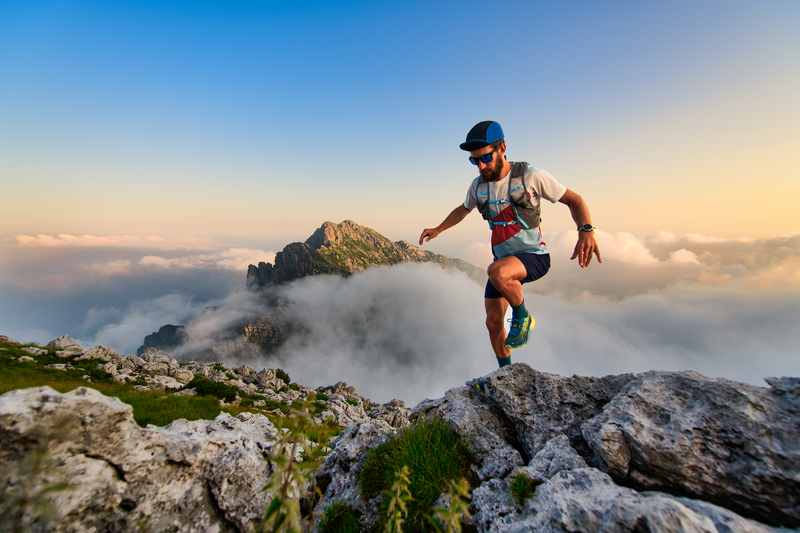
95% of researchers rate our articles as excellent or good
Learn more about the work of our research integrity team to safeguard the quality of each article we publish.
Find out more
REVIEW article
Front. Microbiol. , 27 July 2023
Sec. Microbial Symbioses
Volume 14 - 2023 | https://doi.org/10.3389/fmicb.2023.1208633
This article is part of the Research Topic Ecological interactions between mosquitoes and their microbiota: Implications for pathogen transmission View all 7 articles
The invasive Asian tiger mosquito Aedes albopictus is nowadays broadly distributed with established populations in all continents except Antarctica. In the invaded areas, this species represents an important nuisance for humans and, more relevant, it is involved in the local transmission of pathogens relevant under a public health perspective. Aedes albopictus is a competent vector of parasites such as Dirofilaria and viruses including dengue virus, Zika virus, and chikungunya virus, among others. The mosquito microbiota has been identified as one of the major drivers of vector competence, acting upon relevant vector functions as development or immunity. Here, we review the available literature on the interaction between Ae. albopictus microbiota and pathogen transmission and identify the knowledge gaps on the topic. Most studies are strictly focused on the interplay between pathogens and Wolbachia endosymbiont while studies screening whole microbiota are still scarce but increasing in recent years, supported on Next-generation sequencing tools. Most experimental trials use lab-reared mosquitoes or cell lines, exploring the molecular mechanisms of the microbiota-pathogen interaction. Yet, correlational studies on wild populations are underrepresented. Consequently, we still lack sufficient evidence to reveal whether the microbiota of introduced populations of Ae. albopictus differ from those of native populations, or how microbiota is shaped by different environmental and anthropic factors, but especially, how these changes affect the ability of Ae. albopictus to transmit pathogens and favor the occurrence of outbreaks in the colonized areas. Finally, we propose future research directions on this research topic.
Emerging infectious diseases have spread dramatically during the last decades, driven by human-induced socio-economic and environmental changes (Morand, 2020; Baker et al., 2021). Different components of global change, such as climate change and landscape anthropization, alter the geographic distribution of pathogens and vectors, increasing the exposure of human and animal populations to new diseases. Global trades and travels provide mosquitoes with routes to spread, facilitating and accelerating their expansion, which promotes the colonization of new areas by diseases (Eritja et al., 2017; Ibáñez-Justicia et al., 2020). This scenario creates uncertainty in disease risk for humans (e.g., Schaffner et al., 2013; Akiner et al., 2016), poses a significant threat to people, and implies a global economic (Diagne et al., 2021) and conservation challenge.
Vector-borne diseases are a global concern, particularly those transmitted by mosquitoes (Culicidae) that serve as the primary vectors of pathogens affecting humans and other animals (Tolle, 2009; WHO, 2020). Approximately 10% of known mosquito species are recognized as potential or confirmed vectors of pathogens with public health relevance (Yee et al., 2022). Mosquitoes of the Aedes genus includes numerous vector species of great concern due to their highly invasive character. The yellow fever mosquito (Aedes aegypti), the Asian tiger mosquito (Aedes albopictus), and, to a lesser extent, the Korean bush mosquito (Aedes koreicus) and the rock pool mosquito (Aedes japonicus) are representative examples (Cebrián-Camisón et al., 2020). Aedes aegypti and Ae. albopictus are competent vectors of arboviruses including dengue virus (DENV), chikungunya virus (CHIKV), yellow fever virus (YFV), and Zika virus (ZIKV) (Gutiérrez-López et al., 2023). Although originally from Africa and Asia, respectively, Ae. aegypti and Ae. albopictus are the most invasive mosquitoes in the world. They have been transported globally through international trade and shipping activities (Reiter and Sprenger, 1987; Powell and Tabachnick, 2013; Laporta et al., 2023), creating novel epidemiological scenarios worldwide. Aedes albopictus, in particular, has spread from its native range to, at least, 28 other countries worldwide (ECDC, 2023; Laporta et al., 2023). In Europe, for example, this species was first detected in Albania in 1979 and spread during the 1980s. Nowadays, it is established in more than 15 European countries (ECDC, 2023; Laporta et al., 2023), including all the European Mediterranean Basin. The local abundance of Ae. albopictus, coupled with the presence of infected hosts and other factors, has allowed the occurrence of local outbreaks of imported diseases, such as chikungunya fever in Italy and France (Tomasello and Schlagenhauf, 2013; Calba et al., 2017; Venturi et al., 2017), dengue in Croatia, France and Spain (Tomasello and Schlagenhauf, 2013; Delisle et al., 2015; Succo et al., 2016) and Zika in France (Giron et al., 2019). Moreover, Ae. albopictus has been implicated in the local transmission of Dirofilaria parasites from animal reservoirs to humans in Asia, USA, and Italy (Cancrini et al., 2003, 2007; Gratz, 2004).
