- Centre for Bacterial Cell Biology, Biosciences Institute, Medical School, Newcastle University, Newcastle upon Tyne, United Kingdom
Bacterial cells are surrounded by a peptidoglycan (PG) wall, which is a crucial target for antibiotics. It is well known that treatment with cell wall-active antibiotics occasionally converts bacteria to a non-walled “L-form” state that requires the loss of cell wall integrity. L-forms may have an important role in antibiotic resistance and recurrent infection. Recent work has revealed that inhibition of de novo PG precursor synthesis efficiently induces the L-form conversion in a wide range of bacteria, but the molecular mechanisms remain poorly understood. Growth of walled bacteria requires the orderly expansion of the PG layer, which involves the concerted action not just of synthases but also degradative enzymes called autolysins. Most rod-shaped bacteria have two complementary systems for PG insertion, the Rod and aPBP systems. Bacillus subtilis has two major autolysins, called LytE and CwlO, which are thought to have partially redundant functions. We have dissected the functions of autolysins, relative to the Rod and aPBP systems, during the switch to L-form state. Our results suggest that when de novo PG precursor synthesis is inhibited, residual PG synthesis occurs specifically via the aPBP pathway, and that this is required for continued autolytic activity by LytE/CwlO, resulting in cell bulging and efficient L-form emergence. The failure of L-form generation in cells lacking aPBPs was rescued by enhancing the Rod system and in this case, emergence specifically required LytE but was not associated with cell bulging. Our results suggest that two distinct pathways of L-form emergence exist depending on whether PG synthesis is being supported by the aPBP or RodA PG synthases. This work provides new insights into mechanisms of L-form generation, and specialisation in the roles of essential autolysins in relation to the recently recognised dual PG synthetic systems of bacteria.
Introduction
The peptidoglycan (PG) cell wall is an essential structure for most bacteria and the target for many of our most effective antibiotics. The wall is composed of glycan strands cross-linked by short peptides, forming a huge contiguous meshwork that covers the whole surface of the cell. Expansion of the cell during growth requires the insertion of new PG, which is achieved by the action of glycosyltransferase (GTase) enzymes, to extend the glycan chains, and transpeptidase (TPase) enzymes, which introduce cross-links (Typas et al., 2012; Egan et al., 2020). Recent work has established that most bacteria have two complementary PG insertion systems (Meeske et al., 2016; Emami et al., 2017; Sjodt et al., 2018; Dion et al., 2019; Garner, 2021). The Rod system involves GTases called RodA and FtsW, which work in conjunction with cognate monofunctional TPases called class B penicillin-binding proteins (bPBPs) (Reichmann et al., 2019; Taguchi et al., 2019; Sjodt et al., 2020). They are regulated spatially and temporally to achieve orderly cell extension and then division, governed by cytoskeletal proteins MreB and FtsZ, respectively (Typas et al., 2012; Egan et al., 2020). The aPBP system is based on bifunctional class A PBPs (aPBPs) that have both GTase and TPase activity. In Bacillus subtilis the aPBP system seems to insert new PG in a dispersed manner leading, in the absence of the Rod system, to growth in a spherical form. However, the precise function of the aPBP system is not clear, and all four aPBPs (ponA, pbpD, pbpF and pbpG) can actually be deleted with only mild effects on cell growth and morphology (McPherson and Popham, 2003; Emami et al., 2017; Dion et al., 2019).
Cell wall growth invariably relies also on the action of PG hydrolases, sometimes called autolysins, which break bonds in the PG meshwork. These enzymes are extremely abundant and redundant, B. subtilis has 42 putative autolytic enzymes (Wilson and Garner, 2021). However, their specific functions are poorly characterised. Mutations in several of the genes have mild effects on cell separation after division, and mutations in multiple genes tend to have additive effects, suggesting that they have overlapping functions (Fukushima et al., 2006; Wilson and Garner, 2021). For cell elongation, it has been established that two genes lytE and cwlO, encoding D,L-endopeptidase autolysins, have important and overlapping functions: single deletions have no significant effect on cell growth but the double mutant is inviable, with an apparent complete arrest in cell elongation (Bisicchia et al., 2007; Hashimoto et al., 2012; Meisner et al., 2013; Salzberg et al., 2013; Wilson and Garner, 2021). It has long been assumed that the main role of autolytic enzymes during cell elongation is to break bonds in PG, so as to enable the insertion of new wall material. However, their activities also clearly need to be tightly regulated because autolysins can also be the source of catastrophic cell lysis (Höltje, 1998). Recent studies have started to explore the regulation of autolysins, and the relationships between autolysins and the Rod and aPBP synthetic systems (Dobihal et al., 2019; Patel et al., 2020), although much remains to be understood.
Despite the critical importance of the PG wall for the cell viability, under osmoprotective conditions many bacteria have the ability to switch into a wall-deficient state called the L-form (Klieneberger, 1935), which is completely resistant to antibiotics that work on cell-wall-active antibiotics, such as β-lactams. Historically L-forms have mainly been identified as antibiotic resistant organisms in samples from humans, and have been associated with a wide range of infectious diseases (Domingue and Woody, 1997; Allan et al., 2009; Errington et al., 2016). Our recent findings have provided mechanistic support for the view that L-forms may be involved in chronic or recurrent infections (Kawai et al., 2018; Mickiewicz et al., 2019). However, despite their critical importance for understanding antibiotic resistance and pathogenesis, molecular mechanisms underlying the generation of L-forms are still poorly understood.
We have previously shown that, provided B. subtilis cells have a mutation that downgrades the respiratory chain pathway such as ispA (which works to avoid generation of toxic reactive oxygen species) and are on an isotonic medium, they can avoid killing by antibiotics that inhibit synthesis of the PG precursor, lipid II, by switching into the wall-deficient L-form state (Mercier et al., 2013; Kawai et al., 2015, 2019). The antibiotic D-cycloserine (DCS), which inhibits D-alanine-D-alanine ligase in the lipid II pathway, efficiently induces such a switch. L-form production induced by DCS occurs via a gradual bulging of the walled cell before the L-form emerges (Kawai et al., 2018). We call this critical event in the formation of L-forms “escape.” Escape is dependent on the aPBP PG synthases, as well as the autolytic enzymes, LytE and CwlO (Kawai et al., 2018). Thus, deletion of all four aPBPs (encoded by ponA, pbpD, pbpF and pbpG genes) or the combined absence of LytE and CwlO inhibits L-form escape (Kawai et al., 2018). In contrast, escape is not blocked by mutations affecting RodA. The L-form escape system therefore provides a novel platform for studying the connections between PG synthetic and hydrolytic activities and between the RodA and aPBP systems. Here, we have exploited this system to obtain important new insights into these connections. The results shed light on specialisation of the functions of CwlO and LytE and of the RodA vs. aPBP systems during L-form escape in B. subtilis. They also enhance our understanding of factors that can affect the ability of cells to undertake the L-form switch that results in the evasion of killing by important antibiotics.
Materials and methods
Strains and growth conditions
Nutrient agar and broth (Oxoid) were used for B. subtilis growth. All strains used in this work are listed in Table 1. DNA manipulations and transformations were carried out using standard methods. For selections of transformants, cells were plated on NA plates supplemented with 1 μg/mL erythromycin, 5 μg/mL chlroramphenicol, 60 μg/mL spectinomycin, 1 μg/mL phleomycin, 2.5 μg/mL kanamycin and/or 10 μg/mL tetracycline. IPTG, xylose or Mg2+ was supplemented, as appropriate. ΔmreB and Δmbl mutants were selected on NA containing added 10 mM MgSO4. ΔrodA mutant was selected on isotonic NA plates, composed of 2x magnesium-sucrose-maleic acid (MSM) pH7 (40 mM magnesium chloride, 1 M sucrose, and 40 mM maleic acid) mixed 1:1 with 2x NA. The concentration of kanamycin or tetracycline was increased to 10 μg/mL or 30 μg/mL in the presence of added Mg2+. Since transformation in Δ4 mutant cells was less effective, an temperature sensitive unstable pLOSS-Pspac-ponA plasmid was used to improve the transformation efficiency by providing ponA expression with IPTG in Δ4 mutant backgrounds (Claessen et al., 2008; Emami et al., 2017). Antibiotics were added to media for growth of strains carrying Ωerm-∆cwlO, Ωerm-Pspac-ispA, pLOSS-Pspac-ponA, Ωkan-Pspac-rodA or Ωkan-Pspac-ftsW at the following concentrations: 1 μg/mL erythromycin, 60 μg/mL spectinomycin or 2.5 or 10 μg/mL kanamycin.
