- 1Department of Environment, Constructions, and Design, Institute of Microbiology, University of Applied Sciences and Arts of Southern Switzerland (SUPSI), Mendrisio, Switzerland
- 2Department of Plant Sciences, University of Geneva, Geneva, Switzerland
- 3Alpine Biology Center Foundation, Bellinzona, Switzerland
- 4Department of Surface Waters – Research and Management, Swiss Federal Institute of Aquatic Science and Technology (Eawag), Kastanienbaum, Switzerland
- 5Civil and Environmental Engineering, University of California, Davis, Davis, CA, United States
- 6Faculty of Geosciences and Environment, Institute of Earth Surface Dynamics, University of Lausanne, Lausanne, Switzerland
- 7Department of Physics and Materials Science, Physics of Living Matter Group, Luxembourg City, Luxembourg
Introduction: Bioconvection, a phenomenon characterized by the collective upward swimming of motile microorganisms, has mainly been investigated within controlled laboratory settings, leaving a knowledge gap regarding its ecological implications in natural aquatic environments. This study aims to address this question by investigating the influence of bioconvection on the eco-physiology of the anoxygenic phototrophic sulfur bacteria community of meromictic Lake Cadagno.
Methods: Here we comprehensively explore its effects by comparing the physicochemical profiles of the water column and the physiological traits of the main populations of the bacterial layer (BL). The search for eco-physiological effects of bioconvection involved a comparative analysis between two time points during the warm season, one featuring bioconvection (July) and the other without it (September).
Results: A prominent distinction in the physicochemical profiles of the water column centers on light availability, which is significantly higher in July. This minimum threshold of light intensity is essential for sustaining the physiological CO2 fixation activity of Chromatium okenii, the microorganism responsible for bioconvection. Furthermore, the turbulence generated by bioconvection redistributes sulfides to the upper region of the BL and displaces other microorganisms from their optimal ecological niches.
Conclusion: The findings underscore the influence of bioconvection on the physiology of C. okenii and demonstrate its functional role in improving its metabolic advantage over coexisting phototrophic sulfur bacteria. However, additional research is necessary to confirm these results and to unravel the multiscale processes activated by C. okenii’s motility mechanisms.
Introduction
Bioconvection is an organized behavior observed, mainly in laboratory settings, in diverse groups of motile microorganisms, that share a common upward swimming behavior and a density higher than water (Ochiai et al., 2011; Ghorai and Panda, 2013; Abe et al., 2017). The convective motion is triggered when a large number of such microorganisms accumulate in a specific zone of a water body, leading to an increase in local density. This accumulation generates hydrodynamic instabilities with the underlying water, causing the denser, cell-rich layer to sink due to gravity in the form of characteristic ‘plumes’. The bioconvection cycle is sustained by the microorganisms carried away from the sub-surface layer being replaced by others up swimming from below, generating a convective cycle (Hill and Pedley, 2005; Bouffard and Wüest, 2019; Yanaoka and Nishimura, 2022).
Bioconvection can be distinguished from other drivers of water flow, such as wind, tides, and temperature gradients, due to its reliance on motile microorganisms, such as algae or bacteria (Hill and Pedley, 2005; Bees, 2020). It is characterized by the presence of biological concentration gradients within the fluid medium, which are created by the active movement of these microorganisms. Additionally, bioconvection typically occurs on small length and time scales, with a low Reynolds number compared to other forms of fluid flow, involving a dynamic feedback loop where microorganisms respond to the flow and chemical gradients they generate, making it a unique and complex process in aquatic ecosystems (Ochiai et al., 2011; Williams and Bees, 2011; Yanaoka and Nishimura, 2022).
In laboratory, the focus has been on photo- and gyrotaxis (Bees, 2020; Sengupta, 2023), while more recent works have shown that bioconvective movements can increase oxygen and nutrients transfer, providing benefits to microbial communities (Tuval et al., 2005; Tam and Hosoi, 2011; Ardekani et al., 2017). In the natural environment, it has been demonstrated that biotic communities can cause mixing similar to that produced by wind and tides in marine settings (Dewar et al., 2006; Katija and Dabiri, 2009; Lin et al., 2011). Nevertheless, within oceanic environments, the eco-physiological implications stemming from the convective mixing generated by motile microorganisms are yet to be elucidated (Kils, 1993; Kunze et al., 2006). Thus far, the observation of bioconvection in freshwater ecosystems has remained elusive, resulting in a lack of information on the fluid dynamics and energy balance caused by this process.
This research gap persists despite the potential importance of bioconvection for these ecosystems, where its effects may be more conspicuous than in marine environments because of the smaller size of the water bodies.
Meromictic lakes exhibit permanent stratification and vertical gradients of light and redox conditions (Gulati et al., 2017). Their water column harbors several physiological groups of microorganisms distributed along these vertical gradients, serving as distinct ecological niches (Rogozin et al., 2010; Andrei et al., 2015; Gulati et al., 2017; Block et al., 2021).
Lake Cadagno is an example of crenogenic meromixis, a phenomenon occurring when saline springs discharge dense water into a freshwater lake depression, setting up density-stabilizing conditions (Tonolla et al., 2017). As a result, the water column is permanently stratified into an oxic, electrolyte-poor mixolimnion and a salt-rich, sulfidic monimolimnion. Between the two layers, at a depth of about 12.0 m, is an oxidoreductive transition zone, commonly referred to as the chemocline, where the oxygen disappears and sulfide develops (Di Nezio et al., 2021). A characteristic feature of Lake Cadagno is the presence of a community of anoxygenic phototrophic sulfur oxidizing bacteria, which develop due to the euxinic environment and the presence of light (Ravasi et al., 2012; Danza et al., 2018). This community forms a dense bacterial layer (BL; up to 107 cells ml−1 in the warm season) and is composed of seven species of purple sulfur bacteria (PSB) and two species of green sulfur bacteria (GSB), which compete for the same resources (light and sulfide). The most representative BL species, accounting for over 80% of the total microbial community (Danza et al., 2018), encompass the large motile PSB Chromatium okenii (Luedin et al., 2019a), the small PSB Thiodictyon syntrophicum (Peduzzi et al., 2012) and the two GSB species of the genus Chlorobium, C. phaeobacteroides, and C. clathratiforme (Habicht et al., 2011). These three bacterial phenotypes represent different evolutionary strategies, and due to their different sizes, they can be effectively monitored by flow cytometry (Danza et al., 2017). Large-celled C. okenii (8.0–10.0 μm) displays active motion through positive phototaxis and negative aerotaxis (Pfennig et al., 1968; Luedin et al., 2019a). Small-celled T. syntrophicum (1.4–2.4 μm) has the capacity to create aggregates with microorganisms that produce sulfide, i.e., sulfate-reducing bacteria (SRB) from the genus Desulfocapsa (Tonolla et al., 2000). Conversely, the Chlorobi, due to their small size (0.6–1.3 μm), have a well-developed pigment apparatus that enables them to harvest lower light intensities (Frigaard and Bryant, 2006; Overmann, 2006). In the course of multiple monitoring efforts carried out on Lake Cadagno, spanning over 30 years of scientific research, the annual population dynamics of the BL have been characterized (Tonolla et al., 2005; Peduzzi et al., 2011; Storelli et al., 2013; Luedin et al., 2019b). During the initial warm season, from June to October, C. okenii exhibits dominance, whereas GSB and small-celled PSB T. syntrophicum take over the BL community in late August-beginning September (Tonolla et al., 2003; Danza et al., 2018). This population dynamic during the warm season also coincides with the occurrence of bioconvection, observed in Lake Cadagno only between June and late August, based on the physicochemical profiles of the water column, as recently reported in Sommer et al. (2017). Combining field and laboratory studies with numerical modeling, the authors of the study demonstrated that the motile PSB C. okenii can initiate bioconvection in the BL of meromictic Lake Cadagno and convectively homogenize a one-meter-scale layer, both in the presence of light and in the dark (Sepúlveda Steiner et al., 2019, 2021). This was the first example of bioconvection witnessed in a natural freshwater environment, so far limited to observations in marine ecosystems or laboratory settings. However, the study by Sommer et al. (2017) focused on the physical demonstration of the phenomenon without exploring its consequences on microenvironmental conditions, microbial community, or biogeochemical implications on the lake ecosystem. Furthermore, the BL community plays a crucial role in the lake’s ecology by participating in central biogeochemical cycles like carbon (Posth et al., 2017), sulfur (Di Nezio et al., 2021) and nitrogen (Philippi et al., 2021).
The objective of this study was to investigate whether the presence of bioconvection in Lake Cadagno during the peak of the warm season (July) significantly impacts the physicochemical profiles of the water column and the eco-physiological characteristics of the anoxygenic phototrophic sulfur bacteria of the BL when compared to the absence of bioconvection (September).
Methods
Study site and sampling
Lake Cadagno is located in the Piora Valley (46°33’N, 8°43′E) in the southern Swiss Alps. The sampling was conducted on 16 July and 17 September 2020 using a pump-CTD system (CTD115M, Sea & Sun Technology, Germany), as described in Di Nezio et al. (2021). For the physicochemical characterization of the water column, in situ high vertical resolution data (sampling at 16 Hz) on temperature (°C), conductivity (mS cm−1), dissolved oxygen (mg l−1), light (PPFD) and turbidity (FTU) were obtained during a first continuous downcast of the CTD system from the lake surface down to ~18.0 m depth. During a second downcast, after 30 min and in a different area, discrete water samples were collected from six depths (between 12.0 m and 18.0 m) for bio-chemical analyses and from the top of the BL for incubation experiments (see ‘Radioisotope incubation and uptake analysis’ section). Profiles were recorded at sunrise on 16 July and 17 September 2020 at 05:15 h and 06:30 h, respectively. CTD profiles for determination of light regimes were measured at daytime (17:00 h) on both days.
