed to the Streptococcus pneumoniae endolysins Cpl-1 and Pal increased. However, IgE levels remained low, suggesting a low probability of hypersensitivity or allergic reactions. Additionally, no adverse health consequences in mice with increased levels of pro-inflammatory cytokines or complement activation were seen, suggesting that these endolysins had good safety and toxicity profiles. The Pal and Cpl-1’s endolysin safety and toxicity characteristics justify the safe use of endolysin (Harhala et al., 2018). PlyCD1-174 is effective against Clostridium difficile spores infecting the large intestine in the presence of its intestinal contents. The PlyCD1-174 was injected into the colons of C. difficile-infected mice and 400 μg of endolysin delivered via the colon had no abnormal adverse effects, such as diarrhea or mice weight loss (Wang et al., 2015). In rodent single-and repeated-dose toxicity trials, intravenously administered SAL200 revealed no adverse effect. There were no adverse findings in the dog repeated-dose toxicity test, except temporary abnormal clinical symptoms found in certain dogs when daily injection of SAL200 was continued for more than one week. All aberrant findings found in these safety review investigations were minor and were rapidly recovered and no animal deaths were reported in the safety assessments (Jun et al., 2014). In a clinical trial, healthy male volunteers were administered the endolysin SAL200, and the results showed good endolysin tolerance in human subjects. Therefore, endolysins have an excellent safety profile and revealed no side effects like fever, stomach cramps, or diarrhea in preclinical studies conducted using animal models, demonstrating their safety aim (Jun et al., 2017). The safety and immunogenicity efficacy of endolysin ClyR was investigated in a systemic Streptococcus agalactiae infection mouse model. The ClyR rescued the mice and had no harmful effects on the mice (Yang et al., 2015a). The endolysin MV-L safety activity is evaluated in the mice. The mice who received a single high dosage of endolysin followed by a repeat dose did not show any side effects or a change in their survival rate (Rashel et al., 2007). The endolysin PlyC, immunogenicity, and allergic reactions were tested in mice and human serum samples from healthy volunteers. Mice were challenged with PlyC, and humans lacked PlyC-specific IgE. PlyC was immunogenic but did not cause hypersensitivity. Furthermore, adverse effects, particularly those indicating hypersensitivity, were closely monitored, and no adverse reactions were recorded. These findings significantly support PlyC’s safety as a possible therapeutic enzyme that can be applied to treat severe bacterial infections (Harhala et al., 2022). The endolysin LysGH15 is tested for the influence of endolysin inactivation by antibodies. LysGH15-specific antibodies did not impact LysGH15’s ability to kill MRSA in vitro or in vivo (Zhang et al., 2016). The high safety of endolysins could be because endolysin is very specific for distinctive and conserved structures of bacterial cell wall peptidoglycan or polysaccharides that are not present in mammalian cells (Nau and Eiffert, 2002; Nelson et al., 2012). Although the findings of safety studies to date support the claim of endolysins as a potential safe antibacterial agent. More research in this area is needed to confirm that different classes of endolysin do not pose a significant concern to the host (Murray et al., 2021).
3. The applications of endolysins for food safety
3.1. The applications of endolysin against bacterial biofilm
Biofilms are microorganism communities that are sessile and embedded in a self-produced extracellular environment, being the most widespread bacterial lifestyle (Qian et al., 2022). The well-organized structure of these multilayer colonies guards bacterial cells against external threats, improving their ability to tolerate antibiotic or disinfectant treatment. These colonies are identified by enhanced antibiotic resistance in both hospitals and foods. They are a persistent cause of re-infection and re-contamination of foods (Abee et al., 2011; Fernández et al., 2018). Bacterial biofilm formation is a defense mechanism that bacteria use to deal with unfavorable conditions such as food scarcity or toxic antimicrobial dosage. Antimicrobial drugs often have a limited effect on biofilms, which is hypothesized to be owing to several biofilm-specific features. Low growth rates hamper antibiotics, and evidence showed that sessile bacteria in biofilms have a different metabolic state. Antibiotics’ diffusion velocity is also restricted within biofilms. Reduced antibiotic penetration increases the antibiotic concentration in the biofilm’s deeper layers, allowing biofilm cells to adapt to the antibiotic through stress-induced metabolic and transcriptional changes (Srinivasan et al., 2021). Because of their considerable resistance, the S. aureus biofilms are recognized as a serious concern in the food industry. The reported endolysins LysCSA13, ϕ11, Lys109, LysP108, XZ.700 and LysK did the eradication of bacterial cells of the S. aureus and eliminated its biofilms from different surfaces, including polystyrene, glass, and stainless steel. These endolysins can be used as a potential biocontrol agent in the different food processing sectors (Sass and Bierbaum, 2007; Schmelcher et al., 2015; Cha et al., 2019; Kuiper et al., 2021; Lu et al., 2021; Son et al., 2021).
The L. monocytogenes and Salmonella are also increasingly pressing issues for food industries because of their biofilm formation. The recombinant endolysins PlyLM and 293-amidase showed anti-biofilm activity against L. monocytogenes while the endolysin Lys68 showed a synergistic biofilm-reducing activity against the salmonella in combination with malic or citric acid. Lys68 has good thermo-stability properties so it can be an alternative antibacterial protein against Gram-negative pathogens in the food industry (Simmons et al., 2012; Oliveira et al., 2014; Pennone et al., 2019).
3.2. Endolysin applications for veterinary
Antibiotic misuse and overuse have resulted in a significant emergence of resistant bacteria in meat-producing animals, particularly cattle, poultry, and pigs, which have been identified as crucial carriers of antibiotic-resistant bacteria and their particular genes, which can transfer to humans through direct contact or indirectly through foods (Marshall and Levy, 2011). In response to increasing concern over antibiotic misuse in food-producing animals, many large food companies have agreed to eliminate antibiotic use and improve control over alternative treatments. This creates complex scenarios for the animal-husbandry sector, which can be overcome using bacteriophage-derived endolysins (Love et al., 2018). The poultry market has faced severe problems due to Clostridium perfringens, which can infect 95% of poultry (Timbermont et al., 2011). The endolysin phiSM101 showed a wide range of bactericidal activity against C. perfringens (Tamai et al., 2014). Salmonella can cause widespread disease in chickens, and it causes economic losses in the poultry business (Wernicki et al., 2017). The endolysin LysSE24 derived from the Salmonella bacteriophage LPSE1, showed antibacterial activity against the Salmonella strains (Ding et al., 2020).
3.3. Endolysin applications for meat
Meat is a significant carrier of foodborne infections (Bantawa et al., 2018). The endolysin LysSA11 showed a 3-log CFU/mL of methicillin-resistant S. aureus (MRSA) bacterial reduction in ham meat at a refrigerator temperature of 4°C (Chang et al., 2017a). The endolysin Trx-SA1 effectively reduce S. aureus in the cow udders. Trx-SA1 can be used as an alternate bio-preservative to minimize S. aureus contamination during food storage (Fan et al., 2016).
3.4. Endolysins applications for dairy products
Dairy-borne pathogens, such as L. monocytogenes, S. aureus, Salmonella, and Shiga toxin-producing E. coli (STEC), should be controlled in the dairy production industry. The lysis activities of endolysins have been investigated particularly in dairy products (Lee et al., 2022). S. aureus has been effectively eliminated by several endolysins in milk (Obeso et al., 2008; García et al., 2010; Chang et al., 2017a). The endolysins LysH5, LysSA97 and LysSA11 were tested in milk which showed strong bactericidal activity against S. aureus. LysH5 revealed a synergistic bactericidal activity in pasteurized milk when used in combination with nisin. These antimicrobial proteins can be effective milk preservative candidates (Obeso et al., 2008; García et al., 2010; Chang et al., 2017a,b). The C. tyrobutyricum has been linked to the late blowing of hard and semihard cheese. Even minute quantities of heat-resistant spores can result in food spoilage during pasteurization, processing, and canning procedures. The C. tyrobutyricum phage ϕCTP1 endolysin Ctp1L is an antibacterial protein that showed activity in milk and prevents late blowing during cheese manufacturing (Mayer et al., 2010). Listeria contamination must be eliminated when making fresh cheese since its delicate taste and textures are inconsistent with several antimicrobial treatments and chemicals commonly used in other foodstuffs. The endolysin PlyP100 can potentially prevent the spread of L. monocytogenes in fresh cheese (Van Tassell et al., 2017). The endolysins LysZ5, ply511, and ply118 lysis the L. monocytogenes in milk. LysZ5 has good host specificity and host lysis activity at refrigerator conditions. ply511 and ply118 showed broad-spectrum activity and had high thermal resistance (Zhang et al., 2012; Schmelcher et al., 2012c). The endolysin ply100 showed synergistic bactericidal activity in combination with nisin against the Listeria in the Queso Fresco cheese (Ibarra-Sánchez et al., 2018). Endolysins are also tested against foodborne Listeria in milk, soy milk and Queso Fresco cheese (Zhang et al., 2012; Schmelcher et al., 2012c; Ibarra-Sánchez et al., 2018).
3.5. Endolysins applications in vegetables
To reduce and prevent the antibiotic resistance pathogenic bacteria in vegetables, endolysin has also been tested in vegetable products. Several endolysins have been proposed as possible antibacterial bio-preservative agents for vegetable products against L. monocytogenes in iceberg lettuce (Schmelcher et al., 2012a). The endolysin LysP53 can lysis Salmonella enteritidis on fresh romaine lettuce and can be employed as a biocontrol agent to lower bacterial burdens in fresh vegetable food due to its good activities against a wide range of bacteria, thermal stability, and safety (Li et al., 2023). The endolysin cpp-lys greatly lysis the bacteria C. perfringens which produced various toxins. The activity is checked and confirmed on lettuce. The results of this investigation showed that endolysin cpp-lys has potential uses in the food industry for the control of C. perfringens (Zhao et al., 2023). LysWL59 and LysWL60, are potential bacteriophage LPST10-derived endolysins, which revealed extensive lytic activity and diminished viable S. typhimurium on lettuce (Liu et al., 2019).
3.6. Endolysins and its CBD for fast detection of pathogenic bacteria in food
To ensure food safety, sensitive and specific diagnostic protocols that accurately and rapidly detect pathogenic bacteria in food are essential (Hagens and Loessner, 2007). A useful tool for the detection of a particular food-borne bacteria is CBD which has been fluorescently tagged (Loessner et al., 2002). CBDs derived from bacteriophage-derived endolysins are excellent choices for making use of novel detection tools due to their high degree of specificity and binding affinity. CBDs from Listeria phage endolysins are a prime example, as their binding affinity to the target cells closely resembles or exceeds antigen–antibody interactions (Loessner et al., 2002; Schmelcher et al., 2010). Furthermore, CBDs have highly specific yet varied binding spectra, ranging from the level of the genus to the level of the serovar or even the level of the strain, providing new opportunities for the detection, immobilization, and differentiation of cells (Schmelcher et al., 2010). The endolysin LysCPAS15 inhibited the bacterial cells by up to a 3-log reduction, and enhanced green fluorescent protein EGFP-fused CBD protein (EGFP-LysCPAS15_CBD1) detects C. perfringens (Cho et al., 2021). A novel method for detecting S. aureus in milk is developed by combining immunomagnetic separation with the enzyme-linked CBD of endolysin plyV12, which binds S. aureus with high affinity, tested in the contaminated milk. On the cell surface of S. aureus, there are millions of binding sites for CBD attachment, which significantly improves the detection sensitivity. This method could be used to detect S. aureus in different food samples due to its simplicity, fast detection, and high sensitivity (Yu et al., 2016). Fused CBDs were developed to target and detect other bacterial pathogens, such as B. cereus and L. monocytogenes (Ahmed et al., 2007; Eugster et al., 2011; Kong and Ryu, 2015). However, due to the outer membrane barrier, Gram-negative food-borne pathogens cannot be detected by CBD, which is a significant drawback. CBD cocktails can recognize numerous food-borne bacterial pathogens that are present in a food sample at once by using various colored fluorescent tags. For instance, a multiplex decorating with CBDs allowed the identification of various serovar groups of Listeria. The CBD-P35 and CBD500 were able to recognize various Listeria strains in both milk and camembert cheese samples when they were labeled with different fluorescent markers including RedStar and GFP (green fluorescent protein) (Schmelcher et al., 2010). Due to high specificity and binding activity for pathogenic bacteria, CBD is a perfect option to replace antibodies in the rapid detection of bacterial pathogens (Bai et al., 2016). Even though there has been tremendous advancement in the use of endolysins or their CBDs for detecting pathogenic bacteria in food, future studies should focus on further investigation of endolysins or their CBDs into the quickly developing biosensor technology (Schmelcher and Loessner, 2016).
4. The use of endolysins for the prevention of foodborne bacterial pathogens
4.1. Salmonella
Salmonella is usually recognized as one of the most significant and widespread foodborne pathogens in the entire world. Salmonella has over 2,000 serotypes, some of which can cause human enteric infections, also known as salmonellosis (Majowicz et al., 2010). Salmonella nontyphoidal, which causes food poisoning outbreaks, is China’s second most common foodborne pathogen (Fu et al., 2019). LysSTG2, En4 and SPN9CC bacteriophage-derived endolysins showed bactericidal activity against S. typhimurium. The LysSTG2 (100 μg/mL) and slightly acidic hypochlorous water (40 mg/L of chlorine) eliminated more than 99% of the S. typhimurium. En4 showed a reduction of S. typhimurium 1.0–1.6 log CFU/g in frozen and thawed raw chicken meat with the addition of 0.1% NaHCO3. These results suggested that using endolysin together with permibilizers could be a novel and effective way to combat S. typhimurium in the food industry (Lim et al., 2014; Zhang et al., 2021; Abhisingha et al., 2023). Figure 2 describes the different applications of endolysin.
