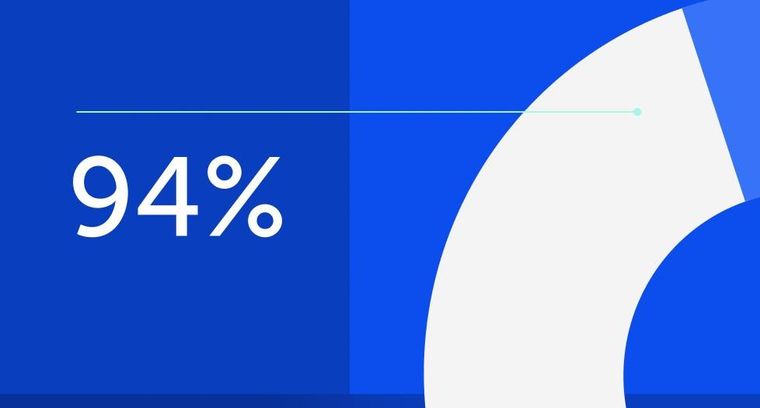
94% of researchers rate our articles as excellent or good
Learn more about the work of our research integrity team to safeguard the quality of each article we publish.
Find out more
ORIGINAL RESEARCH article
Front. Microbiol., 20 September 2023
Sec. Microbiological Chemistry and Geomicrobiology
Volume 14 - 2023 | https://doi.org/10.3389/fmicb.2023.1250330
This article is part of the Research TopicInsights in Microbiological Chemistry and Geomicrobiology : 2022/2023View all 11 articles
Acidithiobacillus caldus plays an important role in bioleaching of low-grade metal ore. It can promote the release of heavy metals in mining-associated habitats and survive in high concentrations of heavy metals. Functions of glutathione reductase (GR) in cell defense against reactive oxygen species caused by heavy metals have been elucidated in some eukaryotic cells and bacteria; however, no information is available in A. caldus. In this research, the methods of bioinformatics, gene expression, GR activity assays were used to detect and characterize the glutathione reductase gene from the A. caldus MTH-04 strain. Then, A. caldus gr knockout mutant and gr overexpression strain were constructed, and the heavy metal tolerant properties and transcriptional levels of ROS related genes of them were compared to study the function of GR. The results showed that, a putative gr gene F0726_RS04210 was detected in the genome of A. caldus MTH-04. The purified recombinant protein of F0726_RS04210 showed remarkable GR activity at optimal pH 7.0 and 30°C using in vitro assay. The evolutionary relationship of GR from A. caldus MTH-04 was close to that from Escherichia coli K12. Gene knockout or overexpression of gr in A. caldus did not affect the growth rate on S0 medium, suggesting that GR did not play a key role in the activation of sulfur. Deletion of gr resulted in increased sensitivity to heavy metals (Cu2+ and Zn2+) in A. caldus, and the gr overexpression strain showed enhanced tolerance to heavy metals. Furthermore, transcription analysis also revealed strong correlations between GR and the antioxidant pathway. The above results suggest that GR can play an important role in heavy metal tolerance in A. caldus.
At present, many polymetallic ores, such as zinc ore and copper ore, are sulfide minerals, which complicate the production of high-grade metal ores and increase the production cost. Acidithiobacillus caldus is an important acidophilic, chemolithoautotrophic sulfur-oxidizing bacterium that is widely used in the bioleaching industry. It can obtain energy and reduce power to achieve autotrophic growth by sulfur oxidation (Hallberg and Lindström, 1994, 1996; Kamimura et al., 1999; Edwards et al., 2000; Okibe et al., 2003). Acidithiobacillus caldus can play an important role in processing low-grade concentrates of non-ferrous metals. During the bioleaching process, the released heavy metals are toxic to the leaching microorganisms, and the stress tolerance process of the microorganisms will result in the production of reactive oxygen species (ROS) inside the cells (Stadtman and Oliver, 1991; Natarajan et al., 1994). In eukaryotic cells, ROS can be scavenged using the glutathione system, including glutathione (GSH), glutathione S-transferases (GST), glutathione synthetase (GS), and glutathione reductase (GR) (Foyer et al., 1991). However, limited studies have been conducted on A. caldus (Dopson et al., 2003; Luo et al., 2008).
GSH, which is widely found in bacteria and eukaryotic cells (Meister and Anderson, 1983; Smith et al., 1990), can protect cells from ROS by providing reducing equivalents for antioxidant defense enzymes or scavenging hydroxyl radicals directly (Noctor and Foyer, 1998). GR can catalyze the conversion of glutathione disulfide (GSSG) to GSH (Scruton et al., 1990; Rice-Evans et al., 1996). By keeping high GSH/GSSG ratios, GR plays a key role in cell defense against ROS (Schirmer et al., 1989; Creissen et al., 1994; Mullineaux and Creissen, 1997). Recently, the glutathione system was reported to participate in the heavy metal tolerance of Acidithiobacillus ferrooxidans (Xia et al., 2011; Zheng et al., 2015, 2016); however, the heavy metal tolerance mechanisms of A. caldus are poorly understood compared with that of A. ferrooxidans.
In this research, we characterized a glutathione reductase gene from A. caldus MTH-04 using the methods of bioinformatic, gene expression, and GR activity assays. Then, we reported the construction of a gr knockout mutant and a gr overexpression strain of A. caldus MTH-04. Finally, we compared the heavy metal tolerance properties of the A. caldus mutants with its wild type and discussed the potential role of gr gene in heavy metal tolerance in A. caldus MTH-04.
