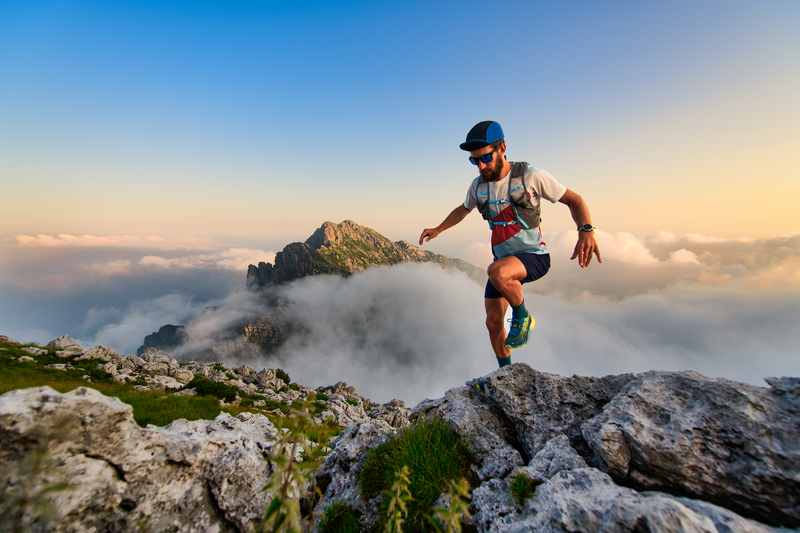
95% of researchers rate our articles as excellent or good
Learn more about the work of our research integrity team to safeguard the quality of each article we publish.
Find out more
REVIEW article
Front. Microbiol. , 19 October 2023
Sec. Ancient DNA and Forensic Microbiology
Volume 14 - 2023 | https://doi.org/10.3389/fmicb.2023.1249884
Recent efforts have been made to review the state of the art on a variety of questions and targets in paleoparasitology, including protozoan taxa. Meanwhile, these efforts seemed to let aside Cryptosporidium, and we then intended to review its paleoparasitological record to assess its past distribution and favored detection methods, and eventually highlight needed research trajectories. This review shows that contrary to other parasites, most of the positive results came from South-American sites and coprolites rather than sediment samples, highlighting the need to test this kind of material, notably in Europe where many negative results were reported in the published literature from sediment samples. Moreover, aDNA-based detections are nearly absent from the paleoparasitological record of this parasite, though punctually shown successful. With their potential to address the evolutionary history of Cryptosporidium species, notably through their 18S rRNA tree, aDNA-based approaches should be encouraged in the future. In sum, and though the limits of currently used methods and materials remain unclear, this review highlights the potential role of coprolites and aDNA for the study of Cryptosporidium species in the past and how this history shaped their current diversity and distribution, notably among human populations but also farm animals.
Paleoparasitology aims to study the remains of parasites extracted from a variety of samples in archeological, paleontological, environmental or medical contexts. At the crossroads between humanities and life-sciences, it can address questions related to the evolution and past ecology of these parasites, but also to past human communities in which these parasites were circulating (in terms of health, hygiene, food habits or waste management for example). Since its beginning, this discipline focused on helminths, and to a lesser extent, protozoans, including Cryptosporidium (Le Bailly et al., 2021).
Ernest Edward Tyzzer identified Cryptosporidium muris in 1907 in the gastric glands of laboratory mice, although the Linnaean name was only formally established in 1910 (Tyzzer, 1907, 1910). Based on many morphological and life cycle details, Tyzzer later identified C. parvum on the basis of its smaller oocyst size and different niche, among other things, that is to say, the small intestine rather than gastric glands when transmitted to laboratory mice (Tyzzer, 1912). However, half a century went by before veterinary and medical scientists and practitioners showed any interest in it. Indeed, Cryptosporidium was shown to be associated with bovine diarrhea in 1971 (leading to economic loss), and several human cases were reported in 1976 by independent working groups (White, 2010). In 1982, several cases of severe protracted diarrhea were reported in the US in men and associated with Acquired Immune Deficiency Syndrome. This definitively triggered the interest of the medical community in this parasite (Fayer et al., 1990).
Cryptosporidium belongs to the phylum Apicomplexa alongside Plasmodium or Toxoplasma, agents of malaria and toxoplasmosis, respectively (Votýpka et al., 2017). Cryptosporidium spp. were classified within the Coccidia class, but as a result of recent evidence, including their newly resolved phylogenetic relationship through their 18S rRNA tree, ultra-structural similarities between C. parvum and gregarines, or their ability to develop their life cycle outside a host cell, they have now been reclassified in the sub-class Cryptogregaria, class Gregarinomorphea, that is to say a sister group of gregarines, rather than among coccidian parasites (Morrison, 2009; Hijjawi et al., 2010; Cavalier-Smith, 2014; Koh et al., 2014; Clode et al., 2015; Aldeyarbi and Karanis, 2016; Thompson et al., 2016).
Up until recently, the field of paleoparasitology mainly dealt with helminths (Nematodes, Cestodes, Trematodes and Acantocephalans). This is easily explained by the possibility to detect their microscopic eggs in archeological, paleoenvironmental, museum or paleontological samples from oviparous species (Le Bailly et al., 2021). Indeed, these microscopic eggs are resistant enough to be preserved through the millennia whereas soft tissue bodies tend to disappear quickly under taphonomical constraints (although mummy remains may preserve such bodies in rare circumstances). Moreover, these eggs can be recognized to the genus level (rarely to the species level) depending on their size, shape, ornamentation, or biological origin. Researchers have thus been looking for fossil or subfossil eggs under light microscopy since the early twentieth century (Ruffer, 1910).
When it comes to protozoans though, this is not a straightforward procedure, as even when producing oocysts, these parasites generally appear to be fragile, small, and lack any specific morphology. This is well exemplified by Cryptosporidium parvum oocysts, ranging from 4 to 6 μm, making them difficult to observe among environmental or fecal residues, and barely distinguishable from other micro-remains, such as fungal spores. Moreover, sample preparation for a microscopic approach includes a micro-sieving step (for example in the Rehydration–Homogenization–Micro-sieving protocol known as RHM protocol) during which the smaller mesh measures 20–25 μm, which is incompatible with the recovery of Cryptosporidium oocysts if the final flow through remains unchecked (Dufour and Le Bailly, 2013).
This does not mean that the paleoparasitology of protozoans is non-existent, far from it. Ten years ago, Frías and colleagues published an impressive review of the published literature on protozoans in ancient samples (Frías et al., 2013). Among other things, they shed light on the paleontological record of protozoans preserved in amber pieces. This is exemplified by a trypanosomatid of the genus Paleoleshmania observed in a female sandfly trapped in Cretaceous Burmese amber and proving the existence of these vector-borne parasites and Early Cretaceous interactions (Poinar and Poinar, 2004). This also encompasses apicomplexans (phylum to which Cryptosporidium spp. belong), as Plasmodium dominicana was recorded in Tertiary Dominican Republic amber. The ancient Apicomplexa record also includes intestinal species such as Eimeria lonatoi oocysts observed in much more recent deer coprolites, dated to 9,000 years BP in Brazil (Ferreira et al., 1992; Poinar, 2005). This record shows that paleoparasitological observations are not necessarily incompatible with the passing millennia, even when it comes to light microscopy-based diagnosis and protozoans.
