- 1College of Animal Science and Technology, Northeast Agricultural University, Harbin, China
- 2Beijing Sunlon Livestock Development Company Limited, Beijing, China
- 3College of Electrical Engineering and Information, Northeast Agricultural University, Harbin, China
- 4College of Animal Science and Technology, Heilongjiang Bayi Agricultural University, Daqing, China
- 5Key Laboratory of Low-carbon Green Agriculture in Northeastern China of Ministry of Agriculture and Rural Affairs, Daqing, China
This study used Silibinin as an additive to conduct fermentation experiments, wherein its effects on rumen gas production, fermentation, metabolites, and microbiome were analyzed in vitro. The silibinin inclusion level were 0 g/L (control group), 0.075 g/L, 0.15 g/L, 0.30 g/L, and 0.60 g/L (experimental group). Fermentation parameters, total gas production, carbon dioxide (CO2), methane (CH4), hydrogen (H2), and their percentages were determined. Further analysis of the rumen microbiome’s relative abundance and α/β diversity was performed on the Illumina NovaSeq sequencing platform. Qualitative and quantitative metabolomics analyses were performed to analyze the differential metabolites and metabolic pathways based on non-targeted metabolomics. The result indicated that with an increasing dose of silibinin, there was a linear reduction in total gas production, CO2, CH4, H2 and their respective percentages, and the acetic acid to propionic acid ratio. Concurrent with a linear increase in pH, when silibinin was added at 0.15 g/L and above, the total volatile fatty acid concentration decreased, the acetic acid molar ratio decreased, the propionic acid molar ratio increased, and dry matter digestibility decreased. At the same time, the relative abundance of Prevotella, Isotricha, Ophryoscolex, unclassified_Rotifera, Methanosphaera, Orpinomyces, and Neocallimastix in the rumen decreased after adding 0.60 g/L of silibinin. Simultaneously, the relative abundance of Succiniclasticum, NK4A214_group, Candidatus_Saccharimonas, and unclassified_Lachnospiraceae increased, altering the rumen species composition, community, and structure. Furthermore, it upregulated the ruminal metabolites, such as 2-Phenylacetamide, Phlorizin, Dalspinin, N6-(1,2-Dicarboxyethyl)-AMP, 5,6,7,8-Tetrahydromethanopterin, Flavin mononucleotide adenine dinucleotide reduced form (FMNH), Pyridoxine 5′-phosphate, Silibinin, and Beta-D-Fructose 6-phosphate, affecting phenylalanine metabolism, flavonoid biosynthesis, and folate biosynthesis pathways. In summary, adding silibinin can alter the rumen fermentation parameters and mitigate enteric methane production by regulating rumen microbiota and metabolites, which is important for developing novel rumen methane inhibitors.
1. Introduction
As the global population continues to rise, more than a third of the protein demand for humans, such as meat and milk, depends on animal agriculture, thus resulting in livestock production intensification and consequently causing the agricultural sector to be a major source of greenhouse gas (GHG) generation (Sakadevan and Nguyen, 2017). The main greenhouse gas emitted from the animal sector is carbon dioxide (CO2), methane (CH4), and nitrous oxide (N2O). Controlling GHG emissions is key to stabilizing the global climate system because its excess in the atmosphere leads to frequent natural disasters, increases the spread of pathogenic diseases, and poses a risk to the health and survival of humans and animals alike. The contribution of methane to global warming is more prominent than N2O because it constitutes about half of the entire greenhouse gas in the atmosphere (Stocker et al., 2013; Myhre et al., 2014; Haque, 2018), with most of the methane emanating from ruminants. Therefore, reducing CH4 emissions from enteric fermentation in ruminants is an effective way to mitigate greenhouse gas emissions in the livestock industry (Vaghar Seyedin et al., 2022).
CH4 produced by ruminants is mainly from the enteric fermentation process initiated by rumen microorganisms through the CO2 reduction pathway under the action of methanogenic bacteria while reducing the partial buildup of rumen H2, maintaining the stability of rumen pH and ensuring normal rumen fermentation and the methane produced is released into the atmosphere through feces or burp. Therefore, it is crucial to mitigate CH4 by regulating the rumen microbiota. Research has found that adding ionic carriers such as monensin to the diet could alter the rumen microbiome, inhibit the production of available substrates by methanogenic bacteria, and reduce CH4 production (Marques and Cooke, 2021). However, with longer feeding times, ion carriers could develop resistance in some bacteria (Vendramini et al., 2016) and have therefore been banned in many countries. The addition of methanogenic inhibitors such as nitro derivatives, on the other hand, though could reduce CH4 production via rumen microbiome alteration, but there are very few reports on their safety assessment (Teng and Kim, 2021).
Plant extracts are gaining popularity due to their safe and non-toxic benefits. Literature has reported that plant extracts have colony-modulating effects (Jubair et al., 2021; Vaou et al., 2021) and that flavonoids extracted from plants not only reduce the number of methanogenic bacteria in the rumen but also reduce the number of protozoa attached to the methanogens to reduce CH4 production (Ku-Vera et al., 2020). Seradj et al. (2014) observed that adding flavonoid extract inhibited hydrogenotrophic methanogenic archaea and Methanosarcina in the rumen. Likewise, the flavonoid-rich extract obtained from Brazilian spinach (Alternanthera sissoo; BS) was found to decrease CH4 production and protozoa counts while elevating total volatile fatty acid and propionic acid levels during in vitro fermentation (Sommai et al., 2021).
Silibinin (C25H22O10), an active ingredient isolated from the fruit of Silybum marianum (chrysanthemum plant), is single in composition and chemistry as a dihydro flavonol compound compared to some other flavonoids extracted from plants. According to recent scientific investigations, gram-positive bacteria are more susceptible to the inhibitory effects of silibinin as compared to their gram-negative counterparts (Janssen and Kirs, 2008; Miller, 2015; Mahmoudi-Rad et al., 2022). Therefore, the potential of silibinin to effectively regulate the activity and population of gram-positive bacteria associated with methane synthesis is critical for methane regulation. Additionally, the mechanism by which silibinin regulates gram-positive bacteria is mainly by inhibiting protein and RNA synthesis (Lee et al., 2003). Some reports have shown that silibinin has a modulating effect on the activity of Staphylococcus aureus (Cai et al., 2018), Bacillus cereus (Mahmoudi-Rad et al., 2022), and Prevotella intermedia (Lee et al., 2012). Also, its anti-inflammatory effects are exerted by regulating the microbial flora (Rakelly de Oliveira et al., 2015).
Even though flavonoids have been shown to have regulatory effects on rumen microflora and amino acids metabolic pathways such as tyrosine metabolism and proline metabolism (Yu et al., 2023), there are no studies on the use of silibinin as an additive in dairy cows. Based on the above, this study hypothesized that silibinin could affect rumen fermentation by regulating rumen microbiota and metabolites, thereby reducing methane production. Therefore, using in vitro fermentation method, we assessed rumen fermentation characteristics and gas production, analyzed the relative abundance and diversity of the rumen microbiome, and revealed the metabolites and metabolic pathways involved. This experiment provides a sufficient theoretical basis and data support for the application of silibinin in regulating ruminal methanogenesis in ruminants.
2. Materials and methods
2.1. Animals, diet, and experimental design
The animal use protocol was approved following the Animal Care and Use Committee of Northeast Agricultural University (protocol number: NEAUEC20230268). Three lactating Chinese Holstein cows (day in milk = 120 ± 11, milk yield = 27.6 ± 3.3 kg) were housed individually in a tethered barn stall, and water was made available freely. All cows were fed the same amount of total mixed ration (TMR) and milked twice daily at 0630 and 1830. The ingredients and chemical composition of the experimental diet are indicated in Table 1.
The anaerobic culture techniques and experimental procedures were similar, as described by Xin et al. (2021). Rumen fluid, sampled from the three fistulated cows before morning feeding, was flushed with O2-free CO2 in a vacuum flask, sieved through a four-layered cheesecloth before mixing with pre-warmed (39°C) buffer, and prepared according to (Soliva and Hess, 2007), at a ratio of 1:2 (buffer: ruminal fluid, v:v) under a continual CO2 flow to maintain an anaerobic environment. After mixing, 150 mL was transferred to a 200 mL glass bottle containing 2 g fermentation TMR substrates. The silibinin, purchased from Daxing’anling Livorcom Biotechnology Co., Ltd. China (purity of silibinin: 98%), with different qualities, was added to the rumen fluid to make 0.00, 0.075, 0.15, 0.30, and 0.60 g/L final concentrations; the blank control glass bottle only contained mixed abomasum fluid. Silicone stoppers with gas-tight collection bags were used to seal the sample bottles (ten replicates per treatment) before incubating them in a shaking water bath at 39°C and 40–50 rpm for 24 h. These incubations were performed in three separate runs over three days.
2.2. Total, hydrogen, carbon dioxide and methane gas production, and rumen fermentation analysis
During fermentation, the total gas produced was collected using gas collection bags. Each gas collection bag was connected to the top of the fermentation bottle via a rubber tube, and after ensuring that the entire device was airtight, incubation was carried out. At the end of the incubation period, the volume of the gas samples was measured using a syringe. Subsequently, a 0.5-mL subsample of the gas was analyzed for its hydrogen, carbon dioxide, and methane content using a Gas chromatograph (GC-8A; Shimadzu Co. Ltd., Tokyo, Japan) with a molecular sieve 13×, 45- to 60-mesh column (2.0 mm × 3.2 mm × 2.0 mm, stainless steel), and thermal conductivity detector. The temperature was set to 60°C, injector and thermal conductivity detector temperatures were set at 120°C, and flame-ionization detector temperature was set at 200°C. The carrier gas (N2) flow rate was 50 mL/min, in line with the procedure outlined by Kim et al. (2014). The pH level of the culture fluid was then measured with a pH meter (Sartorius basic pH meter, Göttingen, Germany). The rumen culture liquor was filtered through a 4-layered cheesecloth and stored at −20°C with 2 mL metaphosphoric acid (25%, wt/vol) per 10 mL before determining the VFA and ammonia-N levels. The VFA concentration was determined using gas chromatography (GC-8A; Shimadzu Corp., Kyoto, Japan) as described by Hu et al. (2005). Meanwhile, ammonia-N levels were determined using the phenol-hypochlorite method (Broderick and Kang, 1980).
2.3. Dry matter digestibility analysis
2 g of TMR sample was weighed into the fiber bag as a fermentation substrate. Ruminal fluid and buffer were mixed in a ratio of 1:2 as described above and added to the glass flask before placing it in the fiber bag. Air bubbles in the glass flasks were removed before sealing and then incubated in a constant temperature water bath at 39°C with shaking. After continuous fermentation for 24 h, the fiber bag was removed, rinsed with distilled water three times, dried for 48 h at 55°C, and then cooled in a desiccator (Kim et al., 2019). The sample was then weighed and used to determine the fermentation substrate’s in vitro dry matter digestibility (IVDMD), according to Wang et al. (2017).
IVDMD (%) = (DM content before fermentation – DM content after fermentation)/ DM content before fermentation × 100%.