The bacterial strains and plasmids used in this study are presented in Table 1. The strains of A. caldus were cultured at 40°C shaken at 150 rpm in liquid Starkey-S0 medium (pH 2.5) or on solid Starkey-Na2S2O3 medium (pH 4.8) (Jin et al., 1992). The liquid Starkey medium contained S0 (8 g/L, boiling sterilized) as the energy source. Kanamycin (200 μg/ml), streptomycin (200 μg/ml), or chloromycetin (68 μg/ml) was used in liquid Starkey-S0 when required, and kanamycin (80 μg/ml), streptomycin (80 μg/ml), or chloromycetin (27.2 μg/ml) was used in the solid Starkey-Na2S2O3 medium for selection. Escherichia coli strains were grown at 37°C shaken at 170 rpm in liquid Luria–Bertani (LB) broth or on solid LB medium, and ampicillin (100 μg/ml), kanamycin (100 μg/ml), streptomycin (100 μg/ml), or chloromycetin (34 μg/ml) was added when required.
NCBI BLASTP (http://blast.ncbi.nlm.nih.gov/Blast.cgi) was used to search for GR homolog in the sequenced genome of A. caldus MTH-04 (CGMCC 1.15711). The protein molecular masses of the homologous proteins and the isoelectric points (pI) were predicted by using an ExPASy Compute pI/Mw tool (http://web.expasy.org/compute_pi/). ClustalX version 1.81 was used to perform the multiple sequence alignment. The phylogenetic tree was constructed by ClustalX version 1.81 and MEGA version 5, with a p-distance distribution, pairwise deletion, and bootstrap analysis of 10,000 repeats. Subcellular localization of protein was predicted using SignalP 4.1 (http://www.cbs.dtu.dk/services/SignalP/), Softberry ProtCompB tool (http://linux1.softberry.com/berry.phtml?topic=pcompb&group=programs&subgroup=proloc), and PSORTB v3.0 (http://www.psort.org/psortb/).
General molecular biological techniques, including restriction enzyme digestion, ligation, gel electrophoresis, and transformation of the plasmids, were conducted according to the standard protocols (Sambrook and Russell, 2001). The genomic DNA of A. caldus was isolated using the TIANamp Bacteria DNA Kit of TIANGEN. Plasmids were isolated using the TIANprep Mini Plasmid Kit of TIANGEN. DNA fragments were recovered from agarose gels using the OMEGA E.Z.N.A.® Gel Extraction Kit of Omega Bio-Tek. DNA polymerase, restriction enzymes, and T4 DNA ligase were purchased from TaKaRa, and primers were generated by Invitrogen.
The strains and plasmids used to clone the potential GR gene are presented in Table 1, and the primers are presented in Table 2. To express and purify the recombinant protein of F0726_RS04210, the coding sequence was amplified using primers F-gr and R-gr. The fragment was digested by NdeI and XhoI and inserted into NdeI-XhoI-treated pET28a, to generate recombinant plasmid pET28a-gr. Successful insertion of the coding sequences of target genes was confirmed by sequencing; then, the recombinant plasmids were transformed into E. coli BL21(DE3) cells. Isopropyl-β-D-thiogalactopyranoside (IPTG) was added to the final concentration of 0.4 mM to induce the expression of recombinant protein at 25°C for at least 5 h. Recombinant proteins were analyzed using 10% SDS-PAGE and purified using HisTrap™ HP Crude Columns (GE Health) and AmiconUltra-15 Centrifugal Filter Units with Ultracel-3 membranes (Merck Millipore). Finally, the protein concentrations were determined using a Pierce®BCA Protein Assay Kit.
GR can catalyze the reaction as follows: NADPH + H + + GSSG → NADP + + 2GSH, and the oxidation of NADPH can be measured by the decrease in absorbance at 340 nm (ε = 6.22 × 103M−1 · cm−1). Purified GR was used in GR activity measurement using the method reported by Greer with minor modifications (Greer and Perham, 1986). In brief, the assay mixture (200 μl) contained 0.1 mM NADPH, 1.2 mM GSSG, and an appropriate amount of purified GR in 1 mM phosphate buffer (pH 7.0) at room temperature. Values of absorbance at 340 nm were recorded every 1 min and continued for at least 5 min. The reaction was started by the addition of NADPH. All reactions were performed with three independent biological replicates. Heat inactivated purified GR was used as the control for each reaction. Specific GR activity was measured as units of GR activities per mg of purified GR protein. Moreover, 1 unit of GR activity is defined as that can reduce 1 μmol of GSSG in 1 min at room temperature.
The effects of pH and temperature on GR activity were determined using the method described above. The optimum pH of GR was determined using purified GR in 1 mM citrate buffer solution (pH 3–5), 1 mM phosphate buffer solution (pH 6–8), or 1 mM Tris–HCl buffer solution (pH 9–10), respectively. The purified GR was incubated in the above buffers for 60 min at room temperature before measuring the activity at various pH conditions. The optimum temperature of GR was measured using purified GR within the range of 20–50°C at pH 7.0. The purified GR was incubated at the same temperature for 60 min before the activity measurement at each temperature.
The effect of metal ions (Cu2+, Zn2+, Cd2+, Ag+, Fe2+, Co2+, Mg2+, Sn2+, and Mn2+) on GR activity was determined by measuring its specific activity in the presence of 0.5 mM, 1 mM, or 1.5 mM of each reagent. The purified GR was incubated in the above reagent for 60 min before the GR activity measurement. The p-value was calculated using a t-test.
Markerless gr knockout mutant was generated as described previously with minor modifications (as shown in Figure 1) (Wang et al., 2016). The plasmids used in generating markerless gr knockout mutants are presented in Table 1, and the primers are presented in Table 2.
Figure 1. Construction process of the A. caldus knockout strain. UHA represents the upstream homologous arm, and DHA represents the downstream homologous arm, respectively.
The upstream and downstream homologous arms of gr were amplified by PCR using primer pairs of grUF/grUR [819 bp upstream homologous arm (UHA)] and grDF/grDR [933 bp downstream homologous arm (DHA)]. The amplified sequences were inserted into the suicide vector pSDUDI after digestion with appropriate restriction enzymes. The sequences of the resulting plasmids pSDUDI-gr were confirmed by sequencing.