More recently, the growing stock of data enabled Le Bailly and colleagues to contribute to this research and to review archeological occurrences of the human amoeba Entamoeba histolytica over the past 6,000 years (Gonçalves et al., 2004; Le Bailly et al., 2016). The authors showed that this protozoan had been circulating in Western Europe since at least the Neolithic period (5,700 years BP) and could have originated in the Old World before spreading toward the pre-Columbian Americas around the twelfth century. Interestingly, this review shows the importance of Enzyme Immunoassays (EIA), as nearly all reviewed papers used Enzyme-Linked immunoSorbent Assay kits (ELISA) to detect E. histolytica in ancient samples. This illustrates how molecular techniques can bypass microscopy limitations and complete classical approaches.
In the last 20 years, the contribution of aDNA-based methods to the field of paleoparasitology has greatly increased, including for protozoans (Côté and Le Bailly, 2017; Wood, 2018). One of the first papers focused on Trypanosoma cruzi aDNA extraction from 2000-year-old Chilean mummies (Madden et al., 2001). Since then, cutting-edge techniques have been applied on several occasions, notably for the recovery and comprehensive evolutionary and ecological history of Plasmodium spp. in humans (e.g., Gelabert et al., 2016; Marciniak et al., 2016) and other infectious pathogens (Arriola et al., 2020).
A rapid overview of protozoans in the paleoparasitological literature suggests that Cryptosporidium lags behind other targets despite its current importance in medical and veterinary domains. Consequently, in this paper, we intend to comprehensively review the topic, highlighting current research based on modern Cryptosporidium strains.
Cryptosporidium is a single-celled parasite with a monoxenous life cycle, meaning that its whole development takes place in a single host before being transmitted to a new one. After sexual and asexual development, thin-walled oocysts excyst inside their current host, leading to autoinfection, while thick-walled oocysts spread in the environment through defecation and are transmitted to a new host (Bouzid et al., 2013). Transmission may occur via the fecal-oral route through the consumption of contaminated water or food, by human-to-human contact (anthroponotic), or non-human-animal-to-human contact (zoonotic) (Xiao, 2010). Cryptosporidium infects the gastrointestinal tract of a wide diversity of animal hosts, including human beings, but also other mammals, birds, reptiles and fish (Fayer, 2010; Zahedi and Ryan, 2020). The severity and form of symptoms can vary widely according to several factors, including host species, age, immunity, nutritional state, genetic background, infection site, or Cryptosporidium species and subtype (Flores and Okhuysen, 2009; Borad and Ward, 2010; Chalmers and Davies, 2010; Sponseller et al., 2014; Checkley et al., 2015). It is one of the main waterborne parasites across the world infecting humans due to its well-known resistance to basic water plant disinfectants, such as chlorination (Fayer et al., 1990; Baldursson and Karanis, 2011), but also as a result of the absence of efficient preventive or curative drugs (Dhal et al., 2022; Helmy and Hafez, 2022; Khan and Witola, 2023). C. hominis and C. parvum are the main strains currently known to infect human hosts. The former is more commonly found in developing countries than the latter although several other strains are known to cause zoonotic transmissions, such as C. cuniculus (Feng et al., 2018; Gilchrist et al., 2018; Zahedi and Ryan, 2020; Tichkule et al., 2021). With a global prevalence of 7.6% between 1960 and 2018 (Dong et al., 2020), Cryptosporidium is an important agent of diarrhea in humans and non-human animals, with noticeable impacts in terms of morbidity and mortality, primarily among immunocompromised people and children in low-income countries (Kotloff et al., 2013; GBD 2016 Diarrhoeal Disease Collaborators, 2018) but also in more developed regions (Costa et al., 2020) where its specific burden may be underestimated (McDonald et al., 2001; White, 2010) as well as its specific etiological agents (Robinson et al., 2008; Chalmers et al., 2009; Morris et al., 2019). Despite varying national surveillance strategies, notably in terms of genotyping efforts, C. parvum and C. hominis are shown to dominate among humans, with marked seasonality peaks of infection in late spring, late summer/early autumn in Europe (McLauchlin et al., 2000; Xiao, 2010; Cacciò and Chalmers, 2016).
The map illustrated in Figure 1 documents detections of the past record of Cryptosporidium and reported negative results from the published literature. The mapping IDs of the archeological sites are reported in Tables 1, 2 with the corresponding site names, dating, sample types and the methods used for Cryptosporidium detection.
Figure 1. Archeological sites where Cryptosporidium detection attempts have been published. Nearly all positive sites are located in the New World, while negative ones are mostly located in the Old World. The correlation coefficient between the approximated central date in years BP and the ratio of positive samples for each site was negatively weak, suggesting a slightly decreasing ratio of positive samples with greater age, but this was not statistically significant (Kendall’s τ = −0.193; p = 0.149).
As far as we know, the first mention of this protozoan appeared in the published literature in 1999. The authors looked for Cryptosporidium oocysts using Immuno Fluorescence Assay under the microscope (IFA), then Enzyme-Linked immunoSorbent Assay from 500 to 3000-year-old feces preserved in human mummies’ intestines from the Andean region in South America (Allison et al., 1999). Out of 39 individuals, 15 tested positive to Cryptosporidium oocysts under IFA and were subsequently tested with ELISA. Eight of these were finally confirmed. The archeological context is barely discussed in the paper, with references limited to the “Andean region” and very loose dating. Nevertheless, it did raise several important points: first, by showing that Cryptosporidium was not beyond the reach of paleoparasitology despite the fact that its oocysts are virtually undistinguishable in ancient samples under light microscopy alone; secondly, by showing that cryptosporidiosis must have plagued humans long before it appeared as a medical concern in the 1980s with massive and newly monitored epidemic events in the 1990s; and third, that C. parvum was certainly rife in pre-contact America, that is to say, before European colonization.
A few years later, a new paper reported Cryptosporidium detection in pre-Columbian Peru (Ortega and Bonavia, 2003). The authors screened 22 archeological feces (nearly always referred to as “coprolites” in archeological science literature) with IFA only. One of these feces collected on the P35-4 archeological site associated with the Peruvian Middle Horizon culture and dated to 770–830 CE was positive to Cryptosporidium sp. oocysts. This paper brought more evidence for pre-contact Cryptosporidium sp. in South America. Interestingly, the authors raised the question of surface antigen taphonomy and preservation over time and if any, their possible evolutionary change on a historical time scale. However, they did not have sufficient evidence to address the question.
