- 1Faculty of Biological and Environmental Sciences, University of Helsinki, Lahti, Finland
- 2Research Institute for Environmental Studies, Parede, Portugal
- 3Natural Resources Institute Finland (Luke), Horticulture Technologies, Turku, Finland
Bioremediation by in situ biostimulation is an attractive alternative to excavation of contaminated soil. Many in situ remediation methods have been tested with some success; however, due to highly variable results in realistic field conditions, they have not been implemented as widely as they might deserve. To ensure success, methods should be validated under site-analogous conditions before full scale use, which requires expertise and local knowledge by the implementers. The focus here is on indigenous microbial degraders and evaluation of their performance. Identifying and removing biodegradation bottlenecks for degradation of organic pollutants is essential. Limiting factors commonly include: lack of oxygen or alternative electron acceptors, low temperature, and lack of essential nutrients. Additional factors: the bioavailability of the contaminating compound, pH, distribution of the contaminant, and soil structure and moisture, and in some cases, lack of degradation potential which may be amended with bioaugmentation. Methods to remove these bottlenecks are discussed. Implementers should also be prepared to combine methods or use them in sequence. Chemical/physical means may be used to enhance biostimulation. The review also suggests tools for assessing sustainability, life cycle assessment, and risk assessment. To help entrepreneurs, decision makers, and methods developers in the future, we suggest founding a database for otherwise seldom reported unsuccessful interventions, as well as the potential for artificial intelligence (AI) to assist in site evaluation and decision-making.
Introduction
Contaminated soils have become a persistent environmental problem that has not received sufficient attention when compared with polluted waters and air. This is illustrated by the fact that there has been a water directive at the EU-level since the year 2000 (EUR-Lex, 2000) and an air quality directive since 2008 (EUR-Lex, 2008), but only now the framework and concrete steps toward protection, restoration and sustainable use of soils are set by the EU in EU soil strategy for 2030 (European Commission, 2017, 2023). As part of this new strategy, a new Soil Monitoring Law has been proposed to address key soil threats in the EU, such as contamination (European Commission, 2017, 2023). The new law puts in place a solid and coherent monitoring framework, makes sustainable soil management the norm in the EU and requests EU Member States to identify potentially contaminated sites, investigate these sites and address unacceptable risks for human health and the environment (European Commission, 2017, 2023). Along with risks to the environment, polluted soil and environmental contaminants pose both direct and indirect risks to human health and well-being (Parajuli et al., 2018; Roslund et al., 2019). The soil, especially clay soil, forms a reservoir for pollutants that can desorb into water and volatilize, or desorb and evaporate into the air (Pukkila and Kontro, 2014; Mattsson et al., 2015).
Bioremediation and in situ treatment methods as concepts usually generate initial enthusiasm and favorable comments from regulatory authorities and remediation contractors. The actual implementation of these techniques in practice is, however, often met with skepticism. Authorities commonly do not want to take responsibility for the effectiveness of what are seen as novel techniques, and entrepreneurs want to use old, familiar methods and avoid unnecessary financial risks (Hannu Silvennoinen, personal communication).
Exceptions to these skeptical attitudes can be found in both the public and private sectors. Hannu Silvennoinen, CEO of Nordic Envicon, referencing Dahl et al. (2013), has pointed out that “even reaching a partial goal of decreasing the volume [of contaminated soil] that requires excavation saves a substantial amount of both [in terms of] money and environment[al impact].” Nikunen et al. (2017), in calculating the results from the SOILI program, found that in situ treatment could achieve average savings of 30% over traditional methods.
The use of monitored natural attenuation may also be a viable solution whenever a thoroughly performed site analysis and risk assessment (RA) has concluded that there is no risk for spread of the contaminant and that contaminant concentration reduction takes place naturally at a satisfactory rate (Simpanen, 2016; Fernandez-Lopez et al., 2022). RA is a sensible way to evaluate whether a site no longer poses an environmental or health risk. Locally applied regulations and tools may be needed, but the principle has backing both in the literature (Öberg and Bergbäck, 2005; Latawiec et al., 2010) and in regulatory recommendations (Ministry of the Environment, 2014). Even so, realistic bioremediation plans are often abandoned due to unrealistic and counterproductive demands to reach below target concentrations in every sample. It is therefore commendable that authorities in Finland (FINLEX, 2007), based on international and EU trends, recommend RA rather than absolute preset targets.
If an organic contaminant is principally biodegradable, but shows no trend of reduction in concentration, there is likely to be one or more bottlenecks for active degradation that need to be removed. Identifying these bottlenecks is therefore crucial. Furthermore, all bottlenecks need to be identified and amended in order to generate a desired rate of biodegradation and contaminant removal (Table 1).
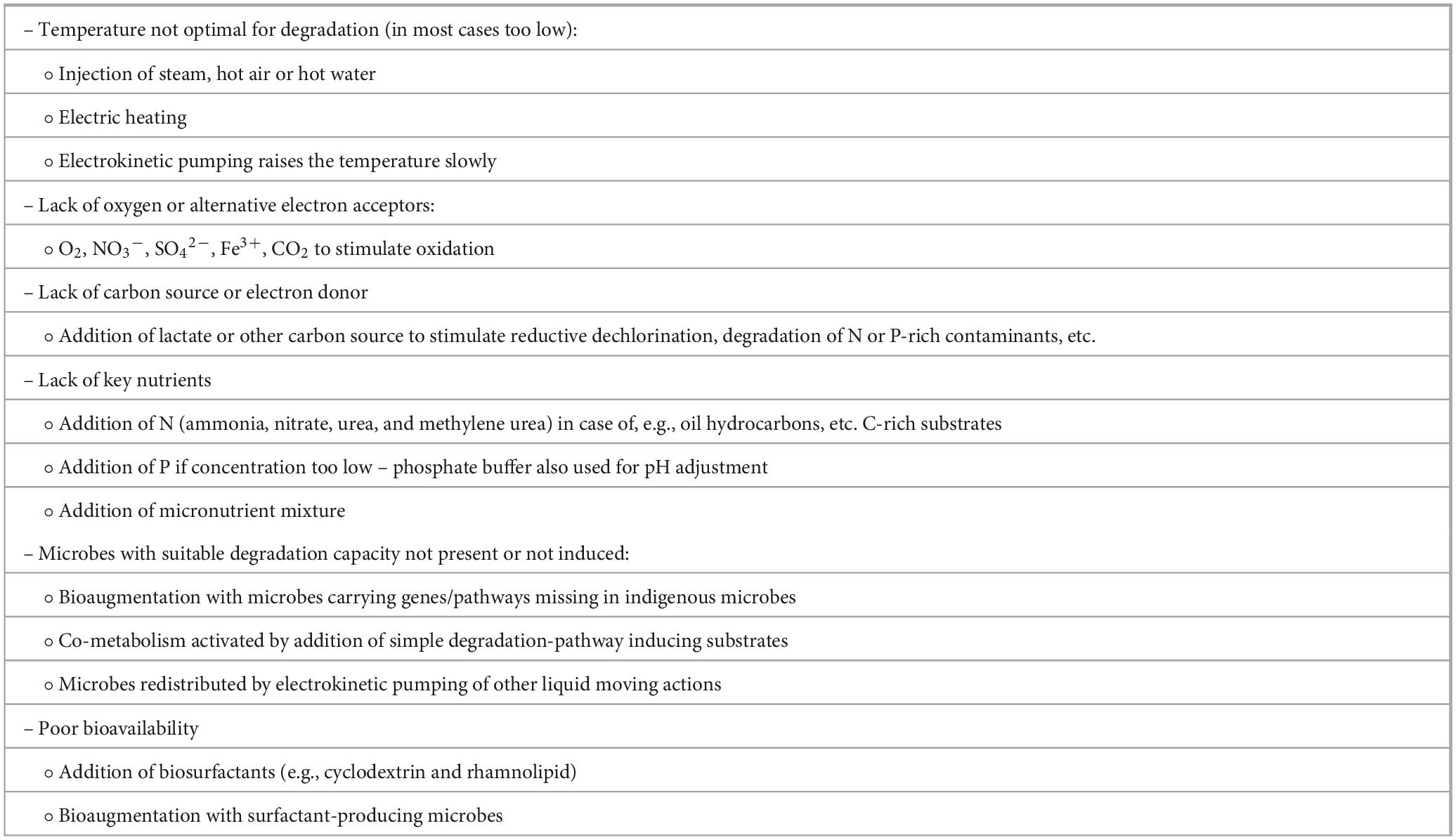
Table 1. Microbial remediation bottlenecks and suggested solutions (activities elucidated in the text).
To speed up bioremediation, the local situation should be modified to allow sufficiently vigorous microbial growth and metabolism in a manner where the target contaminant or some component of it is made to be the limiting factor for growth. If this is achieved, other microbes competing for resources (e.g., electron acceptors) cannot out-compete the relevant degraders. This should be kept in mind, for example, when adding carbon and energy sources, such as easily degradable biomass during bioaugmentation, since competing metabolism may deplete the oxygen supply. If the projected degradation takes place in aerobic conditions any addition that can be oxidized may cause delays in target contaminant degradation (Simpanen et al., 2016b; Talvenmäki et al., 2021), while in contrast, anoxic reducing conditions may be desirable if de-chlorination or denitrification is the goal (Conrad et al., 2010).
In some situations co-degradation of contaminants upon, e.g., addition of general stimulation of microbial activity has been suggested (Conrad et al., 2010) but this approach may lead to degradation of the stimulating agent rather than the contaminant (Talvenmäki et al., 2021).
Environmental parameters such as temperature, availability of oxygen and nutrients, should be optimized and targeted for the bacterial consortium with the highest degradation potential. Thus, if the contaminant is a petroleum hydrocarbon (PHC), this contaminant should be made the limiting carbon and energy source, while if the contaminant is an organonitrogen or organophosphate pesticide, nitrogen or phosphorus, respectively, should be made the limiting factor (Figure 1).
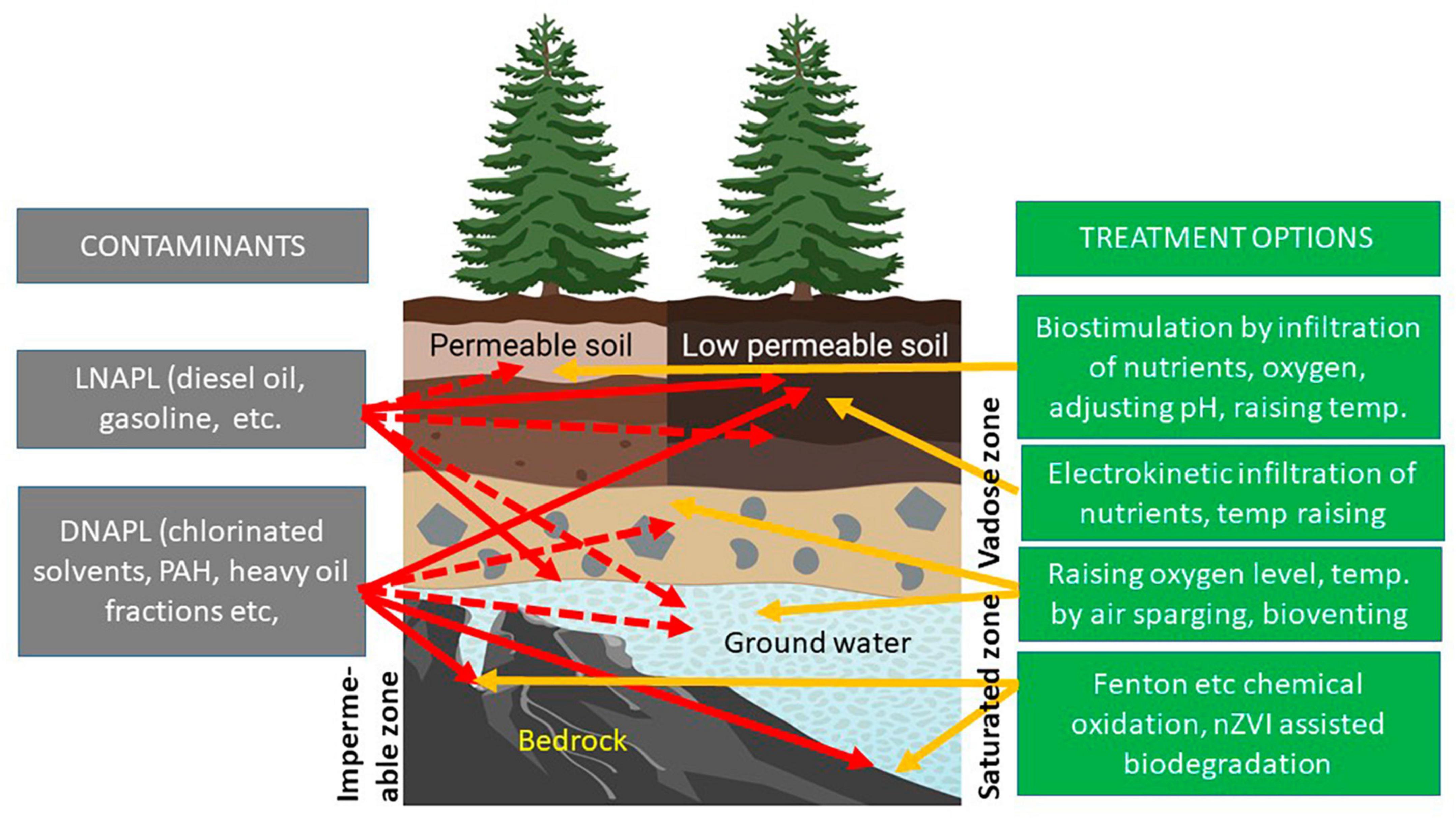
Figure 1. Contaminated scenarios and options for activity. Assumed situation: contamination is not fresh and rapid changes no longer seen. Based on analysis of soil, ground water, and local conditions, a strategy is drawn up, and if possible, tested in laboratory conditions. Red arrows: contaminant location – solid, main contaminant locality; dashed, residual concentrations or area of limited spread. Yellow arrows: suggestion of method and target for intervention. Ground water may contain plumes, depending on contaminant solubility.
The target values to reach with regards to temperature, redox potential, pH, etc. have to be chosen based on an educated guess. Soil temperatures at 1–2 m below the surface varies only a little with the seasons, and deeper down it is almost constant. In boreal conditions subsurface soil temperatures are below 10°C and the soil does not freeze, partly due to the insulating snow cover. At a site in South-Eastern Finland the ground temperature stayed at 4–7°C during a 4 month period, September to December at a depth of 1.5 m (Suni et al., 2007). In such conditions the microbial profile is predominantly psychrophilic and mesophilic, and excessive temperature increases appear to limit microbial activity (Suni et al., 2007), while a modest in situ elevation to between 15 and 20°C has been observed to boost microbial activity (Simpanen et al., 2018; Talvenmäki, 2020).
