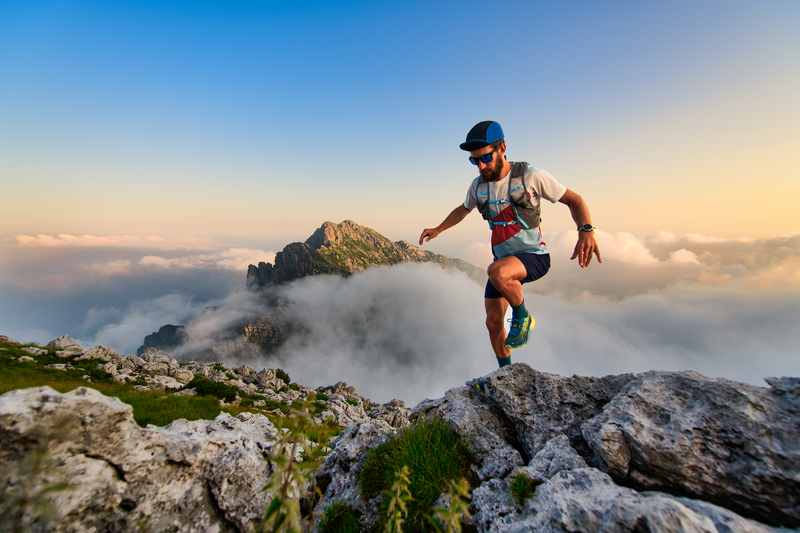
95% of researchers rate our articles as excellent or good
Learn more about the work of our research integrity team to safeguard the quality of each article we publish.
Find out more
REVIEW article
Front. Microbiol. , 14 September 2023
Sec. Microbial Symbioses
Volume 14 - 2023 | https://doi.org/10.3389/fmicb.2023.1244239
This article is part of the Research Topic Frontiers in Wolbachia Biology 2023 View all 15 articles
Wolbachia have been developed as a tool for protecting humans from mosquito populations and mosquito-borne diseases. The success of using Wolbachia relies on the facts that Wolbachia are maternally transmitted and that Wolbachia-induced cytoplasmic incompatibility provides a selective advantage to infected over uninfected females, ensuring that Wolbachia rapidly spread through the target pest population. Most transinfected Wolbachia exhibit a strong antiviral response in novel hosts, thus making it an extremely efficient technique. Although Wolbachia has only been used to control mosquitoes so far, great progress has been made in developing Wolbachia-based approaches to protect plants from rice pests and their associated diseases. Here, we synthesize the current knowledge about the important phenotypic effects of Wolbachia used to control mosquito populations and the literature on the interactions between Wolbachia and rice pest planthoppers. Our aim is to link findings from Wolbachia-mediated mosquito control programs to possible applications in planthoppers.
Wolbachia are a group of gram-negative bacteria that live inside invertebrate cells and have been successfully developed to control mosquitoes and mosquito-borne diseases by decreasing host population density or decreasing host virus transmission. Unlike chemical control approaches, which result in collateral destruction of beneficial insects, Wolbachia-mediated population control has proven to be an excellent vector-control agent because it targets a single species. Moreover, as the target population is suppressed, the chemical control approaches become less effective, while Wolbachia-mediated pest population control is more effective. Because of Wolbachia pervasiveness in nature and lack of genetic modification, Wolbachia-mediated control programs are accepted as environmentally friendly biocontrol strategies to control insect pest populations and disease vectors. To date, the Wolbachia control strategies successfully used have been limited to mosquitoes. There is a question of whether Wolbachia control strategies could be applied more broadly to other pest insects and insect-borne diseases.
Rice (Oryza sativa), cultivated extensively in the tropical and subtropical regions of the world, is the staple food for billions of people worldwide (Sarao et al., 2016). Rice planthoppers (Hemiptera: Delphacidae), the most destructive pests of rice, suck rice sap and oviposit in rice tissues, inducing a substantial threat to rice production. In addition to heavy infestations, rice planthoppers also act as vectors of major plant viruses, such as rice stripe virus, rice black-streaked dwarf virus, rugged stunt virus, grassy stunt virus, and southern rice black-streaked dwarf virus (Hibino, 1996). Various strategies have been developed to control planthoppers. Among those strategies, spraying chemical insecticides is the main method used for controlling this pest. However, blanket application of insecticides has already induced planthopper resistance and disrupted the ecological balance of rice ecosystems in most rice planting countries. Thus, a more practical, economical and environmentally friendly strategy is urgently needed to control planthoppers and their associated diseases.
The success of Wolbachia-mediated mosquito control programs promotes similar strategies that could be applied to planthoppers. Here, we summarize the important properties of Wolbachia used for mosquito control, including stability transmission, host reproduction alteration, and pathogen inhibition. We also review the current knowledge about the interactions between Wolbachia and planthoppers and point out the similarities and differences in biology between mosquitoes and planthoppers to link findings from Wolbachia-mediated mosquito control programs to possible applications in planthoppers.
Wolbachia strains were first identified in the reproductive tissue of Culex pipiens in 1924 (Hertig and Wolbach, 1924). Since then, these bacteria have been found to infect approximately half of all arthropod species from terrestrial and aquatic environments, including nematodes, mites, spiders and all orders of insects (Weinert et al., 2015). Wolbachia formed a monophyletic group with other insect-associated microorganisms using 16S rRNA gene sequences. In recent decades, a large number of Wolbachia with close phylogenetic affinity have been revealed by PCR and sequencing techniques. Based on the variable gene ftsZ, Wolbachia from arthropods form two divergent clades; several different Wolbachia strains from filarial nematodes are assigned to two additional clades (Werren et al., 1995; Bandi et al., 1998). These clades have since been termed supergroups, which are used to describe the divergence of the Wolbachia group. In addition, Wolbachia surface protein (wsp) and groEL genes are used to distinguish the major phylogenetic subdivisions of Wolbachia. Due to extensive recombination and strong diversifying selection in the wsp gene, wsp should therefore be unsuitable for use alone for reliable Wolbachia strain characterization when trying to type and quantify strain diversity (Werren and Bartos, 2001; Baldo et al., 2005; Lo et al., 2007). Considering that a single-locus approach to strain characterization may be misleading, a multilocus sequence typing (MLST) system has been established to type Wolbachia strains using five standard housekeeping genes (gatB, coxA, hcpA, fbpA, and ftsZ) (Baldo et al., 2006). Based on the combination of alleles at a sample of housekeeping genes, the MLST approach defines a strain as a sequence type. This accurate strain typing system MLST using combinations of alleles as molecular markers to genotype strains is considered a universal and unambiguous tool for Wolbachia strain typing, molecular evolutionary, and population genetics studies (Baldo et al., 2006). Overall, the MLST system provides an excellent method for typing Wolbachia strains from diverse hosts and for discriminating among strains in the same host species (Baldo et al., 2006).
Wolbachia strains are subdivided into 17 supergroups from A to R, except for supergroup G, which is controversial (Baldo et al., 2006; Baldo and Werren, 2007; Wang et al., 2016; Zhao et al., 2021). The majority of Wolbachia strains found in insects belong to supergroups A and B. Most Wolbachia strains that infect arthropods are supergroups A, B, D, E, F, and H (Figure 1). As molecular biology techniques have developed, Wolbachia genome sequences are exploited to define genetic diversity and significant genes associated with altering host biology, as well as relationships between Wolbachia and hosts at the gene level (Kaur et al., 2021). To date, over 26 complete Wolbachia genomes have been published, and nearly 1,000 Wolbachia genomes from different arthropod and nematode species have been assembled (Scholz et al., 2020; Kaur et al., 2021). Our understanding of Wolbachia genetic diversity is still developing, which will help us to identify useful Wolbachia variants with desirable phenotypic effects for alternative Wolbachia-mediated population control strategies.
Figure 1. Wolbachia supergroups. Wolbachia strains are subdivided into different supergroups. Most Wolbachia supergroups are listed in the circle graph. Colors correspond to different patterns of Wolbachia-host associations across the supergroups. “?”: controversial supergroup; NA, not annotated at supergroup level.
Numerous studies have shown that Wolbachia exists in diverse cells and somatic tissues of the host, such as the salivary gland, fat bodies, ovary, testis, midgut, and tegument (Dobson et al., 1999; Toomey et al., 2013). Although Wolbachia have been found in host somatic tissues, they exhibit strong reproductive tissue tropism in the host (Frydman et al., 2006; Fast et al., 2011; Toomey et al., 2013). Wolbachia are rarely or not transmitted by sperm, while they accumulate in developing spermatocytes of male hosts (Clark et al., 2002; Ijichi et al., 2002; Ju et al., 2017). In female hosts, Wolbachia enter ovaries and spread into developing oocytes, eventually dispersing within the offspring of the host (Kose and Karr, 1995; Ferree et al., 2005). Thus, Wolbachia is considered as an intracellular maternally transmitted bacterium. The unique ability of Wolbachia to invade host populations has rapidly promoted their exploration as a potential tool in the control of pests.
