- 1Virology Laboratory, Centre for Virus Research, Therapeutics and Vaccines, Translational Health Science and Technology Institute, NCR Biotech Science Cluster, Faridabad, Haryana, India
- 2University School of Biotechnology, Guru Gobind Singh Indraprastha University, New Delhi, India
Viral hepatitis is a major public health concern globally. World health organization aims at eliminating viral hepatitis as a public health threat by 2030. Among the hepatitis causing viruses, hepatitis B and C are primarily transmitted via contaminated blood. Hepatitis A and E, which gets transmitted primarily via the feco-oral route, are the leading cause of acute viral hepatitis. Although vaccines are available against some of these viruses, new cases continue to be reported. There is an urgent need to devise a potent yet economical antiviral strategy against the hepatitis-causing viruses (denoted as hepatitis viruses) for achieving global elimination of viral hepatitis. Although zinc was known to mankind for a long time (since before Christ era), it was identified as an element in 1746 and its importance for human health was discovered in 1963 by the pioneering work of Dr. Ananda S. Prasad. A series of follow up studies involving zinc supplementation as a therapy demonstrated zinc as an essential element for humans, leading to establishment of a recommended dietary allowance (RDA) of 15 milligram zinc [United States RDA for zinc]. Being an essential component of many cellular enzymes and transcription factors, zinc is vital for growth and homeostasis of most living organisms, including human. Importantly, several studies indicate potent antiviral activity of zinc. Multiple studies have demonstrated antiviral activity of zinc against viruses that cause hepatitis. This article provides a comprehensive overview of the findings on antiviral activity of zinc against hepatitis viruses, discusses the mechanisms underlying the antiviral properties of zinc and summarizes the prospects of harnessing the therapeutic benefit of zinc supplementation therapy in reducing the disease burden due to viral hepatitis.
1. Introduction
Zinc is the second most abundant trace element found in humans. A healthy adult body contains 2–4 grams of zinc. Zinc is involved in several biological functions including growth, development, maintenance of immune system and disease resistance. It shows broad anti-inflammatory and anti-oxidant properties. At molecular level, many enzymes and proteins that regulate DNA replication, transcription, signal transduction and apoptosis require zinc for their activities (Maret and Sandstead, 2006; John et al., 2010; Ryu et al., 2020).
Plasma or serum zinc levels between 0.8 and 1.20 μg/ml is considered normal in a healthy individual. Zinc is absorbed in the small intestine by a carrier-mediated mechanism in a concentration dependent manner and increases with increasing dietary zinc (Roohani et al., 2013; Ryu et al., 2020). The portal system delivers absorbed zinc directly to the liver, which directs its circulation for delivery to the other tissues. About 70% of the zinc in circulation is bound to albumin, and any condition that alters serum albumin concentration can have a secondary effect on serum zinc levels (Roohani et al., 2013).
Zinc level gets affected by many conditions such as infections, changes in steroid hormone levels, and muscle catabolism during weight loss or illness (Roohani et al., 2013; Ryu et al., 2020). Around 2 billion people are suggested to have prolonged zinc deficiency worldwide, majority of which includes population from economically weaker countries (Prasad, 2013). Zinc deficiency enhances vulnerability to many viral infections and increasing number of studies support the therapeutic benefit of zinc supplementation in alleviating several viral diseases (Sadeghsoltani et al., 2021). This review provides a comprehensive summary of the antiviral effect of zinc in viral hepatitis and discusses the possible scope of better management of viral hepatitis cases using more potent zinc formulations.
2. Antiviral effect of zinc on hepatitis viruses
Majority of viral hepatitis is caused by the Hepatitis A virus (HAV), Hepatitis B virus (HBV), Hepatitis C virus (HCV), Hepatitis D virus (HDV) and Hepatitis E virus (HEV; Myers et al., 2002). In addition, Herpes viruses such as Epstein–Barr virus (EBV), Cytomegalo virus (CMV), Adenovirus and Varicella zoster virus (VZV) also induce hepatic injury (Lalazar and Ilan, 2014). Herpes simplex virus (HSV) induced hepatitis is a rare cause of acute liver failure (Chaudhary et al., 2017).
2.1. Hepatitis A virus
HAV is a positive stranded nonenveloped RNA virus that is transmitted via the fecal-oral route (Martin and Lemon, 2006; Traore et al., 2012). It is the most common cause of acute viral hepatitis globally (Hauri et al., 2006). It does not cause chronic hepatitis but it adds to further deterioration of liver infected with other hepatotropic viruses (Keeffe, 1995; Vento et al., 1998). A vaccine is available against it (Hauri et al., 2006). No specific treatment is available against it but the disease is self-limiting and there is no lasting injury.
2.2. Hepatitis B virus
HBV is transmitted through exposure to contaminated blood products and body fluids (Myers et al., 2002). It is a DNA virus. Chronic infection with hepatitis B virus (HBV) is estimated to affect 400 million individuals globally, and it is the leading cause of HCC (Lok et al., 2001). Vaccines and antiviral therapies are available against HBV (Das et al., 2019; Zhu et al., 2022).
2.3. Hepatitis C virus
HCV is transmitted through exposure to contaminated blood products (Myers et al., 2002). HCV affects more than 170 million people worldwide (Bhatia et al., 2014; Manns et al., 2017). Coinfections of hepatitis viruses are frequently observed in clinical setting. Furthermore, their propensity for chronicity sets the stage for superinfection with other viruses (Myers et al., 2002). It frequently causes chronic infection, leading to hepatocellular carcinoma (HCC; Vento et al., 1998; Manns et al., 2017). No vaccine is available against it. Treatment options for HCV cases include a combination of broadly-acting antivirals (such as peg-interferon, ribavirin) and specific direct-acting antiviral (Sofosbuvir; Bhatia et al., 2014).
2.4. Hepatitis D virus
HDV is transmitted through exposure to contaminated blood products and body fluids (Myers et al., 2002). It requires the HBV surface antigen (HBsAg) to replicate and is dependent on the latter (Huang and Lo, 2014). Around 5% of HBV carriers (approximately 20 million individuals) are coinfected with the HDV (Myers et al., 2002). No vaccine is available against HDV but HBV vaccinated people are protected from it as it is a significant threat only in HBV infected individuals. Recently, Bulevirtide was shown to be a potential treatment option against HDV (Dietz-Fricke et al., 2023).
2.5. Hepatitis E virus
HEV is a positive stranded quasi-enveloped RNA virus (Nimgaonkar et al., 2018). It is transmitted via the fecal-oral route. It can also get transmitted via blood transfusion. Zoonotic transmission of HEV from animals to human is also reported. It is a major cause of acute viral hepatitis globally (Primadharsini et al., 2021). HAV and HEV are major cause of community level outbreaks and epidemics in areas with poor sanitary conditions (Wu et al., 2016; Primadharsini et al., 2021). It may cause chronic infection in immune compromised individuals. At present, a vaccine against HEV is available in China (Wu et al., 2016). HEV cases are self-limiting in otherwise healthy individuals. A combination of broadly-acting antivirals are the option for off-label therapy in severe HEV cases (Netzler et al., 2019).
There is a need to formulate more potent, side-effect free therapeutics for treatment of viral hepatitis cases (Cowan et al., 2011). Controlled zinc supplementation is known to be a safe, side effect free therapy against Wilson’s disease (Brewer et al., 2000). Zinc is widely used as an antimicrobial agent, without any side effect (Wessels et al., 2022). Zinc supplementation is a part of standard care in the treatment of diarrhea in infants (Bajait and Thawani, 2011). Multiple laboratories have independently evaluated the antiviral potential of zinc against hepatitis viruses using diverse experimental approaches. Compilation and careful interpretation of the available data will be useful in evaluating the therapeutic potential of zinc in the treatment of viral hepatitis cases. Below sections compile majority of the available data on antiviral activity of zinc in vivo and in vitro.
2.6. Clinical trials on evaluation of therapeutic benefit of zinc compounds in viral hepatitis patients
Multiple clinical trials have been performed to determine the therapeutic benefit of zinc supplementation in viral hepatitis patients (Table 1). Majority of the trials involved HCV patients (10 trials), one trial involved HBV patients and one trial involved HEV patients. In some studies, serum zinc level was measured in the patients before and after the treatment regimen and compared to that of the placebo group. Pre-existing zinc deficiency was observed in 4 trials while normal zinc level was observed in 3 trials and zinc level was not measured in 5 trials. Zinc supplementation increased the serum zinc level in three of the four zinc deficient groups tested. Effect of zinc supplementation on disease outcome was evaluated by measuring the levels of serum albumin, ALT (alanine aminotransferase), AST (aspartate aminotransferase) and viral load [sustained viral response (SVR)]. In trials involving only zinc supplementation or zinc supplementation in addition to the standard antiviral therapeutics, there was an increased therapeutic response, compared to the placebo group. However, there was lack of positive clinical outcome with Zinc supplementation in four trials of chronic HCV cases (Table 1). In summary, these studies support the therapeutic benefit of zinc supplementation in a subset of viral hepatitis patients.
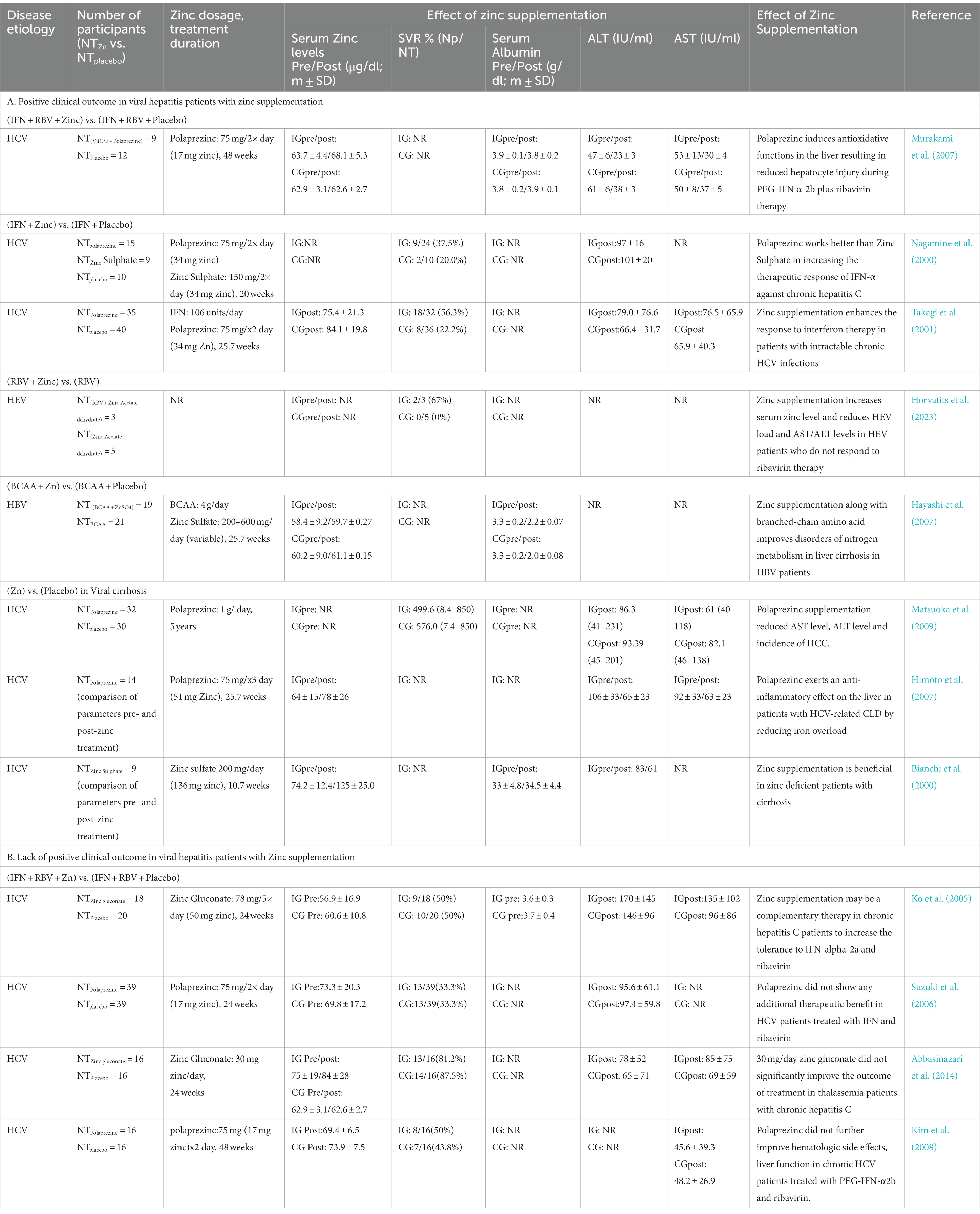
Table 1. Clinical trials on evaluation of therapeutic benefit of zinc compounds in viral hepatitis patients.
Serum zinc levels are decreased in HCV patients and the underlying mechanism is proposed to be due to the requirement of zinc binding by the viral non-structural proteins NS3 and NS5A (Love et al., 1996; Stempniak et al., 1997; Tellinghuisen et al., 2004). Comparison of serum zinc levels in chronic hepatitis C patients before and after treatment with Direct acting antivirals (DAAs) revealed an increase in the serum zinc level after DAA treatment (Suda et al., 2019). Importantly, they showed that the increased zinc level was not attributed to an increase in the albumin level, but it was a direct outcome of the viral RNA clearance (Suda et al., 2019).
Chronic hepatitis due to HCV infection is a known risk factor of HCC. In an interesting study, Hosui et al. evaluated the effect of oral zinc supplementation on the risk of HCC development in DAA treatment-cured chronic hepatitis C suffering individuals (Hosui et al., 2021). One year and three year follow up study after the end of DAA therapy showed cumulative incidence rates of 1.8 and 5.6%, respectively in the no zinc supplemented (control) group. None from the zinc supplemented group developed HCC. Moreover, serum zinc concentration was significantly higher in the no HCC group than the HCC group (Hosui et al., 2021). These data suggest the therapeutic benefit of zinc supplementation in reducing the risk of HCC development in individuals recovered from chronic hepatitis C.
In addition to viral infections, there are other inducers of hepatic dysfunction such as alcohol consumption. Multiple independent clinical trials have been carried out to assess the therapeutic benefit of zinc supplementation in non-viral hepatitis patients with liver cirrhosis (Overbeck et al., 2008; Diglio et al., 2020). Zinc supplementation significantly increased the serum zinc level, reduced the serum albumin level and improved the overall disease condition in those patients, further attesting the therapeutic benefit of zinc in hepatitis patients (Overbeck et al., 2008; Diglio et al., 2020).
2.7. Antiviral effect of zinc compounds in cell culture-based infection/replicon models of hepatitis viruses.
Several independent studies support the antiviral role of zinc on replication and survival of HAV, HCV and HEV (Table 2; Figure 1). All studies used safe dose of zinc, which did not affect the viability of the cells that were used in the experiment. Zinc sulphate partially inhibited the replication of HAV in Huh7 (human hepatoma) cells (Ogawa et al., 2019). Zinc sulphate and zinc chloride inhibit replication of the genomic length HCV RNA at a concentration of 100 μM, with maximum effect at 48 h of treatment (Yuasa et al., 2006). Another study by Gupta et al. compared the HCV inhibitory effect of zinc oxide nanoparticles [ZnO(NP)] and tetrapods [ZnO(TP)] with conventional zinc salts such as ZnSO4, which revealed the superior antiviral potency of the ZnO(TP) against HCV (Gupta et al., 2022). Zinc salts, ZnO(NP) and ZnO(TP) also show antiviral activity against HEV, latter being the most potent (Gupta et al., 2022). ZnO(NP) and ZnO(TP) are nanoparticle conjugated variants of ZnO, which is better absorbed in the intestine, possess better bioavailability and reduced undesirable side effect characteristics (Sirelkhatim et al., 2015; Jiang et al., 2018). Therefore, ZnO(NP) and ZnO(TP) are safer alternatives to the conventional zinc salts for therapeutic use. Inhibitory effect of ZnO(TP) was comparable to that of sofosbuvir, a well-known DAA used in the treatment of HCV cases, further testifying the antiviral potential of ZnO(TP) against HCV (Yuasa et al., 2006; Gupta et al., 2022).
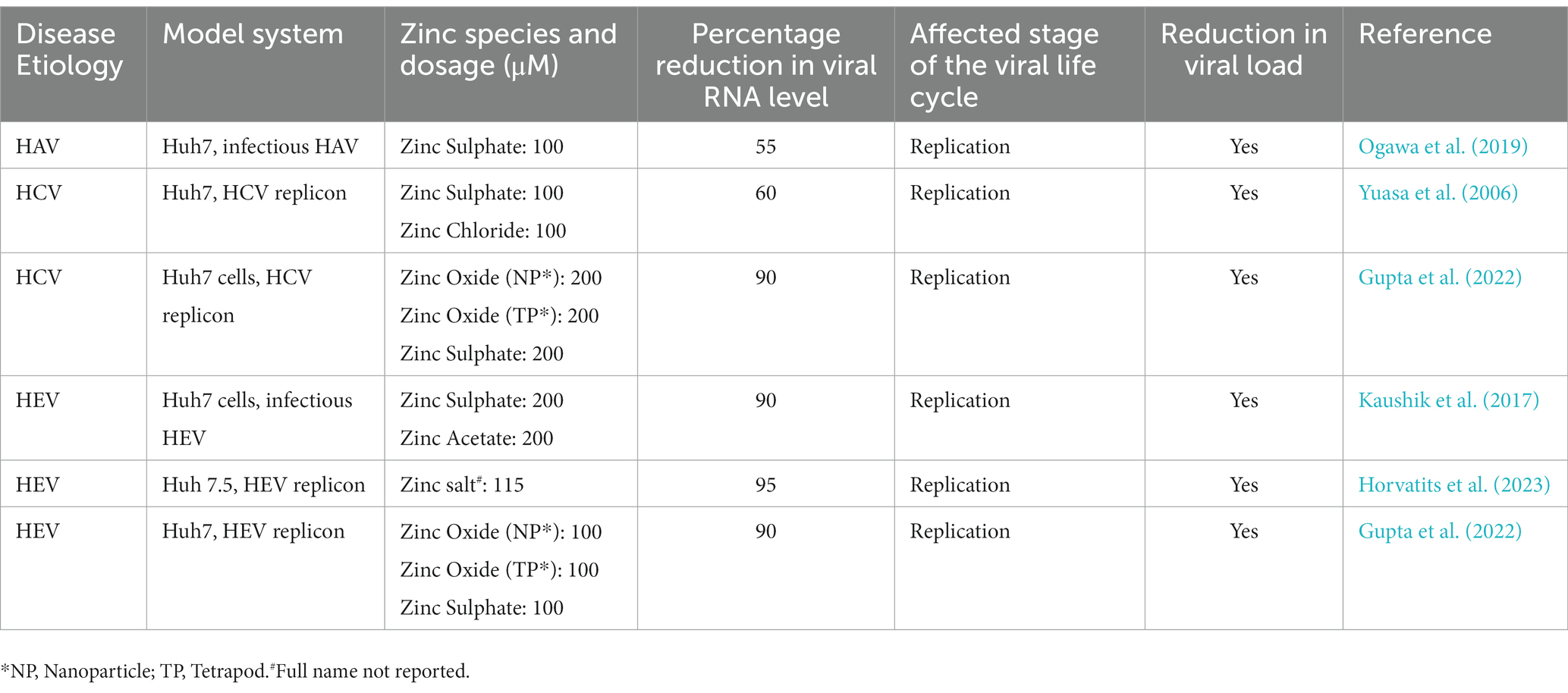
Table 2. Anti-viral effect of zinc on hepatitis viruses in respective cell culture-based infection models/replicon models.