Classification of contaminants, remediation, and site evaluation methods
Soil organic pollutants can be classified into the following, partly overlapping groups: explosives, pesticides (DDT and its metabolites, triazines, etc.), halogenated hydrocarbons (polychlorinated biphenyls, PCBs), flame-retardants (polybrominated diphenyl ethers, PBDEs), surfactants, PHCs and related plastic pollution, polyaromatic hydrocarbons (PAHs), dioxins, and medical waste/pharmaceuticals (Jones and de Voogt, 1999; McGrath et al., 2017; Kraemer et al., 2019; Verla et al., 2019; Tauqeer et al., 2021). Environmental remediation of these pollutants usually starts with identifying the problem, i.e., the extent of the pollution; contaminants, their concentrations and stability of hazardous compounds; soil type(s) including physical, chemical, and microbiological properties of the matrix; water flow conditions; etc. Additionally, the effect of removing the contamination source on the lifetime of the contaminate should be estimated based on its degradation rate (Pande et al., 2020; Patel et al., 2020; Bala et al., 2022). A computational framework is under development for predictive bioremediation to assess environmental fate in remediation approaches based on molecular dynamics simulation, and biodegradation pathway predictions to overcome limited information about the site studies (Singh et al., 2021). Remediation performance must be monitored and that may require the installation of inspection system components, such as piping, pumps, and valves. Parameters typically monitored include pH, dissolved oxygen, temperature, mineral nutrient levels, flow rates (air and water), and pumping rates. Microbial community composition, including the number of specific contaminant-degrading microbial subgroups, and the overall microbial population density should be determined. The decision to end remediation activities should be based on the effectiveness of the remediation results (Pande et al., 2020; Patel et al., 2020; Bala et al., 2022).
In recent years a number of novel methods for remediation of contaminated sites have been developed. Description and classification of these methods and of contaminated sites are presented in the scientific literature and in guidelines (Romantschuk et al., 2000; Volchko et al., 2014; Rosén et al., 2015; Singh et al., 2015; Norrman et al., 2020; Table 1).
Remediation technologies can be subdivided into two broad classes, ex situ and in situ. Ex situ techniques include the more “traditional” remediation methods, which typically require the excavation and transportation of contaminated soils for treatment. In situ techniques, in contrast, do not include excavation, transportation and isolated treatment of the contaminated sites. While ex situ methods, such as landfarming and biopiles, may incorporate bioremediation in the treatment process, as a rule, in situ techniques are gentler, less invasive, and thus have a less negative impact on the environment (Nikunen et al., 2017; USEPA, 2017; Bajagain et al., 2020a; Fernandez-Lopez et al., 2022). Various in situ methods offer important advantages, especially when traditional, off-site “dig and dump” approaches are ruled out by technical or economic demands. When a successful outcome can be achieved in situ, the demands of sustainability are achieved on all fronts: the reduced need for transportation means reduced CO2 emissions and fuel use, resulting also in significant financial savings (Simpanen, 2016). Social and/or economic benefits are reaped from the fact that normal activities at the site can continue and buildings and structures are undisturbed during the treatment, as opposed to off-site methods which are often invasive and disruptive (Simpanen et al., 2016b).
In natural attenuation, or passive in situ treatment, the contaminated soil is remediated by naturally occurring biological, chemical, and physical processes. With bioavailable and easily degradable pollutants a successful end result may be achieved with natural attenuation alone (Penttinen, 2001) largely based on redox-reactions (Tuomi and Vaajasaari, 2004). However, some monitoring is still needed for natural attenuation to be considered an actual treatment method (Wilms et al., 2023).
Active in situ methods for removal of organics from soil may include biological as well as physical or chemical methods. In this review we focus on biodegradation but touch upon physico-chemical techniques when combined or necessary to achieve biodegradation via biostimulation (Table 2).
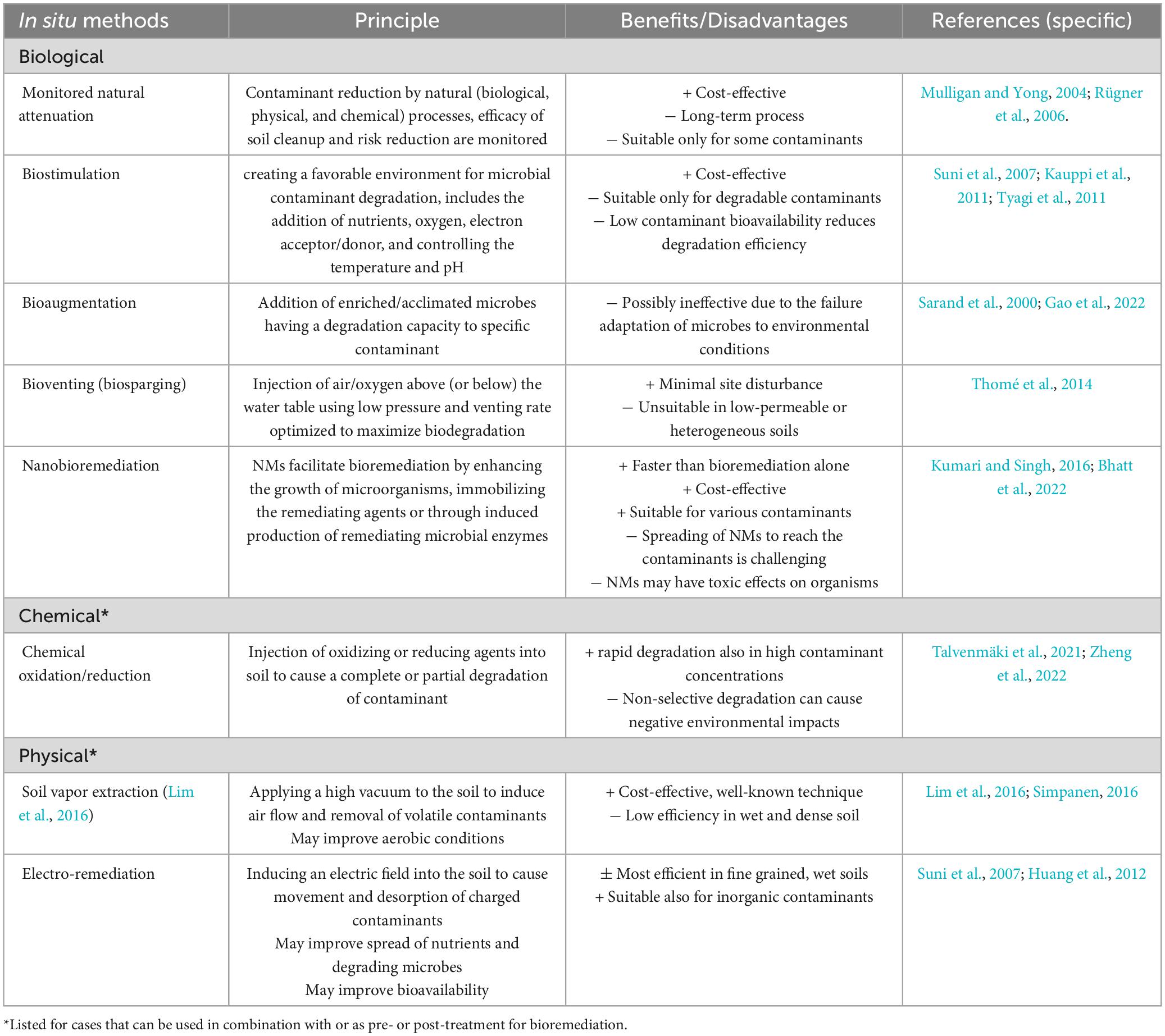
Table 2. Description of various in situ soil remediation methods including some of their benefits and disadvantages (general references: Romantschuk et al., 2000; Penttinen, 2001; Nathanail et al., 2007; USEPA, 2007, 2013; Tomei and Daugulis, 2013).
Microbial bioremediation – forms, prerequisites, and conditions
Bioremediation is a process that mainly relies on the use of microorganisms to remove pollutants from contaminated areas in order to regain the original condition of the site. The process involves biological degradation of organic pollutants to carbon dioxide and water, or to less toxic intermediates under natural conditions (Romantschuk et al., 2000). Although plants and their rhizosphere can also positively influence conditions, the focus here is on active bioremediation via biostimulation.
In bioattenuation, biostimulation, and bioaugmentation, microorganisms, either indigenous or introduced, play the main role (Kauppi et al., 2011, 2012; Simpanen et al., 2016a,b; Talvenmäki et al., 2021). The choice of a particular bioremediation technology should be based on careful planning and is dependent on several factors, such as the characteristics of the polluted soil or water, state of the indigenous microbial population and the type and concentration of pollutants (Nikunen et al., 2017).
In the case of readily biodegradable organic compounds such as PHC, mineralization of the contaminant is largely dictated by bacteria (Romantschuk et al., 2000; Mukherjee et al., 2015; Simpanen et al., 2016a), but recent research shows that Archaea may also be involved (Yan et al., 2018, 2020). To facilitate bioremediation in such cases, soil conditions need to be optimized for bacterial activity and proliferation, which will accelerate removal of the pollutant. Bottlenecks for bacterial activity, such as lack of oxygen or an alternative electron acceptor, suboptimal nutrient balance, and moisture level and/or temperature should be targeted for optimization efforts (Margesin, 2000; Simpanen et al., 2016a,b; Cavazzoli et al., 2022, 2023). Suitable pH and improved bioavailability of the contaminant can also be obtained via additives (Penttinen, 2001; Peltola et al., 2006; Suni et al., 2007; Talvenmäki et al., 2021). An aquatic carrier is often used with an obvious biostimulative role in itself (Khalladi et al., 2009). Aerobic degradation is often more efficient than anaerobic (Tarasov et al., 2004; Kauppi et al., 2011), encouraging the addition of oxygen as a gas straight into the soil or the aquatic phase (Penttinen, 2001), or as a breakdown product of chemicals such as peroxide, persulfate, ozone or permanganate (Tarasov et al., 2004; Goi et al., 2006; Yen et al., 2011; Nykänen et al., 2012; Simpanen et al., 2016a; Bajagain et al., 2018, 2020b). The dissolvability of oxygen is comparatively low; alternatives like nitrate and sulfate sidestep this problem but come with a heightened risk of groundwater pollution (Chen et al., 2022).
In bioremediation, nitrogen and phosphorus are the pivotal macronutrients (Chaîneau et al., 2005) whereas the former is often the sole limiting nutrient in terrestrial systems (Chapin et al., 2011). The unbalanced Corg:N:P ratio resulting from the excess carbon of the organic contaminant can thus be optimized with additions of nitrogen. The optimal Corg:N ratio of 10:1 is difficult to achieve with urea, the most commonly used additive, since the ammonium carbonate producing reaction of urea hydrolysis often increases soil pH to undesired levels (Peltola et al., 2006) that in turn escalates the hydrolysis reaction, resulting in nitrogen leaching trough volatilization of ammonium (Jones et al., 2007). Ideally the availability of nitrogen should be adjusted to the bioavailable portion of the carbon rich contaminant rather than the total amount. This can be facilitated by using methylene urea (MU), a condensation product of urea and formaldehyde in which the length and degree of polymerization affects the pace of release (Koivunen et al., 2004; Peltola et al., 2006). The MU is degraded at a rate that portions out nitrogen in balance with the available carbon. The benefits of using nitrate as the nitrogen source are that it can also function as an electron acceptor (Kim et al., 2013; Chen et al., 2022) and is readily soluble in water as opposed to gaseous oxygen.
Biostimulation and reductive dechlorination
The emerging organohalide contaminants are widely occurring in the environment and suitable for bioremediation by reductive dechlorination or halorespiration. These include chlorinated solvents, PCBs with many different molecular structures, and promising remediation results have also been obtained with perfluoroalkyl and polyfluoroalkyl substances, as well as halogenated flame retardants (Huang and Jaffé, 2019; Yu et al., 2020; He et al., 2021). Contaminants such as chlorinated solvents and PCBs in their oxidized states are not susceptible to oxidation under aerobic conditions, leading to microbial biodegradation via reductive dechlorination under anaerobic conditions, which is the main pathway for their degradation (Dutta et al., 2022). In the reductive dechlorination, cellular energy is produced by replacing one or more chlorine atoms with an electron-donating hydrogen molecule made available by adding organic substances (Tiehm and Schmidt, 2011).
One of the most commonly cited examples of reductive dechlorination is the metabolism of chloroethenes, i.e., the dechlorination of tetrachloroethene (PCE) to trichloroethene (TCE), then to dichloroethene isomers (cis-DCE and trans-DCE), and finally to vinyl chloride (VC) and ethane. Among these metabolites, PCE and TCE, as the most oxidized molecules, are most susceptible to reductive dechlorination, while VC has slower reaction rates and risk of accumulation, co-metabolism being one option for VC degradation. Chloroethenes with the highest chlorine number inhibit the reductive dechlorination of molecules with less chlorine (Tiehm and Schmidt, 2011). Reductive dechlorination of PCBs is more complex and slower than that of chloroethenes, and its progression is related to geochemistry and the specific microorganisms present in the polluted environment (Wiegel and Wu, 2000; Xiao et al., 2020). Brominated molecules, such as PBDEs, and decabromodiphenyl ether can also be degraded by microbial reductive debromination (Gerecke et al., 2005; Robrock et al., 2008). Although the C-F bond is one of the strongest in nature, Acidimicrobium sp. oxidizing ammonium to nitrite and reducing Fe(III) was capable of defluorinating per- and polyfluoroalkyl substances using hydrogen as the sole electron donor instead of ammonium (Huang and Jaffé, 2019).
Reductive dehalogenation can be performed by iron- and sulfate-reducing microbes, as well as by methanogenic and fermentative microbial communities, i.e., reductive dechlorination is a cometabolic or respiratory process (Löffler et al., 1999; Yuan et al., 2021). The energy released in reductive dechlorination of 135–187 kJ/mol is much greater than the energy available from sulfate reduction (38 kJ/mol) and methanogenesis (31 kJ/mol), while as much as 228 kJ/mol of energy is released in Fe(III) reduction (Löffler et al., 1999; Dolfing, 2000). Microorganisms that respire chloroethenes and PCBs most typically belong to Firmicutes, Chloroflexi, or Proteobacteria. In particular, the genus Dehalococcoides is related to the reductive dechlorination of chlorinated hydrocarbons. Other possible genera for dechlorination include Anaeromyxobacter, Dehalobacter, Dehalogenimonas, Desulfitobacterium, Desulfoluna, Desulfomonile, Desulfovibrio, Desulfuromonas, Geobacter, Propionibacterium, and Sulfurospirillum (Xiao et al., 2020). Acidimicrobium sp. strain A6 has been reported to defluorinate per- and polyfluoroalkyl substances (Huang and Jaffé, 2019). In addition, methane oxidizing microorganisms, such as Methylomonas sp. and Methylosinus sp. have been associated with dechlorination through co-metabolism (Xiao et al., 2020). Typically, a decomposing microbial community develops naturally in an environment exposed to pollutants, and the same microorganisms live in similar environments in nature. Because of this, remediating microbes should be isolated from an environment similar to where they are intended to be used in bioaugmentation (Liu et al., 2015).