Wolbachia persist and disperse in arthropods and filarial nematodes that mostly depend on their horizontal and vertical transmission. Wolbachia can transfer from one species to another, that is, horizontal transmission (Figure 2A), though it has low transmission efficiency. Phylogenetic incongruence between Wolbachia and their hosts suggests that horizontal transmission of Wolbachia occurs frequently between many hosts (Baldo et al., 2006; Su et al., 2019). MLST analysis of Wolbachia and successful horizontal transfer of Wolbachia by microinjection have also provided evidence for horizontal transmission (Xi et al., 2005, 2006; Li et al., 2017; Zheng et al., 2019). As recorded, horizontal transmission of Wolbachia could occur by many pathways, such as feeding on common plants (Sintupachee et al., 2006; Le Clec'h et al., 2013; Li et al., 2017; Sanaei et al., 2023), parasitic wasps (Ahmed et al., 2015; Brown and Lloyd, 2015; Goya et al., 2022), parasitic mites (Houck et al., 1991; Jaenike et al., 2007; Gehrer and Vorburger, 2012), hybridization (Jiang et al., 2018; Su et al., 2019), and predation (Goodacre et al., 2006; Wang et al., 2010; Su et al., 2019). Although interspecific horizontal transmission inefficiently occurs, Wolbachia horizontal transmission is found in many insects, including rice planthoppers (Zhang et al., 2013), wasps (Huigens et al., 2004; Goya et al., 2022; Zhou et al., 2022), fruit flies (Turelli et al., 2018), trypetids (Schuler et al., 2013), psyllids (Serbina et al., 2022), moths (Ahmed et al., 2016), ladybirds (Shaikevich and Romanov, 2023), mosquitoes (Shaikevich et al., 2019), mites (Su et al., 2019), butterflies (Ahmed et al., 2016; Zhao et al., 2021) and so forth.
Figure 2. Wolbachia transmission. Wolbachia persist and disperse in hosts by horizontal and vertical transmission. (A) Wolbachia horizontally transmit from one species to another. The most common horizontal transmission of Wolbachia occurs by parasitic wasps. Parasitic wasps infect Wolbachia when they parasitize a Wolbachia-infected host, then transfer Wolbachia to new hosts when they parasitize closest species. (B) Wolbachia vertically transmit from mother to offspring. In female hosts, Wolbachia infect the germinal tissues, enter into the developing oocytes and be incorporated into the embryos, eventually dispersing within the offspring of host. Red dots: Wolbachia.
Wolbachia can also vertically transmit from mother to offspring via the host egg cytoplasm (Figure 2B), which is considered the main pathway for infection transfer across hosts (Werren, 1997). Vertical transmission of symbionts in hosts is generally maternal and occurs through trans eggs and transovarial transmission (Rosen, 1988; Lequime et al., 2016). In trans-egg transmission, Wolbachia spread into eggs at the time of oviposition. In transovarial transmission, Wolbachia infect the germinal tissues and enter into the developing oocytes of the female host. When Wolbachia initially infect a new host, they need to reach the germinal tissues for successful transovarial transmission (Werren et al., 2008). Wolbachia transovarial transmission relies on the infection of developing oocytes, which results in nearly 100% infection of the host progeny (Lequime et al., 2016). Due to the difficultly of detecting trans-egg transmission in vitro and vivo, Wolbachia vertical transmission in the host is mostly focused on transovarial transmission. The factors that impact Wolbachia vertical transmission are complex and undistinguishable and are related to Wolbachia densities, interactions with other symbionts, and the ability of Wolbachia to migrate into the host oocyte.
Wolbachia vertical transmission has been intensively investigated in Diptera insects. Drosophila ovarioles are of the polytrophic meroistic type and divide into the terminal filament, germarium, and vitellarium from tip to pedicel (Szklarzewicz et al., 2007; Swiatoniowska et al., 2013; Szklarzewicz et al., 2013). The female germline stem cell niche (GSCN) is on the apical tip of the germarium, where germline stem cells divide asymmetrically, and one daughter cell exits the GSCN and forms the egg’s germline (Fast et al., 2011). Germline cells divide and form egg chambers in the germarium and finally mature into eggs in the vitellarium. Observation research found an intense accumulation of Wolbachia in the GSCN and the somatic stem cell niche (SSCN), which is located at the germarium and supports somatic stem cells (Frydman et al., 2006; Fast et al., 2011). Further research showed that Wolbachia enter the ovaries of Drosophila from the anterior tip of the germarium (Martinez et al., 2014). After that, Wolbachia utilize the host actin cytoskeleton during oogenesis for efficient transmission and maintenance between Drosophila generations (Newton et al., 2015). Actin-inhibiting drugs significantly abrogate Wolbachia uptake in the host, indicating that the host actin cytoskeleton plays an important role in Wolbachia transmission (Ferree et al., 2005; Newton et al., 2015; Nevalainen et al., 2023).
Wolbachia impact the ecology, evolution, and reproductive biology of their host species to increase their already widespread distribution. Wolbachia are best known for their effects on host reproduction, such as male killing, feminization, thelytokous parthenogenesis, and cytoplasmic incompatibility (CI) (Werren et al., 2008). In 1971, Wolbachia were first verified to be associated with CI, causing the embroys of hosts to perish, which occurs when males carrying Wolbachia mate with females that are uninfected or harboring different Wolbachia strains (Yen and Barr, 1971; Werren, 1997; Hoffmann, 2020). A range of negative fecundity effects or no effects associated with Wolbachia has been described, although the mechanisms responsible for those fitness effects are mostly unknown. Other Wolbachia strains exhibit strong positive fecundity effects on their host, including fecundity increases (Fast et al., 2011; Guo et al., 2018a).
How Wolbachia manipulate the reproduction of hosts, especially Wolbachia-induced CI, has attracted great attention in recent decades. Although the means by which Wolbachia mediate CI are currently unknown, there is a general consensus that Wolbachia modify sperm at an early stage of spermatogenesis, and a rescue activity takes place in the same Wolbachia-infected egg to reverse or neutralize the modification of sperm following fertilization (Werren, 1997; Xiao et al., 2021). Three different models have been proposed to account for the mechanisms of CI induction and rescue: the “lock-and-key,” “slow-motion,” and “titration-restitution” models (Figure 3; Poinsot et al., 2003). Moreover, Wolbachia genes involved in modification and rescue have been identified, which are collectively named cifA and cifB. The two genes are organized into an operon-like genetic element, which encodes the CifA and CifB proteins (Beckmann et al., 2017; LePage et al., 2017). To distinguish the CI-inducing modifications and CifA rescues viability, two types of functional models for CI have been proposed. In the host modification models, Wolbachia Cifs (CifA and CifB) modify the infected sperm, resulting in CI when the modified sperm fertilizes an uninfected egg (Kose and Karr, 1995; Werren, 1997; Bossan et al., 2011). In the “toxin-antidote” model, CifB disrupts the processing of paternally derived chromosomes or nuclease activity and then changes or delays paternal chromatin condensation and separation during the first zygotic mitosis (Tram and Sullivan, 2002). Even so, much remains to be learned about the actual molecular mechanisms of CI induction and rescue, which can help account for CI in insects infected with different Wolbachia strains.
Figure 3. CI induction and rescue models. (A) The “lock-and-key” model. Wolbachia (red dots) produce a “lock” (green triangle) binding on paternal chromosomes. Wolbachia are shed with most of the cytoplasm as spermatogenesis. Cytoplasmic incompatibility occurs in crosses between infected males and uninfected female because the paternal material is “locked-in,” while eggs infected by Wolbachia remain compatible after fertilization because Wolbachia produce a “key” in the egg which removes the lock. (B) The “slow-motion” model. Wolbachia (red dots) produce a slowing down factor (purple star) binding on paternal chromosomes. After that, Wolbachia are shed from the maturing spermatocyte. Embryonic mortality occurs in crosses between infected males and uninfected females because Wolbachia slow down paternal chromosomes movements during the first embryonic mitosis, which is rescued by the similar modification of maternal chromosomes when Wolbachia are present in the egg. (C) The “titration-restitution” model. Wolbachia (red dots) titrate out a protein (semicircles) of paternal and maternal chromosomes. The titrated protein of paternal chromosomes is expelled as Wolbachia are shed from the maturing spermatocyte. Cytoplasmic incompatibility occurs when sperm cell enters an uninfected egg due to lack of the host protein. Rescue occurs between two infected individuals, because the Wolbachia in eggs give back the host protein (blue semicircles) to maternal and paternal chromosomes.