Ten years later, Cryptosporidium was once again detected in ancient samples (Wood et al., 2013). The authors studied the parasitic diversity of several extinct moa in New Zealand through the light microscopy analysis of 84 well-preserved coprolites belonging to three distinct ratite bird species, and 16 were also analyzed with molecular analyses. The authors extracted the whole DNA from coprolite subsamples then amplified the obtained extracts using a previously designed primer set (Table 3) targeting a 350–400-bp-long fragment of the 18S gene, which is conserved in invertebrates (Figure 2A). The amplified extracts were subsequently cloned, and Sanger sequenced before being identified through BLAST alignments. One sequence successfully obtained from a single coprolite collected on the late Holocene Dart River Valley site, South Island, and associated with the extinct moa species Megalopteryx didinus, fell within the clade including the Cryptosporidium species, more specifically as a sister group to C. ‘struthionis’. This genotype is currently known to infect modern-day ostriches. The phylogenetic analysis later supported a ratite clade with the ostrich-infecting Cryptosporidium and basal to the two previously reported Cryptosporidium clades from other vertebrates. The authors suggested that it could hint toward the biogeography hypothesis of the Gondwanan Vicariance, not only documented through host divergence and isolation through time, but also through their parasites. The authors also raised the question of host–parasite coextinction phenomena, as Cryptosporidium was only identified from Megalopteryx in this study, but the scarcity of the sample set prevented further considerations (Wood et al., 2013). As subsequently noted, the long evolutionary C. ‘struthionis’ branch would certainly be refined and split in light of novel, more geographically and temporally diverse isolates, including aDNA (Garcia-R and Hayman, 2016). This highlights the crucial role of aDNA and paleoparasitology in understanding the evolutionary history, current diversity and associations of hosts and parasites.
Table 3. Primer sets designed for the recovery of Cryptosporidium aDNA targeting different regions of its 18S gene.
Figure 2. Alignment of selected complete 18S Cryptosporidium sp. sequences deposited on Genbank showing targeted regions in aDNA based papers (A). Neighbor Joining tree with Jukes-Cantor measure in 1000 bootstrap replicates based on Cryptosporidium 18S complete sequences rooted on C. fragile (EU162753), primary infections sites are adapted from Šlapeta (2017) (B).
In 2016, Cryptosporidium was again detected in human coprolites (Morrow and Reinhard, 2016). Ninety coprolites from the archeological site of La Cueva de los Muertos Chiquitos, in the Rio Zape Valley, Mexico, dated to 600–800 CE and associated with the Loma San Gabriel culture, were analyzed with ELISA to test for several protozoan parasites, including C. parvum. A total of 66 samples corresponding to a 73% detection frequency appeared as positive or likely to be positive to C. parvum in that study. It proved the presence of C. parvum in pre-contact Mesoamerica (previously shown in South America) and enabled the authors to discuss the pathoecology of Cryptosporidium among the ancient Loma San Gabriel people, in particular in terms of subsistence strategy and child mortality in light of previously obtained paleoparasitological and bioanthropological data from the same site. It also yielded the largest number of analyzed coprolites of human origin from a single archeological site to date. In this regard, the high detection frequency may raise some questions and legitimately triggered the authors’ interest (Morrow and Reinhard, 2016). Statistical analysis did not show any spatial association of positive samples with percolation and leaching phenomena in the different archeological squares and strata. Furthermore, the authors took care to cold conserve their samples to avoid fungal and bacterial growth that may lead to cross-reactions in coproantigen-based assays and ultimately to false positives (Morrow and Reinhard, 2016). These questions mirror those raised by Ortega and Bonavia (2003), not only regarding antigen stability, taphonomy and evolution through time leading to false negatives, but also their obliteration in cross-reaction phenomena, leading to false positives, and call for a closer monitoring of this matter in the future.
In 2017, 25 coprolites from Myotragus balearicus were screened for the presence of C. parvum using ELISA tests (Borba Nunes et al., 2017). M. balearicus is an extinct Caprinae endemic to the Eastern Balearic Islands. The source material was collected from Cova Estreta, Pollença, Serra a Tramuntana, Mallorca, and dated to 4,950 ± 38 BP. Among the sample set, nine samples tested positive for C. parvum. This paper brought up several important points. First of all, it was, and as far as we know, remains to date the only paleoparasitological detection of Cryptosporidium in Europe. Secondly, it echoes previously cited work testing for parasites of extinct moas in New Zealand (Wood et al., 2013), raising the same questions about coevolutionary and coextinction process between hosts and parasites during the Holocene. Finally, it also showed that ELISA tests were a functional tool in this matter too, as Wood and colleagues looked for aDNA alongside microscopy, but did not employ any EIA.
More recently, 60 samples consisting of anthropogenized sediment or preserved human feces were collected from 15 archeological sites in South America (Leles et al., 2019). The sites are located in Brazil, Chile and Peru, ranging from 9,800 ± 80 years BP to 400 ± 50 years BP, that is to say an extensive part of the Holocene encompassing pre-and post-contact chrono-cultural contexts. Most sites yielded human coprolites, but a couple also yielded human sediment, canid, feline, and rodent coprolites. ELISA tests were used to detect Cryptosporidium in the samples alongside other protozoans. Sixteen samples appeared positive to Cryptosporidium sp., corresponding to a 26.6% detection rate in the sample set. The oldest positive samples come from human and rodent coprolites from the same archeological site and were dated to 7,230 ± 80 BP. Interestingly, the authors critically tested the yields of immunoenzymatic techniques through the comparison of results obtained with microscopy, ELISA, IFA and PCR. This experimental approach was based on Giardia duodenalis, which was detected in 48 samples (46.6%). In spite of the high detection frequency among archeological samples using immunoenzymatic tests, the authors could not amplify any G. duodenalis sequences, even though specifically designed primer sets were previously shown to work on modern and experimentally taphonomized feces. According to the authors, this discrepancy raises the question of the specificity of immunoenzymatic tests for archeological materials and necessitates further studies (Leles et al., 2019). Their paper echoes previously raised questions and adds experimental data.
At last, 9 coprolites from Puerto Rican indigenous cultures in Vieques and dated to 540–1,400 years BP were analyzed through light microscopy and metagenomic shotgun sequencing (Wiscovitch-Russo et al., 2020). While microscopy allowed the recovery of several helminth taxa, non-targeted whole genome amplification (WGA) and sequencing allowed the recovery of one sequence latter associated to Cryptosporidium spp. HSP70 protein coding gene through BlastX and phylogenetic analysis. Interestingly this paper confirmed the need of integrative approach previously suggested by Côté et al. (2016) as aDNA and microscopy-based data did not overlap. The authors subsequently used network modeling to recreate parasite–host interactions in conjunction with zooarcheological data and highlighted the crucial role of rodents and canids in the transmission of zoonotic parasites among ancient Huecoid and Saladoid communities that co-existed at the Sorcé Site. Meanwhile, Cryptosporidium was not included in this analysis due to the lack of accuracy of the sequence. Though shotgun-based approaches are commonly used for the study of ancient intestinal microbiomes (e.g., Hagan et al., 2020; Jacobson et al., 2020), protozoa like Cryptosporidium may be flooded within the bacterial signal thence leading to false negative or unspecific results.