L-form escape and growth
L-form growth was induced on osmoprotective plates (NA containing MSM) in the presence of 200 μg/mL D-cycloserine (DCS) or genetic inhibition of PG precursor synthesis at 30°C. 1 μg/mL FtsZ inhibitor, 8 J (Adams et al., 2011), was used in L-form culture to prevent the growth of walled cells when required. IPTG or xylose was supplemented, as appropriate. For L-form escape experiments in liquid media, walled cells were pre-cultured in NB at 37°C. The exponentially growing cells (OD600 0.2–0.3) were diluted in the fresh osmoprotective medium (NB containing MSM) with 200 μg/mL D-cycloserine (DCS) or genetic inhibition of PG precursor synthesis, and incubated without shaking at 30°C.
Microscopic imaging
For snapshot live cell imaging, walled cells were mounted on microscope slides covered with a thin film of 1.2% agarose in water, essentially as described previously (Glaser et al., 1997). L-form cultures were mounted on microscope slides covered with MSM. All microscopy experiments were conducted using a Nikon Ti microsope equipped with a Nikon CFI Plan Apo DM Lambda x100 oil objective and a Photometrics Prime camera, using MetaMorph software (version 7.7, Molecular Devices). Images were analysed and processed using FIJI.1
Results
L-form escape Is independent of various components of The rod system
We previously found that L-form escape following the inhibition of de novo PG precursor synthesis requires aPBPs, but not RodA (Figure 1A; Kawai et al., 2018). Figure 1B shows growth and morphology of otherwise wild type B. subtilis in the presence of an ispA* mutation (xseB::Tn-kan) that prevents IspA synthesis (Mercier et al., 2013). We used ispA mutations in all of the L-form experiments in this work so as to enable stable L-form growth by reducing the generation of toxic ROS (Kawai et al., 2015). The cells were cultured on isotonic nutrient agar (NA) plates containing osmoprotective sucrose and extra Mg2+ with (lower panels) or without (upper panels) D-cycloserine (DCS), which inhibits the PG precursor pathway (Figure 1A). Under these conditions, deletion of all four aPBPs (“Δ4 mutant”) prevented the switch to the L-form state (Figure 1C). In contrast, L-form growth occurred significantly in the absence of RodA (Figure 1D, lower panels), whereas it resulted in a spherical mode of growth in the walled state (upper panels) (RodA is normally essential in the walled state but growth can be rescued by the addition of osmolytes) (e.g., sucrose and Mg2+ to standard culture media) (Kawai et al., 2011).
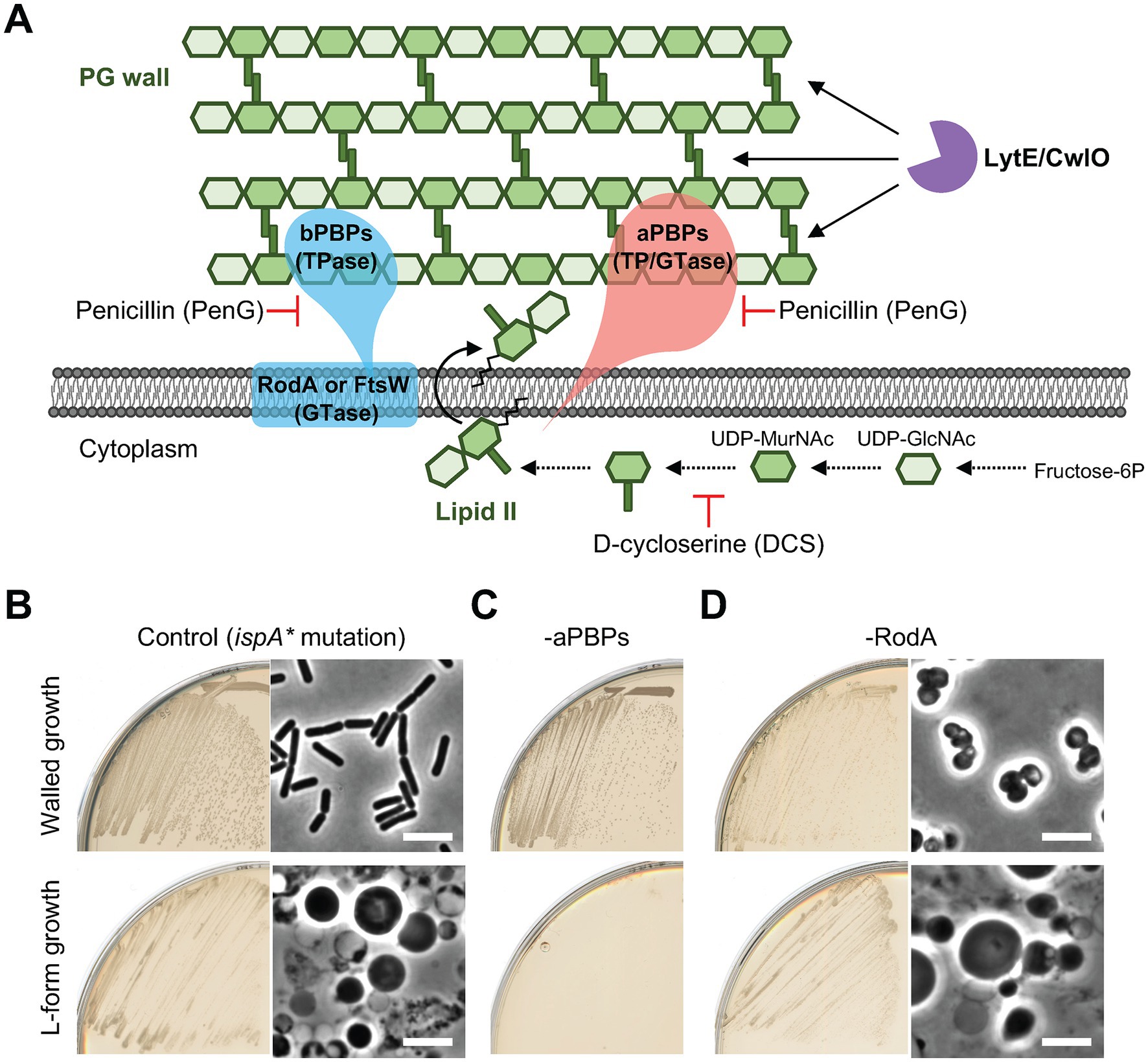
Figure 1. L-form generation requires aPBPs but not RodA (A) Schematic representation of cell wall expansion by dual PG synthetic systems and major autolysins during growth of Bacillus subtilis. PG precursor, lipid II, can be incorporated into the existing PG wall by the Rod and/or aPBP PG synthetic systems. The action of PG hydrolases (LytE and CwlO) is also essential for the PG growth during cell elongation. (B-D) Walled cell and L-form growth of strains YK1395 (ispA*), YK2344 (Δ4 ispA*) and YK2483 (ΔrodA Pspac-ispA). The strains were streaked on isotonic NA plates with or without 200 μg/mL DCS and incubated at 30°C for 1 day (walled growth without DCS) or 2–3 days (L-form growth with DCS). Phase contrast micrographs were taken from colonies on the plates. Scale bars represent 5 μm.
We tested for the effects of repression of other morphogenic proteins required for cell elongation or division (brought about by repression of these genes under IPTG or xylose control). Repression of the division specific PG synthase FtsW did not affect escape (Figure 2, FtsW lower left). L-form escape also occurred efficiently in the absence of the division complex organiser FtsZ, or of the elongation organiser MreB, whether it was eliminated along with its “partners” (MreC and MreD) or its isoforms (Mbl and MreBH) (Figure 2, lower panels). In contrast, the combined absence of LytE and CwlO inhibited L-form growth (Figure 2, lower right), as previously shown (Kawai et al., 2018). All of these mutations abolished growth under selection for the walled state (without added sucrose and Mg2+) (Figure 2, OFF, middle panels), as expected. Thus, L-form escape seems to depend specifically on the aPBP pathway and the autolytic activity of either LytE or CwlO.
