- 1Department of Ecology and Evolutionary Biology, Tulane University, New Orleans, LA, United States
- 2Department of Biological Sciences, University of Pittsburgh, Pittsburgh, PA, United States
- 3Department of Biology, The Pennsylvania State University, State College, PA, United States
- 4Falk School of Sustainability and Environment, Chatham University, Pittsburgh, PA, United States
Symbiotic relationships between animals and microbes are important for a range of functions, from digestion to protection from pathogens. However, the impact of temperature variation on these animal-microbe interactions remains poorly understood. Amphibians have experienced population declines and even extinctions on a global scale due to chytridiomycosis, a disease caused by chytrid fungi in the genus Batrachochytrium. Variation in susceptibility to this disease exists within and among host species. While the mechanisms generating differences in host susceptibility remain elusive, differences in immune system components, as well as variation in host and environmental temperatures, have been associated with this variation. The symbiotic cutaneous bacteria of amphibians are another potential cause for variation in susceptibility to chytridiomycosis, with some bacterial species producing antifungal metabolites that prevent the growth of Bd. The growth of both Bd and bacteria are affected by temperature, and thus we hypothesized that amphibian skin bacteria may be more effective at preventing Bd growth at certain temperatures. To test this, we collected bacteria from the skins of frogs, harvested the metabolites they produced when grown at three different temperatures, and then grew Bd in the presence of those metabolites under those same three temperatures in a three-by-three fully crossed design. We found that both the temperature at which cutaneous bacteria were grown (and metabolites produced) as well as the temperature at which Bd is grown can impact the ability of cutaneous bacteria to inhibit the growth of Bd. While some bacterial isolates showed the ability to inhibit Bd growth across multiple temperature treatments, no isolate was found to be inhibitive across all combinations of bacterial incubation or Bd challenge temperatures, suggesting that temperature affects both the metabolites produced and the effectiveness of those metabolites against the Bd pathogen. These findings move us closer to a mechanistic understanding of why chytridiomycosis outbreaks and related amphibian declines are often limited to certain climates and seasons.
1. Introduction
The importance of symbiotic relationships between animals and microbes has long been appreciated thanks to research in a few well-studied systems, like coral reefs (Li, 2019), hydrothermal vents (Petersen et al., 2011), and the gut microbiomes of animals who specialize on difficult to digest and/or nutrient poor food sources (e.g., termites; Brune and Ohkuma, 2010). However, the recognition that all animals live in symbiotic association with bacteria and other microorganisms is a recent development, and one that is dramatically changing the field of biology (Chow et al., 2010). With this change in our scope of understanding of host-microbiome interactions comes a need to understand how the future of such interactions may be affected by environmental change (Carey and Duddleston, 2014). In many cases, we know little about how variation in temperature, a variable that shapes many aspects of the physiology and behavior of animal hosts, affects the services that microbial symbionts provide. A clearer understanding of temperature’s impact on such symbiotic relationships is needed to predict wildlife responses to climate change and other environmental stressors.
Effects of temperature on the growth and community structure of animal microbiomes has not been well studied. Much more is known about effects of temperature on microbial communities in soil and in other environmental samples. For example, bacterial diversity and community structure is thought to be primarily controlled by environmental temperature in permafrost and other low-temperature environments (Jansson and Taş, 2014). This predominant effect of temperature has also been observed in Arctic fjord soils, with productivity, abundance, and proportion of active bacterial cells increasing during the spring (Piquet et al., 2016). From the few studies available, most of which involve marine animals or insects, the pattern for animal microbiomes appears to be more varied. For example, in one study sponge-associated bacterial communities were found to remain stable across large seasonal shifts in temperature (between 12°C and 26°C; Erwin et al., 2012). Yet, when bacterial communities associated with the sponge Rhopaloeides oborabile were exposed to temperatures ranging from 27°C to 33°C, differences in community structure were observed (Webster et al., 2008). Furthermore, elevated temperatures in R. oborabile have been shown to cause an immediate stress response in both the host and its microbial community (Fan et al., 2013). Though examples are rarer, temperature appears to affect the bacterial communities of insects as well. For example, in an experimental evolution study using Drosophila melanogaster flies as hosts to several strains of Wolbachia, which strain spread in the fly population was found to depend upon the temperature at which the population was maintained (Versace et al., 2014).
In some animals, temperature is known to affect the potential of symbiotic microbes to aid their hosts in resisting infection or other forms of attack. However, the way in which temperature affects the host-enemy-symbiont interaction varies greatly among the systems in which it has been studied (reviewed in Corbin et al., 2017). For example, elevated temperatures have been shown to increase the expression of virulence genes in Vibrio shiloi, which causes bleaching in the coral Oculina patagonica (Rosenberg and Ben-Halm, 2002). On the other side of the coin, the defense of ascidians (sea squirts) against pathogens can be bolstered by diverse secondary metabolites produced by their microbial communities. Differences in the metabolites produced by the bacterial communities of these animals, and correspondingly the ability of these bacteria to protect the animal from infection, may be caused by changes in water temperature (Tianero et al., 2015). In insects the effects of temperature on the protective ability of microbes appear to vary. For example, temperature did not affect the production of antibiotics, which protect the cocoons of the European beewolf from attack by symbiotic Streptomyces (Koehler and Kaltenpoth, 2013). However, protection of pea aphids from attack by parasitic wasps, which is mediated by Hamiltonella defensa, was found to be thermally sensitive (Guay et al., 2009). Because they are ectothermic and threatened globally by a newly-emerged fungal pathogen, Batrachochytrium dendrobatidis (hereafter Bd), amphibians are another potentially fruitful animal study system for investigating the effects of temperature on microbial symbioses.
The symbiotic bacteria on amphibian skin are known to be important in defense against the pathogenic fungus Batrachochytrium dendrobatidis (hereafter Bd; Harris et al., 2009; Woodhams et al., 2015), which causes the disease chytridiomycosis that has been linked to declines and even extinctions of amphibian species on several continents (Berger et al., 1998; Martel et al., 2013; Scheele et al., 2020). Bd is a microscopic chytrid fungus that attacks amphibian skin, feeding on keratin and producing motile zoospores that can be transmitted by contact between infected individuals or through contaminated water or substrate (Longcore et al., 1999; Kolby et al., 2015). Host mortality occurs when infections cause an imbalance of ions across amphibian skin resulting in cardiac arrest (Voyles et al., 2009). Bd-related population declines have been correlated with differences in climate and the seasonality of temperature and humidity (Berger et al., 2004; Kriger and Hero, 2006, 2007; Bishop et al., 2009; Rohr and Raffel, 2010), with declines and extinctions of amphibian hosts more likely to occur in cooler, wetter, less thermally variable areas (Berger et al., 2016).
Variation in susceptibility to chytridiomycosis exists both within and among amphibian species (Tobler and Schmidt, 2010; Martel et al., 2014) and may be caused by several different factors. Differences in virulence among strains of Bd appear to contribute to the global pattern of chytridiomycosis-related declines (O’Hanlon et al., 2018; Byrne et al., 2019). Bd strains can differ in their pathogenicity (Carey et al., 2006; Retallick and Miera, 2007), however, differences in virulence may also come from the host side of this interaction. For example, amphibian host species differ dramatically in the pathogen loads required to trigger clinical symptoms of disease (i.e., pathogen tolerance; Berger et al., 2004; Reeder et al., 2012; Rollins-Smith, 2020).
Amphibians have well-developed immune systems with several defenses that can be effective in combating Bd. One important component of the innate amphibian defense against chytridiomycosis is the secretion of antimicrobial peptides (AMPs), which are synthesized in glands in the skin of amphibians and secreted into the mucus. AMPs, part of the innate immune response, have been shown to inhibit the growth of Bd (Rollins-Smith et al., 2006; Ramsey et al., 2010); however, not all amphibian species produce AMPs and not all AMPs inhibit Bd growth (Conlon, 2011). Amphibians can also deposit antibodies in their mucus, which may contribute to defense against Bd (Ramsey et al., 2010). Acquired immunological resistance to Bd has also been observed (McMahon et al., 2014). However, Bd can also fight back and suppress the acquired immune system by releasing factors that inhibit lymphocyte responses both in vitro and in vivo (Fites et al., 2014; Rollins-Smith et al., 2015).
The microbiome on amphibian skin also appears to be important in defense against Bd (Harris et al., 2006, 2009; Flechas et al., 2012; Bell et al., 2013). The mucus on amphibian skin harbors a diverse community of microbes, and evidence suggests that these microbial communities tend to be species-specific (McKenzie et al., 2012). It has been suggested that AMPs may even play a secondary, supplemental role to bacteria in amphibians’ defense systems (Conlon, 2011), but they can also work synergistically with inhibitory bacteria (Myers et al., 2012). The presence of anti-Bd bacteria in skin mucus can increase host survival after infection with Bd, and this effect appears to be mediated by bacterial production of antifungal metabolites (Harris et al., 2009). For example, violacein, a substance produced by the bacterium Janthinobacterium lividum, has been shown to inhibit the growth of Bd (Becker et al., 2009; Harris et al., 2009). In some cases, a few individual bacterial strains within the cutaneous microbiomes of some amphibian hosts may exhibit broad-spectrum inhibition of Bd growth, but the bacterial community composition as a whole can also be a predictor of an animal’s ability to survive infection (Antwis et al., 2015; Becker et al., 2015a,b; Woodhams et al., 2015). This may be due to additive and synergistic effects of multiple bacterial strains within the community (Loudon et al., 2014), although it has also been noted that Bd infection can alter cutaneous bacterial communities as well (Jani and Briggs, 2014).