Several points emerge from these papers showing positive results for Cryptosporidium in the past. First, to our knowledge, only seven papers mention positive results for 15 archeological or paleontological sites (Table 1). Moreover, 13 sites are located in South or Meso-America, while the remaining ones (N = 2) are in Europe and Oceania, respectively (Figure 1). Coprolites were the main materials for the recovery of Cryptosporidium on these 15 archeological sites. South and Meso-American sites are mostly dated to the pre-contact period, ranging from 8,170 years BP to the late Inca period. European and Oceanian sites are dated to 4,950 years BP and the late Holocene (<3,000 BP) respectively. On the other hand, we found eight papers reporting negative results on 15 archeological sites, six of which are in Brazil (ranging from 9,670 to 2,290 years BP), while nine sites are in the Old World (Italy, Switzerland, Belgium, France and Israel, ranging from the Iron Age to the nineteenth century) (Figure 1). Among these negative reports, coprolites and sediment were indicated as the source material in eight and eight analyses, respectively, (one site used both). In both groups (positive and negative sites), ELISA was the main diagnostic tool, complemented with IFA and PCR/Sanger sequencing on rare occasions (Table 2).
This contrasts with the reviewed literature regarding E. histolytica (Le Bailly et al., 2016). Indeed, Le Bailly and colleagues identified 27 archeological sites, most of which (N = 19) were located in the Old World, more specifically in Europe (Switzerland, Greece, France, Belgium, Italy, Latvia and Israel), while a minority (N = 8) were located in the New World (USA, Guadeloupe, Chile, Peru, Argentina). We could suggest that light microscopy detection leads to more observations of E. histolytica, yet only two papers mentioned light microscopy while all the other diagnoses were based on ELISA tests. These results could also be imputed to the fact that paleoparasitologists’ interest in E. histolytica began earlier than their interest in Cryptosporidium. However, while the first paper regarding the latter was published in 1999, only two earlier papers focus on E. histolytica (Witenberg, 1961; Fouant et al., 1982; Allison et al., 1999). This situation may also imply that Old World researchers or those working with Old World materials, are not interested in Cryptosporidium, and that consequently this protozoan is under explored in the literature and virtually absent in the past record. But this is refuted by the published literature mentioning many failed attempts to detect Cryptosporidium in the Old World (Le Bailly, 2005; Mitchell et al., 2008; Yeh et al., 2015; Graff et al., 2020; Ledger et al., 2021; Rollins et al., 2021; Mitchell et al., 2023), to which we can add our own unpublished negative results. Overall, these attempts employ similar methods to those used for positive results, mainly ELISA tests, with a few exceptions. Moreover, other gastrointestinal parasites, including protozoans, are systematically detected with ELISA (e.g., Mitchell et al., 2023; Table 2). Therefore, we can suggest that a generally smaller number of tested samples in the Old World and/or taphonomical constraints explain this asymmetrical record.
It is important to underline that nearly all the positive results for Cryptosporidium come from coprolites (Figure 3). Interestingly, this is also true for the only two positive results from outside the Americas, namely M. balearicus coprolites from the Balearic Islands in Spain (Western Mediterranean), and M. didinus coprolites from New Zealand (Wood et al., 2013; Borba Nunes et al., 2017), both from extinct species. In the former, C. parvum was identified through ELISA testing, and a fragment of its 18S gene was sequenced from the extracted aDNA in the latter. A chi-square test of independence was applied to the whole data set compiled for this review to examine the relationship between sample type (coprolite or sediment) and Cryptosporidium detection (positive or negative). Coprolites were significantly associated with a higher detection frequency, both when considering all performed tests (ELISA, IFA, and PCR), χ2(1, N = 305) = 17.2499, p < 0.01, and ELISA tests only, χ2(1, N = 231) = 25.0108, p < 0.01.
Figure 3. Positive and negative outcomes depending on the source material (coprolite or sediment). Only ELISA tests are included.
The association between sample type and detection outcome would appear to confirm that coprolites are indeed a better material for the recovery of Cryptosporidium. However, this is not so clearcut as most of the sediment samples were collected from skeletal pelvic areas or latrines/cesspools, and thus highly spoiled with fecal residues. But we must be extremely cautious as the sediment sample set is very small (N = 50; 47 when considering ELISA only) and sampling bias cannot be excluded.
Overall, immunodiagnostic-based papers report negative and positive controls following manufacturers’ standards, lending credibility to the positive and negative results presented in Tables 1, 2. Meanwhile, it cannot totally exclude taphonomic biases as discussed by Leles et al. (2019). On the other hand, aDNA-based approaches follow stringent protocols from the field of paleogenetics, including extraction blanks. When using newly designed primer sets for Cryptosporidium though, it is not always clear if they were tested and validated on fresh and/or experimentally taphonomized materials beforehand as described by Leles et al. (2019) regarding G. intestinalis. This can make negative results more difficult to interpret, as shown in the study by Rollins et al. (2021), but ELISA tests tended to confirm them.
The previously mentioned possibility of false positive results when using coproantigens from coprolites would not be an issue for aDNA-based results, as published by Wood et al. (2013). But aDNA-based tests only account for a tiny minority of the whole sample set. Moreover, on at least two occasions, sediment materials also yielded positive results in the Americas, namely from the Sítio Fonseca, São Paulo, Brazil, dated to 1,010 ± 100 BP, and from the Vale do Rio Chillon site, Lima, Peru, dated to the Late Inca period (Leles et al., 2019). Coprolites appear to be more prone to Cryptosporidium detection on the one hand, but on the other hand, the use of sediment material does not seem to preclude such detection. These results may be explained by several factors regarding taphonomic conditions in coprolites and sediment samples: (1) remains of parasites are much more diluted in sediment samples compared with coprolites consisting in discrete specimens of concentrated fecal material in stratigraphic units; (2) latrines and cesspools from which originate many negative sediment samples may be particularly subjected to continual degradation due to biological taphonomic agents like fungi, mites, and nematodes as suggested by Reinhard et al. (2019) compared with individual coprolites quickly buried and not triggering the same amount of biological activity. Furthermore, the detection of Cryptosporidium may be due to wider favorable conservation conditions at the local site, the same that actually favored the preservation of coprolites in the first place and explain the vast published literature on this topic from American sites (Shillito et al., 2020).
A few key points seem to emerge for future research. First of all, it is essential to build on previously asked questions about coproantigen sensitivity and specificity in archeological materials. Secondly, larger sample sets and coprolites from the Old World now need to be explored. But coprolites can be harder to find in Europe than in other regions of the world with varying conservation conditions, and could be replaced by human mummified intestinal tissues, known to occur in a wide variety of chrono-cultural contexts around the Mediterranean. Indeed, while we expect to find only thick-walled oocysts released in coprolites, intestinal tissues may still carry thin-walled oocysts, trophozoites and other stages of the previously mentioned asexual and sexual Cryptosporidium life cycle infecting numerous enterocytes. Not only can these stages be theoretically observed through SEM, but the abundance of genetic material may also facilitate aDNA detection. Thirdly, the use of molecular tools must be developed to complement immunoenzymatic tests. The latter are fast and easy to set up, but they are designed for fresh stool samples rather than archeological materials and restricted to a narrow species spectrum. On the other hand, aDNA-based methods have a long track record of archeological-like materials adaptation and allow catching a wide genetic diversity including unknown or extinct taxa, but necessitate deeper and time consuming designs from bioinformatics to wet lab experiments. Indeed, only two papers mentioned aDNA-based targeted attempts to detect Cryptosporidium from archeological or paleoenvironmental materials (Wood et al., 2013; Rollins et al., 2021). Both papers targeted the 18S gene thereby facilitating aDNA recovery. The 18S copy number (20 per oocyst) makes it an appropriate candidate for aDNA extraction considering that Cryptosporidium reductive evolution deprived it of high copy number mitochondrial DNA. Several primer sets were designed and tested with ancient samples (Table 3), both targeting hyper variable regions catching a wide variety of species, and highly conserved ones (Figure 2A). Moreover, a number of primer sets targeting the Cryptosporidium 18S gene were independently designed for the analysis of modern samples, some of which would certainly be suited to aDNA recovery based on their short PCR product (not reported in this paper). Last but not least, 18S allows evolutionary relationship inferences among Cryptosporidium species and genotypes, showing clustering according to primary infection location and host species (Figure 2B). It would be extremely interesting to include ancient Cryptosporidium 18S genes in this tree, be it from extinct or still extant strains.