2.4. DNA extraction, sequencing, and diversity analysis
At the end of the incubation, 20 mL of culture liquor with a maximum dose of 0.60 g/L of silibinin was split into two equal portions before storing at −80°C for 16S amplicon sequencing and untargeted metabolomics analysis.
The procedure involved the extraction of total DNA from the culture liquor using QIAamp Fast DNA stool Mini Kit (Qiagen, Cat# 51604) followed by the amplification of PCR conducted with barcoded specific bacteria primers that targeted the variable region 3–4 (V3-V4) of the 16S rRNA gene: forward primer 338F: 5′- ACTCCTACGGGAGGCAGCA -3′ and reverse primer 806R: 5′- GGACTACHVGGGTWTCTAAT -3′ (Wang et al., 2023). The V3-V4 region of the 16S rRNA gene for archaeal analysis was amplified using primers F341 (5′- GYGCASCAGKCGMGAAW -3′) and R806 (5′- GGACTACVSGGGTATCTAAT -3′). The protozoa analysis involved amplifying the V3-V4 region of the 18S rRNA gene using TAReuk454FWD1 F (5′- CCAGCASCYGCGGTAATTCC -3′) and TAReukREV3 R (5′- ACTTTCGTTCTTGATYRA -3′) primers. For fungi analysis, the ITS region of the 16S rRNA gene for fungi was amplified using primers F (CTTGGTCATTTAGAGGAAGTAA) and R (GCTGCGTTCTTCATCGATGC). According to standard protocols, sequencing libraries and paired-end sequencing was constructed on an Illumina NovaSeq6000 platform at Biomarker Technologies Co, Ltd. (Beijing, China). Following Yang et al. (2020), paired-end reads were merged using FLASH v1.2.7, and tags with over six mismatches were ignored. Trimmomatic (Sheng et al., 2019) was used to identify merged tags having an average quality score of <20 in a 50 bp sliding window, and tags shorter than 350 bps were eliminated. Using USEARCH, potential chimeras were eliminated, and the denoised sequences were grouped into operational taxonomic units (OTUs) with 97% similarity (version 10.0). All OTUs were given a taxonomy via a QIIME search against the Silva databases (Release 128).
The microbiome was compared as beta diversity using partial least squares discriminant analysis (PLS-DA). Taxa abundance at the genus level was statistically compared between the control and treatment groups for exposure to silibinin and the ruminal microbiome abundance.
2.5. Non-targeted metabolomics analysis
For quality control purposes, 10 μL per sample of the prepared rumen fluid was pooled. Metabolomics analysis was conducted using a Waters Acquity I-Class PLUS ultra-high performance liquid tandem and Waters Xevo G2-XS QTOF high-resolution mass spectrometer with a Waters Acquity UPLC HSS T3 (1.8um, 2.1 × 100 mm) column as outlined by Zhang et al. (2021). The injection volume for both positive and negative modes was set at 1 μL, with mobile phase A consisting of 0.1% formate aqueous solution and mobile phase B containing 0.1% formate acetonitrile.
After software acquisition control using MassLynx V4.2 (Waters), the primary and secondary mass spectrometry data were extracted using a Waters Xevo G2-XS QTOF high-resolution mass spectrometer in MSe mode. Dual-channel data acquisition was performed, alternating between a low collision energy of 2 V and a high collision energy of 10–40 V per cycle with a scanning frequency of 0.2 s for a mass spectrum, as described by Feng et al. (2022). The ESI ion source utilized a capillary voltage of 2,000 V (positive ion mode) or −1,500 V (negative ion mode), a 30 V cone voltage, a 150°C ion source temperature, 500°C desolvent gas temperature, 50 L/ h backflush gas flow rate, and 800 L/h desolventizing gas flow rate. Data processing operations such as peak extraction, peak alignment, and identification were performed using the online METLIN database and Biomark’s custom identification library with theoretical fragment identification and mass deviation calculations under 100 ppm, all utilizing Progenesis QI software.
The positive and negative data were combined, and the dependability of the model was assessed through 200 permutations after normalizing the original peak area data using principal component analysis (PCA) and orthogonal projections to latent structures-discriminant analysis (OPLS-DA) analysis. Following the grouping information, the difference multiples were calculated and compared. Compounds identified were classified, and pathway information was searched against KEGG databases. Additionally, a T-test was utilized to determine each compound’s differential significance p value, with multiple cross-validations used to calculate the OPLS-DA model’s VIP value. The differential metabolites were then filtered at FC > 1 or < −1, p value <0.05, and VIP > 1 using a technique that integrates the difference multiple, p value, and VIP value of the OPLS-DA model. A hypergeometric distribution test was conducted to determine different metabolites for KEGG pathway enrichment significance. Volcano plots were generated using ggplot2 in R language to filter the metabolites of interest based on their log2 (Fold change) and - log10 (p-value).
2.6. Statistical analysis
All data from the experiment were analyzed using SAS software (Version 9.4; SAS Institute Inc., Cary, NC). Histograms and formal statistical tests included in the UNIVARIATE procedure of SAS were used to test for normal distribution and homogeneity of variance of gas production and concentration and rumen fermentation parameters. Data were then analyzed using a one-way ANOVA, and orthogonal polynomial contrasts were utilized to analyze the linear, quadratic, and cubic effects of silibinin under different concentrations. Relative abundances (%) of rumen microbiome in vitro after 24 h exposure to silibinin were analyzed using the non-parametric Kruskal-Wallis Test, and results were presented as mean with standard deviation. Significantly different means were declared when p ≤ 0.05, while trends were defined at 0.05 < p ≤ 0.10.
3. Results
3.1. Effect of silibinin on in vitro gas production
To assess the impact of silibinin addition on rumen fermentation, we employed an in vitro gas production technique to measure the corresponding fermentation products. After 24 h of in vitro fermentation, total gas production and CH4, CO2, and H2 production and ratio showed linear decreases (p < 0.001) with the increase in the amount of silibinin additive. Total gas production and CH4, CO2, and H2 production and ratios decreased to the lowest in the high dose 0.60 g/L group (Table 2).
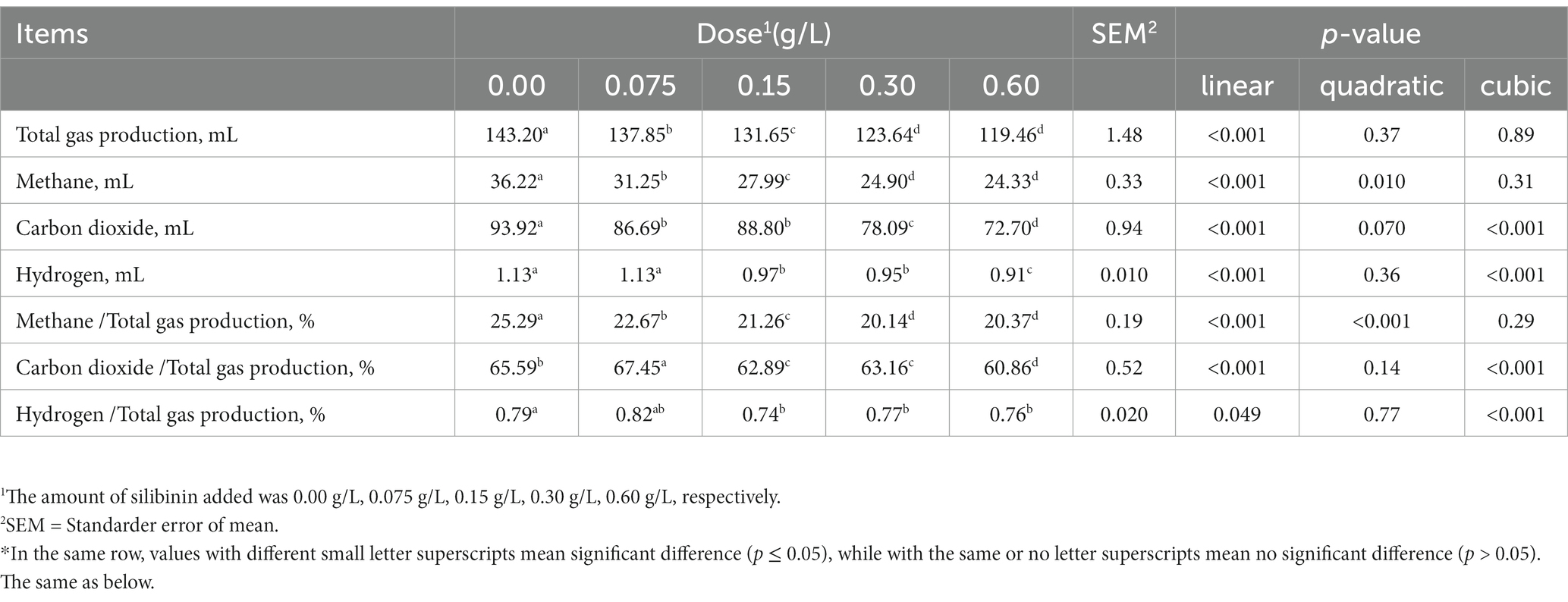
Table 2. Effect of adding different doses of silibinin on gas production after 24 h of in vitro fermentation.
3.2. Effect of silibinin on fermentation characteristics
With the increasing amount of silibinin additive, rumen pH and the molar ratio of propionic acid increased linearly (p < 0.001), and total volatile fatty acid concentration, acetic to propionic acid ratio, and dry matter digestibility decreased linearly (p < 0.001). With the addition of 0.075 g/L silibinin, NH3-N, total volatile fatty acid concentration, the molar ratio of acetic acid, the molar ratio of butyric acid, and dry matter digestibility were not statistically significant differences from the untreated group (Table 3). The result showed that adding low doses of silibinin did not affect rumen fermentation while reducing CH4 production.
