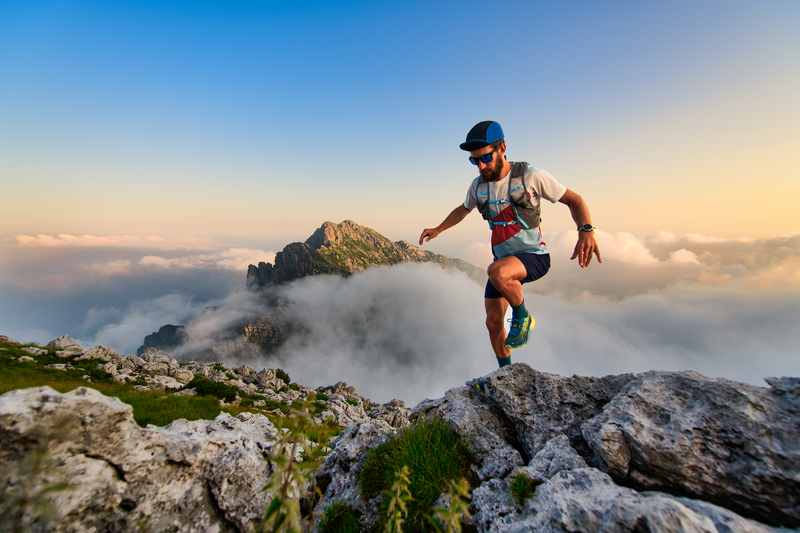
95% of researchers rate our articles as excellent or good
Learn more about the work of our research integrity team to safeguard the quality of each article we publish.
Find out more
ORIGINAL RESEARCH article
Front. Microbiol. , 23 October 2023
Sec. Microbe and Virus Interactions with Plants
Volume 14 - 2023 | https://doi.org/10.3389/fmicb.2023.1251602
Introduction: This work investigates whether rhizosphere microorganisms that colonize halophyte plants thriving in saline habitats can tolerate salinity and provide beneficial effects to their hosts, protecting them from environmental stresses, such as aromatic compound (AC) pollution.
Methods: To address this question, we conducted a series of experiments. First, we evaluated the effects of phenol, tyrosine, 4-hydroxybenzoic acid, and 2,4-dichlorophenoxyacetic (2,4-D) acids on the soil rhizosphere microbial community associated with the halophyte Allenrolfea vaginata. We then determined the ability of bacterial isolates from these microbial communities to utilize these ACs as carbon sources. Finally, we assessed their ability to promote plant growth under saline conditions.
Results: Our study revealed that each AC had a different impact on the structure and alpha and beta diversity of the halophyte bacterial (but not archaeal) communities. Notably, 2,4-D and phenol, to a lesser degree, had the most substantial decreasing effects. The removal of ACs by the rhizosphere community varied from 15% (2,4-D) to 100% (the other three ACs), depending on the concentration. Halomonas isolates were the most abundant and diverse strains capable of degrading the ACs, with strains of Marinobacter, Alkalihalobacillus, Thalassobacillus, Oceanobacillus, and the archaea Haladaptatus also exhibiting catabolic properties. Moreover, our study found that halophile strains Halomonas sp. LV-8T and Marinobacter sp. LV-48T enhanced the growth and protection of Arabidopsis thaliana plants by 30% to 55% under salt-stress conditions.
Discussion: These results suggest that moderate halophile microbial communities may protect halophytes from salinity and potential adverse effects of aromatic compounds through depurative processes.
Aromatic compounds (AC) derived from agro-industrial activities may contaminate saline and hypersaline environments (Fathepure, 2014). Most of these compounds have impacts on animals (including humans), plants, and environmental health because they are toxic, mutagenic, and carcinogenic (Honda and Suzuki, 2020; Krzyszczak and Czech, 2021; Molina and Segura, 2021). Although many microorganisms can degrade AC through both anaerobic and, most frequently, aerobic pathways, cleaving the aromatic ring into Krebs’s cycle intermediate metabolism (Pérez-Pantoja et al., 2010a), it is clear that salinity is one of the main factors that affect AC degradation performance (Li et al., 2019; Mainka et al., 2021). However, halophilic microorganisms from diverse saline environments possess AC degradation capabilities (Bonfá et al., 2013; Li et al., 2019). Moderate halophiles have thus been used to remediate contamination by AC at mild saline concentrations (Ma et al., 2010; Veenagayathri and Vasudevan, 2015; Guo et al., 2016). Some extreme halophiles have also been recommended for bioremediation of saline environments polluted with AC (Arora et al., 2017; Aracil-Gisbert et al., 2018). The application of whole halophilic microbial communities or consortia to degrade xenobiotic compounds under saline and hypersaline conditions has also been proposed (Aracil-Gisbert et al., 2018; Kimbrel et al., 2018).
Halophilic microorganisms that degrade AC belong to different taxa; however, the Pseudomonadota family Halomonadaceae, which mainly comprises marine and moderate halophiles, has been identified as the most diverse group able to degrade phenol and phenol by-products under moderately halophilic conditions (Bonfá et al., 2013; Al Farraj et al., 2020). In addition, species belonging to Bacillaceae (phylum Bacillota), Micrococcaceae, Nocardiaceae, and Dermabacteraceae (phylum Actinomycetota) have also shown potential for bioremediation of organic pollutants (Li et al., 2019). All these halophiles may thrive in environments contaminated by AC (Álvarez and Silva, 2013). Some of these halophilic AC degrading groups have also been associated with plant growth promotion (Mora-Ruiz et al., 2015; Marasco et al., 2016; Sáenz-Mata et al., 2016). Other plant growth promotion rhizobacteria (PGPR) and microbial consortia could degrade organic pollutants found in soil and make plants more tolerant to such abiotic stressors (Chaudhary et al., 2023). Some PGPR strains can protect plants from saline stress (Pinedo et al., 2015). Such beneficial plant-bacteria interactions may be affected by AC (Sampedro et al., 2020). Therefore, identifying and studying halophilic rhizosphere microorganisms able to degrade AC is essential to increase the understanding of how the halophilic microbial community can enhance plant growth and exhibit health protection capacities.
Allenrolfea vaginata, belonging to the family Amaranthaceae, subfamily Salicornioideae (Mora-Ruiz et al., 2015), represents a small group of halophytes that play an essential role in the preservation of coastal ecosystems due to their high salt tolerance and their specialized associated microbiota (Jafari et al., 2012). Salinity-tolerant rhizosphere microorganisms associated with Amaranthaceae species have an essential role in enhancing growth and salt tolerance in this halophyte (Jafari et al., 2012; Mora-Ruiz et al., 2015), and some of these microorganisms have been proposed as biofertilizers for improving the growth of the host-plant in saline environments (Jafari et al., 2012; Mora-Ruiz et al., 2015; Razzaghi Komaresofla et al., 2019). It is worth noting that tolerance to extreme saline conditions varies from one plant species to another, and the impact level of such abiotic stress on growth and development is species-dependent (Sardar et al., 2023). Advances have also been described in the use of halophiles to protect and promote the growth of non-halophyte commercial crops such as tomatoes (Sahu et al., 2023) and rice (Mallick et al., 2018; Kapadia et al., 2022). However, information about rhizosphere microorganisms colonizing halophytes and non-halophytes and their biotechnological potential is still scarce (Sáenz-Mata et al., 2016; Mahmood et al., 2019).
To contribute to the knowledge of catabolic and plant growth promotion abilities of A. vaginata-associated microbiota, the effects on the A. vaginata rhizosphere bacterial and archaeal communities of four low molecular weight AC are reported here. The isolation and initial characterization of microorganisms removing these AC and their promoting plant growth properties under saline stress are also described. 2,4-dichlorophenoxyacetic acid (2,4-D), 4-hydroxybenzoic acid (4-HB), phenol (Phe), and tyrosine (Tyr) were chosen as these four ACs are commonly found in industrial wastewater and have been considered chemical models to study pollution effects and biodegradation capabilities (Pérez-Pantoja et al., 2008; Ning et al., 2015; Warren-Vega et al., 2023).
Soil samples were obtained in January 2013 from Lo Valdivia solar saltern, a sea-salt production site located in central Chile (34°41′50.16″S, 72°00′44.3″W), a place free of AC contamination from agro-industrial sources. Soil samples were collected at six points around the salt ponds (1–1.5 m from the pond edge), where A. vaginata was profusely present and thus defined as A. vaginata rhizosphere soil samples (Figure 1). Soil samples were collected to a maximum depth of 15 cm and stored in a plastic bag. The soil samples, free of root pieces and small stones, were mixed and homogenized and then passed through a 3 mm mesh to prepare the microcosms and perform chemical characterization. The latter was carried out in the Faculty of Agriculture and Forestry laboratory at the Pontificia Universidad Católica de Chile, following standard protocols (Robertson et al., 1999). A fresh, not sifted soil sample was used to isolate rhizosphere halophilic microorganisms.
Figure 1. (A) Lo Valdivia solar salterns localization and an aerial view of Lo Valdivia estuary. (B) Specific sample point on Lo Valdivia solar saltern. (C) Allenrolfea vaginata plants found on the site. The red circle indicates the exact area of the sample site.