Atmospheric radiation data at a 10-min resolution were retrieved from a meteorological station (istSOS)1 close to the lakeshore during both sampling campaigns. Water samples for microbiological (FISH and flow cytometry) and chemical (S2−, SO42− and CaCO3) analyses were stored in 50 mL falcon tubes and processed within the following hour, as described in Di Nezio et al. (2021).
Cell growth
The main characteristics of the phototrophic sulfur bacteria strains used in this study are described in Table 1.
PSB and GSB were grown in Pfennig’s medium I (Eichler and Pfennig, 1988) and cultivated in laboratory under a light/dark photoperiod of 16/8 h with a light intensity of 38.9 μmol m−2 s−1 PPFD (photosynthetic photon flux density) within the photosynthetically active radiation (PAR) range, measured with a portable LI-180 Spectrometer (LI-COR Biosciences, Lincoln, NE), as in Di Nezio et al. (2021).
Bacterial layer microbial community analysis
To describe the composition of the BL microbial community, cell counting was performed through flow-cytometry (FCM), as described in Danza et al. (2017). Bacterial populations were distinguished by applying gates on cell size (forward scatter, FSC) of 0.6–1.3 μm for GSB, 1.4–4.0 μm for small-celled PSB and 8.0–10.0 μm for C. okenii (Supplementary Figure S1). Simultaneously, fluorescent in situ hybridization (FISH) analyses were carried out on bacterial layer (BL, zone with a turbidity >10 FTU) water samples, as previously described in Decristophoris et al. (2009) (Supplementary Table S1), spotting 2 μL of fixed sample on gelatin coated slides (0.1% gelatin, 0.01% KCr(SO4)2) and observing them by epifluorescence microscopy using filter sets F31 (AHF Analysentechnik, Tübingen, Germany; D360/40, 400DCLP, D460/50 for DAPI) and F41 (AHF Analysentechnik, HQ535/50, Q565LP, HQ610/75 for Cy3) at 100X magnification.
Radioisotope incubation and uptake analysis
To test the photosynthetic efficiency of pure bacterial laboratory cultures and describe their different ecological niches, cells were grown up to concentrations of 106 cells ml−1 (mid exponential phase), sealed in 50-cm-long dialysis bags (inflated diameter of 62.8 mm; Karl Roth GmbH Co. KG, Germany), whose membrane allows for diffusive transport of small molecules (< 20 kDa); thus, preventing contamination of the incubated samples from the surrounding environment.
The dialysis bags were acclimatized to the chemocline conditions of Lake Cadagno for a period of 4 weeks (from 15 June to 16 July 2020 and from 21 August to 17 September 2020) before the experiments, at varying depths between 12.20–12.40 m and 13.12–12.94 m in July and September, respectively.
To measure the amount of light reaching the incubation depth, the dialysis bags supporting frame was equipped with HOBO UA-002–64 Pendant passive data loggers (light measurement range: 0 to 320,000 lux; Onset Computer Corporation, MA, USA), one at the top and one at the bottom of the structure, measuring relative light (Lux; 180–1,200 nm) at 60 min intervals during the 4-weeks acclimatization periods, as well as over the course of the 24 h 14C incubations (16–17 July and 17–18 September 2020). Average daylight intensity values for June–July and August–September recorded at the top and bottom of the dialysis support frame are shown in Supplementary Figure S2.
The 14C-radioisotope uptake experiment was carried out on 16 July and 17 September 2020. The 14CO2 assimilation of every selected microorganism, was quantified in sealed glass bottles after a day and night incubation, both in July (at 12.28 m and 12.35 m depth) and September (at 12.63 m and 12.83 m depth). The total inorganic carbon assimilation measured on water sampled from the top of the BL served as a positive control of cell activity. The same water was also filtered through 0.22 μm filters to remove any microorganisms and define a zero value to be used as a negative abiotic control.
The dissolved inorganic carbon concentration needed for the calculation (Gächter and Marès, 1979) was determined using the CaCO3 Merck Spectroquant kit (No. 1.01758.0001) and the Merck Spectroquant Pharo 100 spectrophotometer (Merck, Schaffhausen, Switzerland). Rates were expressed as attomoles (amol) of 14CO2 per cell volume per hour. Cell volumes were calculated as described in Tonolla et al. (2003). The total biovolume of the various species in the control sample was computed using the relative abundances quantified by flow cytometry and FISH.
Light and energy requirements calculations
HOBO light and carbon assimilation data were used to calculate the quantum requirement for the CO2 fixation as the ratio between moles of photons absorbed and moles of 14CO2 fixed by the BL and the dialysis bags pure cultures. Moles were correlated to the surface area (m2) of the glass vials used for the 14C-incubation to calculate how many moles of photons reached the surface of the vials over the entire light period (Brune, 1989).
Statistical analysis
Data are shown as mean ± standard deviation. All the measurements were taken from distinct samples. Statistical significance was assessed by two-way ANOVA for parametrical data, as indicated; Šidák test was used as a post-hoc test. For multiple comparisons, multiplicity adjusted p-values are indicated in the corresponding figures. Statistical analyses comprising calculation of degrees of freedom were done using GraphPad Prism 9.5.1.
Results
Monitoring of the water column
The physicochemical profiles of Lake Cadagno remained largely unchanged between July and September 2020 (Figures 1A,B). Parameters measured with the CTD including dissolved oxygen (DO), temperature, and conductivity showed little difference between the two periods throughout the water column. Nonetheless, upon thorough analysis of the profiles, temperature and conductivity appeared vertically homogenous around 12 m depth in July (as illustrated in Figure 1A), concurrently with the presence of the BL (Figure 1C). Conversely, the profiles observed in September (Figure 1B) did not exhibit such uniformity. The oxygen profile shows little variation between July and September (Figure 1C, right box). In July, oxygen depletes before the top of the BL, whereas in September, it only disappears in the middle of the BL. The dissolved oxygen profile of July exhibits irregularity, notably, a peak in production occurring at 5 m and a minor one at 11 m depth (Figure 1A), indicating the occurrence of oxygenic photosynthesis. The main difference between the July and September physicochemical profiles was observed in the light and turbidity profiles (Figures 1D,E). A turbidity value higher/lower than 10 FTU, is used to define the presence/absence of the BL. On 16 July 2020, the 1.2 m wide BL lied between 12.18 and 13.36 m depth, with a maximum turbidity value of 17.7 FTU at a depth of about 12.70 m (Figure 1D), while on 17 September 2020, it was nearly 20 cm wider (1.4 m) and about 70 cm deeper (12.89–14.30 m depth) with a maximum turbidity peak of 36.9 FTU almost a meter deeper than in July, at 13.40 m (Figure 1E). Such difference in depth resulted in a disparate light profile with an intensity reaching the BL top of 0.44 W m−2 in September, twice lower than in July with 0.88 W m−2 (Figures 1D,E).