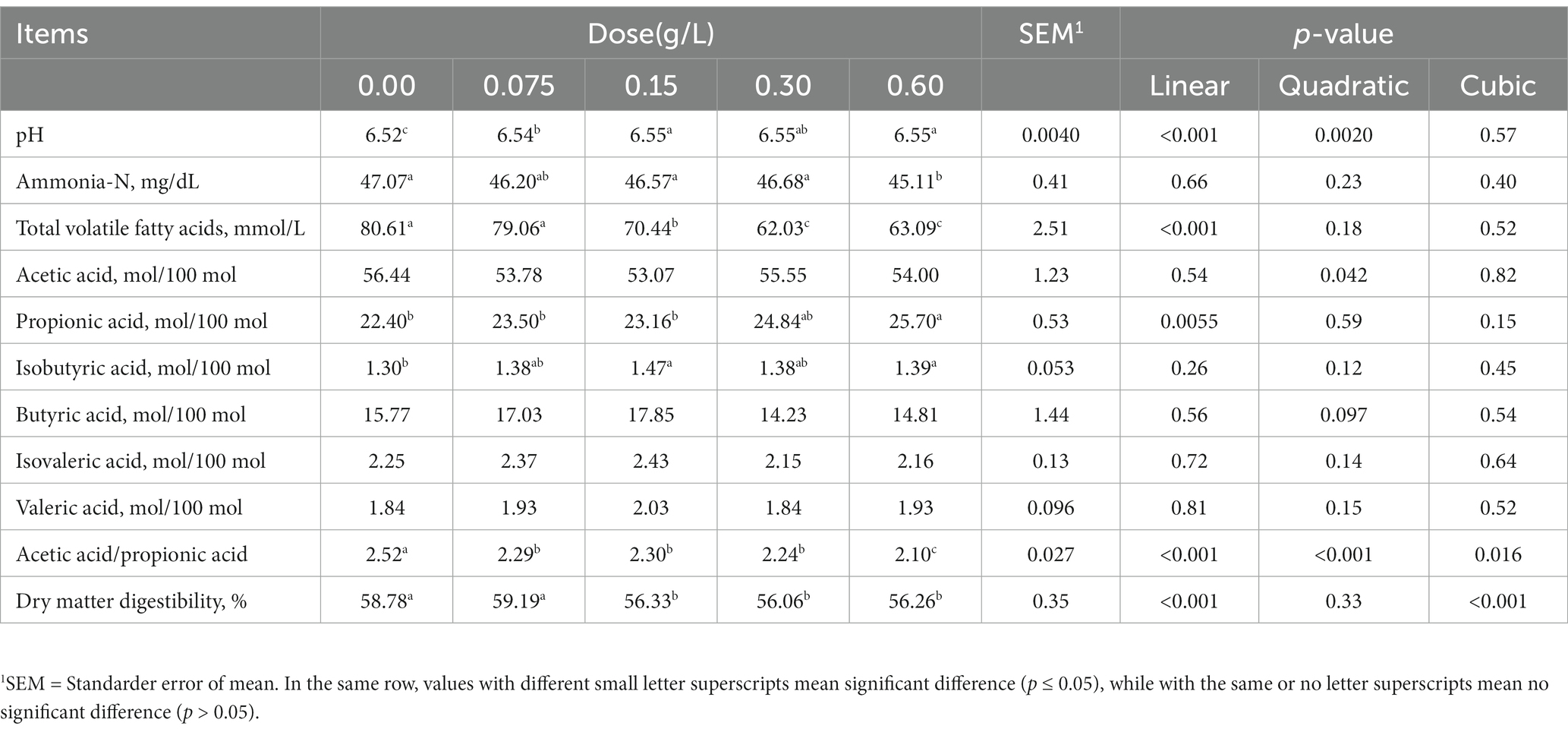
Table 3. Effect of adding different doses of silibinin on rumen fermentation parameters after 24 h of in vitro fermentation.
3.3. Effect of silibinin on the rumen microbiome
To further investigate changes in the rumen microbiome after the addition of silibinin, we select the control group and 0.60 g /L silibinin group, a total of 8 samples. 16S amplicon sequencing was performed by high-throughput sequencing for the rumen microbiome in the control and 0.60 g/L silibinin groups, and the α-diversity and β-diversity of the microbiome, as well as the relative abundance at the genus level, were analyzed. The microbial community diversity of bacteria, archaea, and protozoa in the 0.60 g/L silibinin group was not significantly different from the control group, but Simpson (p = 0.02) and Shannon (p = 0.02) of fungi were significantly different and higher than the untreated group (Table 4). There were also differences in the microbial community structure of bacteria, archaea, protozoa, and fungi in the rumen between the two groups (Figure 1). Figure 2 presents a visual representation of the relative abundance of species at the genus level for both the control group and the 0.60 g/L silibinin group. As the results of the relative abundance of rumen microbiome showed (Table 5), with the addition of 0.60 g/L silibinin, Prevotella, Methanosphaera, Isotricha, Ophryoscolex, and unclassified_Rotifera, Orpinomyces, Neocallimastix were significantly (p < 0.05) less in relative abundance than controls, while Succiniclasticum, NK4A214_group, Candidatus_Saccharimonas, unclassified_Lachnospiraceae, and Others were significantly more abundant than the control group (p < 0.05). These results indicated that supplementation with high doses of silibinin led to alterations in the ruminal microbial community, including changes in its structure and relative abundance.
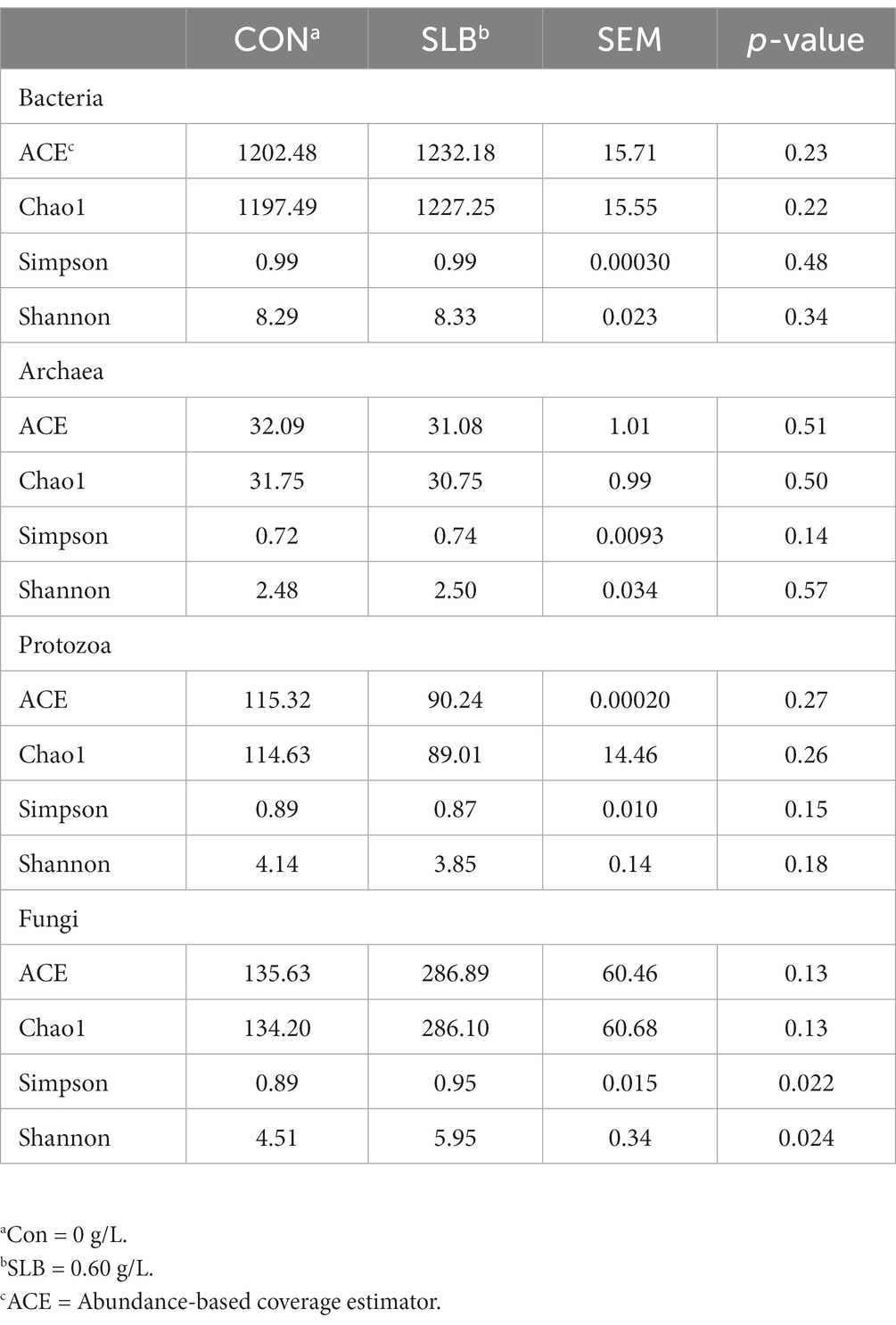
Table 4. Effect of silibinin on the diversity of the most predominant microbial genera in the rumen after 24 h of in vitro incubation (%).
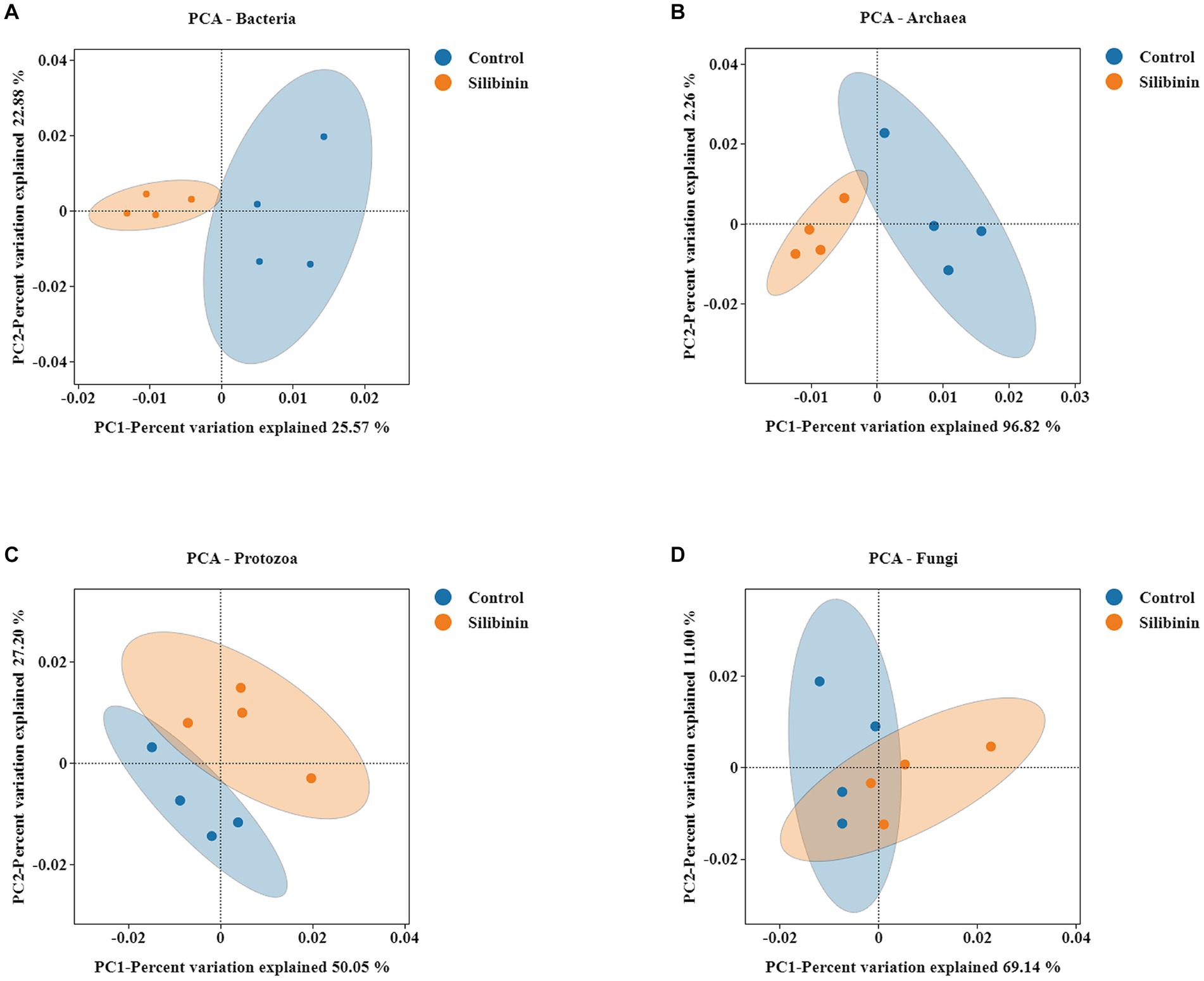
Figure 1. PCA analysis of rumen microbiome in the 0 g/L and 0.60 g/L silibinin addition group (A) Bacteria PCA analysis. (B) Archaea PCA analysis. (C) Protozoa PCA analysis. (D) Fungi PCA analysis.Each point in the figure represents a sample; different colors represent different groups; the oval circle indicates that it is a 95% confidence ellipse (that is, if there are 100 samples in the sample group, 95 will fall into it). The abscissa represents the first principal component, and the percentage represents the contribution value of the first principal component to the sample difference. The ordinate represents the second principal component, and the percentage represents the contribution value of the second principal component to the sample difference.