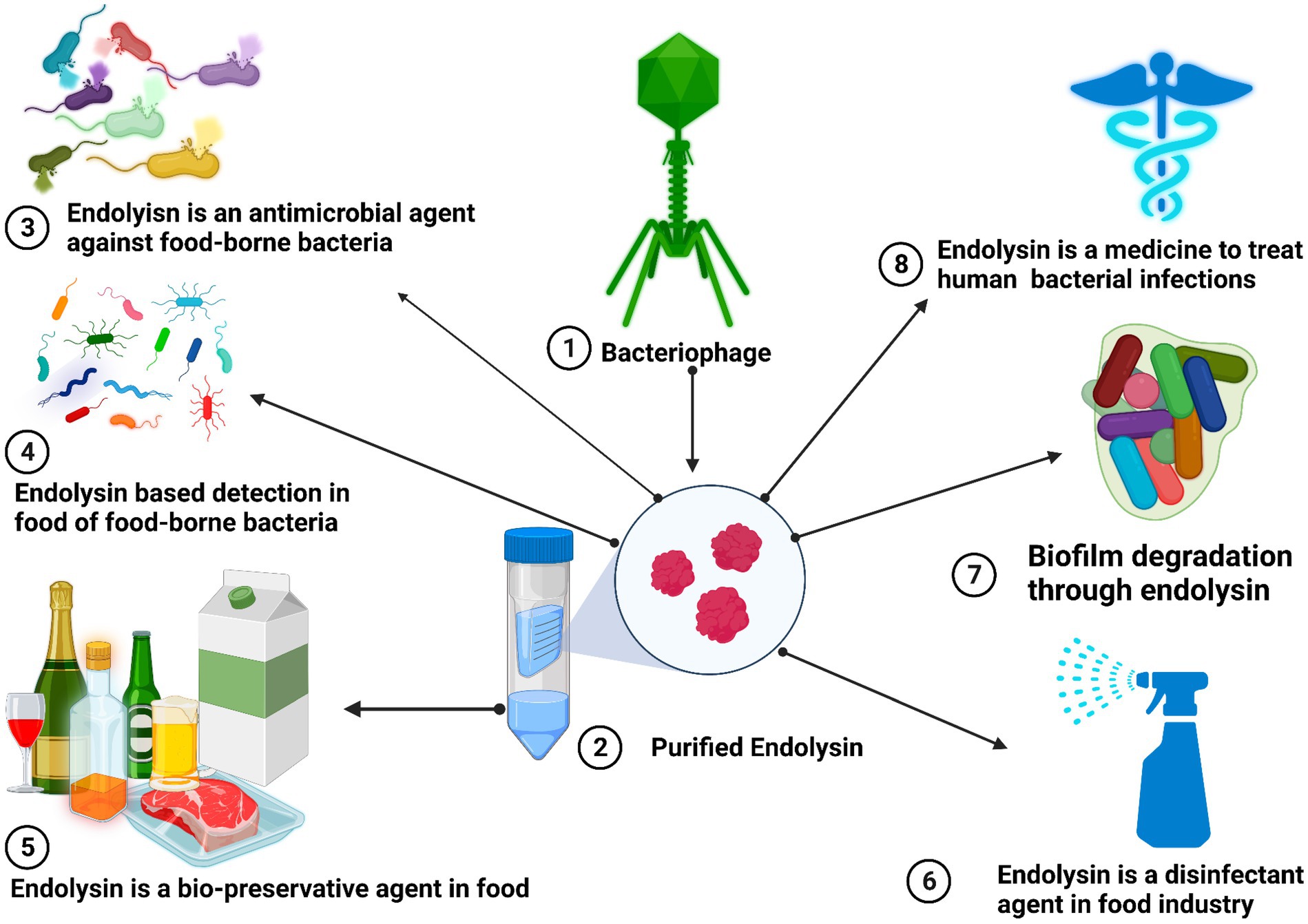
Figure 2. Antimicrobial properties of bacteriophage-derived endolysins for the safety of humans and foods from pathogenic bacteria. Endolysin is a bio-preservative agent in food chains and biofilm degradations through endolysin. Endolysin-based detection in food of food-borne bacteria, endolysin as a disinfectant agent in the food industry, and its use in the clinic as a therapeutic agent to treat and cure human antibiotic-resistant bacterial pathogens.
4.2. Escherichia coli O157: H7
Escherichia coli O157: H7 is a foodborne pathogen that causes a wide range of health issues in humans, including lethargy, nausea, bloody diarrhea, and, in extreme cases, death (Alhadlaq et al., 2023). The fresh vegetables are an essential part of a healthy diet. However, the contamination of salad greens with Escherichia coli O157: H7 has resulted in serious illness and significant economic losses (Xu et al., 2021). The endolysins PlyEc2 and RLysJN01 showed bactericidal activities against the E. coli O157:H7 on lettuce and did not affect the color or taste quality of the lettuce (Xu et al., 2021; Shen et al., 2022).
4.3. Staphylococcus aureus
Staphylococcus aureus is the most common cause of foodborne illness around the globe (Kadariya et al., 2014). MRSA has been a considerable concern to public health worldwide. During the previous decade, an extensive spread of MRSA with cattle origin has been detected along the farm-to-fork food chain (Köck et al., 2014). One potential control technique is purified endolysins to serve as bio-preservatives in food products to control S. aureus food poisoning. The endolysins Lys109 inhibited S. aureus and MRSA in milk and bacon foods and has promising lysis activity on planktonic bacteria and biofilms (Son et al., 2021). CHAPSH3b (which includes the endopeptidase domain of HydH5 and the cell wall targeting domain of lysostaphin) has been reported which eliminate S. aureus in milk to undetectable levels and retained significant activity in raw milk, after pasteurization, and during storage at 4°C. For these reasons, CHAPSH3b has been proposed as a possible bio-preservative component for milk storage (Rodríguez-Rubio et al., 2013).
4.4. Listeria monocytogenes
Listeria monocytogenes is a bacterium found in ready-to-eat foods such as meats, mixed salads, dairy, and vegetables (Zhu et al., 2017; Desai et al., 2019). L. monocytogenes is an infectious pathogen that can transfer to humans through food contamination. It can cause severe infections with possibly deadly outcomes in vulnerable groups like newborns, the old, pregnant women, and immunocompromised people (Vázquez-Boland et al., 2001; de Noordhout et al., 2014). Recently, researchers have studied, examined, and reported endolysin as a natural biocontrol agent against the foodborne pathogen L. monocytogenes. The endolysin PHA_BNPs showed prominent activity against L. monocytogenes (Stone et al., 2022). Fresh Hispanic cheese, known as queso fresco, is frequently linked to outbreaks of listeriosis in the US and human foodborne diseases caused by L. monocytogenes. The endolysins PlyP40 and PlyPSA prevented and eradicated L. monocytogenes contamination in queso fresco at cold storage for 28 days. These endolysins showed activity at low temperatures, pH, and salinity (Holle and Miller, 2021). The heat-stable L. monocytogenes endolysins, PlyP35, Ply511, and Ply118, is a fascinating finding, which shows lysis activity after 30 min of heating to 90°C. Ply511 and Ply118 decreased the amount of live L. monocytogenes when added to artificially spiked food items including iceberg lettuce and whole cow milk (Schmelcher et al., 2012a). These stable heat endolysin can be used in heat-treated food products like pasteurized milk (Schmelcher et al., 2012c). The endolysin LysZ5 from the L. monocytogenes phage FWLLm3 specifically reduced host growth up to 4 log CFU in soya milk in 3 h at 4°C, indicating that LysZ5 has excellent host specificity and host lysis activity at the refrigerator temperature (Zhang et al., 2012). Ply500, a listericidal peptidase, revealed a broad activity range across the genus Listeria (Schmelcher et al., 2012c).
4.5. Clostridium
Clostridium perfringens is a Gram-positive, anaerobic, and rod-shaped bacteria that can cause food poisoning through its enterotoxin (Baldassi, 2005). Fast detection and treatment of C. perfringens infection are essential for maintaining food safety (Cho et al., 2021). It has been reported that C. perfringens frequently contaminates milk (Mcauley et al., 2014). The endolysin LysCPAS15, Psa, Psm, PlyCP10 and PlyCP41 showed lytic activity against C. perfringens and as natural antibacterial proteins for preventing the spread and contamination in milk, meat and other food products. LysCPAS15 was tested in milk which reduced the bacterial load in milk. The PlyCP10 and PlyCP41 inhibited the growth of seventy-five C. perfringens strains isolated from pigs, chickens, and cows (Swift et al., 2018; Cho et al., 2021; Sekiya et al., 2021). Clostridium botulinum is a Gram-positive, anaerobic bacterium that produces spore’s botulinum neurotoxin (BoNT). BoNT causes botulism, a potentially fatal flaccid paralysis, in humans and animals. The germination and development of C. botulinum spores in food can cause different infections in humans and animals (Zhang et al., 2020). The endolysin CBO1751 efficiently lyses C. botulinum and also eliminates neurotoxin production. The antibacterial endolysin CBO1751 against C. botulinum, is a novel anti-botulinum protein for non-thermal applications in food and agriculture (Zhang et al., 2020). Clostridium sporogenes are spoilage bacteria that can cause food spoilage and rotting problems (Mayer et al., 2012). The endolysin CS74L showed lysis activity against the 16 tested strains of C. sporogenes (Mayer et al., 2012).
4.6. Vibrio parahaemolyticus
The main pathogen involved in seafood-borne bacterial infections is Vibrio parahaemolyticus. V. parahaemolyticus is a gram-negative bacterium inhabiting sea foods, including fish, shrimp and oysters (Letchumanan et al., 2014). The V. parahaemolyticus forms biofilms commonly in food manufacturing environments, contaminating food manufacturing machinery and products (Lopatek et al., 2018). V. parahaemolyticus biofilm production can increase resistance to antibacterial drugs commonly used in food, raising the chance of pathogen infections (Ning et al., 2021). Food contaminated with V. parahaemolyticus can cause stomach pain, nausea and vomiting, acute dysentery, and bloodstream infections (Baker-Austin et al., 2017). Additionally, due to antibiotic abuse, V. parahaemolyticus antibiotic resistance is becoming a severe concern to consumers (Shaw et al., 2014). Ninety V. parahaemolyticus strains from the main seafood in the China provinces of Shandong, Hebei, and Liaoning near the Bohai and Yellow Seas were recently examined for antibiotic resistance. Almost half of the isolates had at least three drug resistances, and more than 80% were resistant to ampicillin and cephazolin (Jiang et al., 2019). The demand for alternate antimicrobials to manage V. parahaemolyticus and associated biofilms may have recently increased (Ning et al., 2021). Endolysins are prospective substitutes for conventional antibiotics in the fight against V. parahaemolyticus and its biofilms (Matamp and Bhat, 2019). The recombinant endolysins Lysqdvp001, LysVPMS1 and LysVPp1 inhibited the growth of V. parahaemolyticus. Lysqdvpoo1 and poly-lysine showed robust synergistic antibacterial activity, so these two can be used in combination to tackle V. parahaemolyticus and its biofilms in the food sector (Wang W. et al., 2016; Li et al., 2018; Zermeño-Cervantes et al., 2018; Ning et al., 2021).
4.7. Campylobacter jejuni
Campylobacter jejuni is the most common foodborne bacteria which can cause gastroenteritis worldwide and poses a severe risk to public health and food safety (Moffatt et al., 2016). The endolysin Cj1 and Cj5 can eradicate and control the C. jejuni in chicken food models in a refrigerated 5°C (Zampara et al., 2021).
Figure 3 defines the efficacy of endolysin as an antibacterial agent against foodborne illness caused by foodborne bacterial pathogens. Table 1 summarizes the use of endolysins in different food products, while Table 2 describes the efficacy of endolysin against different pathogenic bacteria.