The optimal environmental conditions for antifungal metabolite production by beneficial amphibian skin bacteria are unknown, yet previous studies have found that temperature does play a role in the production of bacterial products in general (e.g., folate; Kariluoto et al., 2010). Temperature has also been shown to interact with the composition of the amphibian skin microbial community to impact the ability of amphibian hosts to tolerate Bd infections (Robak and Richards-Zawacki, 2018). If temperature can affect the production of key antifungal products by cutaneous bacteria, knowing this may help to explain the timing and locations of disease-related declines in amphibians. In areas and seasons, where environmental conditions are less conducive to the production of these antifungal products, amphibians may be more susceptible to Bd infection and chytridiomycosis and thus, in greater need of human-mediated disease management interventions.
Previous studies have demonstrated the presence of anti-Bd bacteria on amphibian skin, but few have addressed the effects of environmental variables on the ability of those bacteria to inhibit the growth of Batrachochytrium. Daskin et al. (2014) tested whether growth temperature affects the antifungal properties of cutaneous bacteria isolated from the skins of three species of rainforest tree frogs (Litoria nannotis, L. rheocola, and L. serrata). They found that bacteria grown under cooler temperatures produced fewer antifungal products and were less effective at inhibiting the growth of Bd than when these same bacteria were grown at higher temperatures; however, these products were only tested for their effectiveness against Bd at 23°C, a temperature at which Bd grows very well in culture (Piotrowski et al., 2004). If Bd was challenged to grow in the presence of these low-temperature bacterial products away from Bd’s optimal growth temperature (e.g., at the temperature lower temperature they were cultured in), their effectiveness against Bd may have been different.
The objective of the present study was to examine not only whether temperature affects the production of anti-Bd products by amphibian skin bacteria, but also to ask whether temperature modulates the effectiveness of these antifungal products against Bd. Specifically, we tested the hypotheses that (1) extracts from cutaneous bacteria cultured at different temperatures differ in their ability to inhibit Bd growth, perhaps due to differences in the types and/or quantities of metabolites produced, and (2) the effectiveness of these metabolite cocktails at inhibiting Bd growth depends on the temperature at which the interaction with Bd occurs (i.e., challenge temperature). We predicted that the amphibian skin bacteria would produce more metabolites with antifungal properties near their own optimal growth temperatures, which we predict may be more similar to the frogs’ typical body temperatures than to the optimal growth temperature for Bd. We also predicted that antifungal metabolites produced by these bacteria would be more effective at inhibiting Bd growth at temperatures that are suboptimal for Bd growth in vitro than at temperatures near 23°C, which is considered optimal for growth of Bd in culture (Piotrowski et al., 2004). To test these predictions, and to better our understanding of the dynamics and role of antifungal bacteria in this host-pathogen interaction, we collected cutaneous bacteria from two North American frog species with different mean body temperatures and patterns of habitat use in the wild: Blanchard’s cricket frogs (Acris blanchardi) and Southern leopard frogs (Rana sphenocephala). We grew the bacterial isolates from each species to a common optical density under three different temperatures and then challenged Bd to grow in the presence of their metabolic products at the same three temperatures (i.e., a three-by-three fully crossed experimental design).
2. Materials and methods
2.1. Bacteria collection and isolation
We captured five adult Blanchard’s cricket frogs (Acris blanchardi) from Tulane University’s F. Edward Hebert Riverside Research Center near Belle Chasse, Louisiana (29.8852489 °N, 89.9694904 °W) and five adult southern leopard frogs (Rana sphenocephala) from Payne’s Prairie near Gainesville, Florida (29.577939 °N, 82.312629 °W). We chose these species because they are both known to be susceptible to Bd but they differ in their habitat use and thermal ecology, making our findings both ecologically relevant and also potentially informative to conservation actions. Acris blanchardi is often found at the edge of ponds and slow-moving streams and tends to avoid wooded areas and dense vegetation (Hulse et al., 2001; Gamble et al., 2008). Rana sphenocephala can be found in all types of shallow freshwater habitats and will move into moist vegetation in terrestrial habitats to feed during the summer (Wright and Wright, 1949; Conant and Collins, 1991). Based on measurements made across seasons in Central Louisiana, Acris blanchardi (N = 330) have a mean body temperature of 22.5°C and a range of 8.9 to 27.9°C and Rana sphenocephala (N = 425) have a mean temperature of 19.5°C and a range of 8.6 to 30°C (M. Ohmer, unpublished data). We chose two harvest bacteria from two host taxa because skin microbial communities are known to vary greatly from species to species (McKenzie et al., 2012) and we hypothesized that differences in the patterns we see with temperature may arise from differences in the thermal ecology of the host species.
We captured frogs by hand, wearing clean nitrile gloves and changing them between each frog, and then rinsed each frog twice with dechlorinated water to remove transient bacteria. We collected symbiotic bacteria from frog skin by swabbing the frogs’ ventral and dorsal surfaces in the field; we swabbed five times on each surface, with a single dry, sterile swab (MW113, Medical Wire and Equipment Co. LTD.). This was repeated for a total of ten swabs per frog. After swabbing, we released all frogs at their point of capture. We then transported the swabs in 2 mL microcentrifuge tubes without any media, at ambient temperature, to a sterile environment where we streaked five swabs from each frog onto 1% tryptone agar plates and the other five swabs onto R2A agar plates. The total time from swabbing to streaking was 30–60 min. We then incubated each plate at 26°C until colonies could be isolated. We chose a total of 44 bacterial isolates for use in the challenge assays based on differences in morphology, with 22 coming from each frog species. Of the 22 per species, 11 came from the R2A plates and 11 from the 1% tryptone plates. We isolated these into pure culture using standard lab technique. The isolates chosen from the R2A plates were subsequently grown on 1% tryptone plates to ensure that they could be grown in the same broth medium as the other isolates during the challenge assays. We then grew each of the selected isolates in 1 mL of 1% tryptone broth medium for 24 h in two sterile cryotubes. Afterwards, we added 1 mL of glycerol to one tube, and placed the isolate in a − 20°C freezer to preserve it as a working stock and added 0.15 mL of glycerol to the other and placed the isolate in a − 80°C freezer as a preserved culture.
2.2. Bacterial identification
We identified bacterial isolates based on their 16S rRNA gene sequence. We extracted genomic DNA from each isolate using Qiagen’s DNeasy Blood and Tissue kit, following the protocol for gram-negative bacteria. This allowed for extractions from both gram-positive and gram-negative bacteria. We used primers 8F and 1492R (Turner et al., 1999) to amplify an approximately 1.5 kbp fragment of the 16 s rRNA gene. Each polymerase chain reaction (PCR) contained 40% GoTaq Green Master Mix (Promega), 10% 10uM 8F primer, 10% 10uM 1492R primer, 10% template (extracted genomic) DNA, and 30% molecular grade water by volume in a total reaction volume of 10 μL. The PCR was run for 4 min at 94°C followed by 30 cycles of 94°C for 1 min, 53°C for 1 min, and 72°C for 90 s; and then a final extension of 72°C for 10 min. We sent PCR products to Beckman Coulter Genomics for Sanger sequencing. Forward and reverse reads were aligned in Geneious Prime to create consensus sequences for each isolate, and these were then used in a standard nucleotide BLAST against NCBIs 16S rRNA sequences database, with default search parameters to identify the closest genus or species match, where available. We also used the usearch-global tool in VSEARCH (Rognes et al., 2016) to compare our sequences to those in the antifungal isolates database of amphibian skin-associated bacteria (Woodhams et al., 2015), which was last updated with additional culture data (7,340 isolates total) by MC Bletz and DC Woodhams in June of 2020.
2.3. Bacterial preparation for assays
To prepare the bacterial growth assay, we pipetted 200 μL of autoclaved 1% tryptone broth into each well of a sterile 96-well cell culture (Costar 3,595) plates. We then inoculated the wells of each plate with 15 different bacterial isolates, using six replicates of each isolate on each plate. This left six tryptone-only wells as blanks on each plate. We incubated replicate plates at 14°C, 20°C, and 26°C until isolates reached an optical density (OD) of 0.6 on a Spectramax 190 (Molecular Devices, LLC) spectrophotometer with a 492 nm filter. These temperatures were chosen because they are within the range where Bd grows well in culture and also within the range of body temperatures measured for wild individuals of our focal amphibian hosts. Upon reaching our target density, we pipetted isolates into 2 mL collection tubes and centrifuged at 9,000 rpm for 5 min to pellet cells. We then pipetted off the supernatant and pushed it through a syringe with a sterile 0.22 μm Millipore membrane filter, leaving the bacterial extracts in the filtered supernatant to be used in the Bd growth challenge assays. These extracts were stored at −20°C prior to use. We repeated this process for all 44 morphologically distinct isolates.
2.4. Challenge assays
We cultured Bd isolate JEL 412 (provided by Joyce Longcore, University of Maine), in its ninth passage, on TGhL plates at 23°C for use in the challenge assays. We chose JEL 412, which was isolated from a dying Sachatamia ilex frog in El Cope, Panama in 2005 during the chytridiomycosis epizootic there, because it was available at a low passage number, is known to retain its pathogenicity to amphibian hosts, and falls within the BdGPL lineage (Byrne et al., 2019), which has been associated with declines and extinctions globally. We flushed Bd cultures in their seventh day of growth using TGhL broth and filtered them through a 20 μm filter to remove sporangia. We then resuspended the Bd to a working concentration of 1×106 zoospores/ml. To test for inhibition of Bd growth by the bacterial products, we added 50 μL of each bacterial extract, obtained after growing the bacteria under the three temperatures, to three replicate wells of a 96 well culture plate (Costar 3,595). Then, we added 50 μL of Bd to each well containing the extracts. Each plate also had three positive control wells, consisting of 50 μL Bd and 50 μL of 1% tryptone broth, and three negative control wells, consisting of 50 μL heat-killed Bd and 50 μL broth. We heat-killed Bd by incubating it at 60°C for 1 h. Each plate was prepared in triplicate, with one set incubated at each of three temperatures (14, 20, and 26°C). We read each plate daily on a Spectramax 190 spectrophotometer with a 492 nm filter for ten days. We also inspected each plate visually each day during the growth phase to record wells in which Bd growth inhibition had occurred and to exclude any wells in which obvious contamination had developed. Plates were kept in their respective incubators between daily readings. Extracts from all 44 visibly distinct bacterial isolates were included in this experiment.