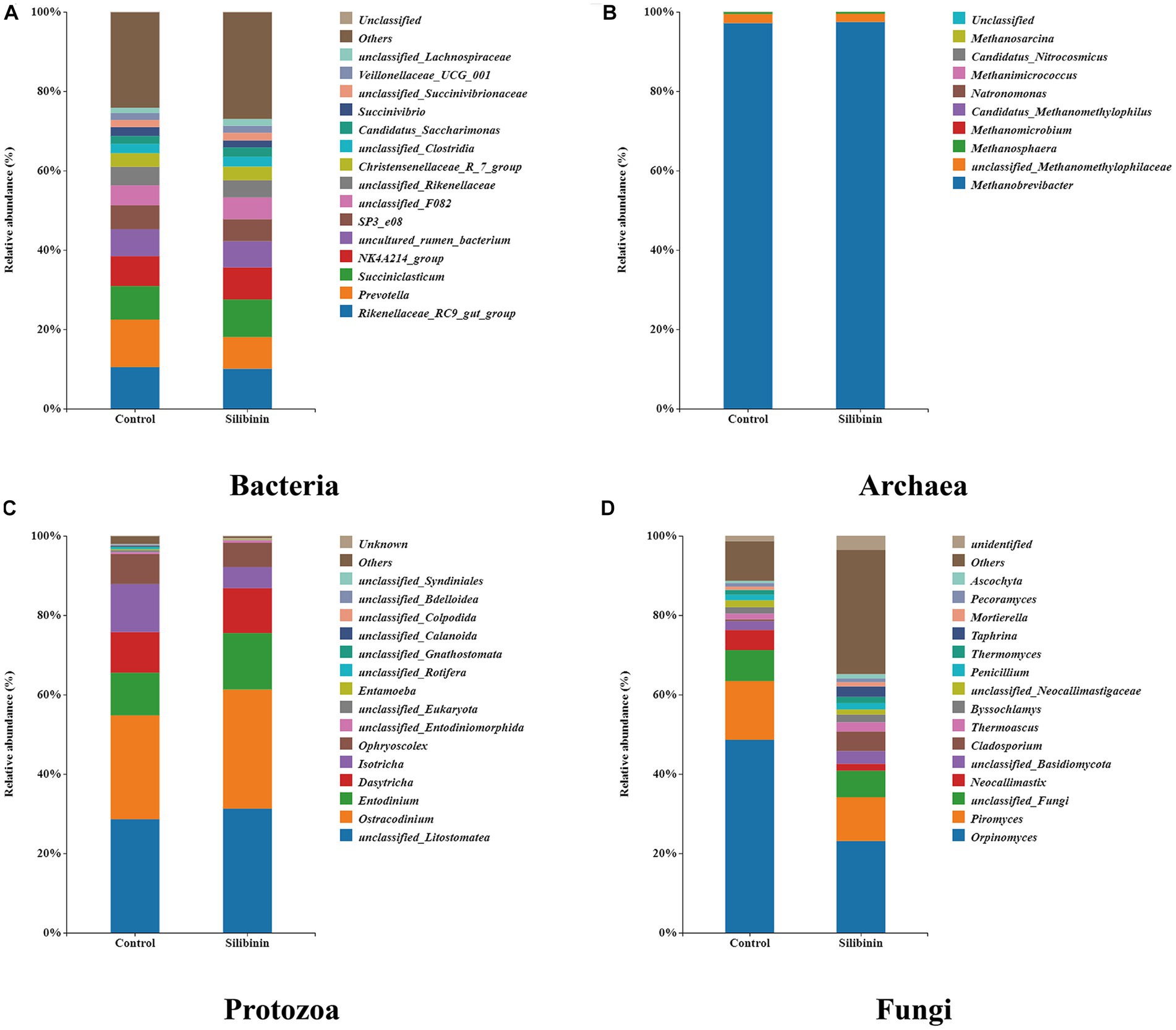
Figure 2. Relative abundance at the genus level of rumen microbiome in the 0 g/L and 0.60 g/L silibinin addition groups. (A) Bacteria relative abundance at the genus level. (B) Archaea relative abundance at the genus level. (C) Protozoa relative abundance at the genus level. (D) Fungi relative abundance at the genus level.
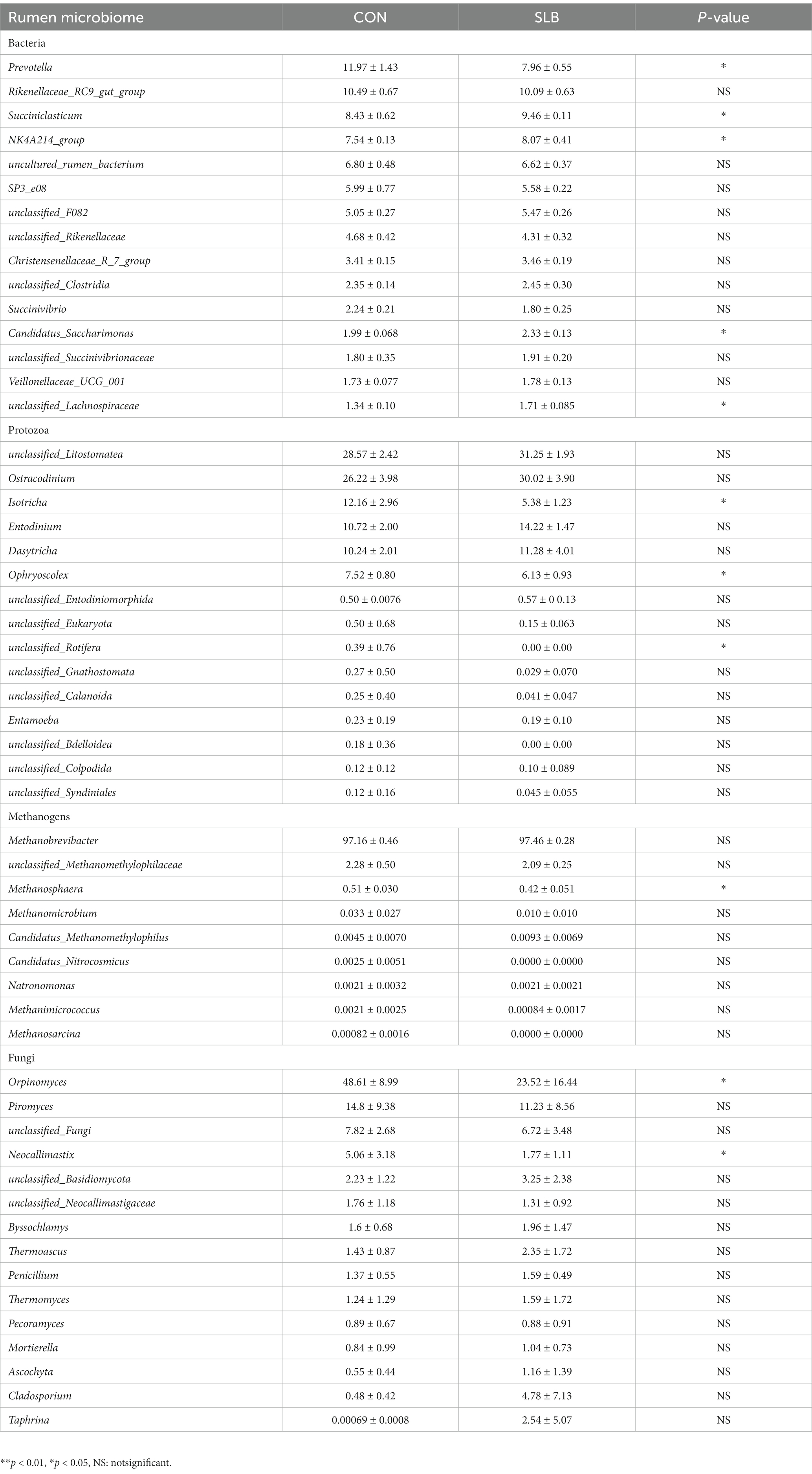
Table 5. Effect of silibinin on the relative abundance of rumen microorganisms after 24 h of in vitro fermentation (%).
3.4. Effect of silibinin on rumen microbial metabolites and their KEGG pathway
Rumen metabolites were analyzed by non-targeted metabolic means to investigate the association between the rumen microbiome and rumen-associated metabolites. A total of 9,162 peaks were detected in 12 samples for qualitative and quantitative metabolism analysis, with 2,414 metabolites annotated for metabolites detected in default mode. The model was evaluated using orthogonal projections to latent structure-discrimination analysis (OPLS-DA) with R2Y = 0.995 and Q2Y = 0.789, which is close to 1, indicating that the model is more stable and reliable, i.e., it can be used to screen for differential metabolites. To check the reliability of the OPLS-DA model, we performed a permutation test. A positive slope of the Q2Y fitted regression line indicates that the model is meaningful. The blue dots are generally above the red dots, indicating that the model’s training and test sets are independent (Figure 3). The OPLS-DA model can be used to identify differences between the two metabolite groups. Based on the results of OPLS-DA, the differential metabolites screened from the variable importance in projection (VIP) of the obtained multivariate analysis OPLS-DA model, combined with fold change and p-value (VIP > 1, p ≤ 0.05, FC > 1 or FC < 0.05) were considered as rumen differential metabolites between the two groups (Table 6). Through conducting a principal component analysis on the samples, we can gather initial insights into the systemic metabolic differences between the control and 0.60 g/L silibinin groups (Figure 4A). The volcano plot (Figure 4B) depicts the degree of difference in metabolite levels between the two groups. Each point on the plot indicates a different metabolite, and its position is determined by the p-value (log base 10) represented on the y-axis, as well as fold change (log base 2) shown on the x-axis. The size of each point is determined by its VIP (Variable Importance in Projection) value for an OPLS-DA model, indicating the significance of differential expression screening with larger scattered points reflecting more reliable differentially expressed metabolites. The plot shows 221 upregulated differentially expressed metabolites represented by red dots and 81 down-regulated differentially expressed metabolites represented by blue dots. Gray dots represent metabolites detected but not significantly different, indicating no significant change of 2,112 metabolites. After sorting by p-value, the top 5 metabolites with significant differences compared to the control group were labeled in the graph. Among them, N-acetylmuramate(beta-methyl)-L-alanyl-D-glutamate, Biotin sulfone, (3Z)-Phycoerythrobilin, L-Dihyd oorotic acid were significantly upregulated (p < 0.05) whereas 2-(7′-methylthio)heptylmalic acid was down-regulated (p < 0.05). Similarly, the Z-score (standard score) based on the quantitative values of metabolites is used to measure the deviation of differences between the experimental group and the control group. The Z-score plot displays the top 30 differential metabolites sorted by p-value, providing an intuitive comparison of metabolite differences between the two groups. Each hollow circle represents the Z-score of metabolite levels in each sample, with the color of the hollow circles representing different groups (Figure 4C).