As mentioned above, aDNA fragments of Cryptosporidium were extracted from extinct flightless birds in New Zealand (Wood et al., 2013). Interestingly, modern C. ‘struthionis’ partial sequences subsequently deposited on Genbank tend to be from farmed mammals (yak, cattle, and pig) in the Qinghai-Tibetan Plateau Area, China (Figure 4). While isolates tend to form distinct clades depending on their host (respectively yak or cattle), the ancient moa sequence from New Zealand clustered with a modern farmed pig isolate, but remained a sister group of the originally identified ostrich isolate (Figure 4). This should not come as a surprise as Cryptosporidium species and genotypes were reported in modern isolates from at least 150 mammal species and 30 bird species, with some very broad host spectrums (Fayer, 2010). Without any doubt, adding ancient sequences, both from farm animals recovered in archeological deposits, and from extinct wildlife, will benefit our understanding of the spread of parasites and host adaptation through time. The need of documenting ancient sequences of Cryptosporidium is also highlighted by recent works based on modern genomes. These works suggested that after initial diversification following the Cretaceous-Paleogene transition (K-Pg boundary), past human activities in historical times (as transatlantic migrations and sanitation development) may explain the current diversity and distribution of this parasite among humans (Garcia-R and Hayman, 2016; Nader et al., 2019; Cabarcas et al., 2021; Tichkule et al., 2022). These hypotheses may be tested through aDNA recovery as illustrated in the case of other infectious agents as Mycobacterium tuberculosis (Brynildsrud et al., 2018). Furthermore, other works including eukaryotic pathogens highlighted the interest to obtain ancient DNA to better understand not only the past distribution of currently neglected infectious agents, but also their molecular evolution including pathogenicity factors over time, or their population genomics up to the present days (Spyrou et al., 2018; Doyle et al., 2022).
Figure 4. Neighbor Joining tree with Jukes-Cantor measure in 1000 bootstrap replicates based on Cryptosporidium ‘struthionis’ 18S partial sequences deposited on Genbank and rooted on C. fragile (EU162753). Icons show animal hosts or environmental samples from which Cryptosporidium was isolated.
In this paper, we aimed to review the published literature regarding Cryptosporidium detection in the past. We showed that positive detections do exist but are rarer when it comes to Cryptosporidium compared to other parasites, including protozoans such as E. histolytica. Nonetheless, the past record of Cryptosporidium is well attested, mainly in the pre-contact Americas. Very few positive results have emerged from European and more recent archeological sites, but this may be due to small sample size and source material (sediment rather than coprolites), as coprolites appear to be a better source material for the recovery of Cryptosporidium. Nearly all reports, positive and negative, used ELISA tests. aDNA-based methods may certainly complement Cryptosporidium detection methods, expand the currently known past record, and participate in a wide array of questions, be it in terms of parasitology, evolutionary, or archeological sciences. Such methods are strategically located at the crossroads of those disciplines, and could potentially relate the emergence of pathogenic strains to past human activities, as previously suggested by modern-day genome studies, shed light on their development and evanescence, and enhance our understanding of their current distribution and diversity.
KR and MLB conceived the manuscript. KR wrote the manuscript and conceived the figures. FD, NC, RB, IJ-B, BV, and FG critically reviewed and edited the final manuscript. All authors contributed to the article and approved the submitted version.
This work was supported by the TRANSBIO Graduate School (contract ISITE BFC ANR-15-IDEX-0003), the Region of Bourgogne-Franche-Comté for the PPG-3 program, and the PEA²T technical platform of the Chrono-environment laboratory.
The authors declare that the research was conducted in the absence of any commercial or financial relationships that could be construed as a potential conflict of interest.
All claims expressed in this article are solely those of the authors and do not necessarily represent those of their affiliated organizations, or those of the publisher, the editors and the reviewers. Any product that may be evaluated in this article, or claim that may be made by its manufacturer, is not guaranteed or endorsed by the publisher.
Aldeyarbi, H. M., and Karanis, P. (2016). The ultra-structural similarities between Cryptosporidium parvum and the gregarines. J. Eukaryot. Microbiol. 63, 79–85. doi: 10.1111/jeu.12250
Allison, M. J., Bergman, T., and Gerszten, E. (1999). Further studies on fecal parasites in antiquity. Am. J. Clin. Pathol. 112, 605–609. doi: 10.1093/ajcp/112.5.605
Arriola, L. A., Cooper, A., and Weyrich, L. S. (2020). Palaeomicrobiology: application of ancient DNA sequencing to better understand bacterial genome evolution and adaptation. Front. Ecol. Evol. 8:40. doi: 10.3389/fevo.2020.00040
Baldursson, S., and Karanis, P. (2011). Waterborne transmission of protozoan parasites: review of worldwide outbreaks - an update 2004-2010. Water Res. 45, 6603–6614. doi: 10.1016/j.watres.2011.10.013
Borad, A., and Ward, H. (2010). Human immune responses in cryptosporidiosis. Future Microbiol. 5, 507–519. doi: 10.2217/fmb.09.128
Borba Nunes, V. H., Alcover, J. A., Silva, V. L., Cruz, P. B., Machado-Silva, J. R., and de Araújo, A. J. G. (2017). Paleoparasitological analysis of the extinct Myotragus balearicus bate 1909 (Artiodactyla, Caprinae) from Mallorca (Balearic Islands, Western Mediterranean). Parasitol. Int. 66, 7–11. doi: 10.1016/j.parint.2016.11.009
Bouzid, M., Hunter, P. R., Chalmers, R. M., and Tyler, K. M. (2013). Cryptosporidium pathogenicity and virulence. Clin. Microbiol. Rev. 26, 115–134. doi: 10.1128/CMR.00076-12