The feature of Wolbachia inducing a conditional sterility CI in infected insects is important for pest and disease control. In recent years, CI has been successfully explored to control the mosquito population and mosquito-borne diseases through population suppression or population replacement approaches. In the population suppression approach, large numbers of Wolbachia-infected male mosquitoes are released into the field, and the male sterility induced by CI causes significant drops in mosquito number. In the population replacement strategy, both Wolbachia-infected male mosquitoes and infected female mosquitoes are released, which can suppress mosquito-borne diseases by decreasing host virus transmission. Overall, Wolbachia-induced CI is central to both population suppression and population replacement programs (Ross et al., 2019).
Reducing the infection or transmission of pathogens is another important property of Wolbachia used for pest and disease control. Wolbachia can inhibit RNA viral replication, which was initially discovered in Drosophila melanogaster (Hedges et al., 2008; Teixeira et al., 2008). Subsequently, Wolbachia were found to be broadly effective against mosquito-borne diseases such as Zika virus, chikungunya virus, dengue virus, yellow fever virus, and West Nile virus, making them less capable of transmitting infection to offspring and humans (Bian et al., 2013a; Ford et al., 2019). Wolbachia can also confer resistance against eukaryotic parasites (Bourtzis et al., 2014), providing a broad range of pathogen protection. Several studies have shown that the antiviral response is dramatically enhanced by Wolbachia newly transinfected to the host, although natural Wolbachia-infected mosquitoes are found to limit virus replication and transmission (Armbruster et al., 2003; Guo et al., 2022). Microinjection technology expands the entry of Wolbachia into new hosts. Once Wolbachia infects, Wolbachia-induced CI produces a frequency-dependent fitness advantage that can drive the spread of Wolbachia within new hosts (Sullivan, 2020). Data have further indicated that the extent of viral inhibition provided by transinfected Wolbachia depends on the Wolbachia variants, host species, virus and host-Wolbachia–virus interactions.
Wolbachia-induced pathogen inhibition is variable between related hosts and different Wolbachia strains in the same host, which is strongly linked to the density of Wolbachia in host tissues. In mosquitoes, virus inhibition correlates with higher Wolbachia density in the salivary glands, midgut, and ovaries. Unlike mosquitoes, high Wolbachia densities in the head, gut, and Malpighian tubules of Drosophila are thought to be important for virus inhibition (Osborne et al., 2012). It is widely believed that higher Wolbachia densities are important for effective antiviral behavior (Chrostek et al., 2013), and Wolbachia may confer virus inhibition by interfering with viral binding, entry into the cell, and RNA replication in the early stages (Schultz et al., 2018; Lu et al., 2020). Regardless of which pathway Wolbachia acts on, the production of progeny viruses from the same Wolbachia-infected cells is reduced, and virus dissemination and transmission are ultimately limited (Kaur et al., 2021). Interestingly, the varied extent of virus inhibition was also associated with viral dose. Recent data suggest that wMel exhibits strong inhibition in high dengue dose mosquitoes, while inhibition appears lower or even increases virus transmission when the dengue dose is low (King et al., 2018).
Wolbachia-induced pathogen inhibition may be related to the upregulation of host innate immunity (Figure 4). This is evident from the inhibition caused by Wolbachia newly transferred to hosts (Moreira et al., 2009; Walker et al., 2011; Ant et al., 2018). In mosquitoes with transinfected Wolbachia strains, Wolbachia upregulate the expression of genes involved in innate defense pathways and then prime insect innate immunity to block pathogen replication (Bian et al., 2013b; Moretti et al., 2018). However, inhibition associated with native Wolbachia variants does not show an immune-priming phenotype but does confer antiviral activity (Mousson et al., 2012). These results suggest that innate immune priming may occur in hosts with newly transinfected Wolbachia variants or novel host-Wolbachia associations (Rances et al., 2012).
Figure 4. Wolbachia upregulate host innate immunity. Wolbachia enhance the synthesis of antimicrobial peptides and melanization by impacting the central genes of host immune-signaling pathways. The increased innate immunity partially accounts for Wolbachia inhibiting pathogens. Red dots: Wolbachia; red arrow: upregulation; red “X”: inhibition.
Another explanation for Wolbachia inhibiting virus replication is the competition for resources between viruses, Wolbachia, and the host cell (Figure 5). Viral replication and Wolbachia growth in the host are tightly regulated by cholesterol metabolism (Lin and Rikihisa, 2003). A recent study has shown that Wolbachia is unable to synthesize cholesterol de novo and that its replication is cholesterol dependent. Thus, cholesterol depletion of host cells by Wolbachia could directly interfere with virus replication in the same host (Rainey et al., 2014). In addition to cholesterol, iron homeostasis needs to be tightly regulated to enable viral replication and bacterial growth. In Wolbachia-infected mosquitoes, the iron-binding proteins transferrin and ferritin were upregulated, suggesting that Wolbachia regulated iron homeostasis (Kremer et al., 2009; Rances et al., 2012). However, this phenomenon is reversed when the host infect is infected with virus (Tchankouo-Nguetcheu et al., 2010). These experiments related to Wolbachia, antiviral activity and host cells are intriguing, clearly suggesting that host cell resources are important for both viral replication and Wolbachia growth. In summary, to develop alternative vector-control strategies, much remains to be learned concerning the mechanisms of Wolbachia-mediated pathogen inhibition.
Figure 5. Competition for host cell resources. Both Wolbachia growth and virus replication rely on host cell resources. Resources depletion of host cells by Wolbachia interferes with virus replication in host limited cell resources. Red dots: Wolbachia; orange drop shapes and gray irregular shapes: host cell resources.
The small brown planthopper (Laodelphax striatellus; SBPH), brown planthopper (Nilaparvata lugens; BPH), and white-backed planthopper (Sogatella furcifera; WBPH) are the three serious and destructive pests of rice that directly cause 20 to 40% of crop loss globally each year (Yang and Zhang, 2016; Sullivan, 2020). Wolbachia in SBPH were first discovered in 1992 using partial sequences of the ribosomal DNA (Rousset et al., 1992), and WBPH was reported to harbor the same Wolbachia strain wStri in 2003 (Kittayapong et al., 2003). In contrast to SBPH and WBPH, BPH was found to be infected with a different Wolbachia strain, wLug. There were significantly different infection statuses among the three planthoppers (Table 1). The infection rate of Wolbachia in SBPHs increased gradually according to the investigation data from 1982 to 1994 (Noda, 1984a; Hoshizaki and Shimada, 1995). A recent study indicated that nearly all SBPHs were infected by Wolbachia in the rice-growing regions of China (Zhang et al., 2013). In WBPH, the infection rate of Wolbachia is different between females and males; nearly 100% of females are infected with Wolbachia, while only half of males are infected (Li et al., 2020). BPH is naturally infected by the Wolbachia strain wLug at a prevalence of only ~18%, showing the lowest infection frequency among the three planthoppers (Qu et al., 2013).
Table 1. Wolbachia in small brown planthopper (SBPH), brown planthopper (BPH), and white-backed planthopper (WBPH).
Wolbachia is located in multiple tissues of planthoppers, including somatic tissues, ovaries, and testes. The somatic localization of Wolbachia is thought to facilitate their horizontal transmission, which also indicates the complex interactions between Wolbachia and the host. The reproductive localization of Wolbachia is thought to facilitate their vertical transmission. Wolbachia exhibit high-efficiency vertical transmission in planthoppers, as they do in the model insect Drosophila (Nakamura et al., 2012; Guo et al., 2018b), which occurs only in female hosts. In contrast to Drosophila ovarioles, planthopper ovarioles are of the telotrophic meroistic type and consist of a terminal filament, tropharium, and vitellarium (Szklarzewicz et al., 2013; Guo et al., 2018b). A cluster of nurse cells connected to the central trophic core radially arranged in the anterior of the tropharium; previtellogenesis arranged on the base of the tropharium (Szklarzewicz et al., 2007). Developing oocytes arrange in the vitellarium, which connects the tropharium through nutritive cords (Szklarzewicz et al., 2007, 2013). Wolbachia bind to Vg outside the ovarioles and endocytose into the tropharium of planthoppers during the early phase of vitellogenesis (Guo et al., 2018b). Wolbachia in the tropharium enter the arrested oocyte and establish an early infection as the trophic core divides. In addition, Wolbachia in the nurse cells spread into the developing oocytes through the nutritive cords that are wide channels formed between nurse cells and establish stable inheritance in host generation (Guo et al., 2018b). Wolbachia behavior during host embryogenesis is also well characterized. Microscopic observations indicated that Wolbachia were mainly localized at the anterior part cells of the embryo in early embryogenesis and then migrated to the posterior region during late embryogenesis, where gonads were formed (Guo et al., 2019). Research related to Wolbachia transmission in host oogenesis and embryogenesis can partially explain how Wolbachia exhibit high vertical transmission in planthoppers.