Slow-growing, anaerobic Dehalococcoides spp. with a preference for neutral pH, carry as many as 14 different reductive dechlorinase genes in their genome. The genes of different strains are typically non-identical, and therefore Dehalococcoides strains have variable ability to degrade organohalides (Hölscher et al., 2004). The dechlorination pathways may even differ between two Dehalococcoides strains (Cheng and He, 2009). The strain Dehalococcoides ethenogens is among the rare isolates that can completely dechlorinate PCE and metabolites to ethene. Examples of different reductive dechlorinase genes include: the tceA gene, which encodes a TCE reductive dehalogenase enzyme that catalyzes the dechlorination of TCE, 1,2-dichloroethane, 1,2-dibromoethane, and dichloroethenes to ethene (Magnuson et al., 2000); the enzyme VC-reductive halogenase of Dehalococcoides sp. strain VS, encoded by the vcrA gene, which reduces VC and all dichloroethene isomers to ethene (Müller et al., 2004); and the bvcA gene of Dehalococcoides sp. strain BAV1, which is involved in the reductive dechlorination of VC to ethene (Krajmalnik-Brown et al., 2004). Real-time PCR assays have been designed to detect Dehalococcoides from 16S rDNA and genes bvcA, vcrA, and tceA to monitor the performance of the reductive dechlorination remediation process, and evaluate solutions for problems in remediation practices (He et al., 2003; Johnson et al., 2005; Ritalahti et al., 2006; Krzmarzick et al., 2012).
In a review of remediation practices at 191 chlorinated solvent-contaminated sites in the United States and Europe, monitored natural attenuation was implemented at approximately 30% of sites, while additional treatment approaches were applied at other sites (McGuire et al., 2004). With PCBs, natural, in situ reductive dechlorination proceeds slowly (Wiegel and Wu, 2000; Xiao et al., 2020). Sources of suitable nutrients and electron donors such as phosphorus or nitrogen, and organic substrates are usually insufficient to achieve natural attenuation. To overcome these problems, biostimulation with nutrient delivery can be used to stimulate the native dechlorinating microbial community, and this has been applied in many aquifers in situ to enhance degradation of chlorinated solvents. The substrates used to provide electrons for reductive dechlorination include methane, acetate, pyruvate, methanol, ethanol, lactate, propionate, butyrate, glucose, etc. Fermentation of these carbon sources produces electron donors, such as hydrogen. In addition to acting as an electron donors, added carbon sources can generally promote microbial growth, oxygen consumption, and creation of anoxic conditions required for reductive dechlorination. Lactate, one of the most commonly used substrates, and ethanol both results in the fermentation of acetate (Hood et al., 2008; Blázquez-Pallí et al., 2019). As the remediation of chlorinated hydrocarbons is often a long-duration process, slowly releasing carbon and electron sources can also be used, such as whey, fatty acid mixtures, vegetable oil, carboxymethylcellulose, polylactic acid, polyhydroxyalkanoates, etc. (Borden et al., 2007; Lee et al., 2008; Kocur et al., 2016; Pierro et al., 2017; Sun et al., 2022).
Other methods combined with biostimulation of reductive dechlorination include the addition of micro- and nano-scale zero valent iron (ZVI), which enhances the simultaneous abiotic dissipation of contaminants (Xie et al., 2017).
Biosurfactants as support
Methods based on water circulation tend to work well for hydrophilic compounds, but the bioavailability of hydrophobic compounds presents a challenge (Khalladi et al., 2009; Martins et al., 2009; Banat et al., 2021), especially since it is closely connected to the risk of contaminant mobilization. Biostimulation in itself does not appear to mobilize contaminants (Simpanen et al., 2016a,b). In a simulation of an oil transportation accident, biostimulation was compared to natural attenuation and chemical oxidation. During the monitoring period, biostimulation had an insignificant effect on the oil contents in the soil, but a pronounced effect on the oil flushed downwards with the water. The logic behind the observation is that microbes do not readily degrade oil that is bound to soil particles, but whatever is dissolved from the particles is bioavailable and degraded (Simpanen et al., 2016a). The bioavailability of hydrophobic compounds can be enhanced by the use of surfactants and biosurfactants that lower the effort required to overcome surface tension by situating themselves at the interface of solvents which differ in polarity (Del Valle, 2004; Khalladi et al., 2009; Talvenmäki et al., 2021; Eras-Muñoz et al., 2022). The use of surfactants accelerates the dissolution of PHCs such as diesel oil from the soil, and may result in the formation of biodegradable aqueous forms, desorption, and even emulsification of hydrocarbons (Calvo et al., 2009; Ayed et al., 2015; Bajagain et al., 2020b). Biosurfactants significantly vary in their structure and molecular weight. Their common characteristics is a high surface activity since they consist of hydrophobic (fatty alcohols, fatty acids, and unsaturated and saturated hydrocarbons) and hydrophilic (oligo-, mono- or polysaccharides, proteins, and peptides) moieties (Lin, 1996). Properly functioning biosurfactants are supposed to improve degradability by dissolving and emulsifying hydrophobic contaminants such as oil compounds or PAHs, while remaining inert long enough so as not to cause competition for resources. If, however, the surfactant itself is too easily degradable it may cause vigorous microbial activity that consumes the oxygen, nitrogen, etc., leaving the contaminant untouched (Talvenmäki et al., 2021). The rate of emulsification should also be matched by the rate of degradation, otherwise there is a risk of mobilizing the contaminant into the soil and groundwater (Simpanen et al., 2016b). A similar risk arises if a component in the contaminant spectrum is dissolved but non-degradable.
Many hydrocarbon degrading microbial species are able to produce biosurfactants that can be both intra- and extracellular. Among them, bacteria belonging to Pseudomonas, Acinetobacter, Bacillus, Rhodococcus, and Stenotrophomonas genera as well as fungi belonging to Candida, Torulopsis, and other yeast genera producing glycolipids (e.g., rhamnolipids, sophorolipids, and trehalose lipids), lipopeptides (e.g., iturin, surfactin, fengycin, lichenysin, and viscosin), and other minor biosurfactant classes, are the most studied (Karlapudi et al., 2018). For in situ bioremediation in cold climates, it is meaningful that many microbially produced surfactants have been shown to be stable at low temperatures and under extreme salinity and pH conditions (Baek et al., 2007; Sharma et al., 2022). Field studies of biosurfactant efficacy in the bioremediation of oil contaminated soils are limited, in contrast to lab studies. However, they demonstrate a significant increase in hydrocarbon decomposition rates under various environmental conditions (Tumeo et al., 1994; Nikolova and Gutierrez, 2021). High bioremediation rates were achieved when biosurfactants were used to enhance biostimulation or bioaugmentation (Baek et al., 2007; Cameotra and Singh, 2008; Kang et al., 2010; Tahseen et al., 2016). Bioaugmentation with the bacteria applied in a surfactant foam was shown to be efficient even at very low temperatures (Jeong et al., 2015).
Particularly interesting from an in situ point of view is the potential to harness biosurfactant producing bacterial strains for colonization of contaminated soils. Pseudomonas aeruginosa producing rhamnolipid was tested for crude oil degradation in combination with nutrition optimization, with encouraging results (Ma et al., 2016) and attempts have been made to implement this concept in in situ scenarios. Monitoring the efficacy is, however, challenging (Decesaro et al., 2017).
Besides crude oil and oily sludge, biosurfactants were demonstrated to enhance bioremediation of PAHs, pesticides and other hydrophobic organic pollutants. The underlying mechanism for organic compounds coincides with that for crude oil, in that an improvement of wettability and emulsification of the pollutant results in higher biodegradability (Raj et al., 2021).
Biostimulation vs. bioaugmentation
Biostimulation requires the presence of microbes possessing the proper degradative capabilities. If the contamination is old pollution with reduced bioavailability, it is highly likely that a degradative community is present, and the slow natural attenuation is due to suboptimal conditions slowing microbial activity (Suni et al., 2007; Kauppi et al., 2011; Dehnavi and Ebrahimipour, 2022). Bioaugmentation may be a viable alternative if the contaminant is highly recalcitrant and/or fresh. However, even freshly contaminated sites can be assumed to harbor microbes capable of degradation of principally biodegradable substances, such as mineral oil (Simpanen et al., 2016a; Banet et al., 2021) or PAHs from creosote (Koivula et al., 2004). Bioaugmentation was also found to significantly improve the remediation results compared to biostimulation alone for soil that lacked indigenous oil degraders (Chaudhary et al., 2021). Examples of the contrary are, however, also reported even in newly contaminated soil. Pilot scale tests in pristine, sandy soil with a humus soil top layer showered with a controlled amount of gasoline-diesel mix showed that biostimulation resulted in cleaning of leachate oil fractions, while natural attenuation did not (Simpanen et al., 2016a). The tested approach was adapted for a real truck accident site with good results (Tervo, 2013; Tervo and Kolehmainen, 2016).
Bioaugmentation is the addition of desired microbes to a system in order to accelerate remediation. This may be beneficial in situations where the indigenous microbial community is suspected to have decreased significantly. For example, in combination with chemical oxidation, such as using Fenton’s reagent for oily hotspots, an inoculum may speed up recovery of microbial degradation, since the high concentration of hydrogen peroxide more or less disinfects the soil locally, even beyond the zone of effective treatment (Talvenmäki et al., 2021). Thermal treatment of hotspots may have similar effects on adjacent areas where the thermal degradation as such does not reach, but the applied heat is still enough to kill soil microflora.
Bioaugmentation is not often needed (Suni et al., 2007; Kauppi et al., 2011) and may even worsen the situation as compared to the application of biostimulation alone. Bioaugmentation only works if conditions are suitable for the microbes to function, and so should always be complemented with biostimulation to guarantee appropriate conditions for microbial activity. In many cases bioaugmentation has no influence on microbial community composition. Even with sufficient biostimulation the added microbes may not perform well in situ, and soon disappear (Sarand et al., 2000; Kauppi et al., 2011). If degradation is limited by electron acceptor availability, adding another source of easily degradable biomass (the added bacterial cells) may slow the degradation of relatively recalcitrant compounds by consuming the available oxygen (Talvenmäki et al., 2021).
Checking the presence of key degradative genes has been used to predict the likelihood of successful remediation of soils contaminated with specific organic compounds (Baelum et al., 2010; Sinkkonen et al., 2014; Castro-Gutierrez et al., 2022; Wilms et al., 2023). A prerequisite to determine the suitability of this approach is that the degradation pathway is known, at least in part (Salminen et al., 2008). This was accomplished for atrazine (Sagarkar et al., 2013; Nousiainen et al., 2014) and chlorophenols in mesocosms (Sinkkonen et al., 2014) and in field applications (Mikkonen et al., 2018) where changes in gene frequencies using qPCR analysis correlated to degradation activity.
Naturally, the presence of microbes carrying relevant genes does not guarantee success, but the absence of such genes can be a strong indicator of poor chances even if biostimulation is applied. In such cases bioaugmentation may have an initial effect on degradation even if the introduced microbial strain or strains do not necessarily survive (Sarand et al., 2000). If the genes encoding for the introduced strain’s degradation capacity are located in a mobilizable position, such as in conjugative plasmids or transposons (Sipilä et al., 2010; Jeffries et al., 2018) this capacity can spread to the indigenous population as observed for the TOL-plasmid (Sarand et al., 2000) in the rhizospheres of pine.
Degradation pathways that have been introduced via a carrier strain may also evolve rapidly under selective pressure within the original carrier or after transfer to new recipients if their original genes are not exactly suited for the task. In this way degradation potential can rapidly diversify toward additional methylated phenols in humus soil (Sarand et al., 2001). This exploits the plasticity of the genes and gene clusters when functioning in natural environments (Ray et al., 2016).
When bioaugmentation is used, it may be of interest to monitor the survival and performance of the introduced microbes. Detecting the presence of the bioaugments can be done with certainty if a detectable marker gene or other unique genetic element is inserted in connection to the operon or gene cluster of interest (Sarand et al., 2000; Boureau et al., 2002), but this may require too much case-specific preparation to be widely applicable.
A simpler approach is to design primer pairs targeting genes of interest. Such targets can be genes known to be active in degradation of the contaminant of interest (Yan et al., 2018) but this will not distinguish between indigenous and introduced microbes. By combining PCR based identification of specific target genes and, e.g., 16S rRNA genes (Juhanson et al., 2009) introduced strains can be recognized in field experiments even years after application. Target gene presence can be quantified with qPCR, which, in combination with analysis of the diversity of 16S rRNA genes in the soil, may give a good estimate of the survival of the introduced strain(s) (Shahi et al., 2016; Li et al., 2021, 2023).
An interesting and novel method that, in addition to presence also monitors activity, is the use of metabolomics to get a snapshot of the in situ metabolic state of a dechlorination-active microbiome following bioaugmentation (May et al., 2022). This technology could be adapted for any genetically well-characterized situation, but performance monitoring requires a high level of technical capability.
An example of exploitation of native degradation capacity was seen when the degradation of pyrene was monitored in humus soil. Humus contains complex, heteropolymeric macromolecules, such as lignin and humic substances, which microbes are capable of degrading. Based on this observation, the conclusion was drawn that microbes normally exposed to such complex, natural substances containing a variety of hetero-aromatic compounds, could also adapt to rapidly degrade polyaromates such as pyrene (Koivula et al., 2004). The adaptability of humic microbial populations was also demonstrated regarding chloro-aromates (Kauppi et al., 2012; Sinkkonen et al., 2013). A different, more “organic” form of bioaugmentation is thus to rely on the microbial community of particular environments, that either contain natural substances structurally similar to the contaminant or that have been previously exposed to and cleaned from substances similar to the target compound, and to use transplantation from such environments into the contaminated site. In the case of PAHs (Koivula et al., 2004), herbicides such as triazine (Sinkkonen et al., 2013) and diesel or light fuel oil (Kauppi et al., 2012; Talvenmäki et al., 2021) transplantation of soil harboring microbes with degradative capacity promotes contaminant degradation when compared to pristine control soil of similar origin. With regards to bioaugmentation in situ, the challenge that remains in field conditions is the introduction of the microbial population into the contaminated site, but in some situations infiltration has proved to be a good strategy (Tyagi et al., 2011; Talvenmäki et al., 2021). Furthermore, infiltration can be increased by addition of surfactants on remedial agents (Gautam et al., 2019).
Phytoremediation, utilizing plants and their rhizosphere to introduce stimulatory compounds and elements (e.g., C) to contaminated soils, and can be considered an indirect form of biostimulation (Salam et al., 2022 and referenced therein, Yrjälä et al., 2010). However, this large topic is not within the scope of this review.
The challenge of non-aqueous phase liquids
Hydrocarbons that are poorly soluble in water may spread in soil and travel rapidly as a non-aqueous phase liquid (NAPL) down to the water table (Caetano et al., 2017). Light NAPL, such as diesel or light fuel oil, spreads on the surface of the groundwater at a rate that is influenced by groundwater stream velocity, soil density, and the viscosity of the NAPL at the current local temperature. However, the distribution of the contaminate can be determined by novel methods (Tsai et al., 2020), possibly aiding in targeted removal by pumping or excavation. As long as the contaminant remains a NAPL (i.e., not emulsified or dissolved by the addition of surfactants), degradation is extremely slow, since microbes have access to the compound only at the NAPL/water interface.
Dense NAPLs, such as creosote that contain large proportions of polycyclic aromatic hydrocarbons, but also many synthetic halogenated organic compounds (Tetrachloroethylene, etc.), do not stay on the surface of groundwater, but travel through the water ending up in bedrock fractures in relatively cold conditions (Yeo et al., 2003; Schaefer et al., 2009). Even if the solubility of the compound is low, the contaminant may spoil groundwater reservoirs and drinking water sources. Using bioremediation for such cases is questionable since removing biodegradation bottlenecks such as increasing the temperature, air sparging to induce aerobic conditions, or injection of surfactants is likely to mobilize the contaminant. Such mobilization, on the other hand, increases the NAPL surface area and the NAPL/water interface, thereby increasing the bioavailability of the contaminant, which may be a prerequisite for successful bioremediation. Thus, if all environmental and health RAs point to an acceptably low risk, in situ bioremediation may be an option (Baldwin et al., 2017; Schaefer et al., 2017). A viable alternative may be to introduce or attempt to favor microbes that produce biosurfactants in situ. Such bacterial preparations are commercially available and have been used with some success (Mariaamalraj et al., 2016).
