- 1Department of Food Safety and Animal Health Research, Norwegian Veterinary Institute, Ås Municipality, Norway
- 2Department of Microbiology, Norwegian Veterinary Institute, Ås Municipality, Norway
- 3Department of Bacteriology, Norwegian Veterinary Institute, Ås Municipality, Norway
The evolution of antimicrobial resistance (AMR) has mainly been studied in planktonic bacteria exposed to sub-inhibitory antimicrobial (AM) concentrations. However, in a number of infections that are treated with AMs the bacteria are located in biofilms where they tolerate high doses of AM. In the present study, we continuously exposed biofilm residing E. coli at body temperature to high ciprofloxacin (CIP) concentrations increasing from 4 to 130 times the minimal inhibitory concentration (MIC), i.e., from 0.06 to 2.0 mg/L. After 1 week, the biofilms were full of CIP resistant bacteria. The evolutionary trajectory observed was the same as described in the literature for planktonic bacteria, i.e., starting with a single mutation in the target gene gyrA followed by mutations in parC, gyrB, and parE, as well as in genes for regulation of multidrug efflux pump systems and outer membrane porins. Strains with higher numbers of these mutations also displayed higher MIC values. Furthermore, the evolution of CIP resistance was more rapid, and resulted in strains with higher MIC values, when the bacteria were biofilm residing than when they were in a planktonic suspension. These results may indicate that extensive clinical AM treatment of biofilm-residing bacteria may not only fail to eradicate the infection but also pose an increased risk of AMR development.
Introduction
Antimicrobial resistance (AMR) is a threat to global human and animal health, food safety and security, and development, and therefore one of the most important challenges facing society today (World Health Organization [WHO], 2020). Acquired AMR in bacteria is a property that is evolved through mutations in chromosomal genes, or by gain of resistance gene(s). A common approach for studying evolution of AMR is to use adaptive evolution to identify resistance-conferring mutations. Mutation rate is a key determinant of evolution. Variability in this rate has been shown in different scenarios to play a key role in evolutionary adaptation, and resistance evolution under stress caused by selective pressure from antimicrobials (AMs) (Engelhardt and Shakhnovich, 2019). Numerous AMR evolution studies have been performed on planktonic bacteria (Nichol et al., 2019; Rosenkilde et al., 2019). In contrast, and despite the predominance of biofilm growth in nature, very few evolution experiments have been performed on biofilm populations (Ahmed et al., 2018; France et al., 2019; Santos-Lopez et al., 2019; Trampari et al., 2021; Usui et al., 2023). Bacterial biofilms consist of bacteria attached to a surface and/or each other and embedded in a self-produced matrix, and have been shown to be present and part of the pathogenesis in bacterial infections in most body system of humans as well as animals (Vestby et al., 2020; Nesse et al., 2023). Bacteria in biofilms show a temporary phenotypic tolerance to antimicrobials (Olsen, 2015; Bowler, 2018). This tolerance is multi-factorial, and it is attributed to restricted penetration of the antibiotics, restricted growth at low-oxygen tension, expression of biofilm-specific genes and the presence of persister cells (Ciofu et al., 2017). Consequently, bacteria in biofilms tolerate higher doses of AMs than planktonic bacteria.
Ciprofloxacin (CIP) is a fluoroquinolone, i.e., a group of AMs considered to be critically important for human medicine where they are used for treatment of a variety of infections (World Health Organization [WHO], 2017; El-Attar et al., 2022). Fluoroquinolones cause bactericidal DNA damage by forming a topoisomerase-quinolone-DNA ternary complex, which leads to double-stranded breaks in DNA and blocking of DNA replication (Dalhoff, 2012). The DNA damage triggers the SOS-response, which induces DNA repair as well as mutagenesis (McKenzie et al., 2000). Development of bacterial resistance against CIP is a growing concern. As many of the diseases that are treated with CIP may be biofilm associated, the role of biofilm in the evolution of CIP resistance is of special interest. Mutations in the gyrase and topoisomerase genes are generally the primary mechanisms of such resistance (Hamed et al., 2018). However, the few studies that have addressed this question, have observed none or few of these target specific mechanisms in biofilm evolved resistance (Ahmed et al., 2018; France et al., 2019; Santos-Lopez et al., 2019; Trampari et al., 2021).
In the present study, we chose to expose biofilm residing E. coli to lethal concentrations of CIP continuously for 2 weeks, as in a clinical treatment plan. The hypothesis was that treatment of biofilms with CIP concentrations above their MIC value might not only fail to eradicate the bacteria, but also stimulate them to evolve a high-level resistance against CIP. We also wanted to see if the evolutionary trajection of resistance under such conditions differed from what would be observed in planktonic cultures. To investigate this, we used the experimental design described in Figure 1.
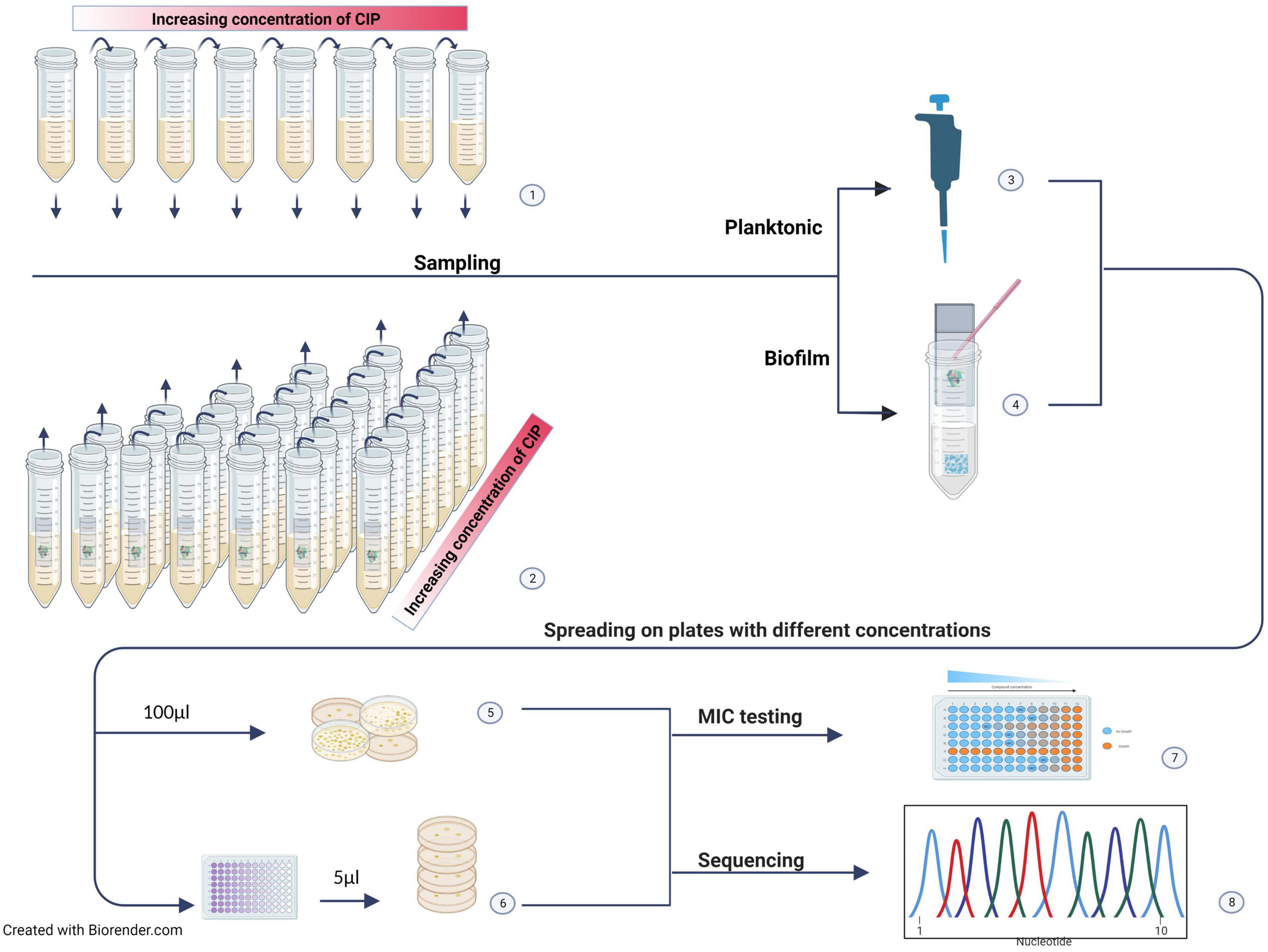
Figure 1. Experimental design. (1) The planktonic suspension was incubated in increasing CIP concentrations starting with 0.5 × MIC. Samples were taken after each incubation period (2–3 days). (2) Seven parallel biofilms on glass slides were separately incubated in increasing CIP concentrations starting with 4 × MIC. One biofilm was sampled after each incubation period. (3) The planktonic sample was used directly for further investigations. (4) The biofilm was scraped off the glass slide, disrupted, and the cells suspended in sterile saline. (5) For enumeration, the suspensions were serially diluted, and (6) spread on LB agar plates without CIP and selective agar plates with different CIP concentrations. Selected colonies were subjected to MIC testing (7) and whole genome sequencing (WGS) (8).