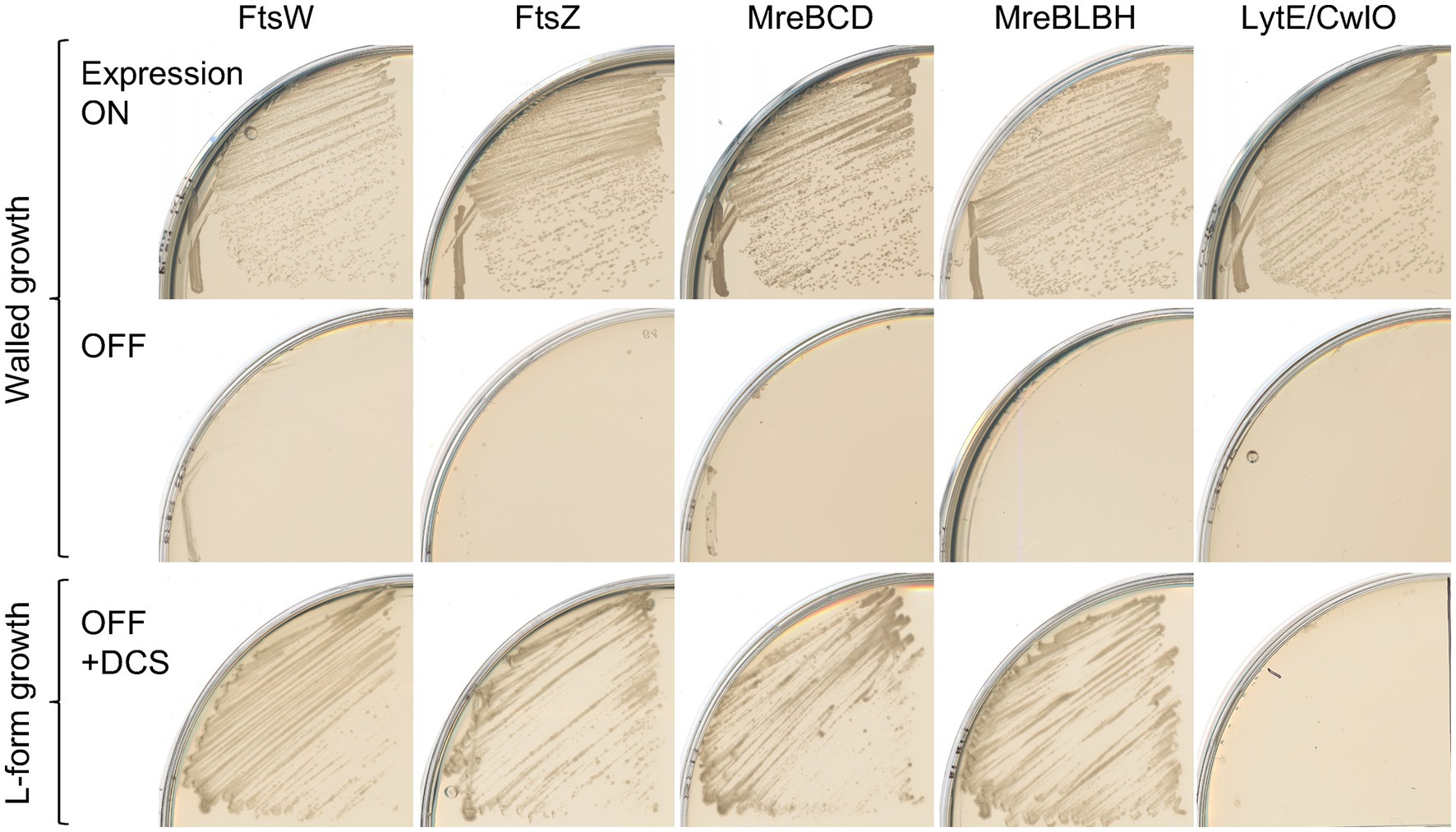
Figure 2. L-form generation does not require the Rod system Walled cell and L-form growth of strains YK2574 (Pspac-ftsW ispA*; FtsW), YK1859 (Pspac-ftsZ ispA*; FtsZ), YK1876 (ΔmreB ΔmreC ΔmreD amyE::Pxyl-mreB-mreC-mreD ΔispA; MreBCD) and YK1874 (ΔmreB Δmbl ΔmreBH amyE::PspacHY-mbl ispA*; MreBLBH) and YK2524 (ΔlytE Pspac-cwlO ispA*; LytE/CwlO) on normal NA plates with or without 0.5 mM IPTG (or 0.5% xylose for YK1876) at 30°C for 1 day (Walled growth), or on isotonic NA plates (containing added sucrose and Mg2+) with 200 μg/mL DCS at 30°C for 2–3 days (L-form growth).
Dispersed PG synthesis by the aPBP pathway during PG precursor depletion
We have previously shown that L-form escape induced by DCS occurs via a gradual bulging of the walled cell before the L-form emerges (Figure 3A; Kawai et al., 2018). Our previous work has also shown that the aPBP system and the autolytic activity of either LytE or CwlO are needed for the bulging and L-form escape in the presence of DCS (Figure 3B). The bulging can also be induced by repressing genes acting in the PG precursor pathway, for example, the murE operon (Figure 3B, MurE OFF) (Kawai et al., 2018). GlmM protein catalyses a key step in the synthesis of uridine 5′-diphospho-N-acetylglucosamine (UDP-GlcNAc), which is an essential intermediate in the lipid II pathway (Figure 1A). The repression of GlmM again induced bulging (Figure 3B, GlmM OFF). Crucially, under each of these conditions, bulging of the walled cells was blocked by deletion of the aPBP genes (Figure 3B, lower panels). Thus, it seems that under conditions of limiting PG precursors, the aPBP pathway takes precedence over the Rod pathway, leading to dispersed PG synthesis and bulging.
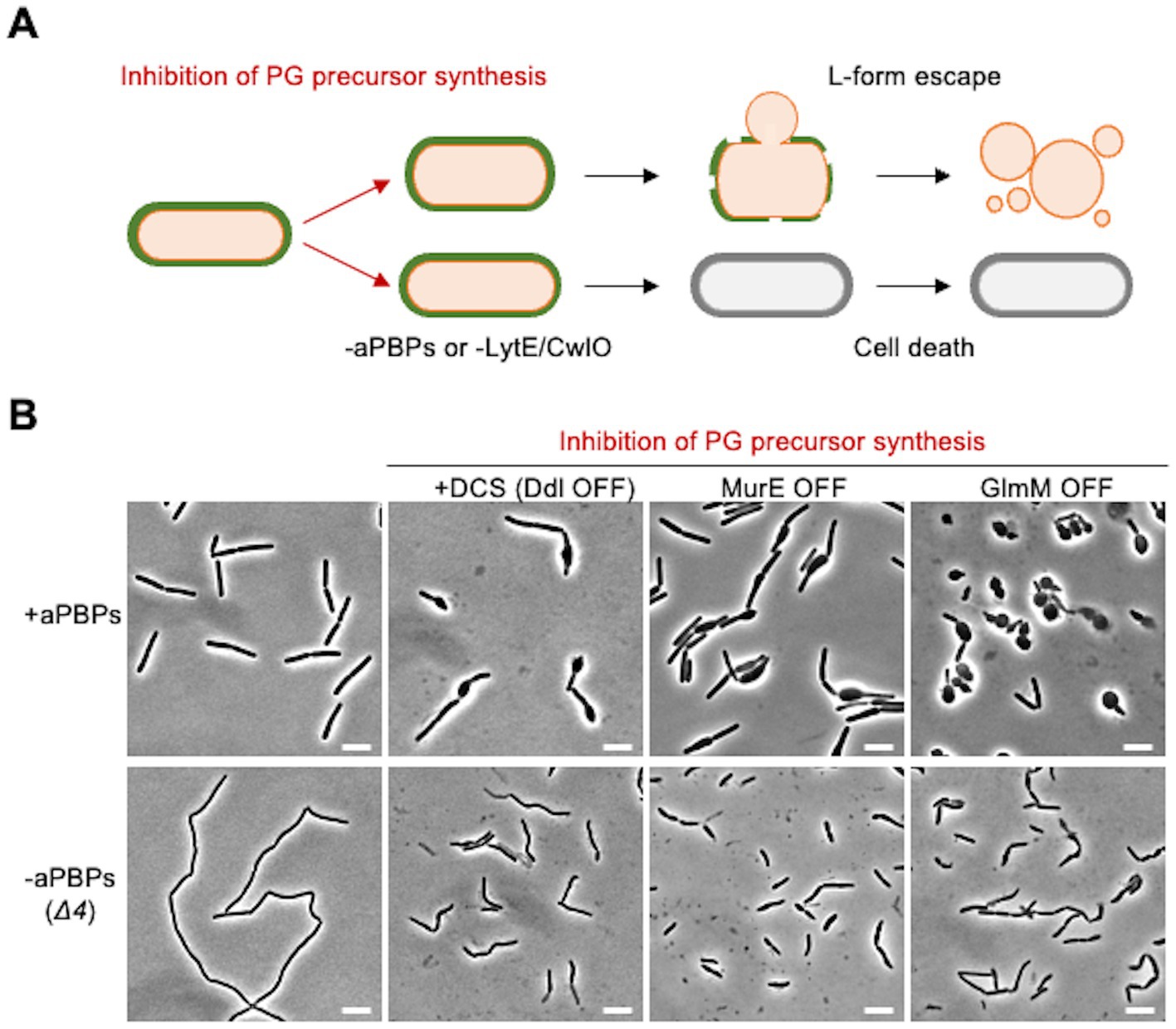
Figure 3. Crucial role for the aPBP pathway in bulging when PG precursors are limiting (A) Schematic representation of L-form escape during PG precursor depletion in B. subtilis. Emergence of L-form cells induced by inhibition of PG precursor synthesis occurs in association with bulging of walled cells. Inactivation of all four aPBPs (Δ4) or the combined absence of LytE and CwlO inhibits the bulging formation and L-form emergence. (B) Phase contrast micrographs of strains YK1395 (ispA*), YK2344 (Δ4 ispA*), LR2 (Pxyl-murE ispA*), YK2342 (Δ4 Pxyl-murE ispA*), YK1559 (Pspac-glmM) and YK2586 (Δ4 Pspac-glmM). The strains YK1395 and YK2344 were cultured in isotonic liquid nutrient broth (NB) at 37°C. The exponentially growing cells were diluted in the fresh isotonic NB with or without 200 μg/mL DCS, and the phase contrast micrographs were captured by 2–3 h after the dilutions. The strains LR2, YK2342, YK1559 and YK2586 were cultured in isotonic NB with or without 0.2% xylose (for murE expression) or 0.1 mM IPTG (for glmM expression) at 37°C. The exponentially growing cells were diluted in the fresh isotonic NB (without xylose and IPTG) and the phase contrast micrographs were captured by 2–3 h after the dilutions (MurE OFF and GlmM OFF). Scale bars represent 5 μm.