2.5. Analyses
We compared the growth of Bd among temperature treatments and bacterial extracts using the mean optical density (OD) value on day 10 of the challenge assay, which occurred during the exponential growth phase. Wells that had visible contamination were excluded from the analyses. To compare the growth of Bd in the presence of bacterial extracts to positive controls (no extracts), we used a relative growth index. To calculate this index, we followed the method in Bell et al. (2013). We first subtracted the negative control from each sample then we subtracted the mean OD on Day 0 from the mean OD on Day 10 for each well containing a bacterial extract or positive control. We then divided this number by the corrected (day 10 minus day 0) OD of the positive controls to give a percentage of Bd growth for each well containing a bacterial extract relative to wells containing no extracts (positive controls). We then subtracted 1 from these values so that negative numbers represent the percent reduction of growth relative to the positive controls (percent inhibition) and positive numbers represent the percent of growth over and above that of the positive controls (percent growth facilitation). A value of 0 represents no difference in growth between the experimental well containing a bacterial extract and the positive Bd growth controls.
To assign bacterial isolates to categories of “inhibitory,” “no effect,” and “facilitating” of Bd growth for each challenge temperature, we used cut-off values based on proportional growth comparisons to positive Bd growth controls: bacterial extracts that consistently (in all 3 replicates) resulted in a ≥ 80% reduction in Bd growth in comparison to positive controls at a given temperature (index values ≤ −0.8) were considered inhibitory, extracts that resulted in a ≥ 20% increase in Bd growth in comparison to controls (index values ≥1.2) were considered facilitating, and extracts producing values in between these ranges were considered to have no effect on Bd growth. Wells that had index values ≥3.0, indicating growth at ≥300% of the positive control (n = 2), were excluded under the assumption that these harbored contamination that was missed in our initial visual screen.
We performed all statistical analyses using R Studio 2022 (Racine, 2012) with R version 2022.02.1 (R Core Team, 2019) and produced figures using ggplot2 version 3.4.3 (Wickham, 2011). We used DHARMa version 0.4.6 (Hartig and Hartig, 2017) and visual assessments of residuals plots to confirm that model assumptions were met. To test for differences in Bd growth index across bacterial incubation and Bd challenge temperatures, we used a linear mixed model (LMM: ‘nlme’ package version 3.1–163, function ‘lme’) (Pinheiro et al., 2023) with bacterial incubation temperature, Bd challenge assay temperature, species, and their interactions as fixed effects and plate as a random effect. We ran this model with the full set of morphologically distinct bacterial isolates as well as a reduced dataset consisting of the set of isolates that were < 99% identical in their 16 s sequences (including 1 randomly chosen isolate per group of ≥99% matching isolates).
3. Results
3.1. Sequence identification
BLAST results for the consensus sequences for each isolate are shown in Table 1. The 44 isolates that we sequenced are representatives of 13 families of bacteria (Table 1). Six of these families were found on both frog species, three were only found on A. blanchardi, and four were only found on R. sphenocephala. These 44 isolates sorted into 26 isolate groups (groups with >99% sequence similarity). Seventeen isolates with at least 1% 16 s sequence difference from one another were obtained from A. blanchardi and 14 were obtained from R. sphenocephala. Only 5 of the isolates from this shorter list were found on both frog species. The “full dataset” for subsequent statistical analysis included all 44 isolates and the “reduced” dataset included just the shorter list of 26 isolates (one chosen haphazardly from each group) that differ by >1% from all other isolate groups in their 16 s sequences.
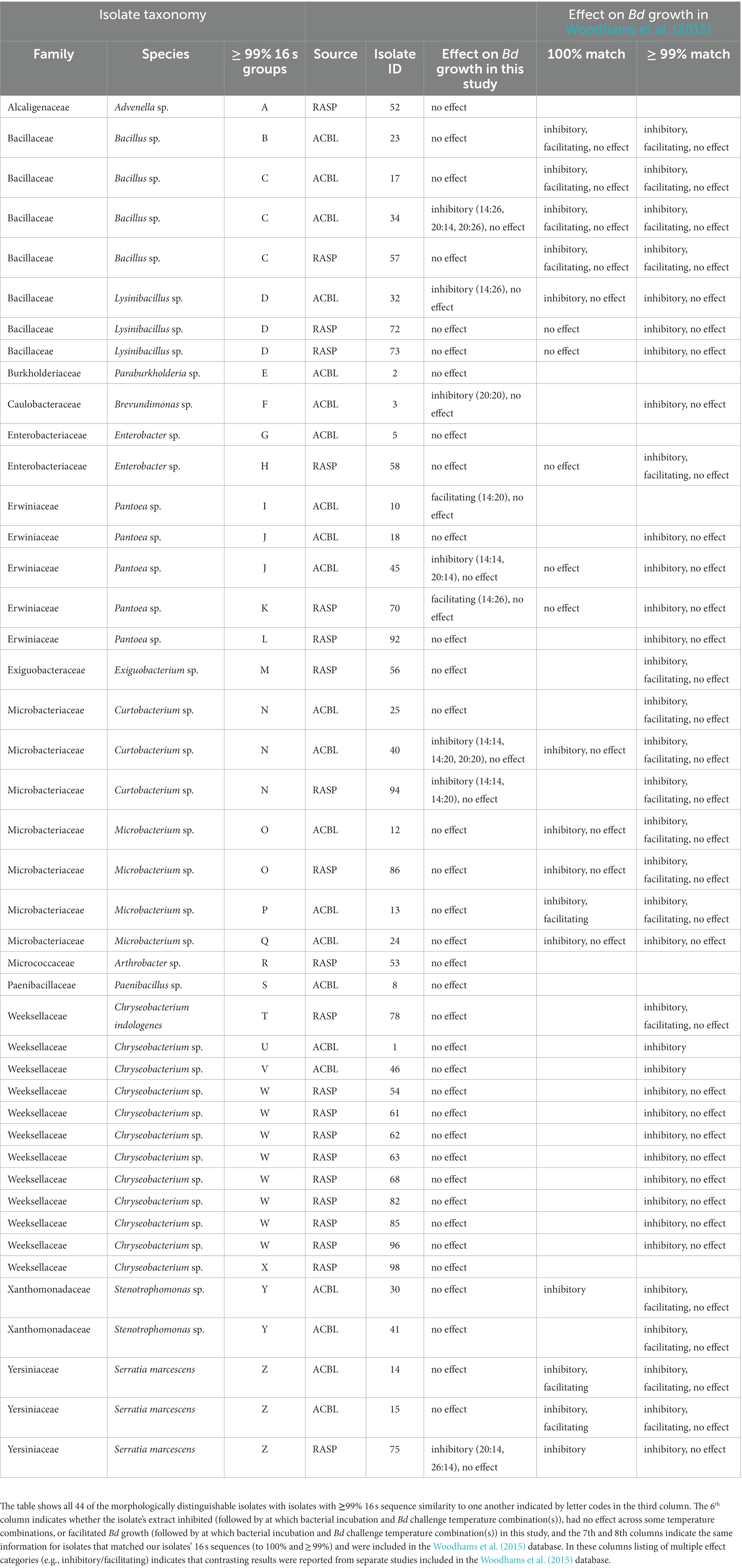
Table 1. BLAST results for sequenced bacterial isolates from A. blanchardi (ACBL) and R. sphenocephala (RASP).
3.2. Differences in relative growth
When considering the full dataset of 44 morphologically distinct bacterial isolates, we found no significant main effect of bacterial incubation temperature on Bd’s ability to grow (relative growth index) in the presence of bacterial extracts (LMM: χ2 = 1.449, p = 0. 485, Supplementary Table S1). There was, however, a significant interaction between bacterial incubation temperature and frog species (LMM: χ2 = 8.515, p = 0.014). Bacterial products from R. sphenocephala isolates inhibited Bd growth more strongly when the isolates were incubated at 14°C than at 26°C (LMM: t = 2.869, p = 0.004; Figure 1A). The temperature at which the growth challenge assay was conducted also affected Bd’s relative growth index (LMM: χ2 = 6.996, p = 0.030, Figure 1B). Bd growth was inhibited more strongly when this growth challenge assay took place at 26°C than at 14°C (LMM: t = −2.639, p = 0.009, Supplementary Table S2). None of the other two- or three-way interactions in the model were significant (LMM: χ2 ≤ 2.657, p ≥ 0.237).