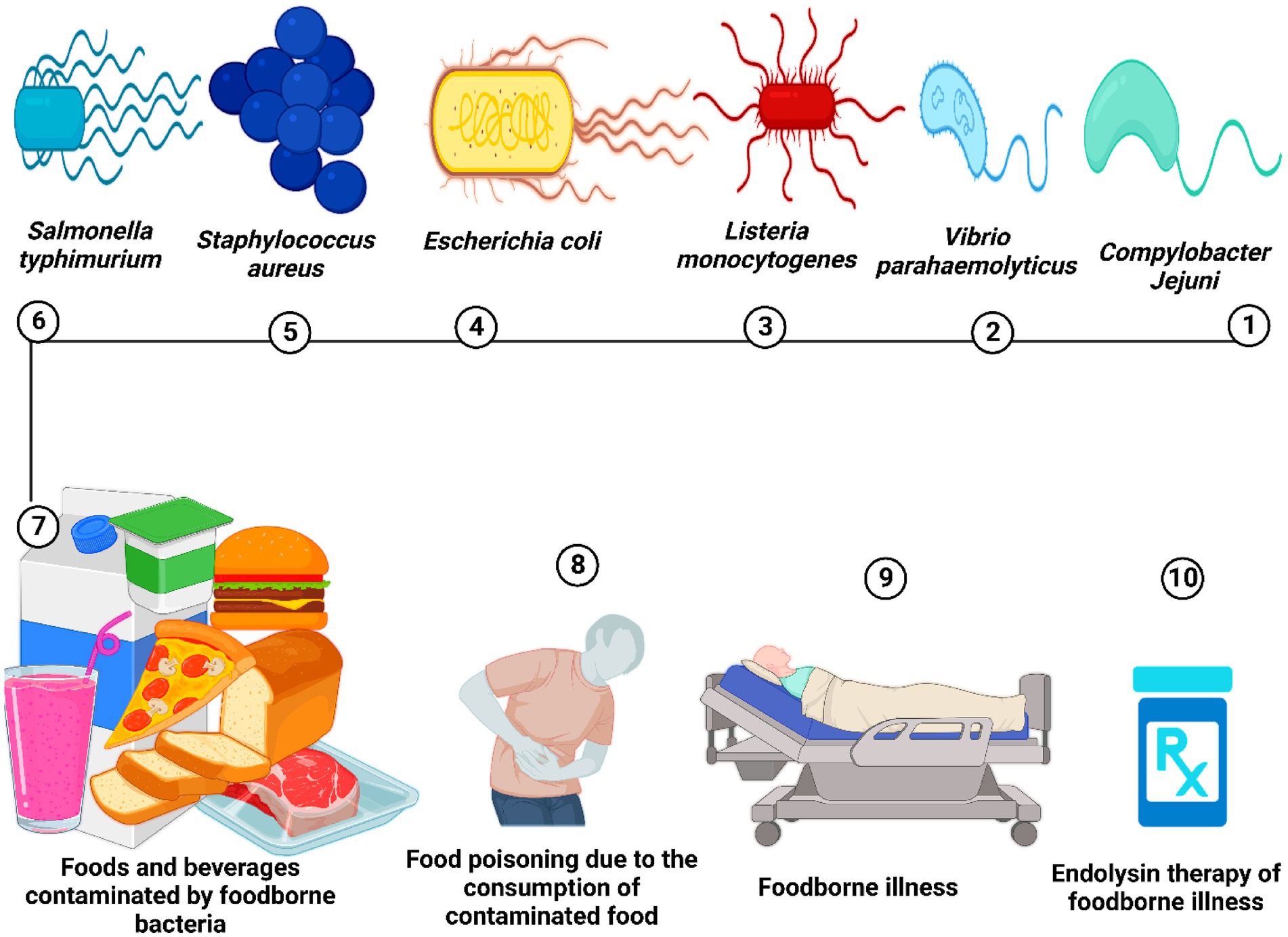
Figure 3. Food is contaminated by food-borne pathogenic bacteria, leading to food poisoning and potentially severe food-borne illnesses. Endolysin therapy could serve as one of the effective treatments for food-borne bacterial infections.
5. Current challenges
Despite their effectiveness, endolysins have significant limits in their practical application in food industries. Although there are several endolysins in nature, identifying unique and potent endolysins necessitates preliminary measures such as phage isolation, propagation, endolysin cloning, and purification (Lee et al., 2021). Certain endolysins have low expression levels and are insoluble (Son et al., 2021). Furthermore, research on endolysins as food supplements and antimicrobial agents is still in its early stages because many endolysin uses have concentrated on their preventive and therapeutic efficiency against bacterial infections in animal models (Garcia et al., 2010). Endolysins’ antibacterial efficacy varies according to the food ingredients including proteins, carbs, fat, minerals, vitamins and biochemical components which mainly include temperature, pH, and ionic strength in foodstuffs (Shannon et al., 2020). All of these factors could have an impact on the protein stability and/or function of endolysins, making them less useful. As a result, only a few scientific investigations have proved their applicability in dietary supplements (Shannon et al., 2020).
5.1. Stability of endolysins
The stability of endolysins used in the food is a main difficulty and concern. On a laboratory scale, natural and engineered endolysins have shown remarkable antibacterial activity. However, given that food is a massively complex of foodstuffs with distinct factors, such as ion concentration, acidity, salt concentration, temperatures, and physical properties, there might be a significant difference in their antimicrobial effectiveness in dietary settings compared to in vitro used (Shannon et al., 2020). The reported endolysins, LysAB54 and PlyA, showed a loss of bactericidal activity in the milk. These may be due to environmental factors like salt and different protein interactions, which can neutralize the endolysins (Yang et al., 2015b; Khan et al., 2021). Additionally, research into the structure of individual domains in endolysins, the location and characteristics of linker sections, the distribution of charges, and the use of cofactors can shed light on how endolysins interact with certain meals (Shannon et al., 2020). Novel delivery ways, such as encapsulating the endolysins into some nano-vesicles, may be attempted to synthesize Gram-negative endolysins for food safety (Gondil and Chhibber, 2021; Lee et al., 2022). At the same time, we suggest that advanced computational and structural analysis ought to serve as the foundation for endolysin designs that are more logically planned and can enhance antibacterial activity in particular foodstuffs.
5.2. Reduced activity of endolysins
During the stationary phase, the bacteria undergo several changes, including spherical cell growth, a hard cell envelope, intense cross-linking of the cell wall, a reduction in membrane fluidity, and the activation of the robust response mechanism to help the cells escape from the disruption (Jaishankar and Srivastava, 2017). Due to this reason, bacterial growth phases have a big effect on the activity of endolysin and endolysins show less activity against the stationary phase of bacteria. Endolysin can be combined with other antibacterial agents to achieve a synergistic effect and improve lysis activity. The engineering of endolysin with the outer membrane-permeabilizing polypeptide, chitosan nanoparticle, and other LPS disrupter molecules including EDTA, and citric acid (Murray et al., 2021; Kocot et al., 2023). Also, we suggest the combination of different endolysins can be used in synergism to enhance the activity of endolysin against the stationary phase of bacteria.
5.3. Challenge against Gram-negative bacteria
It has been discovered that several endolysins function as broad-spectrum antibacterial agents against Gram-positive bacteria (Yang et al., 2015a; Huang et al., 2020; Yang H. et al., 2020). Contrarily, a protective bacterial outer membrane renders most endolysins ineffective against Gram-negative bacteria (Abdelrahman et al., 2021; Khan et al., 2021). Recently, bacteriophage and endolysin encapsulation methods have been reported (Loh et al., 2021). Developing novel phage and endolysin delivery methods and their application are alternative strategies to overcome endolysins’ challenges and improve endolysins’ efficiency (Elkhoury et al., 2020). Therefore, through this strategy, the endolysins can interact with the peptidoglycan layer directly (Oliveira et al., 2012; Deshmukh et al., 2021). Moreover, Yves Briers described novel ways to disrupt the Gram-negative bacterial membrane: (1) physical or chemical methods to disrupt the outer membrane’s integrity; and (2) protein engineering to provide endolysins with the tools needed to overcome the outer membrane (Gutiérrez and Briers, 2021). Another method for combating Gram-negative pathogens is to fuse endolysin with a peptide with outer membrane-permeabilizing features to the endolysin. By attaching the peptide cecropin A to LysMK34 to its N terminus via a linker consisting of three Ala-Gly repeats, resulting in a genetically engineered LysMK34 (eLysMK34). Compared to the parental endolysin, the modified engineered endolysin shows improved antibacterial activity (Abdelkader et al., 2022). To boost outer membrane permeability and increase activity against Gram-negative bacteria, use endolysin in combination with antibiotics. LNT113, an engineered endolysin, was evaluated for synergistic effects with standard-of-care antibiotics. The LNT113 showed a synergistic activity with antibiotics (Hong et al., 2022). Hurdle technology: The use of hurdle technology provides a strategy for enhancing the efficiency of endolysins against Gram-negative bacteria. The makes use of multiple methods combined to eradicate bacteria and ensure the microbiological safety of food. In the case of endolysin, the procedure requires the use of another treatment first, which is to break cell membranes, including the outer membrane allowing the lysin to interact with the target petidoglycan (Oliveira et al., 2012; Mukhopadhyay and Gorris, 2014). The further strategies to increase the bactericidal activity of endolysin against gram-negative bacteria are the development of engineered endolysin. The methods of engineering of endolysin include the development of Lysocins, Innolysins, Lysin-based dendrimers, In silico search for antimicrobial peptides, Liposome-mediated lysin delivery, encapsulation in alginate-chitosan nanoparticles (Kocot et al., 2023).
5.4. The expensive production of endolysin
Endolysins can eradicate Gram-positive bacteria has been proven in food matrices and specific food products, which strongly suggests that they could be used to fight foodborne pathogens in the food industry. However, the necessity for their large-scale production, purifying procedure, and scalability, which may include expensive costs, limits the use of endolysin in food companies (Xu, 2021). We should carefully assess the safety, stability, and manufacturing costs of engineered endolysins before using them in the food sector. Therefore, to address the remaining issues with endolysin, ongoing research into related issues is necessary due to the high needs of the food sector (Lee et al., 2022). Figure 4 shows the endolysin purification process.
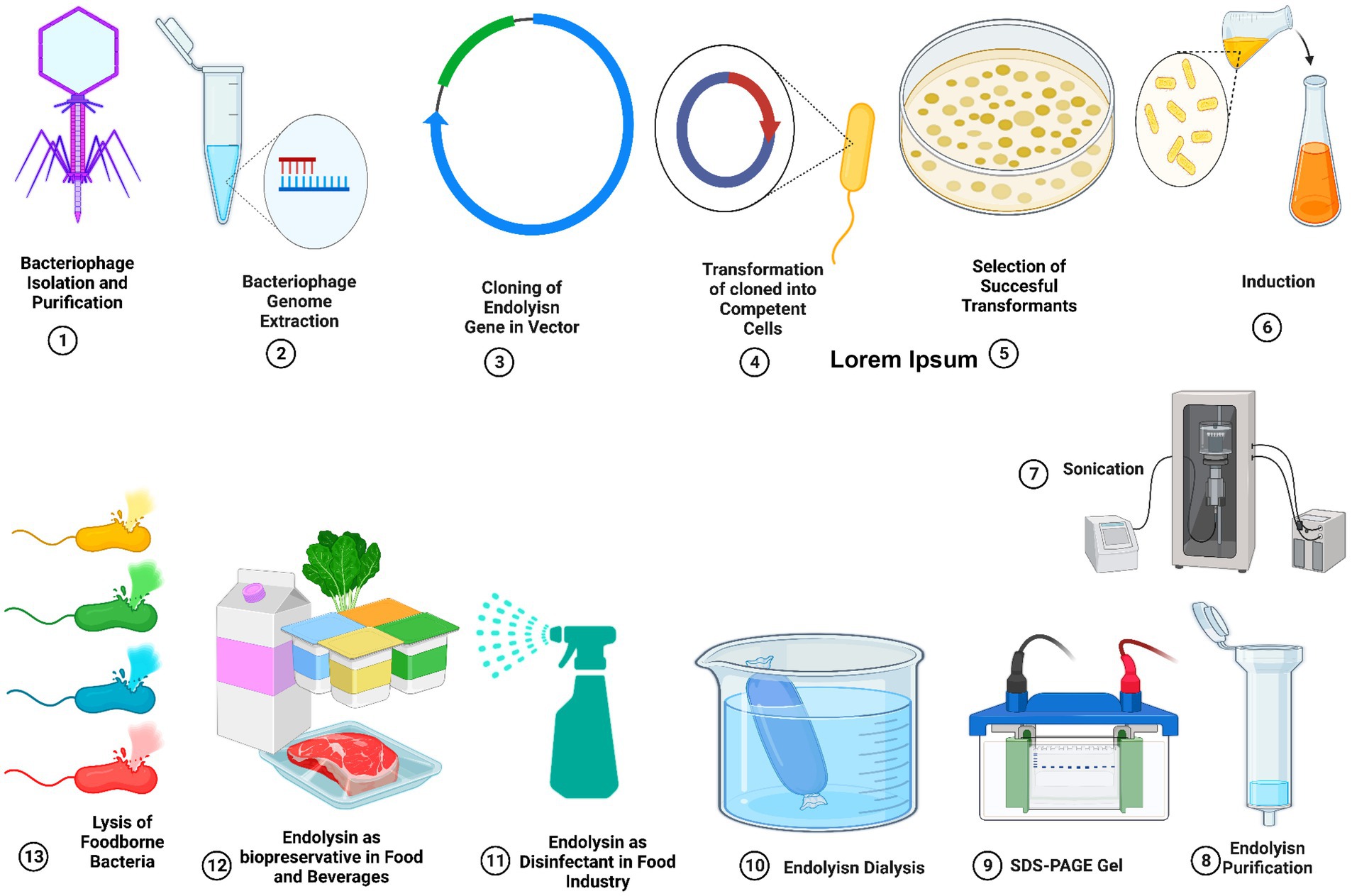
Figure 4. Cloning, expression, and purification of bacteriophage-derived endolysins. Applications of endolysin as a natural bio-preservative agent to reduce the contamination of foods from food-borne pathogenic bacteria.
6. The synthetic engineering of endolysin
Numerous approaches to synthesizing engineering endolysins can overcome the primary limitations of natural endolysins: (1) low bactericidal efficacy and a limited host range in the presence of food ingredients, and (2) lack of capacity to effectively kill Gram-negative bacteria. Endolysins’ low catalytic effectiveness and narrow host spectrum could be modified depending on the feature of endolysins, “modularity.” The modular architecture of general Gram-positive endolysins is made up of various EADs and CBD (Gerstmans et al., 2018). Their active domain units can provide simple and efficient engineering tools such as (i) direct genetic mutations (ii) domain deletion or truncation and (iii) domain shifting (Lee et al., 2022).
6.1. Improving the activity of endolysin through domain truncation or deletion
Endolysins’ antibacterial activity, CBD dependency, and host specificity can all be altered by the truncation or deletion of a domain (Cheng and Fischetti, 2007). The Streptococcus endolysin PlyGBS were randomly mutated. The mutated endolysin had about 20-fold higher lytic activity than full-length PlyGBS. Horgan et al. (2009) synthesized several variants of the Staphylococcus LysK endolysin and reported that CHAPk (a truncated LysK-engineered endolysin) had better lytic activity against staphylococcal strains in vitro than the natural. Low et al. (2011) revealed that eliminating CBD from several endolysins improved their enzymatic activity. They explained this phenomenon in the context of an EAD domain’s CBD dependability being closely linked to its charge. Because lipoteichoic acids are negatively charged in the cell wall, changing the net charge of the catalytic domain from negative to positive may result in enhanced interaction between the catalytic domain and lipoteichoic acids.