The importance of a particular mosquito species for the transmission of pathogens is determined by different components of vector competence (Beerntsen et al., 2000; Stewart Merrill and Johnson, 2020), which refer to the ability of mosquitoes to successfully acquire, maintain, and transmit a pathogen between hosts. Understanding this concept is key to predict the potential of mosquitoes to create new epidemiological scenarios. It is, therefore, crucial to investigate how external factors, such as breeding sites characteristics, and internal factors, such as symbiotic bacteria, affect the vector competence of a particular species in natural ecosystems (Lefèvre et al., 2013; Stewart Merrill and Johnson, 2020). Over the past few decades, researchers have shown that mosquito microbiota plays a critical role in shaping vector competence (Cansado-Utrilla et al., 2021), modulating important functions including the development (Coon et al., 2014, 2016), behavior (Ruiz-Lopez, 2020), digestion and reproduction (Fouda et al., 2001), or physiology and immunity (Dong et al., 2009; Kambris et al., 2010) of insect vectors. Mosquito microbiota has been identified as a key component determining the development of pathogens in the mosquito midgut, as well as mosquito resistance to pathogens and the cost of parasite infections in terms of survival rate [reviewed in Clayton et al. (2014), Gabrieli et al. (2021)]. Consequently, the composition of the microbiota of wild mosquitoes may impact their vector competence and the dynamics of transmission of mosquito-borne pathogens (Boissière et al., 2012; Charan et al., 2013; Souza-Neto et al., 2019). Indeed, previous studies have identified environmental-related differences in the microbiota between mosquito species and populations (Coon et al., 2016; Muturi et al., 2017; Duguma et al., 2019) that could affect their competence (Martínez-de la Puente et al., 2018) and, ultimately, may partially explain the differences in the communities of parasites in host populations.
Based on the significance of mosquito microbiota in the transmission of pathogens, and the relevance of Ae. albopictus as a major vector of mosquito-borne pathogens worldwide, the aim of this article is review the role of Ae. albopictus microbiota in pathogen transmission. While most recent findings rely on Ae. aegypti (see, for example, Ramirez et al., 2012; Monteiro et al., 2019), our knowledge on how microbiota affects pathogen transmission in other species of concern is limited. Given the potential threat posed by the widespread expansion of Ae. albopictus, it is crucial to unravel how environmental heterogeneity affects mosquito microbiota and to determine the mechanisms mediating its effects on vector competence (Stewart Merrill and Johnson, 2020) and disease risk in both endemic and invaded areas. Here, we overview the published knowledge on factors shaping microbiota of individual mosquitoes and populations and how impact pathogen transmission, attending also to the molecular methods used in the studies. Given the significant role of certain endosymbionts, mainly Wolbachia spp. and Asaia spp., in mosquito pathogen transmission (Johnson, 2015; Rosso et al., 2018; Ross et al., 2019), they were also considered in this review. Finally, we identified current knowledge gaps on the topic and suggest future research directions in the area.
One of the earliest studies on the tripartite interaction between Ae. albopictus, pathogens, and mosquito bacterial communities screened chikungunya (CHIKV)-infected and uninfected mosquitoes for differences in Wolbachia abundances (Tortosa et al., 2008). Indeed, a significant amount of publications on the topic aims to determine the presence and/or abundance of certain endosymbionts that may be relevant for pathogen transmission (see Table 1 for examples). Wolbachia is the most paradigmatic example, its potential as vector borne diseases suppressor has been discussed at length in recent decades (e.g., Hedges et al., 2008; Hoffmann et al., 2015). Several naturally-occurring Wolbachia strains have been identified in a range of species of mosquitoes (Bourtzis et al., 2014; Sicard et al., 2019). Specifically, Ae. albopictus is naturally infected by the strains wAlbA and wAlbB, whose impact on viral infections has been assessed in a fair number of studies, mainly on the viruses CHIKV (Tortosa et al., 2008; Raquin et al., 2015), DENV (dengue virus; Mousson et al., 2012; Huang et al., 2020; Sasaki et al., 2022), and ZIKV (Huang et al., 2020), but also in other pathogens, as entomopathogenic fungi (Ramirez et al., 2021). Molecular techniques, such as PCR and qPCR, have been extensively used in all those studies focused on a single endosymbiotic bacterium. Sometimes in combination with other analytical tools as, for example, the use of taxonomic microarrays (Zouache et al., 2012), immunoassays techniques (McLean et al., 2019), or cell lines cultures (Mousson et al., 2012), usually used in transinfection trials.
Table 1. Summary of the relevant information contained in the articles most consulted for the review on the interplay between vector microbial community and pathogen transmission on the invasive Asian tiger mosquito, Aedes albopictus.
Besides natural strains, transfection of Wolbachia among insect species has been suggested as an efficient mechanism to block pathogen transmission in vectors (e.g., Hedges et al., 2008; Hoffmann et al., 2015). Viral infections are limited in Ae. albopictus mosquito cell lines transinfected with the wMel and wMelPop strains from Drosophila melanogaster (McLean et al., 2019; Teramoto et al., 2019; Bhattacharya et al., 2020), the wAu from Drosophila simulans (Mancini et al., 2020), or the wStri isolated from the planthopper Laodelphax striatellus (Schultz et al., 2017, 2018; Bhattacharya et al., 2020). The potential of other endosymbionts, as Asaia or Pantoea, as efficaciously suppressors of vector borne diseases has also been proposed (Guégan et al., 2018b), although we lack specific studies deepening on their effect on Ae. albopictus’ vector competence (but see, for example, Rosso et al., 2018; Seabourn et al., 2020).
The screening of microbial communities has revealed as a practical tool to detect new insect symbionts potentially involved in pathogen transmission. The development of novel molecular techniques, together with the reduction of their costs, have popularized these studies in the last years. New high-throughput sequencing techniques, as next-generation sequencing (NGS), have been used to characterize Ae. albopictus microbiota in relation to its vector competence (Seabourn et al., 2020; Onyango et al., 2021; Zhao et al., 2022). Most studies are aimed to unveil the role of vector microbial communities on ZIKV and DENV transmission (e.g., Duchemin et al., 2017; Schultz et al., 2018; Huang et al., 2020; Mancini et al., 2020). Complementary, these techniques have been applied to detect how microbiota variations of mosquitoes with different statuses, such as food regime, correlate with infections by ZIKV (Onyango et al., 2021), MAYV (Pereira et al., 2021), or DENV (Zhao et al., 2022). In addition, whole microbiota has been screened by Seabourn et al. (2020) using NGS, despite being focused on wAlbA and wAlbB and the infection by A. taiwanensis, while Calle-Tobón et al. (2022) do it to screen whole viromes.
Strictly, vector competence should be assessed using specific trials to discriminate from alternative mechanisms or findings (see, for example, Stewart Merrill and Johnson, 2020), for example, estimating the infection success, dissemination, and transmission rates of pathogens in mosquitoes (see, for example, Gutiérrez-López et al., 2019). Those criteria have been applied to assess Ae. albopictus’ vector competence in relation to Wolbachia and, to a lesser extent, to the whole microbiota (Table 1). Good example of the latter is the experimental trial performed by Onyango et al. (2021) where the effect of ZIKV infection and temperature regimes on microbiota composition was evaluated in three experimental groups: individuals fed only with 10% sucrose, and those fed with infected- and uninfected-blood. Results were consistent with those of Muturi et al. (2016), compared to sucrose-fed individuals, that is mosquitoes fed with blood showed a significant reduction in the microbiota diversity, being more pronounced in those fed with ZIKV-infected blood meals. Moreover, Onyango et al. (2021) observed a negative correlation between ZIKV and the Elizabethkingia anophelis albopictus strain, as well as a reduction in ZIKV infection when mosquitoes were fed with a supplemental diet of E. anophelis aegypti.