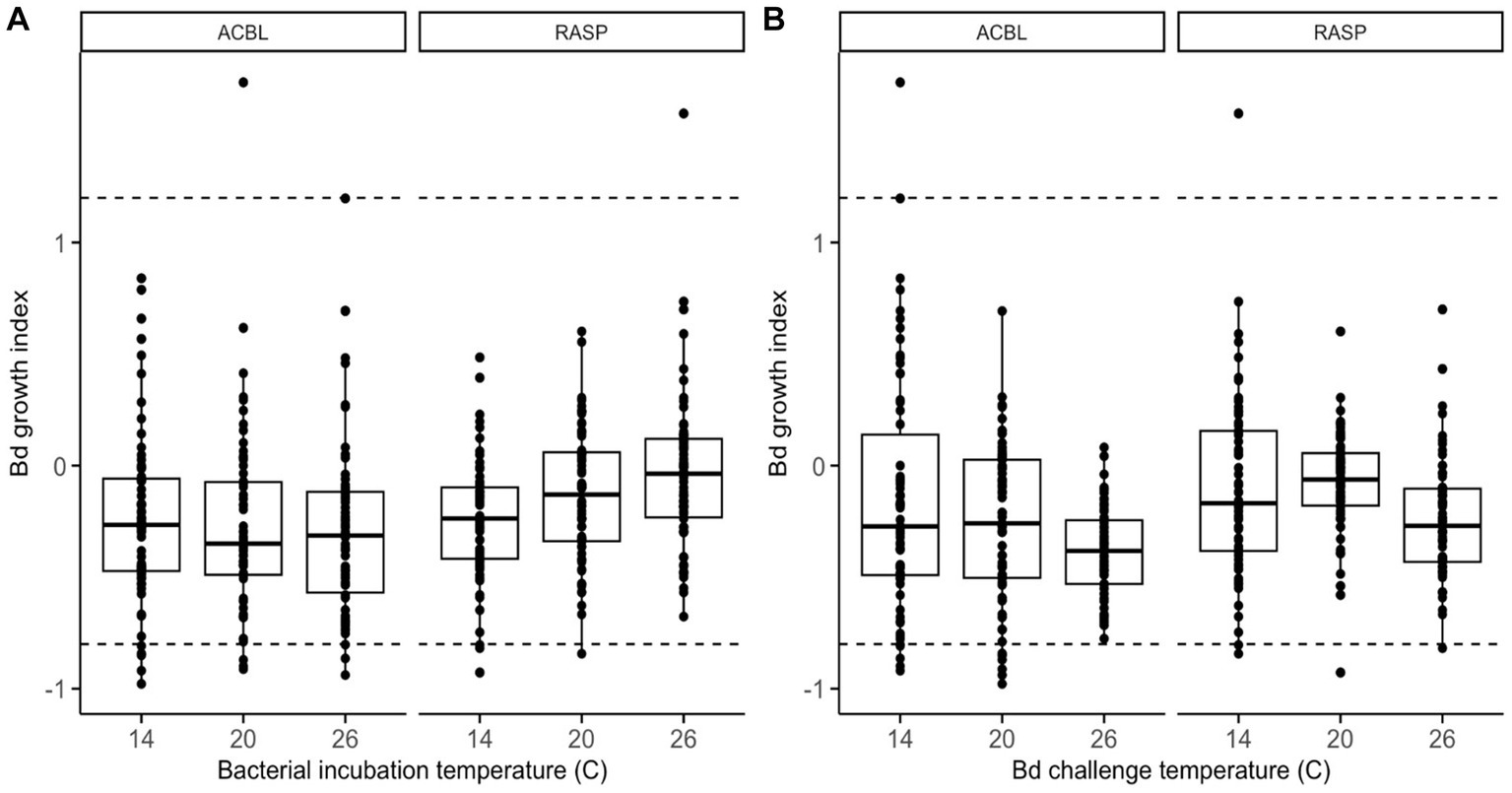
Figure 1. Relative growth of Bd, as compared to positive growth controls, when challenged to grow in the presence of frog skin bacterial extracts from two frog species (ACBL = Acris blanchardi, RASP = Rana sphenocephala) at three different temperatures. In (A), the data are organized by bacterial incubation temperature and in (B) they are organized by Bd challenge temperature. Negative values indicate inhibition of Bd growth and positive values indicate facilitation as compared to no-extract controls. Isolates with values under −0.8 (lower dashed line) were considered inhibitive and isolates with values over 1.2 (upper dashed line) were considered facilitating of Bd growth.
When considering the reduced dataset of only the set of 26 bacterial isolates with 16 s sequences that differed by >1%, we found that none of the main effects, two-, or three-way interactions in the model were significant (Supplementary Tables S3, S4 and Supplementary Figure S1). The interaction between bacterial incubation temperature and frog species was nearly significant (LMM: χ2 = 5.061, p = 0.080) and showed the same pattern as in the full dataset: bacterial products from R. sphenocephala isolates inhibited Bd growth more strongly when they had been incubated at lower temperatures.
3.3. Differences in categorical effects on Bd growth
None of the bacterial isolates were inhibitive of Bd growth across all the bacterial incubation and growth challenge assay temperature combinations (Figure 2, Table 1). Many were inhibitive only in a single combination, but a few were inhibitive in up to three of the nine combinations tested. For example, the extract from isolate 34 (Bacillus sp.) was inhibitive of Bd growth when incubated at 14°C and challenged at 26°C and when incubated at 20°C and challenged at 14°C or 26°C but not under other combinations tested. None of the bacterial extracts were inhibitive across all three bacterial incubation temperatures. Likewise, none of the extracts produced at a given bacterial incubation temperature were inhibitive across all three Bd challenge temperatures.
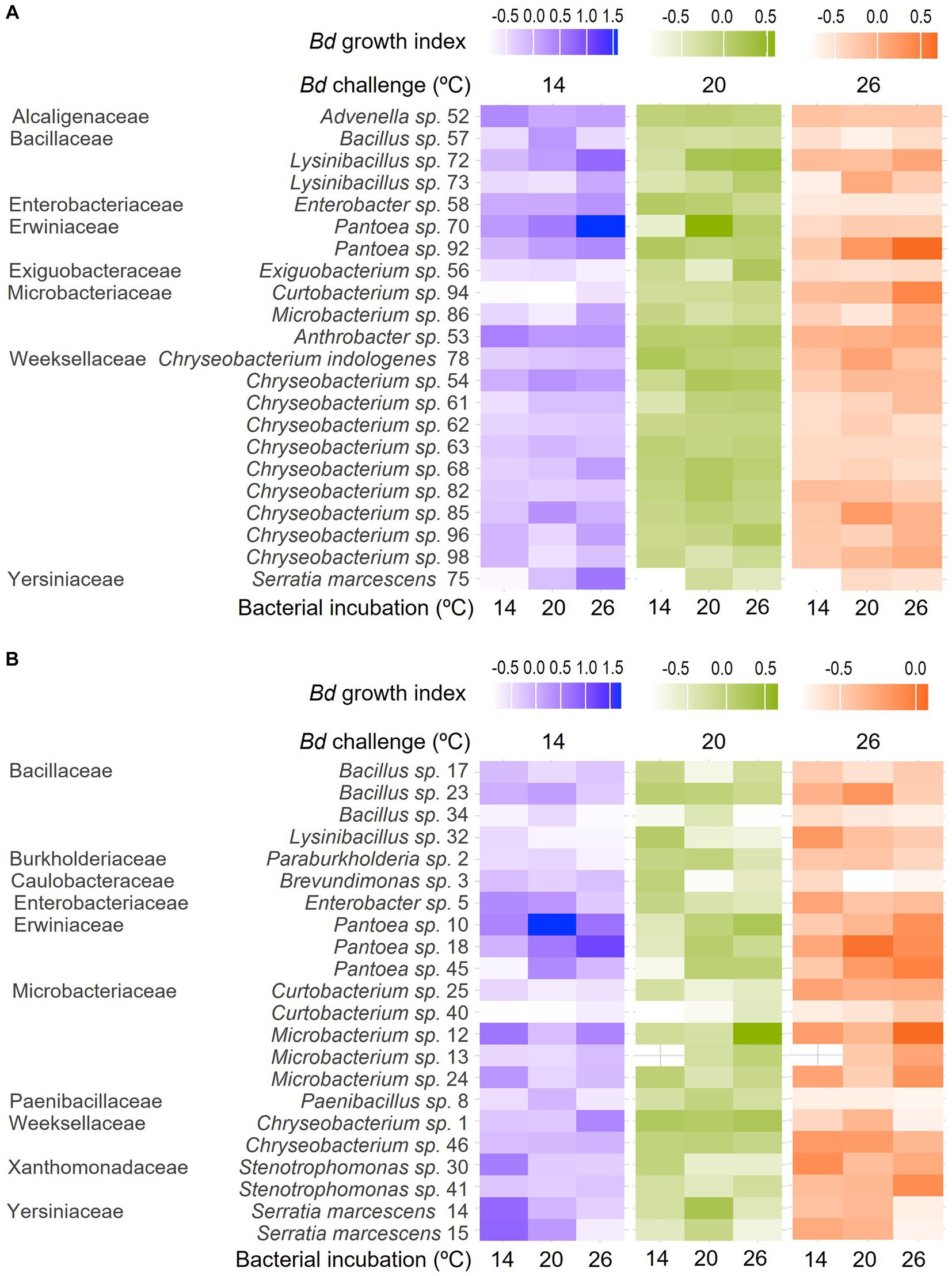
Figure 2. Heat maps showing differences in Bd growth index across bacterial incubation temperatures (individual columns) and Bd challenge temperatures (differently colored groups of columns) for bacteria isolated from the skin of (A) R. sphenocephala and (B) A. blanchardi. Lighter shading (lower Bd growth index) corresponds to more inhibition of the fungal pathogen’s growth in in vitro challenge assays. Bacterial isolates are grouped by family, genus, and isolate ID, as they also are in Table 1.