Wolbachia show different functions on three planthoppers (Table 1). Recent research has shown that Wolbachia provide beneficial effects to BPH. Egg production in Wolbachia-infected BPH females is higher than that in uninfected females. However, the longevity of Wolbachia-infected BPHs is shorter than that of uninfected BPHs, which may partially explain the high egg production and low prevalence of Wolbachia in wild BPH. Similar to BPH, Wolbachia also significantly increased the fecundity of SBPH, which may be associated with the high number of ovarioles that contain apoptotic nurse cells and mitotic germ cells (Guo et al., 2018b, 2020). In addition, Wolbachia affects the miRNA expression of SBPH to alter the expression of genes related to fecundity (Liu et al., 2019). Further experimental and genomic evidence demonstrated that Wolbachia increases the fecundity of BPH and SBPH females by synthesizing the essential nutrients biotin and riboflavin (Ju et al., 2020). In contrast, Wolbachia exhibit negative effects on WBPH; Wolbachia-infected females produce fewer eggs than Wolbachia-uninfected females (Li et al., 2022). Although many studies have focused on the interactions between Wolbachia and planthoppers, the mechanism of Wolbachia-mediated alterations in planthopper oogenesis has not yet been explored.
The CI phenotype in laboratory and wild SBPH populations was found in 1984 (Noda, 1984b). In 1992, the CI phenotype in SBPH was confirmed, which was caused by Wolbachia wStri (Rousset et al., 1992). wStri induced strong CI in SBPH, and the level of CI remained high regardless of the age of Wolbachia-infected males. There are no viable eggs from Wolbachia-infected SBPH females that mated with uninfected SBPH males. RNA-seq comparative analysis of Wolbachia-infected and uninfected SBPH shows that iLvE mediates branched-chain amino acid biosynthesis and may be associated with Wolbachia-induced CI (Ju et al., 2017). Knocking down iLvE expression in Wolbachia-uninfected SBPH males partially rescued fertility in crosses between these males and Wolbachia-infected females. Wild WBPH populations are infected by the same Wolbachia wStri as SBPH are, while the level of CI in WBPH is very weak or even zero. However, a strong CI phenotype was expressed when WBPH was double-infected with Wolbachia and Cardinium bacterium, indicating that Wolbachia may only play an auxiliary role in the CI of WBPH (Li et al., 2022). Interestingly, Wolbachia wLug in wild BPH populations lacks the ability to induce CI. A recent study showed that BPH infected with wStri by microinjection exhibited a high CI level, although the CI level was much lower than that in the original host SBPH (Gong et al., 2020).
In recent years, effects other than reproductive effects on planthoppers have received increasing attention. Studies have shown that Wolbachia of planthoppers increase resistance to insecticides, protect against some RNA viruses, and have other effects. In SBPH, Wolbachia wStri is associated with increased resistance to the insecticide buprofezin, although there is no relationship between Wolbachia density and resistance (Li et al., 2020). BPH increased insecticide susceptibility and decreased detoxification metabolism when the density of Wolbachia was decreased by high temperature (Zhang et al., 2021). Further results indicated that wLug orchestrates the detoxification metabolism of BPH via the CncC pathway to promote host insecticide resistance. In addition, Wolbachia wStri was recently shown to inhibit the growth of positive-sense RNA mosquito viruses, and the inhibition level was up to 99.9%. The presence of wStri did not affect the growth of the negative-sense RNA viruses in the Bunyaviridae and Rhabdoviridae families (Schultz et al., 2018). wStri in Aades albopictus cells has also been shown to repress ZIKV, and the inhibited stages of the ZIKV life cycle were identified to two distinct blocks, including reduction of ZIKV entry into cells and distraction viral genome replication in Wolbachia-infected cells. The addition of a cholesterol-lipid supplement partially rescued ZIKV entry in wStri-infected cells but did not rescue viral replication, showing that viral entry is affected in a cholesterol-dependent manner (Schultz et al., 2018). Wolbachia wStri has the ability to inhibit a wider variety of positive-sense RNA viruses, making it an attractive candidate for future vector-controlled approaches to limit viral infection and spread.
Wolbachia-based mosquito control strategies have been shown to be effective at limiting arbovirus disease spread in approximately 23 countries (Gong et al., 2023). Among them, over 8 countries have used Wolbachia-based mosquito population suppression strategies, which closely depend on Wolbachia-induced CI. The most important aspect of this strategy is that stable and heritable CI-induced Wolbachia infections should be established in target species. To control mosquitoes, adult sterile males with artificial Wolbachia infection have been released to mate with wild females. The eggs produced by these females are perishable, resulting in a target species population decline in a given period. However, the mass release of adult sterile males involves a potential risk of accidentally releasing fertile CI-induced Wolbachia-infected females. Insects are traditionally sterilized by radiation; combining Wolbachia-induced CI with the radiation sterilization technique can sterilize any residual females that are not removed from the released males using low-dose irradiation. Recent field trials indicated that the combination of Wolbachia and radiation sterilization resulted in a near elimination of mosquito populations (Zheng et al., 2019). Another efficient vector-control strategy is Wolbachia-based population replacement, which has been successfully used in 15 countries. The success of this strategy relies on two aspects of Wolbachia: pathogen inhibition and CI drive. Rather than releasing large numbers of Wolbachia-infected males to suppress insect populations, Wolbachia-infected females would be released to replace a wild uninfected population by a CI-based drive, reducing their vector competence and inhibiting arboviral disease (Kaur et al., 2021).
In recent years, great progress has been made in developing possible applications for protecting plants from planthoppers and their associated diseases. BPH is naturally infected with Wolbachia strain wLug at a low prevalence that does not cause CI. Gong et al. (2020) established a wStri-infected BPH line by withdrawing the embryo cytoplasm of SBPH and injecting it into the embryos of BPH. wStri maintained perfect maternal transmission in the new host BPH. The wStri-infected BPH exhibited near 100% CI, although it was slightly lower than that in its native host SBPH (Gong et al., 2020). The high level of CI and low fitness costs of wStri-infected BPHs enable individuals infected with wStri to rapidly invade BPH populations. Furthermore, wStri-infected BPH dramatically reduced planthopper RRSV viral loads and viral transmission to rice plants. The viral load in wStri-infected BPH decreased 75% relative to that in uninfected BPH (Gong et al., 2020). Otherwise, rice seedlings attacked by wStri-infected BPH resulted in a dramatic 82% lower incidence of viral infection compared with that attacked by uninfected BPH (Gong et al., 2020). Above all, the wStri strain appears to be well suited for the Wolbachia-based replacement strategy to control BPHs and their associated diseases, although much work still needs to be done before strategy implementation.
Possible Wolbachia-based population control applications for SBPH and WBPH are more complex than those for in BPH. SBPH and WBPH naturally carry Wolbachia, so double infections are needed for population replacement, whereas double infected strains or novel strains with native Wolbachia removed but carrying another variant added are needed for population suppression. First, a stable and heritable CI-induced Wolbachia infection line should be established by artificial transfection (Figure 6). Although embryonic microinjection technology significantly promotes Wolbachia transfection efficiency from donors to recipients, many problems remain, such as selecting useful Wolbachia variants, which have desirable phenotypic effects for alternative strategies and maintain stability in the longer term. Apart from native Wolbachia, new Wolbachia interactions with other endosymbionts and the complex microbiome could influence host fitness and indirectly affect Wolbachia invasion (Ross et al., 2019).
Figure 6. Properties of Wolbachia used for planthoppers control. The success of Wolbachia-based population control strategies relies on the important properties of Wolbachia including host reproduction alteration, pathogen inhibition, stability transmission and weak or no host fitness cost. CI, cytoplasmic incompatibility.
Host fitness cost is an important determinant in Wolbachia-based pest control strategies. The fitness of hosts is altered when hosts are infected with different Wolbachia strains. In general, natural Wolbachia infections are benign or even beneficial to the host, such as increasing fertility or lifespans as well as inhibiting the virus. In contrast, diverse negative effects on fitness are found when Wolbachia are transferred to novel hosts, depending on the Wolbachia strain and host. It is usually difficult to predict the fitness effects of Wolbachia on novel hosts because Wolbachia densities and tissue distributions dramatically change from native to novel hosts. Most negative effects are that Wolbachia transfections often reduce novel host fecundity or egg hatch, which may prevent transinfected Wolbachia establishment if they are too severe. The overall impact of Wolbachia infections on host fitness is often insufficiently estimated because it strongly depends on the environmental context. The fitness effects of Wolbachia observed in standard laboratory studies are only partial to estimate the dynamics of Wolbachia in natural populations.