RodA overproduction compensates for the loss of aPBPs in L-form generation
Previous reports showed that upregulation of rodA can ameliorate growth deficiencies in walled cells caused by the inactivation of aPBPs under certain conditions (e.g., moenomycin treatment, which blocks aPBP GTase activity) (Meeske et al., 2016; Emami et al., 2017). Consistent with this, overproduction of RodA, using a second copy of the rodA gene under xylose control at an ectopic locus (amyE::Pxyl-rodA), improved the slow growth phenotype that is typical of Δ4 (aPBP) mutants (Figure 4A). We wondered if rodA upregulation might also rescue the defect in L-form escape of a Δ4 mutant. To test this we built a derivative of the Δ4 (ispA repressible) strain containing the extra copy of rodA (Pxyl-rodA). We grew this strain with and without xylose and then streaked the cells on L-form selective plates containing DCS. Cells grown with overproduced RodA gave rise to an abundance of L-form colonies (Figure 4B, middle), whereas no growth occurred for the uninduced cells (Figure 4B, left). Thus, RodA overproduction works not only to compensate for the loss of aPBPs in growth of walled cells but also for L-form escape.
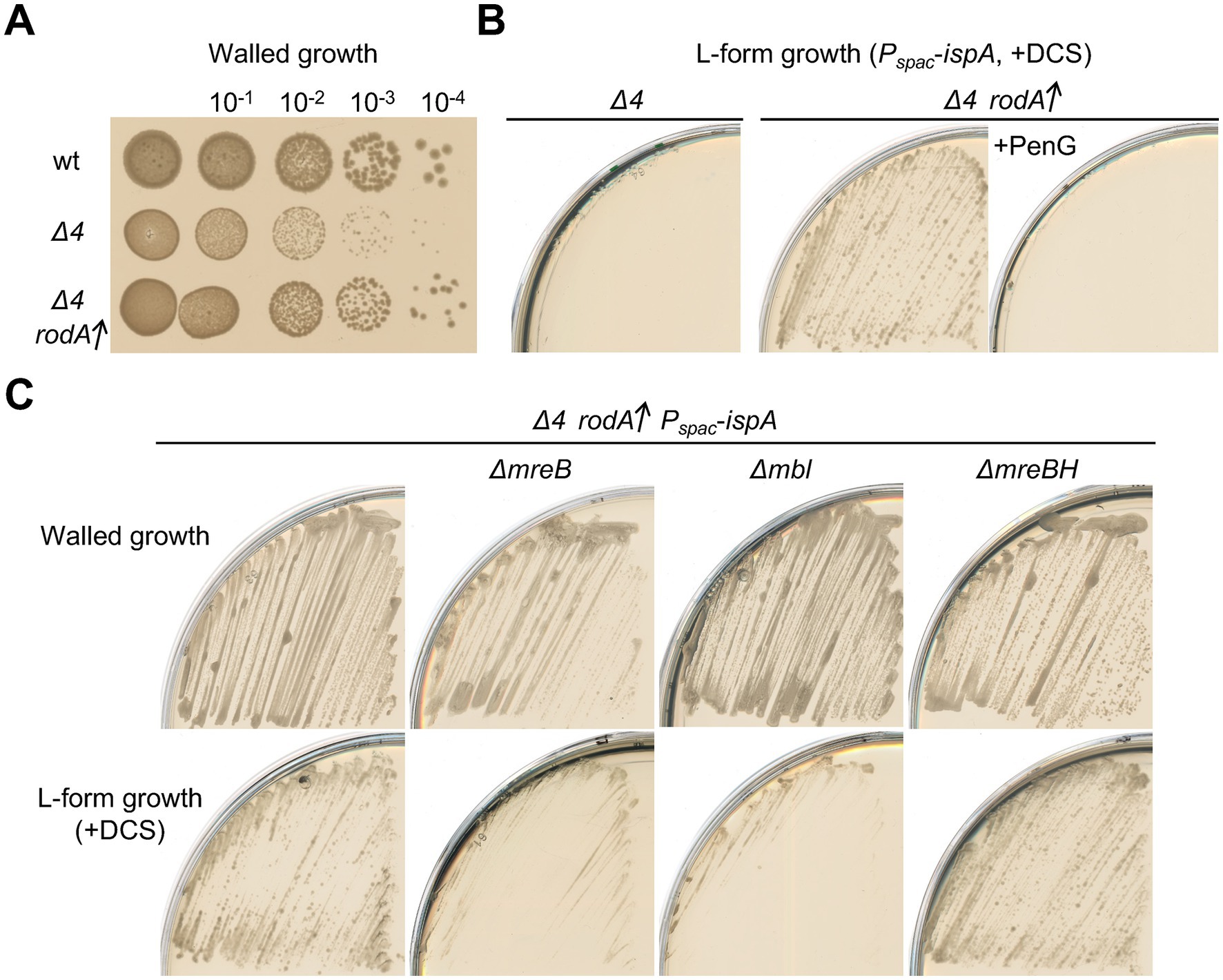
Figure 4. Induction of L-form generation by the Rod pathway (A) Exponentially growing cells of strains 168CA (wild-type), YK2239 (Δ4) and YK2283 (Δ4 amyE::Pxyl-rodA) in NB were diluted (tenfold series) and 6 μL spots were placed on NA plates with 0.5% xylose, and incubated at 30°C for 1 day. (B) L-form growth of strain YK2515 (Δ4 amyE::Pxyl-rodA Pspac-ispA). The strain YK2515 was streaked on isotonic NA plates with or without 1% xylose (for rodA overexpression) in the presence of 200 μg/mL DCS, and incubated at 30°C for 2–3 days. 100 μg/mL PenG was also added to the medium as indicated (right panel). (C) Walled cell and L-form growth of strains YK2515 (Δ4 amyE::Pxyl-rodA Pspac-ispA), YK2596 (ΔmreB Δ4 amyE::Pxyl-rodA Pspac-ispA), YK2535 (Δmbl Δ4 amyE::Pxyl-rodA Pspac-ispA) and YK2538 (ΔmreBH Δ4 amyE::Pxyl-rodA Pspac-ispA). The strains were streaked on isotonic NA plates containing 1% xylose with or without 200 μg/mL DCS, and incubated at 30°C for 2–3 days (L-form growth with DCS) or 1 day (walled cell growth without DCS).