The suicide vector pSDUDI-gr was transformed into E. coli SM10, and the generated transformant was used as the donor. Acidithiobacillus caldus MTH-04 was used as the recipient to construct Δgr. Plasmid pSDUDI-gr was transferred from E. coli SM10 to A. caldus by conjugation, as described earlier (Wu et al., 2017). Colonies on selective Starkey-Na2S2O3 plates containing kanamycin were selected and analyzed using colony PCR. Primer pairs oriTF/oriTR were used to detect the single crossover of Δgr, where a fragment of 465 bp was expected in the case of a single crossover, and no fragment was expected in wild type or Δgr. Colonies with the correct PCR fragments were inoculated into a liquid Starkey-S0 medium, and the genomic DNA of each selected colony was isolated for PCR analysis to confirm the single-recombination event. Primer pairs grIF/grIR were used to confirm the single crossover of Δgr, where both fragments of 3,088 bp and 1,054 bp were expected in the case of a single crossover.
Plasmid pSDU1-I-Sce I was then transferred to the single crossover cells of A. caldus to induce a second homologous recombination, thereby generating the knockout mutants or wild type individuals. The Δgr strain was identified using colony PCR based on screening colonies grown on selective Starkey-Na2S2O3 plate containing chloromycetin using primer pairs grIF/grIR, as described above. A 1,054 bp fragment was expected in the Δgr strain, and a 3,088 bp fragment was expected for wild type cells. Primer pairs oriTF/oriTR, grIF/grIR, and grOF/grOR were used to confirm the deletion of gr. For the primer pairs grOF/grOR, a 2,963 bp fragment was expected in gr knockout mutant, and a 4,325 bp fragment was expected in the wild type cells. The amplified fragment from gr knockout mutant using primer pairs grOF/grOR was sequenced to confirm the mutants.
The pSDU1-I-Sce I plasmid in mutant cells was eliminated by spontaneous loss, as described earlier (Wu et al., 2017).
A Southern blot analysis was performed, as described earlier (Wen et al., 2014), using Sac I digests of the genomic DNA of the Δgr mutant and wild type of A. caldus. The downstream homologous arm of gr gene was labeled with digoxigenin and used as the probe.
The strains and plasmids used to construct the gr gene overexpression strain of A. caldus are presented in Table 1, and the primers are presented in Table 2. The coding sequence of gr along with its SD sequence was amplified using primer pairs O-F/O-R. The amplified fragment was first cloned into plasmid pUC19 for sequencing, after digestion with BamH I and EcoR I. The fragment pJRD215-tac was obtained from plasmid pJRD215-tac-cup after digestion with BamH I and EcoR I. Then, the confirmed gr-coding sequence was obtained from its sequenced recombinant plasmid by digestion with BamH I and EcoR I and ligated with pJRD215-tac to produce pJRD215-tac-gr. Plasmid pJRD215-tac-gr and the control plasmid pJRD215 were transformed into E. coli SM10. The plasmids were then transformed into A. caldus MTH-04 through conjugation as described earlier (Wu et al., 2017).
The A. caldus MTH-04 wild type, Δgr, control strain (wild type carrying plasmid pJRD215), and gr overexpression strain (wild type carrying plasmid pJRD215-tac-gr) were grown in the Starkey-S0 medium. All the strains were grown in the Starkey-S0 medium for 7 days, and then the cells were collected by centrifugation and adjusted to the same cell concentration (OD600 = 20.0). An aliquot (150 μl) of the treated cells was inoculated into 150 ml of fresh Starkey-S0 medium, added with different amounts of CuSO4 with the final concentrations of 0 mM, 5 mM, 10 mM, and 20 mM or different amounts of ZnSO4 with the final concentrations of 0 mM, 40 mM, 80 mM, and 160 mM, respectively, cultivated at 40°C, and shaken at 150 rpm. After low-speed centrifugation at 400 × g for 5 min to remove the solid sulfur (Wang et al., 2016), the growth of A. caldus cultured in different concentrations of CuSO4 or ZnSO4 was monitored by measuring the optical density at 460 or 600 nm, respectively. All measurements were performed in triplicate, and error bars correspond to the standard deviations.
Wild type, Δgr, the control strain (wild type carrying plasmid pJRD215) and the gr overexpression strain (wild type carrying plasmid pJRD215-tac-gr) of A. caldus were grown for 7 days in Starkey-S0 medium without heavy metals and used to measure the transcriptional levels of the glutathione-related genes. The cells were first collected by centrifugation at 12,000 × g for 5 min, resuspended in RNAlater® Solution (Ambion), and harvested from the RNAlater® suspension by centrifugation after overnight storage at 4°C.
To study the responses of the glutathione-related genes to the heavy metal stress at different time points, above A. caldus strains grown for 7 days without heavy metals were supplemented with CuSO4 (final concentration: 5 mM) or ZnSO4 (final concentration: 40 mM), respectively, incubated at 40°C and shaken at 150 rpm. Cells were collected at two time intervals (1 h and 2 h) and treated as described above.
Overall, 100 μl of lysis buffer (1 mg/ml lysozyme, 10 mM Tris, and 1 mM EDTA, pH 8.0) was used to resuspend the cells and then incubated at 26°C for 6 min. TRIzol® Reagent (Ambion) was used to extract total RNA following the manufacturer's instruction. Denaturing formaldehyde agarose gel electrophoresis was used to examine RNA quality, and a NanoDrop-1000 spectrophotometer (NanoDrop Technologies) was used to determine the concentration of RNA. A PrimeScript™ RT Reagent Kit with gDNA Eraser (Perfect Real Time; TAKARA) was used to remove the genomic DNA and synthesize the cDNA.
RT-qPCR was performed with the LightCycler®480 system (Roche), following the manufacturer's instruction with SYBR®Premix Ex Taq (TaKaRa). In this study, all RT-qPCR reactions were performed in triplicate with at least three independent biological replicates. All primers used for RT-qPCR are presented in Table 3. Gene alaS was used for normalization, and transcriptional results were calculated and shown in 2−ΔΔCT (Livaka and Schmittgenb, 2001; Wu et al., 2017). The p-value was calculated using a t-test. The relative mRNA levels of these genes were measured using the gr knockout strain with the wild type as the control or using the gr overexpression strain with the wild type carrying vacant pJRD215 plasmid as the control. Fold change ≥ 2 and p-value ≤ 0.05 were considered to be upregulated, while fold change ≤ 0.5 and p-value ≤ 0.05 were considered to be downregulated.