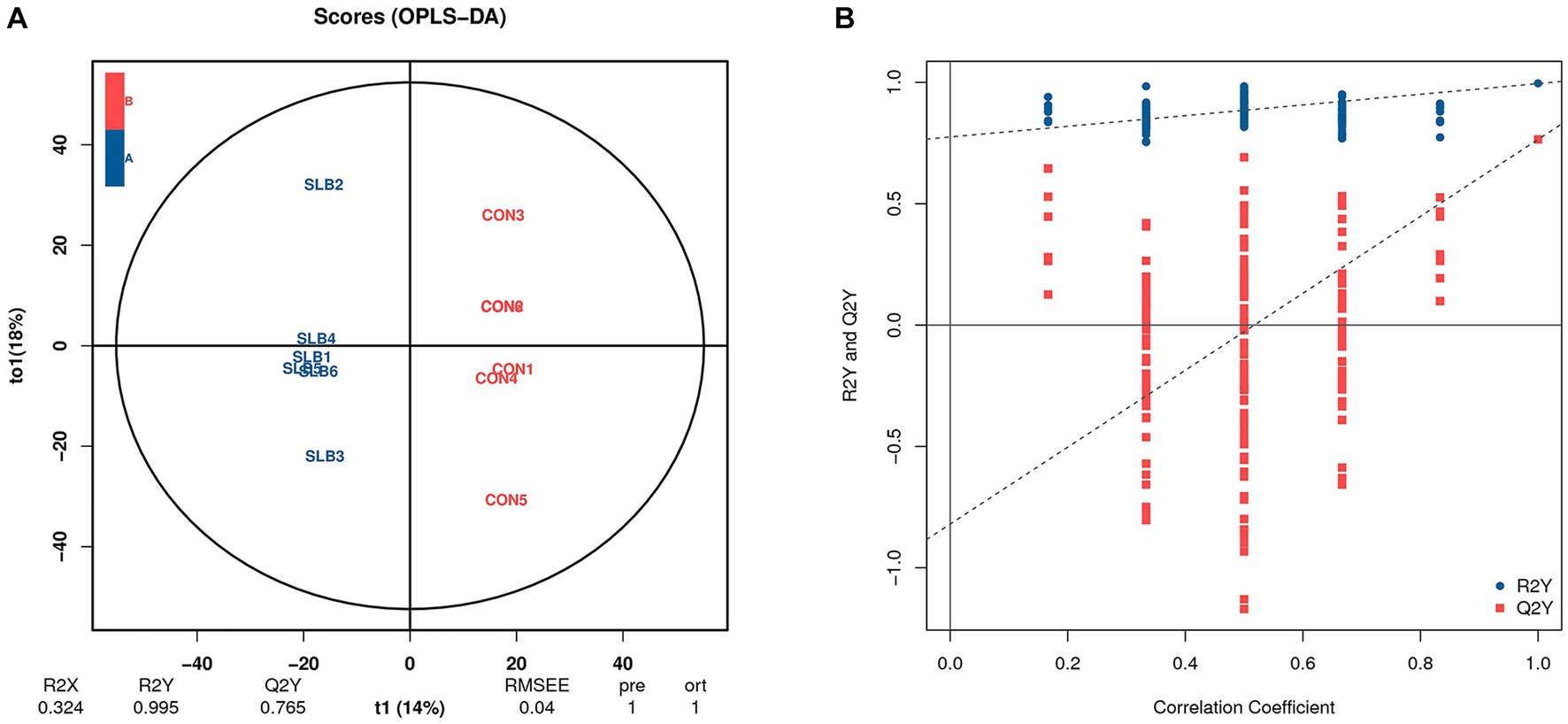
Figure 3. Orthogonal partial least squares-discriminant analysis (OPLS-DA) of the differences in rumen metabolites between 0 g/L and 0.60 g/L silibinin supplementation groups. (A) OPLS-DA score plot. (B) OPLS-DA model substitution test plot.
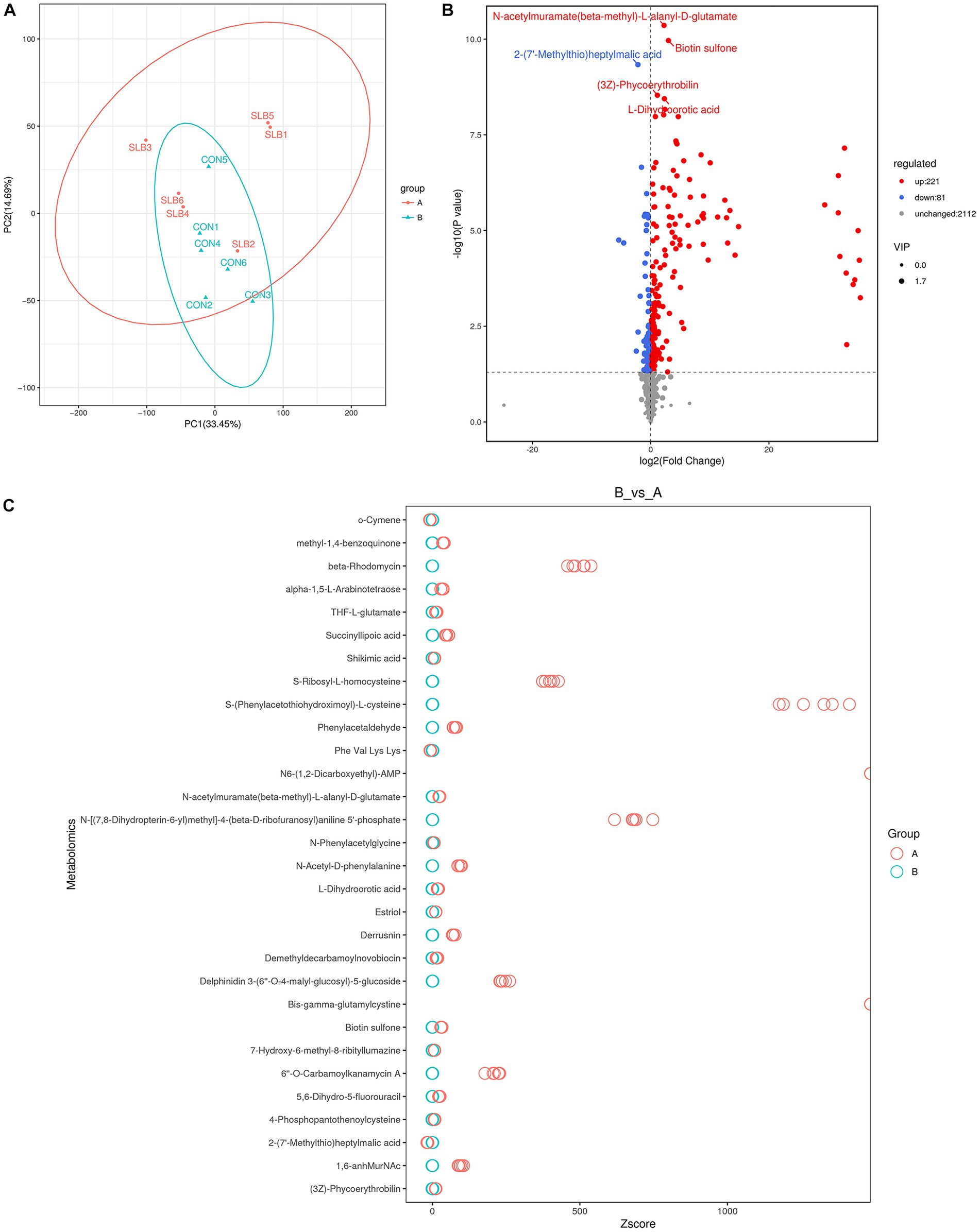
Figure 4. Screening of differential metabolites of rumen metabolites in 0 g/L and 0.60 g/L silibinin supplementation groups (A:SLB; B:CON). (A) Principal component analysis plots for differential groupings, When the number of repetitions in the group was greater than 3, the PCA plot showed the 95% elliptical confidence interval of the group. (B) Heat map of differential metabolite volcanoes, The blue points in the figure represent the down-regulated differentially expressed metabolites, the red points represent the upregulated differentially expressed metabolites, and the gray represents the metabolites detected but the difference is not significant. (C) Differential metabolism Z-score plot, each hollow circle represents the Z-score of the metabolite content in a sample, and the hollow circle color represents different groups.
As different metabolites interact in the organism, different pathways are formed. To gain a more comprehensive understanding of the impact of silibinin on rumen metabolic pathways, we identified and screened for metabolic pathways that displayed significant differences between the two groups. Table 7 showed that the 0.60 g/L silibinin group differed significantly from the control group in the phenylalanine metabolic pathway (p = 0.02), and there was a trend of difference in two metabolic pathways: flavonoid biosynthesis (p = 0.08) and folate biosynthesis (p = 0.10).

Table 7. Significant differences in metabolic pathways between the control and 0.60 g/L silibinin groups.
We screened for differential metabolites in the control and 0.60 g/L silibinin groups with FC < 0.05 or FC > 1 and p ≤ 0.01 (Table 6). 2-Phenylacetamide, N6-(1,2-Dicarboxyethyl)-AMP, phlorizin, Dalspinin, 5,6.7.8-Tetrahydromethanopterin, FMNH, Pyridoxine 5′-phosphate, Silibinin, Beta-D-Fructose 6-phosphate were all up-regulate.
4. Discussion
In this experiment, based on the linear effects of silibinin on most indicators, we selected the maximum dosage group as the research subject for analyzing 16S amplicons and non-targeted metabolomics. By comparing this group with the control group, we aimed to investigate the impact of silibinin on the rumen microbial community, metabolic products, and metabolic pathways to unveil its mechanism in regulating rumen gas production and fermentation parameters. After thoroughly analyzing the results, we discovered that high-dose silibinin influences methane production in the rumen directly and indirectly.