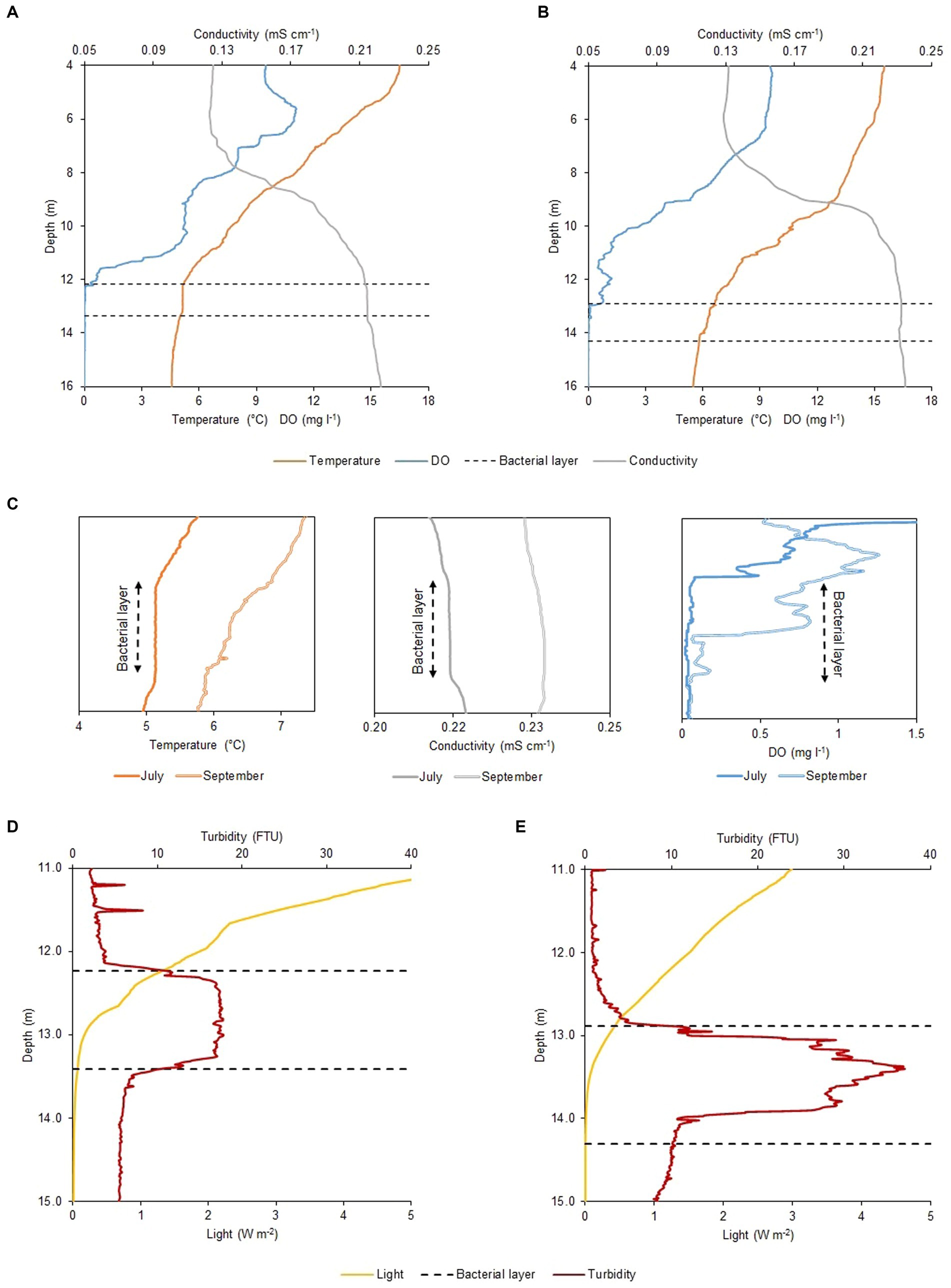
Figure 1. Physicochemical profiles of Lake Cadagno water column. CTD profiles of oxygen (mg l−1), temperature (°C), and temperature-corrected (20°C) conductivity (mS cm−1) in (A) July and (B) September. (C) Temperature (left), temperature-corrected conductivity (central) and DO (right) microstructure profiles for the BL and adjacent regions observed on the two different moments of the year 2020. Different light irradiance (W m−2) and turbidity (FTU) values within the BL observed between (D) July and (E) September. Black dashed lines indicate the BL position on both sampling days (16 July and 17 September 2020).
Differences in light availability
Data collected with the CTD PAR sensor (Figures 1D,E, yellow line) and the pyranometer of the meteorological station (Table 2), revealed a difference in the duration and intensity of light between July and September. The net radiation measurement showed an average photoperiod in July of around 16.0 h (05:15–21:20) while in September it reduced to about 12.5 h (06:40–19:20 h). This resulted in a PAR portion of the average incident radiation below the lake subsurface (1 cm depth) of 100.85 W m−2 day−1 on 16 July and 43.62 W m−2 day−1 on 17 September and, consequently, light radiation reaching the top of the BL was higher in July (Table 1). Moreover, in September the larger values of observed turbidity (Figure 1E) determined a greater degree of light attenuation across the BL. Therefore, the shading effect exerted by the turbidity peak ultimately caused no light to reach the lower part of the BL (Table 2).
Sulfide and intracellular complexity at the top of the BL
In addition to light, anoxygenic phototrophic sulfur bacteria in the BL require reduced sulfur compounds, such as sulfide (S2−) or elemental sulfur (S0). These compounds are present in the water after the SRB metabolism, and in the form of characteristic sulfur globules, produced intracellularly by PSB and extracellularly by GSB (Figure 2A). On 16 July, S2− concentration measured at sunrise, before the light reached the BL, was around 0.14 mg l−1 at the top of the BL and 1.88 mg l−1 at the bottom, while on 17 September, at the same moment of the day, sulfide concentration at the top was zero (below the detection limit of 0.03 mg l−1) and 1.23 mg l−1 at the bottom (Figure 2B, yellow bars).
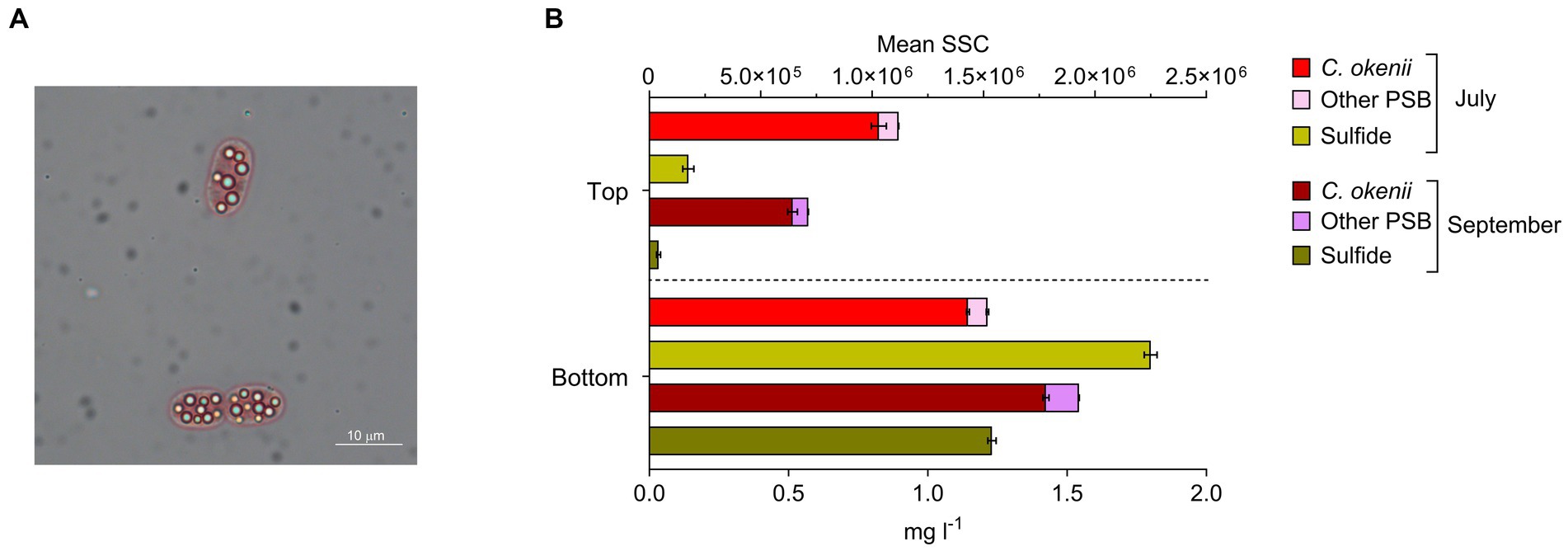
Figure 2. (A) Microscopy image of Chromatium okenii cells (100X magnification) with intracellular sulfur globules. (B) Flow cytometry mean sideward scatter (SSC) of C. okenii and small-celled PSB and corresponding sulfide concentrations in the upper and lower part of the bacterial layer on 16 July and 17 September 2020. Error bars represent standard deviation (n = 3).
The occurrence or lack of sulfide at the top of the BL, where there is still enough light for anoxygenic photosynthesis, shows a positive correlation with cellular complexity (SSC) that is determined by flow cytometry (Danza et al., 2017). In July, when sulfide is present at the top of the BL, we observed a greater intracellular complexity for PSB, with emphasis on C. okenii (Figure 2B, red and pink bars). In September, when, despite the lower light, S2− is no longer available, a marked reduction occurred in the SSC value for C. okenii (Figure 2B, burgundy and purple bars).
Differences in the BL community composition
The total number of cells of C. okenii (Figure 3A, red bars), the other 6 PSB species (Figure 3A, pink bars), and GSB (Figure 3A, green bars) was determined by flow cytometry based on the different cell size (Danza et al., 2017).