We found a putative glutathione reductase encoding gene F0726_RS04210 in the genome of A. caldus MTH-04 by conducting an online BLASTN search. The ORF of F0726_RS04210 encoades a 453 amino acid glutathione disulfide reductase with a theoretical isoelectric point of 6.58 and molecular mass of 49.2 kDa. F0726_RS04210 shareas sequence identity with known GRs. These include Dickeya dadantii (53% identity), E. coli K12 (54% identity), E. coli VRa50 (53% identity), Francisella noatunensis subsp. orientalis str. Toba 04 (45% identity), Nostoc sp. PCC 7120 (40% idaentity), Phaeospirillum molischianum DSM 120 (41% identity), Caulobacter sp. AP07 (38% identity), and Sinorhizobaium meliloti (38% identity), respectively. An unrooted phylogenetic tree was constructed with F0726_RS04210 and the above mentioned GRs to better uanderstand the relationship between the reported GRs and the putative GR detected in A. caldus MTH-04. The evolutionary relationship of F0726_RS04210 is close to the glutathione reductase from E. coli K12, one of the best-understood GR, with a high identitay (54%; Figure 2). By alignment with known GRs, F0726_RS04210 has the highly conserved functional motifs characterized for GR, indicating the close relationship among GRs (Figure 3).
Figure 2. Neighbor-joining tree of GRs from eukaryotic and prokaryotic species. GenBank accession numbers are presented in parentheses.
Figure 3. Sequence alignment of protein sequences homologous to GR. The protein encoded by F0726_RS04210 from Acidithiobacillus caldus MTH-04 is abbreviated to A.c MTH-04 (AUW33524.1). The GRs from Caulobacter sp. AP07, Dickeya dadantii, Escherichia coli K12, Escherichia coli VR50, Francisella noatunensis subsp. orientalis str. Toba 04, Nostoc sp. PCC 7120, Phaeospirillum molischianum DSM 120, and Sinorhizobium meliloti are abbreviated to Caulobacter (WP_007662640.1), D. dadantii (WP_013320090.1), E. coli K12 (P06715.1), E. coli VR50 (AKA92683.1), F. noatunensis (AFJ43905.1), Nostoc sp. (CAA61856.1), P. molischianum (CCG41004.1), and S. meliloti (WP_003535072.1), respectively. Identical amino acid residues are highlighted in black. The box indicates the redox-active disulfide bond domain (CXXXXC), the glutathione-binding residues are marked with , and the conserved arginine residues required for NADP binding are indicated by
, respectively. The symbol * indicates the position of a multiple of 10.
F0726_RS04210 was then expressed and purified from E. coli BL21(DE3) (Figure 4). We observed the GR activity of recombinant protein F0726_RS04210 in vitro. The GR activity of F0726_RS04210 was 415.8 U/mg, which was similar to that from E. coli (Nigel et al., 1987). Based on the activity we detected in the in vitro assays, we designated F0726_RS04210 as glutathione reductase. The optimum pH for the GR was 7.0 (Figure 5A), and the optimum temperature was 30°C (Figure 5B). The effect of metal ions on GR activity was also studied. Cu2+, Zn2+, Cd2+, Ag+, Fe2+, and Co2+ were found to strongly inhibit the activity of GR (P < 0.05), while GR activity was not obviously affected by Mg2+, Mn2+, and Sn2+ (Figure 5C). Similar phenomena were also reported in rainbow trout liver, E. coli, Phaeodactylum tricornutum, and Spinacia oleracea L. Leaves (Asnis, 1955; Michail and James, 1977; Diego et al., 2010; Ekinci and Sentürk, 2013).
Figure 4. Purification of recombinant F0726_RS04210 from E. coli BL21(DE3). The proteins are loaded on 10% (wt/vol) SDS-PAGE gel and stained with Coomassie Brilliant Blue R-250. The protein extract of E. coli BL21(DE3) cells containing pET28a-gr without induction (1), induced with IPTG (2) and the purified recombinant F0726_RS04210 protein (3), is indicated, respectively. M: Blue Plus™ II Protein Marker (TransGen Biotech).
Figure 5. Influence of pH (A), temperature (B), and inhibitors (C) on GR activities of purified recombinant F0726_RS04210.
In addition, the predicted subcellular localization of GR was analyzed using ProtCompB, SignalP 4.1, and PSORTB v3.0, and no signal peptide or transmembrane region was found, suggesting that it may be cytoplasmic protein, which is consistent with the neutral optimum pH of GR.
To better understand the function of GR in A. caldus, we used a markerless gene knockout system to generate gr knockout mutant Δgr (as shown in Figure 1). We identified the candidate Δgr mutant firstly by observing fragment size in a PCR analysis, and all observed PCR fragments were in accordance with the predicted sizes as described in the Materials and Methods section (Figure 6A). Then, Southern blot hybridization was performed using the downstream homologous arm as the probe. After digestion by using Sac I, the expected bands of 1,683 and 3,045 bp were obtained for Δgr mutant and the wild type, respectively, which indicates the successful knockout of the gr gene from A. caldus MTH-04 (Figure 6B). Finally, the obtained Δgr mutant was confirmed by sequencing the mutated region. The gr overexpression strain and a control strain (wild type carrying plasmid pJRD215) were also successfully constructed using the method described in the previous section.