Eight sets of nine microcosms each were set up with homogenized rhizosphere soil samples as follows: 100 mL beakers were filled with 10 g of soil, covered with self-sealing Parafilm M (Bemis, WI, United States), and incubated at room temperature (25°C ± 2°C). Three sampling times were established (1, 15, and 30 days of incubation). At each sampling time, three microcosms from each set were sacrificed, 2 g homogenized soil samples from each microcosm were used for DNA extraction, and the remaining for HPLC analysis. Each microcosm was supplemented with 1 mL of a solution containing 2,4-D, 4-HB, Phe, or Tyr at 5- or 20-mM final concentration. In addition, two control microcosms were used: one treatment without any AC addition and another where only 1 mL of sterilized distilled water was added instead of AC.
Five grams of each saline soil microcosm were mixed with 15 mL of methanol in a conical bottom tube of 50 mL and stirred for 24 h at 200 rpm. The mixture was then centrifuged at 10,860 g for 45 min to recover the supernatant. The supernatant solution was filtered sequentially through 0.45 μm and 0.22 μm pore size filters. To determine the corresponding AC content, the final solution was analyzed by HPLC. HPLC was performed at the Universidad de Chile soil science laboratory in an instrument connected with a Waters 1525 HPLC binary pump and a Waters 2996 diode array. Samples were isocratically eluted using an aqueous solution of phosphoric acid (3%) at 1.5 mL min−1 flow and a mobile phase of 60:40 (methanol/water), using a Kromasil column 100–3.5C18, dimension 4.6 × 150 mm. Absorbance was measured at 228, 254, 211, and 274 nm to detect 2,4-D, 4-HB, Phe, and Tyr, respectively. Data were managed, visualized, and statistically analyzed using RStudio software (RStudio Team, 2020). Statistical analysis of aligned-rank transform (Wobbrock et al., 2011) was applied to detect significant differences among compound and time factors. “ARTool” package was used to perform ANOVA of aligned rank-transformed data (Elkin et al., 2021), and the packages “emmeans” (Lenth et al., 2018) and “multcomp” (Hothorn et al., 2008) were used to perform pairwise contrasts.
Molecular analysis of bacterial and archaeal communities was performed using metagenomic DNA isolated from 1 g of soil from each microcosm soil sample using the FastDNA SPIN Kit for Soil (MP Biomedicals, Santa Ana, California, United States) according to the manufacturer’s instructions. Amplification of 16S rRNA gene was carried out by polymerase chain reaction (PCR) using the primer pairs 63F (6-FAM-5′-AGGCCTAACACATGCAAGTC-3′) and 1087R (5′-CTCGTTGCGGGACTTACCCC-3′) for bacteria domain (Singh et al., 2006), and 21F (6-FAM-5′-TTCCGGTTGATCCTGCCGGA-3′) and 1492R (5′-GGTTACCTTGTTACGACTT-3′) for archaea domain (Waddell et al., 2010). Both forward primers were labeled with the fluorophore 6-carboxy-fluorescein (6-FAM) at the 5′ terminal end. The reaction mixture for the PCR amplification included 5 μL 10× PCR buffer (200 mM Tris-HCl, pH 8.4, 500 mM KCl), 3.5 μL of 25 mM MgCl2, 1 μL each of primer 63F/1087R (0.2 μM) for bacterial sequences and 21F/1492R (0.2 μM) for archaeal sequences, 1 μL of dNTP (0.2 mM), 1 μL of bovine serum albumin (0.5 mg mL−1), 0.4 μL of 1 U Taq DNA polymerase, 2 μL of environmental DNA, in a total reaction volume of 50 μL. Amplification was performed using the Applied Biosystems 2720 Thermocycler (Applied Biosystems, Foster City, CA, United States). Reactions were held at 95°C for 10 min to allow the DNA to denature, followed by 25 cycles at 95°C for 45 s, 56°C for 1 min, and 72°C for 2 min, followed by a final extension at 72°C for 7 min. PCR products were visualized by electrophoresis on a 1% (w/v) agarose gel using GelRed as the DNA intercalating agent. The PCR products were subjected to digestion with the restriction enzymes AluI and MspI at 37°C for three h, followed by 20 min inactivation at 80°C. After digestion, products were purified with 0.1 vol of 3 M sodium acetate and 2.5 vol 100% ethanol and centrifuged at 26,290 g for 30 min at 4°C. After washing with 100 μL of 70% ethanol and centrifuging, DNA was eluted using 10 μL of ultra-pure water. Restriction fragment size analysis was performed through capillary electrophoresis (Macrogen Inc., Korea). Data from profiles of the restriction fragments were read in Peak Scanner version 1.0 Software (Applied Biosystems) using the standard marker 1200 LIZ and exported as a text format archive. Exported data were read and managed using RStudio software (RStudio Team, 2020). Only restriction fragments between 50 and 500 bp were considered for analysis. Fragments comprising less than 1% relative abundance were excluded from the study. The data were standardized by calculating the area of each peak as a percentage of the total area (Osborne et al., 2006). Using the R-package vegan (Oksanen et al., 2020), data were square root transformed, and the Bray–Curtis distance was calculated. The similarity matrix was used to represent the data with an NMDS (Clarke, 1993), and treatment clusters were highlighted using the ordiellipse function. Each compound/concentration cluster was highlighted using the ordispider function of the R package vegan (version 2.5–7) (Oksanen et al., 2020). The ANOSIM test, equivalent to a non-parametric ANOVA, was used to test similarity distances. Ecological diversity was also calculated with the Shannon–Weaver index (H′ = S pi * ln (pi)) and richness index (S) as the total number of OTUs found (Shannon, 1948; Blackwood et al., 2007).
To isolate halophilic microorganisms capable of AC removal, 2 g of saline soil were mixed in a 500 mL flask with 100 mL of the modified HM liquid medium (Ventosa et al., 1982). This medium (HTM) contained 117 g of NaCl, 29.7 g of MgSO4, 20.7 g MgCl2, 3 g KCl, 0.43 g CaCl2, 0.39 g NaBr, and 0.1 g HNaCO3 with 10 mL of 10× phosphate buffer and 10 mL of the micronutrient mix to make 1 L, adjusted to pH = 7.2. To isolate halophiles, HTM medium salt concentration was adjusted to 4.5% salinity by diluting the medium with distilled water. 2,4-D, 4-HB, Phe, or Tyr were added at a 5 mM concentration, and flasks were incubated for 1 month at 25°C. After that, aliquots of 100 μL of a 1:1000 cultures dilution were plated on an HTM medium containing 1.5% agar and 1 mM of the corresponding AC. The agar plates were incubated for 1 month at room temperature. Microorganisms showing visible growth and potentially degrading AC were selected and transferred to a new selective solid medium HTM (4.5% salinity, equivalent to ECsusp = 43.8, Table 1, based on the equation EC = 9.7381*Salinity + 0.9771; R2 = 0.9987) containing the respective compound.
Table 1. Chemical characterization of Allenrolfea vaginata rhizosphere soil from Lo Valdivia solar saltern.
One isolated colony was used as inoculum for liquid culture, using the same diluted HTM medium and the respective compound where each colony was originally cultured. An aliquot of 50 μL was taken when turbidity was observed and plated in a solid medium containing 500 mL of HTM medium plus 5 g tryptone, 2.5% yeast extract, and 1.5% agar. Forty-one isolated colonies were selected for taxonomical identification based on 16S ribosomal gene sequencing. A colony was taken and resuspended in 800 μL of Chelex100 (BioRad, Hercules, CA, United States) for DNA extraction. The mixture was incubated at 80°C for 30 min and immediately chilled on ice for 5 min. Each mix was centrifuged at 26,290 g for 15 min, and the genomic DNA supernatant was recovered. Genomic DNA extracts were amplified by the above PCR program for T-RFLP analysis, using no fluorescent labeled primer pairs 63F and 1087R or 21F and 1492R. The PCR products were sent to sequencing (Macrogen Inc., Korea). The sequences were analyzed using BLAST (NCBI database GenBank). The 16S rRNA gene sequences have been deposited in the GenBank database under accession numbers MZ322696- MZ322737.
To explore the ability of the isolates to grow on the four AC tested, HTM saline medium was prepared at 4.5% salinity, and ACs were added at 0.5, 1.0 or 2.0 mM final concentration. Non-inoculated, sterile cultures were used as controls. To determine the growth rates, cell concentrations were monitored at 600 nm (UV–VIS Spectrophotometer, Shimadzu, Kyoto, Japan) after 1, 3, 7, 14, 30, and 60 days of incubation at 200 rpm and 25°C. To quantify AC removal rates, 1 mL samples of each culture and controls were filtered with a 0.02 μm pore size filter and monitored by UV-spectrophotometry. Absorbance was measured at 228, 254, 211, and 274 nm to quantify 2,4-D 4-HB, Phe, and Tyr concentration, respectively, and removal percentages were estimated using the formula (([control] − [sample])/[control])*100. GraphPad Prism version 6.0.0 for Windows (GraphPad Software, San Diego, California United States, www.graphpad.com) was used to visualize data.