Following a similar approach, Pereira et al. (2021) studied the impact of certain Wolbachia strains on the vector potential of Ae. albopictus for Mayaro virus (MAYV). Adult females were fed with MAYV-infected blood and, several days after, infection and dissemination were assessed by screening head and thorax samples for the presence of the virus. Subsequently, transmission was tested infecting naïve Ae. aegypti individuals with the saliva of MAYV-infected Ae. albopictus. Results from the analyses of head and thorax tissues suggested that Wolbachia wAlbB inhibits MAYV, indicating a possible effect on pathogen transmission. In other trials, neither Duchemin et al. (2017), working with Australian-native Ae. albopictus population, nor Sasaki et al. (2022), comparing native and non-native populations, detected an effect of Wolbachia on ZIKV and DENV transmission, respectively, but infection/dissemination were experimentally proved. Yet, Mousson et al. (2012) demonstrated that Wolbachia limits DENV transmission, estimated as the virus load in the mosquito salivary glands, but not oral infection or viral replication (dissemination). For Ae. albopictus, Wolbachia transinfection from Drosophila simulans (wAu strain) has been proved to effectively blocks ZIKV infection (Mancini et al., 2020).
Beyond the mentioned experimental approaches, valuable information on Ae. albopictus pathogens transmission can be extracted from other studies not directly assessing vector competence but other related components. Zhao et al. (2022) detected changes in the microbiota composition of female mosquitoes before and after DENV experimental infection, identifying those bacterial groups whose abundance varied significantly (e.g., Neurospora crassa, Gammaproteobacteria spp., and Lactobacillus harbinensis). Moreover, authors identified certain microorganisms, such as Alphaproteobacteria, that may be involved in the DENV susceptibility of Ae. albopictus, as they may affect immunity and/or metabolism of mosquitoes. The abundance of Alphaproteobacteria and Gammaproteobacteria, as well as other groups such as the Enterobacteriaceae family, also varied in response to oral CHIKV infections (Zouache et al., 2012). A specially relevant finding is the reduction of endosymbiont abundance, such as Wolbachia, which suggests the existence of competition mechanisms between the virus and the endosymbiont (Hawlena et al., 2022). Studies on single endosymbionts also supported this hypothesis: a slight reduction in the density of Wolbachia in mosquitoes was observed upon CHIKV experimental infection (Tortosa et al., 2008). Complementary, Wolbachia clearance from natural-infected mosquitoes using antibiotics did not affect their susceptibility to fungal entomopathogens, but did affect fungi abundance and some gene expression patterns related to defense against fungal infections (Ramirez et al., 2021).
Cell culture techniques have also contributed to disentangling the mechanisms by which endosymbionts act as suppressors of pathogenic agents (examples in Table 1). Support for the resource-competition theory between Wolbachia and certain pathogens is also provided from cell-lines experiments. In different mosquito cell lines (e.g., C710, C/wStri1, Aa23, C6/36 cells) stably-infected with the Wolbachia wAlbB and wStri (transinfected from Laodelphax striatellus) strains, LaCrosse virus (LCV) or vesicular stomatitis virus (VSV) infections were unaffected, while infections caused by DENV, CHIKV, ZIKV, and yellow fever viruses were constrained (Schultz et al., 2017, 2018). However, cholesterol supplementation allows Wolbachia and ZIKV to grow together. Moreover, Bhattacharya et al. (2020) provided molecular insights on how Wolbachia (strains wAlbB, wStri, and wMel from Drosophila melanogaster) inhibits the early stages of infections by different Flaviviridae and Togaviridae viruses, including ZIKV, CHIKV, and Sindbis virus (SINV) in the cell line RML-12. Similarly, McLean et al. (2019) detected no replication of the flavivirus cell-fusing agent virus (CFAV) in RML-12 Wolbachia-infected cell lines (with the strains wMel and wMelPop-CLA from Drosophila melanogaster), nor even in the Ae. albopictus (C6/36) cell line (which lacks functional antiviral RNAi response) but did for the Phasi Charoen-like virus (PCLV). The replication, assembly, and secretion of CHIKV were also restricted in mosquito C6/36 cell line in presence of the Wolbachia wAlbB strain (Raquin et al., 2015), as occurs for other Flavivirus and Alphavirus (Ekwudu et al., 2020), or with DENV-type2 in the presence of the pathogenic strain wMelPop (Teramoto et al., 2019) using the same cell line.
Correlational studies must definitively contribute to our understanding of the interplay among bacterial communities and pathogen transmission. At early research stages, correlations can provide inputs that must be lately disentangled under experimental conditions. For example, a survey of different natural populations on the island of Maui, Hawai‘I, revealed that bacterial microbiota varied according to geographic location but also to infection by Ascogregarina taiwanensis, a protozoan parasite of mosquitoes (Seabourn et al., 2020). Significant differences in midgut bacterial communities of Ae. albopictus (as well as Ae. aegypti and Aedes vittatus) were also detected in different populations from dengue endemic and non-endemic areas in India (Charan et al., 2016). In Hong Kong metropolitan area, a study failed to detect the presence of DENV and ZIKV in Ae. albopictus, while Wolbachia was stably present in 80% of the samples analyzed (Huang et al., 2020). Another survey in Colombia examined the virome composition in relation to the presence of Wolbachia in field-caught mosquitoes (Calle-Tobón et al., 2022) results showed that Wolbachia was unrelated with any significant change in the virome richness, diversity, or abundance.
Aedes albopictus is a known vector of emerging and re-emerging arboviruses of special concern (Gutiérrez-López et al., 2023), mostly the ZIKV, DENV, CHIKV arboviruses that produced outbreaks in several countries worldwide, raising an unprecedent number of clinical cases (Fritzell et al., 2018). This is reflected in the available literature (see Table 1 for examples): a growing number of publications on the role of Ae. albopictus microbiota in the transmission of ZIKV and DENV were published following the 2015–2016 Zika epidemic (WHO, 2022) and the 2019 dengue outbreaks (WHO, 2019), respectively. The interplay between the Wolbachia and vector competence for ZIKV has been explored for different strains: Cambodia 2010, African MR766, and Puerto Rico PRVABC59 (Duchemin et al., 2017; Schultz et al., 2017, 2018). Apart from the above mentioned study on DENV-2 New Guinea strains (Mancini et al., 2020), other works focused exclusively on the DENV-2 strain, the one causing the more severe health cases in humans, confirmed the capacity of Wolbachia to limit its infection (e.g., Mousson et al., 2012; Teramoto et al., 2019). Differential susceptibilities to the three DENV serotypes (DENV1, DENV2, and DENV3) were found among native and non-native Ae. albopictus populations, naturally infected with Wolbachia-infected (Sasaki et al., 2022). However, it is important to clarify that the different virus strains are not equally represented, although they may respond differential to vector microbiota composition. The work of Teramoto et al. (2019) constitutes a good example since they detected differences in the mosquito susceptibility to infection by three strains of DENV. In. addition, inconclusive results have been found for natural undefined DENV strains (Huang et al., 2020), or among dengue-endemic and non-endemic natural areas (Charan et al., 2016). Further studies have simultaneously assessed the effects of Wolbachia on ZIKV and DENV natural strains (Huang et al., 2020), on ZIKV strain MP1751 and DENV-2 New Guinea C-strain (Mancini et al., 2020), on ZIKV (MR766 Uganda Strain) with SINV-nLuc and CHIKV18125-capsid-mKate (Bhattacharya et al., 2020), and, on three different ZIKV strains (the Brazilian KU365780, the French Polynesian H/PF/2013, and African MR766 strains) together with other arbovirus strains: DENV-2 ET300, WNV-KUN (MRM 16), RRV (T48), BFV (16313), and SINV-MRM39 (Ekwudu et al., 2020). More recently, Onyango et al. (2021) screened the entire bacterial community of Ae. albopictus mosquitoes, evaluating its effect on vector competence for the ZIKV lab strain HND 2016–19563.