Nineteen of the 44 bacterial isolates we isolated and sequenced were a 100% sequence match to isolates in the Woodhams et al. (2015) dataset (version updated in 2020 by MC Bletz and DC Woodhams). Our categorical findings (inhibitory, facilitating, or no effect) for effects on Bd of extracts from these isolates matched with those in the database for all but six of the isolates where either we found no effect on Bd growth and the Woodhams et al. dataset showed evidence of inhibition (4 isolates) or we found evidence of facilitation (1 isolate) or inhibition (1 isolate) but the studies in the Woodhams et al. dataset found no effect. Another 18 isolates from this study were a > 99% but <100% sequence match to the Woodhams et al. dataset. For 16 of these we found no effect of their extract on Bd growth and for two we found evidence Bd inhibition under some conditions. These categorical findings agreed with those in the Woodhams et al. dataset in all but three cases where either we found no effect and the Woodhams et al. dataset contained evidence of inhibition (2 isolates) or where we found facilitation and the Woodhams et al. dataset did not (1 isolate). Seven of the isolates we identified were not found in the Woodhams et al. database (< 99% sequence match) at all. For six of these we found no effect on Bd growth and for one we found facilitation of Bd growth.
4. Discussion
Thirteen of the 15 genera and all 13 families of bacteria identified in this study have been previously found on frog skin. Arthrobacter (Micrococcaceae; Lauer et al., 2008), Bacillus (Bacillaceae; Loudon et al., 2014), Brevundimonas (Caulobacteraceae; Roth et al., 2013), Chryseobacterium (Weeksellaceae; Mauel et al., 2002), Curtobacterium (Microbacteriaceae; Becker et al., 2015a,b), Enterobacter (Enterobacteriaceae; Pasteris et al., 2009), Exiguobacterium (Exiguobacteraceae; Xu et al., 2020), Lysinibacillus (Bacillaceae; Kueng et al., 2014), Microbacterium (Microbacteriaceae; Suzina et al., 2015), Paenibacillus (Paenibacillaceae; Susilawati et al., 2021), Pantoea (Erwiniaceae; Brito de Assis et al., 2016), Serratia (Yersiniaceae; Bresciano et al., 2015), and Stenotrophomonas (Xanthomonadaceae; O’Connell et al., 2011) have all been reported from frog skin, though in some cases ours is the first report from North American frogs. Advenella (Alcaligenaceae) and Paraburkholderia (Burkholderiaceae) were found in this study but to our knowledge these genera not been reported from frog skin previously. However, Pigmentiphaga, another genus in the Alcaligenaceae, has been found on frog skin, specifically in association with arboreal frogs (Bletz et al., 2019). Four of the identified bacterial families were found on A. blanchardi only, three were found on R. sphenocephala only, and six were found on both host species (Table 1).
All 13 of the bacterial families and all 13 of the previously reported genera from this study have members that have been found before, at least under some conditions, to inhibit the growth of Bd in vitro (Woodhams et al., 2015). However, for many of the bacteria we identified the results in the Woodhams et al. (2015) dataset were mixed, suggesting that in some studies their products were found to inhibit Bd growth whereas in other studies they were not. One potential reason for this variation could have been differences in bacterial growth media among the included studies. It stands to reason that bacteria would produce different metabolites when grown on different food sources and this could have impacted the results of Bd growth challenge assays using cell free supernatants. Differences in the Bd strain used and/or its history in culture may have also contributed to the variation seen among the studies in the Woodhams et al. (2015) dataset. Another source of variation could have been differences in the temperatures at which in vitro growth challenge assays were done across the many studies in the Woodhams et al. (2015) dataset. These previous studies ranged in temperature from 15 to 26°C but the vast majority were done at “room temperature,” which was defined as 21 to 23°C. For the two new genera we report here (Advenella and Paraburkholderia) we did not find evidence of Bd inhibition at any temperature. However, for many of the previously reported bacteria we also isolated, despite the fact that we used a consistent bacterial growth medium and Bd strain, we found that whether a bacterial isolate’s extracts facilitated, inhibited, or had no effect on Bd differed among our temperature treatments. In fact, none of the isolates from this study inhibited Bd growth across all the temperature treatments we tried, and most were inhibitive at only one or a few of temperature treatments.
Although temperature has been found to affect Bd growth both in culture and on amphibian skin, this study was the first to consider the effects of temperature on both the production of anti-Bd metabolites by cutaneous bacteria and the ability of those metabolites to inhibit Bd growth. Standardizing the optical density (OD) of bacteria and their products used in the growth challenge assay also permitted us to disentangle effects of temperature on bacterial growth from effects on the anti-fungal properties of bacterial supernatants. We predicted that the bacteria we isolated from amphibian skin would produce more metabolites with anti-Bd properties near their own optimal growth temperatures, which we did not measure but predicted would be similar to the typical body temperatures of their hosts (mean of 19.5°C for R. sphenocephala and 22.5°C for A. blanchardi). Thus, we predicted that Bd inhibition would be greater at lower bacterial incubation temperatures for isolates collected from R. sphenocephala than for those collected from A. blanchardi. We also predicted that antifungal metabolites produced by these bacteria would be more effective at inhibiting Bd growth at temperatures that are suboptimal for Bd growth in vitro, i.e., at temperatures away from 23°C, which is considered optimal for growth of Bd in culture (Piotrowski et al., 2004). Thus, we predicted that Bd growth inhibition would be greater at 14 and 26 than at 23°C.
The pattern we observed among the bacterial incubation temperatures does support the prediction that R. sphenocephala isolates are better at inhibiting Bd growth when incubated at lower temperatures. However, Bd growth was lowest when these isolates were incubated at 14°C whereas the mean body temperature for R. sphenocephala in the southern part of its range, where our collections took place, is closer to our 20°C treatment temperature. There was also no clear pattern with bacterial incubation temperature for isolates collected from A. blanchardi. Taken together, these data suggest that the temperature at which bacterial isolates are grown can, in fact, influence the ability of their metabolites to inhibit Bd growth, though the temperature at which metabolites are most effective may not be closely matched to the thermal ecology of the amphibian host species. It stands to reason that the thermal physiology of the bacteria themselves is an important driver of variation in metabolite production with temperature. However, predicting which temperatures may be optimal for antifungal metabolite production given the suite of bacteria that produce them on amphibian skin, may be beyond our reach without a more detailed understanding of the biology of these bacterial taxa. Future work characterizing differences in metabolites produced across culture temperatures and among bacterial taxa is needed to clarify the mechanisms generating temperature-driven differences in the antimicrobial properties of symbiotic microbes in general, and in frog skin microbes in particular.
Bd has been shown to grow in culture between the temperatures of 4°C and 28°C, but the optimal range for growth in vitro is between 17°C and 25°C (Piotrowski et al., 2004). Our 14°C and 26°C challenge temperature treatments were chosen to be within the range for Bd growth, but outside the optimal growth range. Our second prediction, that Bd growth would be inhibited more by bacterial metabolites when challenged away from its optimal growth temperature, was partially supported. Bd growth index was lower when the challenge temperature was 26°C, a temperature near the upper limit for growth of this pathogen. However, we did not see the same pattern below the optimal growth temperature range. There was no difference in Bd growth index between challenge temperatures of 14 and 20°C. An explanation for this pattern may come from the asymmetrical nature of thermal performance curves. Across many taxa, these curves generally have a steeper decline from the thermal optimum to the critical thermal maximum (CTmax) than they do from the thermal optimum to the critical thermal minimum (CTmin), giving them a “left skewed” appearance (Schulte et al., 2011). As thermal performance curves describe the effects of temperature on biological rate processes, including processes like growth, fecundity, metabolic rate and enzyme activity, it stands to reason that Bd growth might be more easily inhibited by bacterial metabolites at temperatures near CTmax, where these processes begin to fail. Our lower temperature of 14°C is still quite far from Bd’s CTmin, which could explain the lack of a difference in Bd growth index between challenge assays done at this temperature vs. 20°C, which is near the thermal optimum. Given that both amphibians and their Batrachochytrium pathogens experience a range of environmental conditions in the wild, insights like these, that help to clarify our understanding of the impacts of temperature on mechanisms of host defense, are needed to predict susceptibility to chytridiomycosis.
Because we were interested in knowing whether the members of the amphibian skin microbiome produce their antifungal metabolites better or faster at certain temperatures, we chose to grow all bacterial isolates to a set optical density (OD) before removing bacteria and using the supernatants in our Bd growth challenge assays. Our results do, in fact, suggest that temperature can alter the antifungal properties of bacterial supernatants independent of their density in culture, as we saw an effect of bacterial incubation temperature on Bd growth inhibition in the skin microbes from R. sphenocephala. Another approach, and the one taken by Daskin et al. (2014), would have been to grow each bacterial isolate for sufficient time to see a plateau in OD before harvesting extracts for Bd growth challenge assays. With this approach Daskin et al. (2014) also found an effect of incubation temperature on the ability of bacterial extracts to inhibit Bd growth though their findings went in a different direction than our own. Focusing just on isolates that were known to be Bd-inhibitory, they found that Bd growth inhibition by bacterial metabolites was reduced at low temperatures whereas our findings show a trend in the opposite direction. Because the bacteria in Daskin et al. (2014) were not grown to a common density, the differences they found with temperature could stem from differences in bacterial densities more so than differences in the types or quantities of antifungal metabolites produced across incubation temperatures. In other words, the metabolite cocktails from cultures grown under lower temperatures may have been less effective at inhibiting the growth of Bd simply because at low temperatures there were fewer bacteria present to produce those metabolites. This key methodological difference could explain the difference in results between the Daskin et al. (2014) study and our own. An alternative explanation is that the antifungal activity of bacterial communities on the Australian rainforest treefrogs sampled by Daskin et al. (2014) shows a different relationship with temperature than that of the bacterial communities of the subtropical North American pond frogs sampled in this study. Future work comparing the effects of temperature on the antimicrobial properties of amphibian skin microbes across a greater range of host ecologies is needed to tease apart these alternatives.