Five halophilic microorganisms were selected to evaluate their ability to protect A. thaliana plants (Col-0) under salinity conditions and AC chemical stress. A. thaliana seeds were obtained from the Arabidopsis Biological Resource Center (ABRC, Columbus, OH, United States). The selected isolates were Halomonas sp. LV-8T, Alkalihalabacillus sp. LV-39H, Thalassobacillus sp. LV-41P, and Marinobacter sp. LV-48T, representing the genera with the most significant number of isolates. The archaeal isolate Haladaptatus sp. LV-51T was also included as the only isolate belonging to this kingdom. A plant growth-promoting bacteria, Paraburkholderia phytofirmans PsJN (Poupin et al., 2013), was included as a positive control by its ability to promote the growth of A. thaliana under saline stress (Pinedo et al., 2015). Halophile isolates were routinely grown in (100 mM NaCl/10 mM CaCl2) saline R2A liquid medium, and P. phytofirmans PsJN was grown in the same medium without salt added. Cell suspensions from each inoculum were then collected and adjusted to approximately 108 colony-forming units per milliliter (CFU/mL), as determined by plate counting. Seeds were surface sterilized with 50% sodium hypochlorite 100% commercial laundry bleach containing 0.1% Tween 20, rinsed three times with sterile water, and kept at 4°C for 5 days to synchronize germination. Square Petri dishes were prepared with half-strength (Murashige and Skoog 1962) medium (MS½), 0.8% agar. To prepare the inoculated plates, the initial inoculum (108 CFU/mL) was homogenously diluted in MS½ 0.8% agar just before gelling to reach a final concentration of 104 CFU/mL of medium. This final inoculum size has been proven optimal for exploring plant growth promotion features (Poupin et al., 2013), but does not represent true environmental relative abundances. Sterilized and synchronized seeds were sown in the Petri dishes with MS½ medium inoculated or not with one strain. Plates were placed vertically in a growth chamber at 22°C with a photoperiod of 12/12 h (light/dark) for 7 days after transplant to specific conditions according to experimental design.
After 7 days of sowing (7 DAS), 16 A. thaliana seedlings per treatment were transplanted to a new square Petri dish containing the same volume and amount of agar without inoculation. Two treatments per strain were tested, one containing MS½ medium and a concentration of 100 mM NaCl plus 10 mM CaCl2 and another in which only MS½ medium was used. Three non-inoculated treatments (WO_MO treatments) were also run: one in the presence of AC, the other under saline conditions, and a third one combining both AC and salty conditions. Two plates per treatment with 15 seedlings each were incubated in a growth chamber under the same conditions described above for 14 days. At the end of the incubation period, the plates were photographed using a scanner (Epson, Perfection V600 Photo, Nagano, Japan), the root length was measured, and the number of leaves was counted with the naked eye. Subsequently, by analyzing the images taken on day 0 (7 DAS) and day 14 (21 DAS), plant growth was calculated as the difference in the rosette area between 0 and 14 days of incubation.
The effect of AC on the germination rate was tested by sterilizing and synchronizing 500 seeds and subjecting a fraction of these to different AC concentrations using the same medium and conditions described above. A concentration of 0.1 mM of phenol and 2,4-D, 0.5 mM of 4-HB, and Tyr were determined to be toxic to A. thaliana.
The rosette area was image analyzed using Adobe Photoshop Cs3 software. This software allows measurement of the number of pixels corresponding to the area of the Petri dish used (12 × 12 cm). Since all the images were captured at the same resolution and height, this parameter was used to estimate the rosette area, converting the number of pixels into cm2. Rosette area data was obtained from images taken on days 1 and 14.
This work aimed to improve the knowledge of the role of microbial communities associated with halophyte plants, focusing on the effects of ACs. Effects on microbial community structure were evaluated using saline soil microcosms exposed to these ACs and terminal restriction fragment length polymorphism (T-RFLP) fingerprinting analysis. AC removal was determined by high-performance liquid chromatography (HPLC). Conventional culture enrichment and isolation procedures were also used to obtain and preliminary characterize bacteria able to grow on these ACs. In vitro experiments explored the individual capacities of halophiles to promote the growth of Arabidopsis thaliana under saline stress conditions.
Allenrolfea vaginata rhizosphere soil possessed a high content of soluble anions and cations (Table 1), leading to 4.5% salinity. The most conspicuous anions presented were chlorides and sulfates, and cations such as Na+ and Mg2+ were the most abundant in this soil. At the same time, the organic matter was found in the normal range for agricultural soils (Table 1). In addition, the high electrical conductivity of the extract and suspension at neutral pH indicated that this soil might be classified as saline-sodic (Table 1).
Rhizosphere saline soil microcosms were spiked with five and 20 mM of 2,4-D, 4-HB, Phe, or Tyr and monitored on days 1, 15, and 30 using HPLC analysis to quantify soil concentration of each compound and calculate removal percentages (Figure 2). At day one no removal of these compounds was observed, while at day 15, almost all 4-HB and Tyr were removed, at both concentrations. Full removal levels at 5 mM and 20 mM were observed after 30 days for 4-HB (99.8 ± 0.1% and 99.6 ± 0.2%, respectively) and Tyr (98.4 ± 0.3% and 99.1 ± 0.3%, respectively). 2,4-D and Phe removal levels increased in time but were lower than the other two compounds. 2,4-D was the less-removed compound as maximum removal levels were 33.9 ± 7.0% and 14.9 ± 1.1% at 5 and 20 mM, respectively. Phe was significantly removed at 5 mM (93.1 ± 1.5%), but removal levels decreased at 20 mM (76.9 ± 2.3%). Nonparametric statistical analysis of variance of aligned rank transformed data (Wobbrock et al., 2011) confirmed that significant differences in removal were due to time and compound factors interaction at both concentrations 5 mM (F6, 24.0 = 114.726, p = 2.1852E−11) and 20 mM (F6, 24.0 = 87.062, p = 4.3228E−15). A post-hoc pairwise Tukey test showed that significant differences were associated with the non-removal of any compound at day one and removal differences in time of each compound under both compound concentration treatments (Figure 2).
Figure 2. Percent of aromatic compound removal in saline soil microcosms. Initial concentrations of xenobiotic compounds were 5 mM (A) and 20 mM (B). Standard deviations represented by the error bars were calculated using triplicate biological samples. Letters on top of each column represent statistical differences of post-hoc contrasts art-c test analysis using the Tukey method.
To assess the effects of AC on bacterial and archaeal communities, T-RFLP profiles of saline soil microcosms were used to calculate ecological indexes of diversity (H) and richness (S). S and H values for bacterial communities were consistently higher than archaeal communities (Table 2). Archaeal indexes showed almost no variation in time, with differences present only in one or two operational taxonomic units (OTU). The two control conditions not amended with AC displayed higher levels of S and H in archaeal communities than treated microcosms in archaeal communities. Compared with these two controls, bacterial communities were rapidly and strongly affected by the exposure to AC, increasing the number of OTU detected on day 1 (Table 2). When Phe was added, S decreased after 15 and 30 days of incubation. A similar pattern was observed with the H index (Table 2). 2,4-D effects on community indexes highly depended on the compound concentration (Table 2). A noticeable increase of S and H was observed at day 15 with 20 mM 2,4-D. Tyr and 4-HB treatments produced similar changes in community index changes.
Table 2. Richness (S) and Shannon diversity (H) indexes based on terminal restriction fragment length polymorphism profiles of microbial communities from saline soil microcosms exposed to 5 mM or 20 mM aromatic compounds.
The effects of these ACs on the changes in the structure of the saline soil rhizosphere bacterial and archaeal communities were further studied. Non-metric multidimensional scaling (NMDS) comparison of the 16S rRNA gene T-RFLP profiles showed a clear grouping of samples according to treatment (Figures 3A,B). Phe and 2,4-D treatments presented higher dispersion in the NMDS ordering. Particularly noticeable, the 5 mM 2,4-D treatment showed a more substantial dispersion, mainly in archaeal communities (Figure 3A). The finding that the compound component is the more significant dissimilarity factor was confirmed by the analysis of similarity (ANOSIM), achieving a global R of 0.7433 (p-value = 0.001) for bacterial communities, and 0.7802 (p-value = 0.001) for archaeal communities. The R statistic parameter indicates the strength of the difference where 1 is the strongest and 0 is the weakest, representing significant differences between treated communities. T-RFLP profiles from non-treatment or water-only treatment were also clearly different (Figure 3). The results indicated that the increase of 2,4-D concentration had a more significant effect on archaeal community composition (Figure 3A open and solid diamonds) than on bacterial community (Figure 3B). This phenomenon of compositional divergence produced by different concentrations was not observed with the other compounds (Figure 3). On the other hand, there were no signs of resilience within the 30 days, as each compound generated compositional changes that, in general, accentuated through time (Figure 3, black symbols). The pairwise analysis showed that two controls (non-treatment & water-only) presented R values of 0.667 and 0.556 for archaea and bacteria, respectively (Table 3), which indicates that water addition had a low effect on microbial community structure changes. These two controls showed different results at 15 and 30 days, as R values were 1 and 0.481 in archaeal and 0.296 and 0 for bacterial communities, respectively (Table 3). This indicates that the bacterial community structural differences between no-treatment and water-only treatment decreased over time, while archaeal community structural differences peaked at day 15. Bacterial communities exposed to AC showed a fast change in composition after each compound was added (compare treatments and controls), and the amended compound mainly explained the observed differences.
Figure 3. Comparisons of (A) archaeal and (B) bacterial community composition from saline soil microcosm experiments assessed by non-metric multidimensional scaling of MspI-terminal restriction fragment length polymorphisms (T-RFLP) of the 16S rRNA gene analysis. Each symbol corresponds to a single T-RFLP profile representing one of the three replicates from a single community structure at day 1 (blue symbols), day 15 (red symbols), and day 30 (black symbols), exposed to 5 mM (open diamonds) or 20 mM (solid diamonds) 2,4-D; to 5 mM (open triangles) or 20 mM (solid triangles) 4-HB; to 5 mM (open circles) or 20 mM (solid circles) Phe, and 5 mM (open squares) or 20 mM (solid squares) Tyr. T-RFLP profiles from microcosm controls without treatment (*) or without aromatic compound, water-only treatment (x), are also shown. Ellipses and lines were drawn using ordiellipse and ordispider functions from the vegan package.