Studies on the impact of Ae. albopictus microbiota on the development of CHIKV have also been conducted. The E1-226V CHIKV variant has been used to test the responses to infection of both, the microbiota (Zouache et al., 2012) and Wolbachia natural strains (Tortosa et al., 2008). The role of endosymbionts as pathogen blockers has been also tested against the CHIKV 06.21 (Raquin et al., 2015) and the CHIKV 131/25 strains (Schultz et al., 2018). Lastly, CHIKV18125-capsid-mKate infectivity was tested in the presence of Wolbachia (Bhattacharya et al., 2020), along with that of ZIKV and Sindbis nLuc reporter (SINV-nLuc) viruses.
Further research has explored the impact of Ae. albopictus microbiota in the transmission of other pathogens such as Mayaro virus (MAYV; Pereira et al., 2021), Ascogregarina taiwanensis (Seabourn et al., 2020), the entomopathogenic fungi Beauveria bassiana (MBC076) and Beauveria brongniartii (MBC397) (Ramirez et al., 2021), and the Cell-fusing agent virus (CFLV) and Phasi Charoen-like virus (PCLV) (McLean et al., 2019). Calle-Tobón et al. (2022) screened the mosquito virome looking for pathogenic viruses, despite not find any, pathogen screening can be integrated as a feasible solution to not be limited at detecting pathogens of a specific group.
Originally from Asia, Ae. albopictus is currently one of the most invasive mosquito species in the world (ECDC, 2023; Laporta et al., 2023), facing new environmental conditions of colonized areas, which may affect its microbiota and, potentially, its vector competence (e.g., Minard et al., 2015; Coon et al., 2016; Muturi et al., 2017; Duguma et al., 2019). Recent studies have identified simplified microbiota in Ae. albopictus from the invaded areas with respect to those of the native distribution range. The comparison of the microbiota of Ae. albopictus from Vietnam (native area) and France (invaded populations) revealed a lower bacterial diversity in invaded areas (Minard et al., 2015). Similarly, mosquitoes from Italy showed a lower diversity and a different composition of bacteria than those from the native area (Rosso et al., 2018). Apart from the studies focused on bacterial microbiota, a complementary article compared the fungal microbiota (mycobiome) of native versus introduced populations (Luis et al., 2019). These differences in the composition of the mosquito microbiota may impact their ability for the transmission of pathogens under natural conditions, as suggested by the reported variations in mosquito microbiota of wild populations between dengue-endemic and not endemic areas within its natural area of distribution (e.g., Charan et al., 2016; Huang et al., 2020, Hong Kong and India, respectively) or those in the fungal microbiota populations exposed to different environmental conditions (Tawidian et al., 2021). Additional surveys were carried out in colonized countries, including two in South America (Colombia and Brazil) (Pereira et al., 2021; Calle-Tobón et al., 2022, respectively), and in the Pacific island of Maui (USA) (Seabourn et al., 2020), but these studies did not compared their results from native populations of the species.
In addition, different studies have identified the composition of the microbiota of laboratory colonies established from wild eggs, larvae, or adult Ae. albopictus mosquitoes collected, among other places, in China (Zhao et al., 2022), Japan (Mancini et al., 2020; Sasaki et al., 2022), Malaysia, the island of La Reunión (Tortosa et al., 2008; Mousson et al., 2012; Zouache et al., 2012), continental USA (Onyango et al., 2021), or from north Australia (Duchemin et al., 2017). However, Ae. albopictus microbiota may differ between field collected mosquitoes and those reared in the laboratory (Baltar et al., 2023) but also between generations within a laboratory colony or wild collected individuals (Hegde et al., 2015), potentially limiting the conclusions obtained about the potential effects of microbiota composition on pathogen development.
In addition to the differential composition of Ae. albopictus microbiota between geographically distant areas, mosquito microbiota may largely vary at a local scale. Temporal variation in the microbiota of Ae. albopictus have been reported (Saab et al., 2020). Furthermore, different human activities, such as deforestation or urbanization, may alter the mosquito-pathogen relationships (Ferraguti et al., 2016), this is especially relevant for highly anthropogenic species as Ae. albopictus. For example, Thongsripong et al. (2018) found a richer bacterial microbiota of Ae. aegypti in rural than in suburban habitats. The use of insecticides or antibiotics have been found to affect the bacterial community of Ae. albopictus (Guégan et al., 2018a; Juma et al., 2020), modifying the abundance of bacteria with a known role on pathogen transmission including Elizabethkingia spp. and Wolbachia spp. Yet, the direct impact of anthropogenic stressor on vector competence and pathogen transmission remains to be measured for Ae. albopictus.
As far as we know, this is the first review on vector’s microbial community and pathogen transmission on Ae. albopictus. Together with Ae. aegypti, the Asian tiger mosquito is one of the species spreading faster and more globally in the last decades (ECDC, 2023; Laporta et al., 2023) representing a public health concern. Thus, the information contained in this review provides valuable information for the tracking, control, and prevention of arboviruses transmitted by this mosquito species.
The number of publications on this research topic has increased during the last years, reflecting the growing interest in understanding how disease transmission is shaped by the vector-pathogen-environment interplay. However, the simultaneous study of all components of this tripartite interaction has been traditionally neglected. Interestingly, authors such as Onyango et al. (2021) studied vector competence, experimental infection, and bacteriome response of mosquitoes at same time. However, almost all screened publications can be divided into three main categories: (i) those correlating infection patterns with the presence of Wolbachia or, alternatively, to other groups in mosquitoes collected in wild populations, (ii) those detecting changes in the microbiota/endosymbionts of mosquitoes related to geographical locations or sites with differential infection rates by certain pathogens, and (iii) those experimentally assessing the endosymbiont/microbiota-pathogen in cell lines or laboratory colonies. Nonetheless, their contribution to our knowledge on the interactions between mosquitoes, microbiota and pathogen transmission has been crucial. For example, there is accumulate correlative evidence showing a negative relationship between Wolbachia strains and certain pathogens as DENV or ZIKV. Yet, there is limited evidence to reveal whether introduced populations of Ae. albopictus harbor the same, different, or a simplified microbiota than mosquitoes from native populations (but see Minard et al., 2015), as well as those with different origins, but especially, how these changes affect their vector potential affecting the occurrence of outbreaks in the colonized areas. In addition, further studies should explore the impact of the different components of the global change scenario as environmental drivers impacting bacterial communities and pathogen transmission in natural Ae. albopictus populations. This is specially the case of landscape anthropization which affect the exposure to antibiotics and other pollutants in surface water, among other factors, may impact the microbiota composition of mosquitoes and, therefore, their interactions with pathogens. In this respect, the importance of mosquito microbiota in the transmission of Ae. albopictus of different pathogens should be addressed for a number of pathogens that are able to transmit including the zoonotic Dirofilaria for which, to our knowledge, we lack basic information on the relevance of Ae. albopictus microbiota in their transmission.