Choose suitable Wolbachia-based population control strategies, population suppression or population replacement, which closely depend on the biology of the target pest. In mosquitoes, both males and females can feed on damaged and intact vegetative tissue, plant juices, damaged fruits, and homopterans, which act as an energy source for their physiological maintenance and locomotion. Only female mosquitoes bite animals or humans to take a blood meal, which is required for egg development. Therefore, there is no or little threat to animals or humans using Wolbachia-based mosquito suppression strategies by releasing adult sterile males. To efficiently reduce the prevalence of mosquito-borne diseases, Wolbachia-based replacement strategies were carried out by the release of Wolbachia-transinfected antiviral females or eggs. Nevertheless, both female and male planthoppers suck rice sap and transmit viral diseases, and there is no mature biotechnology or equipment for sex sorting to date. Hence, a population replacement strategy may be more suitable for controlling planthoppers than population suppression based on the current knowledge of interactions between Wolbachia and the host (Table 2). Moreover, the chosen strategies should be evaluated under field conditions to demonstrate the possibility of their practical implementation in the future.
YG and YL wrote the manuscript. JS and YW performed the images processing. All authors contributed to the article and approved the submitted version.
This study was supported by the Research and Development program in key areas of Guangdong Province (Grant No. 2021B0707010010), the Special Fund for Scientific Innovation Strategy-Construction of High-Level Academy of Agriculture Science (Grant Nos. R2021YJ-YB2007 and 202117TD), and the National Natural Science Foundation of China (grant no. 31901879).
We thank ZhiHao Zheng of the Plant Protection Research Institute, Guangdong Academy of Agricultural Science, China for his thoughtful suggestions on the review.
The authors declare that the research was conducted in the absence of any commercial or financial relationships that could be construed as a potential conflict of interest.
All claims expressed in this article are solely those of the authors and do not necessarily represent those of their affiliated organizations, or those of the publisher, the editors and the reviewers. Any product that may be evaluated in this article, or claim that may be made by its manufacturer, is not guaranteed or endorsed by the publisher.
Ahmed, M. Z., Breinholt, J. W., and Kawahara, A. Y. (2016). Evidence for common horizontal transmission of Wolbachia among butterflies and moths. BMC Evol. Biol. 16:118. doi: 10.1186/s12862-016-0660-x
Ahmed, M. Z., Li, S. J., Xue, X., Yin, X. J., Ren, S. X., Jiggins, F. M., et al. (2015). The intracellular bacterium Wolbachia uses parasitoid wasps as phoretic vectors for efficient horizontal transmission. PLoS Pathog. 10:e1004672. doi: 10.1371/journal.ppat.1004672
Ant, T. H., Herd, C. S., Geoghegan, V., Hoffmann, A. A., and Sinkins, S. P. (2018). The Wolbachia strain wAu provides highly efficient virus transmission blocking in Aedes aegypti. PLoS Pathog. 14:e1006815. doi: 10.1371/journal.ppat.1006815
Armbruster, P., Damsky, W. E., Giordano, R., Birungi, J., Munstermann, L. E., and Conn, J. E. (2003). Infection of new- and old-world Aedes albopictus (Diptera: culicidae) by the intracellular parasite Wolbachia: implications for host mitochondrial DNA evolution. J. Med. Entomol. 40, 356–360. doi: 10.1603/0022-2585-40.3.356
Baldo, L., Hotopp, J. C. D., Jolley, K. A., Bordenstein, S. R., Biber, S. A., Choudhury, R. R., et al. (2006). Multilocus sequence typing system for the endosymbiont Wolbachia pipientis. Appl. Environ. Microbiol. 72, 7098–7110. doi: 10.1128/AEM.00731-06
Baldo, L., Lo, N., and Werren, J. H. (2005). Mosaic nature of the Wolbachia surface protein. J. Bacteriol. 187, 5406–5418. doi: 10.1128/jb.187.15.5406-5418.2005
Baldo, L., and Werren, J. H. (2007). Revisiting Wolbachia supergroup typing based on WSP: spurious lineages and discordance with MLST. Curr. Microbiol. 55, 81–87. doi: 10.1007/s00284-007-0055-8
Bandi, C., Anderson, T. J. C., Genchi, C., and Blaxter, M. L. (1998). Phylogeny of Wolbachia in filarial nematodes. Proc. R. Soc. Lond. B Biol. Sci. 265, 2407–2413. doi: 10.1098/rspb.1998.0591
Beckmann, J. F., Ronau, J. A., and Hochstrasser, M. (2017). A Wolbachia deubiquitylating enzyme induces cytoplasmic incompatibility. Nat. Microbiol. 2:17007. doi: 10.1038/nmicrobiol.2017.7
Bian, G., Joshi, D., Dong, Y., Lu, P., Zhou, G., Pan, X. L., et al. (2013b). Wolbachia invades Anopheles stephensi populations and induces refractoriness to plasmodium infection. Science 340, 748–751. doi: 10.1126/science.1236192
Bian, G., Zhou, G., Lu, P., and Xi, Z. Y. (2013a). Replacing a native Wolbachia with a novel strain results in an increase in endosymbiont load and resistance to dengue virus in a mosquito vector. PLoS Negl. Trop. Dis. 7:e2250. doi: 10.1371/journal.pntd.0002250
Bossan, B., Koehncke, A., and Hammerstein, P. (2011). A new model and method for understanding Wolbachia-induced cytoplasmic incompatibility. PLoS One 6:e19757. doi: 10.1371/journal.pone.0019757
Bourtzis, K., Dobson, S. L., Xi, Z. Y., Rasgon, J. L., Calvitti, M., Moreira, L. A., et al. (2014). Harnessing mosquito-Wolbachia symbiosis for vector and disease control. Acta Trop. 132, S150–S163. doi: 10.1016/j.actatropica.2013.11.004
Brown, A. N., and Lloyd, V. K. (2015). Evidence for horizontal transfer of Wolbachia by a Drosophila mite. Exp. Appl. Acarol. 66, 301–311. doi: 10.1007/s10493-015-9918-z
Chrostek, E., Marialva, M. S. P., Esteves, S. S., Weinert, L. A., Martinez, J., Jiggins, F. M., et al. (2013). Wolbachia variants induce differential protection to viruses in Drosophila melanogaster: a phenotypic and phylogenomic analysis. PLoS Genet. 9:e1003896. doi: 10.1371/journal.pgen.1003896
Clark, M. E., Veneti, Z., Bourtzis, K., and Karr, T. L. (2002). The distribution and proliferation of the intracellular bacteria Wolbachia during spermatogenesis in Drosophila. Mech. Dev. 111, 3–15. doi: 10.1016/s0925-4773(01)00594-9
Dobson, S. L., Bourtzis, K., Braig, H. R., Jones, B. F., Zhou, W., Rousset, F., et al. (1999). Wolbachia infections are distributed throughout insect somatic and germ line tissues. Insect Biochem. Mol. Biol. 29, 153–160. doi: 10.1016/s0965-1748(98)00119-2
Fast, E. M., Toomey, M. E., Panaram, K., Desjardins, D., Kolaczyk, E. D., and Frydman, H. M. (2011). Wolbachia enhance Drosophila stem cell proliferation and target the germline stem cell niche. Science 334, 990–992. doi: 10.1126/science.1209609
Ferree, P. M., Frydman, H. M., Li, J. M., Cao, J., Wieschaus, E., and Sullivan, W. (2005). Wolbachia utilizes host microtubules and dynein for anterior localization in the Drosophila oocyte. PLoS Pathog. 1:e14. doi: 10.1371/journal.ppat.0010014
Ford, S. A., Allen, S. L., Ohm, J. R., Sigle, L. T., Sebastian, A., Albert, I., et al. (2019). Selection on Aedes aegypti alters Wolbachia-mediated dengue virus blocking and fitness. Nat. Microbiol. 4, 1832–1839. doi: 10.1038/s41564-019-0533-3
Frydman, H. M., Li, J. M., Robson, D. N., and Wieschaus, E. (2006). Somatic stem cell niche tropism in Wolbachia. Nature 441, 509–512. doi: 10.1038/nature04756
Gehrer, L., and Vorburger, C. (2012). Parasitoids as vectors of facultative bacterial endosymbionts in aphids. Biol. Lett. 8, 613–615. doi: 10.1098/rsbl.2012.0144
Gong, J. T., Li, Y. J., Li, T. P., Liang, Y., Hu, L., Zhang, D. J., et al. (2020). Stable introduction of plant-virus-inhibiting Wolbachia into planthoppers for rice protection. Curr. Biol. 30, 4837–4845. doi: 10.1016/j.cub.2020.09.033
Gong, J. T., Li, T. P., Wang, M. K., and Hong, X. Y. (2023). Wolbachia-based strategies for control of agricultural pests. Curr. Opin. Insect Sci. 57:101039. doi: 10.1016/j.cois.2023.101039
Goodacre, S. L., Martin, O. Y., Thomas, C. F. G., and Hewitt, G. M. (2006). Wolbachia and other endosymbiont infections in spiders. Mol. Ecol. 15, 517–527. doi: 10.1111/j.1365-294X.2005.02802.x
Goya, L. F., Lanteri, A. A., Confalonieri, V. A., and Rodriguero, M. S. (2022). New host-parasitoid interactions in Naupactus cervinus (Coleoptera, Curculionidae) raise the question of Wolbachia horizontal transmission. Symbiosis 86, 325–336. doi: 10.1007/s13199-022-00838-z
Guo, Y., Gong, J. T., Mo, P. W., Huang, H. J., and Hong, X. Y. (2019). Wolbachia localization during Laodelphax striatellus embryogenesis. J. Insect Physiol. 116, 125–133. doi: 10.1016/j.jinsphys.2019.05.006
Guo, Y., Guo, J. T., and Li, Y. F. (2022). Wolbachia wPip blocks Zika virus transovarial transmission in Aedes albopictus. Microbiol. Spectr. 10:e0263321. doi: 10.1128/spectrum.02633-21
Guo, Y., Hoffmann, A. A., Xu, X. Q., Mo, P. W., Huang, H. J., Gong, J. T., et al. (2018b). Vertical transmission of Wolbachia is associated with host vitellogenin in Laodelphax striatellus. Front. Microbiol. 9:2016. doi: 10.3389/fmicb.2018.02016
Guo, Y., Hoffmann, A. A., Xu, X. Q., Zhang, X., Huang, H. J., Ju, J. F., et al. (2018a). Wolbachia-induced apoptosis associated with increased fecundity in Laodelphax striatellus (Hemiptera: Delphacidae). Insect Mol. Biol. 27, 796–807. doi: 10.1111/imb.12518
Guo, Y., Khan, J., Zheng, X. Y., and Wu, Y. (2020). Wolbachia increase germ cell mitosis to enhance the fecundity of Laodelphax striatellus. Insect Biochem. Mol. Biol. 127:103471. doi: 10.1016/j.ibmb.2020.103471
Hedges, L. M., Brownlie, J. C., O'Neill, S. L., and Johnson, K. N. (2008). Wolbachia and virus protection in insects. Science 322:702. doi: 10.1126/science.1162418
Hertig, M., and Wolbach, S. B. (1924). Studies on rickettsia-like micro-organisms in insects. J. Med. Res. 44, 329–374.7.