Microorganisms from the rhizospheric saline soil were tested for their ability to remove/degrade ACs. Forty-one isolates from A. vaginata rhizosphere saline soil were obtained using a solid saline medium containing one of the four AC as a sole carbon and energy source. 16S rRNA sequencing analysis revealed that the 41 isolates corresponded to unique 16S rRNA sequences having more than 1% of sequence dissimilarity between the closest sequence of the isolated microorganisms (Figure 4). From these 41 isolates, Halomonas genus was the most represented (21 isolates), of which 16 isolates corresponded to the H. zincidurans species and five isolates corresponded to possibly new Halomonas species, closely related to each other (Figure 4). The isolates included also six possible new species of Alkalihalobacillus, five likely new species of Marinobacter, three potential new species of Oceanobacillus, two isolates closely related to Priestia filamentosa, one isolate closely related to Rossellomorea vietnamensis and possible new species of Halobacillus, Thalassobacillus and Haladaptatus (one isolate each), the latter being the only archaeal isolate obtained (Figure 4). It should be noted that a cautious expression about possible new species was used as only part (about 1,000 bp) of the 16S rRNA sequence was obtained. Among the 41 isolates, 22 were isolated using Tyr, nine using 4-HB, five using Phe, and five using 2,4-D as a sole carbon and energy source.
Figure 4. Phylogenetic analysis of the isolates from Allenrolfea vaginata rhizosphere soil, based on 16S rRNA gene sequence, showing the taxonomic relationship between halophile bacteria and archaea isolates. The tree was constructed using the maximum likelihood method. Sequences for analysis were obtained from GenBank. Bootstrap values are shown next to the branches and were calculated as a percentage of 100 replicates. Isolated microorganism labels indicate the sample site (Lo Valdivia), the isolate number, and the final letter indicates the compound used for its isolation. Access numbers to the gene bank are shown in parentheses. The number of entries, new isolates, and reference sequences is indicated between parenthesis below the taxonomical group.
Nine isolates, including the single archaeal isolate, were selected for preliminary study AC removal versatility in liquid cultures, using two or three concentrations (0.5, 1.0, and 2.0 mM) of each of the four compounds at 4.5% salinity. Isolates of Alkalihalobacillus, Marinobacter, Oceanobacillus, Halobacillus, Thalassobacillus, Haladaptatus, and three Halomonas were selected, as they showed faster growth on solid agar plates. Despite an incubation of up to 60 days, none of these nine isolates could grow on 2,4-D at any concentration, including the Halobacillus sp. LV-2D and Oceanobacillus sp. LV-4D, which were initially isolated using 2,4-D as a growth substrate. Moreover, these two isolates were the only ones unable to grow and remove 4-HB (Figures 5E,G). Thalassobacillus sp. LV-41P showed low removal of 4-HB, presenting maximum removal levels of 48 and 17% at 0.5 and 1 mM after 30 days, respectively (Figure 5H). The other six isolates could almost completely remove 4-HB, although with different time courses (Figures 5A–D,F,I). H. zincidurans was the only isolate that performed better at 0.5 mM than 1 mM (Figure 5C).
Figure 5. Growth and percent of 4-hydroxybenzoic acid (4-HB) removal by selected Allenrolfea vaginata rhizosphere saline soil isolates in incubations with two concentrations of 4-HB at 4.5% salinity. Growth (open symbols) was monitored at O.D.600 nm, and 4-HB removal (closed symbols) was monitored at O.D.254 nm. The more closely related species are indicated in parenthesis. Values correspond to triplicate averages. Standard deviations were lower than 10% and are not shown for clarity.
In contrast to 4-HB, Phe removal was slower (Figure 6), as after 30 days, only Marinobacter sp. LV-48T attained significant removal of 0.5 mM Phe (Figure 6D), whereas complete removal was only observed after 60 days (Figures 6A–H), except for the archaeal isolate Haladaptatus sp. LV-51T (Figure 6I). Significant removal of 1 mM Phe was only detected in two Halomonas isolates (LV-8T and LV-42T) and both Marinobacter sp. LV-48T and Thalassobacillus sp. LV-41P (Figures 6A,C,D,H). As judged by O.D. 600 nm, biomass levels were lower than those observed with 4-HB.
Figure 6. Growth and percent of phenol (Phe) removal by selected Allenrolfea vaginata saline rhizosphere isolates in incubations with two (Phe) concentrations at 4.5% salinity. Growth (open symbols) was monitored at O.D.600 nm, and (Phe) removal (closed symbols) was monitored at O.D.211 nm. The more closely related species are indicated in parenthesis. Values correspond to triplicate averages. Standard deviations were lower than 10% and are not shown for clarity.
Tyr was the only compound that allowed growth and removal at 2 mM after 30 days (Figures 7A,D–F,H,I), except for isolates H. zincidurans LV-24T, LV-42T (Figures 7B,C), and Oceanobacillus sp. LV-4D (Figure 7G). Biomass levels, as judged by O.D.600 nm, were similar to those observed with 4-HB.
Figure 7. Growth and percent of tyrosine (Tyr) removal by selected Allenrolfea vaginata rhizosphere soil isolates in incubations with three Tyr concentrations at 4.5% salinity. Growth (open symbols) was monitored at O.D.600 nm, and Tyr removal (closed symbols) was monitored at O.D.274 nm. The more closely related species are indicated. Values correspond to triplicate averages. Standard deviations were lower than 10% and are not shown for clarity.
To address plant growth promotion and protection from saline stress by A. vaginata rhizosphere microorganisms, the interactions of A. thaliana Col-0 and five selected isolates or P. phytofirmans PsJN, a plant growth promotion rhizobacteria as positive PGPR control, were tested. At 7 DAS, plants were transferred to MS½ medium without salt (control) or with salt (100 mM NaCl/10 mM CaCl2). Plants were photographed 14 days after the transplant, and root length, number of leaves, and rosette area were measured. The rosette area values showed more significant variations, reflecting a two-dimensional parameter. In all the parameters measured, plants in control conditions (without salt) exhibited significant differences from those under saline stress (Figure 8A), represented by a greater root length (F1, 6.205 = 181.138, p < 2.22E−16), number of leaves (F1, 6.205 = 174.138, p < 2.22E−16) and rosette area (F1, 6.203 = 59.2656; p = 5.9191E−13). Under non-saline conditions, Alkalihalobacillus sp. LV-39H, and Marinobacter sp. LV-48T enhanced root length growth compared to the control PsJN or WO_MO (Figure 8A, left panel). No significant differences were found in the other three isolates relative to the WO_MO control. Curiously, strain PsJN did not promote root growth relative to the WO_MO control. Under saline stress conditions, the halophile strains LV-48T and LV-41P showed a clear root growth-enhancing effect (Figure 8A, left panel).
Figure 8. (A) Effects of selected halophilic microorganisms on Arabidopsis thaliana growth in vitro. Data were collected 14 days after transplantation. Bar graphics representation of root length (left panel), the number of leaves (central panel), and rosette area (right panel) of plants treated under saline stress and control conditions. Standard deviations represented by the error bars were calculated using 10 to 15 biological replicate plants. Letters on top of each column represent statistical differences of post-hoc contrasts art-c test analysis using the Tukey method. (B) Effects of selected halophilic microorganisms on A. thaliana growth in vitro under saline stress conditions and the presence or absence of aromatic compounds. Bar graphics representation of root length (left panel), the number of leaves (central panel), and rosette area (right panel) of A thaliana plants under saline conditions (100 mM salinity). Standard deviations represented by the error bars were calculated using ten to 15 biological replicate plants. Letters on top of each column represent statistical differences of post-hoc contrasts art-c test analysis using the Tukey method.
Under non-saline conditions, Thalassobacillus sp. LV-41P and Marinobacter sp. LV-48T showed promotion in the number of leaves compared to PsJN (Figure 8A, central panel). Still, the rest of the halophiles isolated did not present significant differences from the WO_MO control (Figure 8A, central panel). In contrast, only PsJN increased the number of leaves under saline stress with respect to WO_MO control (Figure 8A, central panel). Some halophiles also increased the rosette area under non-saline conditions, as shown for Alkalihalobacillus sp. LV-39H, Marinobacter sp. LV-48T, and Halomonas sp. LV-8T, compared to the WO_MO control (Figure 8A, right panel). Under this treatment, strain PsJN showed a more significant difference, promoting more growth than the halophiles (Figure 8A, right panel).
To study growth promotion produced by these five selected halophiles in A. thaliana, under saline conditions and in the presence of AC, plants were exposed in vitro to salt stress, and in the presence of single AC. A. thaliana seeds were sown in MS½ medium with or without inoculation of selected halophiles, or P. phytofirmans strain PsJN used as a PGPR strain control. At 7 DAS, plants were transferred to MS½ medium containing salt (100 mM NaCl/10 mM CaCl2). Plants were photographed 14 days after the transplant, and root length, number of leaves, and rosette area were measured.