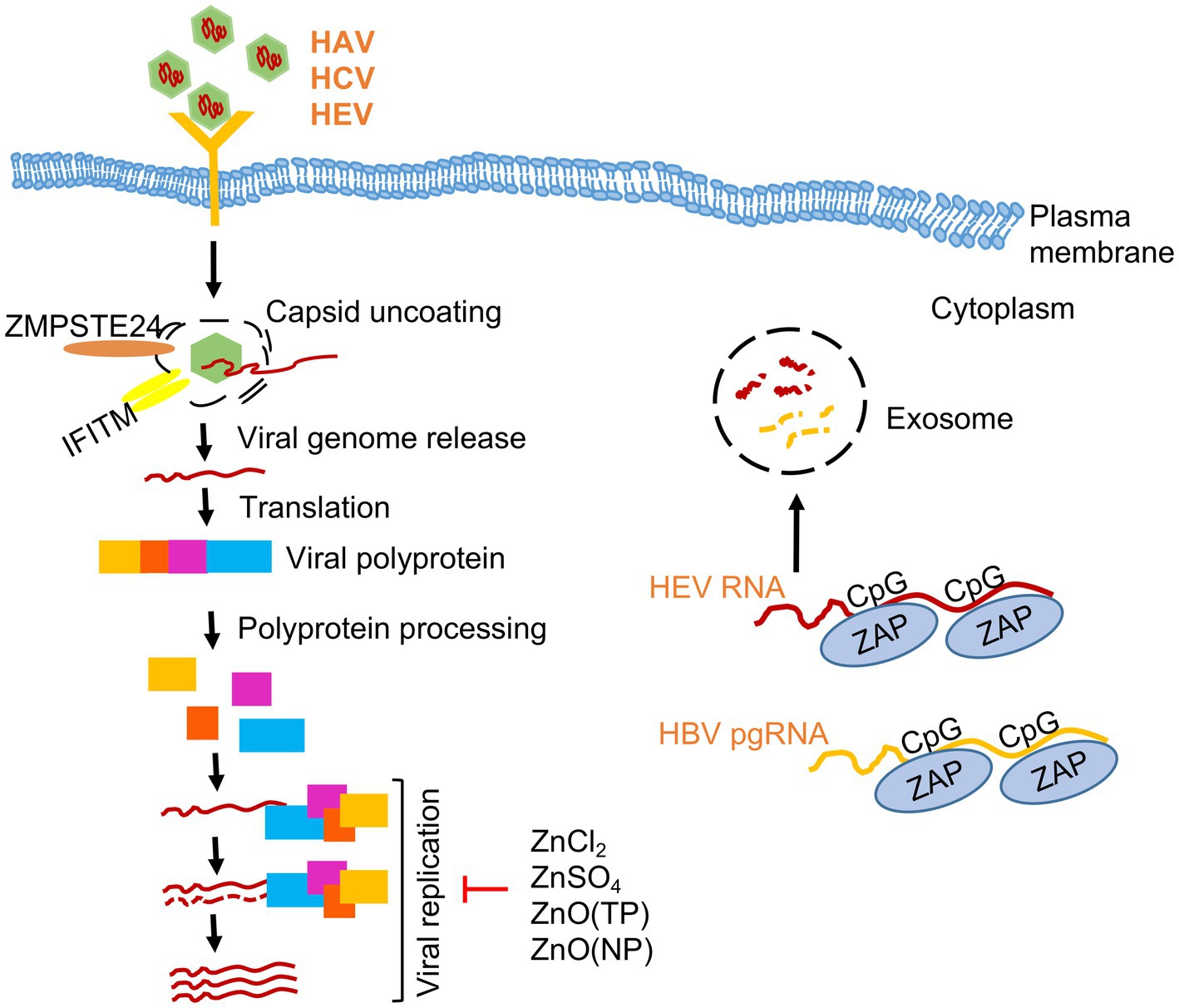
Figure 1. Effect of zinc on hepatitis viruses. A simplified scheme of life cycle of HAV, HCV and HEV is shown. Note that HAV and HEV are quasi-enveloped whereas HCV is an enveloped virus. Zinc salts (ZnCl2, ZnSO4), ZnO nanoparticles (NP) and ZnO tetrapod-shaped nanoparticles (TP) inhibits the replication of Hepatitis A (HAV), Hepatitis C (HCV) and Hepatitis E viruses (HEV). Zinc finger antiviral protein (ZAP) binds to CpG motifs in HEV RNA and HBV pre-genomic (pg) RNA and targets them for degradation.
3. Broad-spectrum antiviral effect of zinc: insight from studies on other viruses
Antiviral effect of zinc have been demonstrated in vivo and in vitro in several viruses, including corona viruses, picornaviruses, papilloma viruses, metapneumoviruses, rhinoviruses, herpes simplex viruses, varicella-zoster viruses, respiratory syncytial viruses, retroviruses, SARS-CoV and SARS-CoV-2 etc. (Bracha and Schlesinger, 1976; Gupta and Rapp, 1976; Polatnick and Bachrach, 1978; Katz and Margalith, 1981; Kümel et al., 1990; Hulisz, 2004; Krenn et al., 2009; Te Velthuis et al., 2010; Liu and Kielian, 2012; Wei et al., 2012; Antoine et al., 2016; Liu et al., 2021; Samad et al., 2021). Based on the available literature, mechanism underlying the antiviral properties of zinc may be broadly classified into two categories: (a) direct inhibitory effect on the different stages of the life cycle of the virus and (b) indirect effect of zinc attributed to its ability to modulate various host cellular processes and immune response. Zinc shows direct inhibitory action against several viruses. It acts by interfering with different steps of the viral life cycle, it inhibits the activity of key viral proteins and competes with other bivalent ions such as manganese, magnesium or calcium to interrupt the function of viral proteins. Direct antiviral activity of zinc against viruses is schematically illustrated in Figures 2, 3.
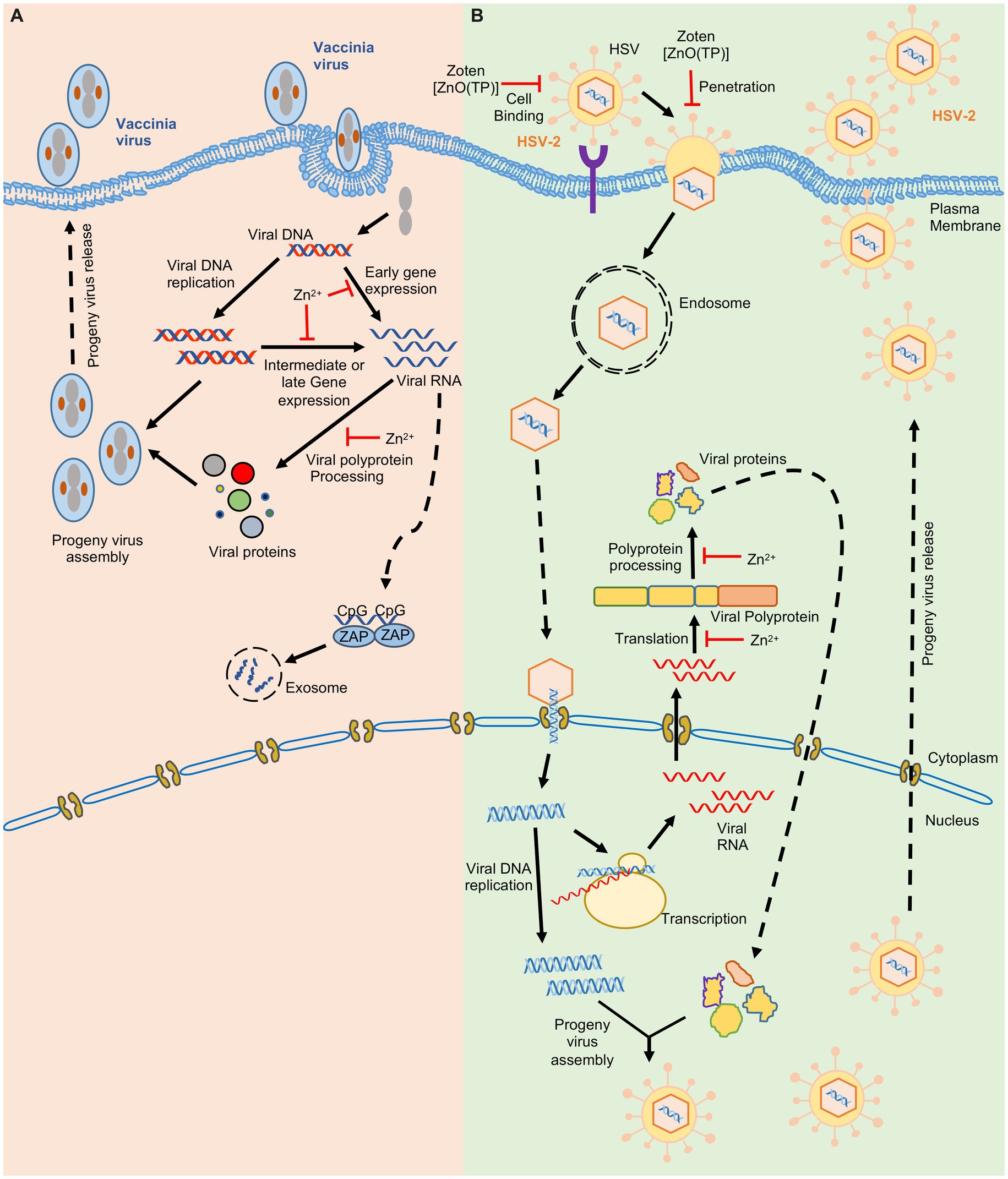
Figure 2. Effect of zinc on Vaccinia and Herpes simplex virus-2. Schematic showing the life cycle of Vaccinia virus (A) Herpes Simplex virus-2 (B). ‘’ indicates the steps inhibited by zinc.
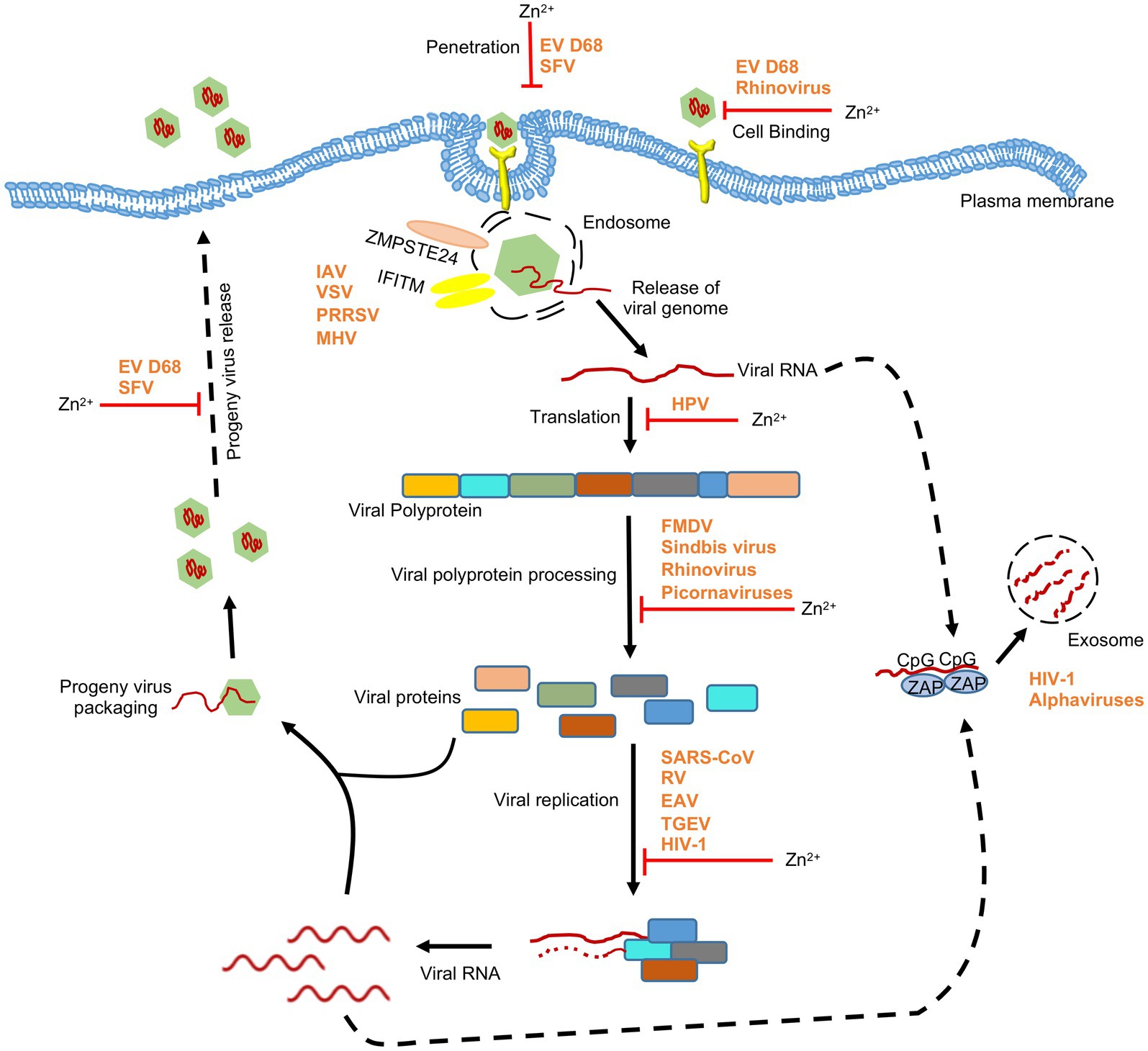
Figure 3. Effect of zinc on RNA viruses. Schematic showing the effect of zinc on life cycle of RNA viruses. Apart from directly inhibiting different stages of viral life cycle, zinc mediates its antiviral activity through zinc-containing proteins such as ZAP and ZMPSTE24. ZAP binds to CpG motif in viral RNA and targets them for exosomal degradation. ZMPSTE24 and IFITM complex interferes with entry of viruses. EV D68, enterovirus D68; HPV, human papilloma virus; RSV, respiratory syncytial virus; TGEV, transmissible gastroenteritis virus; SFV, Semliki forest virus; SARS-CoV, severe acute respiratory syndrome coronavirus; FMDV, foot and mouth disease virus; HIV-1, human immunodeficiency virus-1; IAV, influenza A virus; VSV, vesicular stomatitis virus; PRRSV, porcien reproductive and respiratory syndrome virus; MHV, mouse hepatitis virus. ‘’ indicates the steps inhibited by zinc.
3.1. Effect of zinc on infectivity of the virus and target cell entry
Zinc may directly accumulate on the virus and inactivate it or interfere with its entry into the target cell. Zinc application leads to its deposition on HSV thereby inactivating the virus and inhibiting its cellular entry (Kümel et al., 1990). Recently zinc Oxide tetrapod nanoparticles with engineered oxygen vacancies (Zoten) were shown to possess potent therapeutic benefit in HSV-2 (Herpes simplex virus-2) mediated genital herpes (Antoine et al., 2016). Zoten blocks cellular entry of HSV-2 by efficiently trapping and inactivating the virus, thereby preventing the disease (Figure 2). It also enhances T cell and antibody mediated immunity in mice, have adjuvant like properties and thus reduces chances of reinfection (Antoine et al., 2016; Samad et al., 2021). Zinc treatment was shown to moderately inhibit enterovirus D68 attachment and entry into target cells (Figure 3; Liu et al., 2021). In the case of Rhinovirus, zinc may act as a competitive inhibitor of virus binding to the ICAM1 (intercellular adhesion molecule 1) on the host cell surface, which is the receptor for virus entry (Hulisz, 2004; Figure 3). Zinc and Nickel inhibit membrane fusion of SFV (Semliki forest virus) by targeting the viral transmembrane E1 protein (Figure 3; Liu and Kielian, 2012).
3.2. Effect of zinc on viral protein translation and polyprotein processing
Zinc inhibits proteolytic processing of nonstructural polyproteins of several viruses such as rhinovirus and Picornavirus (Krenn et al., 2009). Zinc ionophores such as Pyrithione and Hinokitol also demonstrate antiviral activity by inhibiting the processing of picornavirus nonstructural polyprotein (Krenn et al., 2009). Zinc treatment inhibits HSV-2, Sindbis, FMDV (Foot and mouth disease virus) and Vaccinia virus growth in infected cells by blocking their polypeptide processing (Figures 2, 3; Bracha and Schlesinger, 1976; Gupta and Rapp, 1976; Polatnick and Bachrach, 1978; Katz and Margalith, 1981).