6.2. Enhancing the activity of endolysin through direct mutagenesis
The direct mutation was used further to improve the endolysins’ lytic activity and thermostability. Direct mutation in conserved sites involved in hydrogen bond networking at the backside of the amidase domain’s catalytic sites resulted in higher lytic activity of mutant CD27L endolysin against multiple L. monocytogenes serotypes (Mayer et al., 2011). Díez-Martínez et al. (2013) synthesized Cpl-7S, a synthetic endolysin, by swapping 15 amino acids into CBD. The alteration enhanced CBD’s net charge, resulting in improved lytic activity. For the changeable sites within the LysF1 fold, Love et al. (2021) altered a single residue in a non-conserved hydrophobic core to increase its heat stability. Using site-directed mutagenesis based on protein structure may be an appropriate approach for enhancing endolysins’ functions.
6.3. Domain shifting approach
Endolysin domains can act independently, they can be replaced or recombined with domains from other endolysins to develop chimeric enzymes with desirable features. Indeed, multiple studies have shown that these tactics are effective in enhancing catalytic efficiency (Mao et al., 2013; Yang et al., 2014b; Díez-Martínez et al., 2015). And increasing host specificity through CBD shifting or addition (Yoong et al., 2004; Becker et al., 2009; Zhou et al., 2017). Fusion endolysins with improved lytic activity and thermostability have been effectively tested on milk and poultry (Rodríguez-Rubio et al., 2012). Rodrguez-Rubio et al. reported that the fusion protein CHAPSH3b which is composed of the CHAP domain of HydH5 and the SH3b domain of lysostaphin eradicated S. aureus in pasteurized milk to undetectable numbers after 15 min of treatment at a relatively low concentration of 1 μM (Rodríguez-Rubio et al., 2012). Mao et al. (2013) increased the lytic activity of Ply187 in milk through the development of a fusion protein CBD of Lysk (KSH3b) and the EAD of Ply187. Using high-throughput synthesis and screening of chimeric endolysins, a random domain-swapping approach coupled with domain shuffling has been developed. Yang et al. (2015a) developed an in vitro screening platform using a two-vector E. coli expression system. Using the in vitro screening platform, they reported ClyR, a new chimeric endolysin that showed a wide, evident lysis zone in the Streptococcus dysgalactiae lawn. In pasteurized milk, ClyR demonstrated a wider host range and lytic activity against Streptococcus spp. Lee et al. (2021) developed a library of chimeric endolysins by rearranging the domains of 12 native staphylococcal endolysins. ClyC, the most efficient chimeric endolysin, maintained its significant antibacterial action in milk, and animal blood, as well as protecting against MRSA-induced bacterial infection in an in vivo model of mouse infection.
6.4. Engineering endolysins for the biological control of Gram-negative bacteria
Since the cell lysis of Gram-negative bacteria through natural endolysin therapy is limited, the basic objective of endolysin engineering for targeting Gram-negative bacteria is to enter the outer membrane (Oliveira et al., 2012). Endolysins targeting Gram-negative bacteria have previously been proven and showed synergistic activities through physical hydrostatic pressure (Nakimbugwe et al., 2006). Gram-negative endolysin was also reported which showed synergistic activities in combination with citric acid, chloroform, EDTA, Triton X-100, trichloroethane (CHCL3) (Briers et al., 2014; Yang et al., 2015b; Guo et al., 2017).
6.4.1. Artilysins
Briers et al. (2014) developed the name “artilysins, “which are chimeric endolysins paired with Gram-negative lysins and an OM-destabilizing peptide. To destabilize the negatively charged lipopolysaccharides at the outer membrane, peptides with various physicochemical properties including cationic, hydrophobic, or amphipathic can be used in the engineering of artilysins. Art-175 is a representative of artilysin, which is a fusion protein composed of a modified form of endolysin KZ144 and SMAP-29. Art-175 showed antibacterial properties against a broad spectrum of Gram-negative bacteria (Briers et al., 2014).
6.4.2. Lysocin and Innolysin
The gram-negative endolysins can be engineered by making use of colicin-like bacteriocins, which function in translocating the OM of bacteria and delivering endolysins to the peptidoglycan (Lukacik et al., 2012; Yan et al., 2017; Heselpoth et al., 2019). Lysocins are fusion proteins that are composed of endolysin and bacteriocin that help transport molecules over the outer membrane. Additionally, a unique strategy for targeting Gram-negative bacteria is the fusion of bacteriophage receptor binding proteins (RBPs) with endolysins, known as Innolysin (Zampara et al., 2020). Innolysin Ec21 showed potential antibacterial activity, resulting in over a 3-log decrease in E. coli cells resistant to third-generation cephalosporins (Zampara et al., 2020).
6.4.3. Encapsulation of endolysin
Despite phages and endolysins’ antibacterial potential, these alternative agents need to overcome various practical obstacles posed by the host system, such as limited bioavailability loss of action, non-targeted delivery, fast clearance by the reticuloendothelial system, and antibody-mediated deactivation (Loh et al., 2021). A formulation approach to Gram-negative endolysins has been engineered to increase its permeability in addition to the fusion engineering method (Bai et al., 2019). Bai et al. (2019) used the cationic liposomes to encapsulate the Salmonella endolysin BSP16Lys. In S. typhimurium and E. coli cells, encapsulated BSPLys revealed improved antimicrobial activity of up to 2.2 logs and 1.6 logs CFU/mL decreases, respectively. T4 lysozyme was immobilized to cellulose nanocrystals, and this immobilization technique significantly improved the antibacterial activity against E. coli and Pseudomonas mendocina compared to the natural enzyme (Abouhmad et al., 2017). As the pneumocidal activity of endolysin, Cpl-1 is retained in chitosan nanoparticles. The alternative encapsulating technique using non-biohazardous and biocompatible particles, such as chitosan, can be used to synthesize Gram-negative endolysins for food safety (Gondil et al., 2020a).
7. Conclusion
Various foodborne outbreaks have persistently put at risk food safety despite developments in modern technology. This threat has increased as a result of antibiotic overuse, abuse, and misuse due to the increase of multidrug-resistant bacteria. To preserve the safety of the food, researchers must develop new techniques for reducing microbial contamination. Bacteriophage-derived endolysins have been suggested as innovative, efficient, and safe antimicrobial agents and applied for the control and eradication of bacterial contaminants in foods and food processing companies. Most bacteria prevalent in the food industry are Gram-negative bacteria. Therefore, the development of new antibacterials should target this group of bacteria. The bacteriophage-derived endolysin has been extensively tested for use as antibacterials. The use of endolysin in a food context is largely limited to Gram-positive bacteria, with only a few studies focusing on the use of phage lysins against foodborne Gram-negative pathogens in food matrices. In recent years, different strategies to improve the effectiveness of endolysin against Gram-negative bacteria have been reported and were focused on clinical application. Therefore, future research should focus on the control of persistent foodborne pathogens. Furthermore, endolysins have some limitations, so new endolysins can be engineered more effectively by being specially adapted for a particular food to increase lysis potential. The use of advanced molecular biology tools will help in engineering new endolysins with high activity against Gram-negative bacteria. This new and enhanced endolysin may allow us to overcome existing barriers and make endolysin used for the control of food-borne pathogens more accessible. To produce an “ideal” engineer endolysin that maintains high activity while exhibiting other desirable properties depending on the conditions of its employment. An engineer endolysin might have thermostability, and high solubility to make it accessible, highly targeted, and broad-spectrum activity depending on the target pathogen, and the production should be cost-effective. Endolysin engineering opens up a plethora of possibilities for the production of ideal novel endolysin. The development of next-generation endolysin can be applied in the food sector.
Author contributions
FK: Conceptualization, Funding acquisition, Investigation, Writing – original draft. J-HC: Conceptualization, Formal analysis, Supervision, Writing – review & editing. RZ: Conceptualization, Supervision, Writing – review & editing, Funding acquisition, Project administration. BL: Conceptualization, Funding acquisition, Supervision, Writing – review & editing, Formal analysis, Project administration.
Funding
The author(s) declare financial support was received for the research, authorship, and/or publication of this article. This work is financially supported by a Research Fund for International Young Scientists from the National Natural Science Foundation of China (NSFC) to FK of Grant number: 32250410292. Also supported by the Guangdong Province Zhujiang Talent Program (grant number 2019ZT08H476); and Shenzhen Science and Technology Program (grant number KQTD20180412181334790).
Acknowledgments
Images (of various bacteria, bacterial cells, phage, protein, and scientific instruments) were used to create figures using BioRender.com.
Conflict of interest
The authors declare that the research was conducted in the absence of any commercial or financial relationships that could be construed as a potential conflict of interest.
Publisher’s note
All claims expressed in this article are solely those of the authors and do not necessarily represent those of their affiliated organizations, or those of the publisher, the editors and the reviewers. Any product that may be evaluated in this article, or claim that may be made by its manufacturer, is not guaranteed or endorsed by the publisher.
References
Abdelkader, K., Gutiérrez, D., Tamés-Caunedo, H., Ruas-Madiedo, P., Safaan, A., Khairalla, A. S., et al. (2022). Engineering a lysin with intrinsic antibacterial activity (LysMK34) by cecropin a fusion enhances its antibacterial properties against Acinetobacter baumannii. Appl. Environ. Microbiol. 88, e01515–e01521. doi: 10.1128/AEM.01515-21
Abdelrahman, F., Easwaran, M., Daramola, O. I., Ragab, S., Lynch, S., Oduselu, T. J., et al. (2021). Phage-encoded endolysins. Antibiotics 10:124. doi: 10.3390/antibiotics10020124
Abee, T., Kovács, Á. T., Kuipers, O. P., and Van Der Veen, S. (2011). Biofilm formation and dispersal in gram-positive bacteria. Curr. Opin. Biotechnol. 22, 172–179. doi: 10.1016/j.copbio.2010.10.016
Abhisingha, M., Dumnil, J., and Pitaksutheepong, C. (2023). Effect of lysin EN4 in combination with sodium bicarbonate on reduction of Salmonella in chilled and thawed chicken meat. Int. J. Food Microbiol. 387:110058. doi: 10.1016/j.ijfoodmicro.2022.110058
Abouhmad, A., Dishisha, T., Amin, M. A., and Hatti-Kaul, R. (2017). Immobilization to positively charged cellulose nanocrystals enhances the antibacterial activity and stability of hen egg white and T4 lysozyme. Biomacromolecules 18, 1600–1608. doi: 10.1021/acs.biomac.7b00219
Ahmed, A. B. F., Noguchi, K., Asami, Y., Nomura, K., Fujii, H., Sakata, M., et al. (2007). Evaluation of cell wall binding domain of Staphylococcus aureus autolysin as affinity reagent for bacteria and its application to bacterial detection. J. Biosci. Bioeng. 104, 55–61. doi: 10.1263/jbb.104.55
Ajuebor, J., Mcauliffe, O., O’mahony, J., Ross, R. P., Hill, C., and Coffey, A. (2016). Bacteriophage endolysins and their applications. Sci. Prog. 99, 183–199. doi: 10.3184/003685016X14627913637705
Alhadlaq, M. A., Mujallad, M. I., and Alajel, S. M. (2023). Detection of Escherichia coli O157: H7 in imported meat products from Saudi Arabian ports in 2017. Sci. Rep. 13:4222. doi: 10.1038/s41598-023-30486-2
Bae, J. Y., Jun, K. I., Kang, C. K., Song, K.-H., Choe, P. G., Bang, J.-H., et al. (2019). Efficacy of intranasal administration of the recombinant endolysin SAL200 in a lethal murine Staphylococcus aureus pneumonia model. Antimicrob. Agents Chemother. 63, e02009–e02018. doi: 10.1128/AAC.02009-18
Bai, J., Kim, Y.-T., Ryu, S., and Lee, J.-H. (2016). Biocontrol and rapid detection of food-borne pathogens using bacteriophages and endolysins. Front. Microbiol. 7:474. doi: 10.3389/fmicb.2016.00474
Bai, J., Yang, E., Chang, P.-S., and Ryu, S. (2019). Preparation and characterization of endolysin-containing liposomes and evaluation of their antimicrobial activities against gram-negative bacteria. Enzym. Microb. Technol. 128, 40–48. doi: 10.1016/j.enzmictec.2019.05.006
Bajovic, B., Bolumar, T., and Heinz, V. (2012). Quality considerations with high pressure processing of fresh and value added meat products. Meat Sci. 92, 280–289. doi: 10.1016/j.meatsci.2012.04.024
Baker-Austin, C., Trinanes, J., Gonzalez-Escalona, N., and Martinez-Urtaza, J. (2017). Non-cholera vibrios: the microbial barometer of climate change. Trends Microbiol. 25, 76–84. doi: 10.1016/j.tim.2016.09.008
Baldassi, L. (2005). Clostridial toxins: potent poisons, potent medicines. J. Venomous Anim. Toxins Includ. Trop. Dis. 11, 391–411. doi: 10.1590/S1678-91992005000400002
Bantawa, K., Rai, K., Subba Limbu, D., and Khanal, H. (2018). Food-borne bacterial pathogens in marketed raw meat of Dharan, eastern Nepal. BMC. Res. Notes 11, 1–5. doi: 10.1186/s13104-018-3722-x
Becker, S. C., Foster-Frey, J., Stodola, A. J., Anacker, D., and Donovan, D. M. (2009). Differentially conserved staphylococcal SH3b_5 cell wall binding domains confer increased staphylolytic and streptolytic activity to a streptococcal prophage endolysin domain. Gene 443, 32–41. doi: 10.1016/j.gene.2009.04.023