Regarding the effect of 4-HB on the growth of A. thaliana (Figure 8B), only two of the three plant growth parameters studied showed significant differences between treatments: the number of leaves (F1, 6.94 = 4.9788; p = 1.815E−4) and rosette area (F1, 6.98 = 4.2686; p = 7.304E−4). Pairwise comparisons through the Tukey test showed that the number of leaves was significantly greater when PsJN was inoculated than treatments inoculated with Alkalihalobacillus sp. LV-39H, Marinobacter sp. LV-48T, and Halomonas sp. LV-8T. However, none of these strains, including PsJN, showed significant differences in control treatment without microorganisms (WO_MO). However, it was observed that Marinobacter sp. LV-48T positively affected the rosette area (Figure 8B). Although there were other significant differences between treatments, they are inconclusive to ensure a positive or negative effect of microorganisms, as they do not differ significantly from the non-inoculated, negative control.
The 2,4-D presence provoked detrimental effects on A. thaliana. The statistical results showed differences between treatment root length (F1, 6.93 = 2.926, p = 1.1167E−2), number of leaves (F1, 6.93 = 7.297, p = 2.09E−6), and rosette area (F1, 6.94 = 8.1197; p = 4.448E−7). However, pairwise analysis showed differences between the control WO_MO and all treatments, indicating that halophile and PsJN strains may protect A. thaliana exposed to 2,4-D under salt stress. Post-hoc contrast analysis showed a significant increase in rosette area in the presence of Marinobacter sp. LV-48T, compared to the control WO_MO (Figure 8B).
Statistical analysis applied to the data related to the presence of Phe and salt stress on the growth of A. thaliana showed significant differences associated with root length (F1, 6.95 = 2.7981, p = 1.499E−2), number of leaves (F1, 6.95 = 1.1217, p = 4.296E−7) and rosette area (F1, 6.96 = 12.856; p = 1.3E−10). A growth promotion effect on root length under salt and Phe stress was observed in treatments with Marinobacter sp. LV-48T and Thalassobacillus sp. LV-41P compared to the control WO_MO (Figure 8B). However, strain LV-41P negatively affected the root length of A. thaliana. On the other hand, the number of leaves decreased in the presence of Alkalihalobacillus sp. LV-39H or Thalassobacillus sp. LV-41P compared to the results obtained in treatments where Marinobacter sp. LV-48T, and Halomonas sp. LV-8T were present. However, these results were not significantly different from those of the control WO_MO, indicating that both promotion and detrimental effects by those microorganisms are mild effects on plants (Figure 8B). Similar results were obtained when P. phytofirmans PsJN was present, which showed a promoter/protective effect but to a lesser extent than the halophiles Halomonas sp. LV-8T, and Marinobacter sp. LV-48T. Similarly, the rosette area decreased in the presence of Thalassobacillus sp. LV-41P compared to control WO_MO, in A. thaliana exposed to salt stress conditions and Phe (Figure 8B).
The presence of Tyr under saline stress showed significant differences in root length (F1, 6.92 = 3.2172, p = 6.4991E−3) between plants inoculated with strain PsJN compared to those inoculated with Alkalihalobacillus sp. LV-39H, and Thalassobacillus sp. LV-41P. Nevertheless, these results do not allow us to conclude whether these halophiles positively or negatively affect root length in A. thaliana because there were no significant differences compared to the control WO_MO (Figure 8B). Moreover, statistical analysis showed differences in leaf numbers of A. thaliana between treatments inoculated with Marinobacter sp. LV-48T and PsJN compared to those inoculated with Alkalihalobacillus sp. LV-39H and Thalassobacillus sp. LV-41P. Differences were observed between the control WO_MO and any of the five halophiles studied. Finally, rosette area significantly decreased in plants inoculated with Thalassobacillus sp. LV-41P, compared to the control WO_MO (Figure 8B).
This work studied the rhizosphere microbial community of the halophyte A. vaginata thriving in soil from Lo Valdivia solar salterns, the most austral salterns in the world. This study focused on AC’s effects on microbial community structure, its depurative potential, a preliminary characterization of microbial isolates growing on such compounds, and an assessment of the effects of these isolates on plant growth promotion and protection to saline stress, using A. thaliana as a model.
The rhizosphere soil used had high conductivity, exceeding four times the conductivities presented in coastal saline soils, which would likely reduce microbial degradation of organic compounds, as high salinity produces water stress in microbial communities (Li et al., 2019). Moreover, salinity decreases the amount of available oxygen, adversely affecting the activity of enzymes such as oxygenases involved in the degradation of organic compounds (Pérez-Pantoja et al., 2010a,b; Campbell and Kirchman, 2013; Guo et al., 2016). In addition, it has been proposed that over 1% of organic matter is required to ensure microbial activity associated with the degradation of organic compounds (Witzgall et al., 2021). The rhizosphere saline soil used in this work had a 2.1% organic matter content, thus positively influencing the high removal levels detected in microcosm experiments.
Salinity is a significant factor influencing the structure of microbial communities, with differential degrees of resilience depending on the community structure (Guo et al., 2016; Viver et al., 2020). It is reported here that changes in microbial community structure produced by dilution of rhizosphere saline soil (water-only) and incubation only (without treatment), observable by differences in ecological alpha and beta indices, reverted after 30 days. This resilience effect was not detected when any four ACs were added to microcosms, as reported on non-saline soil contaminated with AC (Rodríguez-Valdecantos et al., 2017). It was found that the specific compound, rather than its concentration, played a significant role in influencing the bacterial community structure in this rhizosphere saline soil, affecting the removal performance of the microbial community (Figures 2, 3). For example, bacterial communities were strongly affected when 2,4-D was amended at high and low concentrations, increasing richness and diversity. As previously reported, such an increase may be explained by the predominance of cooperative interactions (Rodríguez-Valdecantos et al., 2017). 2,4-D was predictably the less degradable of the four ACs tested here, as the presence of halogen substituents makes their removal difficult during biodegradation (Pieper et al., 2010). In contrast, Phe, Tyr, and 4-HB can be degraded by more than one catabolic aerobic and anaerobic pathway (Pieper et al., 2010; Pérez-Pantoja et al., 2010a,b, 2012).
While the literature associated with 2,4-D degradation by halophiles is very scarce (Maltseva et al., 1996), studies on the degradation of Phe and 4-HB in saline conditions are more common, reporting that degradative aerobic metabolic pathways are similar between halophiles and non-halophiles (Bonfá et al., 2013; Veenagayathri and Vasudevan, 2015; Li et al., 2019; Mahmood et al., 2019). Phe has a general bactericidal effect, but many microorganisms have developed mechanisms to protect themselves and to use it as a sole carbon and energy source through the ortho and the meta ring-cleavage pathways (Pieper et al., 2010; Pérez-Pantoja et al., 2012; Li et al., 2019). For example, Phe is degraded by Halomonas organivorans (Bonfá et al., 2013), and a moderate halophile bacterial consortium, composed of B. cereus, Arthrobacter sp., B. licheniformis, H. salina, B. pumilus, and Pseudomonas aeruginosa (Veenagayathri and Vasudevan, 2015). Our findings are consistent with this as Pseudomonadota and Bacillota isolates (Figure 4) exhibited significant Phe removal levels.
4-HB is expected to be more easily degraded than Phe (Pérez-Pantoja et al., 2010a,b). Higher removal levels in microcosm experiments confirmed this possibility, as the more significant number of isolates obtained and the higher biomass levels reported here. Tyr is also expected to be readily degradable as Tyr catabolic pathways are frequently found in aerobic bacterial species (Pérez-Pantoja et al., 2010b, 2012), including recent reports demonstrating such degradative capacity in a few Halomonas species (Chen et al., 2018; Li et al., 2020), and other halophiles (Dastgheib et al., 2017). Accordingly, halophile organisms belonging to various genera have been shown to degrade hydrocarbons, with members of Halomonas, Alcanivorax, Marinobacter, Haloferax, Haloarcula, and Halobacterium frequently reported in the literature (Haddadi and Shavandi, 2013; Fathepure, 2014; Dastgheib et al., 2017; Chen et al., 2018; Li et al., 2019, 2020).
The study of AC degradation in microorganisms associated with halophytes, such as members of the family Amaranthaceae (Mora-Ruiz et al., 2015; Marasco et al., 2016; Szymańska et al., 2016; Razzaghi Komaresofla et al., 2019), has been less explored and opens possibilities to find new microbial species with catabolic potential. For example, in the Salicornia europaea rhizosphere, microbial community composition varies in sites that differ in their salinity level (Szymańska et al., 2016). Actinomycetota and Bacillota dominate in low salinity sites, whereas Pseudomonadota predominates in saline areas with a salinity level like the soil used in our study. Even though a different selective isolation method was used, Gammaproteobacteria and Bacillota were dominant among the isolates reported here. Members of these two phyla were also described in saline soils and rhizosphere samples associated with six other halophytic plants (Siddikee et al., 2010).
About half of the isolates reported here belong to Halomonas, which, among halophiles, possess the highest versatility to metabolize AC (García et al., 2005; Bonfá et al., 2013; Haddadi and Shavandi, 2013). As Halomonas, Marinobacter species can also degrade AC (Gao et al., 2013), so, unsurprisingly, Marinobacter isolates were also found in our study, removing 4-HB, Phe, and Tyr under moderate salinity conditions. Moreover, it has been demonstrated that Marinobacter hydrocarbonoclasticus resists high salinity levels without losing degradative activity (Fernandez-Linares et al., 1996). M. sedimentalis and M. falvimaris isolated from hypersaline sabkhas use biphenyl, phenanthrene, anthracene, and naphthalene as sole carbon and energy sources at moderate salinity (Al-Mailem et al., 2013; Gao et al., 2013).