3.3. Effect of zinc on viral replication and transcription
Replication of viral genome is an essential step for proliferation and maintenance of genomic integrity of the virus. RNA dependent RNA polymerase (RdRp) produced by proteolytic processing of the viral nonstructural proteins plays the central role in the viral replication process. Zinc inhibits RdRp activity of many viruses, including TGEV (Transmissible gastroenteritis virus), SARS-CoV (Severe acute respiratory syndrome coronavirus), EAV (Equine arteritis virus), Rhinovirus and HEV (Hepatitis E virus; Korant et al., 1974; Hung et al., 2002; Te Velthuis et al., 2010; Wei et al., 2012; Kaushik et al., 2017). Different steps in the replication process have been shown to be targeted by zinc for inhibiting RdRp activity. In case of SARS-CoV, zinc treatment reduced template binding and elongation by the RdRp whereas in case of EAV, initiation step of RNA synthesis was inhibited (Te Velthuis et al., 2010). Zinc also inhibits Rhinovirus RdRp activity in vitro although the mechanism remains to be understood (Korant et al., 1974; Hung et al., 2002). Clinical trials have shown the therapeutic benefit of zinc in alleviating rhinovirus induced common cold symptoms (Eby et al., 1984; Hulisz, 2004; Kurugöl et al., 2006). Zinc inhibits HIV-1 (Human immunodeficiency virus-1) protease and reverse transcriptase activity (Zhang et al., 1991; Haraguchi et al., 1999; Fenstermacher and DeStefano, 2011). Some other HIV-1 encoded proteins are dependent on zinc to carry out their function (Zheng et al., 1996). Effect of zinc supplementation in HIV infected patients have been investigated in clinical trials (Mocchegiani and Muzzioli, 2000; Bobat et al., 2005; Baum et al., 2010). HIV infected children showed a significant decrease in the frequency of watery diarrhea after 3 months of zinc supplementation. However, neither viral load was altered nor CD4+ T lymphocytes level was improved (Bobat et al., 2005). Similar effect of zinc supplementation was observed in HIV infected patients having pneumocystis carinii and candida (Mocchegiani et al., 1995). Another study has shown potent antiviral activity of PEGylated ZnO nanoparticle against H1N1 influenza virus (Ghaffari et al., 2019). Recently, polyamide fibers with embedded zinc ions (zinc oxide) were shown to prevent and deactivate Influenza A virus H1N1 and SARS-CoV-2 (Gopal et al., 2021). Further, higher zinc intake was found to reduce the severity of disease in COVID-19 patients (Asoudeh et al., 2023). In addition, many clinical trials have attempted to evaluate the therapeutic benefit of zinc in COVID-19 patients (Carlucci et al., 2020; Chinni et al., 2021; Gordon and Hardigan, 2021; Thomas et al., 2021; Beran et al., 2022; Tabatabaeizadeh, 2022). Though some studies reported a positive outcome, further investigation is warranted to draw a clear conclusion.
Zinc is also reported to act by inhibiting viral particle production and inhibit viral topoisomerase activity in vaccinia virus, inhibit endosomal membrane fusion in Semliki Forest virus and inhibit viral protein E6 and E7 synthesis (thereby stimulating apoptosis) in Human papilloma virus infected cells (Read et al., 2019).
4. Antiviral function of zinc-containing host proteins
Zinc finger antiviral protein (ZAP) is a well characterized host protein that recognizes the CpG dinucleotide present in RNA and targets them for degradation through exosome (Guo et al., 2007; Gonçalves-Carneiro et al., 2022). Since CpG containing RNA is not produced in human, ZAP efficiently targets viral RNA, justifying its antiviral property. Human ZAP contains four zinc finger motifs, located at the N-terminus. Zinc finger motifs mediate its interaction with CpG RNA and mutation of some cysteine residues in the zinc finger motif of ZAP result in loss of its antiviral activity (Guo et al., 2004). Structural studies have clearly demonstrated the role of zinc finger motif of ZAP in mediating its antiviral function (Chen et al., 2012). ZAP also associates with triphosphate motif-containing protein 25 (TRIM25, an E3 ubiquitin ligase), which acts as a co-factor of ZAP and supports its antiviral function (Zheng et al., 2017). P72 RNA helicase (a DEAD box family RNA helicase) also associates with ZAP and helps in its antiviral function (Chen et al., 2008).
ZAP has been shown to inhibit HIV-I by targeting multiple viral mRNA for degradation (Zhu et al., 2011). ZAP inhibits alphaviruses by targeting the CpG dinucleotides in the NSP2 region containing RNA (Nguyen et al., 2023). ZAP inhibits human cytomegalovirus by targeting its UL4/UL5 transcripts (Gonzalez-Perez et al., 2021). In a recent study, Yu et al. demonstrated that expression of ZAP and IFN-β was significantly reduced upon HEV infection (Yu et al., 2021). ZAP was shown to interact with the 5’UTR region of the HEV genome. Knockdown of ZAP decreased phosphorylation of IRF3, thus limiting host innate immune system, while poly(I:C) induction in cells upregulated IRF3 phosphorylation and ZAP, thus inhibiting HEV replication (Yu et al., 2021). Hence ZAP shows antiviral activity against HEV. ZAP also shows antiviral activity against HBV by interacting with the HBV pgRNA (pre-genomic RNA) and targeting it for degradation (Mao et al., 2013).
Considering the pattern and specificity of ZAP binding to CpG RNA, it is expected that ZAP acts as a broad spectrum antiviral factor that acts by targeting the virus while retaining resistance to evolving mutations in the viral genome. Unless the virus encodes a specific mechanism to antagonize CpG RNA binding property of ZAP or deplete ZAP or its cofactors, it is unlikely to escape the antiviral activity of the ZAP. On top of that ZAP is also reported to stimulate the RIG-I signaling pathway, which is a major antiviral response mechanism of the host (Hayakawa et al., 2011).
ZMPSTE24 is another host zinc finger motif containing protein, which shows antiviral activity against many enveloped viruses, including influenza virus, Vesicular stomatitis virus (VSV), Vaccinia virus, Porcine reproductive and respiratory syndrome virus (PRRSV) and arenaviruses (Fu et al., 2017; Katwal et al., 2022; Stott-Marshall and Foster, 2022). A recent report demonstrates that ZMPSTE24 inhibits infection of SARS-CoV-2-spike pseudotyped lentivirus, suggesting its antiviral function against the SARS-CoV-2. A similar phenomenon was also observed in the case of the mouse hepatitis virus (MHV; Shilagardi et al., 2022). ZMPSTE24 acts by interacting with interferon-inducible membrane proteins (IFITM) and preventing fusion of the viral envelope (Fu et al., 2017; Li et al., 2017).
5. Effect of zinc on host
5.1. Maintenance of zinc homeostasis by metallothioneins and zinc transporters
Metallothionein (MT) is a cysteine rich low molecular weight protein, which binds to zinc and copper to regulate their homeostasis in cells and also sequester heavy metals such as cadmium and mercury to alleviate heavy metal poisoning and superoxide stress. There are four MT isoforms in mice (MT1-4) and several isoform/variants in human (Vašák, 2005). MT1 and MT2 are expressed in all organs, while MT3 is expressed in brain and MT4 in stratified tissues. About 10% of human genome encode zinc binding proteins which play crucial biological functions. The availability of zinc is regulated by MT and zinc transporters. MTs sense the intracellular zinc level and modulate zinc through sequestration, distribution and release. Promoter region for MT1 and MT2 contains several metal and glucocorticoid regulatory elements (MREs and GREs). Metal responsive transcription factor 1 (MTF-1) regulates the transcription of MTs (Figure 4; Vašák, 2005). MTF-1 contains six zinc fingers which is responsible for DNA binding, and thus binds to the promoter proximal MREs. Increased Zinc concentration mediate efficient DNA binding of MTF1 (Grzywacz et al., 2015). Another study has shown that during cellular stress, Nitric Oxide (NO) produced by immune cells induce MT1 and MT2 to release zinc and these free zinc ions bind to MTF1, leading to its activation (Stitt et al., 2006). MTs mobilize zinc to nucleus, cytoplasm, golgi and endoplasmic reticulum. MTs also interact with proteins such as GTP, ATP, Gluthatione and these interactions enable their localization in extracellular milieu (Maret, 1994; Jiang et al., 1998; Subramanian Vignesh and Deepe, 2017).
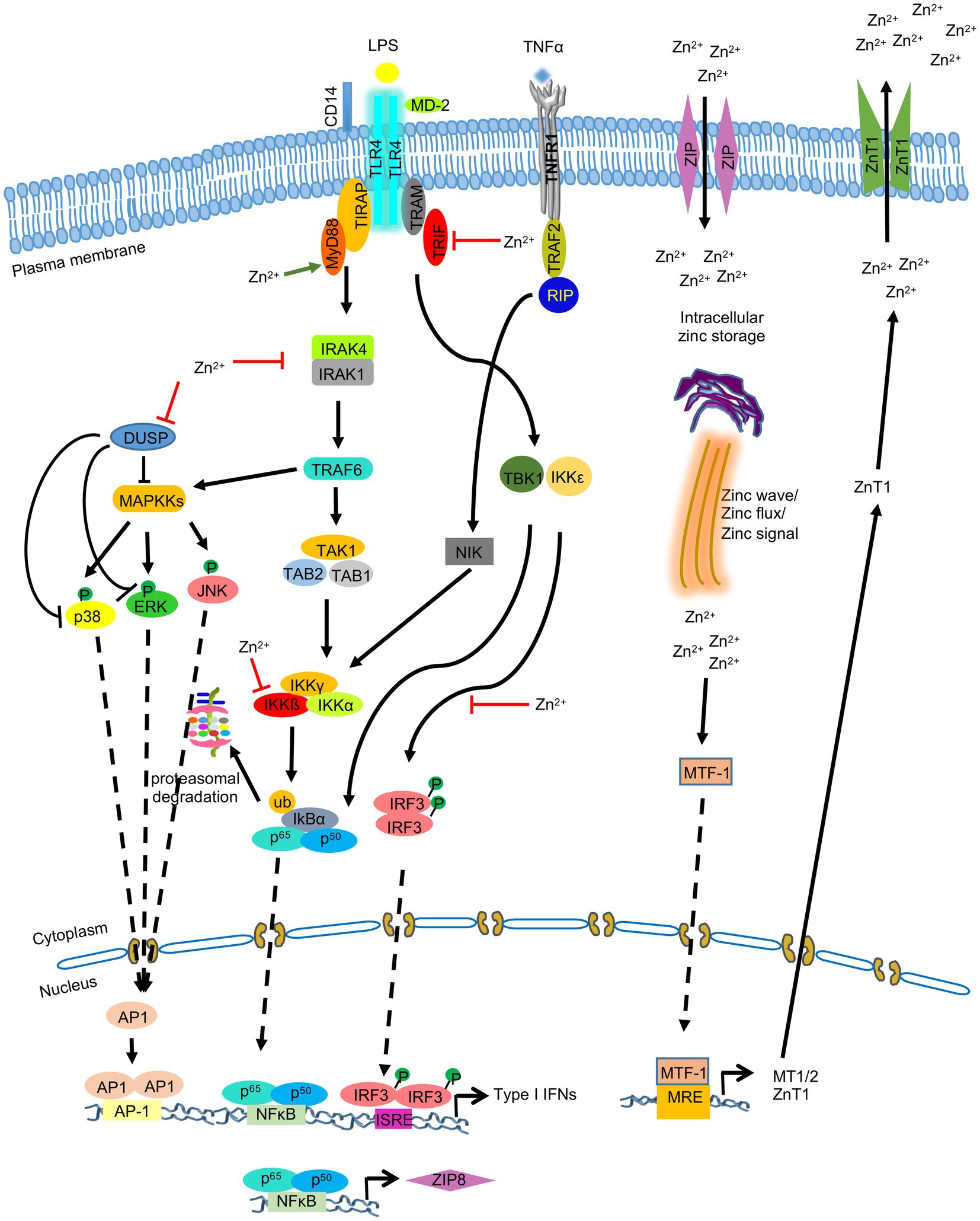
Figure 4. Zinc-Metallothionein homeostasis and zinc signaling in monocytes, macrophages and dendritic cells. LPS mediated stimulation of TLR4 leads to activation of NFκB, IRF3, and MAPK signaling pathways, resulting in production of type I IFNs and ZIP8. Zinc mobilization via ZIP8 increases intracellular zinc level, which acts on MAPK, NFκB, and IRF3 signaling pathways by inhibiting dual specificity phosphatase (DUSP) and by inhibiting IKKβ and IRF3 phosphorylation. Metal Responsive element-binding transcription factor-1 (MTF-1) promotes the synthesis of metallothioneins (MT), which in turn binds to and translocates zinc into organelles. MTF-1 also activates the synthesis of ZnT1, which exports zinc out of the cell. ‘’ indicates the steps inhibited by zinc.
It has been postulated that MTs deliver zinc to the thymulin, which is important for the function of the latter and that aberrant MT regulation can have direct effect on downstream functions of thymulin such as T cell selection, differentiation and function (Subramanian Vignesh and Deepe, 2017). Zinc also regulates the expression of MHC II on dendritic cell (DC) surface. Excess zinc reduces MHC II expression while zinc deficiency elevates MHC II expression on DCs. Thus, it has been proposed that MT-Zn sequestration regulates MHC II expression in DCs, further influencing thymic T cell selection (Kitamura et al., 2006; George et al., 2016).
Several studies also suggest that MT-Zn homeostasis regulates immunological function of bone marrow. Reports have shown that either deficiency of dietary zinc and chronic zinc exposure leads to B-cell and T-cell apoptosis (King et al., 1995; Fraker and King, 2004). Thus, absence of MT expression in bone marrow diminishes zinc homeostasis unless compensatory zinc transporters and MTs are provided. MTs transfer zinc to other metalloproteins including the zinc-dependent transcription factor which regulates the differentiation of precursor cells in bone marrow. For example, Early growth response-1 (Egr-1), a zinc-dependent transcription factor promotes differentiation of monocyte into macrophage (Krishnaraju et al., 1995), while another zinc dependent transcription factor growth factor independent-1 (Gif-1) antagonizes monocyte to macrophage differentiation and rather promotes neutrophil differentiation (Hock et al., 2003). Studies have also shown that exogenously added Zn-MT binds to unknown MT receptor on T-Cell membrane and reduces surface thiol expression. This enhances IL-2 release which promotes T-Cell survival and proliferation (Subramanian Vignesh and Deepe Jr., 2017).
Dendritic cells express MTs in response to thermal stress, which then mediate zinc distribution to regulate intracellular redox environment in DCs. MT1 expressed in DCs induces tolerogenic potential of DCs by promoting differentiation of naïve T cell into FOXP3 expressing Treg cells (Reis e Sousa, 2006; Maldonado and von Andrian, 2010). During inflammation and changed redox state of cells, MTs release zinc. These free zinc ions activate MTs, stimulate MTF-1 and downregulates pro-inflammatory cytokines such as IL6, TNF-α, interleukin IL-1 and also suppresses transcription factor NF-kB. NF-kB induces the expression of pro-inflammatory cytokines 1 L-1, IL-6, TNF- α, which activates MTF-1 transcription factor. MTF-1 upregulates the expression of MTs, and zinc efflux transporter ZnT-1, which helps in maintaining Zn-MT homeostasis and helps recover cells from redox state (Figure 4; Grzywacz et al., 2015).
Zinc transporters play important roles in Zn2+ transport, distribution and homeostasis (Lazarczyk and Favre, 2008; Lichten and Cousins, 2009). Zinc transporters belong to two families: 10 SLC30s/ZnTs and 14 SLC39s/ZIPs. ZIPs mediate influx of Zn2+ from extracellular space to intracellular cytoplasm via diffusion, symporter or secondary active transporter. ZnTs export Zn2+ from cytoplasmic space to extracellular space (Andrews et al., 2004; Lichten and Cousins, 2009). The ZnTs and the ZIPs with their expression and distribution in the different tissues are listed in the Table 3. Inside the cell, specific set of ZnTs and ZIPs are involved in storage of Zn2+ in organelles or its release into cytosol, depending on the state of the cell. Intracellular distribution of ZnTs and ZIPs is schematically shown in Figure 5.
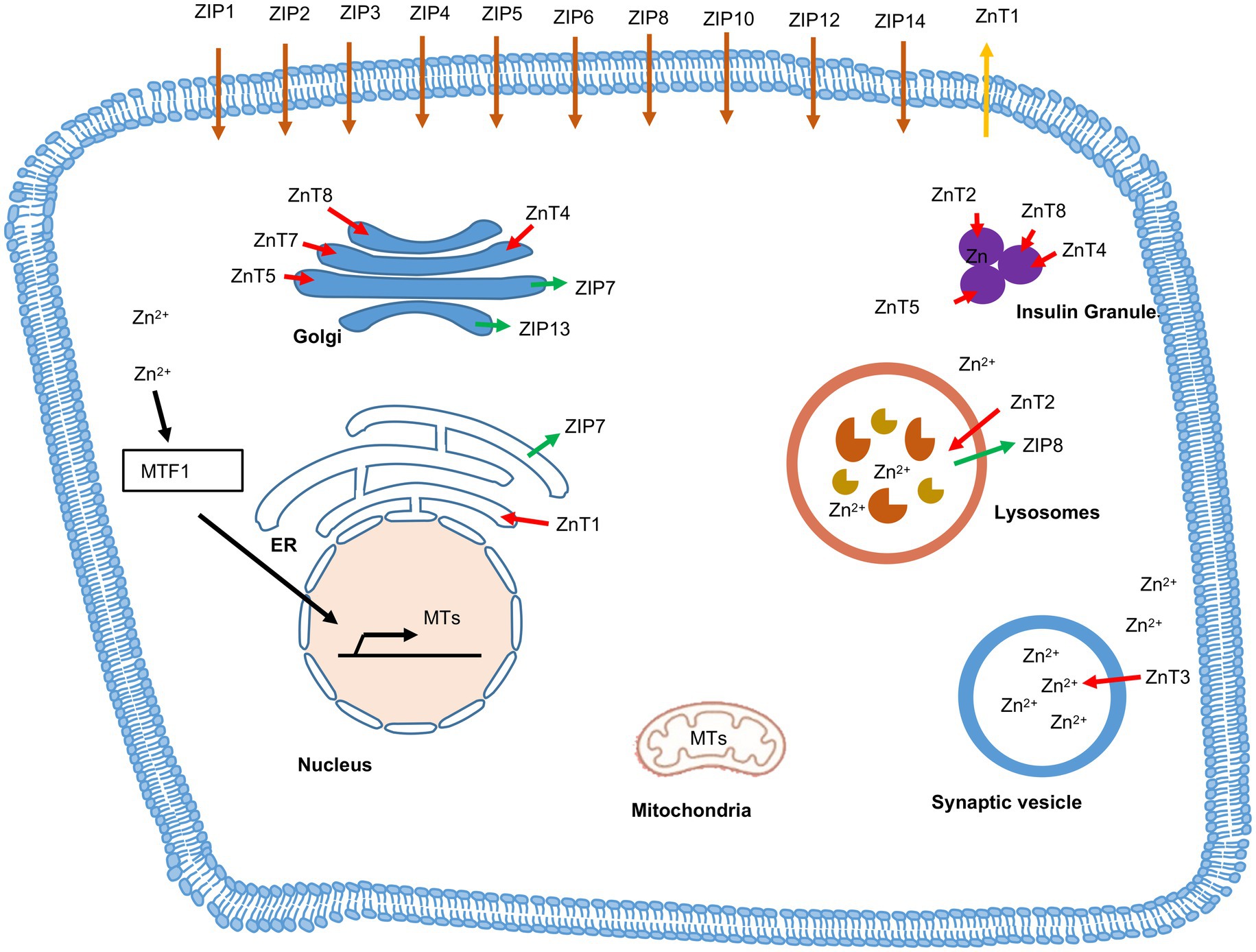
Figure 5. Subcellular localization of Zinc transporters (ZnTs), Zrt- and Irt- like proteins (ZIPs) and Metallothionein (MT). Red arrow indicates the flow of Zn2+ from cytosol to cellular organelles via ZnTs and green arrow indicates the flow of Zn2+ from cellular organelles to cytosol via ZIPs. Brown arrow indicates the ZIPs located on plasma membrane, which transports zinc inside the cytosol. Orange arrow indicates the ZnTs located on plasma membrane, which transports zinc outside the cell. MTs translocate zinc into nucleus, golgi, endoplasmic reticulum (ER), mitochondria and lysosomes.