First, an indirect effect of silibinin is its ability to reduce the concentration of methane precursors, thereby indirectly influencing methane production. Ruminal methane production results from the combined effect of various microorganisms within the rumen, and microorganisms, such as protozoa, bacteria, and fungi, play important roles in methane generation within the rumen (Hook et al., 2010). In the rumen, almost all ciliated protozoa are associated with methane-producing bacteria; there is a portion of methane-producing bacteria in the cytoplasm of rumen ciliate protozoa, forming a mutualistic or symbiotic relationship between them (Finlay et al., 1994) which facilitates methanogens in utilizing the H2 produced by the protozoa to generate methane. Isotricha and Ophryoscolex belong to ciliate protozoa (Schrenk and Bardele, 1987; Ivan et al., 2000), and in this experiment, compared to the control group, the relative abundance of these two protozoa was significantly reduced, which may be one of the reasons for the significant decrease in Methanosphaera. Related research has also shown that plant extracts rich in flavonoids have an inhibitory effect on rumen ciliate protozoa (Kim et al., 2015), consistent with the results of this experiment. Furthermore, rumen ciliate protozoa can adhere to feed particles and degrade cellulose, hemicellulose, and starch (Williams et al., 1984; Mendoza et al., 1993), ultimately producing CO2 and H2 for methanogens to utilize. Additionally, the absence of protozoa has been found to reduce organic matter degradation (Newbold et al., 2015). Moreover, silibinin, chemically belonging to flavonoids, has been found to disrupt the cell membrane of rumen protozoa and reduce their population (Ku-Vera et al., 2020), thereby reducing hydrogen transfer between protozoa and methane-producing bacteria. Based on these findings, we speculate that high doses of silibinin may have acted in a similar way on protozoa, leading to the decreased relative abundance of Isotricha and Ophryoscolex observed. This decrease in abundance could have resulted in changes in metabolite production and a reduction in the concentrations of CO2 and H2, ultimately affecting methane levels (Ranilla et al., 2007).
In addition, other indirect effects of silibinin include increased synthesis of propionate and reduced methane production by competitively utilizing substrate H2. Bacteria and fungi in the rumen play a crucial role in fiber degradation, leading to an increase in dry matter degradation rate and the production of VFAs, CO2, and H2. After adding high doses of silibinin, we observed a significant increase in the relative abundance of Succiniclasticum and NK4A214_group. Succiniclasticum has been noted to promote the metabolism of succinate derived from carbohydrate fermentation into propionate (Van Gylswyk, 1995). The production of propionate reduces H2 partial pressure and ensures normal rumen fermentation. However, the relationship between NK4A214_group and propionic acid production remains unclear, and its specific role in the rumen is still not well understood (Pacifico et al., 2021). Further, N6-(1,2-Dicarboxyethyl)-AMP, an upregulated differential metabolite, can be converted into fumarate, which is a precursor of propionate metabolism, by adenosylsuccinate lyase (Timpani et al., 2020). Fumarate acts as an alternative hydrogen sink in the rumen and competes with methanogenic bacteria for H2, thereby reducing CH4 production (Newbold and Rode, 2006). Based on the above analysis, we speculate that the increase in the proportion of propionate in the rumen is likely attributable to the increased relative abundance of Succiniclasticum. It is equally important to note that after adding a high dose of silibinin, there was a significant decrease in the relative abundances of Prevotella, Orpinomyces, and Neocallimastix. Previous studies have shown that flavonoids are usually present in their glycoside form, which is further deglycosylated by glycosidases secreted by microbial communities, increasing aglycone concentrations (Murota et al., 2018); bacteria have evolved diverse metabolic lifestyles and strategies to harvest energy from glycosides (Wang et al., 2022). Moreover, compared to the Proteobacteria phylum, microorganisms within the Bacteroidetes phylum are selectively inhibited by flavonoids (Yu et al., 2023). This explains the increased relative abundances of Succiniclasticum and NK4A214_group and the decreased relative abundance of Prevotella after adding a high dose of silibinin. Furthermore, Orpinomyces and Neocallimastix ferment CO2 and H2 as end products (Williams et al., 2020), and their relative abundances significantly decrease, reducing not only the proportions of CO2 and H2 but also indirectly lowering methane concentrations. In summary, we speculate that high doses of silibinin regulate the relative abundances and metabolic products of bacteria and fungi in the rumen, affecting the concentration of methane precursors and promoting propionate synthesis through competitive utilization of H2, ultimately leading to a reduction in methane production (Zhou et al., 2021).
The direct effect of silibinin in reducing methane production involves modulating the activity of rumen methanogenic bacteria. Ruminal methanogenic bacteria utilize the reducing power generated through rumen fermentation to reduce carbon dioxide, formic acid, methanol or methylamine to CH4. This study showed that compared to the control group, the relative abundance of Methanosphaera was significantly reduced after adding a high dose of silibinin. It is worth noting that Methanosphaera accounts for a relatively small proportion and may have a limited effect on reducing methane concentration. Previous reports have also found that flavonoids can inhibit the activity of Methanosphaera and other methanogenic bacteria and thus reduce CH4 emissions (Seradj et al., 2014; Hassan et al., 2020). Furthermore, epicatechin, quercetin, isoquercetin, and luteolin-7-glucoside reduced the ratio of CH4 production to total gas production (Sinz et al., 2018). In this study, the percentage of methane in the total gas production also significantly decreased with increasing doses of silibinin, consistent with previous research findings.
Furthermore, after analyzing the metabolites, we found that flavin mononucleotide adenine dinucleotide reduced form (FMNH), Pyridoxine 5′-phosphate, and 5,6,7,8-Tetrahydromethanopterin exhibited upregulation. FMNH plays a role in the electron transfer during methane production (Thauer et al., 2008), ultimately being reduced to FMNH2. Pyridoxine 5′-phosphate can be converted into pyridoxal 5′-phosphate (Garrido-Franco, 2003), which inhibits the activity of the final enzyme involved in methane synthesis, namely coenzyme M methyltransferase (Van Der Meijden et al., 1983). During methane generation, 5,6,7,8-Tetrahydromethanopterin (H4MPT) acts as a carrier for the formyl group through the methyl-reduced C1 unit (Van Beelen et al., 1984), forming 10-formyl-H4MPT in methylotrophic organisms (Keltjens et al., 1990). We speculate that it may be that Methanosphaera, a methylotrophic pathway archaea (Wolin, 1985), whose relative abundance significantly decreased, lead to the obstruction of CH4 synthesis and resulting in the upregulation of intermediates such as FMNH, Pyridoxine 5′-phosphate, and 5,6,7,8-Tetrahydromethanopterin. However, further research is needed to validate this. In addition to impacting the production of metabolites, the addition of 0.60 g/L silibinin also had an effect on the phenylalanine metabolic pathway. We presume that the high dose of silibinin affecting the phenylalanine metabolism resulted in the upregulation of differential metabolites 2-Phenylacetamide, Phlorizin, and Dalspinin. In summary, we hypothesize that silibinin modulates the relevant pathways and metabolites in the rumen in a way that affects methane production in the rumen and reduces the proportion of CH4.
In addition, this experiment found that the concentration of NH3-N did not show a linear decrease with increasing dosage of silibinin, and only the group with 0.60 g/L silibinin showed a significant reduction in NH3-N concentration. Previous reports have also shown that supplementation of citrus flavonoids, flavones, myricetin, naringin, catechin, rutin, quercetin, and kaempferol does not affect rumen NH3-N concentration (Oskoueian et al., 2013; Amin et al., 2021). Additionally, Prevotella is involved in the degradation of plant-derived semi-fibrous materials and participates in the process of protein degradation (Betancur-Murillo et al., 2022). We speculate that the decrease in Prevotella relative abundance, possibly due to the addition of 0.60 g/L silibinin, leads to a reduction in protein degradation and subsequently decreases NH3-N concentration (Zhan et al., 2017). However, the optimal range for rumen microbial growth is between 10–50 mg/dL of NH3-N concentration (Firkins et al., 2007). In this experiment, the rumen NH3-N concentration was within this range, indicating that adding a high dose of silibinin may not have a negative impact on microbial biomass protein synthesis. Moreover, related studies have shown a negative correlation between the relative abundance of rumen family NK4A214_group and NH3-N concentration (Liu et al., 2022), as well as a positive correlation between protozoa and NH3-N concentration (Wanapat and Pimpa, 1999), which is consistent with the results of this study. These findings provide new insights into the role of silibinin in regulating the rumen microbial community and methane production mechanisms. On the other hand, from the experimental results, the ingestion of a high dose of silibinin caused a decrease in dry matter degradation rate, which is likely the main reason for the decrease in total volatile fatty acid concentration, and, consequently, the pH value would correspondingly increase. Relevant literature has also indicated that supplementing mulberry leaf flavonoids did not affect the apparent digestibility of dry matter and organic matter (Chen et al., 2015). These results suggest that different plant-derived flavonoid compounds may produce different effects at varying concentrations. In ruminant animal production, volatile fatty acids are important sources of energy, and changes in VFA concentration can affect milk production in cows (Morvay et al., 2011). Therefore, the aim of our study is to reduce methane production while ensuring cow productivity. In this experiment, we selected the group supplemented with 0.60 g/L of silibinin for analysis to better observe the effect of silibinin on rumen methane production. Compared to the group supplemented with 0.00 g/L of silibinin, the group supplemented with 0.075 g/L of silibinin showed no significant difference in total VFA concentration but a significant decrease in methane concentration. Based on this, it can be preliminarily concluded that adding 0.075 g/L of silibinin in vitro experiments can reduce methane concentration without affecting rumen fermentation. Therefore, this group can serve as an additive for in vivo experiments to evaluate the methane-inhibiting effect of silibinin in living organisms.
5. Conclusion
This study provides a theoretical basis for the application of a novel rumen methane regulator. In an in vitro fermentation experiment, silibinin modulates rumen microbial community diversity and community structure, leading to changes in rumen metabolic pathways and metabolites to reduce methane emissions. Notably, silibinin demonstrated the ability to lower methane emissions at reasonable doses without adversely impacting rumen fermentation. Nevertheless, further verification through long-term in vivo experiments is crucial in light of the possible adaptation of rumen microbes to metabolites and differences between in vitro and in vivo findings.
Data availability statement
The data presented in the study are deposited in the NCBI Sequence Read Archive (SRA) repository, accession number PRJNA975466 and PRJNA976941. https://www.ncbi.nlm.nih.gov/sra.
Ethics statement
The animal use protocol was approved following the Animal Care and Use Committee of Northeast Agricultural University (protocol number: NEAUEC20230268).
Author contributions
RL and YS: methodology, investigation, and writing—original draft. HM, ML, BD, WS, and YQ: investigation and data curation. YL and BD: conceptualization, methodology, resources, funding acquisition, and writing—review and editing. YZ: writing—review and Editing. All authors contributed to the article and approved the submitted version.