Boeckel, T. P. V., Brower, C., Gilbert, M., Grenfell, B. T., Levin, S. A., Robinson, T. P., et al. (2015). Global trends in antimicrobial use in food animals. Proc. Natl. Acad. Sci. 112, 5649–5654. doi: 10.1073/pnas.1503141112
Briers, Y., Volckaert, G., Cornelissen, A., Lagaert, S., Michiels, C. W., Hertveldt, K., et al. (2007). Muralytic activity and modular structure of the endolysins of Pseudomonas aeruginosa bacteriophages φKZ and EL. Mol. Microbiol. 65, 1334–1344. doi: 10.1111/j.1365-2958.2007.05870.x
Briers, Y., Walmagh, M., Van Puyenbroeck, V., Cornelissen, A., Cenens, W., Aertsen, A., et al. (2014). Engineered endolysin-based “Artilysins” to combat multidrug-resistant gram-negative pathogens. MBio 5:e01379-14. doi: 10.1128/mBio.01379-14
Cash, H. L., Whitham, C. V., Behrendt, C. L., and Hooper, L. V. (2006). Symbiotic bacteria direct expression of an intestinal bactericidal lectin. Science 313, 1126–1130. doi: 10.1126/science.1127119
Cha, Y., Son, B., and Ryu, S. (2019). Effective removal of staphylococcal biofilms on various food contact surfaces by Staphylococcus aureus phage endolysin LysCSA13. Food Microbiol. 84:103245. doi: 10.1016/j.fm.2019.103245
Chang, Y. (2020). Bacteriophage-derived endolysins applied as potent biocontrol agents to enhance food safety. Microorganisms 8:724. doi: 10.3390/microorganisms8050724
Chang, Y., Kim, M., and Ryu, S. (2017a). Characterization of a novel endolysin LysSA11 and its utility as a potent biocontrol agent against Staphylococcus aureus on food and utensils. Food Microbiol. 68, 112–120. doi: 10.1016/j.fm.2017.07.004
Chang, Y., Yoon, H., Kang, D.-H., Chang, P.-S., and Ryu, S. (2017b). Endolysin LysSA97 is synergistic with carvacrol in controlling Staphylococcus aureus in foods. Int. J. Food Microbiol. 244, 19–26. doi: 10.1016/j.ijfoodmicro.2016.12.007
Cheng, Q., and Fischetti, V. A. (2007). Mutagenesis of a bacteriophage lytic enzyme PlyGBS significantly increases its antibacterial activity against group B streptococci. Appl. Microbiol. Biotechnol. 74, 1284–1291. doi: 10.1007/s00253-006-0771-1
Cho, J.-H., Kwon, J.-G., O'sullivan, D. J., Ryu, S., and Lee, J.-H. (2021). Development of an endolysin enzyme and its cell wall–binding domain protein and their applications for biocontrol and rapid detection of Clostridium perfringens in food. Food Chem. 345:128562. doi: 10.1016/j.foodchem.2020.128562
De Noordhout, C. M., Devleesschauwer, B., Angulo, F. J., Verbeke, G., Haagsma, J., Kirk, M., et al. (2014). The global burden of listeriosis: a systematic review and meta-analysis. Lancet Infect. Dis. 14, 1073–1082. doi: 10.1016/S1473-3099(14)70870-9
Desai, A. N., Anyoha, A., Madoff, L. C., and Lassmann, B. (2019). Changing epidemiology of Listeria monocytogenes outbreaks, sporadic cases, and recalls globally: a review of ProMED reports from 1996 to 2018. Int. J. Infect. Dis. 84, 48–53. doi: 10.1016/j.ijid.2019.04.021
Deshmukh, S. K., Khan, M. A., Singh, S., and Singh, A. P. (2021). Extracellular nanovesicles: from intercellular messengers to efficient drug delivery systems. ACS Omega 6, 1773–1779. doi: 10.1021/acsomega.0c05539
Díez-Martínez, R., De Paz, H., Bustamante, N., García, E., Menéndez, M., and García, P. (2013). Improving the lethal effect of Cpl-7, a pneumococcal phage lysozyme with broad bactericidal activity, by inverting the net charge of its cell wall-binding module. Antimicrob. Agents Chemother. 57, 5355–5365. doi: 10.1128/AAC.01372-13
Díez-Martínez, R., De Paz, H. D., García-Fernández, E., Bustamante, N., Euler, C. W., Fischetti, V. A., et al. (2015). A novel chimeric phage lysin with high in vitro and in vivo bactericidal activity against Streptococcus pneumoniae. J. Antimicrob. Chemother. 70, 1763–1773. doi: 10.1093/jac/dkv038
Ding, Y., Zhang, Y., Huang, C., Wang, J., and Wang, X. (2020). An endolysin LysSE24 by bacteriophage LPSE1 confers specific bactericidal activity against multidrug-resistant Salmonella strains. Microorganisms 8:737. doi: 10.3390/microorganisms8050737
Donovan, D. M., Lardeo, M., and Foster-Frey, J. (2006). Lysis of staphylococcal mastitis pathogens by bacteriophage phi11 endolysin. FEMS Microbiol. Lett. 265, 133–139. doi: 10.1111/j.1574-6968.2006.00483.x
Elkhoury, K., Koçak, P., Kang, A., Arab-Tehrany, E., Ellis Ward, J., and Shin, S. R. (2020). Engineering smart targeting nanovesicles and their combination with hydrogels for controlled drug delivery. Pharmaceutics 12:849. doi: 10.3390/pharmaceutics12090849
Endersen, L., O’mahony, J., Hill, C., Ross, R. P., Mcauliffe, O., and Coffey, A. (2014). Phage therapy in the food industry. Annu. Rev. Food Sci. Technol. 5, 327–349. doi: 10.1146/annurev-food-030713-092415
Eugster, M. R., Haug, M. C., Huwiler, S. G., and Loessner, M. J. (2011). The cell wall binding domain of Listeria bacteriophage endolysin PlyP35 recognizes terminal GlcNAc residues in cell wall teichoic acid. Mol. Microbiol. 81, 1419–1432. doi: 10.1111/j.1365-2958.2011.07774.x
Fan, J., Zeng, Z., Mai, K., Yang, Y., Feng, J., Bai, Y., et al. (2016). Preliminary treatment of bovine mastitis caused by Staphylococcus aureus, with trx-SA1, recombinant endolysin of S. aureus bacteriophage IME-SA1. Vet. Microbiol. 191, 65–71. doi: 10.1016/j.vetmic.2016.06.001
Fenton, M., Mcauliffe, O., O’mahony, J., and Coffey, A. (2010). Recombinant bacteriophage lysins as antibacterials. Bioeng. Bugs 1, 9–16. doi: 10.4161/bbug.1.1.9818
Fernández, L., González, S., Gutiérrez, D., Campelo, A. B., Martínez, B., Rodríguez, A., et al. (2018). Characterizing the transcriptional effects of Endolysin treatment on established biofilms of Staphylococcus aureus. Bio Protocol 8:e2891. doi: 10.21769/BioProtoc.2891
Fischetti, V. A. (2008). Bacteriophage lysins as effective antibacterials. Curr. Opin. Microbiol. 11, 393–400. doi: 10.1016/j.mib.2008.09.012
Fu, P., Wang, L., Chen, J., Bai, G., Xu, L., Wang, S., et al. (2019). Analysis of foodborne disease outbreaks in China mainland in 2015. Chin. J. Food Hyg. 31, 64–70. doi: 10.13590/j.cjfh.2019.01.014
García, P., Martínez, B., Rodríguez, L., and Rodríguez, A. (2010). Synergy between the phage endolysin LysH5 and nisin to kill Staphylococcus aureus in pasteurized milk. Int. J. Food Microbiol. 141, 151–155. doi: 10.1016/j.ijfoodmicro.2010.04.029
Garcia, P., Rodriguez, L., Rodriguez, A., and Martinez, B. (2010). Food biopreservation: promising strategies using bacteriocins, bacteriophages and endolysins. Trends Food Sci. Technol. 21, 373–382. doi: 10.1016/j.tifs.2010.04.010
Gerstmans, H., Criel, B., and Briers, Y. (2018). Synthetic biology of modular endolysins. Biotechnol. Adv. 36, 624–640. doi: 10.1016/j.biotechadv.2017.12.009
Gondil, V. S., and Chhibber, S. (2021). Bacteriophage and endolysin encapsulation systems: a promising strategy to improve therapeutic outcomes. Front. Pharmacol. 12:675440. doi: 10.3389/fphar.2021.675440
Gondil, V. S., Dube, T., Panda, J. J., Yennamalli, R. M., Harjai, K., and Chhibber, S. (2020a). Comprehensive evaluation of chitosan nanoparticle based phage lysin delivery system; a novel approach to counter S. pneumoniae infections. Int. J. Pharm. 573:118850. doi: 10.1016/j.ijpharm.2019.118850
Gondil, V. S., Harjai, K., and Chhibber, S. (2020b). Endolysins as emerging alternative therapeutic agents to counter drug-resistant infections. Int. J. Antimicrob. Agents 55:105844. doi: 10.1016/j.ijantimicag.2019.11.001
Gondil, V. S., Khan, F. M., Mehra, N., Kumar, D., Khullar, A., Sharma, T., et al. (2021). “Clinical potential of bacteriophage and Endolysin based therapeutics: A futuristic approach” in Microbial Products for Health, Environment and Agriculture. ed. P. K. Arora (London: Springer Nature), 39–58.
Guo, M., Feng, C., Ren, J., Zhuang, X., Zhang, Y., Zhu, Y., et al. (2017). A novel antimicrobial endolysin, LysPA26, against Pseudomonas aeruginosa. Front. Microbiol. 8:293. doi: 10.3389/fmicb.2017.00293
Guo, T., Xin, Y., Zhang, C., Ouyang, X., and Kong, J. (2016). The potential of the endolysin Lysdb from Lactobacillus delbrueckii phage for combating Staphylococcus aureus during cheese manufacture from raw milk. Appl. Microbiol. Biotechnol. 100, 3545–3554. doi: 10.1007/s00253-015-7185-x
Gutiérrez, D., and Briers, Y. (2021). Lysins breaking down the walls of gram-negative bacteria, no longer a no-go. Curr. Opin. Biotechnol. 68, 15–22. doi: 10.1016/j.copbio.2020.08.014
Ha, E., Son, B., and Ryu, S. (2018). Clostridium perfringens virulent bacteriophage CPS2 and its thermostable endolysin LysCPS2. Viruses 10:251. doi: 10.3390/v10050251
Hagens, S., and Loessner, M. J. (2007). Application of bacteriophages for detection and control of foodborne pathogens. Appl. Microbiol. Biotechnol. 76, 513–519. doi: 10.1007/s00253-007-1031-8
Han, H., Li, X., Zhang, T., Wang, X., Zou, J., Zhang, C., et al. (2019). Bioinformatic analyses of a potential Salmonella-virus-FelixO1 biocontrol phage BPS15S6 and the characterisation and anti-Enterobacteriaceae-pathogen activity of its endolysin LyS15S6. Antonie Van Leeuwenhoek 112, 1577–1592. doi: 10.1007/s10482-019-01283-7
Harhala, M. A., Gembara, K., Nelson, D. C., Miernikiewicz, P., and Dąbrowska, K. (2022). Immunogenicity of Endolysin PlyC. Antibiotics 11:966. doi: 10.3390/antibiotics11070966
Harhala, M., Nelson, D., Miernikiewicz, P., Heselpoth, R., Brzezicka, B., Majewska, J., et al. (2018). Safety studies of pneumococcal endolysins Cpl-1 and pal. Viruses-Basel 10:638. doi: 10.3390/v10110638
Heselpoth, R. D., Euler, C. W., Schuch, R., and Fischetti, V. A. (2019). Lysocins: bioengineered antimicrobials that deliver lysins across the outer membrane of gram-negative bacteria. Antimicrob. Agents Chemother. 63:319. doi: 10.1128/aac.00342-00319
Holle, M. J., and Miller, M. J. (2021). Lytic characterization and application of listerial endolysins PlyP40 and PlyPSA in queso fresco. JDS Commun. 2, 47–50. doi: 10.3168/jdsc.2020-0013
Hong, H.-W., Kim, Y. D., Jang, J., Kim, M. S., Song, M., and Myung, H. (2022). Combination effect of engineered endolysin EC340 with antibiotics. Front. Microbiol. 13:821936. doi: 10.3389/fmicb.2022.821936
Horgan, M., O’flynn, G., Garry, J., Cooney, J., Coffey, A., Fitzgerald, G. F., et al. (2009). Phage lysin LysK can be truncated to its CHAP domain and retain lytic activity against live antibiotic-resistant staphylococci. Appl. Environ. Microbiol. 75, 872–874. doi: 10.1128/AEM.01831-08
Huang, L., Luo, D., Gondil, V. S., Gong, Y., Jia, M., Yan, D., et al. (2020). Construction and characterization of a chimeric lysin ClyV with improved bactericidal activity against Streptococcus agalactiae in vitro and in vivo. Appl. Microbiol. Biotechnol. 104, 1609–1619. doi: 10.1007/s00253-019-10325-z
Ibarra-Sánchez, L. A., Van Tassell, M. L., and Miller, M. J. (2018). Antimicrobial behavior of phage endolysin PlyP100 and its synergy with nisin to control Listeria monocytogenes in Queso Fresco. Food Microbiol. 72, 128–134. doi: 10.1016/j.fm.2017.11.013
Imanishi, I., Uchiyama, J., Tsukui, T., Hisatsune, J., Ide, K., Matsuzaki, S., et al. (2019). Therapeutic potential of an endolysin derived from kayvirus S25-3 for staphylococcal impetigo. Viruses 11:769. doi: 10.3390/v11090769
Jado, I., López, R., García, E., Fenoll, A., Casal, J., and García, P. (2003). Phage lytic enzymes as therapy for antibiotic-resistant Streptococcus pneumoniae infection in a murine sepsis model. J. Antimicrob. Chemother. 52, 967–973. doi: 10.1093/jac/dkg485
Jaishankar, J., and Srivastava, P. (2017). Molecular basis of stationary phase survival and applications. Front. Microbiol. 8:2000. doi: 10.3389/fmicb.2017.02000
Jiang, Y., Chu, Y., Xie, G., Li, F., Wang, L., Huang, J., et al. (2019). Antimicrobial resistance, virulence and genetic relationship of Vibrio parahaemolyticus in seafood from coasts of Bohai Sea and Yellow Sea, China. Int. J. Food Microbiol. 290, 116–124. doi: 10.1016/j.ijfoodmicro.2018.10.005
Jiang, Y., Xu, D., Wang, L., Qu, M., Li, F., Tan, Z., et al. (2021). Characterization of a broad-spectrum endolysin LysSP1 encoded by a Salmonella bacteriophage. Appl. Microbiol. Biotechnol. 105, 5461–5470. doi: 10.1007/s00253-021-11366-z
Jun, S. Y., Jang, I. J., Yoon, S., Jang, K., Yu, K.-S., Cho, J. Y., et al. (2017). Pharmacokinetics and tolerance of the phage endolysin-based candidate drug SAL200 after a single intravenous administration among healthy volunteers. Antimicrob. Agents Chemother. 61, e02629–e02616. doi: 10.1128/AAC.02629-16
Jun, S. Y., Jung, G. M., Yoon, S. J., Choi, Y.-J., Koh, W. S., Moon, K. S., et al. (2014). Preclinical safety evaluation of intravenously administered SAL200 containing the recombinant phage endolysin SAL-1 as a pharmaceutical ingredient. Antimicrob. Agents Chemother. 58, 2084–2088. doi: 10.1128/AAC.02232-13
Kadariya, J., Smith, T. C., and Thapaliya, D. (2014). Staphylococcus aureus and staphylococcal food-borne disease: an ongoing challenge in public health. Biomed. Res. Int. 2014:827965. doi: 10.1155/2014/827965
Keeney, K. M., Yurist-Doutsch, S., Arrieta, M.-C., and Finlay, B. B. (2014). Effects of antibiotics on human microbiota and subsequent disease. Annu. Rev. Microbiol. 68, 217–235. doi: 10.1146/annurev-micro-091313-103456
Khan, F. M., Gondil, V. S., Li, C., Jiang, M., Li, J., Yu, J., et al. (2021). A novel Acinetobacter baumannii bacteriophage endolysin LysAB54 with high antibacterial activity against multiple gram-negative microbes. Front. Cell. Infect. Microbiol. 11:637313. doi: 10.3389/fcimb.2021.637313
Kim, S., Lee, D.-W., Jin, J.-S., and Kim, J. (2020). Antimicrobial activity of LysSS, a novel phage endolysin, against Acinetobacter baumannii and Pseudomonas aeruginosa. J. Glob. Antimicrob. Resist. 22, 32–39. doi: 10.1016/j.jgar.2020.01.005
Kirsch, J. M., Brzozowski, R. S., Faith, D., Round, J. L., Secor, P. R., and Duerkop, B. A. (2021). Bacteriophage-bacteria interactions in the gut: from invertebrates to mammals. Ann. Rev. Virol. 8, 95–113. doi: 10.1146/annurev-virology-091919-101238
Köck, R., Ballhausen, B., Bischoff, M., Cuny, C., Eckmanns, T., Fetsch, A., et al. (2014). The impact of zoonotic MRSA colonization and infection in Germany. Berl. Munch. Tierarztl. Wochenschr. 127, 384–398.