Before reaching the water table (LNAPL) or impermeable soil layers (DNAPL) a large portion of the NAPL that travels through soil in the vadose zone is adsorbed to soil particles. Factors influencing the proportion of this adsorption include soil structure, soil moisture (Mercer and Cohen, 1990) and organic matter content, as well as properties of the liquid itself, such as viscosity and hydrophobicity. Biostimulation under such conditions requires mobilization of the contaminant to make it bioavailable (Simpanen et al., 2016a). Even when active mobilization is not attempted, biostimulation appears to be active only on the water accommodated fraction (WAF) portion of the contaminant, that is, only the solubilized contaminant portion is degraded (Mercer and Cohen, 1990; Simpanen et al., 2016a). In case the NAPL fraction does not reach the groundwater level, degradation of any WAF may, however, be sufficient to protect the groundwater from being contaminated. In such a situation biostimulation can be used to protect the groundwater (Simpanen, 2016).
Semi-active forms of in situ bioremediation
Different versions of pump-and-treat (Mikkonen et al., 2018; Zha et al., 2019), that act slowly but keep the contaminant plume from spreading, may be considered less risky than active biostimulation or bioaugmentation, and therefore easier to permit. Also chemical treatment such as the Fenton reaction or use of nano-iron (nZVI) has been used. The reaction is faster than with biostimulation, but the risks of mobilizing part of the contaminant may remain high.
Improved efficiency of in situ chemical oxidation, used as a pretreatment for bioremediation, was achieved by applying a persulfate-oxidation foam mixed with a surfactant to increase infiltration into the soil (Bajagain et al., 2018). Likewise, surfactant foam containing potassium permanganate has been shown to significantly improved the effectiveness of subsequent biodegradation of PHC (Bajagain et al., 2020b).
Construction of reactive barriers to limit the spread of contaminants in groundwater from an identified source is a relatively low risk action since nothing is necessarily done to the source itself (Liu et al., 2018; Naeimi et al., 2021). The remediating action, however, is slow and can be considered more of a prophylactic measure to protect downstream groundwater (Azubuike et al., 2016) than an active treatment of contaminants from an identified source.
Nanobioremediation
To tackle the limitations of bioremediation, such as long duration and formation of toxic degradation products, the combinations of nanotechnology and bioremediation (nanobioremediation) has gained significant attention (Grieger et al., 2015; Shahid et al., 2022). Nanotechnology is based on nano-sciences, i.e., the study of structures and materials on a nanoscale (with a dimensions of <100 nm, nanomaterials (NMs)) and the manipulation and application of these materials. In remediation, NMs have certain advantages that larger particles lack. For example, iron particles in nanosize are 10–100 times more reactive than macro-iron particles thanks to the larger surface-area-to-volume ratio (Zhang et al., 1998). Reactivity of the NMs is based on (a) reductive reactions in which NMs work as an electron donor to transform or convert the contaminant to a less toxic and/or less mobile form, and (b) stabilization and sorption in which the NMs work as a sorbent, precipitant, and/or co-precipitant of the contaminant (Cundy et al., 2008).
NMs used in nanobioremediation can be divided according to their production material into inorganic, such as metals, bimetals, metal oxides, ceramics and synthetic polymers, and organic, such as carbon nanotubes, fullerenes, cellulose nanoparticles, and natural polymers (Rizwan et al., 2014). Successful implementation of nanobioremediation is based on the sequenced use of NMs along with biotechnologies. In such cases, NMs are first applied to reduce high concentrations of contaminants to levels favorable for microbial remediation or as an additional source of growth-limiting substrates (Bhatt et al., 2022). As a result NMs may cause the soil microbial community composition to shift in favor of more resistant and faster-degrading microbial groups (Bhatt et al., 2022). For example, zero-valent iron nanoparticles are known to provide an additional source of electron donors, H2, to bacteria capable of dehalogenation of chlorinated organics (Bruton et al., 2015) and carbon nanotubes have been found to enhance the growth of some potential contaminant degrading genera (Shrestha et al., 2013). Additionally, microbes and enzymes can be immobilized with NMs to enhance the microbial activity and longevity, to ease the reuse and recovery of microbial cells and allow the protection of cells from adverse environmental conditions (Kumari and Singh, 2016).
Nanobioremediation has been tested successfully for many types of contaminants, such as heavy metals (Kumar et al., 2023), hydrocarbons (Galdames et al., 2020; Chauhan et al., 2023), nutrients (Gibert et al., 2022), chlorinated ethenes (Sheu et al., 2016; Summer et al., 2020), and pesticides (Singh and Saxena, 2022; Sunanda and Ghosh Sachan, 2023). However, some concerns have been raised regarding the safety of NMs, since they may have negative impacts on different organisms, such as plants and microbes once released into the environment (Khati et al., 2018; Bhatt et al., 2022). Especially higher concentrations of NMs have shown some toxicity effects (Brayner et al., 2006). Therefore more research is needed regarding suitable concentrations of NMs and their possible risks should be evaluated before larger scale experiments are conducted. Nevertheless, especially in colder climates where microbial degradation is slower (Mohn and Stewart, 2000; Bajaj and Singh, 2015; Miri et al., 2019), use of NMs to speed up and enhance microbial degradation can be beneficial.
In situ methods used in sequence or in combination
As previously noted, raising the rate of soil and groundwater cleaning by biostimulation in practice means removing biodegradation bottlenecks, or to at least substantially lower the threshold for active biodegradation. All bottlenecks hindering relevant microbial activity need to be removed – adding missing nutrients is not sufficient if oxygen is also lacking or vice versa. This means that a comprehensive analysis of the local situation needs to be undertaken to determine the best strategy in each case. This may appear self-evident, but surveys performed at contaminated sites do not always take into account all of the relevant parameters.
Natural attenuation, biostimulation, bioaugmentation, chemical oxidation or reduction, physical treatments, or a combination of different methods are all potentially viable solutions for the in situ remediation of contaminated sites. However, when bioremediation through biostimulation is the chosen course, other methods are almost always included. Thus, the last alternative – a combination of methods – is the most common approach in bioremediation.
Excavation and off-site treatment is often the chosen method for remediation, and may be unavoidable in situations where new building activities will commence within weeks or months of the decision. At the other extreme, natural attenuation may be a valid choice if a site is considered stable, in that the contaminant is not likely to spread to surface or ground waters, or to be exposed at the ground surface. Choosing natural attenuation may make sense when optimizing input-output is a primary concern. Ranking the sites should at that point be based on RA, where environmental (environmental RA) and human health (health RA) issues are prioritized rather than the economic value of a site (discussed further in the next section). Priority for active intervention/remediation should be given to sites where the contaminant situation is not stable, putting adjacent soils and/or groundwater at risk. Monitored natural attenuation, on the other hand, can be a valid choice where the situation is stable and where contaminant levels are reduced at a sufficient rate. With this type of ranking a maximum number of high risk sites can be treated while risk-free sites also progress toward complete removal of contamination.
In most cases in situ bioremediation is not as fast as, e.g., excavation and relocation or chemical oxidation with peroxide (Fenton reaction). However, in contrast to excavation, in situ treatments can take place beneath buildings and roads without the need for demolition. Also, even when such obstacles do not preclude excavation but there is no particular rush, in situ treatment can be considered as a both cost effective and more sustainable option. Removal and transportation of large quantities of soil is not needed, and neither is replacing the excavated soil with pristine soil from elsewhere, significantly reducing the overall environmental impact of the remediation project.
It can be stated that the best results can be achieved by combining methods based on case-by-case evaluation in a goal-oriented manner. The risk is that consultants and contractors favor methods that they represent and/or have expertise in, rather than withdrawing from risky cases. Ideally, a consultant or site evaluator with a sufficiently broad palette of expertise can find combinations of methods and, when needed, combinations of contractors that can tackle the project with a concerted effort. Alternatively, the contractor should represent or have expertise in a sufficient array of techniques so as to be capable of implementing optimal combinations by itself. These consultants and contractors should also be required to acknowledge when the methods that they represent are not likely to work. This means that excavation in some cases may be the most environmentally sustainable solution, but it should never be the only option considered.
Tools to support action: RA, EE, LCA, and AI in the decision making process
Deciding on a particular course of action for a remediation project should be informed by many different factors to help ensure that the strategy with the lowest overall negative impact and maximum effectiveness is chosen. This is often a complex and difficult task, requiring extensive expertise and resources. According to Sorvari and Seppälä (2010), decision support tools (DSTs) can be useful, especially when used with a multi-criteria decision analysis approach, though most existing tools focus on, e.g., site characterization and/or sampling strategy rather than on the evaluation of remediation technologies. DSTs that make use of RA, eco-efficiency (EE), life cycle assessments and, possibly, artificial intelligence (AI) can be used to simplify and improve the process. Here we discuss aspects of DSTs and some software-based tools that are currently available.
Risk assessment examines the human health and environmental impacts of a project and is a widely used method to determine the need for remediation. Mahammedi et al. (2020) examined RA tools used in different countries and that are tailored for specific types of risk and situations. However, the authors found that many of these were lacking in key respects, such as user-friendliness and ability to accurately assess some risks. Additionally, RAs have significant weaknesses that may limit their usefulness in bioremediation projects. For example, RAs rarely consider which remediation methods are to be used or their overall environmental and social impacts (Sorvari and Seppälä, 2010).
Eco-efficiency, based on the ratio of ecological to economic factors involved in a project, is widely used in contaminated land management. While useful in many contexts, Sorvari and Seppälä (2010) found that a lack of established assessment methods and guidelines may be major barriers to the use of EE locally.
Life Cycle Assessments (LCAs) are typically used to evaluate the environmental impacts of various products, processes, and systems throughout their entire life cycle. This process can also be useful in the selection of strategies for bioremediation. By encompassing all aspects of a project, LCA quantifies its environmental impacts from “cradle to grave,” evaluating energy consumption, emissions, resource use, and potential ecological impacts associated with the bioremediation processes being considered. This makes LCA a potentially powerful technique for identifying optimal remediation strategies while helping to minimizing the project’s overall environmental footprint. Although a properly performed LCA is important, alternatives at a specific location should always be assessed based on a number of factors, including those that are not necessarily dealt with in the LCA process. It is especially important to include: direct threats to human health, environmental safety, stability vs. volatility of the contaminant situation, and urgency of continued and/or future use of the site (Romantschuk et al., 2000; Betrie et al., 2013).
Many software-based DSTs exist that can be useful for the evaluation of bioremediation projects, such as Decerns MCDA,1 openLCA,2 SimaPro,3 Sphera (formerly known as GaBi),4 and Umberto.5 Additional tools are in use locally in EU member states, the USA, and elsewhere. One example is the PIRTU EE calculation tool (SYKE, 2013) developed in Finland for assessment of contaminated soil and groundwater sites based on the calculation of risks, environmental impact, costs, and other impacts. However, proper use of the tool requires expertise for evaluation of the numerical results produced. Sorvari and Seppälä (2010) also developed a DST based on multi-attribute value theory for Finnish contaminated sites. Another tool is the SCORE developed in Sweden for assessing sustainability of remediation of contaminated soil (Rosén et al., 2015). In the USA, BIOPLUME III6 is a sophisticated two-dimensional contaminant transport model used to predict natural attenuation of organic contaminants in groundwater under various conditions, which may be modified to include some types of bioremediation (Rifai et al., 2000). Private consultancy companies have also developed their own tools for assessing sustainable remediation, one example of which is SURE by Ramboll (2023).
Recent developments in AI programs such as natural language generation or large language models may also aid in the evaluation and selection of bioremediation strategies for particular sites. Already these programs, such as the web-based ChatGPT,7 are attracting attention in a wide range of fields, such as project management, programming and data analysis (Prieto et al., 2023; Surameery and Shakor, 2023; Zhu et al., 2023). While far from perfect (Lo, 2023; Zhu et al., 2023), given accurate information about site characteristics (i.e., setting, geology, hydrology, in situ microbial community, etc.) and the contaminant of interest, it is possible for a web-based program such as ChatGPT to give apparently useful recommendations for effective remediation strategies based on the literature in its training dataset. It is possible to image that such a model, trained on datasets particular to environmental remediation, could produce effective remediation plans that improve outcomes.
Furthermore, in order to prevent repeating the mistakes of others, a database for—grossly under-reported—unsuccessful in situ bioremediation cases should be established. Such matters have been discussed, e.g., in a report by the National Research Council (U.S.) (1993), and consultancy companies may keep up their own registers, but no widely accessible, updatable database is, to our knowledge, in use. A question that arises is: who should maintain and validate the contents of such a database? The responsible party would need to ensure the integrity of the contents and prevent its miss-use, for example, by using it to generate false negative publicity for a particular method. Ideally, such a database should be maintained by national or international public authorities or by an NGO.
Use of these existing and proposed tools can ease the implementation and efficacy of bioremediation, drawing attention to its possibilities, and reducing the temptation to resort to the most common remediation technique: dig and dump. Actions taken to remediate polluted environments created by human activities should be optimized to achieve the maximum effect and improvement from the often limited available resources. Reaching this goal requires thorough planning and pre-testing, based on solid expertise. A proposed order of actions is presented in Figure 2.