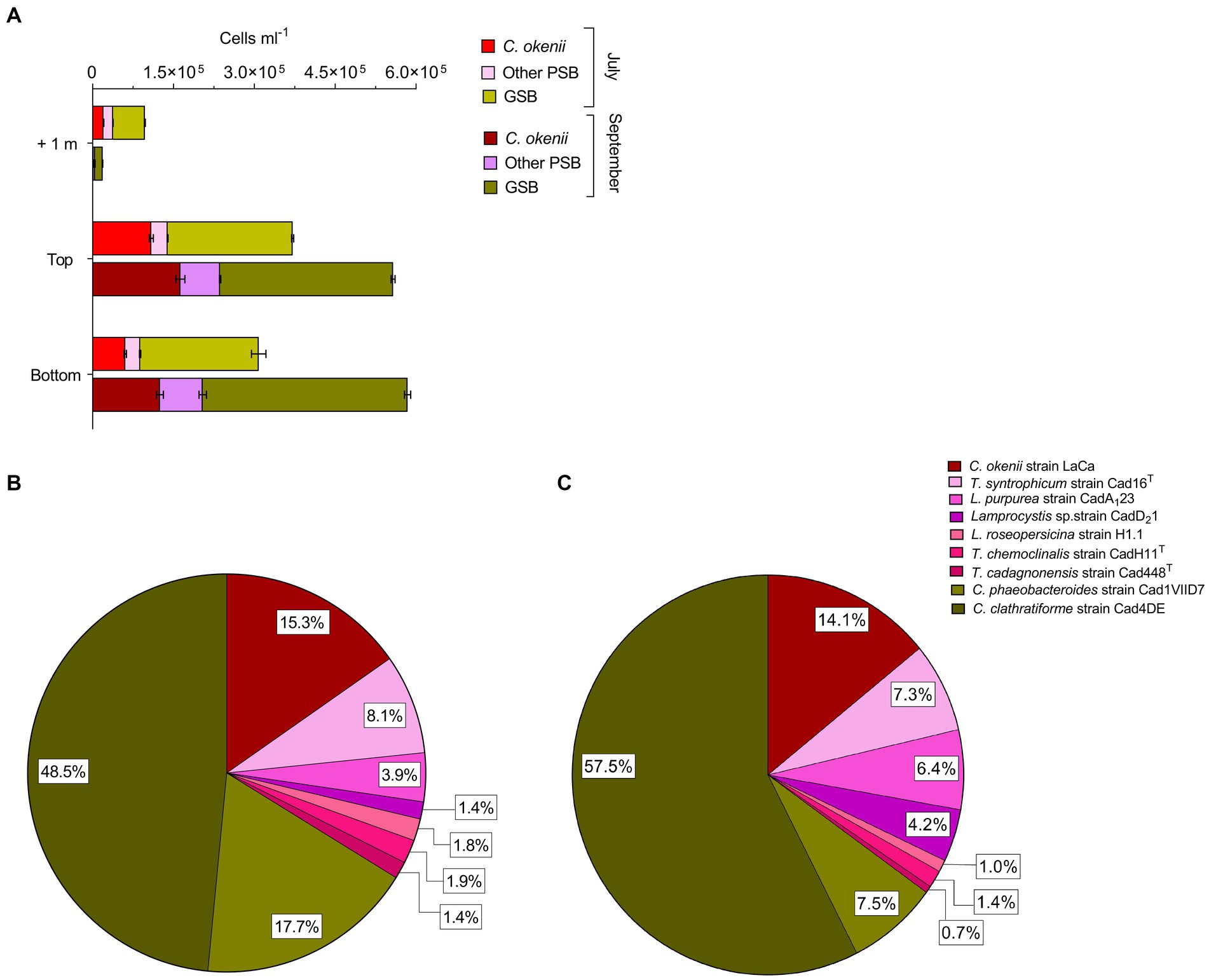
Figure 3. (A) Flow cytometry counts revealed different abundances of anoxygenic phototrophic sulfur bacteria 1 m above, at the top and bottom of the BL between 16 July 2020 (light colors) and 17 September 2020 (dark colors). Cell numbers (×105 mL−1) of all phototrophic sulfur bacteria in the BL obtained by FISH with probes S453F, S453A, S453E, S453H, S453D, S448, CHLP and CHLC at the top of Lake Cadagno BL on (B) 16 July 2020 and (C) 17 September 2020. Error bars represent standard deviation (n = 3).
During both time periods analyzed, the concentration of large-celled C. okenii (approx. Length 8.0–10.0 μm) peaked at the top of the BL, when the turbidity surpassed 10 FTU. FCM red fluorescence signal indicated concentrations of 1.09 ± 0.03 × 105 cells ml−1 in July (Figure 3A, red bars ‘Top’) and 1.63 ± 0.08 × 105 cells ml−1 in September (Figure 3A, burgundy bars ‘Top’). The relative abundance of C. okenii then decreased toward the lower part of the BL, with cell concentrations dropping to 0.61 ± 0.02 × 105 and 1.25 ± 0.06 × 105 cells ml−1 in July (Figure 3A, red bars ‘Bottom’) and September (Figure 3A, burgundy bars ‘Bottom’), respectively. In July, no significant differences in the concentration of small-celled PSB and GSB were detected between the top and bottom of the BL (Figure 3A, pink and light green bars). Conversely, during September, more PSB and GSB were detected at the bottom of the BL compared to the top (Figure 3A, purple and dark green bars).
Interestingly, in July during bioconvection, flow cytometry counts revealed high numbers of small-celled PSB and GSB 1 meter above the BL, at a depth well outside their preferred anaerobic zone (Figure 3A, pink and light green bars ‘+1’). Conversely, in September, as bioconvection faded, their numbers above the BL decreased considerably (Figure 3A, purple and dark green bars ‘+1’) even though the number of cells was larger. Flow cytometry is a useful tool for providing an overview of the major populations in the BL, but it lacks the resolution needed to differentiate cells with the same morphology as the 7 small-celled PSB and 2 GSB Chlorobium species. In contrast, fluorescent in situ hybridization (FISH) allows a precise quantification of the complete spectrum of anoxygenic phototrophic sulfur bacteria species within the BL. This method enabled comparative analysis between samples collected in July (Figure 3B) and September (Figure 3C).
Percentages corresponding to the FISH counts were calculated relative to the total cell population within the BL, allowing for the depiction of variations in species ratios over time. FISH analysis revealed that such increase in small-celled PSB from July to September was mainly due to Lamprocystis sp., strains CadA123 and CadD21 which raised from 3.9 to 6.4%, and from 1.4 to 4.2% of the total BL counts, respectively. Among GSB, from July to September, Chlorobium clathratiforme presence increased by about 9.0%, with a concomitant drop of C. phaeobacteroides of 10.2% (Figures 3B,C, dark and light green). The motile PSB C. okenii, showed a slight decrease of 1.2% in September.
Light-dependent physiological efficiency
The physiological efficiency of the different typology of bacterial strains was assessed by analyzing their primary production over a 24-h period and comparing the results between July and September. We used laboratory-grown pure cultures of the PSB C. okenii strain LaCa, the small PSB T. synthophicum strain Cad16T, and the GSB C. phaeobacteroides strain 1VII D7. The cultures were incubated in Lake Cadagno for 4 weeks inside dialysis bags (50 cm length) at different depths corresponding to the top of the BL in July and September (12 m and 13 m, respectively). During the four-week incubation period in the lake, the presence of light was constantly monitored both above and below the dialysis bags (Supplementary Figure S2). At the time of the experiment, the dialysis bag cultures were transferred into clear glass bottles containing radiocarbon 14 and incubated day (~16 h) and night (~8 h) on July 16 and again day (~ 12.5 h) and night (~11.5 h) on September 17, along with a water sample from the top of the BL as a control.
Rates of 14CO2 assimilation are listed in Table 2. In July, the 14CO2 assimilated by the control sample after daytime incubation was over four times greater than that observed in September.
Among the individual species incubated in the dialysis bags, the large-celled C. okenii exhibited the highest 14CO2 fixation activity in July, and not in September, when maximum assimilation rate was measured for T. syntrophicum. In fact, between July and September, T. syntrophicum increased its photosynthetic activity by more than 4 times, while C. okenii reduced it by more than 5 times. As observed in a previous study (Di Nezio et al., 2021), the photosynthetic activity of GSB C. phaeobacteroides is much lower than that of PSB. Nevertheless, it is noteworthy that its activity experienced a nearly twofold increase in September as compared to July.
In general, the uptake of 14CO2 at night, in the absence of light, was much lower than the diurnal photoassimilation. Despite a rather pronounced difference in photosynthetic activity, the control showed similar assimilation rates in the absence of light, which were quite high at both time points. Among pure cultures, only C. okenii exhibited substantial nocturnal fixation in July, while otherwise, dark assimilation remained consistently low.
Discussion
To date, it remains uncertain whether bioconvection confers positive eco-physiological advantages upon the responsible organism and/or negative effects on analogous microorganisms within its ecological niche. A handful of laboratory-based experiments using pure cultures have suggested that microbes use bioconvection to their benefit for exploiting nutritional microenvironments and improved nutrient absorption efficiency (Ni et al., 2020; Shoup and Ursell, 2021; Sengupta, 2023). For example, experimental observations and fluid dynamic simulations have shown that formation of bioconvection can facilitate oxygen transport, which may in turn benefit aerobic microbial communities, as observed in suspensions of Bacillus subtilis (Tuval et al., 2005).
These experiments underscore the potential advantages bioconvection offers to its producers and, concurrently, to other coexisting microorganisms within the same ecological niche. In the present study, we conducted the first examination of bioconvection in its natural environment, unveiling hitherto unexplored eco-physiological aspects of this phenomenon. This was achieved thanks to the possibility of carrying out field experiments directly on meromictic Lake Cadagno, where we compared two distinct moments of the year, mid-July, when bioconvection is well present in the BL of the lake, and mid-September, when bioconvection is absent (Sommer et al., 2017; Sepúlveda Steiner et al., 2019).
Chemocline physicochemical parameters
First, we monitored and compared the physicochemical parameters of Lake Cadagno water column on 16 July and 17 September 2020, which were consistent with past measurements of the same periods (Danza et al., 2017; Luedin et al., 2019a; Di Nezio et al., 2021). The weather trend for summer 2020 was within the normal range, with good insolation and little precipitation, with the only exception of a heavy thunderstorm in late August that caused a strong mixing of the mixolimnion, and a consequent increase in light penetration to the BL (Storelli et al., under revision). On 16 July, the water column profile revealed the homogeneous temperature and conductivity signatures within the BL, right below the oxic-anoxic interface of the chemocline (Figure 1C), a proxy of the presence of convective turbulence, which has been shown to be caused by the swimming activity of PSB C. okenii (Frigaard and Dahl, 2008; Sommer et al., 2017). Therefore, the lack of a uniform layer in the CTD profile of 17 September confirmed the absence of bioconvection in late Summer, further highlighting its seasonality, as already observed in previous studies on Lake Cadagno (Danza et al., 2017; Barnett et al., 2020).