Funding
This study was financially supported by the Natural Science Foundation of Heilongjiang Province (YQ2023C011), Key Research and Development Program of Heilongjiang Province of China (grant no. 2022ZX01A24), “Academic Backbone” Project of Northeast Agricultural University (22XG35) and Key Laboratory of Low-carbon Green Agriculture in Northeastern China, Ministry of Agriculture and Rural Affairs P. R. China (grant no. LCGANE14).
Conflict of interest
YS was employed by Beijing Sunlon Livestock Development Company Limited.
The remaining authors declare that the research was conducted in the absence of any commercial or financial relationships that could be construed as a potential conflict of interest.
Publisher’s note
All claims expressed in this article are solely those of the authors and do not necessarily represent those of their affiliated organizations, or those of the publisher, the editors and the reviewers. Any product that may be evaluated in this article, or claim that may be made by its manufacturer, is not guaranteed or endorsed by the publisher.
References
Amin, N., Bailoni, L., Wendler, K. R., Caldwell, J., and Pourazad, P. (2021). “Effects of citrus flavonoid, vanillin, and their combination on in vitro rumen fermentation parameters and methane production,’’ in 19th Boku-Symposium Tierernährung. (Vienna: University of Natural Resources and Life Sciences), 1–5.
Betancur-Murillo, C. L., Aguilar-Marin, S. B., and Jovel, J. (2022). Prevotella: a key player in ruminal metabolism. Microorganisms 11:1. doi: 10.3390/microorganisms11010001
Broderick, G. A., and Kang, J. H. (1980). Automated simultaneous determination of ammonia and total amino acids in ruminal fluid and in vitro media. J. Dairy Sci. 63, 64–75. doi: 10.3168/jds.S0022-0302(80)82888-8
Cai, J. Y., Li, J., Hou, Y. N., Ma, K., Yao, G. D., Liu, W. W., et al. (2018). Concentration-dependent dual effects of silibinin on kanamycin-induced cells death in staphylococcus aureus. Biomed. Pharmacother. 102, 782–791. doi: 10.1016/j.biopha.2018.03.133
Chen, D., Chen, X., Tu, Y., Wang, B., Lou, C., Ma, T., et al. (2015). Effects of mulberry leaf flavonoid and resveratrol on methane emission and nutrient digestion in sheep. Anim. Nutr. 1, 362–367. doi: 10.1016/j.aninu.2015.12.008
Feng, Z. D., Zhong, Y. F., He, G. L., Sun, H., Chen, Y. J., Zhou, W. H., et al. (2022). Yeast culture improved the growth performance, liver function, intestinal barrier and microbiota of juvenile largemouth bass (Micropterus salmoides) fed high-starch diet. Fish Shellfish Immunol. 120, 706–715. doi: 10.1016/j.fsi.2021.12.034
Finlay, B. J., Esteban, G., Clarke, K. J., Williams, A. G., Embley, T. M., and Hirt, R. P. (1994). Some rumen ciliates have endosymbiotic methanogens. FEMS Microbiol. Lett. 117, 157–161. doi: 10.1111/j.1574-6968.1994.tb06758.x
Firkins, J., Yu, Z., and Morrison, M. (2007). Ruminal nitrogen metabolism: perspectives for integration of microbiology and nutrition for dairy. J. Dairy Sci. 90, E1–E16. doi: 10.3168/jds.2006-518
Garrido-Franco, M. (2003). Pyridoxine 5′-phosphate synthase: De novo synthesis of vitamin B6 and beyond. Biochim. Biophys. Acta. Proteins. Proteom. 1647, 92–97. doi: 10.1016/S1570-9639(03)00065-7
Haque, M. N. (2018). Dietary manipulation: a sustainable way to mitigate methane emissions from ruminants. J. Anim. Sci. Technol. 60, 1–10. doi: 10.1186/s40781-018-0175-7
Hassan, F. U., Arshad, M. A., Li, M., Rehman, M. S. U., Loor, J. J., and Huang, J. (2020). Potential of mulberry leaf biomass and its flavonoids to improve production and health in ruminants: mechanistic insights and prospects. Animals 10:2076. doi: 10.3390/ani10112076
Hook, S. E., Wright, A. D. G., and McBride, B. W. (2010). Methanogens: methane producers of the rumen and mitigation strategies. Archaea 2010:945785, 1–11. doi: 10.1155/2010/945785
Hu, W. L., Liu, J. X., Ye, J. A., Wu, Y. M., and Guo, Y. Q. (2005). Effect of tea saponin on rumen fermentation in vitro. Anim. Feed Sci. Technol. 120, 333–339. doi: 10.1016/j.anifeedsci.2005.02.029
Ivan, M., Neill, L., Forster, R., Alimon, R., Rode, L., and Entz, T. (2000). Effects of Isotricha, Dasytricha, Entodinium, and total fauna on ruminal fermentation and duodenal flow in wethers fed different diets. J. Dairy Sci. 83, 776–787. doi: 10.3168/jds.S0022-0302(00)74940-X
Janssen, P. H., and Kirs, M. (2008). Structure of the archaeal community of the rumen. Appl. Environ. Microbiol. 74, 3619–3625. doi: 10.1128/AEM.02812-07
Jubair, N., Rajagopal, M., Chinnappan, S., Abdullah, N. B., and Fatima, A. (2021). Review on the antibacterial mechanism of plant-derived compounds against multidrug-resistant bacteria (MDR). Evid. Based Complement. Alternat. Med. 2021, 1–30. doi: 10.1155/2021/3663315
Keltjens, J. T., te Brömmelstroet, B. W., Kengen, S. W., van der Drift, C., and Vogels, G. D. (1990). 5, 6, 7, 8-Tetrahydromethanopterin-dependent enzymes involved in methanogenesis. FEMS Microbiol. Rev. 87, 327–332. doi: 10.1111/j.1574-6968.1990.tb04932.x
Kim, W. Y., Hanigan, M. D., Lee, S. J., Lee, S. M., Kim, D. H., Hyun, J. H., et al. (2014). Effects of cordyceps militaris on the growth of rumen microorganisms and in vitro rumen fermentation with respect to methane emissions. J. Dairy Sci. 97, 7065–7075. doi: 10.3168/jds.2014-8064
Kim, H., Jung, E., Lee, H. G., Kim, B., Cho, S., Lee, S., et al. (2019). Essential oil mixture on rumen fermentation and microbial community - an in vitro study. Asian-Australas J Anim Sci 32, 808–814. doi: 10.5713/ajas.18.0652
Kim, E. T., Lee, S. J., Lee, S. M., Lee, S. S., Lee, I. D., Lee, S. K., et al. (2015). Effects of flavonoid-rich plant extracts on in vitro ruminal methanogenesis, microbial populations and fermentation characteristics. Asian-Australas. J. Anim. Sci. 28, 530–537. doi: 10.5713/ajas.14.0692
Ku-Vera, J. C., Jiménez-Ocampo, R., Valencia-Salazar, S. S., Montoya-Flores, M. D., Molina-Botero, I. C., Arango, J., et al. (2020). Role of secondary plant metabolites on enteric methane mitigation in ruminants. Front. Vet. Sci. 7:584. doi: 10.3389/fvets.2020.00584
Lee, Y. S., Jang, K., and Cha, J. D. (2012). Synergistic antibacterial effect between silibinin and antibiotics in oral bacteria. J. Biomed. Biotechnol. 2012, 1–618087. doi: 10.1155/2012/618081
Lee, D. G., Kim, H. K., Park, Y., Park, S. C., Woo, E. R., Jeong, H. G., et al. (2003). Gram-positive bacteria specific properties of silybin derived from silybum marianum. Arch. Pharm. Res. 26, 597–600. doi: 10.1007/BF02976707
Liu, K., Zhang, Y., Huang, G., Zheng, N., Zhao, S., and Wang, J. (2022). Ruminal bacterial community is associated with the variations of total milk solid content in Holstein lactating cows. Anim. Nutr. 9, 175–183. doi: 10.1016/j.aninu.2021.12.005
Mahmoudi-Rad, Z., Nourafcan, H., Mohebalipour, N., Assadi, A., and Jamshidi, S. (2022). Antibacterial effect of methanolic extract of milk thistle seed on 8 species of gram-positive and negative bacteria. J. Plant Res. 35, 776–785.
Marques, R. D. S., and Cooke, R. F. (2021). Effects of ionophores on ruminal function of beef cattle. Animals 11:2871. doi: 10.3390/ani11102871
Mendoza, G., Britton, R., and Stock, R. (1993). Influence of ruminal protozoa on site and extent of starch digestion and ruminal fermentation. J. Anim. Sci. 71, 1572–1578. doi: 10.2527/1993.7161572x
Miller, T. L. (2015). “Methanobrevibacter” in Bergey's manual of systematics of archaea and bacteria. ed. B. Wolfe (United Kingdom: John Wiley and Sons, Inc., in association with Bergey's Manual Trust), 1–14.
Morvay, Y., Bannink, A., France, J., Kebreab, E., and Dijkstra, J. (2011). Evaluation of models to predict the stoichiometry of volatile fatty acid profiles in rumen fluid of lactating Holstein cows. J. Dairy Sci. 94, 3063–3080. doi: 10.3168/jds.2010-3995
Murota, K., Nakamura, Y., and Uehara, M. (2018). Flavonoid metabolism: the interaction of metabolites and gut microbiota. Biosci. Biotechnol. Biochem. 82, 600–610. doi: 10.1080/09168451.2018.1444467
Myhre, G., Shindell, D., and Pongratz, J. (2014). “Anthropogenic and natural radiative forcing” in Climate change 2013: The physical science basis; working group I contribution to the fifth assessment report of the intergovernmental panel on climate change. ed. T. Stocker (Cambridge: Cambridge University Press), 659–740.