Several members of the Bacillaceae family were also isolated in this work: isolates of Halobacillus, Oceanobacillus, Thalassobacillus, and Alkalihalobacillus grew with Phe and Tyr, and some of them with 4-HB, which agrees with previous reports (Fernandez-Linares et al., 1996; Siddikee et al., 2010; Al-Mailem et al., 2013; Gao et al., 2013). Finally, the only archaeal isolate reported here, able to grow in three of the four compounds tested at salt concentrations of 4.5%, is a possible new species of Haladaptatus, which agrees with the versatility of this archaeal genus (Liu et al., 2015; Acikgoz and Ozcan, 2016).
The study of the interaction between A. thaliana and the halophilic microorganisms isolated from the rhizosphere of A. vaginata found that the halophile Halomonas sp. LV-8T, and Marinobacter sp. LV-48T were the most interesting, as they exhibited a growth-promoting and protective effect on A. thaliana plants under saline stress conditions. This effect was mild on root length and the number of leaves but significant for the rosette area (Figure 8). These results support previous studies that show plant growth-promoting abilities in bacteria belonging to the same genera as Halomonas sp. LV-8T and, Marinobacter sp. LV-48T (Yadav and Saxena, 2018).
In contrast to strains LV-8T and LV-48T, the halophiles Alkalihalobacillus sp. LV-39H and Thalassobacillus sp. LV-41P showed adverse effects on the growth of A. thaliana under saline stress alone and with additional exposure to AC (Figure 8). Adverse effects may be explained when isolates interfere with phytohormone turnover or are inoculated in high numbers (Poupin et al., 2013; Zúñiga et al., 2013) These latter two microorganisms degrade AC and have plant growth promotion abilities under saline stress conditions on salt-sensitive plants (Orhan, 2016; Shah et al., 2017; Khunjamayum et al., 2022). It would be interesting to test combinations of these isolates as they would perform better, promoting plant growth. It is worth mentioning that the four AC differentially affected A. thaliana growth parameters with rosette area >> the number of leaves > root length being more discriminative (Figure 8B). In addition, the detrimental effect of 2,4-D on plant growth parameters, compared to the other three AC, was observed (Figure 8B).
These findings are significant because they suggest halophiles could be biofertilizers for plants grown under high salinity conditions. Furthermore, the ability of these halophiles to protect plants from toxic ACs indicates that they could also be used as bio(phyto)remediation agents for contaminated soils. To do so, plants other than A. thaliana, more field-related conditions for plant growth tests (artificial soils, among others), and isolates from other halophyte’s rhizospheres should be used.
The datasets presented in this study can be found in online repositories. The names of the repository/repositories and accession number(s) can be found in the article/supplementary material.
GR-V and FT-R performed most of the work on isolation and initial characterization of halophiles. GR-V performed soil microcosms studies. FT-R performed HPLC analyses. TL and SM-E carried out plant-microbe interactions studies. MM-R and RR-M prepared and analyzed phylogenetic tree. LC-C helped with further characterization of isolates, and microcosms studies. RR-M and BG conceived the whole idea, guided young researchers and provide funding. GR-V, FT-R, SM-E, MM-R, RR-M, LC-C, TL, and BG helped with draft writing. BG prepared and revised final manuscript. All authors contributed to the article and approved the submitted version.
This work was supported by ANID PIA/BASAL FB0002 grant (to BG), and the Spanish Ministry of Economy Projects CGL2012-39627-C03-03, CLG2015_66686-C3-1-P, and PGC2018-096956-B-C41, and European Regional Development Fund (FEDER) funds (to RR-M).
The authors are grateful to Lo Valdivia salterns miners for providing access to the study site and valuable knowledge on the operation.
The authors declare that the research was conducted in the absence of any commercial or financial relationships that could be construed as a potential conflict of interest.
All claims expressed in this article are solely those of the authors and do not necessarily represent those of their affiliated organizations, or those of the publisher, the editors and the reviewers. Any product that may be evaluated in this article, or claim that may be made by its manufacturer, is not guaranteed or endorsed by the publisher.
Acikgoz, E., and Ozcan, B. (2016). Phenol biodegradation by halophilic archaea. Int. Biodeterior. Biodegrad. 107, 140–146. doi: 10.1016/j.ibiod.2015.11.016
Al Farraj, D. A., Hadibarata, T., Yuniarto, A., Alkufeidy, R. M., Alshammari, M. K., and Syafiuddin, A. (2020). Exploring the potential of halotolerant bacteria for biodegradation of polycyclic aromatic hydrocarbon. Bioprocess Biosyst. Eng. 43, 2305–2314. doi: 10.1007/s00449-020-02415-4
Al-Mailem, D. M., Eliyas, M., and Radwan, S. S. (2013). Oil-bioremediation potential of two hydrocarbonoclastic, diazotrophic Marinobacter strains from hypersaline areas along the Arabian gulf coasts. Extremophiles 17, 463–470. doi: 10.1007/s00792-013-0530-z
Álvarez, H. M., and Silva, R. (2013). “Metabolic diversity and flexibility for hydrocarbon biodegradation by Rhodococcus” in Actinobacteria: application in bioremediation and production of industrial enzymes. eds. M. J. Amoroso, C. S. Benimeli, and S. A. Cuozzo. 1st ed (Boca Raton, FL: CRC Press), 241–273.
Aracil-Gisbert, S., Torregrosa-Crespo, J., and Martínez-Espinosa, R. M. (2018). “Recent trends on bioremediation of polluted salty soils and waters using Haloarchaea” in Advances in bioremediation and phytoremediation. ed. N. Shiomi (London: IntechOpen), 63–77.
Arora, S., Singh, A., and Singh, Y. (2017). “Microbial approach for bioremediation of saline and sodic soils” in Bioremediation of salt affected soils: an Indian perspective. eds. S. Arora, A. Singh, and Y. Singh (Cham: Springer), 87–100.
Blackwood, C. B., Hudleston, D., Zak, D. R., and Buyer, J. S. (2007). Interpreting ecological diversity indices applied to terminal restriction fragment length polymorphism data: insights from simulated microbial communities. Appl. Environ. Microbiol. 73, 5276–5283. doi: 10.1128/AEM.00514-07
Bonfá, M. R. L., Grossman, M. J., Piubeli, F., Mellado, E., and Durrant, L. R. (2013). Phenol degradation by halophilic bacteria isolated from hypersaline environments. Biodegradation 24, 699–709. doi: 10.1007/s10532-012-9617-y
Campbell, B. J., and Kirchman, D. L. (2013). Bacterial diversity, community structure and potential growth rates along an estuarine salinity gradient. ISME J. 7, 210–220. doi: 10.1038/ismej.2012.93
Chaudhary, P., Xu, M., Ahamad, L., Chaudhary, A., Kumar, G., Saanu Adeleke, B., et al. (2023). Application of synthetic consortia for improvement of soil fertility, pollution remediation, and agricultural productivity: a review. Agronomy 13:643. doi: 10.3390/agronomy13030643
Chen, C., Anwar, N., Wu, C., Fu, G., Wang, R., Zhang, C., et al. (2018). Halomonas endophytica sp. nov., isolated from liquid in the stems of Populus euphratica. Int. J. Syst. Evol. Microbiol. 68, 1633–1638. doi: 10.1099/ijsem.0.002585
Clarke, K. (1993). Nonparametric multivariate analyses of changes in community structure. Austral Ecol. 18, 117–143. doi: 10.1111/j.1442-9993.1993.tb00438.x
Dastgheib, S. M. M., Tirandaz, H., Nikou, M. M., Ramezani, M., Shavandi, M., Amoozegar, M. A., et al. (2017). Prauserella oleivorans sp. nov., a halophilic and thermotolerant crude-oil-degrading actinobacterium isolated from an oil-contaminated mud pit. Int. J. Syst. Evol. Microbiol. 67, 3381–3386. doi: 10.1099/ijsem.0.002124
Elkin, L. A., Kay, M., Higgins, J. J., and Wobbrock, J. O. (2021). An aligned rank transform procedure for multifactor contrast tests. The 34th Annual ACM Symposium on User Interface Software and Technology
Fathepure, B. Z. (2014). Recent studies in microbial degradation of petroleum hydrocarbons in hypersaline environments. Front. Microbiol. 5:173. doi: 10.3389/fmicb.2014.00173