Data presented by Sommer et al. (2017) showed how, over the course of three summer seasons (August 2014 and 2015, July 2016), bioconvection activity and thickness of the mixed layer in Lake Cadagno positively correlated with C. okenii cell concentration. In fact, the authors observed that an increase in the concentration of bioconvecting C. okenii cells corresponded with a proportional increase in the thickness of the mixed layer. A higher concentration of C. okenii has also been correlated to augmented convective intensity (Sepúlveda Steiner et al., 2021). We refer to the authors’ direct numerical simulations (Figure 2, Sepúlveda Steiner et al., 2021, Supporting Information Text S3 of the paper) for convincing evidence that the homogeneous layer in Lake Cadagno is due to active biogenic mixing. A detailed explanation of the vertical structure of the mixed layer is provided by Sepúlveda Steiner et al. (2019).
Unfortunately, these observations were limited to the time when bioconvection is present. Interestingly, in September, when bioconvection was absent, C. okenii cell concentration was higher than in July, when bioconvection was active. This result highlights the complexity of the process driven by the C. okenii, seemingly more related to other abiotic or biotic factors investigated in this study, such as light, sulfide or the presence of other phototrophs in the BL, rather than simply to C. okenii population size.
Bioconvection affects sulfide transport across the bacterial layer
The importance of bioconvection in maintaining chemical gradients across the BL, ensuring the constant influx of key elements, has already been proposed in laboratory studies (Bearon and Grünbaum, 2006; Findlay et al., 2017). Interestingly, the higher S2− concentration across the BL observed in July showed that bioconvection transports sulfide from the depths of the lake (Figure 2; Supplementary Figure S3). In addition to carrying more sulfide, an essential requirement for anoxygenic photosynthesis, bioconvection also promotes the removal of oxygen (Figure 1C, right), which is chemically reduced by S2−. The presence of oxygen during the photosynthetic activity of anoxygenic phototrophic sulfur bacteria reduces its effectiveness (42). However, previous studies have also shown that, for PSB, the presence of a small amount of oxygen facilitates CO2 assimilation in the dark (Berg et al., 2019; Luedin et al., 2019b; Di Nezio et al., 2021).
A distinguishing feature of PSB is the production of intracellular sulfur globules (S0) (Frigaard and Dahl, 2008), that contribute to determining cell internal complexity, correlating with the SSC parameter measured with FCM (Danza, 2018). On 16 July at sunrise, when bioconvection was active (Figure 1C), sulfide was detected up to top of the BL, concurrently with a more homogeneous distribution of intracellular complexity between upper and lower BL (Figure 2). Conversely, at sunrise on 17 September, with no mixed layer, no S2− was detected at the top BL and it mostly remained confined to the lower part, in concomitance with a less pronounced PSB cell granularity at the top BL (Figure 2). Given these points, it is evident that bioconvection has the potential to expand the optimal ecological niche by transporting S2− from the bottom, thereby increasing its presence in conjunction with light.
Light period affects bacterial motility and primary production
The importance of photoperiod in shaping bacterial motility has been investigated in several studies. For example, experiments on the flagellated microalga Chlamydomonas reinhardtii (Jin et al., 2020), and other microalgae (Richter et al., 2014), revealed that variations in the light–dark period not only markedly affected cell swimming behavior but also influenced cell orientational and gravitactic transport. Similar trends have been presented by other studies on the influence of photoperiod length on the motility rhythm of swimming microorganisms, with important consequences at the population scale (Fitt and Trench, 1983; Lerch and Cook, 1984; Barnett et al., 2020).
At first the number of cells was identified as a possible factor influencing bioconvection (Sommer et al., 2017). However, our results suggest photoperiod is a key factor for the onset of bioconvection. In fact, under the shorter light period of September (12.5 h) no mixed layer is observed within the BL, despite the number of C. okenii cells being higher than in July (Figure 1). Further evidence comes from laboratory experiments, where a reduction in the growth rate of C. okenii was observed under a photoperiod of 12/12 h, compared to one of 16/8 h. Interestingly, even after changing light intensity from a chemocline-like value of about 4.0 μmol m−2 s−1 PPFD to a 10-fold increase (about 40 μmol m−2 s−1 PPFD), the reduction in growth was maintained under the 12/12 h photoperiod, further emphasizing the importance of light period rather than its intensity (Di Nezio et al., 2023).
Our results indicate that a different length of the light period can significantly impact photosynthesis itself, by determining different light-stimulated rates of inorganic carbon uptake as showed in other photosynthetic microorganisms (Zhou et al., 2015; Johar Gunawan et al., 2018; Xu et al., 2021), and in PSB and GSB in Lake Cadagno as well. It has been observed that CO2 fixation in PSB does not occur at a constant rate throughout the day but reaches the highest values in the first hours of light exposure (Storelli et al., 2013), compared with the hours of highest light intensity in the afternoon (Di Nezio et al., 2021). This trend has also been observed in cyanobacteria (Wyman, 1999; Paul et al., 2000). For this reason, adopting whole day and night incubations allowed us to avoid underestimate carbon assimilation activity due to diel cycles.
In this study, the in situ daily 14C assimilation observed confirmed the strong total inorganic carbon fixation rate in the BL of Lake Cadagno. On 16 July, with 16 h of light, diurnal assimilation was more than three times higher than in September, when values reached only after a daylength of 12.5 h (Table 2). This result is certainly strongly influenced by the physiological activity of C. okenii. In fact, their intense fixation activity measured in July was higher than that of T. syntrophicum and C. phaeobacteroides (Table 3). The situation changed radically in September, when in the absence of bioconvection, C. okenii loses dominance over inorganic carbon fixation in favor of the small-celled PSB T. syntrophicum.
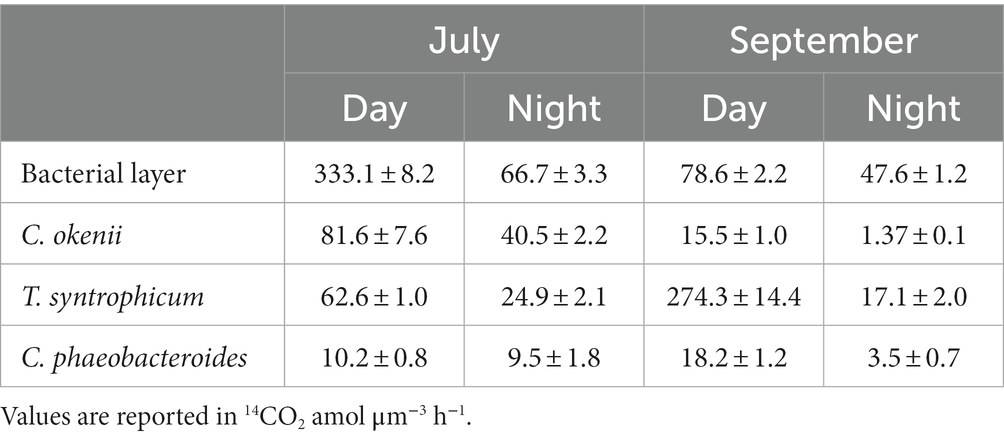
Table 3. July and September 14CO2 mean assimilation rates (±standard deviation) of the BL microbial community and dialysis bags incubated cultures.
Despite being more abundant (Figure 3), GSB were outcompeted by PSB for the uptake of inorganic carbon. Nonetheless, to sustain their photosynthetic growth, these bacteria can rely on different substrates. In fact, GSB have the capacity, although limited, for assimilating organic carbon compounds, such as acetate or pyruvate (Frigaard et al., 2003; Tang and Blankenship, 2010).
Similarly, the higher dark 14C assimilation rates observed in July in the BL and in C. okenii pure culture suggests the persistence of bioconvection during nighttime in Lake Cadagno (Sepúlveda Steiner et al., 2021). Several studies have reported that PSB have the ability fix inorganic carbon in the absence of light by aerobic sulfur-dependent chemoautotrophy (Casamayor et al., 2008, 2012; Berg et al., 2019; Luedin et al., 2019b). In September, our results show good night fixation only for the “ecological control,” while cultures exhibited comparatively diminished fixation rates. One plausible explanation for this observation could be attributed to the elevated presence of Lamprocystis sp. (Figure 3C), which are well-documented for their proficiency in nocturnal assimilation (Musat et al., 2008; Storelli et al., 2013), although this is probably not the only factor. Interestingly, the correlation between the carbon fixation contributions of PSB and GSB and their abundance does not consistently align, as observed in Lake Cadagno (Musat et al., 2008). Recent studies hypothesized that viral infections affecting PSB and GSB, thereby influencing rates of photosynthetic inorganic carbon fixation, may provide an explanation for this observed pattern (Saini et al., 2022; Hesketh-Best et al., 2023).