Our use of 1% tryptone broth, a growth environment that is undoubtedly quite different from amphibian skin, may also have affected what metabolites were produced by the bacterial isolates in this study. Valerio et al. (2016) found that manipulating the growth medium of lactic acid bacteria by adding phenylpyruvic acid improved their antifungal activity. The bacteria utilized in our study may have been able to produce more or different antifungal compounds across temperatures in other growth environments, for example while on the skin of frogs, but not when grown on low nutrient agar. Because our growth challenge assay used only bacterial supernatants, this study also did not address whether and how quickly amphibian skin bacteria would produce antifungal metabolites when grown in the presence of Bd and how that might vary with temperature.
Although it is generally accepted that temperature plays a large role in the dynamics of chytridiomycosis infections, this study helps to clarify some of the mechanisms that may be driving this relationship. While amphibian immune systems in general are thought to function more effectively against Bd at higher temperatures (Rollins-Smith et al., 2011), our findings, the Woodhams et al. (2015) database, and the Daskin et al. (2014) study all suggest that temperature also modulates anti-fungal properties of amphibian skin bacterial communities, which are an important first-line defense against Bd and other skin pathogens. While among the many studies in the Woodhams et al. (2015) database there could be many sources of variation, our results and the results of Daskin et al. (2014) suggest that this variation could be due to differences in bacterial titer or density, the production of different metabolites across temperatures, or possibly both of these factors. A similar effect on bacterial titer to that shown in Daskin et al. (2014) has been seen to affect the benefits that inherited microbial symbionts provide to their hosts (rev. in Corbin et al., 2017). If there is a minimum concentration of metabolites necessary to inhibit Bd growth, it seems plausible that Bd-inhibitory bacteria will produce the necessary amount of metabolites faster when grown at their own optimal temperature than when grown below or above that temperature. However, our understanding of optimal temperatures for growth and antifungal metabolite production in amphibian skin microbes, and in fact in symbiotic bacteria more broadly, remains in its infancy. Filling this gap in our understanding of microbial interactions will be necessary in order to predict the effects of changes in temperature, both natural and anthropogenic, on the symbiotic relationships that animals depend on for a range of functions, from digestion to protection from pathogens.
Data availability statement
Sequence data generated for this project is available on GenBank (Accession Nos. OR228691 - OR228864). The raw data supporting the conclusions of this article will be made available by the authors, without undue reservation.
Ethics statement
The animal study was approved by Tulane University Institutional Animal Care and Use Committee (IACUC). The study was conducted in accordance with the local legislation and institutional requirements.
Author contributions
MR: Conceptualization, Data curation, Investigation, Methodology, Writing – original draft, Writing – review & editing. VS: Formal analysis, Validation, Visualization, Writing – review & editing. EC: Formal analysis, Visualization, Writing – review & editing. CR-Z: Conceptualization, Data curation, Formal analysis, Funding acquisition, Investigation, Methodology, Project administration, Resources, Supervision, Validation, Visualization, Writing – review & editing.
Funding
This work was funded by grants from the National Science Foundation (Award No. 1649443) and Louisiana Board of Regents [Award No. LEQSF (2011-14)-RD-A-26] to CR-Z.
Acknowledgments
We would like to thank Molly Bletz for assistance with cross-checking the microbial sequences and taxa that we found in this study with the updated (in June 2020) version of the Woodhams et al. (2015) database. We would also like to thank Louise Rollins-Smith, David Heins, and Michael Blum for their feedback on an earlier draft of the manuscript.
Conflict of interest
The authors declare that the research was conducted in the absence of any commercial or financial relationships that could be construed as a potential conflict of interest.
Publisher’s note
All claims expressed in this article are solely those of the authors and do not necessarily represent those of their affiliated organizations, or those of the publisher, the editors and the reviewers. Any product that may be evaluated in this article, or claim that may be made by its manufacturer, is not guaranteed or endorsed by the publisher.
Supplementary material
The Supplementary material for this article can be found online at: https://www.frontiersin.org/articles/10.3389/fmicb.2023.1253482/full#supplementary-material
References
Antwis, R. E., Preziosi, R. F., Harrison, X. A., and Garner, T. W. J. (2015). Amphibian symbiotic bacteria do not show a universal ability to inhibit growth of the global panzootic lineage of Batrachochytrium dendrobatidis. Appl Experimental Microbiol 81, 3706–3711. doi: 10.1128/AEM.00010-15
Becker, M. H., Brucker, R. M., Schwantes, C. R., Harris, R. N., and Minbiole, K. P. C. (2009). The bacterially produced metabolite violacein is associated with survival of amphibians infected with a lethal fungus. Appl. Environ. Microbiol. 75, 6635–6638. doi: 10.1128/AEM.01294-09
Becker, M. H., Walke, J. B., Cikanek, S., Savage, A. E., Mattheus, N., Santiago, C. N., et al. (2015a). Composition of symbiotic bacteria predicts survival in Panamanian golden frogs infected with a lethal fungus. Proc Biol Sci 282:20142881. doi: 10.1098/rspb.2014.2881
Becker, M. H., Walke, J. B., Murrill, L., Woodhams, D. C., Reinert, L. K., Rollins-Smith, L. A., et al. (2015b). Phylogenetic distribution of symbiotic bacteria from Panamanian amphibians that inhibit growth of the lethal fungal pathogen Batrachochytrium dendrobatidis. Mol. Ecol. 24, 1628–1641. doi: 10.1111/mec.13135
Bell, S. C., Alford, R. A., Garland, S., Padilla, G., and Thomas, A. D. (2013). Screening bacterial metabolites for inhibitory effects against Batrachochytrium dendrobatidis using a spectrophotometric assay. Dis. Aquat. Org. 103, 77–85. doi: 10.3354/dao02560
Berger, L., Roberts, A. A., Voyles, J., Longcore, J. E., Murray, K. A., and Skerratt, L. F. (2016). History and recent progress on chytridiomycosis in amphibians. Fungal Ecol. 19, 89–99. doi: 10.1016/j.funeco.2015.09.007
Berger, L., Speare, R., Daszak, P., Green, D. E., Cunningham, A. A., Goggin, C. L., et al. (1998). Chytridiomycosis causes amphibian mortality associated with population declines in the rain forests of Australia and Central America. Proc. Natl. Acad. Sci. U. S. A. 95, 9031–9036. doi: 10.1073/pnas.95.15.9031
Berger, L., Speare, R., Hines, H. B., Marantelli, G., Hyatt, A. D., McDonald, K. R., et al. (2004). Effect of season and temperature on mortality in amphibians due to chytridiomycosis. Aust. Vet. J. 82, 434–439. doi: 10.1111/j.1751-0813.2004.tb11137.x
Bishop, P. J., Speare, R., Poulter, R., Butler, M., Speare, B. J., Hyatt, A., et al. (2009). Elimination of the amphibian chytrid fungus Batrachochytrium dendrobatidis by Archey’s frog Leiopelma archeyi. Dis. Aquat. Org. 84, 9–15. doi: 10.3354/dao02028
Bletz, M. C., Bunk, B., Spröer, C., Biwer, P., Reiter, S., Rabemananjara, F. C., et al. (2019). Amphibian skin-associated Pigmentiphaga: genome sequence and occurrence across geography and hosts. PLoS One 14:e0223747. doi: 10.1371/journal.pone.0223747
Bresciano, J. C., Salvador, C. A., Paz-y-Mino, C., Parody-Merino, A. M., Bosch, J., and Woodhams, D. C. (2015). Variation in the presence of anti-Batrachochytrium dendrobatidis bacteria of amphibians across life stages and elevations in Ecuador. EcoHealth 12, 310–319. doi: 10.1007/s10393-015-1010-y
Brito de Assis, A., Dos Santos, C., Dutra, F. P., de Oliveira Motta, A., Costa, F. S., Navas, C. A., et al. (2016). Assessing antibacterial potential of components of Phyllomedusa distincta skin and its associated dermal microbiota. J. Chem. Ecol. 42, 139–148. doi: 10.1007/s10886-016-0665-3
Brune, A., and Ohkuma, M. (2010). “Role of the termite gut microbiota in symbiotic digestion” in Biology of termites: A modern synthesis. eds. D. Bignell, Y. Roisin, and N. Lo (Dordrecht: Springer)
Byrne, A. Q., Vredenburg, V. T., Martel, A., Pasmans, F., Bell, R. C., Blackburn, D. C., et al. (2019). Cryptic diversity of a widespread global pathogen reveals expanded threats to amphibian conservation. Proc. Natl. Acad. Sci. U. S. A. 116, 20382–20387. doi: 10.1073/pnas.1908289116
Carey, C., Bruzgal, J. E., Livo, L. J., Walling, M. L., Kuehl, K. A., Dixon, B. F., et al. (2006). Experimental exposures of boreal toads (Bufo boreas) to a pathogenic chytrid fungus (Batrachochytrium dendrobatidis). EcoHealth 3, 5–21. doi: 10.1007/PL00021734
Carey, H. V., and Duddleston, K. N. (2014). Animal-microbial symbioses in changing environments. J. Therm. Biol. 44, 78–84. doi: 10.1016/j.jtherbio.2014.02.015
Chow, J., Lee, S. M., Shen, Y., Khosravi, A., and Mazmanian, S. K. (2010). Host–bacterial symbiosis in health and disease. Adv. Immunol. 107, 243–274. doi: 10.1016/B978-0-12-381300-8.00008-3
Conant, R., and Collins, J. T. (1991). A field guide to reptiles and amphibians: Eastern and Central North America. Houghton Mifflin Company, Boston, Massachusetts.