Kocot, A. M., Briers, Y., and Plotka, M. (2023). Phages and engineered lysins as an effective tool to combat gram-negative foodborne pathogens. Compr. Rev. Food Sci. Food Saf. 22, 2235–2266. doi: 10.1111/1541-4337.13145
Kong, M., and Ryu, S. (2015). Bacteriophage PBC1 and its endolysin as an antimicrobial agent against Bacillus cereus. Appl. Environ. Microbiol. 81, 2274–2283. doi: 10.1128/AEM.03485-14
Kuiper, J. W. P., Hogervorst, J. M. A., Herpers, B. L., Bakker, A. D., Klein-Nulend, J., Nolte, P. A., et al. (2021). The novel endolysin XZ. 700 effectively treats MRSA biofilms in two biofilm models without showing toxicity on human bone cells in vitro. Biofouling 37, 184–193. doi: 10.1080/08927014.2021.1887151
Kulinkina, A. V., Shinee, E., Guzmán Herrador, B. R., Nygård, K., and Schmoll, O. (2016). The situation of water-related infectious diseases in the pan-European region. Regional Office for Europe: World Health Organization.
Larpin, Y., Oechslin, F., Moreillon, P., Resch, G., Entenza, J. M., and Mancini, S. (2018). In vitro characterization of PlyE146, a novel phage lysin that targets gram-negative bacteria. PLoS One 13:e0192507. doi: 10.1371/journal.pone.0192507
Lee, C., Kim, H., and Ryu, S. (2022). Bacteriophage and endolysin engineering for biocontrol of food pathogens/pathogens in the food: recent advances and future trends. Crit. Rev. Food Sci. Nutr. 1–20. doi: 10.1080/10408398.2022.2116557
Lee, C., Kim, J., Son, B., and Ryu, S. (2021). Development of advanced chimeric endolysin to control multidrug-resistant Staphylococcus aureus through domain shuffling. ACS Infect. Dis. 7, 2081–2092. doi: 10.1021/acsinfecdis.0c00812
Legotsky, S. A., Vlasova, K. Y., Priyma, A. D., Shneider, M. M., Pugachev, V. G., Totmenina, O. D., et al. (2014). Peptidoglycan degrading activity of the broad-range Salmonella bacteriophage S-394 recombinant endolysin. Biochimie 107, 293–299. doi: 10.1016/j.biochi.2014.09.017
Letchumanan, V., Chan, K.-G., and Lee, L.-H. (2014). Vibrio parahaemolyticus: a review on the pathogenesis, prevalence, and advance molecular identification techniques. Front. Microbiol. 5:705. doi: 10.3389/fmicb.2014.00705
Li, C., Jiang, M., Khan, F. M., Zhao, X., Wang, G., Zhou, W., et al. (2021). Intrinsic antimicrobial peptide facilitates a new broad-Spectrum Lysin LysP53 to kill Acinetobacter baumannii in vitro and in a mouse burn infection model. ACS Infect. Dis. 7, 3336–3344. doi: 10.1021/acsinfecdis.1c00497
Li, M., Jin, Y., Lin, H., Wang, J., and Jiang, X. (2018). Complete genome of a novel lytic Vibrio parahaemolyticus phage VPp1 and characterization of its endolysin for antibacterial activities. J. Food Prot. 81, 1117–1125. doi: 10.4315/0362-028X.JFP-17-278
Li, C., Nyaruaba, R., Zhao, X., Xue, H., Yang, H., Li, Y., et al. (2023). LysP53 activity against Salmonella and its application in decontamination of Salmonella on fresh romaine lettuce. Appl. Microbiol. Biotechnol. 107, 5403–5413. doi: 10.1007/s00253-023-12666-2
Li, X., Wang, S., Nyaruaba, R., Liu, H., Yang, H., and Wei, H. (2021). A highly active chimeric lysin with a calcium-enhanced bactericidal activity against Staphylococcus aureus in vitro and in vivo. Antibiotics 10:461. doi: 10.3390/antibiotics10040461
Lim, J.-A., Shin, H., Heu, S., and Ryu, S. (2014). Exogenous lytic activity of SPN9CC endolysin against gram-negative bacteria. J. Microbiol. Biotechnol. 24, 803–811. doi: 10.4014/jmb.1403.03035
Liu, A., Wang, Y., Cai, X., Jiang, S., Cai, X., Shen, L., et al. (2019). Characterization of endolysins from bacteriophage LPST10 and evaluation of their potential for controlling Salmonella Typhimurium on lettuce. LWT 114:108372. doi: 10.1016/j.lwt.2019.108372
Loeffler, J. M., Nelson, D., and Fischetti, V. A. (2001). Rapid killing of Streptococcus pneumoniae with a bacteriophage cell wall hydrolase. Science 294, 2170–2172. doi: 10.1126/science.1066869
Loessner, M. J., Kramer, K., Ebel, F., and Scherer, S. (2002). C-terminal domains of Listeria monocytogenes bacteriophage murein hydrolases determine specific recognition and high-affinity binding to bacterial cell wall carbohydrates. Mol. Microbiol. 44, 335–349. doi: 10.1046/j.1365-2958.2002.02889.x
Loh, B., Gondil, V. S., Manohar, P., Khan, F. M., Yang, H., and Leptihn, S. (2021). Encapsulation and delivery of therapeutic phages. Appl. Environ. Microbiol. 87, e01979–e01920. doi: 10.1128/AEM.01979-20
Lopatek, M., Wieczorek, K., and Osek, J. (2018). Antimicrobial resistance, virulence factors, and genetic profiles of Vibrio parahaemolyticus from seafood. Appl. Environ. Microbiol. 84, e00537–e00518. doi: 10.1128/AEM.00537-18
Love, M. J., Bhandari, D., Dobson, R. C., and Billington, C. (2018). Potential for bacteriophage endolysins to supplement or replace antibiotics in food production and clinical care. Antibiotics 7:17. doi: 10.3390/antibiotics7010017
Love, M. J., Coombes, D., Manners, S. H., Abeysekera, G. S., Billington, C., and Dobson, R. C. (2021). The molecular basis for Escherichia coli O157: H7 phage FAHEc1 endolysin function and protein engineering to increase thermal stability. Viruses 13:1101. doi: 10.3390/v13061101
Low, L. Y., Yang, C., Perego, M., Osterman, A., and Liddington, R. (2011). Role of net charge on catalytic domain and influence of cell wall binding domain on bactericidal activity, specificity, and host range of phage lysins. J. Biol. Chem. 286, 34391–34403. doi: 10.1074/jbc.M111.244160
Lu, Y., Wang, Y., Wang, J., Zhao, Y., Zhong, Q., Li, G., et al. (2021). Phage endolysin LysP108 showed promising antibacterial potential against methicillin-resistant Staphylococcus aureus. Front. Cell. Infect. Microbiol. 11:668430. doi: 10.3389/fcimb.2021.668430
Lukacik, P., Barnard, T. J., Keller, P. W., Chaturvedi, K. S., Seddiki, N., Fairman, J. W., et al. (2012). Structural engineering of a phage lysin that targets gram-negative pathogens. Proc. Natl. Acad. Sci. 109, 9857–9862. doi: 10.1073/pnas.1203472109
Lv, M., Wang, S., Yan, G., Sun, C., Feng, X., Gu, J., et al. (2015). Genome sequencing and analysis of an Escherichia coli phage vB_EcoM-ep3 with a novel lysin, Lysep3. Virus Genes 50, 487–497. doi: 10.1007/s11262-015-1195-8
Ma, F., Xu, S., Tang, Z., Li, Z., and Zhang, L. (2021). Use of antimicrobials in food animals and impact of transmission of antimicrobial resistance on humans. Biosafety Health 3, 32–38. doi: 10.1016/j.bsheal.2020.09.004
Maciejewska, B., Roszniowski, B., Espaillat, A., Ksik-Szeloch, A., Majkowska-Skrobek, G., Kropinski, A. M., et al. (2017). Klebsiella phages representing a novel clade of viruses with an unknown DNA modification and biotechnologically interesting enzymes. Appl. Microbiol. Biotechnol. 101, 673–684. doi: 10.1007/s00253-016-7928-3
Maherani, B., Hossain, F., Criado, P., Ben-Fadhel, Y., Salmieri, S., and Lacroix, M. (2016). World market development and consumer acceptance of irradiation technology. Foods 5:79. doi: 10.3390/foods5040079
Majowicz, S. E., Musto, J., Scallan, E., Angulo, F., Kirk, M., O’brien, S., et al. (2010). International collaboration on enteric disease “burden of illness” studies. 2010. The global burden of nontyphoidal Salmonella gastroenteritis. Clin. Infect. Dis. 50, 882–889. doi: 10.1086/650733
Mao, J., Schmelcher, M., Harty, W. J., Foster-Frey, J., and Donovan, D. M. (2013). Chimeric Ply187 endolysin kills Staphylococcus aureus more effectively than the parental enzyme. FEMS Microbiol. Lett. 342, 30–36. doi: 10.1111/1574-6968.12104
Marshall, B. M., and Levy, S. B. (2011). Food animals and antimicrobials: impacts on human health. Clin. Microbiol. Rev. 24, 718–733. doi: 10.1128/CMR.00002-11
Matamp, N., and Bhat, S. G. (2019). Phage endolysins as potential antimicrobials against multidrug resistant vibrio alginolyticus and Vibrio parahaemolyticus: current status of research and challenges ahead. Microorganisms 7:84. doi: 10.3390/microorganisms7030084
Mayer, M. J., Garefalaki, V., Spoerl, R., Narbad, A., and Meijers, R. (2011). Structure-based modification of a Clostridium difficile-targeting endolysin affects activity and host range. J. Bacteriol. 193, 5477–5486. doi: 10.1128/JB.00439-11
Mayer, M. J., Gasson, M. J., and Narbad, A. (2012). Genomic sequence of bacteriophage ATCC 8074-B1 and activity of its endolysin and engineered variants against Clostridium sporogenes. Appl. Environ. Microbiol. 78, 3685–3692. doi: 10.1128/AEM.07884-11
Mayer, M. J., Payne, J., Gasson, M. J., and Narbad, A. (2010). Genomic sequence and characterization of the virulent bacteriophage ΦCTP1 from Clostridium tyrobutyricum and heterologous expression of its endolysin. Appl. Environ. Microbiol. 76, 5415–5422. doi: 10.1128/AEM.00989-10
Mcauley, C. M., Mcmillan, K., Moore, S. C., Fegan, N., and Fox, E. M. (2014). Prevalence and characterization of foodborne pathogens from Australian dairy farm environments. J. Dairy Sci. 97, 7402–7412. doi: 10.3168/jds.2014-8735
Mehdi, Y., Létourneau-Montminy, M.-P., Gaucher, M.-L., Chorfi, Y., Suresh, G., Rouissi, T., et al. (2018). Use of antibiotics in broiler production: global impacts and alternatives. Anim. Nutri. 4, 170–178. doi: 10.1016/j.aninu.2018.03.002
Mehmood Khan, F., Manohar, P., Singh Gondil, V., Mehra, N., Kayode Oyejobi, G., Odiwuor, N., et al. (2023). The applications of animal models in phage therapy: an update. Hum. Vaccin. Immunother. 19:2175519. doi: 10.1080/21645515.2023.2175519
Misiou, O., Van Nassau, T. J., Lenz, C. A., and Vogel, R. F. (2018). The preservation of Listeria-critical foods by a combination of endolysin and high hydrostatic pressure. Int. J. Food Microbiol. 266, 355–362. doi: 10.1016/j.ijfoodmicro.2017.10.004
Moffatt, C., Greig, A., Valcanis, M., Gao, W., Seemann, T., Howden, B., et al. (2016). A large outbreak of Campylobacter jejuni infection in a university college caused by chicken liver pâté, Australia, 2013. Epidemiol. Infect. 144, 2971–2978. doi: 10.1017/S0950268816001187
Mukhopadhyay, S., and Gorris, L. (2014). Hurdle technology, encyclopedia of food microbiology. 2nd ed. Cambridge, MA, USA: Academic Press.