Hibino, H. (1996). Biology and epidemiology of rice viruses. Annu. Rev. Phytopathol. 34, 249–274. doi: 10.1146/annurev.phyto.34.1.249
Hoshizaki, S., and Shimada, T. (1995). PCR-based detection of Wolbachia, cytoplasmic incompatibility microorganisms, infected in natural populations of Laodelphax striatellus (Homoptera: Delphacidae) in Central Japan: has the distribution of Wolbachia spread recently? Insect Mol. Biol. 4, 237–243. doi: 10.1111/j.1365-2583.1995.tb00029.x
Houck, M. A., Clark, J. B., Peterson, K. R., and Kidwell, M. G. (1991). Possible horizontal transfer of Drosophila genes by the mite Proctolaelaps regalis. Science 253, 1125–1128. doi: 10.1126/science.1653453
Huigens, M. E., de Almeida, R. P., Boons, P. A. H., Luck, R. F., and Stouthamer, R. (2004). Natural interspecific and intraspecific horizontal transfer of parthenogenesis-inducing Wolbachia in Trichogramma wasps. Proc. R. Soc. Lond. B Biol. Sci. 271, 509–515. doi: 10.1098/rspb.2003.2640
Ijichi, N., Kondo, N., Matsumoto, R., Shimada, M., Ishikawa, H., and Fukatsu, T. (2002). Internal spatiotemporal population dynamics of infection with three Wolbachia strains in the adzuki bean beetle, Callosobruchus chinensis (Coleoptera: Bruchidae). Appl. Environ. Microbiol. 68, 4074–4080. doi: 10.1128/AEM.68.8.4074-4080.2002
Jaenike, J., Polak, M., Fiskin, A., Helou, M., and Minhas, M. (2007). Interspecific transmission of endosymbiotic Spiroplasma by mites. Biol. Lett. 3, 23–25. doi: 10.1098/rsbl.2006.0577
Jiang, W., Zhu, J., Wu, Y., Li, L., Li, Y., Ge, C., et al. (2018). Influence of Wolbachia infection on mitochondrial DNA variation in the genus Polytremis (Lepidoptera: Hesperiidae). Mol. Phylogenet. Evol. 129, 158–170. doi: 10.1016/j.ympev.2018.08.001
Ju, J. F., Bing, X. L., Zhao, D. S., Guo, Y., Xi, Z. Y., Hoffmann, A. A., et al. (2020). Wolbachia supplement biotin and riboflavin to enhance reproduction in planthoppers. ISME J. 14, 676–687. doi: 10.1038/s41396-019-0559-9
Ju, J. F., Hoffmann, A. A., Zhang, Y. K., Duan, X. Z., Guo, Y., Gong, J. T., et al. (2017). Wolbachia-induced loss of male fertility is likely related to branch chain amino acid biosynthesis and iLvE in Laodelphax striatellus. Insect Biochem. Mol. Biol. 85, 11–20. doi: 10.1016/j.ibmb.2017.04.002
Kaur, R., Shropshire, J. D., Cross, K. L., Leigh, B., Mansueto, A. J., Stewart, V., et al. (2021). Living in the endosymbiotic world of Wolbachia: a centennial review. Cell Host Microbe 29, 879–893. doi: 10.1016/j.chom.2021.03.006
King, J. G., Souto-Maior, C., Sartori, L. M., Maciel-de-Freitas, R., and Gomes, M. G. M. (2018). Variation in Wolbachia effects on Aedes mosquitoes as a determinant of invasiveness and vectorial capacity. Nat. Commun. 9:1483. doi: 10.1038/s41467-018-03981-8
Kittayapong, P., Jamnongluk, W., Thipaksorn, A., Milne, J. R., and Sindhusake, C. (2003). Wolbachia infection complexity among insects in the tropical rice-field community. Mol. Ecol. 12, 1049–1060. doi: 10.1046/j.1365-294X.2003.01793.x
Kose, H., and Karr, T. L. (1995). Organization of Wolbachia pipientis in the Drosophila fertilized egg and embryo revealed by an anti-Wolbachia monoclonal antibody. Mech. Dev. 51, 275–288. doi: 10.1016/0925-4773(95)00372-x
Kremer, N., Voronin, D., Charif, D., Mavingui, P., Mollereau, B., and Vavre, F. (2009). Wolbachia interferes with ferritin expression and iron metabolism in insects. PLoS Pathog. 5:e1000630. doi: 10.1371/journal.ppat.1000630
Le Clec'h, W., Chevalier, F. D., Genty, L., Bertaux, J., Bouchon, D., and Sicard, M. (2013). Cannibalism and predation as paths for horizontal passage of Wolbachia between terrestrial isopods. PLoS One 8:e60232. doi: 10.1371/journal.pone.0060232
LePage, D. P., Metcalf, J. A., Bordenstein, S. R., On, J., Perlmutter, J. I., Shropshire, J. D., et al. (2017). Prophage WO genes recapitulate and enhance Wolbachia-induced cytoplasmic incompatibility. Nature 543, 243–247. doi: 10.1038/nature21391
Lequime, S., Paul, R. E., and Lambrechts, L. (2016). Determinants of arbovirus vertical transmission in mosquitoes. PLoS Pathog. 12:e1005548. doi: 10.1371/journal.ppat.1005548
Li, S. J., Ahmed, M. Z., Lv, N., Shi, P. Q., Wang, X. M., Huang, J. L., et al. (2017). Plant-mediated horizontal transmission of Wolbachia between whiteflies. ISME J. 11, 1019–1028. doi: 10.1038/ismej.2016.164
Li, F., Li, P., Hua, H., Hou, M., and Wang, F. (2020). Diversity, tissue localization, and infection pattern of bacterial symbionts of the white-backed planthopper, Sogatella furcifera (Hemiptera: Delphacidae). Microb. Ecol. 79, 720–730. doi: 10.1007/s00248-019-01433-4
Li, T. P., Zhou, C. Y., Wang, M. K., Zha, S. S., Chen, J., Bing, X. L., et al. (2022). Endosymbionts reduce microbiome diversity and modify host metabolism and fecundity in the planthopper Sogatella furcifera. Msystems 7:e0151621. doi: 10.1128/msystems.01516-21
Lin, M. Q., and Rikihisa, Y. (2003). Ehrlichia chaffeensis and Anaplasma phagocytophilum lack genes for lipid a biosynthesis and incorporate cholesterol for their survival. Infect. Immun. 71, 5324–5331. doi: 10.1128/iai.71.9.5324-5331.2003
Liu, L., Zhang, K. J., Rong, X., Li, Y. W., and Liu, H. (2019). Identification of Wolbachia-responsive miRNAs in the small brown planthopper, Laodelphax striatellus. Front. Physiol. 10:928. doi: 10.3389/fphys.2019.00928
Lo, N., Paraskevopoulos, C., Bourtzis, K., O'Neill, S., Werren, J., Bordenstein, S., et al. (2007). Taxonomic status of the intracellular bacterium Wolbachia pipientis. Int. J. Syst. Evol. Microbiol. 57, 654–657. doi: 10.1099/ijs.0.64515-0
Lu, P., Sun, Q., Fu, P., Li, K., Liang, X., and Xi, Z. Y. (2020). Wolbachia inhibits binding of dengue and Zika viruses to mosquito cells. Front. Microbiol. 11:1750. doi: 10.3389/fmicb.2020.01750
Martinez, J., Longdon, B., Bauer, S., Chan, Y. S., Miller, W. J., Bourtzis, K., et al. (2014). Symbionts commonly provide broad spectrum resistance to viruses in insects: a comparative analysis of Wolbachia strains. PLoS Pathog. 10:e1004369. doi: 10.1371/journal.ppat.1004369
Moreira, L. A., Iturbe-Ormaetxe, I., Jeffery, J. A., Lu, G., Pyke, A. T., Hedges, L. M., et al. (2009). A Wolbachia symbiont in Aedes aegypti limits infection with dengue, chikungunya, and plasmodium. Cells 139, 1268–1278. doi: 10.1016/j.cell.2009.11.042
Moretti, R., Yen, P. S., Houe, V., Lampazzi, E., Desiderio, A., Failloux, A. B., et al. (2018). Combining Wolbachia-induced sterility and virus protection to fight Aedes albopictus-borne viruses. PLoS Negl. Trop. Dis. 12:e0006626. doi: 10.1371/journal.pntd.0006626