Materials and methods
Bacterial strain and media
The E. coli strain used (2014-01-6355) was originally collected from broiler chicken cecum as part of the Norwegian monitoring program on AMR in the veterinary sector (NORM-VET) (Simonsen and Urdahl, 2014). The isolate was susceptible to CIP with a minimum inhibitory concentration (MIC) ≤0.015 mg/L. Furthermore, it produced biofilm with both curli fimbriae and cellulose in the matrix, i.e., the RDAR morphotype in the Congo red agar assay (Nesse et al., 2021). The strain was stored at −80°C in brain heart infusion broth (BHI; Difco, BD, Franklin Lakes, NJ, USA) supplemented with 15% glycerol (Merck KGaA, Darmstadt, Germany) and recovered on blood agar (cattle blood) at 37.0 ± 1.0°C overnight. Lysogeny broth (LB; Merck) was used for overnight culture and in all experiments on planktonic growth. LB without NaCl (LBwo/NaCl; Bacto-tryptone 10 g/liter, yeast extract 5 g/liter) was used for the biofilm experiments. The antimicrobial CIP (Ciprofloxacin hydrochloride; Bayer; Germany) was added to the media to the desired concentrations.
Experimental design for the evolution of antimicrobial resistance
An overview of the experimental design is given in Figure 1. Evolution was studied under increasing concentrations of CIP at 37.0 ± 1.0°C. Based on the results from preliminary studies, the experiment was performed twice. In each experiment, a start suspension was made by mixing blood agar colonies in LBwo/NaCl to a concentration corresponding to the 1.0 ± 0.1 McFarland standard. The same start suspension was used to produce both biofilms and planktonic test suspensions.
Evolution in biofilm residing bacteria
Parallel biofilms were produced on 16 glass slides by adding 500 μL start suspension to each of 16 centrifuge tubes (50 mL, Sarstedt AG & Co KG, Nürnbrecht, Germany) containing 10 mL LBwo/NaCl and an autoclaved microscope slide (76 by 26 mm; Menzel GmbH + CoKG, Braunschweig, Germany). The tubes were incubated at 37.0 ± 1.0°C for 48.
After incubation, biofilms on two randomly selected slides were harvested (sampling 0), serially diluted, and spread on LB agar plates without CP for enumeration of the total number of colony forming units (cfu), and on selective LB agar plates with 0.25, 0.5 or 1 mg/L CIP for enumeration of CIP resistant cfu (For details, see section “Sampling”).
The rest of the glass slides were randomly allocated to receive CIP (BIO CIP) or control (BIO CTR) treatment, and accordingly each placed in a separate test tube with the designated medium for incubation, i.e., 10 ml LBwo/NaCl with 0.06 mg/L CIP or without CIP, respectively. The initial concentration of CIP was four times the strain’s MIC value. The tubes were incubated in 37.0 ± 1.0°C.
Every second day (third day at weekends), the biofilm of one random slide from each treatment group was harvested and investigated as described for sampling 0. The rest of the slides were moved to tubes with fresh medium with or without CIP, according to their allocated treatment. The CIP concentration was doubled every time the slides were moved (Table 1).
Selected colonies from relevant samplings were subjected to MIC testing and whole genome sequencing (WGS).
Evolution in planktonic bacteria
First, 500 μL of the start suspension were added to a 50 mL centrifuge tube containing 10 mL LB, and incubated at 37.0 ± 1.0°C for 48 h.
After incubation, a sample of the suspension (sampling 0), was serially diluted and spread on both LB agar plates without CIP for enumeration of the total number of colony forming units (cfu), and on selective LB agar plates with 0.25, 0.5, or 1 mg/L CIP for enumeration of CIP resistant cfu (For details, see section “Sampling”).
Thereafter, 500 μL of the planktonic suspension were placed in each of two tubes with 10 mL fresh LB, one with CIP 0.0075 mg/L (PLANK CIP) and the other without CIP (PLANK CTR). The initial concentration of CIP was half the strain’s MIC value. The tubes were incubated in 37.0 ± 1.0°C.
Every second day (third day at weekends), one sample from each incubated tube was investigated as described for sampling 0. Furthermore, 500 μL of the planktonic suspension from the PLANK CIP tube were moved to a new tube with 10 mL fresh LB with CIP. The CIP concentration was doubled every time the slides were moved (Table 1). Likewise, 500 μL of the planktonic suspension from the PLANK CTR tube were moved to a new tube with 10 mL fresh LB without CIP.
Selected colonies from relevant samplings were subjected to MIC testing and WGS.