In addition, quantum requirements of CO2 fixation for each of the three species incubated with carbon-14 were calculated as moles of photons required to assimilate a mole of 14CO2. The value obtained for C. okenii in July (10.6) was similar to that reported by Brune (1989), who calculated quantum requirements of 8.5–10.5 quanta per CO2 fixed for PSB and 3.3–4.5 for GSB, under optimum laboratory conditions, while T. syntrophicum and C. phaeobacteroides had much higher requirements (23.4 and 74.9, respectively). Under the September light regime, C. okenii performed significantly worse (38.5), while T. syntrophicum (13.5) and C. phaeobacteroides (39.1) had both lower quantum requirements than in July. Applying what observed in the 14C-incubated cultures to the broader environmental context of the lake, our data provide enough evidence to sustain that light regime played a key role, as it provided C. okenii cells with the energy required for the onset of bioconvection.
These findings, in combination with the increase in numbers shown by FISH for all species of small-celled PSB (Figure 3B), further indicate that bioconvection could exert a negative influence on other microorganisms competing for the same ecological niche as C. okenii.
Community dynamics in the bacterial layer
The top-bottom small-celled PSB uniform SSC signal in July correlates well with the hypothesis of C. okenii dragging along other microorganisms in the BL, namely small-celled PSB and GSB, that are passively moving floating by means of gas vacuoles (Peduzzi et al., 2012; Luedin et al., 2018). Despite the limitations in differentiating among individual species of small-celled PSB, flow cytometry allows to accurately distinguish C. okenii from the other PSB due to their different cell size. In fact, using specific gates on FSC as constant signature for identification, SSC values were measured for all events contained in the specific gate of the respective population (Supplementary Figure S1). In fact, small-celled PSB and GSB populations distribution across the BL highlighted some interesting patterns (Figure 3A). Interestingly, while C. okenii cells were in general more abundant at the top BL on both times of the season, on 16 July, during active bioconvection, we measured a higher number of small-celled PSB and GSB 1 m above the BL than on 17 September (without bioconvection). This finding underscores a significant fitness advantage for C. okenii over other microorganisms competing for resources such as sulfide and light. Indeed, the displacement by bioconvection of many small-celled PSB and GSB from the optimal BL zone in July (+17%), and not in September (Figure 3A), facilitates an expansion of the ecological niche available to C. okenii.
The consequences of bioconvection also appear to affect trophic links, such as grazing by zooplankton. A recent study on the predatory activity of the ciliate Spirostomum teres, known to feed on PSB (Bolick, 2022), in Lake Cadagno during the years 2020 and 2021 revealed that, in the presence of bioconvection, the amount of C. okenii cells ingested by S. teres is significantly lower than when bioconvection is absent (Bolick et al., in preparation). All together, these observations suggest the effective ecological advantage that C. okenii has in producing bioconvection.
Conclusion
In this paper, we analyzed biological, chemical, and physical factors to elucidate how bioconvection shape the main eco-physiological traits for the phototrophic sulfur bacteria community inhabiting the BL of meromictic Lake Cadagno. We first report the key role of the photoperiod length by comparing two different period of measurements. Moreover, we showed how the presence of bioconvection contributes to maintaining a sulfide gradient across the BL, thereby promoting oxygen removal. It is also interesting to note that bioconvection should negatively affects the fitness of the other ecological competitors of C. okenii, namely small-celled PSB and GSB, present in the BL. Overall, our combined data suggest that C. okenii is able to gain a competitive advantage over other non-motile anoxygenic phototrophic sulfur bacteria in the quest for the optimal environmental conditions by producing mixed layers through bioconvection.
Nevertheless, despite this study provides evidence of the eco-physiological effects of bioconvection in an environmental setting, its consequences on the microenvironmental conditions and the other (micro)organisms involved need to be substantiated with further studies. In particular, the role of bioconvection on the transport of sulfide across the BL, and further insights on its production by sulfate-reducing bacteria in the monimolimnion, require a more detailed investigation. Impacts of bioconvection might also extend outside the mixed layer, influencing the interaction between phototrophic sulfur bacteria and the zooplankton living just above the bacterial layer, e.g., in terms of predation and/or distribution patterns. Lastly, a deeper understanding of the motility mechanisms of C. okenii triggering bioconvection at the single-cell level will help unraveling the nature of this multi-scale process.
Data availability statement
The raw data supporting the conclusions of this article will be made available by the authors, without undue reservation.
Author contributions
FN, SR, AB-D, and NS designed research and collected samples. FN and SR performed laboratory work. FN, SR, OS, and AB-D analyzed data. FN and NS wrote the paper. All authors developed the concepts and hypotheses covered in this work, and have contributed to the revisions of the manuscript.
Funding
Funding was provided by the Swiss National Science Foundation (grant number 315230-179264) and by the Institute of Microbiology (IM) of the University of Applied Sciences and Arts of Southern Switzerland (SUPSI). The quality of the scientific equipment used during monitoring was made possible by cantonal funding for the mandate “Indagini, perizie e consulenza in Microbiologia ambientale” of the Department of “socialità e sanità” (DSS). AS thanks the ATTRACT Investigator Grant (No. A17/MS/11572821/MBRACE) and FNR-CORE Grant (No. C19/MS/13719464/TOPOFLUME/Sengupta) from the Luxembourg National Research Fund for supporting this work.
Acknowledgments
We thank G. Ranieri and M. Thelen for technical assistance with 14C experiment analyses; M. Tonolla and F. Danza for fruitful discussion and constructive comments on the manuscript; the Alpine Biology Centre Foundation (CBA) for laboratory facilities and housing.
Conflict of interest
The authors declare that the research was conducted in the absence of any commercial or financial relationships that could be construed as a potential conflict of interest.
Publisher’s note
All claims expressed in this article are solely those of the authors and do not necessarily represent those of their affiliated organizations, or those of the publisher, the editors and the reviewers. Any product that may be evaluated in this article, or claim that may be made by its manufacturer, is not guaranteed or endorsed by the publisher.
Supplementary material
The Supplementary material for this article can be found online at: https://www.frontiersin.org/articles/10.3389/fmicb.2023.1253009/full#supplementary-material
Footnotes
References
Abe, T., Nakamura, S., and Kudo, S. (2017). Bioconvection induced by bacterial chemotaxis in a capillary assay. Biochem. Biophys. Res. Commun. 483, 277–282. doi: 10.1016/j.bbrc.2016.12.152
Andrei, A. Ş., Robeson, M. S., Baricz, A., Coman, C., Muntean, V., Ionescu, A., et al. (2015). Contrasting taxonomic stratification of microbial communities in two hypersaline meromictic lakes. ISME J. 9, 2642–2656. doi: 10.1038/ismej.2015.60
Ardekani, A. M., Doostmohammadi, A., and Desai, N. (2017). Transport of particles, drops, and small organisms in density stratified fluids. Phys. Rev. Fluids 2:100503. doi: 10.1103/PhysRevFluids.2.100503
Barnett, A., Méléder, V., Dupuy, C., and Lavaud, J. (2020). The vertical migratory rhythm of intertidal microphytobenthos in sediment depends on the light photoperiod, intensity, and Spectrum: evidence for a positive effect of blue wavelengths. Front. Mar. Sci. 7:212. doi: 10.3389/FMARS.2020.00212/BIBTEX
Bearon, R. N., and Grünbaum, D. (2006). Bioconvection in a stratified environment: experiments and theory. Phys. Fluids 18, 1–14. doi: 10.1063/1.2402490
Bees, M. A. (2020, 2020). Advances in bioconvection. Annu. Rev. Fluid Mech. 52, 449–476. doi: 10.1146/annurev-fluid-010518
Berg, J., Pjevac, P., Sommer, T., Buckner, C. R. T., Philippi, M., Hach, P. F., et al. (2019). Dark aerobic sulfide oxidation by anoxygenic phototrophs in anoxic waters. Environ. Microbiol. 21, 1611–1626. doi: 10.1111/1462-2920.14543
Block, K. R., O'Brien, J. M., Edwards, W. J., and Marnocha, C. L. (2021). Vertical structure of the bacterial diversity in meromictic Fayetteville Green Lake. Microbiol. Open 10:e1228. doi: 10.1002/MBO3.1228
Bolick, L. (2022). ‘A microbial whale’ – the giant ciliate Spirostomum teres feeding on phototrophic sulfur bacteria in Lake Cadagno (Ticino). Master thesis, Zürich University of Zürich.