L-form generation by the rod pathway in the absence of aPBPs
PG synthesis by the RodA GTase depends on the TPase activity of its cognate bPBPs, PbpA and/or PbpH (Wei et al., 2003; Dion et al., 2019; Sjodt et al., 2020). The TPase activity of bPBPs, like that of aPBPs, is targeted by β-lactam antibiotics (Figure 1A; Lovering et al., 2012). If the rescue of L-form growth in the absence of aPBPs by RodA overproduction worked by compensating for the loss of PG synthesis, the effect should be blocked by the broad targeting β-lactam penicillin G. This was indeed the case (Figure 4B, right). Thus, escape by this pathway requires not only increased levels of the RodA GTase but also at least one of the cognate bPBPs, and probably other components of the Rod elongation system.
We introduced mutations of the elongation organiser MreB and its isoforms (ΔmreB, Δmbl and ΔmreBH) separately into a Δ4 strain carrying an ectopic copy of Pxyl-rodA. No strong effect of those deletions on normal walled growth was observed on isotonic L-form plates, that contain excess Mg2+ (Figure 4C, upper panels) (The growth deficiency of mreB and mbl mutants under standard conditions [e.g. NA] can be rescued by the addition of high concentrations of Mg2+ (Formstone and Errington, 2005; Leaver and Errington, 2005; Schirner and Errington, 2009) In the presence of DCS, although no significant effect on L-form growth was seen in the ΔmreBH strain, deletion of either of the “major” MreB encoding genes, mreB or mbl, impaired the emergence of L-form colonies (Figure 4C, lower panels), supporting the idea that the escape in a Δ4 RodA overproducing background requires function of the Rod elongation complex.
A distinct form of L-form escape by the Rod pathway
To confirm if RodA overproduction could work to stimulate L-form escape without the need for aPBPs, we cultured Δ4 mutant cells carrying an ectopic Pxyl-rodA construct in liquid nutrient broth (NB) with or without xylose, and then diluted those cells in isotonic NB containing DCS. In the absence of xylose, cells retained their cylindrical shape for at least 60 min but also began to die, as indicated by both phase pale appearance (lysis, arrowheads) and extreme nucleoid condensation (Figure 5, −Xylose). This is consistent with no appearance of L-form colonies of Δ4 mutant cells on L-form selective plates (Figures 1C, 4B). When RodA was overproduced, most cells again retained their cylindrical shape but in this case, neither lysis nor nucleoid condensation occurred, at least during the first hour (Figure 5, +Xylose/60 min). However, after further incubation (> 120 min), L-forms, began to emerge from the still cylindrical parental cells (Figure 5 i), consistent with the L-form growth on plates shown in Figure 4B. Figure 5 ii shows an example of a cell in which the nucleoid appears to have been passing out of the parental shell in association with L-form emergence. These results suggest that two distinct pathways of L-form emergence exist, depending on whether PG synthesis is being supported by the aPBP or RodA PG synthases. Presumably, in both cases the crucial event could be the formation of cell wall lesions large enough to accommodate the exit of an L-form containing an intact nucleoid. This also suggests that RodA overproduction can enable the elongation PG system to function at the reduced PG precursor availability exerted by DCS treatment, and that this can facilitate the autolytic activity needed for L-form escape.
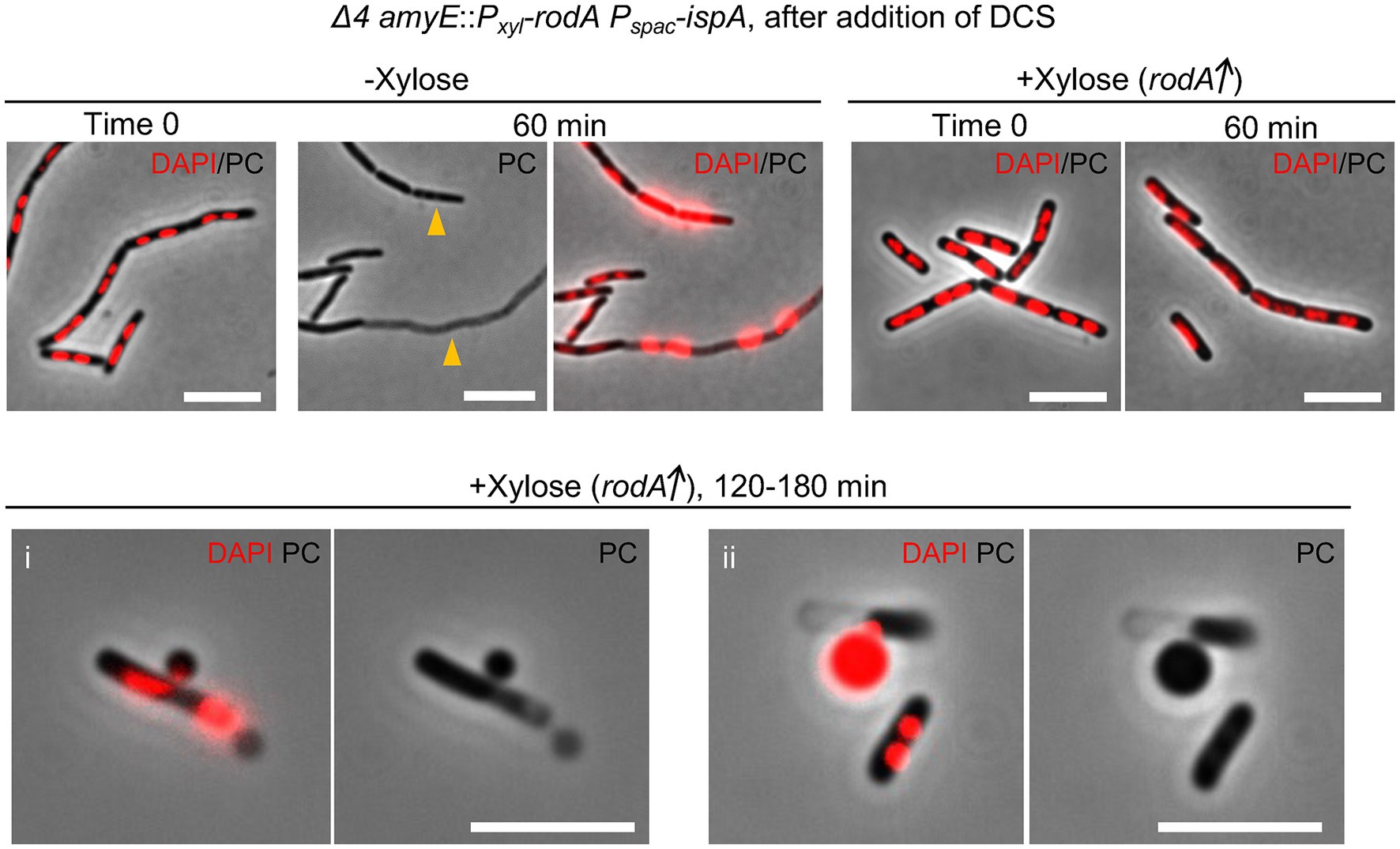
Figure 5. L-form escape by the Rod pathway Time course observation of nucleoid dynamics and cell morphology of strain YK2515 (Δ4 amyE::Pxyl-rodA Pspac-ispA). The YK2515 was cultured in isotonic NB with or without 0.5% xylose at 37°C and the exponentially growing cells were diluted in the fresh isotonic NB containing 200 μg/mL DCS. The cells were incubated at 30°C without shaking. The phase contrast and DAPI staining nucleoid images were captured by several time points as indicated. Arrowheads indicate appearance of dead cells with phase pale effect. Scale bars represent 5 μm.
LytE is the major autolysin acting with the Rod system
Given that L-form escape is dependent on the aPBPs PG synthases, as well as the autolytic enzymes, as described above (Figures 1C, 2), autolytic enzymes could be also crucial in L-form escape by the Rod system in the absence of aPBPs. Since LytE and CwlO were obvious candidates, we introduced ΔlytE and ΔcwlO mutations separately into a Δ4 strain that carried an IPTG inducible copy of the major aPBP gene, ponA. Figure 6A shows that the single deletion of cwlO had little if any effect on cell growth, with or without expression of ponA (left panels). In sharp contrast, however, repression of ponA in the strain with a deletion of lytE severely impaired growth (Figure 6A, right panels), suggesting that LytE has a more important role than CwlO during growth in cells lacking aPBPs. This would be consistent with the suggestion that LytE function is associated with the Rod system (Carballido-Lopez et al., 2006; Patel et al., 2020).