JM-P and MGarrido conceived the original idea. MGarrido wrote the first original draft of the manuscript and subsequent versions with considerable assistance from JM-P, JV, and MGarrigós. All authors contributed to manuscript revision.
This study was financed by the PID2020-118205GB-I00 grant to JM-P funded by MCIN/AEI/10.13039/501100011033. MGarrido was supported by the María Zambrano program and JV received financial support from the Margarita Salas and Juan de la Cierva programs. MGarrigós was supported by a FPI grant (PRE2021-098544).
The authors declare that the research was conducted in the absence of any commercial or financial relationships that could be construed as a potential conflict of interest.
All claims expressed in this article are solely those of the authors and do not necessarily represent those of their affiliated organizations, or those of the publisher, the editors and the reviewers. Any product that may be evaluated in this article, or claim that may be made by its manufacturer, is not guaranteed or endorsed by the publisher.
Akiner, M. M., Demirci, B., Babuadze, G., Robert, V., and Schaffner, F. (2016). Spread of the invasive mosquitoes Aedes aegypti and Aedes albopictus in the black sea region increases risk of chikungunya, dengue, and zika outbreaks in Europe. PLoS. Negl. Trop. Dis. 10:e0004664. doi: 10.1371/journal.pntd.0004664
Baker, R. E., Mahmud, A. S., Miller, I. F., Rajeev, M., Rasambainarivo, F., Rice, B. L., et al. (2021). Infectious disease in an era of global change. Nat. Rev. Microbiol. 20, 193–205. doi: 10.1038/s41579-021-00639-z
Baltar, J. M. C., Pavan, M. G., Corrêa-Antônio, J., Couto-Lima, D., Maciel-De-Freitas, R., and David, M. R. (2023). Gut bacterial diversity of field and laboratory-reared Aedes albopictus populations of Rio de Janeiro, Brazil. Viruses 15:1309. doi: 10.3390/v15061309
Beerntsen, B. T., James, A. A., and Christensen, B. M. (2000). Genetics of mosquito vector competence. Microbiol. Mol. Biol. Rev. 64, 115–137.
Bhattacharya, T., Newton, I. L. G., and Hardy, R. W. (2020). Viral RNA is a target for Wolbachia-mediated pathogen blocking. PLoS Pathog. 16:e1008513. doi: 10.1371/journal.ppat.1008513
Boissière, A., Tchioffo, M. T., Bachar, D., Abate, L., Marie, A., Nsango, S. E., et al. (2012). Midgut microbiota of the malaria mosquito vector anopheles gambiae and interactions with plasmodium falciparum infection. PLoS Pathog. 8:e1002742. doi: 10.1371/journal.ppat.1002742
Bourtzis, K., Dobson, S. L., Xi, Z., Rasgon, J. L., Calvitti, M., Moreira, L. A., et al. (2014). Harnessing mosquito–Wolbachia symbiosis for vector and disease control. Acta Trop. 132, S150–S163. doi: 10.1016/j.actatropica.2013.11.004
Calba, C., Guerbois-Galla, M., Franke, F., Jeannin, C., Auzet-Caillaud, M., Grard, G., et al. (2017). Preliminary report of an autochthonous chikungunya outbreak in France, July to September 2017. Euro Surveill. 22, 17–00647. doi: 10.2807/1560-7917.ES.2017.22.39.17-00647
Calle-Tobón, A., Pérez-Pérez, J., Forero-Pineda, N., Chávez, O. T., Rojas-Montoya, W., Rúa-Uribe, G., et al. (2022). Local-scale virome depiction in Medellín, Colombia, supports significant differences between Aedes aegypti and Aedes albopictus. PLoS One 17:e0263143. doi: 10.1371/journal.pone.0263143
Cancrini, G., Frangipane di Regalbono, A., Ricci, I., Tessarin, C., Gabrielli, S., and Pietrobelli, M. (2003). Aedes albopictus is a natural vector of Dirofilaria immitis in Italy. Vet. Parasitol. 118, 195–202. doi: 10.1016/j.vetpar.2003.10.011
Cancrini, G., Scaramozzino, P., Gabrielli, S., Paolo, M. D., Toma, L., and Romi, R. (2007). Aedes albopictus and culex pipiens implicated as natural vectors of Dirofilaria repens in Central Italy. J. Innate Immun. 44, 1064–1066. doi: 10.1603/0022-2585(2007)44[1064:aaacpi]2.0.co;2
Cansado-Utrilla, C., Zhao, S. Y., Mccall, P. J., Coon, K. L., and Hughes, G. L. (2021). The microbiome and mosquito vectorial capacity: Rich potential for discovery and translation. Microbiome 9:111. doi: 10.1186/s40168-021-01073-2
Cebrián-Camisón, S., Martínez-de la Puente, J., and Figuerola, J. (2020). A literature review of host feeding patterns of invasive aedes mosquitoes in Europe. Insects 11:848. doi: 10.3390/insects11120848
Charan, S. S., Pawar, K. D., Gavhale, S. D., Tikhe, C. V., Charan, N. S., Angel, B., et al. (2016). Comparative analysis of midgut bacterial communities in three aedine mosquito species from dengue-endemic and non-endemic areas of Rajasthan, India. Med. Vet. Entomol. 30, 264–277. doi: 10.1111/mve.12173
Charan, S. S., Pawar, K. D., Severson, D. W., Patole, M. S., and Shouche, Y. S. (2013). Comparative analysis of midgut bacterial communities of Aedes aegypti mosquito strains varying in vector competence to dengue virus. Parasitol. Res. 112, 2627–2637. doi: 10.1007/s00436-013-3428-x
Clayton, A. M., Dong, Y. M., and Dimopoulos, G. (2014). The anopheles innate immune system in the defense against malaria infection. J. Innate Immun. 6, 169–181.
Coon, K. L., Brown, M. R., and Strand, M. R. (2016). Mosquitoes host communities of bacteria that are essential for development but vary greatly between local habitats. Mol. Ecol. 25, 5806–5826. doi: 10.1111/mec.13877
Coon, K. L., Vogel, K. J., Brown, M. R., and Strand, M. R. (2014). Mosquitoes rely on their gut microbiota for development. Mol. Ecol. 23, 2727–2739.