Through sequence analysis it was observed that the ZIP family of transporters contains 8 transmembrane domains (TMDs-1-8). These 8 transmembrane domains are responsible for the zinc transport. A cytoplasmic segment lies between the 3rd and 4th TMD (Taylor and Nicholson, 2003; Bin et al., 2011). These proteins are further divided into four subfamilies- I, II, gufA, and LIV-1 (Taylor and Nicholson, 2003). Amongst these, the LIV-1 sub family members contain a HEXXH motif within TM5 (transmembrane domain 5) and varying lengths of the N-terminal extracellular domain (ECD; Taylor and Nicholson, 2003). Out of the 14 ZIPs identified in mammals, ZIP1, ZIP2, ZIP3 belong to the ZIP II subfamily. ZIP 9 is a member of the ZIP I subfamily and ZIP 11 is of the gufA subfamily (Yu et al., 2013). The remaining 9 ZIPs belong to LIV-1 subfamily (Ryu et al., 2020). The functional roles of LIV-1 subfamily proteins are predominantly due to their ECDs. The role of the ZIP-CTD present between TM3 and TM4 remains unknown (Bin et al., 2018).
The transport mechanisms being used by ZIPs is not yet clearly elucidated and requires further study, but the cell-based assays done using isotopes suggest that ZIP2 controls the zinc flux through a time, temperature and concentration dependent manner as seen in erythroleukemia cells (Gaither and Eide, 2000). It was further seen that ZIP2 was stimulated only by HCO3-, with a high affinity to zinc, suggesting a Zinc- HCO3- symporter mechanism (Gaither and Eide, 2000). ZIP8 has also been shown to act as a Zn- HCO3− symporter in complementary RNA injected Xenopus oocytes (Liu et al., 2008).
ZnTs are part of a superfamily of cation diffusion facilitators. Mammalian ZnTs are predicted to have at least 6 TMDs (Fukada and Kambe, 2011). The ZnTs possess a histidine/serine rich loop of differing lengths between the 4th and 5th TMDs (Fukada and Kambe, 2011). Further ZnTs contain a large cytoplasmic domain at the C- terminus (CTD) with a copper chaperone like architecture (Lu and Fu, 2007). This domain has an important role in diabetes research as mutations in the CTD of ZnT8 increases the risk of developing diabetes (Parsons et al., 2018). The transport mechanism utilized by ZnTs is not clearly understood in the mammalian system, but studies done on E. coli Zinc transporter YiiP suggests that ZnTs control the zinc efflux through a Zn2+/H+ antiporter (Lu and Fu, 2007).
Zinc is an essential component of several cellular processes in the host. It is also essential for normal development and functioning of the innate and adaptive immune systems. The diverse functional properties help in further strengthening the antiviral action of zinc, either by stimulating a better immune response in the host and/or by promoting the synthesis/activation of antiviral factors/pathways, as described in the following sections.
5.2. Modulation of host immune system by zinc
5.2.1. Zinc signaling in monocytes, macrophages, and dendritic cells
There is a reduction in phagocytosis of macrophages, decrease in chemotaxis of polymorphonuclear cells and decrease in the production of proinflammatory cytokines upon zinc deficiency (Rink and Kirchner, 2000; Bonaventura et al., 2015). Upon entering the host, pathogens are recognized by the pattern recognitions receptors (PRRs) such as Toll-like receptors (TLRs), which initiate different signaling cascades, leading to production of host factors essential for survival.
Except TLR3, activation of all other TLRs by various ligands increases intracellular Zn2+ level, which inhibits the phosphorylation of IRF3 in murine macrophages, leading to reduced production of type I interferons such as IFNβ. Conversely, zinc deficiency increases the level of LPS-induced IFNβ (Haase et al., 2008; Brieger et al., 2013; Figure 4). Thus, increased Zn2+ level negatively regulates TRIF/TRAM-dependent signaling pathway (Figure 4). Increased Zn2+ level also activates MAP kinases (mitogen activated protein kinases) in LPS-treated macrophages in a IRAK-TRAF-dependent manner, leading to production of proinflammatory cytokines. Zinc mediated inhibition of MAPK phosphatases such as the dual-specificity phosphatases (DUSPs) and degradation of IRAK1 is proposed to control the effect of zinc on MAPKs (Haase et al., 2008; Wan et al., 2014; Figure 4). Further, zinc modulates MyD88-dependent activity of the transcription factor NFκB. Hasse et al. reported that zinc depeletion reduced LPS-induced phosphorylation of IKKβ in monocytes and reduced DNA binding by NFκB (Haase et al., 2008). However, few other studies reported inhibition of NFκB activity by zinc (Von Bülow et al., 2007; Haase and Rink, 2009; Prasad et al., 2011). Subsequently, it was found that zinc transporter ZIP8 was a target of NFκB (Liu et al., 2013). NFκB mediated upregulation of ZIP8 levels further increases intracellular Zn2+ level, which in turn inhibits IKKβ phosphorylation, leading to the inhibition of NFκB activity. Zinc also inhibits the activity of phosphodiesterase (PDE) in monocytes, leading to the inhibition of NFκB activity (Von Bülow et al., 2007).
5.2.2. Zinc signaling in T cells
Multiple studies have investigated the effect of zinc on T cells, which has been elegantly reviewed by Kim and Woo Lee (Kim et al., 2021). In brief, intracellular Zn2+ level is high in activated T cells. ZIP6 and ZIP8 are the predominant zinc transporters present in T cells. Upon TCR stimulation, ZIP6 mediates Zn2+ influx and loss of ZIP6 impairs T cell activation (Colomar-Carando et al., 2019). Subsequently it was found that Src and/or Syk family kinase ZAP70 mediated phosphorylation of ZIP6 was essential for localization of the latter to the immunological synapse and Zn2+ influx upon TCR stimulation (Kim et al., 2021). Zn2+ influx also inhibits SHP-1 mediated dephosphorylation of the LCK, enabling increased phosphorylation of ZAP70 kinase by LCK at the immunological synapse (Chiang and Sefton, 2001; Štefanová et al., 2003; Figure 6). Note that Zn2+ also facilitates the binding of LCK to CD4 and CD8α, which is important for TCR signaling (Lin et al., 1998).
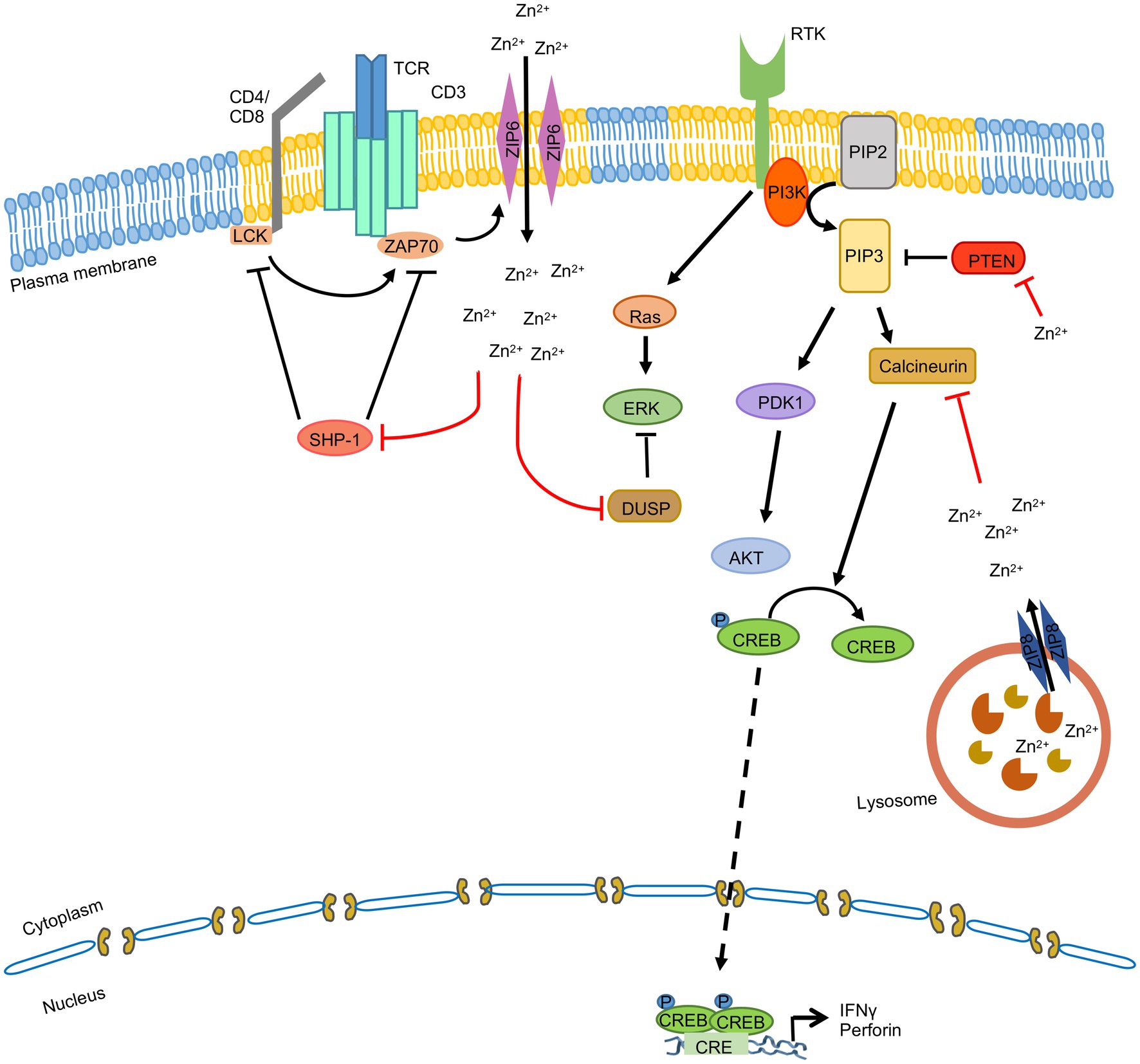
Figure 6. Zinc signaling in T cells. TCR stimulation induces ZAP70 mediated phosphorylation of ZIP6, leading to its localization to the immunological synapse in lipid rafts (yellow). ZIP6 mediates Zn2+ influx, which inhibits SHP-1 mediated dephosphorylation of the LCK and DUSP mediated dephosphorylation of the MAPKs. ZIP8 is present on the lysosome and it mobilizes lysosomal Zn2+ to cytoplasm, which inhibits Calcineurin mediated dephosphorylation of CREB, leading to CREB mediated transcriptional upregulation of IFNγ and perforin. ‘’ indicates the steps inhibited by zinc.
On the other hand, ZIP8 is predominantly localized to the lysosome and it mobilizes lysosomal Zn2+ to cytoplasm. ZIP8 expression is increased upon TCR stimulation, leading to increased translocation of Zn2+ from lysosome to cytoplasm, which inhibits the phosphatase activity of Calcineurin. This leads to CREB (cyclic AMP response element-binding protein) mediated transcriptional upregulation of IFNγ and perforin gene expression, which are key antiviral effectors of the host (Aydemir et al., 2009; Figure 6). Besides, Zn2+ is also known to modulate the activity of ERK, PI3K and STAT3 signaling pathways in T cells, thereby influencing production of proinflammatory cytokines and differentiation of Th17 cells (Kaltenberg et al., 2010; Kitabayashi et al., 2010; Plum et al., 2014).
5.2.3. Zinc signaling in other immune cells
Zinc deficiency reduces natural killer cell cytotoxic activity and impairs host immune response against foreign pathogens, while zinc supplementation can reverse this effect (Fernandes et al., 1979; Fraker et al., 1982). Role of ZnTs in mast cell activation and mast cell mediated allergic reaction has been reported, indicating that ZnT5 mediates FcεRI signaling which leads to activation and translocation of PKC to plasma membrane. PKC stimulates nuclear translocation of NF-κB and cytokine production in mast cells (Nishida et al., 2009). Zinc has also been shown to increase the production of IFNα in leucocytes (Samad et al., 2021). Zinc transporters ZIP7 and ZIP 10 are essential for B cell development and BCR-induced B cell proliferation (Hojyo et al., 2014; Anzilotti et al., 2019).
6. Possible mechanism (s) controlling the antiviral function of zinc in hepatitis viruses
Exact molecular mechanism of antiviral function of zinc against any particular hepatitis virus has not been conclusively demonstrated. Nevertheless, information obtained from several independent studies strongly support the benefit of zinc action against HAV, HBV, HCV, HEV and provide a scientific basis for further investigation. Here, we attempt to extrapolate the possible mechanism (s) (based on information obtained from studies on direct and/or indirect antiviral effect of zinc on all viruses), which enable zinc to antagonize HAV, HBV, HCV and HEV infection and suggest future directions for experimental validation of those possibilities. It is important to obtain clear mechanistic understanding of zinc action in order to harness its complete therapeutic potential for management of viral hepatitis.
As mentioned in previous sections, antiviral effect of zinc against HCV has been evaluated in multiple clinical trials, which provides an overall conclusion that zinc supplementation have therapeutic benefit when taken along with standard antiviral therapy. In vitro, zinc shows potent inhibitory effect on HCV RdRp, although its mechanism of action remains to be explored (Yuasa et al., 2006; Gupta et al., 2022). Recently, our laboratory showed antiviral activity of ZnO nanoparticles [ZnO(NP)] and tetrapods [ZnO(TP)] against genotype 3a-HCV replicon (Gupta et al., 2022). It is possible that zinc acts by chelating magnesium ions, which is required for HCV RdRp activity or acts through other steps in controlling RdRp function (Ouirane et al., 2019).
Zinc was also shown to prevent with IFN-λ3 binding to its receptor IFNLR1, resulting in inhibition of IFN-λ3 signaling, consequently leading to an increase in HCV replication. Single nucleotide polymorphisms rs12979860 and rs809917 in IFNL gene locus clears HCV as well as inflammation and fibrosis progression in viral and non-viral liver disease (Read et al., 2017). Further studies on HCV patients with rs12979860 and rs809917 SNPs should clarify the therapeutic benefit of zinc supplementation in HCV patients.
Previous studies done in our laboratory showed the antiviral activity of zinc against genotypes 1 (g1) and 3 (g3) of HEV, which are major cause of HEV-induced hepatitis in human. Zinc inhibits the activity of HEV RNA-dependent RNA polymerase (RdRp), in vitro (Kaushik et al., 2017). Similar antiviral activity was also observed in cell-based models of g1- and g3-HEV, upon treatment with ZnO nanoparticles [ZnO(NP)] and tetrapods [ZnO(TP)] (Gupta et al., 2022). In agreement with the in vitro data, a recent report demonstrated the anti-HEV activity of zinc in ribavirin nonresponsive HEV patients (Horvatits et al., 2023).
A recent report suggests that the anti-HEV activity of zinc is mediated by its effect on host. Overexpression of Zinc-finger antiviral protein (ZAP), an interferon (IFN)-stimulated gene, inhibits HEV replication, while its knockdown by RNA interference significantly increases HEV RNA level. Silencing of ZAP also decreases interferon regulatory factor 3 (IRF3) phosphorylation in HEV infected cells. Thus, ZAP is an anti-HEV host factor, which blocks viral replication in cooperation with IFN-β (Yu et al., 2021).
An analysis of the protein–protein interactions between human and HEV proteins revealed enrichment of proteins linked to the mitochondrial oxidative phosphorylation pathway (Chandru et al., 2018). It is known that zinc wave results in production of the mitochondrial reactive oxygen species (ROS; Slepchenko et al., 2016). Future study should clarify the involvement, if any, of mitochondrial oxidative phosphorylation pathway in modulating the anti-HEV activity of zinc. Additionally, it has been shown that HEV encoded proteins have an impact on a number of cellular pathways, which may be crucial for survival of the virus inside infected cells (Wißing et al., 2021). Zinc may exert its antiviral effects by interfering with the interplay between HEV and the host signaling pathways.
HEV infection also triggers neuronal disorders such as neurological amyotrophy and Guillain-Barrre syndrome (Jha et al., 2021). HEV infects neuronal cells in culture (Drave et al., 2016). Zinc acts as a neurotransmitter, modulates intracellular and extracellular signaling, which is essential for maintaining normal neuronal physiology (Frederickson et al., 2000). Variations in intracellular level of free zinc decide between survival or death of neurons during ischemic injury (Aras and Aizenman, 2011; Slepchenko et al., 2017). Thus, zinc supplementation might act in a different manner in HEV infected neurons than hepatocytes.
Two studies reported inhibition of HAV replication upon treatment with zinc sulphate and zinc chloride (Ogawa et al., 2019; Kanda et al., 2020). Zinc chloride showed more potent anti-HAV effect than zinc sulphate. It enhanced the antiviral effect of interferon-alpha-2a against HAV (Kanda et al., 2020). Level of mitogen-activated protein kinase 12 (MAPK12) upregulated and six related genes baculoviral IAP repeat containing 3 (BIRC3), interleukin 1 beta (IL1β), proline-serine–threonine phosphatase interacting protein 1 (PSTPIP1), prostaglandin-endoperoxide synthase 2 (PTGS2), PYD and CARD domain containing (PYCARD), and tumor necrosis factor alpha (TNFα) were downregulated in zinc chloride treated cells (Kanda et al., 2020). Further investigations are warranted to uncover the possible direct/indirect role of zinc against HAV.