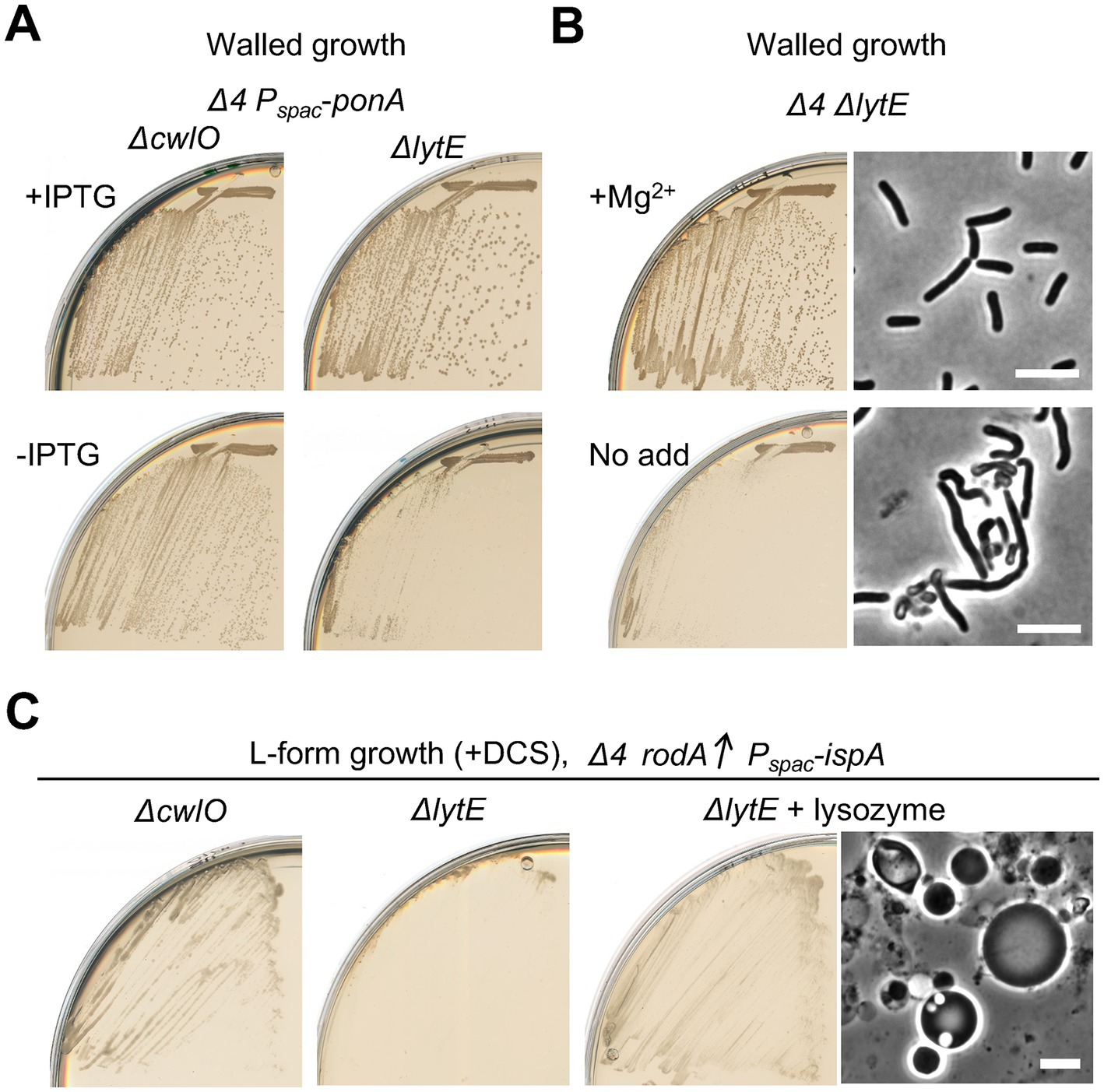
Figure 6. Crucial role for LytE during L-form escape by the Rod pathway (A) Growth of strains YK2562 (Δ4 ΔcwlO Pspac-ponA) and YK2560 (Δ4 ΔlytE Pspac-ponA) on NA plates with or without 0.5 mM IPTG (for ponA expression) at 30°C. (B) Growth and cell morphologies of strain YK2564 (Δ4 ΔlytE) on NA plates with or without added 10 mM Mg2+ at 30°C. Phase contrast micrographs were taken from colonies on the plates. Scale bars represent 5 μm. (C) L-form growth of strains YK2527 (ΔcwlO Δ4 amyE::Pxyl-rodA Pspac-ispA) and YK2526 (ΔlytE Δ4 amyE::Pxyl-rodA Pspac-ispA) on isotonic NA plates containing 1% xylose and 200 μg/mL DCS at 30°C for 2–3 days. YK256 was also cultured in the presence of 100 μg/mL lysoyme as indicated. Phase contrast micrograph was taken from the plate. Scale bars represent 5 μm.
We then tested whether L-form escape also required the LytE. (Note that, during the course of experiments, we found that the growth deficiency of a ΔlytE Δ4 mutant was suppressed in the presence of extra Mg2+ (Figure 6B) as seen for various elongation mutants (Formstone and Errington, 2005; Leaver and Errington, 2005; Schirner and Errington, 2009; Kawai et al., 2011), and taking advantage of this we generated a ΔlytE Δ4 strain carrying an ectopic copy of Pxyl-rodA) Figure 6C shows that the deletion of lytE largely prevented the emergence of L-form colonies. In contrast, no strong effect of ΔcwlO on L-form escape was observed. The escape defect in the ΔlytE Δ4 mutant was rescued by the addition of an exogenous PG hydrolase, lysozyme, leading to the emergence of L-form colonies (Figure 6C, right), confirming a crucial role for LytE activity for the escape via the Rod pathway. Thus, just as for growth of walled cells, activity of the Rod system in supporting L-form escape was largely dependent on LytE, rather than the CwlO autolysin when the aPBP system did not operate.
Discussion
Previous work from this lab had shown that one or other of LytE and CwlO are required for the walled to L-form switch that occurs when PG precursors are depleted (Kawai et al., 2018). In this situation, aPBPs are also required for the L-form switch, whereas the Rod system is not. We have now extended these findings by showing that depletion of PG precursors via antibiotic treatment or genetic repression of glmM also promotes L-form escape via the aPBP-dependent bulging process (Figure 3B) and that mutations affecting various other central components of the Rod or divisome complexes (FtsW, FtsZ, MreBCD, Mbl and MreBH) are not required for escape (Figure 2). Given that the L-form escape process requires both aPBPs and autolytic enzymes, we assume that bulging involves both PG synthetic and hydrolytic activities. Recent work with E. coli suggested that aPBP activity works to enhance the recovery of cells following cell wall inhibition (Vigouroux et al., 2020). In B. subtilis, cell width seems to be determined by the balanced activities of the Rod and aPBP systems (Dion et al., 2019). Increased Rod system activity associates with thinner cells and a more ordered PG matrix, but aPBP activity associates with increased width and less oriented material in the wall (Dion et al., 2019). The aPBPs may fill in the gaps in a foundational structure of oriented PG laid down in the cell cylinder by the Rod system to strengthen and expand its width (Rohs and Bernhardt, 2021). It has also been reported that assembly of the Rod elongation complex is dependent on the cellular abundance of the PG precursors in B. subtilis (Schirner et al., 2015). Based on our results we suggest an alternative or additional role for the aPBP system in supporting continued PG expansion when precursor availability is reduced. Nevertheless, the Rod elongation complex can clearly still work to support L-form escape in the absence of aPBP activity at low PG precursor levels, when RodA is overproduced (Figures 4, 5). Elucidating how the levels of PG precursors selectively influence the PG synthetic systems, thereby impacting on the mode of PG synthesis and L-form escape, is an important challenge for future.