Newbold, C. J., De la Fuente, G., Belanche, A., Ramos-Morales, E., and McEwan, N. R. (2015). The role of ciliate protozoa in the rumen. Front. Microbiol. 6:1313. doi: 10.3389/fmicb.2015.01313
Newbold, C. J., and Rode, L. (2006). Dietary additives to control methanogenesis in the rumen. Elsevier 1293, 138–147. doi: 10.1016/j.ics.2006.03.047
Oskoueian, E., Abdullah, N., and Oskoueian, A. (2013). Effects of flavonoids on rumen fermentation activity, methane production, and microbial population. Biomed. Res. Int. 2013:349129, 1–8. doi: 10.1155/2013/349129
Pacifico, C., Petri, R. M., Ricci, S., Mickdam, E., Wetzels, S. U., Neubauer, V., et al. (2021). Unveiling the bovine epimural microbiota composition and putative function. Microorganisms. 9:342. doi: 10.3390/microorganisms9020342
Rakelly de Oliveira, D., Relison Tintino, S., Morais Braga, M. F. B., Boligon, A. A., Linde Athayde, M., Douglas Melo Coutinho, H., et al. (2015). In vitro antimicrobial and modulatory activity of the natural products silymarin and silibinin. Biomed. Res. Int. 2015:292797. doi: 10.1155/2015/292797
Ranilla, M. J., Jouany, J. P., and Morgavi, D. P. (2007). Methane production and substrate degradation by rumen microbial communities containing single protozoal species in vitro. Lett. Appl. Microbiol. 45, 675–680. doi: 10.1111/j.1472-765X.2007.02251.x
Sakadevan, K., and Nguyen, M. L. (2017). Livestock production and its impact on nutrient pollution and greenhouse gas emissions. Adv. Agron. 141, 147–184. doi: 10.1016/bs.agron.2016.10.002
Schrenk, H. G., and Bardele, C. F. (1987). The fine structure of the paralabial organelle in the rumen ciliate Ophryoscolex purkinjei stein, 1858. J. Protozool. 34, 97–104. doi: 10.1111/j.1550-7408.1987.tb03141.x
Seradj, A. R., Abecia, L., Crespo, J., Villalba, D., Fondevila, M., and Balcells, J. (2014). The effect of Bioflavex® and its pure flavonoid components on in vitro fermentation parameters and methane production in rumen fluid from steers given high concentrate diets. Anim. Feed Sci. Technol. 197, 85–91. doi: 10.1016/j.anifeedsci.2014.08.013
Sheng, D. D., Zhao, S. M., Gao, L., Zheng, H. F., Liu, W. T., Hou, J., et al. (2019). Babaodan attenuates high-fat diet-induced non-alcoholic fatty liver disease via activation of AMPK signaling. Cell Biosci. 9, 1–10. doi: 10.1186/s13578-019-0339-2
Sinz, S., Kunz, C., Liesegang, A., Braun, U., Marquardt, S., Soliva, C. R., et al. (2018). In vitro bioactivity of various pure flavonoids in ruminal fermentation, with special reference to methane formation. Czech J. Anim. Sci. 63, 293–304. doi: 10.17221/118/2017-CJAS
Soliva, C., and Hess, H. (2007). “Measuring methane emission of ruminants by in vitro and in vivo techniques” in Measuring methane production from ruminants. eds. H. P. S. Makkar and E. Vercoe Philip (Berlin, Germany: Springer), 15–31. doi: 10.1007/978-1-4020-6133-2_2
Sommai, S., Cherdthong, A., Suntara, C., So, S., Wanapat, M., and Polyorach, S. (2021). In vitro fermentation characteristics and methane mitigation responded to flavonoid extract levels from alternanthera sissoo and dietary ratios. Fermentation 7:109. doi: 10.3390/fermentation7030109
Stocker, T. F., Qin, D., Plattner, G., Tignor, M., Allen, S., Boschung, J., et al. (2013). Contribution of working group I to the fifth assessment report of the intergovernmental panel on climate change. Climate Change 5, 1–1552. doi: 10.1017/CBO9781107415324
Teng, P. Y., and Kim, W. K. (2021). Roles of nitrocompounds in inhibition of foodborne bacteria, parasites, and methane production in economic animals. Animals 11:923. doi: 10.3390/ani11040923
Thauer, R. K., Kaster, A. K., Seedorf, H., Buckel, W., and Hedderich, R. (2008). Methanogenic archaea: ecologically relevant differences in energy conservation. Nat. Rev. Microbiol. 6, 579–591. doi: 10.1038/nrmicro1931
Timpani, C. A., Goodman, C. A., Stathis, C. G., White, J. D., Mamchaoui, K., Butler-Browne, G., et al. (2020). Adenylosuccinic acid therapy ameliorates murine duchenne muscular dystrophy. Sci. Rep. 10, 1–18. doi: 10.1038/s41598-020-57610-w
Vaghar Seyedin, S. M., Zeidi, A., Chamanehpour, E., Nasri, M. H. F., and Vargas-Bello-Pérez, E. (2022). Methane emission: strategies to reduce global warming in relation to animal husbandry units with emphasis on ruminants. Sustainability 14:16897. doi: 10.3390/su142416897
Van Beelen, P., Van Neck, J. W., De Cock, R. M., Vogels, G. D., Guijt, W., and Haasnoot, C. A. (1984). 5, 10-Methenyl-5, 6, 7, 8-tetrahydromethanopterin, a one-carbon carrier in the process of methanogenesis. Biochemistry 23, 4448–4454. doi: 10.1021/bi00314a032
Van Der Meijden, P., Heythuysen, H. J., Sliepenbeek, H. T., Houwen, F. P., Van Der Drift, C., and Vogels, G. D. (1983). Activation and inactivation of methanol: 2-mercaptoethanesulfonic acid methyltransferase from Methanosarcina barkeri. J. Bacteriol. 153, 6–11. doi: 10.1128/jb.153.1.6-11.1983
Van Gylswyk, N. (1995). Succiniclasticum ruminis gen. Nov., sp. nov., a ruminal bacterium converting succinate to propionate as the sole energy-yielding mechanism. Int. J. Syst. Evol. Microbiol. 45, 297–300. doi: 10.1099/00207713-45-2-297
Vaou, N., Stavropoulou, E., Voidarou, C., Tsigalou, C., and Bezirtzoglou, E. (2021). Towards advances in medicinal plant antimicrobial activity: a review study on challenges and future perspectives. Microorganisms 9:2041. doi: 10.3390/microorganisms9102041
Vendramini, T. H. A., Takiya, C. S., Silva, T., Zanferari, F., Rentas, M. F., Bertoni, J., et al. (2016). Effects of a blend of essential oils, chitosan or monensin on nutrient intake and digestibility of lactating dairy cows. Feed Sci. Technol. 214, 12–21. doi: 10.1016/j.anifeedsci.2016.01.015
Wanapat, M., and Pimpa, O. (1999). Effect of ruminal NH3-N levels on ruminal fermentation, purine derivatives, digestibility and rice straw intake in swamp buffaloes. Asian-Australas J. Anim. Sci. 12, 904–907. doi: 10.5713/ajas.1999.904
Wang, Z., Tauzin, A. S., Laville, E., and Potocki-Veronese, G. (2022). Identification of glycoside transporters from the human gut microbiome. Front. Microbiol. 13:816462. doi: 10.3389/fmicb.2022.816462
Wang, Y., Yu, Q., Wang, X., Song, J., Lambo, M. T., Huang, J., et al. (2023). Replacing alfalfa hay with industrial hemp ethanol extraction byproduct and Chinese wildrye hay: effects on lactation performance, plasma metabolites, and bacterial communities in Holstein cows. Front. Vet. Sci. 10:31. doi: 10.3389/fvets.2023.1061219
Wang, L., Zhao, C., Zeng, Y., Chen, Z., and Zheng, C. (2017). Effects of adding mannan-oligosaccharides to diets with different concentrate to roughage ratios on nutrient degradation rates in sheep rumen. Chinese J. Anim. Nutr. 29, 2556–2564. doi: 10.3969/j.issn.1006-267x.2017.07.041
Wei, Z., Zhang, B., and Liu, J. (2018). Effects of the dietary nonfiber carbohydrate content on lactation performance, rumen fermentation, and nitrogen utilization in mid-lactation dairy cows receiving corn Stover. J. Anim. Sci. Biotechnol. 9, 699–705. doi: 10.1186/s40104-018-0239-z
Williams, C. L., Thomas, B. J., McEwan, N. R., Rees Stevens, P., Creevey, C. J., and Huws, S. A. (2020). Rumen protozoa play a significant role in fungal predation and plant carbohydrate breakdown. Front. Microbiol. 11:720. doi: 10.3389/fmicb.2020.00720
Williams, A. G., Withers, S. E., and Coleman, G. S. (1984). Glycoside hydrolases of rumen bacteria and protozoa. Curr. Microbiol. 10, 287–293. doi: 10.1007/BF01577143
Wolin, T. L. M. M. J. (1985). Methanosphaera stadtmaniae gen. Nov., sp. nov. a species that forms methane by reducing methanol with hydrogen. Arch. Microbiol. 141, 116–122. doi: 10.1007/BF00423270
Xin, H. S., Khan, N. A., Liu, X., Jiang, X., Sun, F., Zhang, S. Z., et al. (2021). Profiles of odd-and branched-chain fatty acids and their correlations with rumen fermentation parameters, microbial protein synthesis, and bacterial populations based on pure carbohydrate incubation in vitro. Front. Nutr. 8:673. doi: 10.3389/fnut.2021.733352
Yang, W. Z., and Beauchemin, K. A. (2006). Increasing the physically effective fiber content of dairy cow diets may lower efficiency of feed use. J. Dairy Sci. 89, 2694–2704.
Yang, C., Xu, Z., Deng, Q., Huang, Q., Wang, X., and Huang, F. (2020). Beneficial effects of flaxseed polysaccharides on metabolic syndrome via gut microbiota in high-fat diet fed mice. Food Res. Int. 131:108994. doi: 10.1016/j.foodres.2020.108994
Yu, S., Li, L., Zhao, H., Zhang, S., Tu, Y., Liu, M., et al. (2023). Dietary citrus flavonoid extract improves lactational performance through modulating rumen microbiome and metabolites in dairy cows. Food Funct. 14, 94–111. doi: 10.1039/D2FO02751H
ZeidAli-Nejad, A., Ghorbani, G. R., Kargar, S., Sadeghi-Sefidmazgi, A., Pezeshki, A., and Ghaffari, M. H. (2018). Nutrient intake, rumen fermentation and growth performance of dairy calves fed extruded full-fat soybean as a replacement for soybean meal. Animal 12, 733–740.
Zhan, J., Liu, M., Wu, C., Su, X., Zhan, K., and Zhao, G. (2017). Effects of alfalfa flavonoids extract on the microbial flora of dairy cow rumen. Asian-Australas. J. Anim. 30, 1261–1269. doi: 10.5713/ajas.16.0839
Zhang, N. N., Hu, G. J., Guo, K., Fu, T., Lian, H. X., Wang, L. F., et al. (2021). Rumen bacteria and epithelial metabolism contribute to improving N utilization efficiency of calves. Anim. Biotechnol. 33, 1480–1491. doi: 10.1080/10495398.2021.1909056
Keywords: silibinin, methane, rumen fermentation, microbiome, metabolite
Citation: Liu R, Shen Y, Ma H, Li Y, Lambo MT, Dai B, Shen W, Qu Y and Zhang Y (2023) Silibinin reduces in vitro methane production by regulating the rumen microbiome and metabolites. Front. Microbiol. 14:1225643. doi: 10.3389/fmicb.2023.1225643
Edited by:
Raffaella Tudisco, University of Naples Federico II, ItalyReviewed by:
Stefan Muetzel, AgResearch Ltd, New ZealandYu Pi, Feed Research Institute (CAS), China
Copyright © 2023 Liu, Shen, Ma, Li, Lambo, Dai, Shen, Qu and Zhang. This is an open-access article distributed under the terms of the Creative Commons Attribution License (CC BY). The use, distribution or reproduction in other forums is permitted, provided the original author(s) and the copyright owner(s) are credited and that the original publication in this journal is cited, in accordance with accepted academic practice. No use, distribution or reproduction is permitted which does not comply with these terms.
*Correspondence: Yang Li, bGl5YW5nMTQwNTA1M0BuZWF1LmVkdS5jbg==; Baisheng Dai, YnNkYWlAbmVhdS5lZHUuY24=
†These authors have contributed equally to this work