Brynildsrud, O. B., Pepperell, C. S., Suffys, P., Grandjean, L., Monteserin, J., Debech, N., et al. (2018). Global expansion of Mycobacterium tuberculosis lineage 4 shaped by colonial migration and local adaptation. Sci. Adv. 4:eaat5869. doi: 10.1126/sciadv.aat5869
Cabarcas, F., Galvan-Diaz, A. L., Arias-Agudelo, L. M., García-Montoya, G. M., Daza, J. M., and Alzate, J. F. (2021). Cryptosporidium hominis Phylogenomic analysis reveals separate lineages with continental segregation. Front. Genet. 12:740940. doi: 10.3389/fgene.2021.740940
Cacciò, S. M., and Chalmers, R. M. (2016). Human cryptosporidiosis in Europe. Clin. Microbiol. Infect. 22, 471–480. doi: 10.1016/j.cmi.2016.04.021
Cavalier-Smith, T. (2014). Gregarine site-heterogeneous 18S rDNA trees, revision of gregarine higher classification, and the evolutionary diversification of Sporozoa. Eur. J. Protistol. 50, 472–495. doi: 10.1016/j.ejop.2014.07.002
Chalmers, R. M., and Davies, A. P. (2010). Minireview: clinical cryptosporidiosis. Exp. Parasitol. 124, 138–146. doi: 10.1016/j.exppara.2009.02.003
Chalmers, R. M., Robinson, G., Elwin, K., Hadfield, S. J., Xiao, L., Ryan, U., et al. (2009). Cryptosporidium sp. rabbit genotype, a newly identified human pathogen. Emerg. Infect. Dis. 15, 829–830. doi: 10.3201/eid1505.081419
Checkley, W., White, A. C. Jr., Jaganath, D., Arrowood, M. J., Chalmers, R. M., Chen, X. M., et al. (2015). A review of the global burden, novel diagnostics, therapeutics, and vaccine targets for Cryptosporidium. Lancet Infect. Dis. 15, 85–94. doi: 10.1016/S1473-3099(14)70772-8
Clode, P. L., Koh, W. H., and Thompson, R. C. A. (2015). Life without a host cell: what is Cryptosporidium? Trends Parasitol. 31, 614–624. doi: 10.1016/j.pt.2015.08.005
Costa, D., Razakandrainibe, R., Valot, S., Vannier, M., Sautour, M., Basmaciyan, L., et al. (2020). Epidemiology of cryptosporidiosis in France from 2017 to 2019. Microorganisms 8:1358. doi: 10.3390/microorganisms8091358
Côté, N. M., Daligault, J., Pruvost, M., Bennett, E. A., Gorgé, O., Guimaraes, S., et al. (2016). A new high-throughput approach to genotype ancient human gastrointestinal parasites. PLoS One 11:e0146230. doi: 10.1371/journal.pone.0146230
Côté, N. M.-L., and Le Bailly, M. (2017). Palaeoparasitology and palaeogenetics: review and perspectives for the study of ancient human parasites. Parasitology 145, 656–664. doi: 10.1017/S003118201700141X
Dhal, A. K., Panda, C., Yun, S. I., and Mahapatra, R. K. (2022). An update on Cryptosporidium biology and therapeutic avenues. J. Paras. Dis. 46, 923–939. doi: 10.1007/s12639-022-01510-5
Dong, S., Yang, Y., Wang, Y., Dongjian, Y., Yang, Y., Shi, Y., et al. (2020). Prevalence of Cryptosporidium infection in the global population: a systematic review and Meta-analysis. Acta Parasitol. 65, 882–889. doi: 10.2478/s11686-020-00230-1
Doyle, S. R., Søe, M. J., Nejsum, P., Betson, M., Cooper, P. J., Peng, L., et al. (2022). Population genomics of ancient and modern Trichuris trichiura. Nat. Commun. 13:3888. doi: 10.1038/s41467-022-31487-x
Dufour, B., and Le Bailly, M. (2013). Testing new parasite egg extraction methods in paleoparasitology and an attempt at quantification. Int. J. Paleopathol. 3, 199–203. doi: 10.1016/j.ijpp.2013.03.008
Fayer, R. (2010). Taxonomy and species delimitation in Cryptosporidium. Exp. Parasitol. 124, 90–97. doi: 10.1016/j.exppara.2009.03.005
Fayer, R., Speer, C. A., and Dubey, J. P. (1990). “General biology of Cryptosporidium” in Cryptosporidiosis of man and animals (Boca Raton: CRC Press, Inc), 1–30.
Feng, Y., Ryan, U. M., and Xiao, L. (2018). Genetic diversity and population structure of Cryptosporidium. Trends Parasitol. 34, 997–1011. doi: 10.1016/j.pt.2018.07.009
Ferreira, L. F., Araújo, A., Confalonieri, U., Chame, M., and Ribeiro, B. (1992). Eimeria oocysts in deer coprolites dated from 9,000 years BP. Mem. Inst. Oswaldo Cruz 87, 105–106. doi: 10.1590/S0074-02761992000500021
Flores, J., and Okhuysen, P. C. (2009). Genetics of susceptibility to infection with enteric pathogens. Curr. Opin. Infect. Dis. 22, 471–476. doi: 10.1097/QCO.0b013e3283304eb6
Fouant, M. M., Allison, M., Gerszten, E., and Focacci, G. (1982). Parásitos intestinales entre los indígenas precolombinos. Chungara 9, 285–299.
Frías, L., Leles, D., and Araújo, A. (2013). Studies on protozoa in ancient remains - a review. Mem Inst Oswaldo Cruz 108, 1–12. doi: 10.1590/S0074-02762013000100001
Garcia-R, J. C., and Hayman, D. T. (2016). Origin of a major infectious disease in vertebrates: the timing of Cryptosporidium evolution and its hosts. Parasitology 143, 1683–1690. doi: 10.1017/S0031182016001323
GBD 2016 Diarrhoeal Disease Collaborators (2018). Estimates of the global, regional, and national morbidity, mortality, and aetiologies of diarrhoea in 195 countries: a systematic analysis for the global burden of disease study 2016. Lancet Infect. Dis. 18, 1211–1228. doi: 10.1016/S1473-3099(18)30362-1
Gelabert, P., Sandoval-Velasco, M., Olalde, I., Fregel, R., Rieux, A., Escosa, R., et al. (2016). Mitochondrial DNA from the eradicated European plasmodium vivax and P. falciparum from 70-year-old slides from the Ebro Delta in Spain. Proc. Natl. Acad. Sci. U. S. A. 113, 11495–11500. doi: 10.1073/pnas.1611017113
Gilchrist, C. A., Cotton, J. A., Burkey, C., Arju, T., Gilmartin, A., Lin, Y., et al. (2018). Genetic diversity of Cryptosporidium hominis in a Bangladeshi community as revealed by whole-genome sequencing. J. Infect. Dis. 218, 259–264. doi: 10.1093/infdis/jiy121
Gonçalves, M. L., da Silva, V. L., de Andrade, C. M., Reinhard, K., da Rocha, G. C., Le Bailly, M., et al. (2004). Amoebiasis distribution in the past: first steps using an immunoassay technique. Trans. R. Soc. Trop. Med. Hyg. 98, 88–91. doi: 10.1016/s0035-9203(03)00011-7
Graff, A., Bennion-Pedley, E., Jones, A. K., Ledger, M. L., Deforce, K., Degraeve, A., et al. (2020). A comparative study of parasites in three latrines from medieval and renaissance Brussels, Belgium (14th-17th centuries). Parasitology 147, 1443–1451. doi: 10.1017/S0031182020001298