Sampling
During incubation, biofilm was formed on both sides of the microscope slides at the liquid-air interface. When a biofilm was harvested, the glass slide was washed three times in sterile saline to remove loosely adhered bacteria. Thereafter, the biofilm was removed by scraping with a sterile cell scraper (BD Falcon, Bedford, MA, USA) into a sterile reagent tube containing 5 mL sterile saline and 20–30 glass beads (3 mm; Assistent, Glaswarenfabrik Karl Hecht GmbH & Co KG, Bavaria, Germany). The tube was vortexed at 2000 rpm for 1 min before the suspension was used for enumeration of total cfu by serially diluting 200 μL suspension in sterile saline and placing 5 μL dilution on agar plates. In addition, 100 μL undiluted suspension were spread directly on agar plates. Same procedure was used for enumerating total cfu in the planktonic samples, using the test suspensions of these tubes without further preparation. LB agar plates without CIP were used for enumeration of total cfu, whereas LB agar plates with three different concentrations of CIP, namely 0.25 mg/L (CIP low), 0.5 mg/L (CIP medium), and 1.0 mg/L (CIP high), were used to calculate the number of CIP resistant bacteria. The results were expressed as log10 cfu in the biofilm or in the 10 mL planktonic test suspension. The limit of detection was 1.7 log10 cfu.
Selection of colonies for further testing
One colony from each of the samplings 0, 1, 2, 4, 6, and 7 was randomly picked from the LB agar plates without CIP used for the enumeration of BIO CTR (n = 6) and PLANK CTR (n = 6) in the experiment 2. Colonies from BIO CIP (n = 8) and PLANK CIP (n = 16) were selected from agar plates with different CIP concentrations at relevant samplings at both experiments. All selected colonies (n = 37) were subjected to MIC testing and whole genome sequencing together with the wildtype strain.
Antimicrobial susceptibility testing (MIC testing)
Minimal inhibitory concentrations were determined by broth microdilution with Sensititre™ gram-negative antimicrobial susceptibility plates (EUVSEC, Sensititre, TREK Diagnostic LTD, Thermo Scientific USA), according to the manufacturer’s instructions. The CIP MIC values were classified according to the clinical breakpoints given in European Committee on Antimicrobial Susceptibility Testing Version 11.0, 20211 as resistant: MIC > 0.5 mg/L, intermediate: MIC = 0.5 mg/L, or susceptible: MIC ≤ 0.25 mg/L.
DNA extraction and whole genome sequencing (WGS)
DNA from each isolate was extracted using the QIAamp® DNA Mini Kit (Qiagen) according to the manufacturer’s protocol, with some minor modifications. The optional RNase A step was included, and 100 μL 10 mM Tris, pH 8, was used as elution buffer. The DNA extracts purity was determined using MySpec (VWR®) and DNA concentrations using Tecan Spark Fluorometer (Tecan Trading AG, Switzerland) with the Qubit broad range kit. The DNA was prepared using the Nextera™ DNA Flex library preparation kit (Illumina) and sequenced on Illumina MiSeq resulting in 300 bp paired-end reads.
Bioinformatic analyses
All reads were quality checked using fastQC2 and multiQC (Ewels et al., 2016). The reads from the wildtype isolate were trimmed using Trimgalore 20193 and assembled using Unicycler (Wick et al., 2017) in normal mode. Thereafter, Prokka (Seemann, 2014) was used to annotate the genome. In order to determine changes in the genome (i.e., SNPs and indels) of isolates grown in biofilm and planktonic suspension with and without CIP, we used Snippy4 with the annotated wildtype genome as reference.
Statistical analysis
A two-tailed Student’s t-test was used for comparisons among populations. The differences were considered statistically significant when the p-value was ≤ 0.05.
Results
Total and resistant log10 cfu during evolution in biofilm
Throughout the experiments, the total log10 cfu in the biofilms of BIO CIP was lower than that of BIO CTR (mean difference ± SD = 1.44 ± 0.65, p = 0.01). The BIO CIP treated bacteria displayed the same resistance evolution pattern in both experiments (Figure 2). Bacteria growing on CIP low and CIP medium agar plates were first observed at sampling 2, and the mean log10 cfu on these plates were not statistically different from the total mean log10 cfu in the biofilm throughout the rest of the experiments (p = 0.78 and 0.61, respectively). At the same sampling, low numbers of cfu growing on CIP high agar plates were also observed. Unfortunately, results from sampling 3 were missing from both experiments. Consequently, it is not known whether these numbers reached the level of the total mean log10 cfu at sampling 3 or at sampling 4 where this was first observed.
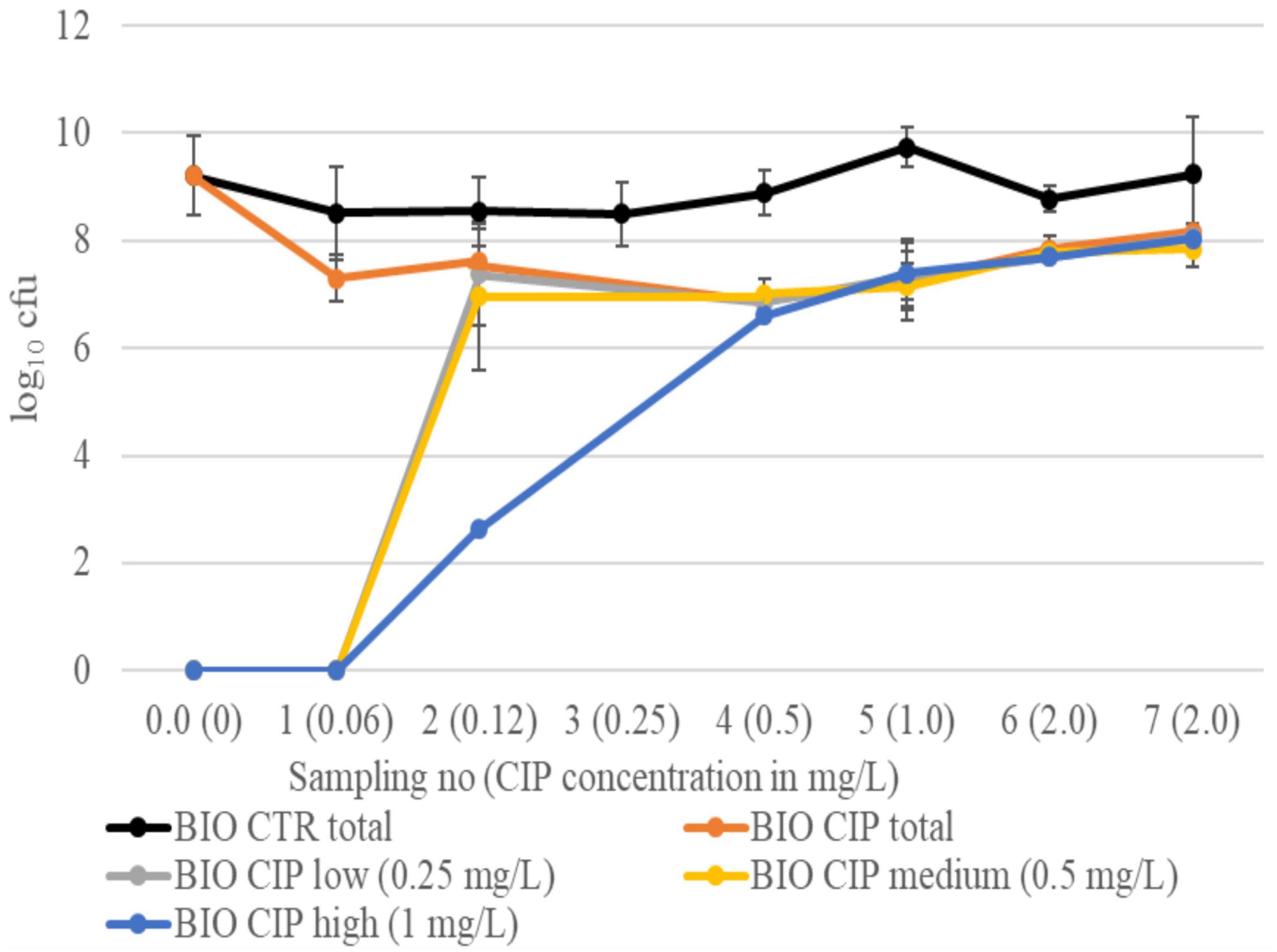
Figure 2. Total log10 cfu in BIO CIP and BIO CTR, as well as log10 cfu growing on selective plates with low (0.25 mg/L), medium (0.5 mg/L), and high (1.0 mg/L) CIP concentrations, at different sampling points. Results are given as means of experiments 1 and 2. Bars represent standard deviations. Results from sampling 3 in BIO CIP are missing in both experiments.