Delisle, E., Rousseau, C., Broche, B., Leparc-Goffart, I., L’AMBERT, G., Cochet, A., et al. (2015). Chikungunya outbreak in Montpellier, France, September to October 2014. Euro Surveill. 20:21108. doi: 10.2807/1560-7917.es2015.20.17.21108
Diagne, C., Leroy, B., Vaissière, A.-C., Gozlan, R. E., Roiz, D., Jarić, I., et al. (2021). High and rising economic costs of biological invasions worldwide. Nature 592, 571–576.
Dong, Y. M., Manfredini, F., and Dimopoulos, G. (2009). Implication of the mosquito midgut microbiota in the defense against malaria parasites. PLoS Pathog. 5:e1000423. doi: 10.1371/journal.ppat.1000423
Duchemin, J. B., Mee, P. T., Lynch, S. E., Vedururu, R., Trinidad, L., and Paradkar, P. (2017). Zika vector transmission risk in temperate Australia: A vector competence study. Virol. J. 14, 108. doi: 10.1186/s12985-017-0772-y
Duguma, D., Hall, M. W., Smartt, C. T., Debboun, M., and Neufeld, J. D. (2019). Microbiota variations in Culex nigripalpus disease vector mosquito of West Nile virus and Saint Louis Encephalitis from different geographic origins. PeerJ 6:e6168. doi: 10.7717/peerj.6168
Ekwudu, O. M., Devine, G. J., Aaskov, J. G., and Frentiu, F. D. (2020). Wolbachia strain wAlbB blocks replication of flaviviruses and alphaviruses in mosquito cell culture. Parasit. Vectors 13:54. doi: 10.1186/s13071-020-3936-3
Eritja, R., Palmer, J. R. B., Roiz, D., Sanpera-Calbet, I., and Bartumeus, F. (2017). Direct evidence of adult Aedes albopictus Dispersal by Car. Sci. Rep. 7:14399. doi: 10.1038/s41598-017-12652-5
Ferraguti, M., Martínez-de la Puente, J., Roiz, D., Ruiz, S., Soriguer, R., and Figuerola, J. (2016). Effects of landscape anthropization on mosquito community composition and abundance. Sci. Rep. 6:29002.
Fouda, M. A., Hassan, M. I., Al-Daly, A. G., and Hammad, K. M. (2001). Effect of midgut bacteria of Culex pipiens L. on digestion and reproduction. J. Egypt Soc. Parasitol. 31, 767–780.
Fritzell, C., Rousset, D., Adde, A., Kazanji, M., Van Kerkhove, M. D., and Flamand, C. (2018). Current challenges and implications for dengue, chikungunya and Zika seroprevalence studies worldwide: A scoping review. PLoS. Negl. Trop. Dis. 12:e0006533. doi: 10.1371/journal.pntd.0006533
Gabrieli, P., Caccia, S., Varotto-Boccazzi, I., Arnoldi, I., Barbieri, G., Comandatore, F., et al. (2021). Mosquito trilogy: Microbiota, immunity and pathogens, and their implications for the control of disease transmission. Front. Microbiol. 12:630438. doi: 10.3389/fmicb.2021.630438
Giron, S., Franke, F., Decoppet, A., Cadiou, B., Travaglini, T., Thirion, L., et al. (2019). Vector-borne transmission of Zika virus in Europe, southern France, August 2019. Eurosurveillance 24, 1900655. doi: 10.2807/1560-7917.ES.2019.24.45.1900655
Gratz, N. G. (2004). Critical review of the vector status of Aedes albopictus. Med. Vet. Entomol. 18, 215–227. doi: 10.1111/j.0269-283X.2004.00513.x
Guégan, M., Zouache, K., Démichel, C., Minard, G., Tran, V., Potier, P., et al. (2018b). The mosquito holobiont: Fresh insight into mosquito-microbiota interactions. Microbiome 6:49. doi: 10.1186/s40168-018-0435-2
Guégan, M., Minard, G., Tran, F.-H., Tran, V., Dubost, A., and Valiente, M. (2018a). Short-term impacts of anthropogenic stressors on Aedes albopictus mosquito vector microbiota. FEMS Microbiol. Ecol. 94:fiy188. doi: 10.1093/femsec/fiy188
Gutiérrez-López, R., Bialosuknia, S. M., Ciota, A. T., Montalvo, T., Martínez-De, L. P., Gangoso, L., et al. (2019). Vector competence of Aedes caspius and A. albopictus mosquitoes for zika virus, Spain. Emerg. Infect. Dis. 25, 346–348. doi: 10.3201/eid2502.171123
Gutiérrez-López, R., Figuerola, J., and Martínez-de la Puente, J. (2023). Methodological procedures explain observed differences in the competence of European populations of Aedes albopictus for the transmission of Zika virus. Acta Trop. 237:106724. doi: 10.1016/j.actatropica.2022.106724
Hawlena, H., Garrido, M., Cohen, C., Halle, S., and Cohen, S. (2022). Bringing the mechanistic approach back to life: A Systematic review of the experimental evidence for coexistence and four of its classical mechanisms. Front. Ecol. Evol. 10:898074. doi: 10.3389/fevo.2022.898074
Hedges, L. M., Brownlie, J. C., O’NEILL, S. L., and Johnson, K. N. (2008). Wolbachia and virus protection in insects. Science 322:702. doi: 10.1126/science.1162418
Hegde, S., Rasgon, J. L., and Hughes, G. L. (2015). The microbiome modulates arbovirus transmission in mosquitoes. Curr. Opin. Virol. 15, 97–102.
Hoffmann, A. A., Ross, P. A., and Rašić, G. (2015). Wolbachia strains for disease control: Ecological and evolutionary considerations. Evol. Appl. 8, 751–768. doi: 10.1111/eva.12286
Huang, E. Y. Y., Wong, A. Y. P., Lee, I. H. T., Qu, Z., Yip, H. Y., Leung, C. W., et al. (2020). Infection patterns of dengue, Zika and endosymbiont Wolbachia in the mosquito Aedes albopictus in Hong Kong. Parasit. Vectors 13:361. doi: 10.1186/s13071-020-04231-x
Ibáñez-Justicia, A., Smitz, N., den Hartog, W., van de Vossenberg, B., De Wolf, K., Deblauwe, I., et al. (2020). Detection of exotic mosquito species (Diptera: Culicidae) at international airports in Europe. Int. J. Environ. Res. Public Health 17:3450.