Murray, E., Draper, L. A., Ross, R. P., and Hill, C. (2021). The advantages and challenges of using endolysins in a clinical setting. Viruses 13:680. doi: 10.3390/v13040680
Nakimbugwe, D., Masschalck, B., Atanassova, M., Zewdie-Bosüner, A., and Michiels, C. W. (2006). Comparison of bactericidal activity of six lysozymes at atmospheric pressure and under high hydrostatic pressure. Int. J. Food Microbiol. 108, 355–363. doi: 10.1016/j.ijfoodmicro.2005.11.021
Nau, R., and Eiffert, H. (2002). Modulation of release of proinflammatory bacterial compounds by antibacterials: potential impact on course of inflammation and outcome in sepsis and meningitis. Clin. Microbiol. Rev. 15, 95–110. doi: 10.1128/CMR.15.1.95-110.2002
Navarro, F., and Muniesa, M. (2017). Phages in the human body. Front. Microbiol. 8:566. doi: 10.3389/fmicb.2017.00566
Nelson, D. C., Schmelcher, M., Rodriguez-Rubio, L., Klumpp, J., Pritchard, D. G., Dong, S., et al. (2012). Endolysins as antimicrobials. Adv. Virus Res. 83, 299–365. doi: 10.1016/B978-0-12-394438-2.00007-4
Ning, H.-Q., Lin, H., and Wang, J.-X. (2021). Synergistic effects of endolysin Lysqdvp001 and ε-poly-lysine in controlling Vibrio parahaemolyticus and its biofilms. Int. J. Food Microbiol. 343:109112. doi: 10.1016/j.ijfoodmicro.2021.109112
Obeso, J. M., Martínez, B., Rodríguez, A., and García, P. (2008). Lytic activity of the recombinant staphylococcal bacteriophage ΦH5 endolysin active against Staphylococcus aureus in milk. Int. J. Food Microbiol. 128, 212–218. doi: 10.1016/j.ijfoodmicro.2008.08.010
Oliveira, H., Azeredo, J., Lavigne, R., and Kluskens, L. D. (2012). Bacteriophage endolysins as a response to emerging foodborne pathogens. Trends Food Sci. Technol. 28, 103–115. doi: 10.1016/j.tifs.2012.06.016
Oliveira, H., São-José, C., and Azeredo, J. (2018). Phage-derived peptidoglycan degrading enzymes: challenges and future prospects for in vivo therapy. Viruses 10:292. doi: 10.3390/v10060292
Oliveira, H., Thiagarajan, V., Walmagh, M., Sillankorva, S., Lavigne, R., Neves-Petersen, M. T., et al. (2014). A thermostable Salmonella phage endolysin, Lys68, with broad bactericidal properties against gram-negative pathogens in presence of weak acids. PLoS One 9:e108376. doi: 10.1371/journal.pone.0108376
Oyejobi, G. K., Sule, W. F., Akinde, S. B., Khan, F. M., and Ogolla, F. (2022). Multidrug-resistant enteric bacteria in Nigeria and potential use of bacteriophages as biocontrol. Sci. Total Environ. :153842. doi: 10.1016/j.scitotenv.2022.153842
Park, Y., Lim, J. A., Kong, M., Ryu, S., and Rhee, S. (2014). Structure of bacteriophage SPN 1 S endolysin reveals an unusual two-module fold for the peptidoglycan lytic and binding activity. Mol. Microbiol. 92, 316–325. doi: 10.1111/mmi.12555
Pastagia, M., Euler, C., Chahales, P., Fuentes-Duculan, J., Krueger, J. G., and Fischetti, V. A. (2011). A novel chimeric lysin shows superiority to mupirocin for skin decolonization of methicillin-resistant and-sensitive Staphylococcus aureus strains. Antimicrob. Agents Chemother. 55, 738–744. doi: 10.1128/AAC.00890-10
Pennone, V., Sanz-Gaitero, M., O’connor, P., Coffey, A., Jordan, K., Van Raaij, M. J., et al. (2019). Inhibition of L. monocytogenes biofilm formation by the amidase domain of the phage vB_LmoS_293 endolysin. Viruses 11:722. doi: 10.3390/v11080722
Qian, P.-Y., Cheng, A., Wang, R., and Zhang, R. (2022). Marine biofilms: diversity, interactions and biofouling. Nat. Rev. Microbiol. 20, 671–684. doi: 10.1038/s41579-022-00744-7
Ragland, S. A., and Criss, A. K. (2017). From bacterial killing to immune modulation: recent insights into the functions of lysozyme. PLoS Pathog. 13:e1006512. doi: 10.1371/journal.ppat.1006512
Rahimzadeh, G., Gill, P., and Rezai, M. S. (2018). Endolysins of bacteriophages as an anti-methicillin resistant staphylococcus aureus infection in children: a narrative review. J. Pediatr. Rev. 6, 36–43. doi: 10.5812/jpr.11562
Rashel, M., Uchiyama, J., Ujihara, T., Uehara, Y., Kuramoto, S., Sugihara, S., et al. (2007). Efficient elimination of multidrug-resistant Staphylococcus aureus by cloned lysin derived from bacteriophage ϕMR11. J. Infect. Dis. 196, 1237–1247. doi: 10.1086/521305
Rodríguez-Rubio, L., Gerstmans, H., Thorpe, S., Mesnage, S., Lavigne, R., and Briers, Y. (2016). DUF3380 domain from a Salmonella phage endolysin shows potent N-acetylmuramidase activity. Appl. Environ. Microbiol. 82, 4975–4981. doi: 10.1128/AEM.00446-16
Rodríguez-Rubio, L., Martínez, B., Donovan, D. M., García, P., and Rodríguez, A. (2013). Potential of the virion-associated peptidoglycan hydrolase HydH5 and its derivative fusion proteins in milk biopreservation. PLoS One 8:e54828. doi: 10.1371/journal.pone.0054828
Rodríguez-Rubio, L., Martínez, B., Rodríguez, A., Donovan, D. M., and García, P. (2012). Enhanced staphylolytic activity of the Staphylococcus aureus bacteriophage vB_SauS-phiIPLA88 HydH5 virion-associated peptidoglycan hydrolase: fusions, deletions, and synergy with LysH5. Appl. Environ. Microbiol. 78, 2241–2248. doi: 10.1128/AEM.07621-11
Sass, P., and Bierbaum, G. (2007). Lytic activity of recombinant bacteriophage φ11 and φ12 endolysins on whole cells and biofilms of Staphylococcus aureus. Appl. Environ. Microbiol. 73, 347–352. doi: 10.1128/AEM.01616-06
Schirmeier, E., Zimmermann, P., Hofmann, V., Biebl, M., Gerstmans, H., Maervoet, V. E., et al. (2018). Inhibitory and bactericidal effect of Artilysin® Art-175 against colistin-resistant mcr-1-positive Escherichia coli isolates. Int. J. Antimicrob. Agents 51, 528–529. doi: 10.1016/j.ijantimicag.2017.08.027
Schmelcher, M., Donovan, D. M., and Loessner, M. J. (2012a). Bacteriophage endolysins as novel antimicrobials. Future Microbiol. 7, 1147–1171. doi: 10.2217/fmb.12.97
Schmelcher, M., and Loessner, M. J. (2016). Bacteriophage endolysins: applications for food safety. Curr. Opin. Biotechnol. 37, 76–87. doi: 10.1016/j.copbio.2015.10.005
Schmelcher, M., Powell, A. M., Becker, S. C., Camp, M. J., and Donovan, D. M. (2012b). Chimeric phage lysins act synergistically with lysostaphin to kill mastitis-causing Staphylococcus aureus in murine mammary glands. Appl. Environ. Microbiol. 78, 2297–2305. doi: 10.1128/AEM.07050-11
Schmelcher, M., Shabarova, T., Eugster, M. R., Eichenseher, F., Tchang, V. S., Banz, M., et al. (2010). Rapid multiplex detection and differentiation of Listeria cells by use of fluorescent phage endolysin cell wall binding domains. Appl. Environ. Microbiol. 76, 5745–5756. doi: 10.1128/AEM.00801-10
Schmelcher, M., Shen, Y., Nelson, D. C., Eugster, M. R., Eichenseher, F., Hanke, D. C., et al. (2015). Evolutionarily distinct bacteriophage endolysins featuring conserved peptidoglycan cleavage sites protect mice from MRSA infection. J. Antimicrob. Chemother. 70, 1453–1465. doi: 10.1093/jac/dku552
Schmelcher, M., Waldherr, F., and Loessner, M. J. (2012c). Listeria bacteriophage peptidoglycan hydrolases feature high thermoresistance and reveal increased activity after divalent metal cation substitution. Appl. Microbiol. Biotechnol. 93, 633–643. doi: 10.1007/s00253-011-3372-6
Schuch, R., Nelson, D., and Fischetti, V. A. (2002). A bacteriolytic agent that detects and kills Bacillus anthracis. Nature 418, 884–889. doi: 10.1038/nature01026
Seijsing, J., Sobieraj, A. M., Keller, N., Shen, Y., Zinkernagel, A. S., Loessner, M. J., et al. (2018). Improved biodistribution and extended serum half-life of a bacteriophage endolysin by albumin binding domain fusion. Front. Microbiol. 9:2927. doi: 10.3389/fmicb.2018.02927
Sekiya, H., Okada, M., Tamai, E., Shimamoto, T., Shimamoto, T., and Nariya, H. (2021). A putative amidase endolysin encoded by Clostridium perfringens St13 exhibits specific lytic activity and synergizes with the muramidase endolysin psm. Antibiotics 10:245. doi: 10.3390/antibiotics10030245
Shannon, R., Radford, D. R., and Balamurugan, S. (2020). Impacts of food matrix on bacteriophage and endolysin antimicrobial efficacy and performance. Crit. Rev. Food Sci. Nutr. 60, 1631–1640. doi: 10.1080/10408398.2019.1584874
Shavrina, M., Zimin, A., Molochkov, N., Chernyshov, S., Machulin, A., and Mikoulinskaia, G. (2016). In vitro study of the antibacterial effect of the bacteriophage T5 thermostable endolysin on Escherichia coli cells. J. Appl. Microbiol. 121, 1282–1290. doi: 10.1111/jam.13251
Shaw, K. S., Rosenberg Goldstein, R. E., He, X., Jacobs, J. M., Crump, B. C., and Sapkota, A. R. (2014). Antimicrobial susceptibility of Vibrio vulnificus and Vibrio parahaemolyticus recovered from recreational and commercial areas of Chesapeake Bay and Maryland coastal bays. PLoS One 9:e89616. doi: 10.1371/journal.pone.0089616
Shen, K.-S., Shu, M., Tang, M.-X., Yang, W.-Y., Wang, S.-C., Zhong, C., et al. (2022). Molecular cloning, expression and characterization of a bacteriophage JN01 endolysin and its antibacterial activity against E. coli O157: H7. LWT 165:113705. doi: 10.1016/j.lwt.2022.113705
Simmons, M., Morales, C. A., Oakley, B. B., and Seal, B. S. (2012). Recombinant expression of a putative amidase cloned from the genome of Listeria monocytogenes that lyses the bacterium and its monolayer in conjunction with a protease. Probiotics Antimicrob. Proteins 4, 1–10. doi: 10.1007/s12602-011-9084-5
Sohaib, M., Anjum, F. M., Arshad, M. S., and Rahman, U. U. (2016). Postharvest intervention technologies for safety enhancement of meat and meat based products; a critical review. J. Food Sci. Technol. 53, 19–30. doi: 10.1007/s13197-015-1985-y
Solanki, K., Grover, N., Downs, P., Paskaleva, E. E., Mehta, K. K., Lee, L., et al. (2013). Enzyme-based listericidal nanocomposites. Sci. Rep. 3, 1–6. doi: 10.1038/srep01584
Son, B., Kong, M., Cha, Y., Bai, J., and Ryu, S. (2020). Simultaneous control of Staphylococcus aureus and Bacillus cereus using a hybrid endolysin LysB4EAD-LysSA11. Antibiotics 9:906. doi: 10.3390/antibiotics9120906
Son, B., Kong, M., Lee, Y., and Ryu, S. (2021). Development of a novel chimeric endolysin, Lys109 with enhanced lytic activity against Staphylococcus aureus. Front. Microbiol. 11:615887. doi: 10.3389/fmicb.2020.615887
Srinivasan, R., Santhakumari, S., Poonguzhali, P., Geetha, M., Dyavaiah, M., and Xiangmin, L. (2021). Bacterial biofilm inhibition: a focused review on recent therapeutic strategies for combating the biofilm mediated infections. Front. Microbiol. 12:676458. doi: 10.3389/fmicb.2021.676458