Bouffard, D., and Wüest, A. (2019). Convection in lakes. Annu. Rev. Fluid Mech. 51, 189–215. doi: 10.1146/annurev-fluid-010518-040506
Brune, D. C. (1989). Sulfur oxidation by phototrophic bacteria. Biochim. Biophys. Acta 975, 189–221. doi: 10.1016/S0005-2728(89)80251-8
Casamayor, E. O., García-Cantizano, J., and Pedrós-Alió, C. (2008). Carbon dioxide fixation in the dark by photosynthetic bacteria in sulfide-rich stratified lakes with oxic-anoxic interfaces. Limnol. Oceanogr. 53, 1193–1203. doi: 10.4319/lo.2008.53.4.1193
Casamayor, E. O., Llirós, M., Picazo, A., Barberán, A., Borrego, C. M., and Camacho, A. (2012). Contribution of deep dark fixation processes to overall CO2 incorporation and large vertical changes of microbial populations in stratified karstic lakes. Aquat. Sci. 74, 61–75. doi: 10.1007/s00027-011-0196-5
Danza, F. (2018) Flow cytometry as a tool to investigate the anoxygenic phototrophic sulfur bacteria coexistence in the chemocline of meromictic Lake Cadagno. Geneva Université de Genève, 12
Danza, F., Ravasi, D., Storelli, N., Roman, S., Lüdin, S., Bueche, M., et al. (2018). Bacterial diversity in the water column of meromictic Lake Cadagno and evidence for seasonal dynamics. PLoS One 13, e0209743–e0209717. doi: 10.1371/journal.pone.0209743
Danza, F., Storelli, N., Roman, S., Lüdin, S., and Tonolla, M. (2017). Dynamic cellular complexity of anoxygenic phototrophic sulfur bacteria in the chemocline of meromictic Lake Cadagno. PLoS One 12, e0189510–e0189517. doi: 10.1371/journal.pone.0189510
Decristophoris, P. M. A., Peduzzi, S., Ruggeri-Bernardi, N., Hahn, D., and Tonolla, M. (2009). Fine scale analysis of shifts in bacterial community structure in the chemocline of meromictic Lake Cadagno, Switzerland. J. Limnol. 68, 16–24. doi: 10.4081/jlimnol.2009.16
Dewar, W. K., Bingham, R. J., Iverson, R. L., Nowacek, D. P., St. Laurent, L. C., and Wiebe, P. H. (2006). Does the marine biosphere mix the ocean? J. Mar. Res. 64, 541–561. doi: 10.1357/002224006778715720
Di Nezio, F., Beney, C., Roman, S., Danza, F., Buetti-Dinh, A., Tonolla, M., et al. (2021). Anoxygenic photo- and chemo-synthesis of phototrophic sulfur bacteria from an alpine meromictic lake. FEMS Microbiol. Ecol. 97, 1–17. doi: 10.1093/femsec/fiab010
Di Nezio, F., Ong, I. L. H., Riedel, R., Goshal, A., Dhar, J., Roman, S., et al. (2023). Synergistic phenotypic shifts during domestication promote plankton-to-biofilm transition in purple sulfur bacterium Chromatium okenii. bioRxiv [Preprint]. Available at: https://www.biorxiv.org/content/10.1101/2023.10.20.563228v1 (Accessed October 20, 2023).
Eichler, B., and Pfennig, N. (1988). A new purple sulfur bacterium from stratified freshwater lakes, Amoebobacter purpureus sp. nov. Arch. Microbiol. 149, 395–400. doi: 10.1007/BF00425577
Findlay, A. J., Di Toro, D. M., and Luther, G. W. (2017). A model of phototrophic sulfide oxidation in a stratified estuary. Limnol. Oceanogr. 62, 1853–1867. doi: 10.1002/lno.10539
Fitt, W. K., and Trench, R. K. (1983). The relation of diel patterns of cell division to diel patterns of motility in the symbiotic dinoflagellate Symbiodinium microadriaticum Freudenthal in culture. New Phytol. 94, 421–432. doi: 10.1111/j.1469-8137.1983.tb03456.x
Frigaard, N. U., and Bryant, D. A. (2006). “Chlorosomes: antenna organelles in photosynthetic green Bacteria” in Complex intracellular structures in prokaryotes. ed. J. M. Shively (Berlin Heidelberg: Springer), 79–114.
Frigaard, N. U., and Dahl, C. (2008). Sulfur metabolism in phototrophic sulfur bacteria. Adv. Microb. Physiol. 54, 103–200. doi: 10.1016/S0065-2911(08)00002-7
Frigaard, N. U., Gomez Maqueo Chew, A., Li, H., Maresca, J. A., and Bryant, D. A. (2003). Chlorobium Tepidum: insights into the structure, physiology, and metabolism of a green sulfur bacterium derived from the complete genome sequence. Photosynth. Res. 78, 93–117. doi: 10.1023/B:PRES.0000004310.96189.B4
Gächter, R., and Marès, A. (1979). Comments to the acidification and bubbling method for determining phytoplankton production. Oikos 33, 69–73. doi: 10.2307/3544513
Ghorai, S., and Panda, M. K. (2013). Bioconvection in an anisotropic scattering suspension of phototactic algae. Eur. J. Mechan. B/Fluids 41, 81–93. doi: 10.1016/J.EUROMECHFLU.2012.07.001
Gulati, R., Zadereev, E., and Degermendzhi, A. (2017) in Ecology of meromictic lakes. eds. R. D. Gulati, E. S. Zadereev, and A. G. Degermendzhi (Cham (CH): Springer International Publishing), 398.
Habicht, K. S., Miller, M., Cox, R. P., Frigaard, N. U., Tonolla, M., Peduzzi, S., et al. (2011). Comparative proteomics and activity of a green sulfur bacterium through the water column of Lake Cadagno, Switzerland. Environ. Microbiol. 13, 203–215. doi: 10.1111/j.1462-2920.2010.02321.x
Hesketh-Best, P. J., Bosco-Santos, A., Garcia, S. L., O’Beirne, M. D., Werne, J. P., Gilhooly, W. P. III, et al. (2023). Viruses of sulfur oxidizing phototrophs encode genes for pigment, carbon, and sulfur metabolisms. Commun. Earth Environ. 4, 1–12. doi: 10.1038/s43247-023-00796-4
Hill, N. A., and Pedley, T. J. (2005). Bioconvection. Fluid Dynam. Res. 37, 1–20. doi: 10.1016/J.FLUIDDYN.2005.03.002
Jin, D., Kotar, J., Silvester, E., Leptos, K. C., and Croze, O. A. (2020). Diurnal variations in the motility of populations of biflagellate microalgae. Biophys. J. 119, 2055–2062. doi: 10.1016/J.BPJ.2020.10.006
Johar Gunawan, T., Ikhwan, Y., Restuhadi, F., and Pato, U. (2018). Effect of light intensity and photoperiod on growth of Chlorella pyrenoidosa and CO2 biofixation. E3S Web Conferen. 31:3003. doi: 10.1051/E3SCONF/20183103003
Katija, K., and Dabiri, J. O. (2009). A viscosity-enhanced mechanism for biogenic ocean mixing. Nature 460, 624–626. doi: 10.1038/nature08207
Kils, U. (1993). Formation of micropatches by zooplankton-driven microturbulences. Bull. Mar. Sci. 53, 160–169.
Kunze, E., Dower, J. F., Beveridge, I., Dewey, R., and Bartlett, K. P. (2006). Observations of biologically generated turbulence in a coastal inlet. Science 313, 1768–1770. doi: 10.1126/SCIENCE.1129378
Lerch, K. A., and Cook, C. B. (1984). Some effects of photoperiod on the motility rhytm of cultured zooxanthellae. Bull. Mar. Sci. 34, 477–483.