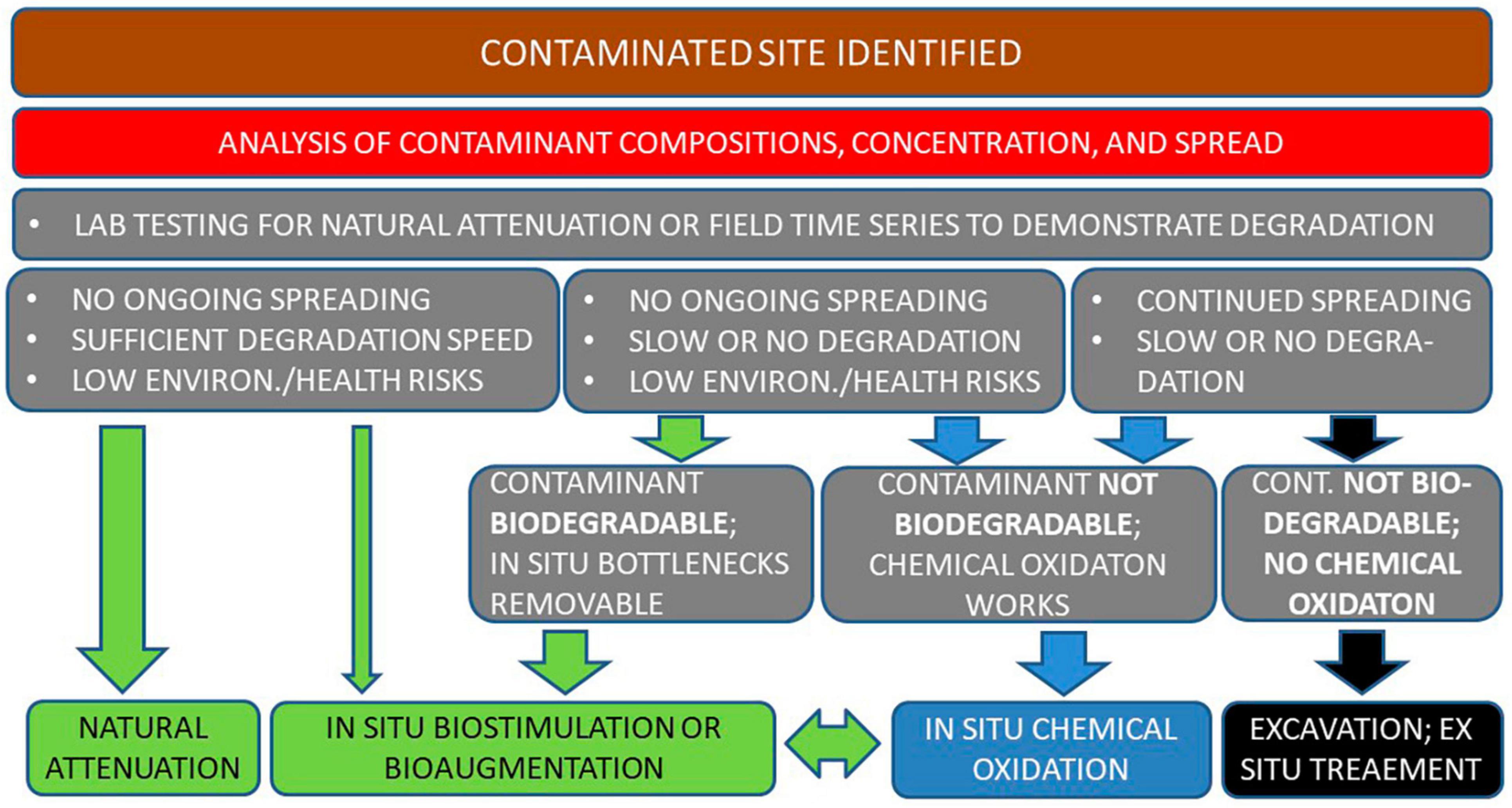
Figure 2. Stepwise progression toward decision for action at a contaminated site. Following site identification (brown box) the contaminant composition, site characteristics and area contaminated are determined (red). A sequence of lab tests (gray) commence to determine degradability, stability, and risk aspects. Depending on lab results field activities are chosen: biological [natural attenuation, biostimulation, and bioaugmentation (green)], chemical oxidation (blue), or excavation/ex situ treatment (black) as a last resort. Biological and chemical remediation may in some situations be used in combination or in sequence (vertical double arrow).
Author contributions
MR: Conceptualization, Writing—original draft, Writing—review and editing. KL-L: Writing—original draft, Writing—review and editing. MK: Writing—review and editing. PG: Conceptualization, Writing—original draft, Writing—review and editing. HT: Writing—original draft, Writing—review and editing. SS: Writing—original draft, Writing—review and editing. JA: Writing—review and editing. AS: Writing—review and editing.
Funding
The author(s) declare that no financial support was received for the research, authorship, and/or publication of this article.
Conflict of interest
The authors declare that the research was conducted in the absence of any commercial or financial relationships that could be construed as a potential conflict of interest.
Publisher’s note
All claims expressed in this article are solely those of the authors and do not necessarily represent those of their affiliated organizations, or those of the publisher, the editors and the reviewers. Any product that may be evaluated in this article, or claim that may be made by its manufacturer, is not guaranteed or endorsed by the publisher.
Footnotes
- ^ http://decerns.com
- ^ https://openlca.org
- ^ https://simapro.com
- ^ https://sphera.com
- ^ https://ifu.com/umberto/
- ^ https://epa.gov/water-research/bioplume-iii
- ^ https://chat.openai.com
References
Ayed, H. B., Jemil, N., Maalej, H., Bayoudh, A., Hmidet, N., and Moncef, N. (2015). Enhancement of solubilization and biodegradation of diesel oil by biosurfactant from Bacillus amyloliquefaciens An6. Int. Biodeterior. Biodegrad. 99, 8–14.
Azubuike, C. C., Chikere, C. B., and Okpokwasili, G. C. (2016). Bioremediation techniques–classification based on site of application: Principles, advantages, limitations and prospects. World J. Microbiol. Biotechnol. 32:180. doi: 10.1007/s11274-016-2137-x
Baek, K.-H., Yoon, B.-D., Kim, B.-H., Cho, D.-H., Lee, I.-S., Oh, H.-M., et al. (2007). Monitoring of microbial diversity and activity during bioremediation of crude oil-contaminated soil with different treatments. J. Microbiol. Biotechnol. 17, 67–73.
Baelum, J., Jacobsen, C. S., and Holben, W. E. (2010). Comparison of 16S rRNA gene phylogeny and functional tfdA gene distribution in thirty-one different 2,4-dichlorophenoxyacetic acid and 4-chloro-2-methylphenoxyacetic acid degraders. Syst. Appl. Microbiol. 33, 67–70. doi: 10.1016/j.syapm.2010.01.001
Bajagain, R., Gautam, P., and Jeong, S.-W. (2020a). Biodegradation and post-oxidation of fuel-weathered field soil. Sci. Total Environ. 734:139452. doi: 10.1016/j.scitotenv.2020.139452
Bajagain, R., Gautam, P., and Jeong, S.-W. (2020b). Degradation of petroleum hydrocarbons in unsaturated soil and effects on subsequent biodegradation by potassium permanganate. Environ. Geochem. Health 42, 1705–1714. doi: 10.1007/s10653-019-00346-y
Bajagain, R., Lee, S., and Jeong, S.-W. (2018). Application of persulfate-oxidation foam spraying as a bioremediation pretreatment for diesel oil-contaminated soil. Chemosphere 207, 565–572. doi: 10.1016/j.chemosphere.2018.05.081
Bajaj, S., and Singh, D. K. (2015). Biodegradation of persistent organic pollutants in soil, water and pristine sites by cold-adapted microorganisms: Mini review. Int. Biodeterior. Biodegrad. 100, 98–105. doi: 10.1016/j.ibiod.2015.02.023
Bala, S., Garg, D., Thirumalesh, B. V., Sharma, M., Sridhar, K., Inbaraj, B. S., et al. (2022). Recent strategies for bioremediation of emerging pollutants: A review for a green and sustainable environment. Toxics 10:484. doi: 10.3390/toxics10080484
Baldwin, B. R., Taggart, D., Chai, Y., Wandor, D., Biernacki, A. L., Sublette, K., et al. (2017). Bioremediation management reduces mass discharge at a chlorinated DNAPL Site. Groundwater Monitor. Remed. 37, 58–70. doi: 10.1111/gwmr.12211
Banat, I. M., Carboué, Q., Saucedo-Castañeda, G., and de Jesús Cázares-Marinero, J. (2021). Biosurfactants: The green generation of speciality chemicals and potential production using Solid-State fermentation (SSF) technology. Bioresour. Technol. 320:124222. doi: 10.1016/j.biortech.2020.124222
Banet, G., Turaani, A. K., Farber, R., Armoza- Zvuloni, R., Rotem, N., Stavi, I., et al. (2021). The effects of biostimulation and bioaugmentation on crude oil biodegradation in two adjacent terrestrial oil spills of different age, in a hyper-arid region. J. Environ. Manage. 286:112248. doi: 10.1016/j.jenvman.2021.112248
Betrie, G. D., Sadiq, R., Morin, K. A., and Tesfamariam, S. (2013). Selection of remedial alternatives for mine sites: A multicriteria decision analysis approach. J. Environ. Manage. 119, 36–46. doi: 10.1016/j.jenvman.2013.01.024
Bhatt, P., Pandey, S. C., Joshi, S., Chaudhary, P., Pathak, V. M., Huang, Y., et al. (2022). Nanobioremediation: A sustainable approach for the removal of toxic pollutants from the environment. J. Hazard. Mater. 427:128033. doi: 10.1016/j.jhazmat.2021.128033
Blázquez-Pallí, N., Rosell, M., Varias, J., Bosch, M., Soler, A., Vicent, T., et al. (2019). Multi-method assessment of the intrinsic biodegradation potential of an aquifer contaminated with chlorinated ethenes at an industrial area in Barcelona (Spain). Environ. Pollut. 244, 165–173. doi: 10.1016/j.envpol.2018.10.013
Borden, R. C., Beckwith, W. J., Lieberman, M. T., Akladiss, N., and Hill, S. R. (2007). Enhanced anaerobic bioremediation of a TCE source at the Tarheel Army Missile Plant using EOS. Remediation Journal 17, 5–19. doi: 10.1002/rem.20130
Boureau, T., Routtu, J., Roine, E., Taira, S., and Romantschuk, M. (2002). Localization of hrpA-induced Pseudomonas syringae pv. tomato DC3000 in infected tomato leaves. Mol. Plant Pathol. 3, 451–460. doi: 10.1046/j.1364-3703.2002.00139.x
Brayner, R., Ferrari-Iliou, R., Brivois, N., Djediat, S., Benedetti, M. F., and Fiévet, F. (2006). Toxicological Impact Studies Based on Escherichia coli Bacteria in Ultrafine ZnO nanoparticles colloidal medium. Nano Lett. 6, 866–870. doi: 10.1021/nl052326h
Bruton, T. A., Pycke, B. F. G., and Halden, R. U. (2015). Effect of nanoscale zero-valent iron treatment on biological reductive dechlorination: A review of current understanding and research needs. Crit. Rev. Environ. Sci. Technol. 45, 1148–1175. doi: 10.1080/10643389.2014.924185
Caetano, M. O., Schneider, I. A. H., Gomes, L. P., Kieling, A. G., and Miranda, L. A. S. (2017). A compact remediation system for the treatment of groundwater contaminated with BTEX and TPH. Environ. Technol. 38, 1408–1420. doi: 10.1080/09593330.2016.1231222
Calvo, C., Manzanera, M., Silva-Castro, G. A., Uad, I., and Gonzalez-Lopez, J. (2009). Application of bioemulsifiers in soil oil bioremediation process. Future prospects. Sci. Total Environ. 407, 3634–3640.
Cameotra, S. S., and Singh, P. (2008). Bioremediation of oil sludge using crude biosurfactants. Int. Biodeterior. Biodegrad. 62, 274–280. doi: 10.1016/j.ibiod.2007.11.009
Castro-Gutierrez, V. M., Hassard, F., and Moir, J. W. B. (2022). Probe-based qPCR assay enables the rapid and specific detection of bacterial degrading genes for the pesticide metaldehyde in soil. J. Microbiol. Methods 195:106447. doi: 10.1016/j.mimet.2022.106447
Cavazzoli, S., Selonen, V., Rantalainen, A.-L., Sinkkonen, A., Romantschuk, M., and Squartini, A. (2022). Natural additives contribute to hydrocarbon and heavy metal co-contaminated soil remediation. Environ. Pollut. 307:119569. doi: 10.1016/j.envpol.2022.119569
Cavazzoli, S., Squartini, A., Sinkkonen, A., Romantschuk, M., Rantalainen, A.-L., Selonen, V., et al. (2023). Nutritional additives dominance in driving the bacterial communities succession and bioremediation of hydrocarbon and heavy metal contaminated soil microcosms. Microbiol. Res. 270:127343. doi: 10.1016/j.micres.2023.127343
Chaîneau, C. H., Rougeux, G., Yéprémian, C., and Oudot, J. (2005). Effects of nutrient concentration on the biodegradation of crude oil and associated microbial populations in the soil. Soil Biol. Biochem. 37, 1490–1497. doi: 10.1016/j.soilbio.2005.01.012
Chapin, F. S., Matson, P. A., and Vitousek, P. M. (2011). Principles of Terrestrial Ecosystem Ecology. New York, NY: Springer, doi: 10.1007/978-1-4419-9504-9
Chaudhary, D. K., Bajagain, R., Jeong, S.-W., and Kim, J. (2021). Effect of consortium bioaugmentation and biostimulation on remediation efficiency and bacterial diversity of diesel-contaminated aged soil. World J. Microbiol. Biotechnol. 37, 1–12. doi: 10.1007/s11274-021-02999-3
Chauhan, P., Imam, A., Kanaujia, P. K., and Suman, S. K. (2023). Nano-bioremediation: an eco-friendly and effective step towards petroleum hydrocarbon removal from environment. Environ. Res. 231:116224. doi: 10.1016/j.envres.2023.116224
Chen, X., Zhang, S., Yi, L., Liu, Z., Ye, X., Yu, B., et al. (2022). Evaluation of Biodegradation of BTEX in the Subsurface of a Petrochemical Site near the Yangtze River, China. Int. J. Environ. Res. Public Health 19:16449. doi: 10.3390/ijerph192416449
Cheng, D., and He, J. (2009). Isolation and Characterization of “Dehalococcoides” sp. Strain MB, Which Dechlorinates Tetrachloroethene to trans-1,2-Dichloroethene. Appl. Environ. Microbiol. 75, 5910–5918. doi: 10.1128/AEM.00767-09
Conrad, M. E., Brodie, E. L., Radtke, C. W., Bill, M., Delwiche, M. E., Lee, M. H., et al. (2010). Field evidence for co-metabolism of trichloroethene stimulated by addition of electron donor to groundwater. Environ. Sci. Technol. 44, 4697–4704. doi: 10.1021/es903535j
Cundy, A. B., Hopkinson, L., and Whitby, R. L. D. (2008). Use of iron-based technologies in contaminated land and groundwater remediation: A review. Sci. Total Environ. 400, 42–51. doi: 10.1016/j.scitotenv.2008.07.002
Dahl, M., Lepikkö, K., Malk, V., and Simpanen, S. (eds) (2013). Kemikaalionnettomuuksien riskinhallinta ja pilaantuneiden alueiden kunnostus [RIMA-Risk Management and remediation of Chemical accidents report]. Kotka: Helsingin yliopisto, koulutus- ja kehittämiskeskus Palmenia.
Decesaro, A., Machado, T. S., Cappellaro, ÂC., Reinehr, C. O., Thomé, A., and Colla, L. M. (2017). Biosurfactants during in situ bioremediation: factors that influence the production and challenges in evalution. Environ. Sci. Pollut. Res. Int. 24, 20831–20843. doi: 10.1007/s11356-017-9778-7
Dehnavi, S. M., and Ebrahimipour, G. (2022). Comparative remediation rate of biostimulation, bioaugmentation, and phytoremediation in hydrocarbon contaminants. Int. J. Environ. Sci. Technol. 19, 11561–11586. doi: 10.1007/s13762-022-04343-0
Del Valle, E. M. M. (2004). Cyclodextrins and their uses: a review. Process Biochem. 39, 1033–1046. doi: 10.1016/S0032-9592(03)00258-9
Dolfing, J. (2000). Energetics of Anaerobic Degradation Pathways of Chlorinated Aliphatic Compounds. Microb. Ecol. 40, 2–7. doi: 10.1007/s002480000039
Dutta, N., Usman, M., Ashraf, M. A., Luo, G., and Zhang, S. (2022). A critical review of recent advances in the bio-remediation of chlorinated substances by microbial dechlorinators. Chem. Eng. J. Adv. 12:100359. doi: 10.1016/j.ceja.2022.100359
Eras-Muñoz, E., Farré, A., Sánchez, A., Font, X., and Gea, T. (2022). Microbial biosurfactants: a review of recent environmental applications. Bioengineered 13, 12365–12391. doi: 10.1080/21655979.2022.2074621
EUR-Lex (2000). Directive 2000/60/EC of the European Parliament and of the Council of 23 October 2000 establishing a framework for Community action in the field of water policy. Available online at: http://data.europa.eu/eli/dir/2000/60/oj/eng (accessed August 30, 2023).