Fernandez-Linares, L., Acquaviva, M., Bertrand, J. C., and Gauthier, M. (1996). Effect of sodium chloride concentration on growth and degradation of eicosane by the marine halotolerant bacterium Marinobacter hydrocarbonoclasticus. Syst. Appl. Microbiol. 19, 113–121. doi: 10.1016/S0723-2020(96)80018-X
Gao, W., Cui, Z., Li, Q., Xu, G., Jia, X., and Zheng, L. (2013). Marinobacter nanhaiticus sp. nov., polycyclic aromatic hydrocarbon-degrading bacterium isolated from the sediment of the South China Sea. Antonie Van Leeuwenhoek 103, 485–491. doi: 10.1007/s10482-012-9830-z
García, M. T., Ventosa, A., and Mellado, E. (2005). Catabolic versatility of aromatic compound-degrading halophilic bacteria. FEMS Microbiol. Ecol. 54, 97–109. doi: 10.1016/j.femsec.2005.03.009
Guo, G., He, F., Tian, F., Huang, Y., and Wang, H. (2016). Effect of salt contents on enzymatic activities and halophilic microbial community structure during phenanthrene degradation. Int. Biodeterior. Biodegrad. 110, 8–15. doi: 10.1016/j.ibiod.2016.02.007
Haddadi, A., and Shavandi, M. (2013). Biodegradation of phenol in hypersaline conditions by Halomonas sp. strain PH2-2 isolated from saline soil. Int. Biodeterior. Biodegrad. 85, 29–34. doi: 10.1016/j.ibiod.2013.06.005
Honda, M., and Suzuki, N. (2020). Toxicities of polyciclic aromatic hydrocarbons for aquatic animals. Int. J. Environ. Res. Public Health 17:1363. doi: 10.3390/ijerph17041363
Hothorn, T., Bretz, F., and Westfall, P. (2008). Simultaneous inference in general parametric models. Biom. J. 50, 346–363. doi: 10.1002/bimj.200810425
Jafari, B., Hanifezadeh, M., and Parvin, M. S. J. (2012). Molecular study of bacteria associated with Salicornia symbiotic bacteria as a candidate for Hormozgan salty zone culturing by Persian gulf water irrigation. Afr. Microbiol. Res. 6, 4687–4695. doi: 10.5897/AJMR11.1132
Kapadia, C., Patel, N., Rana, A., Vaidya, H., Alfarraj, S., Ansari, M. J., et al. (2022). Evaluation of plant growth-promoting and salinity ameliorating potential of halophilic bacteria isolated from saline soil. Front. Plant Sci. 13:946217. doi: 10.3389/fpls.2022.946217
Khunjamayum, R., Tamreihao, K., Asem, R. S., Singh, Y. R., Nongthombam, A., Devi, K. M., et al. (2022). Fungal disease suppression and growth promotion potential of endophytic bacteria from ethnomedicinal plants. Arch. Microbiol. 204:539. doi: 10.1007/s00203-022-03136-w
Kimbrel, J. A., Ballor, N., Wu, Y.-W., David, M. M., Hazen, T. C., Simmons, B. A., et al. (2018). Microbial community structure and functional potential along a hypersaline gradient. Front. Microbiol. 9:1492. doi: 10.3389/fmicb.2018.01492
Krzyszczak, A., and Czech, B. (2021). Ocurrence and toxicity of polyciclic aromatic hydrocarbons derivatives in environmental matrices. Sci. Total Environ. 788:147738. doi: 10.1016/j.scitotenv.2021.147738
Lenth, R., Singmann, H., Love, J., Buerkner, P., and Herve, M. (2018). Package Emmeans. R package version 4.0.3. (Comprehensive R Archive Network). R Core Team. Available at: http://cran.r-project.org/package=emmeans
Li, X., Gan, L., Hu, M., Wang, S., Tian, Y., and Shi, B. (2020). Halomonas pellis sp. nov., a moderately halophilic bacterium isolated from wetsalted hides. Int. J. Syst. Evol. Microbiol. 70, 5417–5424. doi: 10.1099/ijsem.0.004426
Li, H., Meng, F., Duan, W., Lin, Y., and Zheng, Y. (2019). Biodegradation of phenol in saline or hypersaline environments by bacteria: a review. Ecotoxicol. Environ. Saf. 184:109658. doi: 10.1016/j.ecoenv.2019.109658
Liu, G., Zhang, M., Mo, T., He, L., Zhang, W., Yu, Y., et al. (2015). Metabolic flux analysis of the halophilic archaeon Haladaptatus paucihalophilus. Biochem. Biophys. Res. Commun. 467, 1058–1062. doi: 10.1016/j.bbrc.2015.09.174
Ma, Y., Galinski, E. A., Grant, W. D., Oren, A., and Ventosa, A. (2010). Halophiles 2010: life in saline environments. Appl. Environ. Microbiol. 76, 6971–6981. doi: 10.1128/AEM.01868-10
Mahmood, A., Kataoka, R., Turgay, O. C., and Yaprak, A. E. (2019). “Halophytic microbiome in ameliorating the stress” in Ecophysiology, abiotic stress responses and utilization of halophytes. eds. M. Hasanuzzaman, K. Nahar, and M. Öztürk (Singapore: Springer), 171–194.
Mainka, T., Weirathmüller, D., Herwig, C., and Pflügl, S. (2021). Potential applications of halophilic microorganisms for biological treatment of industrial process brines contaminated with aromatics. J. Ind. Microbiol. Biotechnol. 48:kuab015. doi: 10.1093/jimb/kuab015
Mallick, I., Bhattacharyya, C., Mukherji, S., Dey, D., Sarkar, S. C., Mukhopadhyay, U. K., et al. (2018). Effective rhizoinoculation and biofilm formation by arsenic immobilizing halophilic plant growth promoting bacteria (PGPB) isolated from mangrove rhizosphere: a step towards arsenic rhizoremediation. Sci. Total Environ. 610-611, 1239–1250. doi: 10.1016/j.scitotenv.2017.07.234
Maltseva, O., McGowan, C., Fulthorpe, R., and Oriel, P. (1996). Degradation of 2,4-dichlorophenoxyacetic acid by haloalkaliphilic bacteria. Microbiology 142, 1115–1122. doi: 10.1099/13500872-142-5-1115
Marasco, R., Mapelli, F., Rolli, E., Mosqueira, M. J., Fusi, M., Bariselli, P., et al. (2016). Salicornia strobilacea (synonym of Halocnemum strobilaceum) grown under different tidal regimes selects rhizosphere bacteria capable of promoting plant growth. Front. Microbiol. 7:1286. doi: 10.3389/fmicb.2016.01286
Molina, L., and Segura, A. (2021). Biochemical and metabolic plant responses towards polyciclic aromatic hydrocarbons and heavy metals present in atmospheric pollution. Plan. Theory 10:2305. doi: 10.3390/plants10112305
Mora-Ruiz, M. R., Font-Verdera, F., Díaz-Gil, C., Urdiain, M., Rodríguez-Valdecantos, G., González, B., et al. (2015). Moderate halophilic bacteria colonizing the phylloplane of halophytes of the subfamily Salicornioideae (Amaranthaceae). Syst. Appl. Microbiol. 38, 406–416. doi: 10.1016/j.syapm.2015.05.004
Murashige, T., and Skoog, F. (1962). A revised medium for rapid growth and bioassays with tobacco tissue cultures. Physiol. Plant. 15, 473–497. doi: 10.1111/j.1399-3054.1962.tb08052.x
Ning, X., Shen, L., Sun, J., Lin, C., Zhang, Y., Yang, Z., et al. (2015). Degradation of polycyclic aromatic hydrocarbons (PAHs) in textile dyeing sludge by O3/H2O2 treatment. RSC Adv. 5, 38021–38029. doi: 10.1039/C5RA03307A
Oksanen, J., Blanchet, F. G., Friendly, M., Kindt, R., Legendre, P., Mc Glinn, D., et al. (2020). Vegan: community ecology package. R package version 2, 5–7. Available at: https://CRAN.R-project.org/package=vegan
Orhan, F. (2016). Alleviation of salt stress by halotolerant and halophilic plant growth-promoting bacteria in wheat (Triticum aestivum). Braz. J Microbiol. 47, 621–627. doi: 10.1016/j.bjm.2016.04.001
Osborne, C. A., Rees, G. N., Bernstein, Y., and Janssen, P. H. (2006). New threshold and confidence estimates for terminal restriction fragment length polymorphism analysis of complex bacterial communities. Appl. Environ. Microbiol. 72, 1270–1278. doi: 10.1128/AEM.72.2.1270-1278.2006
Pérez-Pantoja, D., De la Iglesia, R., Pieper, D. H., and González, B. (2008). Metabolic reconstruction of aromatic compounds degradation from the genome of the amazing pollutant-degrading bacterium Cupriavidus necator JMP134. FEMS Microbiol. Rev. 32, 736–794. doi: 10.1111/j.1574-6976.2008.00122.x
Pérez-Pantoja, D., Donoso, R., Agulló, L., Córdova, M., Seeger, M., Pieper, D. H., et al. (2012). Genomic analysis of aromatic compounds biodegradation in Burkholderiales. Environ. Microbiol. 14, 1091–1117. doi: 10.1111/j.1462-2920.2011.02613.x
Pérez-Pantoja, D., Donoso, R., Junca, H., González, B., and Pieper, D. H. (2010b). “Phylogenomics of aerobic bacterial degradation of aromatics” in Handbook of hydrocarbon and lipid microbiology. ed. K. N. Timmis (Berlin: Springer-Verlag), 1356–1397.
Pérez-Pantoja, D., González, B., and Pieper, D. H. (2010a). “Aerobic degradation of aromatic hydrocarbons” in Handbook of hydrocarbon and lipid microbiology. ed. K. N. Timmis (Berlin: Springer), 799–837.
Pieper, D. H., González, B., Cámara, B., Pérez-Pantoja, D., and Reineke, W. (2010). “Aerobic degradation of chloroaromatics” in Handbook of hydrocarbon and lipid microbiology. ed. K. N. Timmis (Berlin: Springer), 839–864.