Total and resistant log10 cfu during evolution in planktonic suspension
Interestingly, the pattern of resistance development in CIP-exposed planktonic bacteria differed in the two experiments. In experiment 1, a sharp drop in total log10 cfu of PLANK CIP was observed at sampling 3, and bacteria growing on CIP agar plates were not observed until sampling 4 (CIP low and CIP medium) and sampling 5 (CIP high) (Figure 3). Although the total log10 cfu of PLANK CIP increased at sampling 4, it remained lower than the total log10 cfu of PLANK CTR for the rest of the experiment (p = 0.006). In experiment 2, bacteria growing on CIP low and CIP medium agar plates were observed already at sampling 1, whereas bacteria growing on CIP high plates were not seen before sampling 6 (Figure 4). Also in this experiment, the total log10 cfu of PLANK CIP total was lower than PLANK CTR in the last three samplings (p = 0.05).
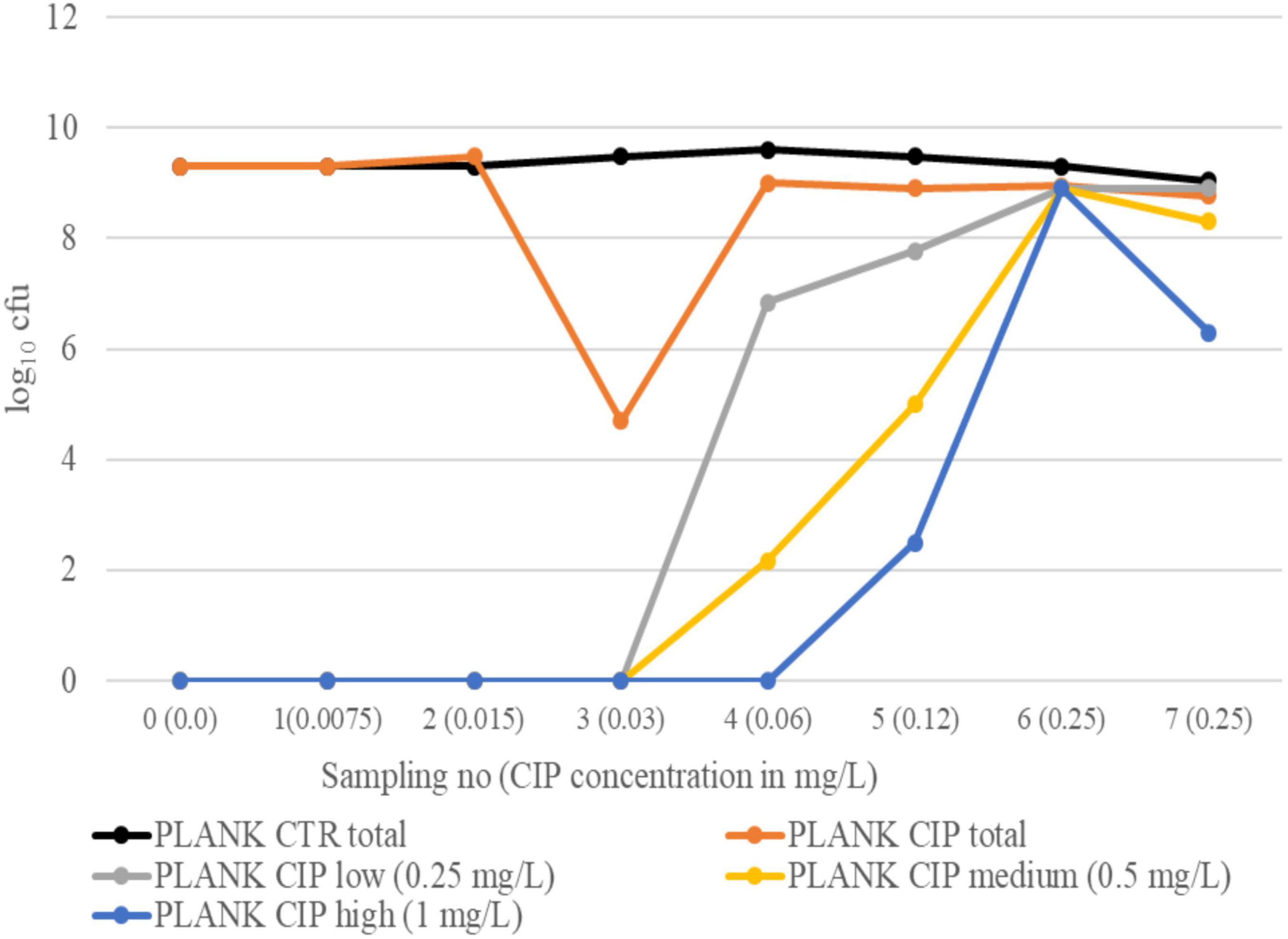
Figure 3. Experiment 1. Total log10 cfu in PLANK CIP and PLANK CTR, as well as log10 cfu growing on selective plates with low (0.25 mg/L), medium (0.5 mg/L), and high (1.0 mg/L) CIP concentrations, at different sampling points.