Hagan, R. W., Hofman, C. A., Hübner, A., Reinhard, K., Schnorr, S., Lewis, C. M. Jr., et al. (2020). Comparison of extraction methods for recovering ancient microbial DNA from paleofeces. Am. J. Phys. Anthropol. 171, 275–284. doi: 10.1002/ajpa.23978
Helmy, Y. A., and Hafez, H. M. (2022). Cryptosporidiosis: from prevention to treatment, a narrative review. Microorganisms 10:2456. doi: 10.3390/microorganisms10122456
Hijjawi, N., Estcourt, A., Yang, R., Monis, P., and Ryan, U. (2010). Complete development and multiplication of Cryptosporidium hominis in cell free culture. Vet. Parasitol. 169, 29–36. doi: 10.1016/j.vetpar.2009.12.021
Jacobson, D. K., Honap, T. P., Monroe, C., Lund, J., Houk, B. A., Novotny, A. C., et al. (2020). Functional diversity of microbial ecologies estimated from ancient human coprolites and dental calculus. Philos. Trans. R. Soc. Lond. Ser. B Biol. Sci. 375:20190586. doi: 10.1098/rstb.2019.0586
Khan, S. M., and Witola, W. H. (2023). Past, current, and potential treatments for cryptosporidiosis in humans and farm animals: a comprehensive review. Front. Cell. Infect. Microbiol. 13:1115522. doi: 10.3389/fcimb.2023.1115522
Koh, W., Thompson, R. C., Edwards, H., Monis, P., and Clode, P. L. (2014). Extracellular excystation and development of Cryptopsoridium: tracing the fate of oocysts within Pseudomonas aquatic biofilm systems. BMC Microbiol. 14:281. doi: 10.1186/s12866-014-0281-8
Kotloff, K. L., Nataro, J. P., Blackwelder, W. C., Nasrin, D., Farag, T. H., Panchalingam, S., et al. (2013). Burden and aetiology of diarrhoeal disease in infants and young children in developing countries (the global enteric multicenter study, GEMS): a prospective, case-control study. Lancet 382, 209–222. doi: 10.1016/S0140-6736(13)60844-2
Le Bailly, M. (2005). Evolution de la relation hôte/parasite dans le système lacustre nord alpins au Néolithique (3900–2900 BC), et nouvelles données dans la détection des paléoantigènes de Protozoa. PhD thesis, Université de Reims Champagne-Ardenne.
Le Bailly, M., Maicher, C., and Dufour, B. (2016). Archaeological occurrences and historical review of the human amoeba, Entamoeba histolytica, over the past 6000 years. Infect. Genet. Evol. 42, 34–40. doi: 10.1016/j.meegid.2016.04.030
Le Bailly, M., Maicher, C., Roche, K., and Dufour, B. (2021). Accessing ancient population lifeways through the study of gastrointestinal parasites: paleoparasitology. Appl. Sci. 11:4868. doi: 10.3390/app11114868
Ledger, M. L., Micarelli, I., Ward, D., Prowse, T. L., Carroll, M., Killgrove, K., et al. (2021). Gastrointestinal infection in Italy during the Roman Imperial and Longobard periods: a paleoparasitological analysis of sediment from skeletal remains and sewer drains. Int. Jo. Paleopathol. 33, 61–71. doi: 10.1016/j.ijpp.2021.03.001
Leles, D., Frías, L., Araújo, A., Brener, B., Sudré, A., Chame, M., et al. (2019). Are immunoenzymatic tests for intestinal protozoans reliable when used on archaeological material? Exp. Parasitol. 205:107739. doi: 10.1016/j.exppara.2019.107739
Madden, M., Salo, W. L., Streitz, J., Aufderheide, A. C., Fornaciari, G., Jaramillo, C., et al. (2001). Hybridization screening of very short PCR products for paleoepidemiological studies of Chagas' disease. BioTechniques 30, 102–109. doi: 10.2144/01301st07
Marciniak, S., Prowse, T. L., Herring, D. A., Klunk, J., Kuch, M., Duggan, A. T., et al. (2016). Plasmodium falciparum malaria in 1st-2nd century CE southern Italy. Curr. Biol. 26, R1220–R1222. doi: 10.1016/j.cub.2016.10.016
McDonald, A. C., Mac Kenzie, W. R., Addis, D. G., Gradus, M. S., Linke, G., Zembrowski, E., et al. (2001). Cryptosporidium parvum-specific antibody responses among children residing in Milwaukee during the 1993 waterborne outbreak. J. Infect. Dis. 183, 1373–1379. doi: 10.1086/319862
McLauchlin, J., Amar, C., Pedraza-Díaz, S., and Nichols, G. L. (2000). Molecular epidemiological analysis of Cryptosporidium spp. in the United Kingdom: results of genotyping Cryptosporidium spp. in 1,705 fecal samples from humans and 105 fecal samples from livestock animals. J. Clin. Microbiol. 38, 3984–3990. doi: 10.1128/JCM.38.11.3984-3990.2000
Mitchell, P. D., Stern, E., and Tepper, Y. (2008). Dysentery in the crusader kingdom of Jerusalem: an ELISA analysis of two medieval latrines in the City of acre (Israel). J. Archaeol. Sci. 35, 1849–1853. doi: 10.1016/j.jas.2007.11.017
Mitchell, P. D., Wang, T., Billig, Y., Gadot, Y., Warnock, P., and Langgut, D. (2023). Giardia duodenalis and dysentery in Iron age Jerusalem (7th-6th century BCE). Parasitology 150, 693–699. doi: 10.1017/S0031182023000410
Morris, A., Robinson, G., Swain, M. T., and Chalmers, R. M. (2019). Direct sequencing of Cryptosporidium in stool samples for public health. Front. Public Health 7:360. doi: 10.3389/fpubh.2019.00360
Morrison, D. A. (2009). Evolution of the Apicomplexa: where are we now? Trends Parasitol. 25, 375–382. doi: 10.1016/j.pt.2009.05.010
Morrow, J. J., and Reinhard, K. J. (2016). Cryptosporidium parvum among coprolites from La Cueva de los Muertos Chiquitos (600-800 CE), Rio Zape Valley, Durango, Mexico. J. Parasitol. 102, 429–435. doi: 10.1645/15-916
Nader, J. L., Mathers, T. C., Ward, B. J., Pachebat, J. A., Swain, M. T., Robinson, G., et al. (2019). Evolutionary genomics of anthroponosis in Cryptosporidium. Nat. Microbiol. 4, 826–836. doi: 10.1038/s41564-019-0377-x
Ortega, Y. R., and Bonavia, D. (2003). Cryptosporidium, Giardia, and Cyclospora in ancient Peruvians. J. Parasitol. 89, 635–636. doi: 10.1645/GE-3083RN
Poinar, G. J. (2005). Plasmodium dominicana n. sp. (Plasmodiidae: Haemospororida) from Tertiary Dominican amber. Syst. Parasitol. 61, 47–52. doi: 10.1007/s11230-004-6354-6
Poinar, G. J., and Poinar, R. (2004). Paleoleishmania proterus n. gen., n. sp., (Trypanosomatidae: Kinetoplastida) from cretaceous Burmese amber. Protist 155, 305–310. doi: 10.1078/1434461041844259