Pinedo, I., Ledger, T., Greve, M., and Poupin, M. J. (2015). Burkholderia phytofirmans PsJN induces long-term metabolic and transcriptional changes involved in A. thaliana salt tolerance. Front. Plant Sci. 6:466. doi: 10.3389/fpls.2015.00466
Poupin, M. J., Timmermann, T., Vega, A., Zúñiga, A., and González, B. (2013). Effects of the plant growth promoting bacterium Burkholderia phytofirmans PsJN throughout the life cycle of Arabidopsis thaliana. PLoS One 8:2013. doi: 10.1371/journal.pone.0069435
Razzaghi Komaresofla, B., Alikhani, H. A., Etesami, H., and Khoshkholgh-Sima, N. A. (2019). Improved growth and salinity tolerance of the halophyte Salicornia sp. by co-inoculation with endophytic and rhizosphere bacteria. Appl. Soil Ecol. 138, 160–170. doi: 10.1016/j.apsoil.2019.02.022
Robertson, G. P., Coleman, D. C., Sollins, P., and Bledsoe, C. S., (1999). Standard soil methods for long-term ecological research New York. Oxford University Press
Rodríguez-Valdecantos, G., Manzano, M., Sánchez, R., Urbina, F., Hengst, M. B., Lardies, M. A., et al. (2017). Early successional patterns of bacterial communities in soil microcosms reveal changes in bacterial community composition and network architecture, depending on the successional condition. Appl. Soil Ecol. 120, 44–54. doi: 10.1016/j.apsoil.2017.07.015
RStudio Team. (2020). RStudio: integrated development for R. RStudio PBC, Boston, MA. Available at: http://www.rstudio.com/
Sáenz-Mata, J., Palacio-Rodríguez, R., Sánchez-Galván, H., and Balagurusamy, N. (2016). “Plant growth promoting rhizobacteria associated to halophytes: potential applications in agriculture” in Sabkha ecosystems. Tasks for vegetation science. eds. M. Khan, B. Boër, M. Ȫzturk, M. Clüsener-Godt, B. Gul, and S. W. Breckle (Cham: Springer), 411–425.
Sahu, P. K., Shafi, Z., Singh, S., Ojha, K., Jayalakshmi, K., Tilgam, J., et al. (2023). Colonization potential of endophytes from halophytic plants growing in the “Runn of Kutch” salt marshes and their contribution to mitigating salt stress in tomato cultivation. Front. Microbiol. 14:1226149. doi: 10.3389/fmicb.2023.1226149
Sampedro, I., Pérez-Mendoza, D., Toral, L., Palacios, E., Arriagada, C., and Llamas, I. (2020). Effects of halophyte root exudates and their components on chemotaxis, biofilm formation and colonization of the halophilic bacterium Halomonas anticariensis FP35(T). Microorganisms 8:575. doi: 10.3390/microorganisms8040575
Sardar, H., Khalid, Z., Ahsan, M., Naz, S., Nawaz, A., Ahmad, R., et al. (2023). Enhancement of salinity stress tolerance in lettuce (Lactuca sativa L.) via foliar application of nitric oxide. Plan. Theory 12:1115. doi: 10.3390/plants12051115
Shah, G., Jan, M., Afreen, M., Anees, M., Rehman, S., Daud, M. K., et al. (2017). Halophilic bacteria mediated phytoremediation of salt—affected soils cultivated with rice. J. Geochem. Explor. 174, 59–65. doi: 10.1016/j.gexplo.2016.03.011
Shannon, C. E. (1948). A mathematical theory of communication. Bell Syst. Tech J. 27, 623–656. doi: 10.1002/j.1538-7305.1948.tb01338.x
Siddikee, M. A., Chauhan, P. S., Anandham, R., Han, G. H., and Sa, T. M. (2010). Isolation, characterization, and use for plant growth promotion under salt stress, of ACC deaminase-producing halotolerant bacteria derived from coastal soil. J. Microbiol. Biotechnol. 20, 1577–1584. doi: 10.4014/jmb.1007.07011
Singh, B. K., Nazaries, L., Munro, S., Anderson, I. C., and Campbell, C. D. (2006). Use of multiplex terminal restriction fragment length polymorphism for rapid and simultaneous analysis of different components of the soil microbial community. Appl. Environ. Microbiol. 72, 7278–7285. doi: 10.1128/AEM.00510-06
Szymańska, S., Płociniczak, T., Piotrowska-Seget, Z., and Hrynkiewicz, K. (2016). Endophytic and rhizosphere bacteria associated with the roots of the halophyte Salicornia europaea L.—community structure and metabolic potential. Microbiol. Res. 192, 37–51. doi: 10.1016/j.micres.2016.05.012
Veenagayathri, K., and Vasudevan, N. (2015). Degradation of dual phenolics by a moderately halophilic bacterial consortium and its degradation products. Int. J. Curr. Microbiol. App. Sci. 3, 513–524. doi: 10.9734/BMRJ/2013/3171
Ventosa, A., Quesada, E., Rodriguez-Valera, F., Ruiz-Berraquero, F., and Ramos-Cormenzana, A. (1982). Numerical taxonomy of moderately halophilic Gram-negative rods. Microbiology 128, 1959–1968. doi: 10.1099/00221287-128-9-1959
Viver, T., Conrad, R. E., Orellana, L. H., Urdiain, M., González-Pastor, J. E., Hatt, J. K., et al. (2020). Distinct ecotypes within a natural haloarchaeal population enable adaptation to changing environmental conditions without causing population sweeps. ISME J. 15, 1178–1191. doi: 10.1038/s41396-020-00842-5
Waddell, E. J., Elliott, T. J., Vahrenkamp, J. M., Roggenthen, W. M., Sani, R. K., Anderson, C. M., et al. (2010). Phylogenetic evidence of noteworthy microflora from the subsurface of the former Homestake gold mine, Lead, South Dakota. Environ. Technol. 31, 979–991. doi: 10.1080/09593331003789511
Warren-Vega, W. M., Campos-Rodríguez, A. C., Zárate-Guzmán, A. I., and Romero-Cano, L. A. (2023). A current review of water pollutants in American continent: trends and perspectives in detection, health risks and treatment technologies. Int. J. Environ. Res. Public Health 20:4099. doi: 10.3390/ijerph20054499
Witzgall, K., Vidal, A., Schubert, D. I., Höschen, C., Schweizer, S. A., Buegger, F., et al. (2021). Particulate organic matter as a functional soil componente for persistent soil organic carbon. Nat. Commun. 12:4115. doi: 10.1038/s41467-021-24192-8
Wobbrock, J. O., Findlater, L., Gergle, D., and Higgins, J. J. (2011). The aligned rank transform for nonparametric factorial analyses using only ANOVA procedures. Proceedings of the SIGCHI Conference on Human Factors in Computing Systems. pp. 143–146.
Yadav, A. N., and Saxena, A. K. (2018). Biodiversity and biotechnological applications of halophilic microbes for sustainable agriculture. J. Appl. Biol. Biotechnol. 6, 48–55. doi: 10.7324/JABB.2018.60109
Zúñiga, A., Poupin, M. J., Donoso, R. A., Ledger, T., Guiliani, N., Gutiérrez, R. A., et al. (2013). Quorum sensing and indole-3-acetic acid degradation play a role in colonization and plant growth promotion of Arabidopsis thaliana by Burkholderia phytofirmans PsJN. Mol. Plant Microbe. Interact. 26, 546–553. doi: 10.1094/MPMI-10-12-0241-R
Keywords: aromatic compounds, halophiles, PGPR, rhizosphere, salterns
Citation: Rodríguez-Valdecantos G, Torres-Rojas F, Muñoz-Echeverría S, del Rocío Mora-Ruiz M, Rosselló-Móra R, Cid-Cid L, Ledger T and González B (2023) Aromatic compounds depurative and plant growth promotion rhizobacteria abilities of Allenrolfea vaginata (Amaranthaceae) rhizosphere microbial communities from a solar saltern hypersaline soil. Front. Microbiol. 14:1251602. doi: 10.3389/fmicb.2023.1251602
Received: 02 July 2023; Accepted: 09 October 2023;
Published: 23 October 2023.
Edited by:
Asma Imran, National Institute for Biotechnology and Genetic Engineering, PakistanReviewed by:
Satish Kumar, Directorate of Onion and Garlic Research (ICAR), IndiaCopyright © 2023 Rodríguez-Valdecantos, Torres-Rojas, Muñoz-Echeverría, del Rocío Mora-Ruiz, Rosselló-Móra, Cid-Cid, Ledger and González. This is an open-access article distributed under the terms of the Creative Commons Attribution License (CC BY). The use, distribution or reproduction in other forums is permitted, provided the original author(s) and the copyright owner(s) are credited and that the original publication in this journal is cited, in accordance with accepted academic practice. No use, distribution or reproduction is permitted which does not comply with these terms.
*Correspondence: Bernardo González, YmVybmFyZG8uZ29uemFsZXpAdWFpLmNs
†These authors have contributed equally to this work and share first authorship
Disclaimer: All claims expressed in this article are solely those of the authors and do not necessarily represent those of their affiliated organizations, or those of the publisher, the editors and the reviewers. Any product that may be evaluated in this article or claim that may be made by its manufacturer is not guaranteed or endorsed by the publisher.
Research integrity at Frontiers
Learn more about the work of our research integrity team to safeguard the quality of each article we publish.