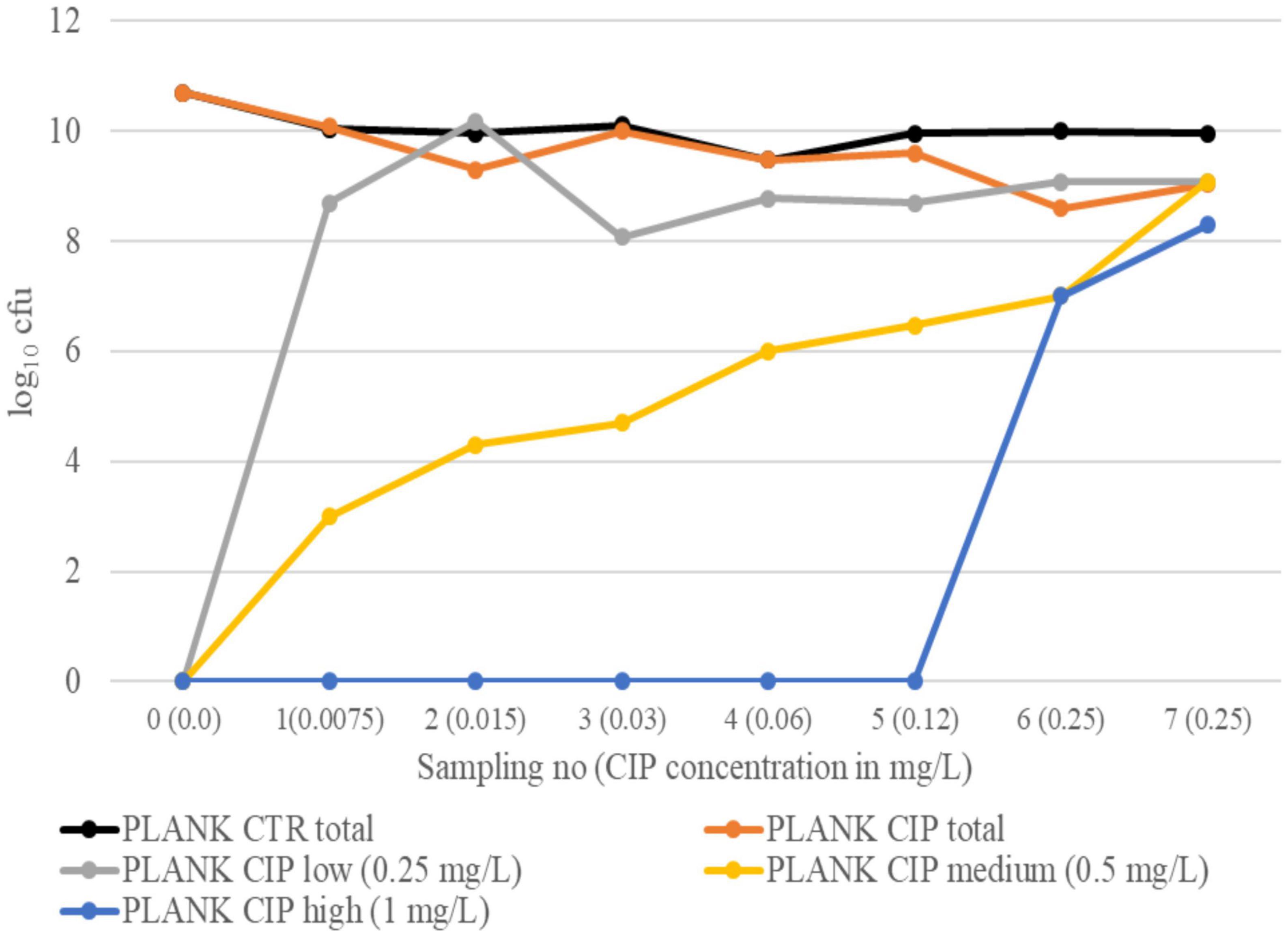
Figure 4. Experiment 2. Total log10 cfu in PLANK CIP and PLANK CTR, as well as log10 cfu growing on selective plates with low (0.25 mg/L), medium (0.5 mg/L), and high (1.0 mg/L) CIP concentrations, at different sampling points.
MIC testing
The wildtype strain had a CIP MIC value of ≤0.015 mg/L. All isolates from the controls displayed CIP MIC values in the range of ≤0.015–0.03 mg/L, i.e., the same as the wildtype strain within the accepted error range of one dilution step (European Food Safety Authority [EFSA], Aerts et al., 2019; Supplementary Tables 1, 2). The BIO CIP isolates had CIP MIC values ranging from 1 to >8 mg/L (Table 2), whereas the PLANK CIP isolates displayed values from 0.5 to 2 mg/L (Tables 3, 4). In addition, a number of the BIO CIP and PLANK CIP isolates, but none of the control isolates, had increased MIC values for chloramphenicol (CHL) and tetracycline (TET).
Genetic studies
The BIO CIP isolates from the two experiments displayed from 4 to 10 mutations as compared to the wildtype strain (Table 2), whereas 1 to 5 mutations was seen in the BIO CTR isolates (Supplementary Table 1). Mutations in the QRDR of gyrA were observed in all BIO CIP isolates. Several isolates also had mutations in the relevant regions of gyrB, parC, and parE, as well as in regulator genes of multidrug efflux systems and outer membrane porin F. The isolates with the highest CIP MIC values had the highest number of mutations in these groups of genes. Other mutations appeared randomly distributed. Mutations in the BIO CTR isolates also seemed randomly distributed except for mutations at different positions in the fimH2 gene of four isolates, and identical mutations in the pgm gene of three isolates. The pgm mutation was also observed in one BIO CIP isolate, whereas none of the BIO CIP isolates carried any of the fimH2 mutations.
The two planktonic experiments, on the other hand, displayed different mutation patterns in their PLANK CIP isolates. In experiment 1 (Table 3), four to ten mutations per isolate were observed. All isolates had mutations in gyrB, as well as in regulator genes of multidrug efflux systems. In the isolates from the two last samplings, mutations in the QRDR of gyrA were also present. In addition, four of the five isolates investigated displayed an identical frameshift variant in the gene rfbC, whereas the fifth isolate had a conservative inframe insertion in rfbD. In contrast, all isolates in experiment 2 (Table 4) had an identical mutation in the QRDR of gyrA. Half of the isolates also displayed a mutation in either marR or soxR. The total number of mutations per isolate in experiment 2, was one to seven. A total of zero to six mutations per isolate was observed in the PLANK CTR isolates (Supplementary Table 2). Three isolates displayed identical mutations in the genes fimH2, flu1, and yohF, whereas the rest of the mutations appeared randomly distributed. The fimH2 mutation was different from those observed in BIO CTR isolates.
Discussion
The present study demonstrated rapid evolution of CIP resistance in biofilm-residing E. coli subjected to prolonged treatment with high, increasing concentrations of CIP, where the initial concentration corresponded to 4 × MIC of the wildtype strain. Already after 1 week, the biofilms were dominated by CIP resistant E. coli. To the best of our knowledge, this is the first time evolution of AMR in bacteria in biofilm continuously treated with high AM concentrations has been demonstrated. Our results indicate that prolonged AM treatment may not only fail to eradicate chronic bacterial infections associated with the presence of biofilms, but also result in development of high level AMR.
Strikingly, the evolutionary trajectory of AMR in the biofilms was similar in both our experiments, even though six separately developed biofilms were studied in each experiment. All CIP resistant isolates investigated displayed mutations in the QRDR of gyrA, and 62.5% had additional mutations in gyrB and/or parC/parE. Furthermore, 62.5% of the isolates had mutations in genes associated to multidrug efflux pumps and outer membrane porin regulation. This probably also contributed to the increased MIC values for CHL and TET, in addition to CIP. Higher numbers of CIP resistance-conferring mutations were correlated with higher CIP MIC values. On selective plates, colonies were first observed on those with the low and medium CIP concentrations, followed by increasing cfu numbers on the plates with high concentration. All these observations fit a stepwise evolution model as described in literature. DNA gyrase is the primary target of fluoroquinolones in E. coli, but if gyrA is mutated, topoisomerase IV becomes a target (Redgrave et al., 2014). Accordingly, the primary event of evolving resistance in planktonic cultures is believed to be a single mutation in gyrA followed by mutations in parC and the mar operon, where each new mutation increases the level of resistance (Huseby et al., 2017). This is also what was seen in the biofilm residing bacteria in our study. The observed positions substituted in GyrA (Ser-83 or Asp-87) and ParC (Ser-80) are reported to be the most commonly found in CIP resistant E. coli (Jacoby, 2005). The position mutated in GyrB interacts with the quinolone molecule when this is bound to the QRDR of GyrA (Bhatnagar and Wong, 2019). Mutations at the same position in ParE as in our experiment have also been related to increased MIC-values (Sorlozano et al., 2007). Although the biofilm lifestyle itself has been reported to induce AMR conferring mutations (Nesse and Simm, 2018; France et al., 2019), no growth was observed on selective CIP plates in our BIO CTR experiments, nor did any of the isolates investigated have mutations related to quinolone resistance. This confirms that the evolved resistance in BIO CIP was due to the CIP exposure.