Conlon, J. M. (2011). The contribution of skin antimicrobial peptides to the system of innate immunity in anurans. Cell Tissue Res. 343, 201–212. doi: 10.1007/s00441-010-1014-4
Corbin, C., Heyworth, E. R., Ferrari, J., and Hurst, G. D. D. (2017). Heritable symbionts in a world of varying temperature. Heredity 118, 10–20. doi: 10.1038/hdy.2016.71
Daskin, J. H., Bell, S. C., Schwarzkopf, L., and Alford, R. A. (2014). Cool temperatures reduce antifungal activity of symbiotic bacteria of threatened amphibians—implications for disease management and patterns of decline. PLoS One 9:e100378. doi: 10.1371/journal.pone.0100378
Erwin, P. M., Pita, L., Lopez-Legentil, S., and Turon, X. (2012). Stability of sponge-associated bacteria over large seasonal shifts in temperature and irradiance. Appl. Environ. Microbiol. 78, 7358–7368. doi: 10.1128/AEM.02035-12
Fan, L., Liu, M., Simister, R., Webster, N. S., and Thomas, T. (2013). Marine microbial symbiosis heats up: the phylogenetic and functional response of a sponge holobiont to thermal stress. ISME J. 7, 991–1002. doi: 10.1038/ismej.2012.165
Fites, J. S., Reinert, L. K., Chappell, T. M., and Rollins-Smith, L. A. (2014). Inhibition of local immune responses by the frog-killing fungus Batrachochytrium dendrobatidis. Infect. Immun. 82, 4698–4706. doi: 10.1128/IAI.02231-14
Flechas, S. V., Sarmiento, C., Cardenas, M. E., Medina, E. M., Restrepo, S., and Amezquita, A. (2012). Surviving chytridiomycosis: differential anti-Batrachochytrium dendrobatidis activity in bacterial isolates from three lowland species of Atelopus. PLoS One 7:e44832. doi: 10.1371/journal.pone.0044832
Gamble, T., Berendzen, P. B., Shaffer, H. B., Starkey, D. E., and Simons, A. M. (2008). Species limits and phylogeography of north American cricket frogs (Acris: Hylidae). Mol. Phylogenet. Evol. 48, 112–125. doi: 10.1016/j.ympev.2008.03.015
Guay, J. -F., Boudreault, S., Michaud, D., and Cloutier, C. (2009). Impact of environmental stress on aphid clonal resistance to parasitoids: role of Hamiltonella defensa bacterial symbiosis in association with a new facultative symbiont of the pea aphid. J. Insect Physiol. 55, 919–926. doi: 10.1016/j.jinsphys.2009.06.006
Harris, R. N., Brucker, R. M., Walke, J. B., Becker, M. H., Schwantes, C. R., Flaherty, D. C., et al. (2009). Skin microbes on frogs prevent morbidity and mortality caused by a lethal skin fungus. ISME J. 3, 818–824. doi: 10.1038/ismej.2009.27
Harris, R. N., James, T. Y., Lauer, A., Simon, M. A., and Patel, A. (2006). Amphibian pathogen Batrachochytrium dendrobatidis is inhibited by the cutaneous bacteria of amphibian species. EcoHealth 3, 53–56. doi: 10.1007/s10393-005-0009-1
Hartig, F., and Hartig, M. F. (2017). Package ‘DHARMa’. R package. Available at: http://florianhartig.github.io/DHARMa/.
Hulse, A. C., McCoy, C. J., and Censky, E. J. (2001). Amphibians and reptiles of Pennsylvania and the northeast. Comstock Publishing Associates. NY.
Jani, A. J., and Briggs, C. J. (2014). The pathogen Batrachochytrium dendrobatidis disturbs the frog skin microbiome during a natural epidemic and experimental infection. Proc. Natl. Acad. Sci. U. S. A. 111, E5049–E5058. doi: 10.1073/pnas.1412752111
Jansson, J. K., and Taş, N. (2014). The microbial ecology of permafrost. Nat. Rev. Microbiol. 12, 414–425. doi: 10.1038/nrmicro3262
Kariluoto, S., Edelmann, M., Herranen, M., Lampi, A., Shmelev, A., Salovaara, H., et al. (2010). Production of folate by bacteria from oat bran. Int. J. Food Microbiol. 143, 41–47. doi: 10.1016/j.ijfoodmicro.2010.07.026
Koehler, S., and Kaltenpoth, M. (2013). Maternal and environmental effects on symbiont mediated antimicrobial defense. J. Chem. Ecol. 39, 978–988. doi: 10.1007/s10886-013-0304-1
Kolby, J. E., Ramirez, S. D., Berger, L., Richards-Hrdlicka, K. L., Jocque, M., and Skerratt, L. F. (2015). Terrestrial dispersal and potential environmental transmission of the amphibian chytrid fungus (Batrachochytrium dendrobatidis). PLoS One 10:e0125386. doi: 10.1371/journal.pone.0125386
Kriger, K. M., and Hero, J. M. (2006). Survivorship in wild frogs infected with chytridiomycosis. EcoHealth 3, 171–177. doi: 10.1007/s10393-006-0027-7
Kriger, K. M., and Hero, J. M. (2007). Large-scale seasonal variation in the prevalence and severity of chytridiomycosis. J. Zool. 271, 352–359. doi: 10.1111/j.1469-7998.2006.00220.x
Kueng, D., Bigler, L., Davis, L. R., Gratwicke, B., Griffith, E., and Woodhams, D. C. (2014). Stability of microbiota facilitated by host immune regulation: informing probiotic strategies to manage amphibian disease. PLoS One 9:e87101. doi: 10.1371/journal.pone.0087101
Lauer, A., Simon, M. A., Banning, J. L., Lam, B. A., and Harris, R. N. (2008). Diversity of cutaneous bacteria with antifungal activity isolated from female four-toed salamanders. ISME J. 2, 145–157. doi: 10.1038/ismej.2007.110
Longcore, J. E., Pessier, A. P., and Nichols, D. K. (1999). Batrachochytrium dendrobatidis gen. Et sp. nov., a chytrid pathogenic to amphibians. Mycologia 91, 219–227. doi: 10.1080/00275514.1999.12061011
Loudon, A. H., Holland, J. A., Umile, T. P., Burzynski, E. A., Minbiole, K. P. C., and Harris, R. N. (2014). Interactions between amphibians’ symbiotic bacteria cause the production of emergent anti-fungal metabolites. Front. Microbiol. 5:441. doi: 10.3389/fmicb.2014.00441
Martel, A., Blooi, M., Adriaensen, C., Van Rooij, P., Beukema, W., Fisher, M. C., et al. (2014). Recent introduction of a chytrid fungus endangers Western Palearctic salamanders. Science 346, 630–631. doi: 10.1126/science.1258268
Martel, A., Spitzen-van der Slujis, A., Blooi, M., Bert, W., Ducatell, R., Fisher, M. C., et al. (2013). Batrachochytrium salamandrivorans sp nov causes lethal chytridiomycosis in amphibians. Proc. Natl. Acad. Sci. U. S. A. 110, 15325–15329. doi: 10.1073/pnas.1307356110
Mauel, M. J., Miller, D. L., Frazier, K. S., and Hines, M. E. (2002). Bacterial pathogens isolated from cultured bullfrogs (Rana catesbeiana). J. Vet. Diagn. Investig. 14, 431–433. doi: 10.1177/104063870201400515
McKenzie, V. J., Bowers, R. M., Fierer, N., Knight, R., and Lauber, C. L. (2012). Co-habiting amphibian species harbor unique skin bacterial communities in wild populations. ISME J. 6, 588–596. doi: 10.1038/ismej.2011.129
McMahon, T. A., Sears, B. F., Venesky, M. D., Bessler, S. M., Brown, J. M., Deutsch, K., et al. (2014). Amphibians acquire resistance to live and dead fungus overcoming fungal immunosuppression. Nature 511, 224–227. doi: 10.1038/nature13491
Myers, J. M., Ramsey, J. P., Blackman, A. L., Nichols, A. E., Minbiole, K. P. C., and Harris, R. N. (2012). Synergistic inhibition of the lethal fungal pathogen Batrachochytrium dendrobatidis: the combined effect of symbiotic bacterial metabolites and antimicrobial peptides of the frog Rana muscosa. J. Chem. Ecol. 38, 958–965. doi: 10.1007/s10886-012-0170-2
O’Connell, D., Mruk, K., Rocheleau, J. M., and Kobertz, W. R. (2011). Xenopus laevis oocytes infected with multi-drug-resistant bacteria: implications for electrical recordings. J. Gen. Physiol. 138, 271–277. doi: 10.1085/jgp.201110661
O’Hanlon, S. J., Rieux, A., Farrer, R. A., Rosa, G. M., Waldman, B., Bataille, A., et al. (2018). Recent Asian origin of chytrid fungi causing global amphibian declines. Science 360, 621–627. doi: 10.1126/science.aar1965
Pasteris, S. E., Babot, G. R., Otero, M. C., Buhler, M. I., and Nader-Macias, M. E. (2009). Beneficial properties of lactic acid bacteria isolated from a Rana catesbeiana hatchery. Aquac. Res. 40, 1605–1615. doi: 10.1111/j.1365-2109.2009.02261.x
Petersen, J. M., Zielinski, F. U., Pape, T., Seifet, R., Moraru, C., Amann, R., et al. (2011). Hydrogen is an energy source for hydrothermal vent symbioses. Nature 476, 176–180. doi: 10.1038/nature10325