Figure 6. Identification of the gr knockout mutant of Acidithiobacillus caldus MTH-04 using PCR (A) and Southern blot hybridization (B). (A) Specific primer pairs oriTF/oriTR (2, 5), grIF/grIR (3, 6), and grOF/grOR (4, 7) were used to detect the presence of corresponding sequence on genomic DNA of A. caldus MTH-04 wild type (2, 3, 4) and Δgr (5, 6, 7). The numbers on the left indicate the sizes of the fragments based on the molecular size marker (lane 1). (B) Line 2: Sac I-digested genomic DNA from Δgr, line 3: Sac I-digested genomic DNA from wild type. The molecular size marker was loaded on the left lane, and the sizes of its fragments are indicated (lane 1).
To study the effect of gr gene on heavy metal tolerance of A. caldus, Δgr, the gr overexpression strain and the control strains of wild type and the wild type carrying plasmid pJRD215 of A. caldus were grown in the Starkey-S0 medium with different concentrations of CuSO4 or ZnSO4. Without heavy metals, knockout or overexpression of gr did not affect the growth curves on S0 compared with the control strains (Figure 7A), suggesting that GR did not play a key role in the growth on S0.
Figure 7. Growth curves of the Acidithiobacillus caldus MTH-04 wild type, gr knockout mutant, gr overexpression strain, and control strain (wild type carrying pJRD215) grown in S0 medium under copper stress. A. caldus (wild) represents the wild type, Δgr represents the gr knockout mutant, A. caldus (pJRD215) represents the control strain, and A. caldus (pJRD215-tac-gr) represents the gr overexpression strain of A. caldus MTH-04, respectively. The concentrations of copper are indicated as well. (A) 0 mM Cu2+; (B) 5 mM Cu2+; (C) 10 mM Cu2+; (D) 20 mM Cu2+. OD460nm indicates the optical density at 460 nm, all measurements were performed in triplicate, and error bars correspond to the standard deviations.
All strains showed obvious growth lags under low concentrations of copper ions, and the inhibition was increased with the increase in copper ion concentrations. The growth of Δgr mutant was completely inhibited under 20 mM copper ions, while the gr overexpression strain showed a growth advantage over the wild type (Figure 7). The high sensitivity to copper ions of the Δgr mutant and the enhanced tolerance to copper ions of the gr overexpression strain indicated the involvement of gr gene in copper tolerance in A. caldus MTH-04.
The zinc tolerance of A. caldus strains was investigated as well. The increased growth inhibitions with the concentration of zinc ions were also observed in all A. caldus strains. Moreover, the Δgr mutant grew lowest with a longer growth delay, while the gr overexpression strain grew highest with a shorter growth delay under the same concentration of zinc ions (Figure 8). The above results indicated the important role of the gr gene in zinc tolerance in A. caldus MTH-04.
Figure 8. Growth curves of Acidithiobacillus caldus MTH-04 wild type, gr knockout mutant, gr overexpression strain, and control strain (wild type carrying pJRD215) grown in S0 medium under zinc stress. Acidithiobacillus caldus (wild) represents the wild type, Δgr represents the gr knockout mutant, A. caldus (pJRD215) represents the control strain, and A. caldus (pJRD215-tac-gr) represents the gr overexpression strain of A. caldus MTH-04, respectively. The concentrations of zinc are indicated as well. (A) 0 mM Zn2+; (B) 40 mM Zn2+; (C) 80 mM Zn2+; (D) 160 mM Zn2+. OD600nm indicates the optical density at 600 nm, all measurements were performed in triplicate, and error bars correspond to the standard deviations.
To further understand how gr may influence the expression patterns of glutathione-related genes in A. caldus, the relative mRNA levels of these genes were measured using the gr knockout strain with the wild type as the control or using the gr overexpression strain with the wild type carrying vacant pJRD215 plasmid as the control using the RT-qPCR measurement method. Without heavy metal stress, gr knockout resulted in increased expression levels of glutathione synthetase and glutathione peroxidase, while gr overexpression resulted in increased expression levels of glutathione reductase, thioredoxin reductase, and heterodisulfide reductase subunit C and decreased expression levels of peroxiredoxin, glutathione synthetase, and glutathione S-transferase (Table 4). These results suggest that the GR activity was involved in the glutathione system in A. caldus during elemental sulfur oxidization.
Table 4. Changes in the expression of genes in Acidithiobacillus caldus MTH-04 in gr knockout and gr overexpression strains.
When the strains were incubated with CuSO4, as shown in Table 5, most of the investigated genes were apparently upregulated in Δgr compared with the wild type. On the other hand, the increased expression levels of the genes were somewhat different from others. For instance, the expression levels of peroxiredoxin, thioredoxin-disulfide reductase, and heterodisulfide reductase subunit C were increased after incubation for either 1 or 2 h, while the expression levels of thiol peroxidase and sulfur dioxygenase only increased after incubation for 1 h. When the gr overexpression strain was compared with the control strain (wild type carrying plasmid pJRD215), the expression levels of glutathione reductase and heterodisulfide reductase subunit C were increased after incubation for 1 and 2 h, respectively, while the expression levels of thioredoxin-disulfide reductase and sulfur dioxygenase were decreased after incubation for 1 h, and the expression levels of peroxiredoxin were decreased after incubation for 2 h, respectively.
Table 5. Changes in the expression of genes in Acidithiobacillus caldus MTH-04 in gr knockout and gr overexpression strains under copper stress.
Table 6 presents the responses of the selected genes to ZnSO4. It shows that when compared with the wild type, the deletion of gr resulted in the upregulation of peroxiredoxin and heterodisulfide reductase subunit C and the downregulation of thioredoxin reductase, glutathione S-transferase, and sulfur dioxygenase after incubation for 1 h. The absence of gr also resulted in the upregulation of thiol peroxidase, peroxiredoxin, and thioredoxin reductase after incubation for 2 h. When compared with the control strain (wild type carrying plasmid pJRD215), the overexpression of gr resulted in the upregulation of glutathione reductase and heterodisulfide reductase subunit C after incubation for either 1 or 2 h and downregulation of peroxiredoxin after incubation for 2 h. The above results suggest that the GR activity was involved in heavy metal tolerance in A. caldus.