Reinhard, K., Camacho, M., Geyer, B., Hayek, S., Horn, C., Otterson, K., et al. (2019). Imaging coprolite taphonomy and preservation. Archaeol. Anthropol. Sci. 11, 6017–6035. doi: 10.1007/s12520-019-00946-w
Robinson, G., Elwin, K., and Chalmers, R. M. (2008). Unusual Cryptosporidium genotypes in human cases of diarrhea. Emerg. Infect. Dis. 14, 1800–1802. doi: 10.3201/eid1411.080239
Rollins, A., Krupa, K., Millward, G., Piombino-Mascali, D., Reinhard, K., and Kaestle, F. (2021). Molecular identification of parasites in an intestinal coprolite from a mummified religious dignitary of the Piraino mother church crypt, Sicily. J. Archaeol. Sci. 38:103022. doi: 10.1016/j.jasrep.2021.103022
Ruffer, M. A. (1910). Note on the presence of bilharzia haematobia in Egyptian mummies of twentieth dynasty (1250-1000 BC). Br. Med. J. 1:16. doi: 10.1136/bmj.1.2557.16-a
Shillito, L.-M., Blong, J. C., Green, E. J., and van Asperen, E. N. (2020). The what, how and why of archaeological coprolite analysis. Earth Sci. Rev. 207:103196. doi: 10.1016/j.earscirev.2020.103196
Šlapeta, J. (2017). DNA barcoding of Cryptosporidium. Parasitology 145, 574–584. doi: 10.1017/S0031182017001809
Sponseller, J. K., Griffiths, J., and Tzipori, S. (2014). The evolution of respiratory cryptosporidiosis: evidence for transmission by inhalation. Clin. Microbiol. Rev. 27, 575–586. doi: 10.1128/CMR.00115-13
Spyrou, M. A., Tukhbatova, R. I., Wang, C. C., Valtueña, A. A., Lankapalli, A. K., Kondrashin, V. V., et al. (2018). Analysis of 3800-year-old Yersinia pestis genomes suggests bronze age origin for bubonic plague. Nat. Commun. 9:2234. doi: 10.1038/s41467-018-04550-9
Thompson, R. C. A., Koh, W. H., and Clode, P. L. (2016). Cryptosporidium—what is it? Food Waterborne Parasitol. 4, 54–61. doi: 10.1016/j.fawpar.2016.08.004
Tichkule, S., Cacciò, S. M., Robinson, G., Chalmers, R. M., Mueller, I., Emery-Corbin, S. J., et al. (2022). Global population genomics of two subspecies of Cryptosporidium hominis during 500 years of evolution. Mol. Biol. Evol. 39:msac056. doi: 10.1093/molbev/msac056
Tichkule, S., Jex, A. R., van Oosterhout, C., Sannella, A. R., Krumkamp, R., Aldrich, C., et al. (2021). Comparative genomics revealed adaptive admixture in Cryptosporidium hominis in Africa. Microb. Genom. 7:mgen000493. doi: 10.1099/mgen.0.000493
Tyzzer, E. E. (1907). A sporozoan found in the peptic glands of the common mouse. Proc. Soc. Exp. Biol. Med. 5, 12–13. doi: 10.3181/00379727-5-5
Tyzzer, E. E. (1910). An extracellular coccidium, Cryptosporidium muris (gen. Et sp. nov.), of the gastric glands of the common mouse. J. Med. Res. 23, 487–510.3.
Tyzzer, E. E. (1912). Cryptosporidium parvum (sp. nov.), a coccidium found in the small intestine of the common mouse. Arch. Protistenkd. 26:394.
Votýpka, J., Modrý, D., Oborník, M., Šlapeta, J., and Lukeš, J. (2017). “Apicomplexa” in Handbook of the protists. eds. J. M. Archibald, A. G. B. Simpson, and C. H. Slamovits (Switzerland: Springer International Publishing), 567–624.
White, A. J. J. (2010). Cryptosporidiosis and the ears of the Hippopotamus. Clin. Infect. Dis. 50, 1373–1374. doi: 10.1086/652141
Wiscovitch-Russo, R., Rivera-Perez, J., Narganes-Storde, Y. M., García-Roldán, E., Bunkley-Williams, L., Cano, R., et al. (2020). Pre-Columbian zoonotic enteric parasites: an insight into Puerto Rican indigenous culture diets and life styles. PLoS One 15:e0227810. doi: 10.1371/journal.pone.0227810
Wood, J. R. (2018). DNA barcoding of ancient parasites. Parasitology 145, 646–655. doi: 10.1017/S0031182018000380
Wood, J. R., Wilmshurst, J. M., Rawlence, N. J., Bonner, K. I., Worthy, T. H., Kinsella, J. M., et al. (2013). A megafauna's microfauna: gastrointestinal parasites of New Zealand's extinct moa (Aves: Dinornithiformes). PLoS One 8:e57315. doi: 10.1371/journal.pone.0057315
Xiao, L. (2010). Molecular epidemiology of cryptosporidiosis: an update. Exp. Parasitol. 124, 80–89. doi: 10.1016/j.exppara.2009.03.018
Yeh, H. Y., Prag, K., Clamer, C., Humbert, J. B., and Mitchell, P. D. (2015). Human intestinal parasites from a Mamluk period cesspool in the Christian quarter of Jerusalem: potential indicators of long distance travel in the 15th century AD. Int. J. Paleopathol. 9, 69–75. doi: 10.1016/j.ijpp.2015.02.003
Keywords: paleoparasitology, Cryptosporidium parvum , Cryptosporidium hominis , ELISA, ANCIENT DNA, sediment sample, coprolite, paleomicrobiology
Citation: Roche K, Dalle F, Capelli N, Borne R, Jouffroy-Bapicot I, Valot B, Grenouillet F and Le Bailly M (2023) From modern-day parasitology to paleoparasitology: the elusive past record and evolution of Cryptosporidium. Front. Microbiol. 14:1249884. doi: 10.3389/fmicb.2023.1249884
Received: 29 June 2023; Accepted: 05 October 2023;
Published: 19 October 2023.
Edited by:
Sara C. Zapico, New Jersey Institute of Technology, United StatesReviewed by:
Francisco Medina Paz, New Jersey Institute of Technology, United StatesCopyright © 2023 Roche, Dalle, Capelli, Borne, Jouffroy-Bapicot, Valot, Grenouillet and Le Bailly. This is an open-access article distributed under the terms of the Creative Commons Attribution License (CC BY). The use, distribution or reproduction in other forums is permitted, provided the original author(s) and the copyright owner(s) are credited and that the original publication in this journal is cited, in accordance with accepted academic practice. No use, distribution or reproduction is permitted which does not comply with these terms.
*Correspondence: Kévin Roche, a2V2aW4ucm9jaGVAdW5pdi1mY29tdGUuZnI=
Disclaimer: All claims expressed in this article are solely those of the authors and do not necessarily represent those of their affiliated organizations, or those of the publisher, the editors and the reviewers. Any product that may be evaluated in this article or claim that may be made by its manufacturer is not guaranteed or endorsed by the publisher.
Research integrity at Frontiers
Learn more about the work of our research integrity team to safeguard the quality of each article we publish.