EUR-Lex (2008). Directive 2008/50/EC of the European Parliament and of the Council of 21 May 2008 on ambient air quality and cleaner air for Europe. Available online at: http://data.europa.eu/eli/dir/2008/50/oj/eng (accessed August 30, 2023).
European Commission (2017). European achievements in soil remediation and brownfield redevelopment: a report of the European Information and Observation Network’s National Reference Centres for Soil (Eionet NRC Soil). Brussels: , Joint Research Centre.
Fernandez-Lopez, C., Posada-Baquero, R., and Ortega-Calvo, J.-J. (2022). Nature-based approaches to reducing the environmental risk of organic contaminants resulting from military activities. Sci. Total Environ. 843:157007. doi: 10.1016/j.scitotenv.2022.157007
FINLEX (2007). Government decree on the assessment of soil contamination and remediation needs. Available online at: https://www.finlex.fi/en/laki/kaannokset/2007/en20070214?search%5Btype%5D=pika&search%5Bkieli%5D%5B0%5D=en&search%5Bpika%5D=214%2F2007 (accessed October 2, 2023).
Galdames, A., Ruiz-Rubio, L., Orueta, M., Sánchez-Arzalluz, M., and Vilas-Vilela, J. L. (2020). Zero-valent iron nanoparticles for soil and groundwater remediation. Int. J. Environ. Res. Public Health 17, 5817. doi: 10.3390/ijerph17165817
Gao, D., Zhao, H., Wang, L., Li, Y., Tang, T., Bai, Y., et al. (2022). Current and emerging trends in bioaugmentation of organic contaminated soils: A review. J. Environ. Manage. 320:115799. doi: 10.1016/j.jenvman.2022.115799
Gautam, P., Bajagain, R., and Jeong, S.-W. (2019). Soil infiltration capacity of chemical oxidants used for risk reduction of soil contamination. Ecotoxicol. Environ. Safety 183:109548.
Gerecke, A. C., Hartmann, P. C., Heeb, N. V., Kohler, H.-P. E., Giger, W., Schmid, P., et al. (2005). Anaerobic degradation of decabromodiphenyl ether. Environ. Sci. Technol. 39, 1078–1083. doi: 10.1021/es048634j
Gibert, O., Sánchez, D., and Cortina, J. L. (2022). Removal of nitrate and pesticides from groundwater by nano zero-valent iron injection pulses under biostimulation and bioaugmentation scenarios in continuous-flow packed soil columns. J. Environ. Manage. 321:115965. doi: 10.1016/j.jenvman.2022.115965
Goi, A., Trapido, M., Kulik, N., Palmroth, M. R. T., and Tuhkanen, T. (2006). Ozonation and fenton treatment for remediation of diesel fuel contaminated soil. Ozone 28, 37–46. doi: 10.1080/01919510500479130
Grieger, K. D., Hjorth, R., Rice, J., Kumar, N., and Bang, J. (2015). Nano-remediation: tiny particles cleaning up big environmental problems. Geneva: IUCN.
He, H., Li, Y., Shen, R., Shim, H., Zeng, Y., Zhao, S., et al. (2021). Environmental occurrence and remediation of emerging organohalides: A review. Environ. Pollut. 290:118060. doi: 10.1016/j.envpol.2021.118060
He, J., Ritalahti, K. M., Aiello, M. R., and Löffler, F. E. (2003). Complete detoxification of vinyl chloride by an anaerobic enrichment culture and identification of the reductively dechlorinating population as a dehalococcoides species. Appl. Environ. Microbiol. 69, 996–1003. doi: 10.1128/AEM.69.2.996-1003.2003
Hölscher, T., Krajmalnik-Brown, R., Ritalahti, K. M., von Wintzingerode, F., Görisch, H., Löffler, F. E., et al. (2004). Multiple nonidentical reductive-dehalogenase-homologous genes are common in dehalococcoides. Appl. Environ. Microbiol. 70, 5290–5297. doi: 10.1128/AEM.70.9.5290-5297.2004
Hood, E. D., Major, D. W., Quinn, J. W., Yoon, W.-S., Gavaskar, A., and Edwards, E. A. (2008). Demonstration of Enhanced Bioremediation in a TCE Source Area at Launch Complex 34, Cape Canaveral Air Force Station. Groundwater Monitor. Remed. 28, 98–107. doi: 10.1111/j.1745-6592.2008.00197.x
Huang, D., Xu, Q., Cheng, J., Lu, X., and Zhang, H. (2012). Electrokinetic remediation and its combined technologies for removal of organic pollutants from contaminated soils. Int. J. Electrochem. Sci. 7, 4528–4544. doi: 10.1016/S1452-3981(23)19558-7
Huang, S., and Jaffé, P. R. (2019). Defluorination of perfluorooctanoic acid (PFOA) and perfluorooctane sulfonate (PFOS) by Acidimicrobium sp, Strain A6. Environ. Sci. Technol. 53, 11410–11419. doi: 10.1021/acs.est.9b04047
Jeffries, T. C., Rayu, S., Nielsen, U. N., Lai, K., Ijaz, A., Nazaries, L., et al. (2018). Metagenomic functional potential predicts degradation rates of a model organophosphorus xenobiotic in pesticide contaminated soils. Front. Microbiol. 9:147. doi: 10.3389/fmicb.2018.00147
Jeong, S.-W., Jeong, J., and Kim, J. (2015). Simple surface foam application enhances bioremediation of oil-contaminated soil in cold conditions. J. Hazard. Mater. 286, 164–170. doi: 10.1016/j.jhazmat.2014.12.058
Johnson, D. R., Lee, P. K. H., Holmes, V. F., and Alvarez-Cohen, L. (2005). An internal reference technique for accurately quantifying specific mRNAs by Real-Time PCR with application to the tcea reductive dehalogenase Gene. Appl. Environ. Microbiol. 71, 3866–3871. doi: 10.1128/AEM.71.7.3866-3871.2005
Jones, C. A., Koenig, R. T., Ellsworth, J. W., Brown, B. D., and Jackson, G. D. (2007). Management of Urea Fertilizer to Minimize Volatilization. Bozeman, MT: Montana State University Cooperative Extension Service Publication.
Jones, K. C., and de Voogt, P. (1999). Persistent organic pollutants (POPs): state of the science. Environ. Pollut. 100, 209–221. doi: 10.1016/S0269-7491(99)00098-6
Juhanson, J., Truu, J., Heinaru, E., and Heinaru, A. (2009). Survival and catabolic performance of introduced Pseudomonas strains during phytoremediation and bioaugmentation field experiment. FEMS Microbiol. Ecol. 70, 446–455. doi: 10.1111/j.1574-6941.2009.00754.x
Kang, S.-W., Kim, Y.-B., Shin, J.-D., and Kim, E.-K. (2010). Enhanced Biodegradation of Hydrocarbons in Soil by Microbial Biosurfactant, Sophorolipid. Appl. Biochem. Biotechnol. 160, 780–790. doi: 10.1007/s12010-009-8580-5
Karlapudi, A. P., Venkateswarulu, T. C., Tammineedi, J., Kanumuri, L., Ravuru, B. K., Dirisala, V. R., et al. (2018). Role of biosurfactants in bioremediation of oil pollution-a review. Petroleum 4, 241–249. doi: 10.1016/j.petlm.2018.03.007
Kauppi, S., Romantschuk, M., Strömmer, R., and Sinkkonen, A. (2012). Natural attenuation is enhanced in previously contaminated and coniferous forest soils. Environ. Sci. Pollut. Res. 19, 53–63. doi: 10.1007/s11356-011-0528-y
Kauppi, S., Sinkkonen, A., and Romantschuk, M. (2011). Enhancing bioremediation of diesel-fuel-contaminated soil in a boreal climate: Comparison of biostimulation and bioaugmentation. Int. Biodeterior. Biodegrad. 65, 359–368. doi: 10.1016/j.ibiod.2010.10.011
Khalladi, R., Benhabiles, O., Bentahar, F., and Moulai-Mostefa, N. (2009). Surfactant remediation of diesel fuel polluted soil. J. Hazard. Mater. 164, 1179–1184. doi: 10.1016/j.jhazmat.2008.09.024
Khati, P., Parul Bhatt, P., Nisha Kumar, R., and Sharma, A. (2018). Effect of nanozeolite and plant growth promoting rhizobacteria on maize. 3 Biotech 8:141. doi: 10.1007/s13205-018-1142-1
Kim, D.-J., Park, M.-R., Lim, D.-S., and Choi, J.-W. (2013). Impact of Nitrate Dose on Toluene Degradation under Denitrifying Condition. Appl. Biochem. Biotechnol. 170, 248–256. doi: 10.1007/s12010-013-0176-4
Kocur, C. M. D., Lomheim, L., Molenda, O., Weber, K. P., Austrins, L. M., Sleep, B. E., et al. (2016). Long-Term Field Study of Microbial Community and Dechlorinating Activity Following Carboxymethyl Cellulose-Stabilized Nanoscale Zero-Valent Iron Injection. Environ. Sci. Technol. 50, 7658–7670. doi: 10.1021/acs.est.6b01745
Koivula, T. T., Salkinoja-Salonen, M., Peltola, R., and Romantschuk, M. (2004). Pyrene Degradation in Forest Humus Microcosms with or without Pine and its Mycorrhizal Fungus. J. Environ. Qual. 33, 45–53. doi: 10.2134/jeq2004.4500
Koivunen, M. E., Morisseau, C., Horwath, W. R., and Hammock, B. D. (2004). Isolation of a strain of Agrobacterium tumefaciens (Rhizobium radiobacter) utilizing methylene urea (ureaformaldehyde) as nitrogen source. Can. J. Microbiol. 50, 167–174. doi: 10.1139/w04-001
Kraemer, S. A., Ramachandran, A., and Perron, G. G. (2019). Antibiotic pollution in the environment: from microbial ecology to public policy. Microorganisms 7:180. doi: 10.3390/microorganisms7060180
Krajmalnik-Brown, R., Hölscher, T., Thomson, I. N., Saunders, F. M., Ritalahti, K. M., and Löffler, F. E. (2004). Genetic identification of a putative vinyl chloride reductase in dehalococcoides sp. Strain BAV1. Appl. Environ. Microbiol. 70, 6347–6351. doi: 10.1128/AEM.70.10.6347-6351.2004
Krzmarzick, M. J., Crary, B. B., Harding, J. J., Oyerinde, O. O., Leri, A. C., Myneni, S. C. B., et al. (2012). Natural niche for organohalide-respiring chloroflexi. Appl. Environ. Microbiol. 78, 393–401. doi: 10.1128/AEM.06510-11
Kumar, A., Krishan, B., Shivani Dhiman, S., and Sharma, A. (2023). “Nanotechnology for Bioremediation of Heavy Metals,” in Modern Approaches in Waste Bioremediation: Environmental Microbiology, ed. M. P. Shah (Cham: Springer International Publishing), doi: 10.1007/978-3-031-24086-7_24
Kumari, B., and Singh, D. P. (2016). A review on multifaceted application of nanoparticles in the field of bioremediation of petroleum hydrocarbons. Ecol. Eng. 97, 98–105. doi: 10.1016/j.ecoleng.2016.08.006
Latawiec, A. E., Simmons, P., and Reid, B. J. (2010). Decision-makers’ perspectives on the use of bioaccessibility for risk-based regulation of contaminated land. Environ. Int. 36, 383–389. doi: 10.1016/j.envint.2010.02.007
Lee, P. K. H., Macbeth, T. W., Sorenson, K. S., Deeb, R. A., and Alvarez-Cohen, L. (2008). Quantifying genes and transcripts to assess the in situ physiology of “ Dehalococcoides ” spp. in a trichloroethene-contaminated groundwater site. Appl. Environ. Microbiol. 74, 2728–2739. doi: 10.1128/AEM.02199-07
Li, F., Deng, D., Wadden, A., Parvis, P., Cutt, D., and Li, M. (2023). Effective removal of trace 1,4-dioxane by biological treatments augmented with propanotrophic single culture versus synthetic consortium. J. Hazard. Mater. Adv. 9:100246. doi: 10.1016/j.hazadv.2023.100246
Li, X., Chen, Y., Du, X., Zheng, J., Lu, D., and Liu, Z. (2021). Ecological response in the integrated process of biostimulation and bioaugmentation of diesel-contaminated soil. Appl. Sci. 11:6305. doi: 10.3390/app11146305
Lim, M. W., Lau, E. V., and Poh, P. E. (2016). A comprehensive guide of remediation technologies for oil contaminated soil — Present works and future directions. Marine Pollut. Bull. 109, 14–45. doi: 10.1016/j.marpolbul.2016.04.023
Liu, N., Zhang, Y., An, Y., and Wang, L. (2018). Preparation of integrative cubes as a novel biological permeable reactive barrier medium for the enhancement of in situ aerobic bioremediation of nitrobenzene-contaminated groundwater. Environ. Earth Sci. 77:707. doi: 10.1007/s12665-018-7890-8
Liu, X., Ecarnot, M., and Kontro, M. H. (2015). The physicochemical conditions of isolation source determine the occurrence of Pseudomonas fluorescens group species. Ann. Microbiol. 65, 2363–2377. doi: 10.1007/s13213-015-1078-1
Lo, C. K. (2023). What Is the Impact of ChatGPT on Education? A Rapid Review of the Literature. Educ. Sci. 13:410. doi: 10.3390/educsci13040410
Löffler, F. E., Tiedje, J. M., and Sanford, R. A. (1999). Fraction of electrons consumed in electron acceptor reduction and hydrogen thresholds as indicators of halorespiratory physiology. Appl. Environ. Microbiol. 65, 4049–4056.
Ma, K.-Y., Sun, M.-Y., Dong, W., He, C.-Q., Chen, F.-L., and Ma, Y.-L. (2016). Effects of nutrition optimization strategy on rhamnolipid production in a Pseudomonas aeruginosa strain DN1 for bioremediation of crude oil. Biocatal. Agricult. Biotechnol. 6, 144–151. doi: 10.1016/j.bcab.2016.03.008
Magnuson, J. K., Romine, M. F., Burris, D. R., and Kingsley, M. T. (2000). Trichloroethene reductive dehalogenase from Dehalococcoides ethenogenes: Sequence of tceA and Substrate Range Characterization. Appl. Environ. Microbiol. 66, 5141–5147.