Mousson, L., Zouache, K., Arias-Goeta, C., Raquin, V., Mavingui, P., and Failloux, A. B. (2012). The native Wolbachia symbionts limit transmission of dengue virus in Aedes albopictus. PLoS Negl. Trop. Dis. 6:e1989. doi: 10.1371/journal.pntd.0001989
Nakamura, Y., Yukuhiro, F., Matsumura, M., and Noda, H. (2012). Cytoplasmic incompatibility involving Cardinium and Wolbachia in the white-backed planthopper Sogatella furcifera (Hemiptera: Delphacidae). Appl. Entomol. Zool. 47, 273–283. doi: 10.1007/s13355-012-0120-z
Nevalainen, L. B., Layton, E. M., and Newton, I. L. G. (2023). Wolbachia promotes its own uptake by host cells. Infect. Immun. 91:e0055722. doi: 10.1128/iai.00557-22
Newton, I. L., Savytskyy, O., and Sheehan, K. B. (2015). Wolbachia utilize host actin for efficient maternal transmission in Drosophila melanogaster. PLoS Pathog. 11:e1004798. doi: 10.1371/journal.ppat.1004798
Noda, H. (1984b). Cytoplasmic incompatibility in allopatric field populations of the small brown planthopper, Laodelphax striatellus. Japan. Entomol. Exp. Appl. 35, 263–267.
Osborne, S. E., Iturbe-Ormaetxe, I., Brownlie, J. C., O'Neill, S. L., and Johnson, K. N. (2012). Antiviral protection and the importance of Wolbachia density and tissue tropism in Drosophila simulans. Appl. Environ. Microbiol. 78, 6922–6929. doi: 10.1128/aem.01727-12
Poinsot, D., Charlat, S., and Mercot, H. (2003). On the mechanism of Wolbachia-induced cytoplasmic incompatibility: confronting the models with the facts. BioEssays 25, 259–265. doi: 10.1002/bies.10234
Qu, L. Y., Lou, Y. H., Fan, H. W., Ye, Y. X., Huang, H. J., Hu, M. Q., et al. (2013). Two endosymbiotic bacteria, Wolbachia and Arsenophonus, in the brown planthopper Nilaparvata lugens. Symbiosis 61, 47–53. doi: 10.1007/s13199-013-0256-9
Rainey, S. M., Shah, P., Kohl, A., and Dietrich, I. (2014). Understanding the Wolbachia-mediated inhibition of arboviruses in mosquitoes: progress and challenges. J. Gen. Virol. 95, 517–530. doi: 10.1099/vir.0.057422-0
Rances, E., Ye, Y. H., Woolfit, M., McGraw, E. A., and O'Neill, S. L. (2012). The relative importance of innate immune priming in Wolbachia-mediated dengue interference. PLoS Pathog. 8:e1002548. doi: 10.1371/journal.ppat.1002548
Rosen, L. (1988). Further observations on the mechanism of vertical transmission of flaviviruses by Aedes mosquitoes. Am. J. Trop. Med. Hyg. 39, 123–126. doi: 10.4269/ajtmh.1988.39.123
Ross, P. A., Turelli, M., and Hoffmann, A. A. (2019). Evolutionary ecology of Wolbachia releases for disease control. Annu. Rev. Genet. 53, 93–116. doi: 10.1146/annurev-genet-112618-043609
Rousset, F., Bouchon, D., Pintureau, B., Juchault, P., and Solignac, M. (1992). Wolbachia endosymbionts responsible for various alterations of sexuality in arthropods. Proc. Natl. Acad. Sci. U. S. A. 250, 91–98. doi: 10.1098/rspb.1992.0135
Sanaei, E., Albery, G. F., Yeoh, Y. K., Lin, Y. P., Cook, L. G., and Engelstadter, J. (2023). Host phylogeny and ecological associations best explain Wolbachia host shifts in scale insects. Mol. Ecol. 32, 2351–2363. doi: 10.1111/mec.16883
Sarao, P. S., Sahi, G. K., Neelam, K., Mangat, G. S., Patra, B. C., and Singh, K. (2016). Donors for resistance to brown planthopper Nilaparvata lugens (Stal) from wild rice species. Rice Sci. 23, 219–224. doi: 10.1016/j.rsci.2016.06.005
Scholz, M., Albanese, D., Tuohy, K., Donati, C., Segata, N., and Rota-Stabelli, O. (2020). Large scale genome reconstructions illuminate Wolbachia evolution. Nat. Commun. 11:5235. doi: 10.1038/s41467-020-19016-0
Schuler, H., Bertheau, C., Egan, S. P., Feder, J. L., Riegler, M., Schlick-Steiner, B. C., et al. (2013). Evidence for a recent horizontal transmission and spatial spread of Wolbachia from endemic Rhagoletis cerasi (Diptera: Tephritidae) to invasive Rhagoletis cingulata in Europe. Mol. Ecol. 22, 4101–4111. doi: 10.1111/mec.12362
Schultz, M. J., Tan, A. L., Gray, C. N., Isern, S., Michael, S. F., Frydman, H. M., et al. (2018). Wolbachia wStri blocks Zika virus growth at two independent stages of viral replication. mBio 9, e00738–e00718. doi: 10.1128/mBio.00738-18
Serbina, L. S., Gajski, D., Malenovsky, I., Corretto, E., Schuler, H., and Dittmer, J. (2022). Wolbachia infection dynamics in a natural population of the pear psyllid Cacopsylla pyri (Hemiptera: Psylloidea) across its seasonal generations. Sci. Rep. 12:16502. doi: 10.1038/s41598-022-20968-0
Shaikevich, E., Bogacheva, A., Rakova, V., Ganushkina, L., and Ilinsky, Y. (2019). Wolbachia symbionts in mosquitoes: intra- and intersupergroup recombinations, horizontal transmission and evolution. Mol. Phylogenet. Evol. 134, 24–34. doi: 10.1016/j.ympev.2019.01.020
Shaikevich, E., and Romanov, D. (2023). Symbiotic Wolbachia bacteria in coccinellid parasitoids: genetic diversity, horizontal transfer, and recombination. Int. J. Microbiol. 26, 269–280. doi: 10.1007/s10123-022-00295-0
Sintupachee, S., Milne, J. R., Poonchaisri, S., Baimai, V., and Kittayapong, P. (2006). Closely related Wolbachia strains within the pumpkin arthropod community and the potential for horizontal transmission via the plant. Microb. Ecol. 51, 294–301. doi: 10.1007/s00248-006-9036-x
Su, Q. H., Hu, G. W., Yun, Y. L., and Peng, Y. (2019). Horizontal transmission of Wolbachia in Hylyphantes graminicola is more likely via intraspecies than interspecies transfer. Symbiosis 79, 123–128. doi: 10.1007/s13199-019-00623-5
Sullivan, W. (2020). Vector control: Wolbachia expands its protective reach from humans to plants. Curr. Biol. 30, R1489–R1491. doi: 10.1016/j.cub.2020.11.005
Swiatoniowska, M., Ogorzalek, A., Golas, A., Michalik, A., and Szklarzewicz, T. (2013). Ultrastructure, distribution, and transovarial transmission of symbiotic microorganisms in Nysius ericae and Nithecus jacobaeae (Heteroptera: Lygaeidae: Orsillinae). Protoplasma 250, 325–332. doi: 10.1007/s00709-012-0416-4
Szklarzewicz, T., Jankowska, W., Łukasiewicz, K., and Szymańska, B. (2007). Structure of the ovaries and oogenesis in Cixius nervosus (Cixiidae), Javesella pellucida and Conomelus anceps (Delphacidae) (Insecta, Hemiptera, Fulgoromorpha). Arthropod Struct. Dev. 36, 199–207. doi: 10.1016/j.asd.2006.09.001
Szklarzewicz, T., Kalandyk-Kolodziejczyk, M., Kot, M., and Michalik, A. (2013). Ovary structure and transovarial transmission of endosymbiotic microorganisms in Marchalina hellenica (Insecta, Hemiptera, Coccomorpha: Marchalinidae). Acta Zool. 94, 184–192. doi: 10.1111/j.1463-6395.2011.00538.x