As mentioned above, overproduction of RodA could rescue L-form generation in a Δ4 mutant background during DCS treatment (Figure 4). Under these circumstances, bulging growth did not occur and the cells retained an approximately cylindrical shape (Figure 5). Nevertheless, L-form escape did occur, often from polar or approximately mid-cell locations. We previously reported that a strain with what turned out to be a complex mutant background, 168ED, also gave rise to L-forms, albeit less efficiently than in the experiments described here. In that case, L-form emergence also occurred without extensive cell bulging. The best understood mutation conferring this phenotype lay in the sepF gene (Domínguez-Cuevas et al., 2012). sepF mutations give rise to defective malformed division sites (Gündoğdu et al., 2011). Taken together with our new findings, we suggest that L-form escape requires the formation of a substantial cell wall lesion large enough to allow the passage of an L-form cell and its nuclear and cytoplasmic components, without serious damage to the chromosome or spillage of contents. It will be interesting in the future to explore the mechanisms involved in the successful exit of L-forms from their walled parent cells.
The cell wall-independent growth of L-forms allowed us to test whether either or both of the “essential” autolysins was required for L-form escape. As shown in Figure 6C, LytE was required but CwlO not. Thus, just as for growth of vegetative cells, it appears that LytE is partially specialised for functioning in the Rod pathway during L-form escape. Further work is needed to elucidate the molecular mechanisms coupling synthetic and autolytic activities during normal cell growth when these activities are balanced. Our results suggest that L-form escape will provide a useful experimental system for studying the coupling when lytic activity becomes dominant.
Data availability statement
The original contributions presented in the study are included in the article/supplementary material, further inquiries can be directed to the corresponding author.
Author contributions
YK designed concepts, performed experiments, and wrote the original draught of the manuscript. JE designed concepts, reviewed and edited the manuscript, and funding acquisition. All authors contributed to the article and approved the submitted version.
Funding
This work was supported by a European Research Council Advanced award (670980), a Wellcome Investigator Award (209500) and an ARC Laureate Fellowship (FL210100071).
Conflict of interest
The authors declare that this work carried out in the absence of any personal, commercial or financial relationships that could potentially be construed as a conflict of interest.
Publisher’s note
All claims expressed in this article are solely those of the authors and do not necessarily represent those of their affiliated organizations, or those of the publisher, the editors and the reviewers. Any product that may be evaluated in this article, or claim that may be made by its manufacturer, is not guaranteed or endorsed by the publisher.
Footnotes
References
Adams, D. W., Wu, L. J., Czaplewski, L. G., and Errington, J. (2011). Multiple effects of benzamide antibiotics on FtsZ function. Mol. Microbiol. 80, 68–84. doi: 10.1111/j.1365-2958.2011.07559.x
Allan, E. J., Hoischen, C., and Gumpert, J. (2009). Bacterial L-forms. Adv. Appl. Microbiol. 68, 1–39. doi: 10.1016/S0065-2164(09)01201-5
Bisicchia, P., Noone, D., Lioliou, E., Howell, A., Quigley, S., Jensen, T., et al. (2007). The essential YycFG two-component system controls cell wall metabolism in Bacillus subtilis. Mol. Microbiol. 65, 180–200. doi: 10.1111/j.1365-2958.2007.05782.x
Carballido-Lopez, R., Formstone, A., Li, Y., Ehrlich, S. D., Noirot, P., and Errington, J. (2006). Actin homolog MreBH governs cell morphogenesis by localization of the cell wall hydrolase LytE. Dev. Cell 11, 399–409. doi: 10.1016/j.devcel.2006.07.017
Claessen, D., Emmins, R., Hamoen, L. W., Daniel, R. A., Errington, J., and Edwards, D. H. (2008). Control of the cell elongation-division cycle by shuttling of PBP1 protein in Bacillus subtilis. Mol. Microbiol. 68, 1029–1046. doi: 10.1111/j.1365-2958.2008.06210.x
Dion, M. F., Kapoor, M., Sun, Y., Wilson, S., Ryan, J., Vigouroux, A., et al. (2019). Bacillus subtilis cell diameter is determined by the opposing actions of two distinct cell wall synthetic systems. Nat. Microbiol. 4, 1294–1305. doi: 10.1038/s41564-019-0439-0
Dobihal, G. S., Brunet, Y. R., Flores-Kim, J., and Rudner, D. Z. (2019). Homeostatic control of cell wall hydrolysis by the WalRK two-component signaling pathway in Bacillus subtilis. elife 8:e52088. doi: 10.7554/eLife.52088
Domingue, G. J., and Woody, H. B. (1997). Bacterial persistence and expression of disease. Clin. Microbiol. Rev. 10, 320–344. doi: 10.1128/CMR.10.2.320
Domínguez-Cuevas, P., Mercier, R., Leaver, M., Kawai, Y., and Errington, J. (2012). The rod to L-form transition of Bacillus subtilis is limited by a requirement for the protoplast to escape from the cell wall sacculus. Mol. Microbiol. 83, 52–66. doi: 10.1111/j.1365-2958.2011.07920.x
Domínguez-Cuevas, P., Porcelli, I., Daniel, R. A., and Errington, J. (2013). Differentiated roles for MreB-actin isologues and autolytic enzymes in Bacillus subtilis morphogenesis. Mol. Microbiol. 89, 1084–1098. doi: 10.1111/mmi.12335
Egan, A. J. F., Errington, J., and Vollmer, W. (2020). Regulation of peptidoglycan synthesis and remodelling. Nat. Rev. Microbiol. 18, 446–460. doi: 10.1038/s41579-020-0366-3
Emami, K., Guyet, A., Kawai, Y., Devi, J., Wu, L. J., Allenby, N., et al. (2017). RodA as the missing glycosyltransferase in Bacillus subtilis and antibiotic discovery for the peptidoglycan polymerase pathway. Nat. Microbiol. 2:16253. doi: 10.1038/nmicrobiol.2016.253
Errington, J., Mickiewicz, K., Kawai, Y., and Wu, L. J. (2016). L-form bacteria, chronic diseases and the origins of life. Philos. Trans. R. Soc. Lond. Ser. B Biol. Sci. 371:20150494. doi: 10.1098/rstb.2015.0494
Formstone, A., and Errington, J. (2005). A magnesium-dependent mreB null mutant: implications for the role of mreB in Bacillus subtilis. Mol. Microbiol. 55, 1646–1657. doi: 10.1111/j.1365-2958.2005.04506.x
Fukushima, T., Afkham, A., Kurosawa, S., Tanabe, T., Yamamoto, H., and Sekiguchi, J. (2006). A new D,L-endopeptidase gene product, YojL (renamed CwlS), plays a role in cell separation with LytE and LytF in Bacillus subtilis. J. Bacteriol. 188, 5541–5550. doi: 10.1128/JB.00188-06
Gamba, P., Hamoen, L. W., and Daniel, R. A. (2016). Cooperative recruitment of FtsW to the division site of Bacillus subtilis. Front. Microbiol. 7:1808. doi: 10.3389/fmicb.2016.01808
Garner, E. C. (2021). Toward a mechanistic understanding of bacterial rod shape formation and regulation. Annu. Rev. Cell Dev. Biol. 37, 1–21. doi: 10.1146/annurev-cellbio-010521-010834
Glaser, P., Sharpe, M. E., Raether, B., Perego, M., Ohlsen, K., and Errington, J. (1997). Dynamic, mitotic-like behavior of a bacterial protein required for accurate chromosome partitioning. Genes Dev. 11, 1160–1168. doi: 10.1101/gad.11.9.1160
Gündoğdu, M. E., Kawai, Y., Pavlendova, N., Ogasawara, N., Errington, J., Scheffers, D. J., et al. (2011). Large ring polymers align FtsZ polymers for normal septum formation. EMBO J. 30, 617–626. doi: 10.1038/emboj.2010.345
Hashimoto, M., Ooiwa, S., and Sekiguchi, J. (2012). Synthetic lethality of the lytE cwlO genotype in Bacillus subtilis is caused by lack of D,L-endopeptidase activity at the lateral cell wall. J. Bacteriol. 194, 796–803. doi: 10.1128/JB.05569-11