Johnson, K. N. (2015). The impact of wolbachia on virus infection in mosquitoes. Viruses 7, 5705–5717. doi: 10.3390/v7112903
Juma, E. O., Allan, B. F., Kim, C.-H., Stone, C., Dunlap, C., and Muturi, E. J. (2020). Effect of life stage and pesticide exposure on the gut microbiota of Aedes albopictus and Culex pipiens L. Sci. Rep. 10:9489. doi: 10.1038/s41598-020-66452-5
Kambris, Z., Blagborough, A. M., Pinto, S. B., Blagrove, M. S. C., Godfray, H. C. J., Sinden, R. E., et al. (2010). Wolbachia stimulates immune gene expression and inhibits plasmodium development in anopheles gambiae. PLoS Pathog. 6:e1001143. doi: 10.1371/journal.ppat.1001143
Laporta, G. Z., Potter, A. M., Oliveira, J. F., Bourke, B. P., Pecor, D. B., and Linton, Y.-M. (2023). Global distribution of Aedes aegypti and Aedes albopictus in a Climate change scenario of regional rivalry. Insects 14:49. doi: 10.3390/insects14010049
Lefèvre, T., Vantaux, A., Dabiré, K. R., Mouline, K., and Cohuet, A. (2013). Non-genetic determinants of mosquito competence for malaria parasites. PLoS Pathog. 9:e1003365. doi: 10.1371/journal.ppat.1003365
Luis, P., Vallon, L., Tran, F.-H., Hugoni, M., Tran-Van, V., Mavingui, P., et al. (2019). Aedes albopictus mosquitoes host a locally structured mycobiota with evidence of reduced fungal diversity in invasive populations. Fungal Ecol. 39, 257–266.
Mancini, M. V., Herd, C. S., Ant, T. H., Murdochy, S. M., and Sinkins, S. P. (2020). Wolbachia strain wAu efficiently blocks arbovirus transmission in Aedes albopictus. PLoS. Negl. Trop. Dis. 14:e0007926. doi: 10.1371/journal.pntd.0007926
Martínez-de la Puente, J., Gutiérrez-López, R., and Figuerola, J. (2018). Do avian malaria parasites reduce vector longevity? Curr. Opin. Insect Sci. 28, 113–117.
McLean, B. J., Dainty, K. R., Flores, H. A., and O’NEILL, S. L. (2019). Differential suppression of persistent insect specific viruses in trans-infected wMel and wMelPop-CLA Aedes-derived mosquito lines. Virology 527, 141–145. doi: 10.1016/j.virol.2018.11.012
Minard, G., Tran, F.-H., Tran-Van, V., Goubert, C., Bellet, C., Lambert, G., et al. (2015). French invasive Asian tiger mosquito populations harbor reduced bacterial microbiota and genetic diversity compared to Vietnamese autochthonous relatives. Front. Microbiol. 6:970. doi: 10.3389/fmicb.2015.00970
Monteiro, V., Navegantes-Lima, K., de Lemos, A., da Silva, G., de Souza Gomes, R., Reis, J., et al. (2019). Aedes–chikungunya virus interaction: Key role of vector midguts microbiota and its saliva in the host infection. Front. Microbiol. 10:492. doi: 10.3389/fmicb.2019.00492
Morand, S. (2020). Emerging diseases, livestock expansion and biodiversity loss are positively related at global scale. Biol. Conserv. 248:108707. doi: 10.1016/j.biocon.2020.108707
Mousson, L., Zouache, K., Arias-Goeta, C., Raquin, V., Mavingui, P., and Failloux, A. B. (2012). The native Wolbachia symbionts limit transmission of dengue virus in Aedes albopictus. PLoS. Negl. Trop. Dis. 6:e1989. doi: 10.1371/journal.pntd.0001989
Muturi, E. J., Bara, J. J., Rooney, A. P., and Hansen, A. K. (2016). Midgut fungal and bacterial microbiota of Aedes triseriatus and Aedes japonicus shift in response to La Crosse virus infection. Mol. Ecol. 25, 4075–4090. doi: 10.1111/mec.13741
Muturi, E. J., Ramirez, J. L., Rooney, A. P., and Kim, C.-H. (2017). Comparative analysis of gut microbiota of mosquito communities in central Illinois. PLoS. Negl. Trop. Dis. 11:e0005377. doi: 10.1371/journal.pntd.0005377
Onyango, M. G., Lange, R., Bialosuknia, S., Payne, A., Mathias, N., Kuo, L., et al. (2021). Zika virus and temperature modulate Elizabethkingia anophelis in Aedes albopictus. Parasit. Vectors 14:573. doi: 10.1186/s13071-021-05069-7
Pereira, T. N., Carvalho, F. D., Rugani, J. N., de Carvalho, V., Jarusevicius, J., Souza-Neto, J. A., et al. (2021). Mayaro virus: The potential role of microbiota and wolbachia. Pathogens 10:525. doi: 10.3390/pathogens10050525
Powell, J. R., and Tabachnick, W. J. (2013). History of domestication and spread of Aedes aegypti–a review. Mem. Inst. Oswaldo Cruz 108(Suppl. 1), 11–17. doi: 10.1590/0074-0276130395
Ramirez, J. L., Schumacher, M. K., Ower, G., Palmquist, D. E., and Juliano, S. A. (2021). Impacts of fungal entomopathogens on survival and immune responses of Aedes albopictus and Culex pipiens mosquitoes in the context of native Wolbachia infections. PLoS. Negl. Trop. Dis. 15:e0009984. doi: 10.1371/journal.pntd.0009984
Ramirez, J. L., Souza-Neto, J. A., Torres Cosme, R., Rovira, J., Ortiz, A., Pascale, J. M., et al. (2012). Reciprocal tripartite interactions between the Aedes aegypti midgut microbiota, innate immune system and dengue virus influences vector competence. PLoS. Negl. Trop. Dis. 6:e1561. doi: 10.1371/journal.pntd.0001561
Raquin, V., Valiente, M., Saucereau, Y., Tran, F.-H., Potier, P., and Mavingui, P. (2015). Native Wolbachia from Aedes albopictus blocks chikungunya virus infection in cellulo. PLoS One 10:e0125066. doi: 10.1371/journal.pone.0125066
Reiter, P., and Sprenger, D. (1987). The used tire trade: A mechanism for the worldwide dispersal of container breeding mosquitoes. J. Am. Mosq. Control Assoc. 3, 494–501.
Ross, P. A., Turelli, M., and Hoffmann, A. A. (2019). Evolutionary ecology of wolbachia releases for disease control. Annu. Rev. Genet. 53, 93–116. doi: 10.1146/annurev-genet-112618-043609
Rosso, F., Tagliapietra, V., Albanese, D., Pindo, M., Baldacchino, F., Arnoldi, D., et al. (2018). Reduced diversity of gut microbiota in two Aedes mosquitoes species in areas of recent invasion. Sci. Rep. 8:16091. doi: 10.1038/s41598-018-34640-z
Ruiz-Lopez, M. J. (2020). Mosquito behavior and vertebrate microbiota interaction: Implications for pathogen transmission. Front. Microbiol. 11:573371. doi: 10.3389/fmicb.2020.573371
Saab, S. A., Dohna, H. Z., Nilsson, L. K. J., Onorati, P., Nakhleh, J., Terenius, O., et al. (2020). The environment and species affect gut bacteria composition in laboratory co-cultured Anopheles gambiae and Aedes albopictus mosquitoes. Sci. Rep. 10:3352. doi: 10.1038/s41598-020-60075-6
Sasaki, T., Moi, M. L., Saito, K., Isawa, H., Takasaki, T., and Sawabe, K. (2022). Aedes albopictus strain and dengue virus serotype in the dengue fever outbreaks in japan: Implications of Wolbachia infection. Jpn. J. Infect. Dis. 75, 140–143. doi: 10.7883/yoken.JJID.2021.376
Schaffner, F., Medlock, J. M., and Bortel, W. V. (2013). Public health significance of invasive mosquitoes in Europe. Clin. Microbiol. Infect. 19, 685–692.