Stone, E., Pennone, V., Reilly, K., Grant, I. R., Campbell, K., Altermann, E., et al. (2022). Inhibition of Listeria monocytogenes by phage lytic enzymes displayed on tailored bionanoparticles. Foods 11:854. doi: 10.3390/foods11060854
Swift, S. M., Waters, J. J., Rowley, D. T., Oakley, B. B., and Donovan, D. M. (2018). Characterization of two glycosyl hydrolases, putative prophage endolysins, that target Clostridium perfringens. FEMS Microbiol. Lett. 365:fny179. doi: 10.1093/femsle/fny179
Sykilinda, N. N., Nikolaeva, A. Y., Shneider, M. M., Mishkin, D. V., Patutin, A. A., Popov, V. O., et al. (2018). Structure of an Acinetobacter broad-range prophage endolysin reveals a C-terminal α-helix with the proposed role in activity against live bacterial cells. Viruses 10:309. doi: 10.3390/v10060309
Tamai, E., Yoshida, H., Sekiya, H., Nariya, H., Miyata, S., Okabe, A., et al. (2014). X-ray structure of a novel endolysin encoded by episomal phage phiSM 101 of C lostridium perfringens. Mol. Microbiol. 92, 326–337. doi: 10.1111/mmi.12559
Thummeepak, R., Kitti, T., Kunthalert, D., and Sitthisak, S. (2016). Enhanced antibacterial activity of Acinetobacter baumannii bacteriophage ØABP-01 endolysin (LysABP-01) in combination with colistin. Front. Microbiol. 7:1402. doi: 10.3389/fmicb.2016.01402
Timbermont, L., Haesebrouck, F., Ducatelle, R., and Van Immerseel, F. (2011). Necrotic enteritis in broilers: an updated review on the pathogenesis. Avian Pathol. 40, 341–347. doi: 10.1080/03079457.2011.590967
Van Tassell, M. L., Ibarra-Sánchez, L. A., Hoepker, G. P., and Miller, M. J. (2017). Hot topic: Antilisterial activity by endolysin PlyP100 in fresh cheese. J. Dairy Sci. 100, 2482–2487. doi: 10.3168/jds.2016-11990
Vázquez-Boland, J. A., Kuhn, M., Berche, P., Chakraborty, T., DomliNguez-Bernal, G., Goebel, W., et al. (2001). Listeria pathogenesis and molecular virulence determinants. Clin. Microbiol. Rev. 14, 584–640. doi: 10.1128/CMR.14.3.584-640.2001
Wang, Q., Euler, C. W., Delaune, A., and Fischetti, V. A. (2015). Using a novel lysin to help control Clostridium difficile infections. Antimicrob. Agents Chemother. 59, 7447–7457. doi: 10.1128/AAC.01357-15
Wang, C.-Y., Huang, H.-W., Hsu, C.-P., and Yang, B. B. (2016). Recent advances in food processing using high hydrostatic pressure technology. Crit. Rev. Food Sci. Nutr. 56, 527–540. doi: 10.1080/10408398.2012.745479
Wang, W., Li, M., Lin, H., Wang, J., and Mao, X. (2016). The Vibrio parahaemolyticus-infecting bacteriophage qdvp001: genome sequence and endolysin with a modular structure. Arch. Virol. 161, 2645–2652. doi: 10.1007/s00705-016-2957-x
Wang, B., Yao, M., Lv, L., Ling, Z., and Li, L. (2017). The human microbiota in health and disease. Engineering 3, 71–82. doi: 10.1016/J.ENG.2017.01.008
Wernicki, A., Nowaczek, A., and Urban-Chmiel, R. (2017). Bacteriophage therapy to combat bacterial infections in poultry. Virol. J. 14, 1–13. doi: 10.1186/s12985-017-0849-7
Wu, M., Hu, K., Xie, Y., Liu, Y., Mu, D., Guo, H., et al. (2019). A novel phage PD-6A3, and its endolysin Ply6A3, with extended lytic activity against Acinetobacter baumannii. Front. Microbiol. 9:3302. doi: 10.3389/fmicb.2019.00196
Xu, Y. (2021). Phage and phage lysins: new era of bio-preservatives and food safety agents. J. Food Sci. 86, 3349–3373. doi: 10.1111/1750-3841.15843
Xu, S., Campisi, E., Li, J., and Fischetti, V. A. (2021). Decontamination of Escherichia coli O157: H7 on fresh Romaine lettuce using a novel bacteriophage lysin. Int. J. Food Microbiol. 341:109068. doi: 10.1016/j.ijfoodmicro.2021.109068
Yan, G., Liu, J., Ma, Q., Zhu, R., Guo, Z., Gao, C., et al. (2017). The N-terminal and central domain of colicin a enables phage lysin to lyse Escherichia coli extracellularly. Antonie Van Leeuwenhoek 110, 1627–1635. doi: 10.1007/s10482-017-0912-9
Yang, Q., Ding, Y., Nie, R., Yao, L., Wang, X., Zhou, M., et al. (2020). Characterization of a novel T7-like Salmonella Typhimurium (ATCC13311) bacteriophage LPST144 and its endolysin. LWT 123:109034
Yang, W., Gondil, V. S., Luo, D., He, J., Wei, H., and Yang, H. (2021). Optimized silica-binding peptide-mediated delivery of bactericidal Lysin efficiently prevents Staphylococcus aureus from adhering to device surfaces. Int. J. Mol. Sci. 22:12544. doi: 10.3390/ijms222212544
Yang, Y., Le, S., Shen, W., Chen, Q., Huang, Y., Lu, S., et al. (2018). Antibacterial activity of a lytic enzyme encoded by Pseudomonas aeruginosa double stranded RNA bacteriophage phiYY. Front. Microbiol. 9:1778. doi: 10.3389/fmicb.2018.01778
Yang, H., Linden, S. B., Wang, J., Yu, J., Nelson, D. C., and Wei, H. (2015a). A chimeolysin with extended-spectrum streptococcal host range found by an induced lysis-based rapid screening method. Sci. Rep. 5, 1–12. doi: 10.1038/srep17257
Yang, H., Luo, D., Etobayeva, I., Li, X., Gong, Y., Wang, S., et al. (2020). Linker editing of pneumococcal lysin ClyJ conveys improved bactericidal activity. Antimicrob. Agents Chemother. 64, e01610–e01619. doi: 10.1128/AAC.01610-19
Yang, H., Wang, M., Yu, J., and Wei, H. (2015b). Antibacterial activity of a novel peptide-modified lysin against Acinetobacter baumannii and Pseudomonas aeruginosa. Front. Microbiol. 6:1471. doi: 10.3389/fmicb.2015.01471
Yang, H., Zhang, Y., Huang, Y., Yu, J., and Wei, H. (2014a). Degradation of methicillin-resistant Staphylococcus aureus biofilms using a chimeric lysin. Biofouling 30, 667–674. doi: 10.1080/08927014.2014.905927
Yang, H., Zhang, H., Wang, J., Yu, J., and Wei, H. (2017). A novel chimeric lysin with robust antibacterial activity against planktonic and biofilm methicillin-resistant Staphylococcus aureus. Sci. Rep. 7, 1–13.
Yang, H., Zhang, Y., Yu, J., Huang, Y., Zhang, X.-E., and Wei, H. (2014b). Novel chimeric lysin with high-level antimicrobial activity against methicillin-resistant Staphylococcus aureus in vitro and in vivo. Antimicrob. Agents Chemother. 58, 536–542. doi: 10.1128/AAC.01793-13
Yoong, P., Schuch, R., Nelson, D., and Fischetti, V. A. (2004). Identification of a broadly active phage lytic enzyme with lethal activity against antibiotic-resistant enterococcus faecalis and Enterococcus faecium. J. Bacteriol. 186, 4808–4812. doi: 10.1128/JB.186.14.4808-4812.2004
Yu, J., Zhang, Y., Li, H., Yang, H., and Wei, H. (2016). Sensitive and rapid detection of Staphylococcus aureus in milk via cell binding domain of lysin. Biosens. Bioelectron. 77, 366–371. doi: 10.1016/j.bios.2015.09.058
Yuan, Y., Li, X., Wang, L., Li, G., Cong, C., Li, R., et al. (2021). The endolysin of the Acinetobacter baumannii phage vB_AbaP_D2 shows broad antibacterial activity. Microb. Biotechnol. 14, 403–418. doi: 10.1111/1751-7915.13594
Zampara, A., Sørensen, M. C. H., Gencay, Y. E., Grimon, D., Kristiansen, S. H., Jørgensen, L. S., et al. (2021). Developing innolysins against campylobacter jejuni using a novel prophage receptor-binding protein. Front. Microbiol. 12:619028. doi: 10.3389/fmicb.2021.619028
Zampara, A., Sørensen, M. C. H., Grimon, D., Antenucci, F., Vitt, A. R., Bortolaia, V., et al. (2020). Exploiting phage receptor binding proteins to enable endolysins to kill gram-negative bacteria. Sci. Rep. 10:12087. doi: 10.1038/s41598-020-68983-3
Zermeño-Cervantes, L. A., Makarov, R., Lomelí-Ortega, C. O., Martínez-Díaz, S. F., and Cardona-Félix, C. S. (2018). Recombinant Lys VPMS 1 as an endolysin with broad lytic activity against Vibrio parahaemolyticus strains associated to acute hepatopancreatic necrosis disease. Aquac. Res. 49, 1723–1726. doi: 10.1111/are.13577
Zhang, H., Bao, H., Billington, C., Hudson, J. A., and Wang, R. (2012). Isolation and lytic activity of the Listeria bacteriophage endolysin LysZ5 against Listeria monocytogenes in soya milk. Food Microbiol. 31, 133–136. doi: 10.1016/j.fm.2012.01.005
Zhang, Y., Huang, H.-H., Duc, H. M., Masuda, Y., Honjoh, K.-I., and Miyamoto, T. (2021). Endolysin LysSTG2: characterization and application to control Salmonella Typhimurium biofilm alone and in combination with slightly acidic hypochlorous water. Food Microbiol. 98:103791. doi: 10.1016/j.fm.2021.103791
Zhang, Z., Lahti, M., Douillard, F. P., Korkeala, H., and Lindström, M. (2020). Phage lysin that specifically eliminates Clostridium botulinum group I cells. Sci. Rep. 10, 1–10. doi: 10.1038/s41598-020-78622-6
Zhang, L., Li, D., Li, X., Hu, L., Cheng, M., Xia, F., et al. (2016). LysGH15 kills Staphylococcus aureus without being affected by the humoral immune response or inducing inflammation. Sci. Rep. 6, 1–9. doi: 10.1038/srep29344
Zhao, X., Li, L., Zhang, Q., Li, M., Hu, M., Luo, Y., et al. (2023). Characterization of the Clostridium perfringens phage endolysin cpp-lys and its application on lettuce. Int. J. Food Microbiol. 405:110343. doi: 10.1016/j.ijfoodmicro.2023.110343
Zhou, Y., Zhang, H., Bao, H., Wang, X., and Wang, R. (2017). The lytic activity of recombinant phage lysin LysKΔamidase against staphylococcal strains associated with bovine and human infections in the Jiangsu province of China. Res. Vet. Sci. 111, 113–119. doi: 10.1016/j.rvsc.2017.02.011
Keywords: antimicrobial resistance, bacteriophage, endolysin, foodborne bacterial pathogens, food safety
Citation: Khan FM, Chen J-H, Zhang R and Liu B (2023) A comprehensive review of the applications of bacteriophage-derived endolysins for foodborne bacterial pathogens and food safety: recent advances, challenges, and future perspective. Front. Microbiol. 14:1259210. doi: 10.3389/fmicb.2023.1259210
Edited by:
Chunlei Shi, Shanghai Jiao Tong University, ChinaReviewed by:
Heejoon Myung, Hankuk University of Foreign Studies, Republic of KoreaAhmed Askora, Zagazig University, Egypt
Copyright © 2023 Khan, Chen, Zhang and Liu. This is an open-access article distributed under the terms of the Creative Commons Attribution License (CC BY). The use, distribution or reproduction in other forums is permitted, provided the original author(s) and the copyright owner(s) are credited and that the original publication in this journal is cited, in accordance with accepted academic practice. No use, distribution or reproduction is permitted which does not comply with these terms.
*Correspondence: Rui Zhang, cnVpemhhbmdAc3p1LmVkdS5jbg==; Bin Liu, bGl1YmluQHN6dS5lZHUuY24=