Table 6. Changes in the expression of genes in Acidithiobacillus caldus MTH-04 in gr knockout and gr overexpression strains under zinc stress.
A putative gr gene (F0726_RS04210) was detected in the genome of A. caldus MTH-04 by conducting a BLASTP search. The proteins with GR activity contain conserved functional motifs, including an NADPH binding site, a GSSG binding site, and a redox-active disulfide bond domain (Figure 3). Most of the GR homologs contain a highly conserved NADPH binding site sequence (RX5R). Moreover, the first arginine residue in the RX5R motif is conserved up to 100%, while the last arginine residue is replaced by different residues in different strains. In F0726_RS04210, a histidine residue replaces the last conserved arginine residue (RX5H). Similar phenomena were also reported in F. noatunensis subsp. orientalis str. Toba 04 (RX5M), S. meliloti (RX5S), and Nostoc sp. PCC7120 (RX5K). Replacing arginine with other basic amino acids (H or K) may affect the affinity of NADPH and result in a wider range of electronic sources, such as NADPH and NADH. In addition, the evolutionary relationship of F0726_RS04210 is close to the glutathione reductase from E. coli K12. Finally, we detected GR activity in the protein of F0726_RS04210. The above results indicate that F0726_RS04210 is a GR and may play a similar role as the reported GRs.
It is well established that A. caldus can obtain energy by oxidizing reduced inorganic sulfur compounds (RISCs) during chemolithoautotrophic growth in acidic environments. Oxidation of RISCs requires elemental sulfur (S0) as the initial primary and intermediate metabolite; however, limited information is available on the activation of elemental sulfur. Previous studies have proposed that elemental sulfur may be activated by GSH in Acidithiobacillus (Silver and Lundgren, 1968). However, the Δgr mutant of A. caldus still grew well when using elemental sulfur as the sole energy substrate. The results indicate that GR is not fatal in the oxidation of elemental sulfur in A. caldus.
In biometallurgy, ore leaching microorganisms are in an environment of high osmotic pressure and high concentration of heavy metals. Our recent research has explained the essential role of OmpR in A. caldus adapting to the high osmolarity (Chen et al., 2022), but little is known about the mechanism of heavy metal resistance. A copper-sensitive operon repressor was identified in A. caldus, which might be involved in putative copper resistance mechanisms (Hou et al., 2021). Due to the lack of effective genetic tools, this specific mechanism still needs to be verified. Heavy metals accumulate during the bioleaching process, and the stress tolerance process will result in reactive oxygen species (ROS) (Stadtman and Oliver, 1991; Natarajan et al., 1994). Recently, GR was reported to participate in the heavy metal tolerance of A. ferrooxidans (Xia et al., 2011; Zheng et al., 2015, 2016), so the role of GR in the heavy metal tolerance of A. caldus was investigated in this research. Deletion of gr resulted in increased sensitivity to heavy metals, while the overexpression of gr enhanced tolerance to heavy metals, which suggests the involvement of the gr gene in heavy metal tolerance in A. caldus MTH-04. Moreover, enzymes involved in the antioxidant pathway (for instance, thioredoxin reductase) and GSH-producing pathway (for instance, glutathione synthetase and heterodisulfide reductase subunit C) were altered when gr was deleted or overexpressed in A. caldus under heavy metal stress. Previous studies also reported that GR plays a key role in heavy metal tolerance by keeping high GSH/GSSG ratios (Schirmer et al., 1989; Creissen et al., 1994; Mullineaux and Creissen, 1997). The results indicate that GR may play a key role in heavy metal tolerance in A. caldus by sustaining the reduced status of glutathione.
We detect a gr gene in A. caldus and provide the report characterizing the gr gene by constructing a gr knockout mutant and a gr overexpression strain. We found that gr knockout results in increased sensitivity to heavy metals (Cu2+ and Zn2+) and revealed the strong correlations between GR and the antioxidant pathway in A. caldus. Finally, we propose the function of GR is to play an important role in heavy metal tolerance. Our findings provide a template for further investigation of GR in other microorganisms and can be further used to construct improved bioleaching strains.
The original contributions presented in the study are included in the article/supplementary material, further inquiries can be directed to the corresponding authors.
YS, YY, and XiaoL: methodology. JianqiL: formal analysis. XianL: validation. JianquL and XP: investigation and supervision. WW: initial draft and revised draft writing and editing. JianquL and XP: funding acquisition. All authors have read and agreed to the published version of the manuscript.
This study was supported by grants from the National Natural Science Foundation of China (30800011 and 31872621), the State Key Laboratory of Microbial Technology Foundation (M2017-01), the Natural Science Foundation of Shandong Province (ZR2020MC006), People's Republic of China, the Instrument Improvement Funds of Shandong University Public Technology Platform (ts20220104), the State Key Laboratory of Microbial Technology Open Projects Fund (Project No. M2022-03), and the Cooperation Project on Bioleaching Between Shandong University and Guangxi Senhe High-tech Co., Ltd.
The authors would like to thank the Core Facilities Sharing Platform for Life and Environment Sciences of Shandong University, including Cheng-Jia Zhang and Nan-Nan Dong for providing the bacteriological incubator and Zhi-Feng Li for RT-qPCR instruction.
The authors declare that the research was conducted in the absence of any commercial or financial relationships that could be construed as a potential conflict of interest.
All claims expressed in this article are solely those of the authors and do not necessarily represent those of their affiliated organizations, or those of the publisher, the editors and the reviewers. Any product that may be evaluated in this article, or claim that may be made by its manufacturer, is not guaranteed or endorsed by the publisher.