Schultz, M. J., Isern, S., Michael, S. F., Corley, R. B., Connor, J. H., and Frydman, H. M. (2017). Variable inhibition of zika virus replication by different Wolbachia strains in mosquito cell cultures. J. Virol. 91, e00339-17. doi: 10.1128/JVI.00339-17
Schultz, M. J., Tan, A. L., Gray, C. N., Isern, S., Michael, S. F., Frydman, H. M., et al. (2018). Wolbachia wStri blocks zika virus growth at two independent stages of viral replication. mBio 9, e00738-18. doi: 10.1128/mBio.00738-18
Seabourn, P., Spafford, H., Yoneishi, N., and Medeiros, M. (2020). The Aedes albopictus (Diptera: Culicidae) microbiome varies spatially and with Ascogregarine infection. PLoS. Negl. Trop. Dis. 14:e0008615. doi: 10.1371/journal.pntd.0008615
Sicard, M., Bonneau, M., and Weill, M. (2019). Wolbachia prevalence, diversity, and ability to induce cytoplasmic incompatibility in mosquitoes. Curr. Opin. Insect Sci. 34, 12–20. doi: 10.1016/j.cois.2019.02.005
Souza-Neto, J. A., Powell, J. R., and Bonizzoni, M. (2019). Aedes aegypti vector competence studies: A review. Infect. Genet. Evol. 67, 191–209. doi: 10.1016/j.meegid.2018.11.009
Stewart Merrill, T., and Johnson, P. T. J. (2020). Towards a mechanistic understanding of competence: A missing link in diversity–disease research. Parasitology 147, 1159–1170. doi: 10.1017/S0031182020000943
Succo, T., Leparc-Goffart, I., Ferré, J. B., Roiz, D., Broche, B., Maquart, M., et al. (2016). Autochthonous dengue outbreak in Nîmes, South of France, July to September 2015. Euro Surveill. 21:30240. doi: 10.2807/1560-7917.ES.2016.21.21.30240
Tawidian, P., Coon, K. L., Jumpponen, A., Cohnstaedt, L. W., and Michel, K. (2021). Host-environment interplay shapes fungal diversity in mosquitoes. mSphere 6:e0064621. doi: 10.1128/mSphere.00646-21
Teramoto, T., Huang, X., Armbruster, P. A., and Padmanabhan, R. (2019). Infection of Aedes albopictus mosquito C6/36 cells with the wmelpop strain of Wolbachia modulates dengue virus-induced host cellular transcripts and induces critical sequence alterations in the dengue viral genome. J. Virol. 93, e00581-19. doi: 10.1128/JVI.00581-19
Thongsripong, P., Chandler, J. A., Green, A. B., Kittayapong, P., Wilcox, B. A., Kapan, D. D., et al. (2018). Mosquito vector-associated microbiota: Metabarcoding bacteria and eukaryotic symbionts across habitat types in Thailand endemic for dengue and other arthropod-borne diseases. Ecol. Evol. 8, 1352–1368. doi: 10.1002/ece3.3676
Tolle, M. A. (2009). Mosquito-borne Diseases. Curr. Probl. Pediatr. Adolesc. Health Care 39, 97–140.
Tomasello, D., and Schlagenhauf, P. (2013). Chikungunya and dengue autochthonous cases in Europe, 2007–2012. Travel Med. Infect. Dis. 11, 274–284. doi: 10.1016/j.tmaid.2013.07.006
Tortosa, P., Courtiol, A., Moutailler, S., Failloux, A. B., and Weill, M. (2008). Chikungunya-Wolbachia interplay in Aedes albopictus. Insect. Mol. Biol. 17, 677–684. doi: 10.1111/j.1365-2583.2008.00842.x
Venturi, G., Di Luca, M., Fortuna, C., Remoli, M. E., Riccardo, F., Severini, F., et al. (2017). Detection of a chikungunya outbreak in Central Italy, August to September 2017. Euro Surveill. 22, 17–00646. doi: 10.2807/1560-7917.ES.2017.22.39.17-00646
WHO (2020). Evaluation of genetically modified mosquitoes for the control of vector-borne diseases: Position statement, October 2020. Geneva: WHO.
Yee, D. A., Dean, B., Reyes-Torres, L. J., Fijman, N. S., Scavo, N. A., Nelsen, J., et al. (2022). Robust network stability of mosquitoes and human pathogens of medical importance. Parasit. Vectors 15:216. doi: 10.1186/s13071-022-05333-4
Zhao, T., Li, B. Q., Gao, H. T., Xing, D., Li, M. J., Dang, Y. Q., et al. (2022). Metagenome sequencing reveals the microbiome of Aedes albopictus and its possible relationship with dengue virus susceptibility. Front. Microbiol. 13:891151. doi: 10.3389/fmicb.2022.891151
Keywords: Aedes, bacteria, invasive species, metabarcoding, microbiota, vectors, mosquito-borne pathogens, Wolbachia
Citation: Garrido M, Veiga J, Garrigós M and Martínez-de la Puente J (2023) The interplay between vector microbial community and pathogen transmission on the invasive Asian tiger mosquito, Aedes albopictus: current knowledge and future directions. Front. Microbiol. 14:1208633. doi: 10.3389/fmicb.2023.1208633
Received: 19 April 2023; Accepted: 10 July 2023;
Published: 27 July 2023.
Edited by:
Claire Valiente Moro, Université Claude Bernard Lyon 1, FranceReviewed by:
Benjamin Cull, University of Minnesota Twin Cities, United StatesCopyright © 2023 Garrido, Veiga, Garrigós and Martínez-de la Puente. This is an open-access article distributed under the terms of the Creative Commons Attribution License (CC BY). The use, distribution or reproduction in other forums is permitted, provided the original author(s) and the copyright owner(s) are credited and that the original publication in this journal is cited, in accordance with accepted academic practice. No use, distribution or reproduction is permitted which does not comply with these terms.
*Correspondence: Mario Garrido, bS5nYXJyaWRvQHVnci5lcw==
Disclaimer: All claims expressed in this article are solely those of the authors and do not necessarily represent those of their affiliated organizations, or those of the publisher, the editors and the reviewers. Any product that may be evaluated in this article or claim that may be made by its manufacturer is not guaranteed or endorsed by the publisher.
Research integrity at Frontiers
Learn more about the work of our research integrity team to safeguard the quality of each article we publish.