Lin, Z., Thiffeault, J. L., and Childress, S. (2011). Stirring by squirmers. J. Fluid Mech. 669, 167–177. doi: 10.1017/S002211201000563X
Luedin, S. M., Liechti, N., Cox, R. P., Danza, F., Frigaard, N. U., Posth, N. R., et al. (2019a). Draft genome sequence of Chromatium okenii isolated from the stratified alpine Lake Cadagno. Sci. Rep. 9, 1936–1914. doi: 10.1038/s41598-018-38202-1
Luedin, S. M., Pothier, J. F., Danza, F., Storelli, N., Frigaard, N. U., Wittwer, M., et al. (2018). Complete genome sequence of “Thiodictyon syntrophicum” sp. nov. strain Cad16 T, a photolithoautotrophic purple sulfur bacterium isolated from the alpine meromictic Lake Cadagno. Stand. Genomic Sci. 13, 14–13. doi: 10.1186/s40793-018-0317-z
Luedin, S. M., Storelli, N., Danza, F., Roman, S., Wittwer, M., Pothier, J. F., et al. (2019b). Mixotrophic growth under micro-oxic conditions in the purple sulfur bacterium “Thiodictyon syntrophicum.”. Front. Microbiol. 10:384. doi: 10.3389/fmicb.2019.00384
Musat, N., Halm, H., Winterholler, B., Hoppe, P., Peduzzi, S., Hillion, F., et al. (2008). A single-cell view on the ecophysiology of anaerobic phototrophic bacteria. Proc. Natl. Acad. Sci. U. S. A. 105, 17861–17866. doi: 10.1073/pnas.0809329105
Ni, B., Colin, R., Link, H., Endres, R. G., and Sourjik, V. (2020). Growth-rate dependent resource investment in bacterial motile behavior quantitatively follows potential benefit of chemotaxis. Proc. Natl. Acad. Sci. U. S. A. 117, 595–601. doi: 10.1073/PNAS.1910849117/SUPPL_FILE/PNAS.1910849117.SD04.TXT
Ochiai, N., DragIila, M. I., and Parke, J. L. (2011). Pattern swimming of Phytophthora citricola zoospores: an example of microbial bioconvection. Fungal Biol. 115, 228–235. doi: 10.1016/J.FUNBIO.2010.12.006
Overmann, J. (2006). The family chlorobiaceae. Prokaryotes 7, 359–378. doi: 10.1007/0-387-30747-8_13
Paul, J. H., Kang, J. B., and Tabita, F. R. (2000). Diel patterns of regulation of rbcL transcription in a cyanobacterium and a prymnesiophyte. Mar. Biotechnol. 2, 429–436. doi: 10.1007/S101260000016/METRICS
Peduzzi, S., Storelli, N., Welsh, A., Peduzzi, R., Hahn, D., Perret, X., et al. (2012). Candidatus “Thiodictyon syntrophicum”, sp. nov., a new purple sulfur bacterium isolated from the chemocline of Lake Cadagno forming aggregates and specific associations with Desulfocapsa sp. Syst. Appl. Microbiol. 35, 139–144. doi: 10.1016/j.syapm.2012.01.001
Peduzzi, S., Welsh, A., Demarta, A., Decristophoris, P., Peduzzi, R., Hahn, D., et al. (2011). Thiocystis chemoclinalis sp. nov. and Thiocystis cadagnonensis sp. nov., motile purple sulfur bacteria isolated from the chemocline of a meromictic lake. Int. J. Syst. Evol. Microbiol. 61, 1682–1687. doi: 10.1099/ijs.0.010397-0
Pfennig, N., Höflin, K.-H., and Kusmierz, H. (1968). “Chromatium okenii (Thiorhodaceae) Biokonvektion, aero- und phototaktisches Verhalten” in Encyclopedia Cinematographica. doi: 10.3203/IWF/E-1036
Philippi, M., Kitzinger, K., Berg, J. S., Tschitschko, B., Kidane, A. T., Littmann, S., et al. (2021). Purple sulfur bacteria fix N2 via molybdenumnitrogenase in a low molybdenum Proterozoic Ocean analogue. Nat. Commun. 12, 1–12. doi: 10.1038/s41467-021-25000-z
Posth, N. R., Bristow, L. A., Cox, R. P., Habicht, K. S., Danza, F., Tonolla, M., et al. (2017). Carbon isotope fractionation by anoxygenic phototrophic bacteria in euxinic Lake Cadagno. Geobiology 15, 798–816. doi: 10.1111/gbi.12254
Ravasi, D. F., Peduzzi, S., Guidi, V., Peduzzi, R., Wirth, S. B., Gilli, A., et al. (2012). Development of a real-time PCR method for the detection of fossil 16S rDNA fragments of phototrophic sulfur bacteria in the sediments of Lake Cadagno. Geobiology 10, 196–204. doi: 10.1111/j.1472-4669.2012.00326.x
Richter, P. R., Strauch, S. M., Ntefidou, M., Schuster, M., Daiker, V., Nasir, A., et al. (2014). Influence of different light-dark cycles on motility and photosynthesis of Euglena gracilis in closed bioreactors. Astrobiology 14, 848–858. doi: 10.1089/AST.2014.1176
Rogozin, D. Y., Trusova, M. Y., Khromechek, E. B., and Degermendzhy, A. G. (2010). Microbial community of the chemocline of the meromictic Lake Shunet (Khakassia, Russia) during summer stratification. Microbiology 79, 253–261. doi: 10.1134/S0026261710020189/METRICS
Saini, J. S., Hassler, C., Cable, R., Fourquez, M., Danza, F., Roman, S., et al. (2022). Bacterial, phytoplankton, and viral distributions and their biogeochemical contexts in meromictic Lake Cadagno offer insights into the proterozoic ocean microbial loop. MBio 13:e0005222. doi: 10.1128/MBIO.00052-22/SUPPL_FILE/MBIO.00052-22-S0002.PDF
Sepúlveda Steiner, O. R., Bouffard, D., and Wüest, A. (2019). Convection-diffusion competition within mixed layers of stratified natural waters. Geophys. Res. Lett. 46, 13199–13208. doi: 10.1029/2019GL085361
Sepúlveda Steiner, O. R., Bouffard, D., and Wüest, A. (2021). Persistence of bioconvection-induced mixed layers in a stratified lake. Limnol. Oceanogr. 66, 1531–1547. doi: 10.1002/lno.11702
Shoup, D., and Ursell, T. (2021). Bacterial bioconvection confers context-dependent growth benefits and is robust under varying metabolic and genetic conditions. bio Rxiv :2021.04.20.440374. doi: 10.1101/2021.04.20.440374
Sommer, T., Danza, F., Berg, J., Sengupta, A., Constantinescu, G., Tokyay, T., et al. (2017). Bacteria-induced mixing in natural waters. Geophys. Res. Lett. 44, 9424–9432. doi: 10.1002/2017GL074868
Storelli, N., Peduzzi, S., Saad, M. M., Frigaard, N. U., Perret, X., and Tonolla, M. (2013). CO2 assimilation in the chemocline of Lake Cadagno is dominated by a few types of phototrophic purple sulfur bacteria. FEMS Microbiol. Ecol. 84, 421–432. doi: 10.1111/1574-6941.12074
Tam, D., and Hosoi, A. E. (2011). Optimal feeding and swimming gaits of biflagellated organisms. Proc. Natl. Acad. Sci. U. S. A. 108, 1001–1006. doi: 10.1073/PNAS.1011185108/ASSET/BBE295AA-A7F8-42CA-A4B7-61ED1400332B/ASSETS/GRAPHIC/PNAS.1011185108EQ72.GIF
Tang, K. H., and Blankenship, R. E. (2010). Both forward and reverse TCA cycles operate in green sulfur bacteria. J. Biol. Chem. 285, 35848–35854. doi: 10.1074/jbc.M110.157834
Tonolla, M., Demarta, A., Peduzzi, S., Hahn, D., and Peduzzi, R. (2000). In situ analysis of sulfate-reducing bacteria related to Desulfocapsa thiozymogenes in the chemocline of meromictic Lake Cadagno (Switzerland). Appl. Environ. Microbiol. 66, 820–824. doi: 10.1128/AEM.66.2.820-824.2000
Tonolla, M., Peduzzi, R., and Hahn, D. (2005). Long-term population dynamics of phototrophic sulfur bacteria in the chemocline of Lake Cadagno, Switzerland. Appl. Environ. Microbiol. 71, 3544–3550. doi: 10.1128/AEM.71.7.3544
Tonolla, M., Peduzzi, S., Hahn, D., and Peduzzi, R. (2003). Spatio-temporal distribution of phototrophic sulfur bacteria in the chemocline of meromictic Lake Cadagno (Switzerland). FEMS Microbiol. Ecol. 43, 89–98. doi: 10.1016/S0168-6496(02)00354-9
Tonolla, M., Storelli, N., Danza, F., Ravasi, D., Peduzzi, S., Posth, N. R., et al. (2017). “Lake Cadagno: microbial life in Crenogenic Meromixis” in Ecology of meromictic lakes. eds. R. Gulati, E. S. Zadereev, and A. Degermendzhi (Cham: Springer International Publishing), 155–186.
Tuval, I., Cisneros, L., Dombrowski, C., Wolgemuth, C. W., Kessler, J. O., and Goldstein, R. E. (2005). Bacterial swimming and oxygen transport near contact lines. Proc. Natl. Acad. Sci. U. S. A. 102, 2277–2282. doi: 10.1073/pnas.0406724102
Williams, C. R., and Bees, M. A. (2011). A tale of three taxes: photo-gyro-gravitactic bioconvection. J. Exp. Biol. 214, 2398–2408. doi: 10.1242/JEB.051094
Wyman, M. (1999). Diel rhythms in ribulose-1, 5-bisphosphate carboxylase/oxygenase and glutamine synthetase gene expression in a natural population of marine picoplanktonic cyanobacteria (Synechococcus spp.). Appl. Environ. Microbiol. 65, 3651–3659. doi: 10.1128/AEM.65.8.3651-3659.1999/ASSET/BB10E355-6789-44E2-9643-E1A8EBC70A14/ASSETS/GRAPHIC/AM0890383005.JPEG
Xu, K., Zou, X., Xue, Y., Qu, Y., and Li, Y. (2021). The impact of seasonal variations about temperature and photoperiod on the treatment of municipal wastewater by algae-bacteria system in lab-scale. Algal Res. 54:102175. doi: 10.1016/J.ALGAL.2020.102175
Yanaoka, H., and Nishimura, T. (2022). Pattern wavelengths and transport characteristics in three-dimensional bioconvection generated by chemotactic bacteria. J. Fluid Mech. 952:A13. doi: 10.1017/jfm.2022.898
Keywords: bioconvection, meromixis, Lake Cadagno, sulfur bacteria, eco-physiology
Citation: Di Nezio F, Roman S, Buetti-Dinh A, Sepúlveda Steiner O, Bouffard D, Sengupta A and Storelli N (2023) Motile bacteria leverage bioconvection for eco-physiological benefits in a natural aquatic environment. Front. Microbiol. 14:1253009. doi: 10.3389/fmicb.2023.1253009
Edited by:
Joshua A. Steele, Southern California Coastal Water Research Project, United StatesReviewed by:
Katharine J. Thompson, University of Stuttgart, GermanyAlice Bosco Santos, Université de Lausanne, Switzerland
Copyright © 2023 Di Nezio, Roman, Buetti-Dinh, Sepúlveda Steiner, Bouffard, Sengupta and Storelli. This is an open-access article distributed under the terms of the Creative Commons Attribution License (CC BY). The use, distribution or reproduction in other forums is permitted, provided the original author(s) and the copyright owner(s) are credited and that the original publication in this journal is cited, in accordance with accepted academic practice. No use, distribution or reproduction is permitted which does not comply with these terms.
*Correspondence: Francesco Di Nezio, ZnJhbmNlc2NvLmRpbmV6aW9Ac3Vwc2kuY2g=; Nicola Storelli, bmljb2xhLnN0b3JlbGxpQHN1cHNpLmNo