Limited information exists regarding direct antiviral effect of zinc against HBV to draw any mechanistic insight. One clinical trial evaluated the therapeutic benefit of zinc supplementation in HBV patients, indicating some improvement in liver function (Hayashi et al., 2007; Himoto et al., 2007). It is noteworthy that zinc binding is essential for function of the HBX protein, which is a key protein encoded by HBV (Ramakrishnan et al., 2019). Further, many host antiviral proteins and ISGs inhibit HBV replication either by targeting protein viral proteins and/or RNA. For example, host antiviral factor ZAP inhibits HBV replication (Mao et al., 2013). ISG20 selectively degrades HBV RNA (Liu et al., 2017; Imam et al., 2020). Myeloid differentiation primary response 88 (MYD88) inhibits HBV replication by promoting viral pregenomic RNA degradation and retention of viral preS/S RNA in the nucleus (Li et al., 2010). Myxovirus Resistance Gene A (MxA), an ISG, interacts with viral core protein and inhibits HBV replication (Gordien et al., 2001; Li et al., 2012). Since zinc is known to influence the activity of these genes/pathways under different conditions, additional studies involving suitable model systems are required to assess the possible therapeutic benefit of zinc in HBV cases. Knowledge obtained from our understanding of the mechanism of zinc action in other viruses should be the guiding criteria for designing experiments in HBV cases.
7. Challenges associated with zinc supplementation therapy
7.1. Rigorous cellular zinc homeostasis
Zinc homeostasis in the cell is a multistep process constituting three main stages, namely the zinc influx into the cells, zinc efflux from the cells and the storage within the cells. As described earlier, this process is realized through zinc transporters and the zinc binding proteins. In normal conditions, zinc homeostasis is tightly and neatly controlled, but while considering zinc supplementation, the dosage and the amount need to be judiciously and carefully curated. Any imbalance of zinc levels due to supplementation might cause disruption of zinc transport, leading to zinc accumulation in the cells causing toxicity. One of the major challenges to zinc supplementation is that zinc is needed in a large number of cellular processes and therefore interacts with a large number of proteins. It is really difficult to keep track of the effect of zinc supplementation on all these proteins.
7.2. Expression of zinc transporters in target cells and their effects
A number of reports suggest that differential expression and activity of ZIP and ZnTs are responsible for the pathogenesis and progression of chronic diseases. ZIP transporters are known to promote the invasive activity of cancers. Reduced expression in ZIPs has been seen in prostate cancer, where the cellular zinc is reduced, which accelerates the disease progression. ZIP4 is known to get atypically overexpressed in case of “acrodermatitis enteropathica.” ZIPs are known to play roles in epithelial–mesenchymal transition, anoikis resistance, and metastasis. In such cases, abnormal expression of ZIPs elevates the cytosolic zinc levels, which may extend the zinc dependent growth factor signaling and cause aberrant activation of cellular signaling pathways (Kagara et al., 2007). A myriad of secretory and membrane-bound enzymes which need zinc like matrix metalloproteinases are involved in cancer metastasis, and capture zinc in the early secretory pathway. Thus, ZnT transporters like ZnT5, ZnT6, and ZnT7 might be facilitating zinc uptake by these enzymes and their activation (Kambe, 2012). These examples merely illustrate that abnormal dysregulation of the zinc transporters have been observed in various disease and zinc supplementation could have a similar effect on these transporters and could lead to deleterious effects if not administered correctly.
Zinc interferes with the copper absorption in the body, even at a very little dose above the RDA (Recommended Dietary Allowance). Actually, higher concentration of zinc induces more expression of metallothioneins in the lumen to absorb more amount of zinc. However, this protein also has a high affinity for copper, resulting in a copper deficiency, and may cause anemia, neutropenia and myelopathy (Francis et al., 2022).
7.3. Zinc toxicity
Zinc is known to have many beneficial functions and has seen to play a role in protection in various infectious diseases, but excess zinc can cause zinc toxicity or side effects. Most often the zinc toxicity depends on the route of exposure to zinc or on the dosage. Zinc toxicity can be divided into two types- (a) acute and (b) chronic toxicity. Acute toxicity is caused by ingestion of salts of zinc such as zinc sulphate and zinc chloride which manifests into gastrointestinal symptoms like diarrhea, renal injury, acute respiratory distress syndrome (ARDS), liver necrosis, thrombocytopenia, coagulopathy and might lead to death in extreme cases (Barceloux, 1999). Chronic toxicity causes bone marrow damage and neurological symptoms. One of the major causes of concern in case of chronic toxicity is that if zinc is chronically ingested it can result in copper deficiency can lead to sideroblastic anemia, granulocytopenia, and myelodysplastic syndrome (Irving et al., 2003; Sheqwara and Alkhatib, 2013). Orally consumed zinc is absorbed by the jejunum of the small intestine. This process is facilitated by the metallothionein complex in the enterocyte villi (Ruttkay-Nedecky et al., 2013). Zinc binds to the metallothionine, which is known to be involved in the regulation of other metals as well, especially copper having the highest affinity to it (Ruttkay-Nedecky et al., 2013). To remove the excess zinc, the human body produces more metallothionein to prevent excess free zinc but in turn also decreases copper levels, thus, forming a dynamic antagonistic relationship. Here, the zinc homeostasis can only be maintained by the excretion of the metallothionein-zinc complex via bile and faeces. In case of zinc overdose, excretion of zinc will not be fast enough and thus it will get accumulated and cause clinical complications (Agnew and Slesinger, 2020).
8. Advances in zinc supplementation strategy and future perspectives
8.1. Zinc derivatives
Due to the advancement in nanotechnology, biomedical nanoparticles have gained considerable attention due to their prominence in biomedical applications and are being explored for molecular diagnostics, drug delivery, gene therapy. In case of Zinc based nanotechnology, zinc oxide-based nanoparticles have taken the center stage (Zhang and Xiong, 2015; Bashandy et al., 2018).
Zinc oxide nanoparticles (ZnO NPs) are important and are used in a variety of fields due to their unusual properties. ZnO nanoparticles have a small particle size which helps in the easier absorption of zinc by the body and are used as food additives and the US FDA has classified it as a GRAS (Jiang et al., 2018). In addition to this ZnO NPs are relatively inexpensive and less toxic. These properties make ZnOs an excellent candidate for biomedical applications. ZnOs have been used in anti-cancer treatment, drug delivery, antibacterial, diabetes treatment, anti-inflammation, wound healing and bioimaging (Zhang and Xiong, 2015; Mishra et al., 2017). In recent years nanoparticles of gold and silver have been predominantly studied and deployed against viral diseases. However, Zn nanoparticles might be relatively less toxic than silver and gold nanoparticles and more cost efficient. ZnO nanoparticles have proven to be successful as a therapeutic strategy for various viral diseases, although they have not been very extensively studied in animal as well as human models, but promising results have been obtained in various cell-based models (Aderibigbe, 2017). Zinc oxide nanoparticles have been shown to have a virostatic effect against HSV-1 and were able to efficiently trap virions from entering the human corneal fibroblasts (Mishra et al., 2011). Surface modified zinc oxide nanoparticles have been shown to alter the infection of Herpes simplex virus -I by neutralizing the virus by utilizing the electrostatic interference caused by the hydrophobic zinc nanoparticles (Farouk and Shebl, 2018). PEGylated zinc oxide nanoparticles have been shown to be successful in inhibiting H1N1 viral infection and there was a 1.2 log10 TCID50 decrease in the viral titer (Ghaffari et al., 2019). Further ZnO nanoparticles suppressed replication in case of nidovirus and also have been shown to disrupt the replication of a range of RNA viruses (Gurunathan et al., 2020). In MA104 cells (African green monkey fetal kidney), ZnO nanoparticles led to a 10-fold decrease in the chikungunya viral load (Kumar et al., 2018).
In addition to this, ZnO tetrapods have also been used as an alternative for the traditional nanoparticles (Mishra and Adelung, 2018). The unique 3D structure gives them a higher flexibility as a biomedical engineering tool than traditional spherical or 1D nanoparticles as tetrapods avoid the agglomeration issues faced by the other two. The first use of ZnO nano tetrapods was for the efficient delivery of plasmid DNA (Nie et al., 2006). Furthermore, zinc tetrapods have been used to trap virus particles using oxygen vacancies on the synthesized ZnO tetrapods. Polar surfaces were created in the tetrapods due to the oxide, which helped in trapping the negatively charged functional glycoprotein groups present on the virion surface (Mishra et al., 2011). Dendritic cells could easily take up such entrapped virus particles and neutralize them. This success of this strategy has been shown in humans and mice in the cases of HPV, ZIKA, HIV and Dengue. Apart from this ZnO nanoparticles and tetrapods have been shown to have an antiviral role in HSV, HEV and HCV by suppressing the viral replication (Mishra et al., 2011; Gupta et al., 2022).
Although zinc based nanoparticles have been shown to play antiviral roles they need to be further characterized to be successfully established as a commercially available therapeutic for viral diseases.
8.2. Modulators of zinc transporter
Zinc transport can be modulated by zinc ionophores. Zinc ionophores have been shown to inhibit replication of many viruses in vitro. Four zinc ionophores have shown to have antiviral roles which are Pyrithione, Hinokitiol, PDTC and Chloroquine. Pyrithione, Hinokitiol and PDTC inhibit the replication of the following viruses in vitro: coxsackievirus, equine arteritis virus, SARS-CoV, HSV, mengovirus and rhinovirus (Lanke et al., 2007; Krenn et al., 2009; Te Velthuis et al., 2010; Qiu et al., 2013). Chloroquine inhibits the replication of the following viruses: HCV, HCoV-229E, MERS-CoV, SARS-CoV, HIV-1 and Zika virus (Romanelli et al., 2004; Delvecchio et al., 2016; Read et al., 2017; Chandru et al., 2018). In addition to this, another zinc ionophore, Quercetin is known to inhibit replication of Influenza A virus and Rhino virus (Wu et al., 2016; Mehrbod et al., 2021). These studies indicate that zinc ionophores may be used as antivirals and since zinc has a significant role to play in liver homeostasis, the use of these ionophores is likely be an excellent antiviral strategy against hepatitis causing viruses.
8.3. Future perspectives
ZnO NPs have shown promise in biomedical applications due to their anti-viral, anti-bacterial and anti-diabetic roles. Inherently toxic, the ZnO NPs inhibit cancerous cells as well as bacteria by generating intracellular ROS generation. This activates the apoptotic signaling pathway making these nanoparticles excellent anticancer and anti-bacterial agents. Furthermore, Zinc nanoparticles and tetrapods have shown significant antiviral activities ranging from affecting replication, to neutralizing viruses and influencing viral entry. As drug carriers, ZnO NPs enhance therapeutic efficiency by promoting the bioavailability of the drugs or biomolecules.
The FDA has listed ZnO NPs as a safe substance. However, they should be further explored and studied as there are no comparative analysis of the biological advantages of Zinc nanoparticles over other metal nanoparticles. Further, there is lack of information on evidence based randomized trials and animal studies emphasizing their therapeutic roles. Focused studies on these aspects would help us better understand their diagnostic and therapeutic potential.
It is also important to be cautious and careful while interpreting and extrapolating the data obtained from cell-based experiments to patient studies. Generally zinc compounds are used in micromolar (μM)-millimolar (mM) concentration in the cell-based studies to evaluate their antiviral potential whereas plasma zinc concentration ranges between 10 and 18 μM in human (Rükgauer et al., 1997). On top of that free zinc levels are very tightly controlled in vivo. Although intracellular level of zinc maybe in micromolar concentration, most of it is bound to metallothioneins, thereby reducing the free zinc concentration to nanomolar scale (Krężel and Maret, 2006; Zheng et al., 2017). Such complex regulation makes it difficult to fully harness the therapeutic benefit of zinc based on the data obtained in cell-based experiments. Therefore, even though a zinc compound may exhibit very potent antiviral effect in laboratory condition, it needs stringent evaluation in the clinic.
The treatment of viral hepatitis has evolved rapidly over the last few years especially with the introduction of curated therapies for Hepatitis C. In addition to this the improvement in HAV, HBV and HEV vaccination has also led to a significant advance in viral hepatitis management. Viral hepatitis treatment is still dependent on a few drugs like Ribavirin and PEGylated Interferon but none of these are known to specifically target these viruses. Thus, targeted therapies can be further explored; one such strategy could be the use of zinc salt, zinc nanoparticles and zinc ionophore based therapies. We have recently shown the antiviral activity of zinc oxide nanoparticles and tetrapods against HEV and HCV, which could pave the way for the development of new therapeutic strategies against these viruses (Gupta et al., 2022).
Zinc oxide nanoparticles have shown promising antiviral effects on various viruses, but further research needs to be performed to explore and understand the underlying mechanism of zinc nanoparticles dependent antiviral activities. Zinc seems to inhibit the enzyme activities of viral protease and polymerases and is also involved in the physical processes of viral attachment and uncoating (Mishra et al., 2011). However, it is important to study these in clinical scenarios as zinc could become a viable supplement to the traditional viral treatments. A number of in vitro studies have demonstrated that free zinc possesses a strong antiviral effect through the trials with creams and tablets containing higher amounts of zinc (Mishra et al., 2011). These studies also tell us that when zinc is used at therapeutic doses and in the right form could improve viral clearance in case of acute and chronic infections. Zinc supplementation can be successful in two ways: it can be either be administered to improve the systemic immunity and the antiviral response in zinc deficient patients or can be used to specifically target viral replication and the symptoms of infection (Mishra et al., 2011). Although zinc-based strategies have been shown to have various roles in inhibiting the viral replication, there is a lack of information on whether these zinc-based strategies are sufficient as a standalone treatment or in enhancement of the effect of other antiviral treatments. Zinc supplementation with Ribavirin or PEGylated IFN-α reduced the side effects of Ribavirin/PEGylated IFN-α in case of HCV and HEV chronic patients but had no additive effect on these treatments (Farouk and Shebl, 2018; Suda et al., 2019). It has also been proven that in chronic patients of HCV and HBV zinc supplementation induces anti oxidative functioning of the liver preventing further liver damage during the treatment with Ribavirin and PEGylated IFN-α (Murakami et al., 2007). Zinc supplementation has also been shown to reduce inflammation and production of cytokines like NF-κB in chronic HCV and HBV patients (Nagamine et al., 2000). Accumulating data, through both in vitro and clinical studies, suggest that zinc supplementation in addition to other antiviral therapy is a viable treatment regimen for viral hepatitis. Although this needs to be further elucidated through large cohort based clinical studies.
Author contributions
SK, SA, SN, CR-K, and MS wrote the manuscript. MS and CR-K edited the manuscript draft. MS conceptualized the review. All authors contributed to the article and approved the submitted version.
Conflict of interest
The authors declare that the research was conducted in the absence of any commercial or financial relationships that could be construed as a potential conflict of interest.
Publisher’s note
All claims expressed in this article are solely those of the authors and do not necessarily represent those of their affiliated organizations, or those of the publisher, the editors and the reviewers. Any product that may be evaluated in this article, or claim that may be made by its manufacturer, is not guaranteed or endorsed by the publisher.
References
Abbasinazari, M., Alavian, S. M., Behnava, B., Asgharinia, M., Salimi, S., Keshvari, M., et al. (2014). Effect of zinc supplementation on viral response in patients with chronic hepatitis C and Beta thalassemia major, a pilot study. J. Clin. Diagn. Res. 8, HC16–HC19. doi: 10.7860/JCDR/2014/10403.5305
Aderibigbe, B. A. (2017). Metal-based nanoparticles for the treatment of infectious diseases. Molecules 22:1370. doi: 10.3390/molecules22081370
Agnew, U. M., and Slesinger, T. L. (2020). Zinc toxicity Florida, United States: Stat Pearls Publishing.