Mahammedi, C., Mahdjoubi, L., Booth, C. A., Akram, H., and Butt, T. E. (2020). A systematic review of risk assessment tools for contaminated sites – Current perspectives and future prospects. Environ. Res. 191:110180. doi: 10.1016/j.envres.2020.110180
Margesin, R. (2000). Potential of cold-adapted microorganisms for bioremediation of oil-polluted Alpine soils. Int. Biodeterior. Biodegrad. 46, 3–10. doi: 10.1016/S0964-8305(00)00049-4
Mariaamalraj, S. K., Pasumarthi, R., Achary, A., and Mutnuri, S. (2016). Effect of rhamnolipid on biodegradation of hydrocarbons in non-aqueous-phase liquid (NAPL). Bioremed. J. 20, 183–193. doi: 10.1080/10889868.2016.1212807
Martins, V. G., Kalil, S. J., and Costa, J. A. V. (2009). In situ bioremediation using biosurfactant produced by solid state fermentation. World J. Microbiol. Biotechnol. 25, 843–851. doi: 10.1007/s11274-009-9955-z
Mattsson, M. K., Liu, X., Yu, D., and Kontro, M. H. (2015). Depth, soil type, water table, and site effects on microbial community composition in sediments of pesticide-contaminated aquifer. Environ. Sci. Pollut. Res. 22, 10263–10279. doi: 10.1007/s11356-015-4224-1
May, A. L., Xie, Y., Kara Murdoch, F., Michalsen, M. M., Löffler, F. E., and Campagna, S. R. (2022). Metabolome patterns identify active dechlorination in bioaugmentation consortium SDC-9TM. Front. Microbiol. 13:981994. doi: 10.3389/fmicb.2022.981994
McGrath, T. J., Ball, A. S., and Clarke, B. O. (2017). Critical review of soil contamination by polybrominated diphenyl ethers (PBDEs) and novel brominated flame retardants (NBFRs); concentrations, sources and congener profiles. Environ. Pollut. 230, 741–757. doi: 10.1016/j.envpol.2017.07.009
McGuire, T. M., Newell, C. J., Looney, B. B., Vangelas, K. M., and Sink, C. H. (2004). Historical analysis of monitored natural attenuation: A survey of 191 chlorinated solvent sites and 45 solvent plumes. Remediation 15, 99–112. doi: 10.1002/rem.20036
Mercer, J. W., and Cohen, R. M. (1990). A review of immiscible fluids in the subsurface: Properties, models, characterization and remediation. J. Contam. Hydrol. 6, 107–163.
Mikkonen, A., Yläranta, K., Tiirola, M., Dutra, L. A. L., Salmi, P., Romantschuk, M., et al. (2018). Successful aerobic bioremediation of groundwater contaminated with higher chlorinated phenols by indigenous degrader bacteria. Water Res. 138, 118–128. doi: 10.1016/j.watres.2018.03.033
Ministry of the Environment (2014). Pilaantuneen maa-alueen riskinarviointi ja kestävä riskinhallinta [Risk assessment and sustainable risk management of contaminated land]. Helsinki: Ministry of the Environment.
Miri, S., Naghdi, M., Rouissi, T., Kaur Brar, S., and Martel, R. (2019). Recent biotechnological advances in petroleum hydrocarbons degradation under cold climate conditions: A review. Crit. Rev. Environ. Sci. Technol. 49, 553–586. doi: 10.1080/10643389.2018.1552070
Mohn, W. W., and Stewart, G. R. (2000). Limiting factors for hydrocarbon biodegradation at low temperature in Arctic soils. Soil Biol. Biochem. 32, 1161–1172. doi: 10.1016/S0038-0717(00)00032-8
Mukherjee, S., Sipilä, T., Pulkkinen, P., and Yrjälä, K. (2015). Secondary successional trajectories of structural and catabolic bacterial communities in oil-polluted soil planted with hybrid poplar. Mol. Ecol. 24, 628–642. doi: 10.1111/mec.13053
Müller, J. A., Rosner, B. M., von Abendroth, G., Meshulam-Simon, G., McCarty, P. L., and Spormann, A. M. (2004). Molecular Identification of the Catabolic Vinyl Chloride Reductase from Dehalococcoides sp. Strain VS and Its Environmental Distribution. Appl. Environ. Microbiol. 70, 4880–4888. doi: 10.1128/AEM.70.8.4880-4888.2004
Mulligan, C. N., and Yong, R. N. (2004). Natural attenuation of contaminated soils. Environ. Int. 30, 587–601. doi: 10.1016/j.envint.2003.11.001
Naeimi, M., Shavandi, M., and Alaie, E. (2021). Determining the impact of biofilm in the bioaugmentation process of benzene-contaminated resources. J. Environ. Chem. Eng. 9:104976. doi: 10.1016/j.jece.2020.104976
Nathanail, J., Bardos, P., and Nathanail, P. (2007). Contaminated land management: Ready reference. London: Land Quality Press and EPP Publications Ltd.
National Research Council (U.S.) ed. (1993). In situ bioremediation: when does it work? Washington, DC: National Academy Press.
Nikolova, C., and Gutierrez, T. (2021). Biosurfactants and Their Applications in the Oil and Gas Industry: Current State of Knowledge and Future Perspectives. Front. Bioeng. Biotechnol. 9:626639. doi: 10.3389/fbioe.2021.626639
Nikunen, S., Pyysing, S., Talvenmäki, H., Allen, J., Romantschuk, M., and Silvennoinen, H. (2017). “Utilization of in situ techniques in remediation of oil-polluted sites in Finland,” in European achievements in soil remediation and brownfield redevelopment: A report of the European Information and Observation Network’s National Reference Centres for Soil (Eionet NRC Soil), eds A. Paya and S. Pelaez (Geneva: European Commission).
Norrman, J., Söderqvist, T., Volchko, Y., Back, P.-E., Bohgard, D., Ringshagen, E., et al. (2020). Enriching social and economic aspects in sustainability assessments of remediation strategies – Methods and implementation. Sci. Total Environ. 707:136021. doi: 10.1016/j.scitotenv.2019.136021
Nousiainen, A. O., Björklöf, K., Sagarkar, S., Mukherjee, S., Purohit, H. J., Kapley, A., et al. (2014). Atrazine degradation in boreal nonagricultural subsoil and tropical agricultural soil. J. Soils Sediments 14, 1179–1188. doi: 10.1007/s11368-014-0868-6
Nykänen, A., Kontio, H., Klutas, O., Penttinen, O.-P., Kostia, S., Mikola, J., et al. (2012). Increasing lake water and sediment oxygen levels using slow release peroxide. Sci. Total Environ. 429, 317–324. doi: 10.1016/j.scitotenv.2012.04.044
Öberg, T., and Bergbäck, B. (2005). A Review of Probabilistic Risk Assessment of Contaminated Land (12 pp). J. Soils Sediments 5, 213–224. doi: 10.1065/jss2005.08.143
Pande, V., Pandey, S. C., Sati, D., Pande, V., and Samant, M. (2020). Bioremediation: an emerging effective approach towards environment restoration. Environ. Sustainability 3, 91–103. doi: 10.1007/s42398-020-00099-w
Parajuli, A., Grönroos, M., Siter, N., Puhakka, R., Vari, H. K., Roslund, M. I., et al. (2018). Urbanization Reduces Transfer of Diverse Environmental Microbiota Indoors. Front. Microbiol. 9:84. doi: 10.3389/fmicb.2018.00084
Patel, N., Khan, M. D. Z. A., Shahane, S., Rai, D., Chauhan, D., Kant, C., et al. (2020). Emerging Pollutants in Aquatic Environment: Source, Effect, and Challenges in Biomonitoring and Bioremediation- A Review. Pollution 6:646. doi: 10.22059/poll.2019.285116.646
Peltola, R., Salkinoja-Salonen, M., Pulkkinen, J., Koivunen, M., Turpeinen, A.-R., Aarnio, T., et al. (2006). Nitrification in polluted soil fertilized with fast- and slow-releasing nitrogen: A case study at a refinery landfarming site. Environ. Pollut. 143, 247–253. doi: 10.1016/j.envpol.2005.11.029
Penttinen, R. (2001). Maaperän ja pohjaveden kunnostus - yleisimpien menetelmien esittely [Soil and ground water remediation - presentation of the most common methods]. Helsinki: Helda.
Pierro, L., Matturro, B., Rossetti, S., Sagliaschi, M., Sucato, S., Alesi, E., et al. (2017). Polyhydroxyalkanoate as a slow-release carbon source for in situ bioremediation of contaminated aquifers: From laboratory investigation to pilot-scale testing in the field. N. Biotechnol. 37, 60–68. doi: 10.1016/j.nbt.2016.11.004
Prieto, S. A., Mengiste, E. T., and García de Soto, B. (2023). Investigating the Use of ChatGPT for the Scheduling of Construction Projects. Buildings 13:857. doi: 10.3390/buildings13040857
Pukkila, V., and Kontro, M. H. (2014). Dichlobenil and 2,6-dichlorobenzamide (BAM) dissipation in topsoil and deposits from groundwater environment within the boreal region in southern Finland. Environ. Sci. Pollut. Res. 21, 2289–2297. doi: 10.1007/s11356-013-2164-1
Raj, A., Kumar, A., and Dames, J. F. (2021). Tapping the role of microbial biosurfactants in pesticide remediation: An eco-friendly approach for environmental sustainability. Front. Microbiol. 12:791723. doi: 10.3389/fmicb.2021.791723
Ray, S., Gunzburg, M. J., Wilce, M., Panjikar, S., and Anand, R. (2016). Structural basis of selective aromatic pollutant sensing by the effector binding domain of mopr, an NTRC family transcriptional regulator. ACS Chem. Biol. 11, 2357–2365. doi: 10.1021/acschembio.6b00020
Rifai, H. S., Newell, C. J., Gonzales, J. R., and Wilson, J. T. (2000). Modeling natural attenuation of fuels with bioplume III. J. Environ. Eng. 126, 428–438. doi: 10.1061/(ASCE)0733-93722000126:5(428)
Ritalahti, K. M., Amos, B. K., Sung, Y., Wu, Q., Koenigsberg, S. S., and Löffler, F. E. (2006). Quantitative PCR Targeting 16S rRNA and reductive dehalogenase genes simultaneously monitors multiple dehalococcoides strains. Appl. Environ. Microbiol. 72, 2765–2774. doi: 10.1128/AEM.72.4.2765-2774.2006
Rizwan, M., Singh, M., Mitra, C. K., and Morve, R. K. (2014). Ecofriendly application of nanomaterials: nanobioremediation. J. Nanoparticles 2014, e431787. doi: 10.1155/2014/431787
Robrock, K. R., Korytár, P., and Alvarez-Cohen, L. (2008). Pathways for the Anaerobic Microbial Debromination of Polybrominated Diphenyl Ethers. Environ. Sci. Technol. 42, 2845–2852. doi: 10.1021/es0720917
Romantschuk, M., Sarand, I., Petänen, T., Peltola, R., Jonsson-Vihanne, M., Koivula, T., et al. (2000). Means to improve the effect of in situ bioremediation of contaminated soil: an overview of novel approaches. Environ. Pollut. 107, 179–185. doi: 10.1016/S0269-7491(99)00136-0
Rosén, L., Back, P.-E., Söderqvist, T., Norrman, J., Brinkhoff, P., Norberg, T., et al. (2015). SCORE: A novel multi-criteria decision analysis approach to assessing the sustainability of contaminated land remediation. Sci. Total Environ. 511, 621–638. doi: 10.1016/j.scitotenv.2014.12.058
Roslund, M. I., Rantala, S., Oikarinen, S., Puhakka, R., Hui, N., Parajuli, A., et al. (2019). Endocrine disruption and commensal bacteria alteration associated with gaseous and soil PAH contamination among daycare children. Environ. Int. 130:104894. doi: 10.1016/j.envint.2019.06.004
Rügner, H., Finkel, M., Kaschl, A., and Bittens, M. (2006). Application of monitored natural attenuation in contaminated land management—A review and recommended approach for Europe. Environ. Sci. Policy 9, 568–576. doi: 10.1016/j.envsci.2006.06.001
Sagarkar, S., Mukherjee, S., Nousiainen, A., Björklöf, K., Purohit, H. J., Jørgensen, K. S., et al. (2013). Monitoring bioremediation of atrazine in soil microcosms using molecular tools. Environ. Pollut. 172, 108–115. doi: 10.1016/j.envpol.2012.07.048
Salam, M. M. A., Ruhui, W., Sinkkonen, A., Pappinen, A., and Pulkkinen, P. (2022). Effects of Contaminated Soil on the Survival and Growth Performance of European (Populus tremula L.) and Hybrid Aspen (Populus tremula L. × Populus tremuloides Michx.) Clones Based on Stand Density. Plants 11:1970. doi: 10.3390/plants11151970
Salminen, J. M., Tuomi, P. M., and Jørgensen, K. S. (2008). Functional Gene Abundances (nahAc, alkB, xylE) in the Assessment of the Efficacy of Bioremediation. Appl. Biochem. Biotechnol. 151, 638–652. doi: 10.1007/s12010-008-8275-3
Sarand, I., Haario, H., Jørgensen, K. S., and Romantschuk, M. (2000). Effect of inoculation of a TOL plasmid containing mycorrhizosphere bacterium on development of Scots pine seedlings, their mycorrhizosphere and the microbial flora in m-toluate-amended soil. FEMS Microbiol. Ecol. 31, 127–141. doi: 10.1111/j.1574-6941.2000.tb00678.x
Sarand, I., Skärfstad, E., Forsman, M., Romantschuk, M., and Shingler, V. (2001). Role of the DmpR-Mediated Regulatory Circuit in Bacterial Biodegradation Properties in Methylphenol-Amended Soils. Appl. Environ. Microbiol. 67, 162–171. doi: 10.1128/AEM.67.1.162-171.2001
Schaefer, C. E., Callaghan, A. V., King, J. D., and McCray, J. E. (2009). Dense Nonaqueous Phase Liquid Architecture and Dissolution in Discretely Fractured Sandstone Blocks. Environ. Sci. Technol. 43, 1877–1883. doi: 10.1021/es8011172
Schaefer, C. E., Lavorgna, G. M., White, E. B., and Annable, M. D. (2017). Bioaugmentation in a Well-Characterized Fractured Rock DNAPL Source Area. Groundwater Monitor. Remed. 37, 35–42. doi: 10.1111/gwmr.12208
Shahi, A., Aydin, S., Ince, B., and Ince, O. (2016). Evaluation of microbial population and functional genes during the bioremediation of petroleum-contaminated soil as an effective monitoring approach. Ecotoxicol. Environ. Safety 125, 153–160. doi: 10.1016/j.ecoenv.2015.11.029
Shahid, M., Yaseen, N., Noman, M., Ahmed, T., and Javed, M. T. (2022). “Nanoremediation: An Innovative Approach for Environmental Safety,” in Managing Plant Production Under Changing Environment, eds M. Hasanuzzaman, G. J. Ahammed, and K. Nahar (Singapore: Springer Nature), doi: 10.1007/978-981-16-5059-8_1
Sharma, N., Lavania, M., and Lal, B. (2022). Biosurfactant: A next-generation tool for sustainable remediation of organic pollutants. Front. Microbiol. 12:821531. doi: 10.3389/fmicb.2021.821531
Sheu, Y. T., Lien, P. J., Chen, K. F., Ou, J. H., and Kao, C. M. (2016). Application of NZVI-contained emulsified substrate to bioremediate PCE-contaminated groundwater – A pilot-scale study. Chem. Eng. J. 304, 714–727. doi: 10.1016/j.cej.2016.06.126
Shrestha, B., Acosta-Martinez, V., Cox, S. B., Green, M. J., Li, S., and Cañas-Carrell, J. E. (2013). An evaluation of the impact of multiwalled carbon nanotubes on soil microbial community structure and functioning. J. Hazard. Mater. 261, 188–197. doi: 10.1016/j.jhazmat.2013.07.031
Simpanen, S. (2016). Evaluation of in situ remediation methods in soils contaminated with organic pollutants. Helsinki: HELDA.