Interestingly, our results differed from earlier studies on evolution of CIP resistance in biofilm with Pseudomonas aeruginosa (Ahmed et al., 2018, 2020) and Acinetobacter baumannii (Penesyan et al., 2019; Santos-Lopez et al., 2019). In these studies, the biofilm cells only acquired mutations in regulators of efflux pumps, and not in the topoisomerase target genes. Accordingly, their CIP MIC values were lower than those of planktonic evolved cells. Likewise, a CIP resistance evolution experiment in Salmonella enterica serovar Typhimurium biofilms, displayed in a wide variety of mutations resulting in porin loss, efflux changes and in some cases target site changes, indicating multiple paths of evolution and resistance (Trampari et al., 2021). However, the experimental design in all these studies deviated from our study at two major points. Firstly, their biofilms were exposed to sub-inhibitory CIP concentrations, either throughout the experiment, or initially with subsequent increasing concentrations. Secondly, the lifespan of the exposed biofilms were short, i.e., 1 to 3 days. One study had a total exposure time of 3 days (Penesyan et al., 2019), whereas the others investigated short term biofilms repeatedly propagated from the previous biofilms. Our results show that the biofilm residing bacteria can mount a target based evolutionary trajectory and high CIP MICs, if exposed to high CIP concentrations and given sufficient time.
Ciprofloxacin is known to be able to penetrate into biofilms (Anderl et al., 2000; Araujo et al., 2014), but the active concentrations achieved in the different layers of our biofilms are unknown. However, a statistically significant decrease in the total log10 cfu was observed for BIO CIP compared to BIO CTR during both experiments. This may indicate that the high levels of CIP treatment was a stressful challenge for the bacteria, even under the protection offered by the biofilm lifestyle. CIP is known to induce an SOS-response leading to mutagenesis (Qin et al., 2015). Our study was not designed to measure mutations rates. Still, crude observations of the total number of mutations observed in selected isolates may indicate a higher number of mutations in BIO CIP than in BIO CTR.
In the BIO CTR, isolates from four out of six parallel biofilms displayed different non-synonymous mutations in fimH2, and three had identical mutations in pgm (Gly494Val). This might indicate a selective advantage in long term biofilms for these mutants, as both these genes have been reported to be involved in biofilm formation. The FimH protein, which is the receptor-recognizing part of type 1 fimbriae (Krogfelt et al., 1990), has been shown to be important for biofilm formation by E. coli K-12 under static growth conditions (Pratt and Kolter, 1998), i.e., the same conditions as in our experiments. Furthermore, studies using E. coli transposon mutants showed that pgm, encoding phosphoglucomutase, influenced motility and curli production (Hadjifrangiskou et al., 2012), both factors that contribute to biofilm formation by E. coli (Nesse et al., 2021). Although we did not observe increased numbers of cfu in our control biofilms, these mutations might still be beneficious in other ways, e.g., by contributing to better adhesion.
Our experiments with planktonic bacteria were originally included as comparison for the biofilm experiments. However, interesting differences in the evolutionary trajectory of the two planktonic experiments were also observed. In experiment 2, the evolution followed the commonly reported trajectory, i.e., early observations of reduced susceptibility, and rapid mutations in the gyrA followed by mutations in genes encoding multidrug efflux-pump systems. In experiment 1, however, no growth on the selective agar plates was observed at the first three samplings. In addition, the total log10 cfu in the biofilm fell dramatically at sampling 3 where the CIP concentration was twice the MIC value of the wildtype strain. This indicates that no or only a very low level of reduced susceptibility had developed at this stage. The total log10 cfu was almost restored at sampling 4 where it included colonies growing on low and medium selective plates. Isolates from these plates harbored the same mutation in gyrB as observed in BIO CIP isolates, in addition to various mutations in multidrug efflux pump system genes. Colonies with mutation in gyrA were not observed until the last two samplings. The gyrB mutation can augment resistance if gyrA is already mutated, but it is less effective in a bacterial cell with wild-type gyrA (Jacoby, 2005; Bhatnagar and Wong, 2019). A small reduction in susceptibility due to the gyrB mutation observed may thus have given sufficient protection under the early exposures to sub-inhibitory and low concentrations. If so, this might have reduced the competitive advantage of other mutations at these concentrations. Furthermore, all isolates investigated displayed either an identical frameshift variant in the gene rfbC (4 isolates) or a conservative inframe insertion in rfbD (1 isolate). These genes belong to the rfb cluster encoding enzymes involved in O-antigen synthesis (Coimbra et al., 2000). The gene rfbC has also been reported to be involved in the response of E. coli to lethal stress, e.g., from exposure to the quinolone nalidixic acid (Han et al., 2010). It is therefore possible the mutations in rfbC, and maybe also in rfbB, may have contributed to reduced susceptibility to CIP.
In the PLANK CTR, three isolates from different samplings displayed identical mutations in the genes fimH2, flu1, and yohF. The two first genes encode surface structures involved in adhesion and agglutination (Krogfelt et al., 1990; Hasman et al., 1999), whereas the last one is a putative oxidoreductase. In contrast to the biofilm experiments, the planktonic samples were not taken from separately evolved cultures, but from cultures that were propagated from the preceding one. Whether the presence of these mutations over time in fact reflects a selective advantage is therefore uncertain.
Resistance to CIP evolved more rapidly in biofilm-residing bacteria than in planktonic ones. Colonies growing on selective plates with high CIP concentration were first observed at sampling 2 from BIO CIP and at sampling 5 and 6 from PLANK CIP. However, as the biofilms were subjected to an initial CIP concentration eight times higher than the planktonic bacteria, the CIP concentration at sampling 2 in the biofilm experiments was the same as at sampling 5 in the planktonic. The fast development in the biofilm residing bacteria therefore appears primarily to be due to the biofilm lifestyle permitting susceptible bacteria being exposed to the higher CIP concentrations. This is probably also the reason for the biofilm residing bacteria displaying higher numbers of resistance-conferring mutations and higher MIC values than the planktonic. This may indicate that the risk of resistance development is higher during AM treatment of biofilm infections than non-biofilm infections.