Asnis, R. E. (1955). A glutathione reductase from Escherichia coli. J. Biol. Chem. 213, 77–85. doi: 10.1016/S0021-9258(18)71046-6
Chen, L., Liu, X., Gao, C., Guan, Y., Lin, J., Liu, X., et al. (2022). The essential role of OmpR in Acidithiobacillus caldus adapting to the high osmolarity and its regulation on the tetrathionate-metabolic pathway. Microorganisms 11, 35. doi: 10.3390/microorganisms11010035
Creissen, G., Edwards, E. A., and Mullineaux, P. (1994). “Glutathione reductase and ascorbate peroxidase,” in Causes of Photooxidative Stress and Amelioration of Defense Systems in Plants, eds C. H. Foyer, and P. M. Mullineaux (Boca Raton, FL: CRC Press), 343–364. doi: 10.1201/9781351070454-13
Cui, S. (2011). Function Comparison and Analysis of Gene-cox, Cup, Iro of Acidithiobacillus ferrooxidans in Ferrous Iron Oxidation [M.Sc]. Jinan: Shandong University.
Davison, J., Heusterspreute, M., Chevalier, N., Ha-Thi, V., and Brunei, F. (1987). Vectors with restriction site banks V. pJRD215, a wide-host-range cosmid vector with multiple cloning sites. Gene 51, 275–280. doi: 10.1016/0378-1119(87)90316-7
Diego, G. A., Vanina, E. M., Alejandro, J. B., Sergio, A. G., and Alberto, A. I. (2010). Purification and characterization of a glutathione reductase from Phaeodactylum tricornutum. Protist 161, 91–101. doi: 10.1016/j.protis.2009.06.001
Dopson, M., Baker-Austin, C., Koppineedi, P. R., and Bond, P. L. (2003). Growth in sulfidic mineral environments: metal resistance mechanisms in acidophilic micro-organisms. Microbiology 149, 1959–1970. doi: 10.1099/mic.0.26296-0
Edwards, K. J., Bond, P. L., and Banfield, J. F. (2000). Characteristics of attachment and growth of Thiobacillus caldus on sulphide minerals: a chemotactic response to sulphur minerals? Environ. Microbiol. 2, 324–332. doi: 10.1046/j.1462-2920.2000.00111.x
Ekinci, D., and Sentürk, M. (2013). Assessment of metal inhibition of antioxidant enzyme glutathione reductase from rainbow trout liver. J. Enzyme Inhib. Med. Chem. 28, 11–15. doi: 10.3109/14756366.2011.615745
Foyer, C., Lelandais, M., Galap, C., and Kunert, K. J. (1991). Effects of elevated cytosolic glutathione reductase activity on the cellular glutathione pool and photosynthesis in leaves under normal and stress conditions. Plant Physiol. 97, 863–872. doi: 10.1104/pp.97.3.863
Greer, S., and Perham, R. N. (1986). Glutathione reductase from Escherichia coli: cloning and sequence analysis of the gene and relationship to other flavoprotein disulfide oxidoreductases. J. Biochem. 25, 2736–2742. doi: 10.1021/bi00357a069
Hallberg, K. B., and Lindström, E. B. (1994). Characterization of Thiobacillus caldus sp.nov., a moderately thermophilic acidophile. Microbiology 140, 3451–3456. doi: 10.1099/13500872-140-12-3451
Hallberg, K. B., and Lindström, E. B. (1996). Multiple serotypes of the moderate thermophile Thiobacillus caldus, a limitation of immunological assays for biomining microorganisms. Appl. Environ. Microbiol. 62, 4243–4246. doi: 10.1128/aem.62.11.4243-4246.1996
Hou, S., Tong, Y., Yang, H., and Feng, S. (2021). Molecular insights into the copper-sensitive operon repressor in Acidithiobacillus caldus. Appl. Environ. Microbiol. 87, e0066021. doi: 10.1128/AEM.00660-21
Jin, S., Yan, W., and Wang, Z. (1992). Transfer of IncP plasmids to extremely acidophilic Thiobacillus thiooxidans. Appl. Environ. Microbiol. 58, 429–430. doi: 10.1128/aem.58.1.429-430.1992
Kamimura, K., Okayama, T., Murakami, K., and Sugio, T. (1999). Isolation and characterization of a moderately thermophilic sulfur-oxidizing bacterium. Microbios 99, 7–18.
Liu, Y., Qi, F., Lin, J., Tian, K., and Yan, W. (2004). Isolation and phylogenetic analysis of a moderately thermophilic acidophilic sulfur oxidizing bacterium. Acta Microbiol. Sin. 44, 382–385. doi: 10.13343/j.cnki.wsxb.2004.03.025
Livaka, K. J., and Schmittgenb, T. D. (2001). Analysis of relative gene expression data using real-time quantitative PCR and the 2-[Delta][Delta] CT method. Methods 25, 402–408. doi: 10.1006/meth.2001.1262
Luo, Y., Liu, Y., Zhang, C., Luo, H., Guan, H., Liao, H., et al. (2008). Insights into two high homogenous genes involved in copper homeostasis in Acidithiobacillus ferrooxidans. Curr. Microbiol. 57, 274–280. doi: 10.1007/s00284-008-9189-6
Meister, A., and Anderson, M. E. (1983). Glutathione. Ann. Rev. Biochem. 52, 711–760. doi: 10.1146/annurev.bi.52.070183.003431
Michail, S., and James, A. B. (1977). Chloroplast glutathione reductase. J. Plant Physiol. 59, 1011–1012. doi: 10.1104/pp.59.5.1011
Mullineaux, P. M., and Creissen, G. P. (1997). “Glutathione reductase: regulation and role in oxidative stress,” in Oxidative Stress and the Molecular Biology of Antioxidant Defenses ed J. Scandalios (New York, NY: Cold Spring Harbor Laboratory Press), 667–713.