Andrews, G. K. (2008). Regulation and function of Zip 4, the acrodermatitis enteropathica gene. Biochem. Soc. Trans. 36, 1242–1246. doi: 10.1042/BST0361242
Andrews, G. K., Wang, H., Dey, S. K., and Palmiter, R. D. (2004). Mouse zinc transporter 1 gene provides an essential function during early embryonic development. Genesis 40, 74–81. doi: 10.1002/gene.20067
Antoine, T. E., Hadigal, S. R., Yakoub, A. M., Mishra, Y. K., Bhattacharya, P., Haddad, C., et al. (2016). Intravaginal zinc oxide tetrapod nanoparticles as novel immunoprotective agents against genital herpes. J. Immunol. 196, 4566–4575. doi: 10.4049/jimmunol.1502373
Anzilotti, C., Swan, D. J., Boisson, B., Deobagkar-Lele, M., Oliveira, C., Chabosseau, P., et al. (2019). An essential role for the Zn2+ transporter ZIP7 in B cell development. Nat. Immunol. 20, 350–361. doi: 10.1038/s41590-018-0295-8
Aras, M. A., and Aizenman, E. (2011). Redox regulation of intracellular zinc: molecular signalling in the life and death of neurons. Antioxid. Redox Signal. 15, 2249–2263. doi: 10.1089/ars.2010.3607
Asoudeh, F., Ebrahimzadeh, A., Ghoreishy, S. M., Imani, H., Mousavi, S. M., Zargarzadeh, N., et al. (2023). The association between dietary intakes of zinc, vitamin C and COVID-19 severity and related symptoms: A cross-sectional study. Clin. Nut. ESPEN 55, 244–250. doi: 10.1016/j.clnesp.2023.03.013
Aydemir, T. B., Liuzzi, J. P., McClellan, S., and Cousins, R. J. (2009). Zinc transporter ZIP8 (SLC39A8) and zinc influence IFN-γ expression in activated human T cells. J. Leukoc. Biol. 86, 337–348. doi: 10.1189/jlb.1208759
Bajait, C., and Thawani, V. (2011). Role of zinc in pediatric diarrhea. Indian J. Pharm. 43, 232–235. doi: 10.4103/0253-7613.81495
Bashandy, S. A., Alaamer, A., Moussa, S. A. A., and Omara, E. A. (2018). Role of zinc oxide nanoparticles in alleviating hepatic fibrosis and nephrotoxicity induced by thioacetamide in rats. Can. J. Physiol. Pharmacol. 96, 337–344. doi: 10.1139/cjpp-2017-0247
Baum, M. K., Lai, S., Sales, S., Page, J. B., and Campa, A. (2010). Randomized, controlled clinical trial of zinc supplementation to prevent immunological failure in HIV-infected adults. Clin. Infect. Dis. 50, 1653–1660. doi: 10.1086/652864
Beran, A., Mhanna, M., Srour, O., Ayesh, H., Stewart, J. M., Hjouj, M., et al. (2022). Clinical significance of micronutrient supplements in patients with coronavirus disease 2019: a comprehensive systematic review and meta-analysis. Clin. Nut. ESPEN 48, 167–177. doi: 10.1016/j.clnesp.2021.12.033
Bhatia, H. K., Singh, H., Grewal, N., and Natt, N. K. (2014). Sofosbuvir: a novel treatment option for chronic hepatitis C infection. J. Pharmacol. Pharmacother. 5, 278–284. doi: 10.4103/0976-500X.142464
Bianchi, G. P., Marchesini, G., Brizi, M., Rossi, B., Forlani, G., Boni, P., et al. (2000). Nutritional effects of oral zinc supplementation in cirrhosis. Nutr. Res. 20, 1079–1089. doi: 10.1016/S0271-5317(00)00194-9
Bin, B. H., Fukada, T., Hosaka, T., Yamasaki, S., Ohashi, W., Hojyo, S., et al. (2011). Biochemical characterization of human ZIP13 protein: a homo-dimerized zinc transporter involved in the spondylocheiro dysplastic Ehlers-Danlos syndrome. J. Biol. Chem. 286, 40255–40265. doi: 10.1074/jbc.M111.256784
Bin, B. H., Seo, J., and Kim, S. T. (2018). Function, structure, and transport aspects of ZIP and ZnT zinc transporters in immune cells. J Immunol Res 2018, 1–9. doi: 10.1155/2018/9365747
Bobat, R., Coovadia, H., Stephen, C., Naidoo, K. L., McKerrow, N., Black, R. E., et al. (2005). Safety and efficacy of zinc supplementation for children with HIV-1 infection in South Africa: a randomised double-blind placebo-controlled trial. Lancet 366, 1862–1867. doi: 10.1016/S0140-6736(05)67756-2
Bonaventura, P., Benedetti, G., Albarède, F., and Miossec, P. (2015). Zinc and its role in immunity and inflammation. Autoimmun. Rev. 14, 277–285. doi: 10.1016/j.autrev.2014.11.008
Bosomworth, H. J., Thornton, J. K., Coneyworth, L. J., Ford, D., and Valentine, R. A. (2012). Efflux function, tissue-specific expression and intracellular trafficking of the Zn transporter ZnT10 indicate roles in adult Zn homeostasis. Metallomics 4, 771–779. doi: 10.1039/c2mt20088k
Bracha, M., and Schlesinger, M. J. (1976). Inhibition of Sindbis virus replication by zinc lons. Virology 72, 272–277. doi: 10.1016/0042-6822(76)90330-5
Brewer, G. J., Johnson, V. D., Dick, R. D., Hedera, P., Fink, J. K., and Kluin, K. J. (2000). Treatment of Wilson's disease with zinc. XVII: treatment during pregnancy. Hepatology 31, 364–370. doi: 10.1002/hep.510310216
Brieger, A., Rink, L., and Haase, H. (2013). Differential regulation of TLR-dependent MyD88 and TRIF signaling pathways by free zinc ions. J. Immunol. 191, 1808–1817. doi: 10.4049/jimmunol.1301261
Carlucci, P. M., Ahuja, T., Petrilli, C., Rajagopalan, H., Jones, S., and Rahimian, J. (2020). Zinc sulfate in combination with a zinc ionophore may improve outcomes in hospitalized COVID-19 patients. J. Med. Microbiol. 69, 1228–1234. doi: 10.1099/jmm.0.001250
Chandru, S., Nair, V. P., Saumya, A., Das, M. S., Madhu, P., Nidhi, K., et al. (2018). Host-virus protein interaction network reveals the involvement of multiple host processes in the life cycle of hepatitis E virus. mSystems 3, 1–21. doi: 10.1128/mSystems.00135-17
Chaudhary, D., Ahmed, S., Liu, N., and Marsano-Obando, L. (2017). Acute liver failure from herpes simplex virus in an immunocompetent patient due to direct inoculation of the peritoneum. ACG Case Rep. J. 4:e23. doi: 10.14309/crj.2017.23
Chen, G., Guo, X., Lv, F., Xu, Y., and Gao, G. (2008). P 72 DEAD box RNA helicase is required for optimal function of the zinc-finger antiviral protein. Proc. Natl. Acad. Sci. 105, 4352–4357. doi: 10.1073/pnas.0712276105
Chen, S., Xu, Y., Zhang, K., Wang, X., Sun, J., Gao, G., et al. (2012). Structure of N-terminal domain of ZAP indicates how a zinc-finger protein recognizes complex RNA. Nat. Struct. Mol. Biol. 19, 430–435. doi: 10.1038/nsmb.2243
Chiang, G. G., and Sefton, B. M. (2001). Specific dephosphorylation of the Lck tyrosine protein kinase at Tyr-394 by the SHP-1 protein-tyrosine phosphatase. J. Biol. Chem. 276, 23173–23178. doi: 10.1074/jbc.M101219200
Chinni, V., El-Khoury, J., Perera, M., Bellomo, R., Jones, D., Bolton, D., et al. (2021). Zinc supplementation as an adjunct therapy for COVID-19: challenges and opportunities. Br. J. Clin. Pharmacol. 87, 3737–3746. doi: 10.1111/bcp.14826
Colomar-Carando, N., Meseguer, A., Jutz, S., Herrera-Fernández, V., Olvera, A., Kiefer, K., et al. (2019). Zip 6 transporter is an essential component of the lymphocyte activation machinery. J. Immunol. 202, 441–450. doi: 10.4049/jimmunol.1800689
Cowan, M. L., Thomas, H. C., and Foster, G. R. (2011). Therapy for chronic viral hepatitis: current indications, optimal therapies and delivery of care. Clin. Med. 11, 184–189. doi: 10.7861/clinmedicine.11-2-184
Das, S., Ramakrishnan, K., Behera, S. K., Ganesapandian, M., Xavier, A. S., and Selvarajan, S. (2019). Hepatitis B vaccine and immunoglobulin: key concepts. J. Clin. Transl. Hepatol. 7, 165–171. doi: 10.14218/JCTH.2018.00037
Delvecchio, R., Higa, L. M., Pezzuto, P., Valadão, A. L., Garcez, P. P., Monteiro, F. L., et al. (2016). Chloroquine, an endocytosis blocking agent, inhibits Zika virus infection in different cell models. Viruses 8:322. doi: 10.3390/v8120322
Dietz-Fricke, C., Tacke, F., Zöllner, C., Demir, M., Schmidt, H. H., Schramm, C., et al. (2023). Treating hepatitis D with bulevirtide–real-world experience from 114 patients. JHEP Rep. 5:100686. doi: 10.1016/j.jhepr.2023.100686
Diglio, D. C., Fernandes, S. A., Stein, J., Azeredo-da-Silva, A., de Mattos, A. A., and Tovo, C. V. (2020). Role of zinc supplementation in the management of chronic liver diseases: a systematic review and meta-analysis. Ann. Hepatol. 19, 190–196. doi: 10.1016/j.aohep.2019.08.011
Drave, S. A., Debing, Y., Walter, S., Todt, D., Engelmann, M., Friesland, M., et al. (2016). Extra-hepatic replication and infection of hepatitis E virus in neuronal-derived cells. J. Viral Hepat. 23, 512–521. doi: 10.1111/jvh.12515
Dufner-Beattie, J., Huang, Z. L., and Geiser, J. (2006). Mouse ZIP1 and ZIP3 genes together are essential for adaptation to dietary zinc deficiency during pregnancy. Genesis 44, 239–251. doi: 10.1002/dvg.20211
Eby, G. A., Davis, D. R., and Halcomb, W. W. (1984). Reduction in duration of common colds by zinc gluconate lozenges in a double-blind study. Antimicrob. Agents Chemother. 25, 20–24. doi: 10.1128/AAC.25.1.20
Farouk, F., and Shebl, R. I. (2018). Comparing surface chemical modifications of zinc oxide nanoparticles for modulating their antiviral activity against herpes simplex virus type-1. Int. J. Nanopart. Nanotechnol. 4:21. doi: 10.35840/2631-5084/5521
Fenstermacher, K. J., and DeStefano, J. J. (2011). Mechanism of HIV reverse transcriptase inhibition by zinc: formation of a highly stable enzyme-(primer-template) complex with profoundly diminished catalytic activity. J. Biol. Chem. 286, 40433–40442. doi: 10.1074/jbc.M111.289850
Fernandes, G., Nair, M., Onoe, K., Tanaka, T., Floyd, R., and Good, R. A. (1979). Impairment of cell-mediated immunity functions by dietary zinc deficiency in mice. Proc. Natl. Acad. Sci. 76, 457–461. doi: 10.1073/pnas.76.1.457
Fraker, P. J., Caruso, R., and Kierszenbaum, F. (1982). Alteration of the immune and nutritional status of mice by synergy between zinc deficiency and infection with Trypanosoma cruzi. J. Nutr. 112, 1224–1229. doi: 10.1093/jn/112.6.1224
Fraker, P. J., and King, L. E. (2004). Reprogramming of the immune system during zinc deficiency. Annu. Rev. Nutr. 24, 277–298. doi: 10.1146/annurev.nutr.24.012003.132454
Francis, Z., Book, G., Litvin, C., and Kalivas, B. (2022). The COVID-19 pandemic and zinc-induced copper deficiency: an important link. Am. J. Med. 135, e290–e291. doi: 10.1016/j.amjmed.2022.03.008
Frederickson, C. J., Suh, S. W., Silva, D., Frederickson, C. J., and Thompson, R. B. (2000). Importance of zinc in the central nervous system: the zinc-containing neuron. J. Nutr. 130, 1471S–1483S. doi: 10.1093/jn/130.5.1471S
Fu, B., Wang, L., Li, S., and Dorf, M. E. (2017). ZMPSTE24 defends against influenza and other pathogenic viruses. J. Exp. Med. 214, 919–929. doi: 10.1084/jem.20161270
Fukada, T., and Kambe, T. (2011). Molecular and genetic features of zinc transporters in physiology and pathogenesis. Metallomics 3, 662–674. doi: 10.1039/c1mt00011j
Gaither, L. A., and Eide, D. J. (2000). Functional expression of the human hZIP2 zinc transporter. J. Biol. Chem. 275, 5560–5564. doi: 10.1074/jbc.275.8.5560
George, M. M., Vignesh, K. S., Figueroa, J. A. L., Caruso, J. A., and Deepe, G. S. (2016). Zinc induces dendritic cell tolerogenic phenotype and skews regulatory T cell–Th17 balance. J. Immunol. 197, 1864–1876. doi: 10.4049/jimmunol.1600410
Ghaffari, H., Tavakoli, A., Moradi, A., Tabarraei, A., Bokharaei-Salim, F., Zahmatkeshan, M., et al. (2019). Inhibition of H1N1 influenza virus infection by zinc oxide nanoparticles: another emerging application of nanomedicine. J. Biomed. Sci. 26, 70–10. doi: 10.1186/s12929-019-0563-4
Gonçalves-Carneiro, D., Mastrocola, E., Lei, X., DaSilva, J., Chan, Y. F., and Bieniasz, P. D. (2022). Rational attenuation of RNA viruses with zinc finger antiviral protein. Nat. Microbiol. 7, 1558–1567. doi: 10.1038/s41564-022-01223-8
Gonzalez-Perez, A. C., Stempel, M., Wyler, E., Urban, C., Piras, A., Hennig, T., et al. (2021). The zinc finger antiviral protein ZAP restricts human cytomegalovirus and selectively binds and destabilizes viral UL4/UL5 transcripts. MBio 12, 10–1128. doi: 10.1128/mBio.02683-20
Gopal, V., Nilsson-Payant, B. E., French, H., Siegers, J. Y., Yung, W. S., Hardwick, M., et al. (2021). Zinc-embedded polyamide fabrics inactivate SARS-CoV-2 and influenza A virus. ACS Appl. Mater. Interfaces 13, 30317–30325. doi: 10.1021/acsami.1c04412
Gordien, E., Rosmorduc, O., Peltekian, C., Garreau, F., Bréchot, C., and Kremsdorf, D. (2001). Inhibition of hepatitis B virus replication by the interferon-inducible MxA protein. J. Virol. 75, 2684–2691. doi: 10.1128/JVI.75.6.2684-2691.2001
Gordon, A. M., and Hardigan, P. C. (2021). A case-control study for the effectiveness of oral zinc in the prevention and mitigation of COVID-19. Front. Med. 8:756707. doi: 10.3389/fmed.2021.756707
Groth, C., Sasamura, T., Khanna, M. R., Whitley, M., and Fortini, M. E. (2013). Protein trafficking abnormalities in Drosophila tissues with impaired activity of the ZIP7 zinc transporter catsup. Development 140, 3018–3027. doi: 10.1242/dev.088336
Grzywacz, A., Gdula-Argasińska, J., Muszyńska, B., Tyszka-Czochara, M., Librowski, T., and Opoka, W. (2015). Metal responsive transcription factor 1 (MTF-1) regulates zinc dependent cellular processes at the molecular level. Acta Biochim. Pol. 62, 491–498. doi: 10.18388/abp.2015_1038
Guo, X., Carroll, J. W. N., Mac Donald, M. R., Goff, S. P., and Gao, G. (2004). The zinc finger antiviral protein directly binds to specific viral mRNAs through the CCCH zinc finger motifs. J. Virol. 78, 12781–12787. doi: 10.1128/JVI.78.23.12781-12787.2004
Guo, H., Jin, X., and Zhu, T. (2014). SLC39A5 mutations interfering with the BMP/TGF-b pathway in non-syndromic high myopia. J. Med. Genet. 51, 518–525. doi: 10.1136/jmedgenet-2014-102351
Guo, X., Ma, J., Sun, J., and Gao, G. (2007). The zinc-finger antiviral protein recruits the RNA processing exosome to degrade the target mRNA. Proc. Natl. Acad. Sci. 104, 151–156. doi: 10.1073/pnas.0607063104
Gupta, J., Irfan, M., Ramgir, N., Muthe, K. P., Debnath, A. K., Ansari, S., et al. (2022). Antiviral activity of zinc oxide nanoparticles and Tetrapods against the hepatitis E and hepatitis C viruses. Front. Microbiol. 13:881595. doi: 10.3389/fmicb.2022.881595
Gupta, P., and Rapp, F. (1976). Effect of zinc ions on synthesis of herpes simplex virus type 2-induced polypeptides. Proc. Soc. Exp. Biol. Med. 152, 455–458. doi: 10.3181/00379727-152-39417
Gurunathan, S., Qasim, M., Choi, Y., Do, J. T., Park, C., Hong, K., et al. (2020). Antiviral potential of nanoparticles—can nanoparticles fight against coronaviruses? Nano 10:1645. doi: 10.3390/nano10091645
Haase, H., Ober-Blöbaum, J. L., Engelhardt, G., Hebel, S., Heit, A., Heine, H., et al. (2008). Zinc signals are essential for lipopolysaccharide-induced signal transduction in monocytes. J. Immunol. 181, 6491–6502. doi: 10.4049/jimmunol.181.9.6491
Haase, H., and Rink, L. (2009). Functional significance of zinc-related signaling pathways in immune cells. Annu. Rev. Nutr. 29, 133–152. doi: 10.1146/annurev-nutr-080508-141119
Hara, T., Takeda, T. A., Takagishi, T., Fukue, K., Kambe, T., and Fukada, T. (2017). Physiological roles of zinc transporters: molecular and genetic importance in zinc homeostasis. J. Physiol. Sci. 67, 283–301. doi: 10.1007/s12576-017-0521-4
Haraguchi, Y., Sakurai, H., Hussain, S., Anner, B. M., and Hoshino, H. (1999). Inhibition of HIV-1 infection by zinc group metal compounds. Antivir. Res. 43, 123–133. doi: 10.1016/s0166-3542(99)00040-6
Hauri, A. M., Fischer, E., Fitzenberger, J., Uphoff, H., and Koenig, C. (2006). Active immunisation during an outbreak of hepatitis a in a German day-care Centre. Vaccine 24, 5684–5689. doi: 10.1016/j.vaccine.2006.04.053
Hayakawa, S., Shiratori, S., Yamato, H., Kameyama, T., Kitatsuji, C., Kashigi, F., et al. (2011). ZAPS is a potent stimulator of signaling mediated by the RNA helicase RIG-I during antiviral responses. Nat. Immunol. 12, 37–44. doi: 10.1038/ni.1963
Hayashi, M., Ikezawa, K., Ono, A., Okabayashi, S., Hayashi, Y., Shimizu, S., et al. (2007). Evaluation of the effects of combination therapy with branched-chain amino acid and zinc supplements on nitrogen metabolism in liver cirrhosis. Hepatol. Res. 37, 615–619. doi: 10.1111/j.1872-034X.2007.00095.x
Hildebrand, M. S., Phillips, A. M., and Mullen, S. A. (2015). Loss of synaptic Zn2? Transporter function increases risk of febrile seizures. Sci. Rep. 5:17816. doi: 10.1038/srep17816
Himoto, T., Hosomi, N., Nakai, S., Deguchi, A., Kinekawa, F., Matsuki, M., et al. (2007). Efficacy of zinc administration in patients with hepatitis C virus-related chronic liver disease. Scand. J. Gastroenterol. 42, 1078–1087. doi: 10.1080/00365520701272409
Hock, H., Hamblen, M. J., Rooke, H. M., Traver, D., Bronson, R. T., Cameron, S., et al. (2003). Intrinsic requirement for zinc finger transcription factor Gfi-1 in neutrophil differentiation. Immunity 18, 109–120. doi: 10.1016/s1074-7613(02)00501-0
Hojyo, S., Miyai, T., Fujishiro, H., Kawamura, M., Yasuda, T., Hijikata, A., et al. (2014). Zinc transporter SLC39A10/ZIP10 controls humoral immunity by modulating B-cell receptor signal strength. Proc. Natl. Acad. Sci. 111, 11786–11791. doi: 10.1073/pnas.1323557111
Horvatits, T., Behrendt, P., Schuebel, N., Guthoff, M., Wiegand, J., Harth, A., et al. (2023). Oral zinc supplementation in chronically HEV-infected patients not responding to ribavirin monotherapy. Hepat. Mon. 23:e130865. doi: 10.5812/hepatmon-130865
Hosui, A., Tanimoto, T., Okahara, T., Ashida, M., Ohnishi, K., Wakahara, Y., et al. (2021). Oral zinc supplementation decreases the risk of HCC development in patients with HCV eradicated by DAA. Hepatol. Commun. 5, 2001–2008. doi: 10.1002/hep4.1782
Huang, L., and Gitschier, J. (1997). A novel gene involved in zinc transport is deficient in the lethal milk mouse. Nat. Genet. 17, 292–297. doi: 10.1038/ng1197-292
Huang, C. R., and Lo, S. J. (2014). Hepatitis D virus infection, replication and cross-talk with the hepatitis B virus. WJG 20, 14589–14597. doi: 10.3748/wjg.v20.i40.14589
Huang, L., Yu, Y. Y., and Kirschke, C. P. (2007). Znt 7 (Slc 30a7)- deficient mice display reduced body zinc status and body fat accumulation. J. Biol. Chem. 282, 37053–37063. doi: 10.1074/jbc.M706631200
Hulisz, D. (2004). Efficacy of zinc against common cold viruses: an overview. J. Am. Pharm. Assoc. 44, 594–603. doi: 10.1331/1544-3191.44.5.594.Hulisz
Hung, M., Gibbs, C. S., and Tsiang, M. (2002). Biochemical characterization of rhinovirus RNA-dependent RNA polymerase. Antivir. Res. 56, 99–114. doi: 10.1016/s0166-3542(02)00101-8
Imam, H., Kim, G. W., Mir, S. A., Khan, M., and Siddiqui, A. (2020). Interferon-stimulated gene 20 (ISG20) selectively degrades N6-methyladenosine modified hepatitis B virus transcripts. PLoS Pathog. 16:e1008338. doi: 10.1371/journal.ppat.1008338
Inoue, K., Matsuda, K., and Itoh, M. (2002). Osteopenia and male specific sudden cardiac death in mice lacking a zinc transporter gene, Znt 5. Hum. Mol. Genet. 11, 1775–1784. doi: 10.1093/hmg/11.15.1775
Irving, J. A., Mattman, A., Lockitch, G., Farrell, K., and Wadsworth, L. D. (2003). Element of caution: a case of reversible cytopenias associated with excessive zinc supplementation. CMAJ 169, 129–131.