Tchankouo-Nguetcheu, S., Khun, H., Pincet, L., Roux, P., Bahut, M., Huerre, M., et al. (2010). Differential protein modulation in midguts of Aedes aegypti infected with chikungunya and dengue 2 viruses. PLoS One 5:e13149. doi: 10.1371/journal.pone.0013149
Teixeira, L., Ferreira, A., and Ashburner, M. (2008). The bacterial symbiont Wolbachia induces resistance to RNA viral infections in Drosophila melanogaster. PLoS Biol. 6, 2753–2763. doi: 10.1371/journal.pbio.1000002
Toomey, M. E., Panaram, K., Fast, E. M., Beatty, C., and Frydman, H. M. (2013). Evolutionarily conserved Wolbachia-encoded factors control pattern of stem-cell niche tropism in Drosophila ovaries and favor infection. Proc. Natl. Acad. Sci. U. S. A. 110, 10788–10793. doi: 10.1073/pnas.1301524110
Tram, U., and Sullivan, W. (2002). Role of delayed nuclear envelope breakdown and mitosis in Wolbachia-induced cytoplasmic incompatibility. Science 296, 1124–1126. doi: 10.1126/science.1070536
Turelli, M., Cooper, B. S., Richardson, K. M., Ginsberg, P. S., Peckenpaugh, B., Antelope, C. X., et al. (2018). Rapid global spread of wRi-like Wolbachia across multiple Drosophila. Curr. Biol. 28, 963–971. doi: 10.1016/j.cub.2018.02.015
Walker, T., Johnson, P., Moreira, L., Iturbe-Ormaetxe, I., Frentiu, F., McMeniman, C., et al. (2011). The wMel Wolbachia strain blocks dengue and invades caged Aedes aegypti populations. Nature 476:450. doi: 10.1038/nature10355
Wang, Z. Y., Deng, C., Yun, Y. L., Jian, C., and Peng, Y. (2010). Molecular detection and the phylogenetics of Wolbachia in Chinese spiders (Araneae). J. Arachnol. 38, 237–241. doi: 10.1636/joa_b09-69.1
Wang, G. H., Jia, L. Y., Xiao, J. H., and Huang, D. W. (2016). Discovery of a new Wolbachia supergroup in cave spider species and the lateral transfer of phage WO among distant hosts. Infect. Genet. Evol. 41, 1–7. doi: 10.1016/j.meegid.2016.03.015
Weinert, L. A., Araujo-Jnr, E. V., Ahmed, M. Z., and Welch, J. J. (2015). The incidence of bacterial endosymbionts in terrestrial arthropods. Proc. R. Soc. Lond. B Biol. Sci. 282:20150249. doi: 10.1098/rspb.2015.0249
Werren, J. H. (1997). Biology of Wolbachia. Annu. Rev. Entomol. 42, 587–609. doi: 10.1146/annurev.ento.42.1.587
Werren, J. H., Baldo, L., and Clark, M. E. (2008). Wolbachia: master manipulators of invertebrate biology. Nat. Rev. Microbiol. 6, 741–751. doi: 10.1038/nrmicro1969
Werren, J. H., and Bartos, J. D. (2001). Recombination in Wolbachia. Curr. Biol. 11, 431–435. doi: 10.1016/s0960-9822(01)00101-4
Werren, J. H., Zhang, W., and Guo, L. R. (1995). Evolution and phylogeny of Wolbachia: reproductive parasites of arthropods. Proc. R. Soc. Lond. B Biol. Sci. 261, 55–63. doi: 10.1098/rspb.1995.0117
Xi, Z. Y., Dean, J. L., Khoo, C., and Dobson, S. L. (2005). Generation of a novel Wolbachia infection in Aedes albopictus (Asian tiger mosquito) via embryonic microinjection. Insect Biochem. Mol. Biol. 35, 903–910. doi: 10.1016/j.ibmb.2005.03.015
Xi, Z. Y., Khoo, C. C. H., and Dobson, S. L. (2006). Interspecific transfer of Wolbachia into the mosquito disease vector Aedes albopictus. Proc. R. Soc. Lond. B Biol. Sci. 273, 1317–1322. doi: 10.1098/rspb.2005.3405
Xiao, Y., Chen, H., Wang, H., Zhang, M., Chen, X., Berk, J. M., et al. (2021). Structural and mechanistic insights into the complexes formed by Wolbachia cytoplasmic incompatibility factors. Proc. Natl. Acad. Sci. U. S. A. 118:e2107699118. doi: 10.1073/pnas.2107699118
Yang, L., and Zhang, W. (2016). Genetic and biochemical mechanisms of rice resistance to planthopper. Plant Cell Rep. 35, 1559–1572. doi: 10.1007/s00299-016-1962-6
Yen, J. H., and Barr, A. R. (1971). New hypothesis of the cause of cytoplasmic incompatibility in Culex pipiens L. Nature 232, 657–658. doi: 10.1038/232657a0
Zhang, Y., Cai, T., Ren, Z., Liu, Y., Yuan, M., Cai, Y., et al. (2021). Decline in symbiont-dependent host detoxification metabolism contributes to increased insecticide susceptibility of insects under high temperature. ISME J. 15, 3693–3703. doi: 10.1038/s41396-021-01046-1
Zhang, K. J., Han, X., and Hong, X. Y. (2013). Various infection status and molecular evidence for horizontal transmission and recombination of Wolbachia and Cardinium among rice planthoppers and related species. Insect Sci. 20, 329–344. doi: 10.1111/j.1744-7917.2012.01537.x
Zhao, Z., Zhu, J., Hoffmann, A. A., Cao, L., Shen, L., Fang, J., et al. (2021). Horizontal transmission and recombination of Wolbachia in the butterfly tribe Aeromachini Tutt, 1906 (Lepidoptera: Hesperiidae). G3-genes genomes. Genetics 11:jkab221. doi: 10.1093/g3journal/jkab221
Zheng, X. Y., Zhang, D. J., Li, Y. J., Yang, C., Wu, Y., Liang, X., et al. (2019). Incompatible and sterile insect techniques combined eliminate mosquitoes. Nature 572, 56–61. doi: 10.1038/s41586-019-1407-9
Keywords: Wolbachia , mosquitoes, planthoppers, transmission, cytoplasmic incompatibility, pathogen inhibition
Citation: Guo Y, Shao J, Wu Y and Li Y (2023) Using Wolbachia to control rice planthopper populations: progress and challenges. Front. Microbiol. 14:1244239. doi: 10.3389/fmicb.2023.1244239
Received: 22 June 2023; Accepted: 24 August 2023;
Published: 14 September 2023.
Edited by:
Daisuke Kageyama, National Agriculture and Food Research Organization (NARO), JapanReviewed by:
Sonam Popli, University of Toledo, United StatesCopyright © 2023 Guo, Shao, Wu and Li. This is an open-access article distributed under the terms of the Creative Commons Attribution License (CC BY). The use, distribution or reproduction in other forums is permitted, provided the original author(s) and the copyright owner(s) are credited and that the original publication in this journal is cited, in accordance with accepted academic practice. No use, distribution or reproduction is permitted which does not comply with these terms.
*Correspondence: Yifeng Li, eWlmZW5nbGlAZ2RhYXMuY24=
Disclaimer: All claims expressed in this article are solely those of the authors and do not necessarily represent those of their affiliated organizations, or those of the publisher, the editors and the reviewers. Any product that may be evaluated in this article or claim that may be made by its manufacturer is not guaranteed or endorsed by the publisher.
Research integrity at Frontiers
Learn more about the work of our research integrity team to safeguard the quality of each article we publish.