Höltje, J. V. (1998). Growth of the stress-bearing and shape-maintaining Murein Sacculus ofEscherichia coli. Microbiol. Mol. Biol. Rev. 62, 181–203. doi: 10.1128/MMBR.62.1.181-203.1998
Kawai, Y., Asai, K., and Errington, J. (2009). Partial functional redundancy of MreB isoforms, MreB, Mbl and MreBH, in cell morphogenesis of Bacillus subtilis. Mol. Microbiol. 73, 719–731. doi: 10.1111/j.1365-2958.2009.06805.x
Kawai, Y., Marles-Wright, J., Cleverley, R. M., Emmins, R., Ishikawa, S., Kuwano, M., et al. (2011). A widespread family of bacterial cell wall assembly proteins. EMBO J. 30, 4931–4941. doi: 10.1038/emboj.2011.358
Kawai, Y., Mercier, R., Mickiewicz, K., Serafini, A., Sório de Carvalho, L. P., and Errington, J. (2019). Crucial role for central carbon metabolism in the bacterial L-form switch and killing by β-lactam antibiotics. Nat. Microbiol. 4, 1716–1726. doi: 10.1038/s41564-019-0497-3
Kawai, Y., Mercier, R., Wu, L. J., Domínguez-Cuevas, P., Oshima, T., and Errington, J. (2015). Cell growth of wall-free L-form bacteria is limited by oxidative damage. Curr. Biol. 25, 1613–1618. doi: 10.1016/j.cub.2015.04.031
Kawai, Y., Mickiewicz, K., and Errington, J. (2018). Lysozyme counteracts beta-lactam antibiotics by promoting the emergence of L-form Bacteria. Cells 172:e10. doi: 10.1016/j.cell.2018.01.021
Klieneberger, E. (1935). The natural occurrence of pleuropneumonia-like organism in apparent symbiosis withStrrptobacillus moniliformis and other bacteria. J. Pathol. Bacteriol. 40, 93–105. doi: 10.1002/path.1700400108
Leaver, M., Domínguez-Cuevas, P., Coxhead, J. M., Daniel, R. A., and Errington, J. (2009). Life without a wall or division machine in Bacillus subtilis. Nature 457, 849–853. doi: 10.1038/nature07742
Leaver, M., and Errington, J. (2005). Roles for MreC and MreD proteins in helical growth of the cylindrical cell wall in Bacillus subtilis. Mol. Microbiol. 57, 1196–1209. doi: 10.1111/j.1365-2958.2005.04736.x
Lovering, A. L., Safadi, S. S., and Strynadka, N. C. (2012). Structural perspective of peptidoglycan biosynthesis and assembly. Annu. Rev. Biochem. 81, 451–478. doi: 10.1146/annurev-biochem-061809-112742
McPherson, D. C., and Popham, D. L. (2003). Peptidoglycan synthesis in the absence of class a penicillin-binding proteins in Bacillus subtilis. J. Bacteriol. 185, 1423–1431. doi: 10.1128/JB.185.4.1423-1431.2003
Meeske, A. J., Riley, E. P., Robins, W. P., Uehara, T., Mekalanos, J. J., Kahne, D., et al. (2016). SEDS proteins are a widespread family of bacterial cell wall polymerases. Nature 537, 634–638. doi: 10.1038/nature19331
Meisner, J., Montero Llopis, P., Sham, L. T., Garner, E., Bernhardt, T. G., and Rudner, D. Z. (2013). FtsEX is required for CwlO peptidoglycan hydrolase activity during cell wall elongation in Bacillus subtilis. Mol. Microbiol. 89, 1069–1083. doi: 10.1111/mmi.12330
Mercier, R., Kawai, Y., and Errington, J. (2013). Excess membrane synthesis drives a primitive mode of cell proliferation. Cells 152, 997–1007. doi: 10.1016/j.cell.2013.01.043
Mickiewicz, K. M., Kawai, Y., Drage, L., Gomes, M. C., Davison, F., Pickard, R., et al. (2019). Possible role of L-form switching in recurrent urinary tract infection. Nat. Commun. 10:4379. doi: 10.1038/s41467-019-12359-3
Patel, Y., Zhao, H., and Helmann, J. D. (2020). A regulatory pathway that selectively up-regulates elongasome function in the absence of class a PBPs. elife 9:e57902. doi: 10.7554/eLife.57902
Reichmann, N. T., Tavares, A. C., Saraiva, B. M., Jousselin, A., Reed, P., Pereira, A. R., et al. (2019). SEDS-bPBP pairs direct lateral and septal peptidoglycan synthesis in Staphylococcus aureus. Nat. Microbiol. 4, 1368–1377. doi: 10.1038/s41564-019-0437-2
Rohs, P. D. A., and Bernhardt, T. G. (2021). Growth and division of the peptidoglycan matrix. Annu. Rev. Microbiol. 75, 315–336. doi: 10.1146/annurev-micro-020518-120056
Salzberg, L. I., Powell, L., Hokamp, K., Botella, E., Noone, D., and Devine, K. M. (2013). The WalRK (YycFG) and sigma(I) RsgI regulators cooperate to control CwlO and LytE expression in exponentially growing and stressed Bacillus subtilis cells. Mol. Microbiol. 87, 180–195. doi: 10.1111/mmi.12092
Schirner, K., and Errington, J. (2009). The cell wall regulator σI specifically suppresses the lethal phenotype of mbl mutants in Bacillus subtilis. J. Bacteriol. 191, 1404–1413. doi: 10.1128/JB.01497-08
Schirner, K., Eun, Y. J., Dion, M., Luo, Y., Helmann, J. D., Garner, E. C., et al. (2015). Lipid-linked cell wall precursors regulate membrane association of bacterial actin MreB. Nat. Chem. Biol. 11, 38–45. doi: 10.1038/nchembio.1689
Sjodt, M., Brock, K., Dobihal, G., Rohs, P. D. A., Green, A. G., Hopf, T. A., et al. (2018). Structure of the peptidoglycan polymerase RodA resolved by evolutionary coupling analysis. Nature 556, 118–121. doi: 10.1038/nature25985
Sjodt, M., Rohs, P. D. A., Gilman, M. S. A., Erlandson, S. C., Zheng, S., Green, A. G., et al. (2020). Structural coordination of polymerization and crosslinking by a SEDS-bPBP peptidoglycan synthase complex. Nat. Microbiol. 5, 813–820. doi: 10.1038/s41564-020-0687-z
Taguchi, A., Welsh, M. A., Marmont, L. S., Lee, W., Sjodt, M., Kruse, A. C., et al. (2019). FtsW is a peptidoglycan polymerase that is functional only in complex with its cognate penicillin-binding protein. Nat. Microbiol. 4, 587–594. doi: 10.1038/s41564-018-0345-x
Typas, A., Banzhaf, M., Gross, C. A., and Vollmer, W. (2012). From the regulation of peptidoglycan synthesis to bacterial growth and morphology. Nat. Rev. Microbiol. 10, 123–136. doi: 10.1038/nrmicro2677
Vigouroux, A., Cordier, B., Aristov, A., Alvarez, L., Ozbaykal, G., Chaze, T., et al. (2020). Class-a penicillin binding proteins do not contribute to cell shape but repair cell-wall defects. elife 9:e51998. doi: 10.7554/eLife.51998
Wei, Y., Havasy, T., McPherson, D. C., and Popham, D. L. (2003). Rod shape determination by the Bacillus subtilis class B penicillin-binding proteins encoded by pbpA and pbpH. J. Bacteriol. 185, 4717–4726. doi: 10.1128/JB.185.16.4717-4726.2003
Keywords: L-form bacteria, antibiotic resistance, Bacillus subtilis , cell wall, RodA, penicillin-binding proteins, LytE
Citation: Kawai Y and Errington J (2023) Dissecting the roles of peptidoglycan synthetic and autolytic activities in the walled to L-form bacterial transition. Front. Microbiol. 14:1204979. doi: 10.3389/fmicb.2023.1204979
Edited by:
Chang-Ro Lee, Myongji University, Republic of KoreaReviewed by:
Wonsik Lee, School of Pharmacy, Sungkyunkwan University, Republic of KoreaChristoph Mayer, University of Tübingen, Germany
Copyright © 2023 Kawai and Errington. This is an open-access article distributed under the terms of the Creative Commons Attribution License (CC BY). The use, distribution or reproduction in other forums is permitted, provided the original author(s) and the copyright owner(s) are credited and that the original publication in this journal is cited, in accordance with accepted academic practice. No use, distribution or reproduction is permitted which does not comply with these terms.
*Correspondence: Yoshikazu Kawai, eW9zaGlrYXp1Lmthd2FpQHN5ZG5leS5lZHUuYXU=
†Present addresses: Yoshikazu Kawai, Faculty of Medicine and Health, University of Sydney, Sydney, NSW, AustraliaJeff Errington, Faculty of Medicine and Health, University of Sydney, Darlington, Australia
‡ORCID: Jeff Errington, https://orcid.org/0000-0002-6977-9388