Itsumura, N., Inamo, Y., and Okazaki, F. (2013). Compound heterozygous mutations in SLC30A2/ZnT2 results in low milk zinc concentrations: a novel mechanism for zinc deficiency in a breast-fed infant. PLoS One 8:e64045. doi: 10.1371/journal.pone.0064045
Jha, A. K., Kumar, G., Dayal, V. M., Ranjan, A., and Suchismita, A. (2021). Neurological manifestations of hepatitis E virus infection: an overview. World J. Gastroenterol. 27, 2090–2104. doi: 10.3748/wjg.v27.i18.2090
Jiang, J., Jiang, P., and Jiye, C. (2018). The advancing of zinc oxide nanoparticles for biomedical applications. Bioinorg. Chem. Appl. 2018, 1–18. doi: 10.1155/2018/1062562
Jiang, L. J., Maret, W., and Vallee, B. L. (1998). The ATP–metallothionein complex. Proc. Natl. Acad. Sci. 95, 9146–9149. doi: 10.1073/pnas.95.16.9146
John, E., Laskow, T. C., Buchser, W. J., Pitt, B. R., Basse, P. H., Butterfield, L. H., et al. (2010). Zinc in innate and adaptive tumor immunity. J. Transl. Med. 8:118. doi: 10.1186/1479-5876-8-118
Kagara, N., Tanaka, N., Noguchi, S., and Hirano, T. (2007). Zinc and its transporter ZIP10 are involved in invasive behaviour of breast cancer cells. Cancer Sci. 98, 692–697. doi: 10.1111/j.1349-7006.2007.00446.x
Kaltenberg, J., Plum, L. M., Ober-Blöbaum, J. L., Hönscheid, A., Rink, L., and Haase, H. (2010). Zinc signals promote IL-2-dependent proliferation of T cells. Eur. J. Immunol. 40, 1496–1503. doi: 10.1002/eji.200939574
Kambe, T. (2012). Molecular architecture and function of ZnT transporters. Curr. Top. Membr. 69, 199–220. doi: 10.1016/B978-0-12-394390-3.00008-2
Kanda, T., Sasaki, R., Masuzaki, R., Takahashi, H., Fujisawa, M., Matsumoto, N., et al. (2020). Additive effects of zinc chloride on the suppression of hepatitis A virus replication by interferon in human hepatoma huh 7 cells. In Vivo 34, 3301–3308. doi: 10.21873/invivo.12168
Katwal, P., Aftab, S., Nelson, E., Hildreth, M., Li, S., and Wang, X. (2022). Role of zinc metalloprotease (ZMPSTE24) in porcine reproductive and respiratory syndrome virus (PRRSV) replication in vitro. Arch. Virol. 167, 2281–2286. doi: 10.1007/s00705-022-05529-0
Katz, E. H. U. D., and Margalith, E. (1981). Inhibition of vaccinia virus maturation by zinc chloride. Antimicrob. Agents Chemother. 19, 213–217. doi: 10.1128/aac.19.2.213
Kaushik, N., Subramani, C., Anang, S., Muthumohan, R., Shalimar,, Nayak, B., et al. (2017). Zinc salts block hepatitis E virus replication by inhibiting the activity of viral RNA-dependent RNA polymerase. J. Virol. 91, e00754–e00717. doi: 10.1128/JVI.00754-17
Keeffe, E. B. (1995). Is hepatitis a more severe in patients with chronic hepatitis B and other chronic liver diseases? Am. J. Gastroenterol. 90, 201–205.
Kim, B., Kim, H. Y., and Lee, W. W. (2021). Zap 70 regulates TCR-mediated zip 6 activation at the immunological synapse. Front. Immunol. 12:687367. doi: 10.3389/fimmu.2021.687367
Kim, K. I., Kim, S. R., Sasase, N., Akimoto, Y., Shikata, M., Ohtani, A., et al. (2008). Blood cell, liver function, and response changes by PEG-interferon-α2b plus ribavirin with polaprezinc therapy in patients with chronic hepatitis C. Hepatol. Int. 2, 111–115. doi: 10.1007/s12072-007-9029-y
King, L. E., Osati-Ashtiani, F., and Fraker, P. J. (1995). Depletion of cells of the B lineage in the bone marrow of zinc-deficient mice. Immunology 85, 69–73.
Kitabayashi, C., Fukada, T., Kanamoto, M., Ohashi, W., Hojyo, S., Atsumi, T., et al. (2010). Zinc suppresses Th17 development via inhibition of STAT3 activation. Int. Immunol. 22, 375–386. doi: 10.1093/intimm/dxq017
Kitamura, H., Morikawa, H., Kamon, H., Iguchi, M., Hojyo, S., Fukada, T., et al. (2006). Toll-like receptor–mediated regulation of zinc homeostasis influences dendritic cell function. Nat. Immunol. 7, 971–977. doi: 10.1038/ni1373
Ko, W. S., Guo, C. H., Hsu, G. S. W., Chiou, Y. L., Yeh, M. S., and Yaun, S. R. (2005). The effect of zinc supplementation on the treatment of chronic hepatitis C patients with interferon and ribavirin. Clin. Biochem. 38, 614–620. doi: 10.1016/j.clinbiochem.2005.04.003
Korant, B. D., Kauer, J. C., and Butterworth, B. E. (1974). Zinc ions inhibit replication of rhinoviruses. Nature 248, 588–590. doi: 10.1038/248588a0
Krenn, B. M., Gaudernak, E., Holzer, B., Lanke, K., Van Kuppeveld, F. J. M., and Seipelt, J. (2009). Antiviral activity of the zinc ionophores pyrithione and hinokitiol against picornavirus infections. J. Virol. 83, 58–64. doi: 10.1128/JVI.01543-08
Krężel, A., and Maret, W. (2006). Zinc-buffering capacity of a eukaryotic cell at physiological pZn. JBIC J. Biol. Inorgan. Chem. 11, 1049–1062. doi: 10.1007/s00775-006-0150-5
Krishnaraju, K., Nguyen, H. Q., Liebermann, D. A., and Hoffman, B. (1995). The zinc finger transcription factor Egr-1 potentiates macrophage differentiation of hematopoietic cells. Mol. Cell. Biol. 15, 5499–5507. doi: 10.1128/MCB.15.10.5499
Kumar, R., Sahoo, G., Pandey, K., Nayak, M. K., Topno, R., Rabidas, V., et al. (2018). Virostatic potential of zinc oxide (ZnO) nanoparticles on capsid protein of cytoplasmic side of chikungunya virus. Int. J. Infect. Dis. 73:368. doi: 10.1016/j.ijid.2018.04.4247
Kümel, G., Schrader, S., Zentgraf, H., Daus, H., and Brendel, M. (1990). The mechanism of the antiherpetic activity of zinc sulphate. J. Gen. Virol. 71, 2989–2997. doi: 10.1099/0022-1317-71-12-2989
Kurugöl, Z., Akilli, M., Bayram, N., and Koturoglu, G. (2006). The prophylactic and therapeutic effectiveness of zinc sulphate on common cold in children. Acta Paediatr. 95, 1175–1181. doi: 10.1080/08035250600603024
Lalazar, G., and Ilan, Y. (2014). Viral diseases of the liver. Liver Immunology: Principles and Practice, 159–171.
Lanke, K., Krenn, B. M., Melchers, W. J. G., Seipelt, J., and Van Kuppeveld, F. J. M. (2007). PDTC inhibits picornavirus polyprotein processing and RNA replication by transporting zinc ions into cells. J. Gen. Virol. 88, 1206–1217. doi: 10.1099/vir.0.82634-0
Lazarczyk, M., and Favre, M. (2008). Role of Zn2+ ions in host-virus interactions. J. Virol. 82, 11486–11494. doi: 10.1128/JVI.01314-08
Li, D., Achkar, J.-P., and Haritunians, T. (2016). A pleiotropic missense variant in SLC39A8 is associated with Crohn’s disease and human gut microbiome composition. Gastroenterology 151, 724–732. doi: 10.1053/j.gastro.2016.06.051
Li, S., Fu, B., Wang, L., and Dorf, M. E. (2017). ZMPSTE24 is downstream effector of interferon-induced transmembrane antiviral activity. DNA Cell Biol. 36, 513–517. doi: 10.1089/dna.2017.3791
Li, J., Lin, S., Chen, Q., Peng, L., Zhai, J., Liu, Y., et al. (2010). Inhibition of hepatitis B virus replication by MyD88 involves accelerated degradation of pregenomic RNA and nuclear retention of pre-S/S RNAs. J. Virol. 84, 6387–6399. doi: 10.1128/JVI.00236-10
Li, N., Zhang, L., Chen, L., Feng, W., Xu, Y., Chen, F., et al. (2012). MxA inhibits hepatitis B virus replication by interaction with hepatitis B core antigen. Hepatology 56, 803–811. doi: 10.1002/hep.25608
Lichten, L. A., and Cousins, R. J. (2009). Mammalian zinc transporters: nutritional and physiologic regulation. Annu. Rev. Nutr. 29, 153–176. doi: 10.1146/annurev-nutr-033009-083312
Lin, R. S., Rodriguez, C., Veillette, A., and Lodish, H. F. (1998). Zinc is essential for binding of p56lck to CD4 and CD8α. J. Biol. Chem. 273, 32878–32882. doi: 10.1074/jbc.273.49.32878
Liu, M. J., Bao, S., Gálvez-Peralta, M., Pyle, C. J., Rudawsky, A. C., Pavlovicz, R. E., et al. (2013). ZIP8 regulates host defense through zinc-mediated inhibition of NF-κB. Cell Rep. 3, 386–400. doi: 10.1016/j.celrep.2013.01.009
Liu, S., Cao, X., Guo, H., and Wei, W. (2021). Zinc influx restricts enterovirus D68 replication. Front. Microbiol. 12:748546. doi: 10.3389/fmicb.2021.748546
Liu, C. Y., and Kielian, M. (2012). Identification of a specific region in the e1 fusion protein involved in zinc inhibition of semliki forest virus fusion. J. Virol. 86, 3588–3594. doi: 10.1128/JVI.07115-11
Liu, Z., Li, H., Soleimani, M., Girijashanker, K., Reed, J. M., He, L., et al. (2008). Cd2+ versus Zn2+ uptake by the ZIP8 HCO3--dependent symporter: kinetics, electrogenicity and trafficking. Biochem. Biophys. Res. Commun. 365, 814–820. doi: 10.1016/j.bbrc.2007.11.067
Liu, Y., Nie, H., Mao, R., Mitra, B., Cai, D., Yan, R., et al. (2017). Interferon-inducible ribonuclease ISG20 inhibits hepatitis B virus replication through directly binding to the epsilon stem-loop structure of viral RNA. PLoS Pathog. 13:e1006296. doi: 10.1371/journal.ppat.1006296
Lok, A. S., Heathcote, E. J., and Hoofnagle, J. H. (2001). Management of hepatitis B: 2000—summary of a workshop. Gastroenterology 120, 1828–1853. doi: 10.1053/gast.2001.24839
Love, R. A., Parge, H. E., Wickersham, J. A., Hostomsky, Z., Habuka, N., Moomaw, E. W., et al. (1996). The crystal structure of hepatitis C virus NS3 proteinase reveals a trypsin-like fold and a structural zinc binding site. Cells 87, 331–342. doi: 10.1016/S0092-8674(00)81350-1
Lu, M., and Fu, D. (2007). Structure of the zinc transporter Yii P. Science 317, 1746–1748. doi: 10.1126/science.1143748
Maldonado, R. A., and von Andrian, U. H. (2010). How tolerogenic dendritic cells induce regulatory T cells. Adv. Immunol. 108, 111–165. doi: 10.1016/B978-0-12-380995-7.00004-5
Manns, M. P., Buti, M., Gane, E. D., Pawlotsky, J. M., Razavi, H., Terrault, N., et al. (2017). Hepatitis C virus infection. Nat. Rev. Dis. Primers 3, 1–19. doi: 10.1038/nrdp.2017.6
Mao, R., Nie, H., Cai, D., Zhang, J., Liu, H., Yan, R., et al. (2013). Inhibition of hepatitis B virus replication by the host zinc finger antiviral protein. PLoS Pathog. 9:e1003494. doi: 10.1371/journal.ppat.1003494
Maret, W. (1994). Oxidative metal release from metallothionein via zinc-thiol/disulfide interchange. Proc. Natl. Acad. Sci. 91, 237–241. doi: 10.1073/pnas.91.1.237
Maret, W., and Sandstead, H. H. (2006). Zinc requirements and the risks and benefits of zinc supplementation. J. Trace Elem. Med. Biol. 20, 3–18. doi: 10.1016/j.jtemb.2006.01.006
Martin, A., and Lemon, S. M. (2006). Hepatitis a virus: from discovery to vaccines. Hepatology 43, S164–S172. doi: 10.1002/hep.21052
Mathews, W. R., Ong, D., Milutinovich, A. B., and Van Doren, M. (2006). Zinc transport activity of fear of intimacy is essential for proper gonad morphogenesis and DE-cadherin expression. J. Embryol. Exp. Morpholog. 133, 1143–1153. doi: 10.1242/dev.02256
Matsuoka, S., Matsumura, H., Nakamura, H., Oshiro, S., Arakawa, Y., Hayashi, J., et al. (2009). Zinc supplementation improves the outcome of chronic hepatitis C and liver cirrhosis. J. Clin. Biochem. Nutr. 45, 292–303. doi: 10.3164/jcbn.jcbn08-246
Mehrbod, P., Hudy, D., Shyntum, D., Markowski, J., Łos, M. J., and Ghavami, S. (2021). Quercetin as a natural therapeutic candidate for the treatment of influenza virus. Biomol. Ther. 11:10. doi: 10.3390/biom11010010
Mishra, Y. K., and Adelung, R. (2018). ZnO tetrapod materials for functional applications. Mater. Today 21, 631–651. doi: 10.1016/j.mattod.2017.11.003
Mishra, Y. K., Adelung, R., Röhl, C., Shukla, D., Spors, F., and Tiwari, V. (2011). Virostatic potential of micro–nano filopodia-like ZnO structures against herpes simplex virus-1. Antivir. Res. 92, 305–312. doi: 10.1016/j.antiviral.2011.08.017
Mishra, P. K., Mishra, H., Ekielski, A., Talegaonkar, S., and Vaidya, B. (2017). Zinc oxide nanoparticles: a promising nanomaterial for biomedical applications. Drug Discov. Today 22, 1825–1834. doi: 10.1016/j.drudis.2017.08.006
Mocchegiani, E., and Muzzioli, M. (2000). Therapeutic application of zinc in human immunodeficiency virus against opportunistic infections. J. Nutr. 130, 1424S–1431S. doi: 10.1093/jn/130.5.1424S
Mocchegiani, E., Veccia, S., Ancarani, F., Scalise, G., and Fabris, N. (1995). Benefit of oral zinc supplementation as an adjunct to zidovudine (AZT) therapy against opportunistic infections in AIDS. Int. J. Immunopharmacol. 17, 719–727. doi: 10.1016/0192-0561(95)00060-f
Murakami, Y., Koyabu, T., Kawashima, A., Kakibuchi, N., Kawakami, T., Takaguchi, K., et al. (2007). Zinc supplementation prevents the increase of transaminase in chronic hepatitis C patients during combination therapy with pegylated interferon α-2b and ribavirin. J. Nutr. Sci. Vitaminol. 53, 213–218. doi: 10.3177/jnsv.53.213
Myers, S. A., Nield, A., and Myers, M. (2012). Zinc transporters, mechanisms of action and therapeutic utility: implications for type 2 diabetes mellitus. J. Nut. Metabol. 2012, 1–13. doi: 10.1155/2012/173712
Myers, R. P., Ratziu, V., Benhamou, Y., Di Martino, V., Moussalli, J., Hélène Tainturier, M., et al. (2002). Infections with multiple hepatotropic viruses. Polymicro. Dis., 51–73. doi: 10.1128/9781555817947.ch4
Nagamine, T., Takagi, H., Takayama, H., Kojima, A., Kakizaki, S., Mori, M., et al. (2000). Preliminary study of combination therapy with interferon-α and zinc in chronic hepatitis C patients with genotype 1b. Biol. Trace Elem. Res. 75, 53–63. doi: 10.1385/BTER:75:1-3:53
Netzler, N. E., Enosi Tuipulotu, D., Vasudevan, S. G., Mackenzie, J. M., and White, P. A. (2019). Antiviral candidates for treating hepatitis E virus infection. Antimicrob. Agents Chemother. 63, e00003–e00019. doi: 10.1128/aac.00003-19
Nguyen, L. P., Aldana, K. S., Yang, E., Yao, Z., and Li, M. M. (2023). Alphavirus evasion of zinc finger antiviral protein (ZAP) correlates with CpG suppression in a specific viral ns P 2 gene sequence. Viruses 15:830. doi: 10.3390/v15040830
Nie, L., Gao, L., Feng, P., Zhang, J., Fu, X., Liu, Y., et al. (2006). Three-dimensional functionalized tetrapod-like ZnO nanostructures for plasmid DNA delivery. Small 2, 621–625. doi: 10.1002/smll.200500193
Nimgaonkar, I., Ding, Q., Schwartz, R. E., and Ploss, A. (2018). Hepatitis E virus: advances and challenges. Nat. Rev. Gastroenterol. Hepatol. 15, 96–110. doi: 10.1038/nrgastro.2017.150
Nishida, K., Hasegawa, A., Nakae, S., Oboki, K., Saito, H., Yamasaki, S., et al. (2009). Zinc transporter Znt 5/Slc 30a5 is required for the mast cell–mediated delayed-type allergic reaction but not the immediate-type reaction. J. Exp. Med. 206, 1351–1364. doi: 10.1084/jem.20082533
Ogawa, M., Kanda, T., Suganami, A., Nakamoto, S., Win, N. N., Tamura, Y., et al. (2019). Antiviral activity of zinc sulfate against hepatitis A virus replication. Futur. Virol. 14, 399–406. doi: 10.2217/fvl-2019-0031
Ouirane, K. B., Boulard, Y., and Bressanelli, S. (2019). The hepatitis C virus RNA-dependent RNA polymerase directs incoming nucleotides to its active site through magnesium-dependent dynamics within its F motif. J. Biol. Chem. 294, 7573–7587. doi: 10.1074/jbc.RA118.005209
Overbeck, S., Rink, L., and Haase, H. (2008). Modulating the immune response by oral zinc supplementation: a single approach for multiple diseases. Arch. Immunol. Ther. Exp. 56, 15–30. doi: 10.1007/s00005-008-0003-8
Parsons, D. S., Hogstrand, C., and Maret, W. (2018). The C-terminal cytosolic domain of the human zinc transporter ZnT8 and its diabetes risk variant. FEBS J. 285, 1237–1250. doi: 10.1111/febs.14402
Peters, J. L., Dufner-Beattie, J., and Xu, W. (2007). Targeting of the mouse Slc 39a2 (Zip 2) gene reveals highly cell-specific patterns of expression, and unique functions in zinc, iron, and calcium homeostasis. Genesis 45, 339–352. doi: 10.1002/dvg.20297
Plum, L. M., Brieger, A., Engelhardt, G., Hebel, S., Nessel, A., Arlt, M., et al. (2014). PTEN-inhibition by zinc ions augments interleukin-2-mediated Akt phosphorylation. Metallomics 6, 1277–1287. doi: 10.1039/c3mt00197k
Polatnick, J., and Bachrach, H. L. (1978). Effect of zinc and other chemical agents on foot-and-mouth disease virus replication. Antimicrob. Agents Chemother. 13, 731–734. doi: 10.1128/AAC.13.5.731
Prasad, A. S. (2013). Discovery of human zinc deficiency: its impact on human health and disease. Adv. Nutr. 4, 176–190. doi: 10.3945/an.112.003210
Prasad, A. S., Bao, B., Beck, F. W., and Sarkar, F. H. (2011). Zinc-suppressed inflammatory cytokines by induction of A20-mediated inhibition of nuclear factor-κB. Nutrition 27, 816–823. doi: 10.1016/j.nut.2010.08.010
Primadharsini, P. P., Nagashima, S., and Okamoto, H. (2021). Mechanism of cross-species transmission, adaptive evolution and pathogenesis of hepatitis E virus. Viruses 13:909. doi: 10.3390/v13050909
Qiu, M., Chen, Y. U., Chu, Y., Song, S., Yang, N. A., Gao, J., et al. (2013). Zinc ionophores pyrithione inhibits herpes simplex virus replication through interfering with proteasome function and NF-κB activation. Antivir. Res. 100, 44–53. doi: 10.1016/j.antiviral.2013.07.001
Ramakrishnan, D., Xing, W., Beran, R. K., Chemuru, S., Rohrs, H., Niedziela-Majka, A., et al. (2019). Hepatitis B virus X protein function requires zinc binding. J. Virol. 93, e00250–e00219. doi: 10.1128/JVI.00250-19
Read, S. A., O’Connor, K. S., Suppiah, V., Ahlenstiel, C. L., Obeid, S., Cook, K. M., et al. (2017). Zinc is a potent and specific inhibitor of IFN-λ3 signalling. Nat. Commun. 8, 1–15. doi: 10.1038/ncomms15245
Read, S. A., Obeid, S., Ahlenstiel, C., and Ahlenstiel, G. (2019). The role of zinc in antiviral immunity. Adv. Nutr. 10, 696–710. doi: 10.1093/advances/nmz013
Reis e Sousa, C. (2006). Dendritic cells in a mature age. Nat. Rev. Immunol. 6, 476–483. doi: 10.1038/nri1845
Rink, L., and Kirchner, H. (2000). Zinc-altered immune function and cytokine production. J. Nutr. 130:1407S. doi: 10.1093/jn/130.5.1407S
Romanelli, F., Smith, K. M., and Hoven, A. D. (2004). Chloroquine and hydroxychloroquine as inhibitors of human immunodeficiency virus (HIV-1) activity. Curr. Pharm. Des. 10, 2643–2648. doi: 10.2174/1381612043383791
Roohani, N., Hurrell, R., Kelishadi, R., and Schulin, R. (2013). Zinc and its importance for human health: an integrative review. J. Res. Med. Sci. 18, 144–157.