Conclusion
This study displayed evolution of high level CIP resistance in biofilm-residing E. coli subjected to lethal CIP concentrations for up to 2 weeks. This is the first study showing evolution of AMR in bacteria continuously exposed to high AM concentrations while located within a biofilm. The resistance that evolved in our study was target based, i.e., conferred by mutations in topoisomerase genes. This is in contrast to earlier studies using short-term biofilms exposed to sub-inhibitory concentrations of CIP, where the resistance observed was low level and non-target based. Furthermore, we found that the resistance evolved more rapidly within the biofilm than in a planktonic suspension. This was most probably because the protection provided by the biofilm lifestyle allowed the susceptible bacteria to survive exposure to CIP concentrations above their MIC value. Biofilms are present and part of the pathogenesis in a number of bacterial infections, which are often treated with AM. Therefore, this study was performed at body temperature. Our findings indicate that AM treatment of biofilm-associated infections may pose a high risk of stimulating AMR development rather than eradication of the pathogens.
Data availability statement
The datasets presented in this study can be found in online repositories. The names of the repository/repositories and accession number(s) can be found below: https://www.ebi.ac.uk/ena, PRJEB63533.
Author contributions
SM: project administration and funding acquisition. BA, AO, and SM: experiments. LN and SM: data analyses. LN, AO, and SM: writing—original draft. All authors have conceptualization, writing—review and editing, read, and agreed to the submitted version of the manuscript.
Funding
This study was funded by the Norwegian Veterinary Institute.
Acknowledgments
We are grateful for valuable scientific advice from research scientist Jannice Schau Slettemeås and Dr. Amar Anandrao Telke.
Conflict of interest
The authors declare that the research was conducted in the absence of any commercial or financial relationships that could be construed as a potential conflict of interest.
Publisher’s note
All claims expressed in this article are solely those of the authors and do not necessarily represent those of their affiliated organizations, or those of the publisher, the editors and the reviewers. Any product that may be evaluated in this article, or claim that may be made by its manufacturer, is not guaranteed or endorsed by the publisher.
Supplementary material
The Supplementary Material for this article can be found online at: https://www.frontiersin.org/articles/10.3389/fmicb.2023.1246895/full#supplementary-material
Footnotes
- ^ http://www.eucast.org
- ^ https://www.bioinformatics.babraham.ac.uk/projects/fastqc/
- ^ https://github.com/FelixKrueger/TrimGalore
- ^ https://github.com/tseemann/snippy
References
Ahmed, M. N., Abdelsamad, A., Wassermann, T., Porse, A., Becker, J., Sommer, M. O. A., et al. (2020). The evolutionary trajectories of P. aeruginosa in biofilm and planktonic growth modes exposed to ciprofloxacin: Beyond selection of antibiotic resistance. NPJ Biofilms. Microbiomes 6:28. doi: 10.1038/s41522-020-00138-8
Ahmed, M. N., Porse, A., Sommer, M. O. A., Hoiby, N., and Ciofu, O. (2018). Evolution of antibiotic resistance in biofilm and planktonic Pseudomonas aeruginosa populations exposed to subinhibitory levels of ciprofloxacin. Antimicrob. Agents Chemother. 62, e00320-18. doi: 10.1128/AAC.00320-18
Anderl, J. N., Franklin, M. J., and Stewart, P. S. (2000). Role of antibiotic penetration limitation in Klebsiella pneumoniae biofilm resistance to ampicillin and ciprofloxacin. Antimicrob. Agents Chemother. 44, 1818–1824. doi: 10.1128/AAC.44.7.1818-1824.2000
Araujo, P. A., Mergulhao, F., Melo, L., and Simoes, M. (2014). The ability of an antimicrobial agent to penetrate a biofilm is not correlated with its killing or removal efficiency. Biofouling 30, 675–683. doi: 10.1080/08927014.2014.904294
Bhatnagar, K., and Wong, A. (2019). The mutational landscape of quinolone resistance in Escherichia coli. PLoS One 14:e0224650. doi: 10.1371/journal.pone.0224650
Bowler, P. G. (2018). Antibiotic resistance and biofilm tolerance: A combined threat in the treatment of chronic infections. J. Wound Care 27, 273–277. doi: 10.12968/jowc.2018.27.5.273
Ciofu, O., Rojo-Molinero, E., Macia, M. D., and Oliver, A. (2017). Antibiotic treatment of biofilm infections. Apmis 125, 304–319.
Coimbra, R. S., Grimont, F., Lenormand, P., Burguiere, P., Beutin, L., and Grimont, P. A. (2000). Identification of Escherichia coli O-serogroups by restriction of the amplified O-antigen gene cluster (rfb-Rflp). Res. Microbiol. 151, 639–654. doi: 10.1016/s0923-2508(00)00134-0
Dalhoff, A. (2012). Global fluoroquinolone resistance epidemiology and implictions for clinical use. Interdiscip. Perspect. Infect. Dis. 2012:976273. doi: 10.1155/2012/976273
El-Attar, M. S., Sadeek, S. A., Abd El-Hamid, S. M., and Elshafie, H. S. (2022). Spectroscopic analyses and antimicrobial activity of novel ciprofloxacin and 7-Hydroxy-4-methylcoumarin, the plant-based natural benzopyrone derivative. Int. J. Mol. Sci. 23:8019. doi: 10.3390/ijms23148019
Engelhardt, D., and Shakhnovich, E. I. (2019). Mutation rate variability as a driving force in adaptive evolution. Phys. Rev. E 99:022424. doi: 10.1103/PhysRevE.99.022424
European Food Safety Authority [EFSA], Aerts, M., Battisti, A., Hendriksen, R., Kempf, I., Teale, C., et al. (2019). Technical specifications on harmonised monitoring of antimicrobial resistance in zoonotic and indicator bacteria from food-producing animals and food. EFSA J. 17:e05709. doi: 10.2903/j.efsa.2019.5709
Ewels, P., Magnusson, M., Lundin, S., and Kaller, M. (2016). Multiqc: Summarize analysis results for multiple tools and samples in a single report. Bioinformatics 32, 3047–3048. doi: 10.1093/bioinformatics/btw354
France, M. T., Cornea, A., Kehlet-Delgado, H., and Forney, L. J. (2019). Spatial structure facilitates the accumulation and persistence of antibiotic-resistant mutants in biofilms. Evol. Appl. 12, 498–507. doi: 10.1111/eva.12728
Hadjifrangiskou, M., Gu, A. P., Pinkner, J. S., Kostakioti, M., Zhang, E. W., Greene, S. E., et al. (2012). Transposon mutagenesis identifies uropathogenic Escherichia coli biofilm factors. J. Bacteriol. 194, 6195–6205. doi: 10.1128/JB.01012-12
Hamed, S. M., Elkhatib, W. F., El-Mahallawy, H. A., Helmy, M. M., Ashour, M. S., and Aboshanab, K. M. A. (2018). Multiple mechanisms contributing to ciprofloxacin resistance among gram negative bacteria causing infections to cancer patients. Sci. Rep. 8:12268. doi: 10.1038/s41598-018-30756-4
Han, X., Dorsey-Oresto, A., Malik, M., Wang, J. Y., Drlica, K., Zhao, X., et al. (2010). Escherichia coli genes that reduce the lethal effects of stress. BMC Microbiol. 10:35. doi: 10.1186/1471-2180-10-35
Hasman, H., Chakraborty, T., and Klemm, P. (1999). Antigen-43-mediated autoaggregation of Escherichia coli is blocked by fimbriation. J. Bacteriol. 181, 4834–4841. doi: 10.1128/JB.181.16.4834-4841.1999
Huseby, D. L., Pietsch, F., Brandis, G., Garoff, L., Tegehall, A., and Hughes, D. (2017). Mutation supply and relative fitness shape the genotypes of ciprofloxacin-resistant Escherichia coli. Mol. Biol. Evol. 34, 1029–1039. doi: 10.1093/molbev/msx052
Jacoby, G. A. (2005). Mechanisms of resistance to quinolones. Clin. Infect. Dis. 41(Suppl. 2), S120–S126.