Pinheiro, J., and Bates, D., R Core Team (2023). Nlme: linear and nonlinear mixed effects models. R package version 3, 1–162. Available at: https://CRAN.R-project.org/package=nlme
Piotrowski, J. S., Annis, S. L., and Longcore, J. E. (2004). Physiology of Batrachochytrium dendrobatidis, a chytrid pathogen of amphibians. Mycologia 96, 9–15. doi: 10.1080/15572536.2005.11832990
Piquet, A. M. T., Maat, D. S., Confurius-Guns, V., Sintes, E., Herndl, G. J., Van De Poll, W. H., et al. (2016). Springtime dynamics, productivity and activity of prokaryotes in two Arctic fjords. Polar Biol. 39, 1749–1763. doi: 10.1007/s00300-015-1866-x
R Core Team (2019) R: A language and environment for statistical computing. R Foundation for Statistical Computing, Vienna, Austria
Racine, J. S. (2012). RStudio: A platform-independent IDE for R and Sweave. J. Appl. Econ. 27, 167–172. doi: 10.1002/jae.1278
Ramsey, J. P., Reinert, L. K., Harper, L. K., Woodhams, D. C., and Rollins-Smith, L. A. (2010). Immune defenses against Batrachochytrium dendrobatidis, a fungus linked to global amphibian declines, in the south African clawed frog, Xenopus laevis. Infect. Immun. 78, 3981–3992. doi: 10.1128/IAI.00402-10
Reeder, N. M. M., Pessier, A. P., and Vredenburg, V. T. (2012). A reservoir species for the emerging amphibian pathogen Batrachochytrium dendrobatidis thrives in a landscape decimated by disease. PLoS One 7:e33567. doi: 10.1371/journal.pone.0033567
Retallick, R. W. R., and Miera, V. (2007). Strain differences in the amphibian chytrid Batrachochytrium dendrobatidis and non-permanent, sub-lethal effects of infection. Dis. Aquat. Org. 75, 201–207. doi: 10.3354/dao075201
Robak, M. J., and Richards-Zawacki, C. L. (2018). Temperature-dependent effects of cutaneous bacteria on a frog’s tolerance of fungal infection. Front. Microbiol. 9:410. doi: 10.3389/fmicb.2018.00410
Rognes, T., Flouri, T., Nichols, B., Quince, C., and Mahe, F. (2016). VSEARCH: a versatile open source tool for metagenomics. PeerJ 4:e2584. doi: 10.7717/peerj.2584
Rohr, J. R., and Raffel, T. R. (2010). Linking global climate and temperature variability to widespread amphibian declines putatively caused by disease. Proc Natl Acad Sci U S A 107, 8269–8274. doi: 10.1073/pnas.0912883107
Rollins-Smith, L. A. (2020). Global amphibian declines, disease, and the ongoing battle between Batrachochytrium fungi and the immune system. Herpetologica 76, 178–188. doi: 10.1655/0018-0831-76.2.178
Rollins-Smith, L. A., Fites, J. S., Reinert, L. K., Shiakolas, A. R., Umile, T. P., and Minbiole, K. P. C. (2015). Immunomodulatory metabolites released by the frog-killing fungus Batrachochytrium dendrobatidis. Infect. Immun. 83, 4565–4570. doi: 10.1128/IAI.00877-15
Rollins-Smith, L. A., Ramsey, J. P., Pask, J. D., Reinert, L. K., and Woodhams, D. C. (2011). Amphibian immune defenses against chytridiomycosis: impacts of changing environments. Integr. Comp. Biol. 51, 552–562. doi: 10.1093/icb/icr095
Rollins-Smith, L. A., Woodhams, D. C., Reinert, L. K., Vredenburg, V. T., Briggs, C. J., Nielsen, P. F., et al. (2006). Antimicrobial peptide defense of the mountain yellow-legged frog (Rana muscosa). Dev. Comp. Immunol. 30, 831–842. doi: 10.1016/j.dci.2005.10.005
Rosenberg, E., and Ben-Halm, Y. (2002). Microbial diseases of corals and global warming. Environ. Microbiol. 4, 318–326. doi: 10.1046/j.1462-2920.2002.00302.x
Roth, T., Foley, J., Worth, J., Piovia-Scott, J., Pope, K., and Lawler, S. (2013). Bacterial flora on cascades frogs in the Klamath mountains of California. Comp. Immunol. Microbiol. Infect. Dis. 36, 591–598. doi: 10.1016/j.cimid.2013.07.002
Scheele, B. C., Pasmans, R., Skerratt, L. F., Berger, L., Martel, A., Beukema, W., et al. (2020). Response to comment on "amphibian fungal panzootic causes catastrophic and ongoing loss of biodiversity". Science 367, 1459–1463. doi: 10.1126/science.aay2905
Schulte, P. M., Health, T. M., and Fangue, N. A. (2011). Thermal performance curves, phenotypic plasticity, and the time scales of temperature exposure. Integr. Comp. Biol. 51, 691–702. doi: 10.1093/icb/icr097
Susilawati, L., Iwai, N., Komatsu, K., and Arie, T. (2021). Antifungal activity of bacteria isolated from Japanese frog skin against plant pathogenic fungi. Biol. Control 153:104498. doi: 10.1016/j.biocontrol.2020.104498
Suzina, N. E., Esikova, T. Z., Oleinikov, R. R., Gafarov, A. B., Shorokhova, A. P., Polivtseva, V. N., et al. (2015). Comparative characteristics of free-living ultramicroscopical bacteria obtained from natural biotopes. Appl. Biochem. Microbiol. 51, 159–168. doi: 10.1134/S0003683815020192
Tianero, M. D. B., Kwan, J. C., Wyche, J. P., Presson, A. P., Koch, M., Barrows, L. R., et al. (2015). Species specificity of symbiosis and secondary metabolism in ascidians. ISME J. 9, 615–628. doi: 10.1038/ismej.2014.152
Tobler, U., and Schmidt, B. R. (2010). Within- and among-population variation in chytridiomycosis-induced mortality in the toad Alytes obstetricians. PLoS One 5:e10927. doi: 10.1371/journal.pone.0010927
Turner, S., Pryer, K. M., Miao, V. P. W., and Palmer, J. D. (1999). Investigating deep phylogenetic relationships among cyanobacteria and plastids by small subunit rRNA sequence analysis. J. Eukaryot. Microbiol. 46, 327–338. doi: 10.1111/j.1550-7408.1999.tb04612.x
Valerio, F., Di Biase, M., Lattanzio, V. M. T., and Lavermicocca, P. (2016). Improvement of the antifungal activity of lactic acid bacteria by addition to the growth medium of phenylpyruvic acid, a precursor of phenyllactic acid. Int. J. Food Microbiol. 222, 1–7. doi: 10.1016/j.ijfoodmicro.2016.01.011
Versace, E., Nolte, V., Pandey, R. V., Tobler, R., and Schlötterer, C. (2014). Experimental evolution reveals habitat-specific fitness dynamics among Wolbachia clades in Drosophila melanogaster. Mol. Ecol. 23, 802–814. doi: 10.1111/mec.12643
Voyles, J., Young, S., Berger, L., Campbell, C., Voyles, W. F., Dinudom, A., et al. (2009). Pathogenesis of chytridiomycosis, a cause of catastrophic amphibian declines. Science 326, 582–585. doi: 10.1126/science.1176765
Webster, N. S., Cobb, R. E., and Negri, A. P. (2008). Temperature thresholds for bacterial symbiosis with a sponge. ISME J. 2, 830–842. doi: 10.1038/ismej.2008.42
Wickham, H. (2011). ggplot2. Wiley Interdisciplinary Reviews: Computational Statistics 3, 180–185. doi: 10.1002/wics.147
Woodhams, D. C., Alford, R. A., Antwis, R. E., Archer, H., Becker, M. H., Belden, L. K., et al. (2015). Antifungal isolates database of amphibian skin-associated bacteria and function against emerging fungal pathogens: ecological archives E096-059. Ecology 96:595. doi: 10.1890/14-1837.1
Wright, A. H., and Wright, A. A. (1949). Handbook of frogs and toads of the United States and Canada. Comstock Publishing Associates, Ithaca, New York.
Keywords: Chytridiomycosis, antifungal bacteria, host-pathogen interactions, amphibian, microbiome
Citation: Robak MJ, Saenz V, de Cortie E and Richards-Zawacki CL (2023) Effects of temperature on the interaction between amphibian skin bacteria and Batrachochytrium dendrobatidis. Front. Microbiol. 14:1253482. doi: 10.3389/fmicb.2023.1253482
Edited by:
Zhiyong Li, Shanghai Jiao Tong University, ChinaReviewed by:
Anbu Poosakkannu, University of Turku, FinlandPaul Greenfield, Commonwealth Scientific and Industrial Research Organisation (CSIRO), Australia
Copyright © 2023 Robak, Saenz, de Cortie and Richards-Zawacki. This is an open-access article distributed under the terms of the Creative Commons Attribution License (CC BY). The use, distribution or reproduction in other forums is permitted, provided the original author(s) and the copyright owner(s) are credited and that the original publication in this journal is cited, in accordance with accepted academic practice. No use, distribution or reproduction is permitted which does not comply with these terms.
*Correspondence: Corinne L. Richards-Zawacki, Y29yaS56YXdhY2tpQHBpdHQuZWR1