Rükgauer, M., Klein, J., and Kruse-Jarres, J. D. (1997). Reference values for the trace elements copper, manganese, selenium, and zinc in the serum/plasma of children, adolescents, and adults. J. Trace Elem. Med. Biol. 11, 92–98. doi: 10.1016/S0946-672X(97)80032-6
Ruttkay-Nedecky, B., Nejdl, L., Gumulec, J., Zitka, O., Masarik, M., Eckschlager, T., et al. (2013). The role of metallothionein in oxidative stress. Int. J. Mol. Sci. 14, 6044–6066. doi: 10.3390/ijms14036044
Ryu, M. S., Aydemir, T.B., Marriott, B.P., Birt, D.F., Stallings, V.A., and Yates, A.A. (2020). Present knowledge in nutrition.11th ed. Cambridge, Massachusetts: Wiley-Blackwell. 393–408.
Sadeghsoltani, F., Mohammadzadeh, I., Safari, M. M., Hassanpour, P., Izadpanah, M., Qujeq, D., et al. (2021). Zinc and respiratory viral infections: important trace element in anti-viral response and immune regulation. Biol. Trace Elem. Res. 200, 2556–2571. doi: 10.1007/s12011-021-02859-z
Samad, N., Sodunke, T. E., Abubakar, A. R., Jahan, I., Sharma, P., Islam, S., et al. (2021). The implications of zinc therapy in combating the COVID-19 global pandemic. J. Inflamm. Res. 14, 527–550. doi: 10.2147/JIR.S295377
Sheqwara, J., and Alkhatib, Y. (2013). Sideroblastic anemia secondary to zinc toxicity. Blood 122:311. doi: 10.1182/blood-2012-12-469239
Shilagardi, K., Spear, E. D., Abraham, R., Griffin, D. E., and Michaelis, S. (2022). The integral membrane protein ZMPSTE24 protects cells from SARS-CoV-2 spike-mediated pseudovirus infection and syncytia formation. MBio 13:e0254322. doi: 10.1128/mbio.02543-22
Sirelkhatim, A., Mahmud, S., Seeni, A., Kaus, N. H. M., Ann, L. C., Bakhori, S. K. M., et al. (2015). Review on zinc oxide nanoparticles: antibacterial activity and toxicity mechanism. Nano Lett. 7, 219–242. doi: 10.1007/s40820-015-0040-x
Slepchenko, K. G., Lu, Q., and Li, Y. V. (2016). Zinc wave during the treatment of hypoxia is required for initial reactive oxygen species activation in mitochondria. Int. J. Physiol. Pathophysiol. Pharmacol. 8, 44–51.
Slepchenko, K. G., Lu, Q., and Li, Y. V. (2017). Cross talk between increased intracellular zinc (Zn2+) and accumulation of reactive oxygen species in chemical ischemia. Am. J. Phys. Cell Phys. 313, C448–C459. doi: 10.1152/ajpcell.00048.2017
Štefanová, I., Hemmer, B., Vergelli, M., Martin, R., Biddison, W. E., and Germain, R. N. (2003). TCR ligand discrimination is enforced by competing ERK positive and SHP-1 negative feedback pathways. Nat. Immunol. 4, 248–254. doi: 10.1038/ni895
Stempniak, M., Hostomska, Z., Nodes, B. R., and Hostomsky, Z. (1997). The NS3 proteinase domain of hepatitis C virus is a zinc-containing enzyme. J. Virol. 71, 2881–2886. doi: 10.1128/jvi.71.4.2881-2886.1997
Stitt, M. S., Wasserloos, K. J., Tang, X., Liu, X., Pitt, B. R., and Croix, C. M. S. (2006). Nitric oxide-induced nuclear translocation of the metal responsive transcription factor, MTF-1 is mediated by zinc release from metallothionein. Vasc. Pharmacol. 44, 149–155. doi: 10.1016/j.vph.2005.10.004
Stott-Marshall, R. J., and Foster, T. L. (2022). Inhibition of arenavirus entry and replication by the cell-intrinsic restriction factor ZMPSTE24 is enhanced by IFITM antiviral activity. Front. Microbiol. 13:840885. doi: 10.3389/fmicb.2022.840885
Subramanian Vignesh, K., and Deepe, G. S. Jr. (2017). Metallothioneins: emerging modulators in immunity and infection. Int. J. Mol. Sci. 18:2197. doi: 10.3390/ijms18102197
Suda, T., Okawa, O., Shirahashi, R., Tokutomi, N., and Tamano, M. (2019). Changes in serum zinc levels in hepatitis C patients before and after treatment with direct-acting antiviral agents. Hepatol. Res. 49, 1353–1356. doi: 10.1111/hepr.13409
Suzuki, H., Takagi, H., Sohara, N., Kanda, D., Kakizaki, S., Sato, K., et al. (2006). Gunma liver study group triple therapy of interferon and ribavirin with zinc supplementation for patients with chronic hepatitis C: a randomized controlled clinical trial. World J. Gastroenterol. 12, 1265–1269. doi: 10.3748/wjg.v12.i8.1265
Tabatabaeizadeh, S. A. (2022). Zinc supplementation and COVID-19 mortality: a meta-analysis. Eur. J. Med. Res. 27, 70–76. doi: 10.1186/s40001-022-00694-z
Takagi, H., Nagamine, T., Abe, T., Takayama, H., Sato, K., Otsuka, T., et al. (2001). Zinc supplementation enhances the response to interferon therapy in patients with chronic hepatitis C. J. Viral Hepat. 8, 367–371. doi: 10.1046/j.1365-2893.2001.00311.x
Taylor, K. M., and Nicholson, R. I. (2003). The LZT proteins; the LIV-1 subfamily of zinc transporters. Biochim. Biophys. Acta - Biomembr. 1611, 16–30. doi: 10.1016/s0005-2736(03)00048-8
Te Velthuis, A. J., van den Worm, S. H., Sims, A. C., Baric, R. S., Snijder, E. J., and van Hemert, M. J. (2010). Zn2+ inhibits coronavirus and arterivirus RNA polymerase activity in vitro and zinc ionophores block the replication of these viruses in cell culture. PLoS Pathog. 6:e1001176. doi: 10.1371/journal.ppat.1001176
Tellinghuisen, T. L., Marcotrigiano, J., Gorbalenya, A. E., and Rice, C. M. (2004). The NS5A protein of hepatitis C virus is a zinc metalloprotein. J. Biol. Chem. 279, 48576–48587. doi: 10.1074/jbc.M407787200
Thomas, S., Patel, D., Bittel, B., Wolski, K., Wang, Q., Kumar, A., et al. (2021). Effect of high-dose zinc and ascorbic acid supplementation vs usual care on symptom length and reduction among ambulatory patients with SARS-CoV-2 infection: the COVID A to Z randomized clinical trial. JAMA Netw. Open 4:e210369. doi: 10.1001/jamanetworkopen.2021.0369
Traore, K. A., Rouamba, H., Nebie, Y., Sanou, M., Traore, A. S., Barro, N., et al. (2012). Seroprevalence of fecal-oral transmitted hepatitis A and E virus antibodies in Burkina Faso. PLoS One 7:e48125. doi: 10.1371/journal.pone.0048125
Vašák, M. (2005). Advances in metallothionein structure and functions. J. Trace Elem. Med. Biol. 19, 13–17. doi: 10.1016/j.jtemb.2005.03.003
Vento, S., Garofano, T., Renzini, C., Cainelli, F., Casali, F., Ghironzi, G., et al. (1998). Fulminant hepatitis associated with hepatitis a virus superinfection in patients with chronic hepatitis C. N. Engl. J. Med. 338, 286–290. doi: 10.1056/NEJM199801293380503
Von Bülow, V., Dubben, S., Engelhardt, G., Hebel, S., Plümäkers, B., Heine, H., et al. (2007). Zinc-dependent suppression of TNF-α production is mediated by protein kinase A-induced inhibition of Raf-1, IκB kinase β, and NF-κB. J. Immunol. 179, 4180–4186. doi: 10.4049/jimmunol.179.6.4180
Wan, Y., Petris, M. J., and Peck, S. C. (2014). Separation of zinc-dependent and zinc-independent events during early LPS-stimulated TLR4 signaling in macrophage cells. FEBS Lett. 588, 2928–2935. doi: 10.1016/j.febslet.2014.05.043
Wei, Z., Burwinkel, M., Palissa, C., Ephraim, E., and Schmidt, M. F. (2012). Antiviral activity of zinc salts against transmissible gastroenteritis virus in vitro. Vet. Microbiol. 160, 468–472. doi: 10.1016/j.vetmic.2012.06.019
Wenzlau, J. M., Juhl, K., and Yu, L. (2007). The cation efflux transporter ZnT8 (Slc 30A8) is a major autoantigen in human type 1 diabetes. Proc. Natl. Acad. Sci. U. S. A. 104, 17040–17045. doi: 10.1073/pnas.0705894104
Wessels, I., Rolles, B., Slusarenko, A. J., and Rink, L. (2022). Zinc deficiency as a possible risk factor for increased susceptibility and severe progression of Corona virus disease 19. Br. J. Nutr. 127, 214–232. doi: 10.1017/S0007114521000738
Wißing, M. H., Brüggemann, Y., Steinmann, E., and Todt, D. (2021). Virus–host cell interplay during hepatitis E virus infection. Trends Microbiol. 29, 309–319. doi: 10.1016/j.tim.2020.07.002
Wu, X., Chen, P., Lin, H., Hao, X., and Liang, Z. (2016). Hepatitis E virus: current epidemiology and vaccine. Hum. Vaccin. Immunother. 12, 2603–2610. doi: 10.1080/21645515.2016.1184806
Wu, W., Li, R., Li, X., He, J., Jiang, S., Liu, S., et al. (2016). Quercetin as an antiviral agent inhibits influenza A virus (IAV) entry. Viruses 8:6. doi: 10.3390/v8010006
Yu, W., Ji, H., Long, F., Chen, S., He, Q., Xia, Y., et al. (2021). Inhibition of hepatitis E virus replication by zinc-finger antiviral protein synergizes with IFN-β. J. Viral Hepat. 28, 1219–1229. doi: 10.1111/jvh.13522
Yu, Y., Wu, A., Zhang, Z., Yan, G., Zhang, F., Zhang, L., et al. (2013). Characterization of the Guf a subfamily member SLC39A11/Zip 11 as a zinc transporter. J. Nutr. Biochem. 24, 1697–1708. doi: 10.1016/j.jnutbio.2013.02.010
Yuasa, K., Naganuma, A., Sato, K., Ikeda, M., Kato, N., Takagi, H., et al. (2006). Zinc is a negative regulator of hepatitis C virus RNA replication. Liver Int. 26, 1111–1118. doi: 10.1111/j.1478-3231.2006.01352.x
Zhang, Z. Y., Reardon, I. M., Hui, J. O., O'Connell, K. L., Poorman, R. A., Tomasselli, A. G., et al. (1991). Zinc inhibition of renin and the protease from human immunodeficiency virus type 1. Biochemistry 30, 8717–8721. doi: 10.1021/bi00100a001
Zhang, Z. Y., and Xiong, H. M. (2015). Photoluminescent ZnO nanoparticles and their biological applications. Materials 8, 3101–3127. doi: 10.3390/ma8063101
Zheng, R., Jenkins, T. M., and Craigie, R. (1996). Zinc folds the N-terminal domain of HIV-1 integrase, promotes multimerization, and enhances catalytic activity. Proc. Natl. Acad. Sci. 93, 13659–13664. doi: 10.1073/pnas.93.24.13659
Zheng, X., Wang, X., Tu, F., Wang, Q., Fan, Z., and Gao, G. (2017). TRIM25 is required for the antiviral activity of zinc finger antiviral protein. PLoS Pathog. 13, e1006145–e1001128. doi: 10.1371/journal.ppat.1006145
Zhu, Y., Chen, G., Lv, F., Wang, X., Ji, X., Xu, Y., et al. (2011). Zinc-finger antiviral protein inhibits HIV-1 infection by selectively targeting multiply spliced viral mRNAs for degradation. Proc. Natl. Acad. Sci. 108, 15834–15839. doi: 10.1073/pnas.1101676108
Keywords: zinc, viral hepatitis, hepatitis A virus, hepatitis B virus, hepatitis C, hepatitis E virus
Citation: Kumar S, Ansari S, Narayanan S, Ranjith-Kumar CT and Surjit M (2023) Antiviral activity of zinc against hepatitis viruses: current status and future prospects. Front. Microbiol. 14:1218654. doi: 10.3389/fmicb.2023.1218654
Edited by:
Cécile E. Malnou, Université Toulouse III Paul Sabatier, FranceReviewed by:
Perumal Vivekanandan, Indian Institute of Technology Delhi, IndiaSabine Chapuy-Regaud, Institut National de la Santé et de la Recherche Médicale (INSERM), France
Copyright © 2023 Kumar, Ansari, Narayanan, Ranjith-Kumar and Surjit. This is an open-access article distributed under the terms of the Creative Commons Attribution License (CC BY). The use, distribution or reproduction in other forums is permitted, provided the original author(s) and the copyright owner(s) are credited and that the original publication in this journal is cited, in accordance with accepted academic practice. No use, distribution or reproduction is permitted which does not comply with these terms.
*Correspondence: Milan Surjit, bWlsYW5AdGhzdGkucmVzLmlu