Natarajan, K. A., Sudeesha, K., and Rao, G. R. (1994). Stability of copper tolerance in Thiobacillus ferrooxidans. Antonie Van Leeuwenhoek 66, 303–306. doi: 10.1007/BF00882764
Nigel, S. S., Alan, B., and Richard, N. P. (1987). Purification and characterization of glutathione reductase encoded by a cloned and over-expressed gene in Escherichia coli. J. Biol. Chem. 245, 875–880. doi: 10.1042/bj2450875
Noctor, G., and Foyer, C. H. (1998). Ascorbate and glutathione: keeping active oxygen under control. Ann. Rev. Plant Physiol. Plant Biol. Med. 49, 249–279. doi: 10.1146/annurev.arplant.49.1.249
Okibe, N., Gericke, M., Hallberg, K. B., and Johnson, D. B. (2003). Enumeration and characterization of acidophilic microorganisms isolated from apilot plant stirredtank bioleaching operation. Appl. Environ. Microbiol. 69, 1936–1943. doi: 10.1128/AEM.69.4.1936-1943.2003
Rice-Evans, C. A., Miller, N. J., and Paganga, G. (1996). Structure-antioxidant activity relationships of flavonoids and phenolic acids. Free Rad. Biol. Med. 20, 933–956. doi: 10.1016/0891-5849(95)02227-9
Sambrook, J., and Russell, D. W. (2001). Molecular Cloning: A Laboratory Manual, 3rd ed. Cold Spring Harbor, NY: Cold Spring Harbor Laboratory Press.
Schirmer, R. H., Krauth-Siegel, R. L., and Schulz, G. E. (1989). “Glutathione reductase,” in Coenzymes and Cofactors: Glutathione, Vol. 3, eds D. Dolphin, O. Avaramovic, and R. Poulson (New York, NY: Wiley), 553–596.
Scruton, N. S., Berry, A., and Perham, R. N. (1990). Redesign of the coenzyme specificity of a dehydrogenase by protein engineering. Nature 343, 38–43. doi: 10.1038/343038a0
Silver, M., and Lundgren, D. G. (1968). Sulfur-oxidizing enzyme of Ferrobacillus ferrooxidans (Thiobacillus ferrooxidans). Biochem. Cell Biol. 46, 1215–1220. doi: 10.1139/o68-069
Simon, R., Priefer, U., and Pühler, A. (1983). A broad host range mobilization system for in vivo genetic engineering: transposon mutagenesis in gram negative bacteria. Nat. Biotechnol. 1, 784–791. doi: 10.1038/nbt1183-784
Smith, I. K., Polle, A., and Rennenberg, H. (1990). “Glutathione,” in Stress Responses in Plant Adaptation and Acclimation Mechanisms, eds R. G. Alscher, and J. R. Cumming (New York, NY: Wiley-Liss), 201–215.
Stadtman, E. R., and Oliver, C. N. (1991). Metal-catalyzed oxidation of proteins. J. Biol. Chem. 266, 2005–2008. doi: 10.1016/S0021-9258(18)52199-2
Wang, Z., Li, Y., Lin, J., Pang, X., Liu, X., Liu, B., et al. (2016). The two-component system RsrS-RsrR regulates the tetrathionate intermediate pathway for thiosulfate oxidation in Acidithiobacillus caldus. Front. Microbiol. 7, 1755. doi: 10.3389/fmicb.2016.01755
Wen, Q., Liu, X., Wang, H., and Lin, J. (2014). A versatile and efficient markerless gene disruption system for Acidithiobacillus thiooxidans: application for characterizing a copper tolerance related multicopper oxidase gene. Environ. Microbiol. 16, 3499–3514. doi: 10.1111/1462-2920.12494
Wu, W., Pang, X., Lin, J., Liu, X., Wang, R., Lin, J., et al. (2017). Discovery of a new subgroup of sulfur dioxygenases and characterization of sulfur dioxygenases in the sulfur metabolic network of Acidithiobacillus caldus. PLoS ONE 12, e0183668. doi: 10.1371/journal.pone.0183668
Xia, J. L., Wu, S., Zhang, R. Y., Zhang, C. G., He, H., Jiang, H. C., et al. (2011). Effects of copper exposure on expression of glutathione-related genes in Acidithiobacillus ferrooxidans. Curr. Microbiol. 62, 1460–1466. doi: 10.1007/s00284-011-9881-9
Zheng, C., Chen, M., Tao, Z., Zhang, L., Zhang, X. F., Wang, J. Y., et al. (2015). Differential expression of sulfur assimilation pathway genes in Acidithiobacillus ferrooxidans under Cd2+ stress: evidence from transcriptional, enzymatic, and metabolic profiles. Extremophiles 19, 429–4436. doi: 10.1007/s00792-014-0728-8
Keywords: glutathione reductase, Acidithiobacillus caldus, heavy metal tolerance, bioleaching, antioxidation
Citation: Shi Y, Wu W, Yang Y, Liu X, Lin J, Liu X, Lin J and Pang X (2023) Gene knockout of glutathione reductase results in increased sensitivity to heavy metals in Acidithiobacillus caldus. Front. Microbiol. 14:1250330. doi: 10.3389/fmicb.2023.1250330
Received: 30 June 2023; Accepted: 09 August 2023;
Published: 20 September 2023.
Edited by:
Ruiyong Zhang, Chinese Academy of Sciences (CAS), ChinaReviewed by:
Santosh Kumar, University of Wisconsin-Madison, United StatesCopyright © 2023 Shi, Wu, Yang, Liu, Lin, Liu, Lin and Pang. This is an open-access article distributed under the terms of the Creative Commons Attribution License (CC BY). The use, distribution or reproduction in other forums is permitted, provided the original author(s) and the copyright owner(s) are credited and that the original publication in this journal is cited, in accordance with accepted academic practice. No use, distribution or reproduction is permitted which does not comply with these terms.
*Correspondence: Jianqun Lin, amlhbnF1bmxpbkBzZHUuZWR1LmNu; Xin Pang, cGFuZ3hpbkBzZHUuZWR1LmNu
†These authors have contributed equally to this work
Disclaimer: All claims expressed in this article are solely those of the authors and do not necessarily represent those of their affiliated organizations, or those of the publisher, the editors and the reviewers. Any product that may be evaluated in this article or claim that may be made by its manufacturer is not guaranteed or endorsed by the publisher.
Research integrity at Frontiers
Learn more about the work of our research integrity team to safeguard the quality of each article we publish.