Simpanen, S., Dahl, M., Gerlach, M., Mikkonen, A., Malk, V., Mikola, J., et al. (2016a). Biostimulation proved to be the most efficient method in the comparison of in situ soil remediation treatments after a simulated oil spill accident. Environ. Sci. Pollut. Res. 23, 25024–25038. doi: 10.1007/s11356-016-7606-0
Simpanen, S., Mäkelä, R., Mikola, J., Silvennoinen, H., and Romantschuk, M. (2016b). Bioremediation of creosote contaminated soil in both laboratory and field scale: Investigating the ability of methyl-β-cyclodextrin to enhance biostimulation. Int. Biodeterior. Biodegrad. 106, 117–126. doi: 10.1016/j.ibiod.2015.10.013
Simpanen, S., Yu, D., Mäkelä, R., Talvenmäki, H., Sinkkonen, A., Silvennoinen, H., et al. (2018). Soil vapor extraction of wet gasoline-contaminated soil made possible by electroosmotic dewatering–lab simulations applied at a field site. J. Soils Sediments 18, 3303–3309. doi: 10.1007/s11368-017-1717-1
Singh, A. K., Bilal, M., Iqbal, H. M. N., and Raj, A. (2021). Trends in predictive biodegradation for sustainable mitigation of environmental pollutants: Recent progress and future outlook. Sci. Total Environ. 770, 144561. doi: 10.1016/j.scitotenv.2020.144561
Singh, R., Singh, S., Parihar, P., Singh, V. P., and Prasad, S. M. (2015). Arsenic contamination, consequences and remediation techniques: A review. Ecotoxicol. Environ. Safety 112, 247–270. doi: 10.1016/j.ecoenv.2014.10.009
Singh, Y., and Saxena, M. K. (2022). Insights into the recent advances in nano-bioremediation of pesticides from the contaminated soil. Front. Microbiol. 13:982611. doi: 10.3389/fmicb.2022.982611
Sinkkonen, A., Kauppi, S., Pukkila, V., Nan, H., Płociniczak, T., Kontro, M., et al. (2013). Previous exposure advances the degradation of an anthropogenic s-triazine regardless of soil origin. J. Soils Sediments 13, 1430–1438. doi: 10.1007/s11368-013-0742-y
Sinkkonen, A., Ollila, S., and Romantschuk, M. (2014). Changes in TcpA gene frequency explain 2,4,6-trichlorophenol degradation in mesocosms. J. Environ. Sci. Health Part B 49, 756–759. doi: 10.1080/03601234.2014.929865
Sipilä, T. P., Väisänen, P., Paulin, L., and Yrjälä, K. (2010). Sphingobium sp. HV3 degrades both herbicides and polyaromatic hydrocarbons using ortho- and meta-pathways with differential expression shown by RT-PCR. Biodegradation 21, 771–784. doi: 10.1007/s10532-010-9342-3
Sorvari, J., and Seppälä, J. (2010). A decision support tool to prioritize risk management options for contaminated sites. Sci. Total Environ. 408, 1786–1799. doi: 10.1016/j.scitotenv.2009.12.026
Summer, D., Schöftner, P., Wimmer, B., Pastar, M., Kostic, T., Sessitsch, A., et al. (2020). Synergistic effects of microbial anaerobic dechlorination of perchloroethene and nano zero-valent iron (nZVI) – A lysimeter experiment. N. Biotechnol. 57, 34–44. doi: 10.1016/j.nbt.2020.02.005
Sun, C., Wei, S., Tan, H., Huang, Y., and Zhang, Y. (2022). Progress in upcycling polylactic acid waste as an alternative carbon source: A review. Chem. Eng. J. 446:136881. doi: 10.1016/j.cej.2022.136881
Sunanda Ghosh Sachan, S. (2023). Nanobioremediation of pesticides by immobilization technique: a review. Int. J. Environ. Sci. Technol. 20, 3455–3466. doi: 10.1007/s13762-021-03759-4
Suni, S., Malinen, E., Kosonen, J., Silvennoinen, H., and Romantschuk, M. (2007). Electrokinetically enhanced bioremediation of creosote-contaminated soil: Laboratory and field studies. J. Environ. Sci. Health Part A 42, 277–287. doi: 10.1080/10934520601134213
Surameery, N. M. S., and Shakor, M. Y. (2023). Use chat GPT to solve programming bugs. International Journal of Information Technology & Computer Eng. 3, 17–22. doi: 10.55529/ijitc.31.17.22
SYKE (2013). PIRTU-ekotehokkuuslaskentatyökalu [PIRTU ecoefficiency calculation tool]. Available online at: https://www.syke.fi/fi-FI/Tutkimus__kehittaminen/Tutkimus_ja_kehittamishankkeet/Hankkeet/Pilaantuneen_maaperan_ja_pohjaveden_riskinhallintaratkaisujen_ekotehokkuus_PIRRE_PIRRE2/PIRTUekotehokkuuslaskentatyokalu (accessed August 29, 2023).
Tahseen, R., Afzal, M., Iqbal, S., Shabir, G., Khan, Q. M., Khalid, Z. M., et al. (2016). Rhamnolipids and nutrients boost remediation of crude oil-contaminated soil by enhancing bacterial colonization and metabolic activities. Int. Biodeterior. Biodegrad. 115, 192–198. doi: 10.1016/j.ibiod.2016.08.010
Talvenmäki, H. (2020). Advantages and limitations of combined in situ remediation methods and mechanisms at petroleum fuel product contaminated sites. Helsinki: HELDA.
Talvenmäki, H., Saartama, N., Haukka, A., Lepikkö, K., Pajunen, V., Punkari, M., et al. (2021). In situ bioremediation of Fenton’s reaction–treated oil spill site, with a soil inoculum, slow release additives, and methyl-β-cyclodextrin. Environ. Sci. Pollut. Res. 28, 20273–20289. doi: 10.1007/s11356-020-11910-w
Tarasov, A. L., Borzenkov, I. A., Milekhina, E. I., Mysiakina, I. S., and Beliaev, S. S. (2004). [Utilization of H2O2 as the oxygen source by bacteria of the genera Pseudomonas and Rhodococcus]. Mikrobiologiia 73, 465–471.
Tauqeer, H. M., Karczewska, A., Lewińska, K., Fatima, M., Khan, S. A., Farhad, M., et al. (2021). “Environmental concerns associated with explosives (HMX, TNT, and RDX), heavy metals and metalloids from shooting range soils: Prevailing issues, leading management practices, and future perspectives,” in Handbook of Bioremediation, eds M. Hasanuzzaman and M. N. V. Prasad (Cambridge, MA: Academic Press), doi: 10.1016/B978-0-12-819382-2.00036-3
Tervo, J.-P. (2013). Polttoaineen pilaaman maaperän kunnostusmenetelmän valinta pilotointikokeilla [Selection of Remediation Method for Fuel Contaminated Soil, Iisalmi Eteläntie Pilot Experiment]. Kuopio: Savonia.
Tervo, J. P., and Kolehmainen, A. (2016). Eteläntien säiliöauto-onnettomuus, Iisalmi Eteläntie. Öljyvahingon jälkihoitotoimenpiteiden loppuraportti. Kuopio: Ramboll.
Thomé, A., Reginatto, C., Cecchin, I., and Colla, L. M. (2014). Bioventing in a residual clayey soil contaminated with a blend of biodiesel and diesel oil. J. Environ. Eng. 140:06014005. doi: 10.1061/(ASCE)EE.1943-7870.0000863
Tiehm, A., and Schmidt, K. R. (2011). Sequential anaerobic/aerobic biodegradation of chloroethenes—aspects of field application. Curr. Opin. Biotechnol. 22, 415–421. doi: 10.1016/j.copbio.2011.02.003
Tomei, M. C., and Daugulis, A. J. (2013). Ex Situ Bioremediation of Contaminated Soils: An Overview of Conventional and Innovative Technologies. Crit. Rev. Environ. Sci. Technol. 43, 2107–2139. doi: 10.1080/10643389.2012.672056
Tsai, Y.-J., Chou, Y.-C., Wu, Y.-S., and Lee, C.-H. (2020). Noninvasive survey technology for LNAPL-contaminated site investigation. J. Hydrol. 587, 125002. doi: 10.1016/j.jhydrol.2020.125002
Tumeo, M., Braddock, J., Venator, T., Rog, S., and Owens, D. (1994). Effectiveness of a biosurfactant in removing weathered crude oil from subsurface beach material. Spill Sci. Technol. Bull. 1, 53–59. doi: 10.1016/1353-2561(94)90007-8
Tuomi, P., and Vaajasaari, K. (2004). Monitoroidun luontaisen puhdistumisen (MLP) käyttö pilaantuneiden alueiden kunnostuksessa [Monitored Natural Attenuation (MNA) as a remediation method for contaminated soil areas]. Helsinki: Edita Publishig Ltd.
Tyagi, M., da Fonseca, M. M. R., and de Carvalho, C. C. C. R. (2011). Bioaugmentation and biostimulation strategies to improve the effectiveness of bioremediation processes. Biodegradation 22, 231–241. doi: 10.1007/s10532-010-9394-4
USEPA (2017). EPA 510-B-17-003 - How To Evaluate Alternative Cleanup TechnologiesFor Underground Storage Tank Sites: A Guide For Corrective Action Plan Reviewers. Washington, DC: United States Environmental Protection Agency.
Verla, A. W., Enyoh, C. E., Verla, E. N., and Nwarnorh, K. O. (2019). Microplastic–toxic chemical interaction: a review study on quantified levels, mechanism and implication. SN Appl. Sci. 1, 1400. doi: 10.1007/s42452-019-1352-0
Volchko, Y., Norrman, J., Rosén, L., Bergknut, M., Josefsson, S., Söderqvist, T., et al. (2014). Using soil function evaluation in multi-criteria decision analysis for sustainability appraisal of remediation alternatives. Sci. Total Environ. 485, 785–791. doi: 10.1016/j.scitotenv.2014.01.087
Wiegel, J., and Wu, Q. (2000). Microbial reductive dehalogenation of polychlorinated biphenyls. FEMS Microbiol. Ecol. 32, 1–15. doi: 10.1111/j.1574-6941.2000.tb00693.x
Wilms, W., Woźniak-Karczewska, M., Niemczak, M., Parus, A., Frankowski, R., Wolko, Ł, et al. (2023). 2,4-D versus 2,4-D based ionic liquids: Effect of cation on herbicide biodegradation, tfdA genes abundance and microbiome changes during soil bioaugmentation. J. Hazard. Mater. 452, 131209. doi: 10.1016/j.jhazmat.2023.131209
Xiao, Z., Jiang, W., Chen, D., and Xu, Y. (2020). Bioremediation of typical chlorinated hydrocarbons by microbial reductive dechlorination and its key players: A review. Ecotoxicol. Environ. Safety 202, 110925. doi: 10.1016/j.ecoenv.2020.110925
Xie, Y., Dong, H., Zeng, G., Tang, L., Jiang, Z., Zhang, C., et al. (2017). The interactions between nanoscale zero-valent iron and microbes in the subsurface environment: A review. J. Hazard. Mater. 321, 390–407. doi: 10.1016/j.jhazmat.2016.09.028
Yan, L., Hui, N., Simpanen, S., Tudeer, L., and Romantschuk, M. (2020). Simulation of microbial response to accidental diesel spills in basins containing brackish sea water and sediment. Front. Microbiol. 11:593232. doi: 10.3389/fmicb.2020.593232
Yan, L., Yu, D., Hui, N., Naanuri, E., Viggor, S., Gafarov, A., et al. (2018). Distribution of archaeal communities along the coast of the Gulf of Finland and their response to oil contamination. Front. Microbiol. 9:15. doi: 10.3389/fmicb.2018.00015
Yen, C.-H., Chen, K.-F., Kao, C.-M., Liang, C.-H., and Chen, T.-Y. (2011). Application of persulfate to remediate petroleum hydrocarbon-contaminated soil: Feasibility and comparison with common oxidants. J. Hazard. Mater. 186, 2097–2102.
Yeo, I. W., Ji, S.-H., and Lee, K.-K. (2003). Density-surfactant-motivated removal of DNAPL trapped in dead-end fractures. Geophys. Res. Lett. 30, 4. doi: 10.1029/2003GL017186
Yrjälä, K., Keskinen, A.-K., Åkerman, M.-L., Fortelius, C., and Sipilä, T. P. (2010). The rhizosphere and PAH amendment mediate impacts on functional and structural bacterial diversity in sandy peat soil. Environ. Pollut. 158, 1680–1688. doi: 10.1016/j.envpol.2009.11.026
Yu, Y., Zhang, K., Li, Z., Ren, C., Chen, J., Lin, Y.-H., et al. (2020). Microbial Cleavage of C–F Bonds in Two C 6 Per- and Polyfluorinated Compounds via Reductive Defluorination. Environ. Sci. Technol. 54, 14393–14402. doi: 10.1021/acs.est.0c04483
Yuan, J., Li, S., Cheng, J., Guo, C., Shen, C., He, J., et al. (2021). Potential role of methanogens in microbial reductive dechlorination of organic chlorinated pollutants in situ. Environ. Sci. Technol. 55, 5917–5928. doi: 10.1021/acs.est.0c08631
Zha, Y., Yeh, T.-C. J., Illman, W. A., Mok, C. M. W., Tso, C.-H. M., Carrera, B. A., et al. (2019). Exploitation of pump-and-treat remediation systems for characterization of hydraulic heterogeneity. J. Hydrol. 573, 324–340. doi: 10.1016/j.jhydrol.2019.03.089
Zhang, W., Wang, C.-B., and Lien, H.-L. (1998). Treatment of chlorinated organic contaminants with nanoscale bimetallic particles. Catalysis Today 40, 387–395. doi: 10.1016/S0920-5861(98)00067-4
Zheng, W., Cui, T., and Li, H. (2022). Combined technologies for the remediation of soils contaminated by organic pollutants. A review. Environ Chem Lett 20, 2043–2062. doi: 10.1007/s10311-022-01407-y
Keywords: biostimulation, bioremediation, biodegradation, in situ, bacteria, organic contaminants
Citation: Romantschuk M, Lahti-Leikas K, Kontro M, Galitskaya P, Talvenmäki H, Simpanen S, Allen JA and Sinkkonen A (2023) Bioremediation of contaminated soil and groundwater by in situ biostimulation. Front. Microbiol. 14:1258148. doi: 10.3389/fmicb.2023.1258148
Received: 13 July 2023; Accepted: 22 September 2023;
Published: 06 November 2023.
Edited by:
Francis Hassard, Cranfield University, United KingdomReviewed by:
Rishikesh Bajagain, Korea University, Republic of KoreaAlif Chebbi, Roma Tre University, Italy
Copyright © 2023 Romantschuk, Lahti-Leikas, Kontro, Galitskaya, Talvenmäki, Simpanen, Allen and Sinkkonen. This is an open-access article distributed under the terms of the Creative Commons Attribution License (CC BY). The use, distribution or reproduction in other forums is permitted, provided the original author(s) and the copyright owner(s) are credited and that the original publication in this journal is cited, in accordance with accepted academic practice. No use, distribution or reproduction is permitted which does not comply with these terms.
*Correspondence: Martin Romantschuk, bWFydGluLnJvbWFudHNjaHVrQGhlbHNpbmtpLmZp