Krogfelt, K. A., Bergmans, H., and Klemm, P. (1990). Direct evidence that the FimH protein is the mannose-specific adhesin of Escherichia coli type 1 Fimbriae. Infect. Immun. 58, 1995–1998. doi: 10.1128/iai.58.6.1995-1998.1990
McKenzie, G. J., Harris, R. S., Lee, P. L., and Rosenberg, S. M. (2000). The Sos response regulates adaptive mutation. Proc. Natl. Acad. Sci. U.S.A. 97, 6646–6651. doi: 10.1073/pnas.120161797
Nesse, L. L., Mo, S. S., Ramstad, S. N., Witso, I. L., Sekse, C., Bruvoll, A. E. E., et al. (2021). The effect of antimicrobial resistance plasmids carrying bla(Cmy-2) on biofilm formation by Escherichia coli from the broiler production chain. Microorganisms 9:104. doi: 10.3390/microorganisms9010104
Nesse, L. L., Osland, A. M., and Vestby, L. K. (2023). The Role of biofilms in the pathogenesis of animal bacterial infections. Microorganisms 11:608. doi: 10.3390/microorganisms11030608
Nesse, L. L., and Simm, R. (2018). Biofilm: A hotspot for emerging bacterial genotypes. Adv. Appl. Microbiol. 103, 223–246. doi: 10.1016/bs.aambs.2018.01.003
Nichol, D., Rutter, J., Bryant, C., Hujer, A. M., Lek, S., Adams, M. D., et al. (2019). Antibiotic collateral sensitivity is contingent on the repeatability of evolution. Nat. Commun. 10:334. doi: 10.1038/s41467-018-08098-6
Olsen, I. (2015). Biofilm-specific antibiotic tolerance and resistance. Eur. J. Clin. Microbiol. Infect. Dis. 34, 877–886.
Penesyan, A., Nagy, S. S., Kjelleberg, S., Gillings, M. R., and Paulsen, I. T. (2019). Rapid microevolution of biofilm cells in response to antibiotics. NPJ Biofilms. Microbiomes 5:34. doi: 10.1038/s41522-019-0108-3
Pratt, L. A., and Kolter, R. (1998). Genetic analysis of Escherichia coli biofilm formation: Roles of flagella, motility, chemotaxis and type I pili. Mol. Microbiol. 30, 285–293. doi: 10.1046/j.1365-2958.1998.01061.x
Qin, T. T., Kang, H. Q., Ma, P., Li, P. P., Huang, L. Y., and Gu, B. (2015). Sos response and its regulation on the fluoroquinolone resistance. Ann. Transl. Med. 3:358. doi: 10.3978/j.issn.2305-5839.2015.12.09
Redgrave, L. S., Sutton, S. B., Webber, M. A., and Piddock, L. J. (2014). Fluoroquinolone resistance: Mechanisms, impact on bacteria, and role in evolutionary success. Trends Microbiol. 22, 438–445. doi: 10.1016/j.tim.2014.04.007
Rosenkilde, C. E. H., Munck, C., Porse, A., Linkevicius, M., Andersson, D. I., and Sommer, M. O. A. (2019). Collateral sensitivity constrains resistance evolution of the Ctx-M-15 beta-lactamase. Nat. Commun. 10:618. doi: 10.1038/s41467-019-08529-y
Santos-Lopez, A., Marshall, C. W., Scribner, M. R., Snyder, D. J., and Cooper, V. S. (2019). Evolutionary pathways to antibiotic resistance are dependent upon environmental structure and bacterial lifestyle. eLife 8:e47612. doi: 10.7554/eLife.47612
Seemann, T. (2014). Prokka: Rapid prokaryotic genome annotation. Bioinformatics 30, 2068–2069. doi: 10.1093/bioinformatics/btu153
Simonsen, G. S., and Urdahl, A. M. (2014). Norm/Norm-Vet 2013 usage of antimicrobial agents and occurrence of antimicrobial resistance in Norway. Tromsø: Norm/Normvet.
Sorlozano, A., Gutierrez, J., Jimenez, A., De Dios Luna, J., and Martinez, J. L. (2007). Contribution of a new mutation in parE to quinolone resistance in extended-spectrum-beta-lactamase-producing Escherichia coli isolates. J. Clin. Microbiol. 45, 2740–2742. doi: 10.1128/JCM.01093-07
Trampari, E., Holden, E. R., Wickham, G. J., Ravi, A., Martins, L. O., Savva, G. M., et al. (2021). Exposure of Salmonella biofilms to antibiotic concentrations rapidly selects resistance with collateral tradeoffs. NPJ Biofilms. Microbiomes. 7:3. doi: 10.1038/s41522-020-00178-0
Usui, M., Yoshii, Y., Thiriet-Rupert, S., Ghigo, J. M., and Beloin, C. (2023). Intermittent antibiotic treatment of bacterial biofilms favors the rapid evolution of resistance. Commun. Biol. 6:275. doi: 10.1038/s42003-023-04601-y
Vestby, L. K., Gronseth, T., Simm, R., and Nesse, L. L. (2020). Bacterial biofilm and its role in the pathogenesis of disease. Antibiotics 9, 59. doi: 10.3390/antibiotics9020059
World Health Organization [WHO] (2017). Critically important antimicrobials for human medicine – 5th rev. Geneva: WHO.
World Health Organization [WHO] (2020). Antibiotic resistance. Available online at: https://www.who.int/news-room/fact-sheets/detail/antibiotic-resistance (accessed April 13, 2023).
Keywords: antimicrobial resistance, biofilm, evolution, ciprofloxacin, E. coli
Citation: Nesse LL, Osland AM, Asal B and Mo SS (2023) Evolution of antimicrobial resistance in E. coli biofilm treated with high doses of ciprofloxacin. Front. Microbiol. 14:1246895. doi: 10.3389/fmicb.2023.1246895
Received: 24 June 2023; Accepted: 07 August 2023;
Published: 05 September 2023.
Edited by:
Hazem Salaheldin Elshafie, University of Basilicata, ItalyReviewed by:
Ahmed S. Khairalla, University of Regina, CanadaIppolito Camele, University of Basilicata, Italy
Copyright © 2023 Nesse, Osland, Asal and Mo. This is an open-access article distributed under the terms of the Creative Commons Attribution License (CC BY). The use, distribution or reproduction in other forums is permitted, provided the original author(s) and the copyright owner(s) are credited and that the original publication in this journal is cited, in accordance with accepted academic practice. No use, distribution or reproduction is permitted which does not comply with these terms.
*Correspondence: Live L. Nesse, bGl2ZS5uZXNzZUB2ZXRpbnN0Lm5v