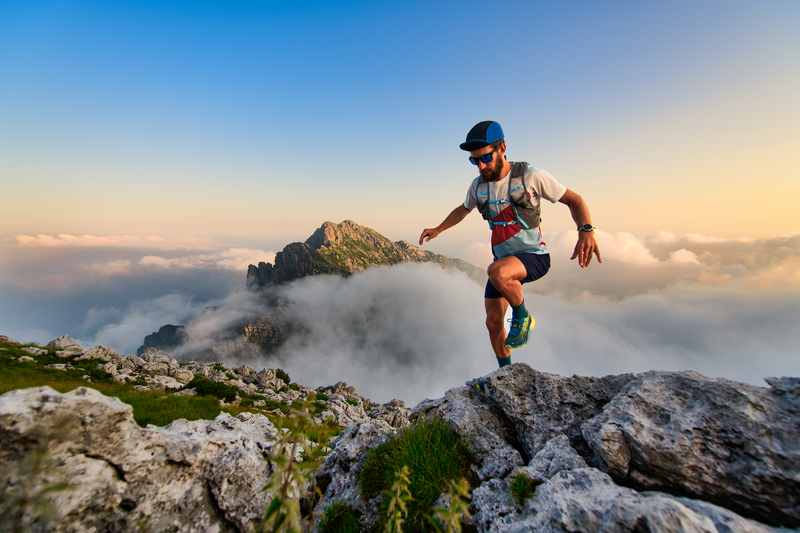
94% of researchers rate our articles as excellent or good
Learn more about the work of our research integrity team to safeguard the quality of each article we publish.
Find out more
REVIEW article
Front. Microbiol. , 22 June 2023
Sec. Microorganisms in Vertebrate Digestive Systems
Volume 14 - 2023 | https://doi.org/10.3389/fmicb.2023.1194419
With the increasing number of cholecystectomy and the high proportion of colorectal cancer in malignant tumors, the question of whether cholecystectomy is a risk factor for colorectal disease has been widely concerned. After reviewing the literature at home and abroad, the authors will summarize the research progress of the correlation between the occurrence of colorectal tumors after cholecystectomy, in order to provide help for the prevention and treatment of colorectal tumors.
The gallbladder is one of the most important organs of the digestive system, which has the functions of movement, transport, absorption, and secretion and can concentrate and store bile between meals. After eating food, the gallbladder in the human body drains bile into the intestinal cavity through its own contraction, which can not only regulate the enterohepatic circulation of bile acids but also promote the absorption of dietary lipids (Qi et al., 2019). During fasting, liver bile continues to flow into the gallbladder cavity, where it is concentrated and acidified before being stored in the gallbladder (Housset et al., 2016). The movement function of the gallbladder has a great influence on the metabolism and enterohepatic circulation of the bile acid. Around the world, gallstones are one of the most common digestive disorders, with a worldwide prevalence of 10–15% (Shabanzadeh, 2018). In recent years, the prevalence of gallstones has gradually increased due to the increase in high-calorie, high-carbohydrate, and low-fiber diets and the decrease in physical activity (Tsai et al., 2008). Although most affected individuals remain asymptomatic during their lifetime, 10–25% will develop symptomatic gallstone disease, the consequences of which can range from simple biliary colic to potentially life-threatening complications such as acute or chronic cholecystitis, choledocholithiasis, cholangitis, biliary pancreatitis, and rarely, gallbladder cancer (Sakorafas et al., 2007). Currently, cholecystectomy is internationally recognized as the best treatment for symptomatic gallstones. Cholecystectomy can be performed in two main ways as follows: a laparoscopic or a classic open operation technique. Compared with the classic way, laparoscopic cholecystectomy is a relatively minimally invasive surgical procedure and has basically replaced the open technique for cholecystectomies since the early 1990s (Chattopadhyay and Das, 2020). Several advantages have made it a popular procedure over the past few decades, including a short hospital stay, quick return to normal activities, reduced pain after surgery, more acceptable cosmetic results, less morbidity, and less mortality (Islam et al., 2021). With the emergence of laparoscopic technology, cholecystectomy has been further widely applied worldwide, and more and more patients are undergoing or have undergone cholecystectomy (Harrison et al., 2012; Tiderington et al., 2016). However, in addition to serious surgery-related complications (such as bile duct injury, bleeding, bile leakage, and infection), surgical removal of the functional gallbladder in patients with gallstones may also lead to some physiological changes in the gastrointestinal tract (Sicklick et al., 2005). Cholecystectomy changes the way bile drains into the intestinal cavity and increases the enterohepatic recirculation rate of bile acids. It also increases the exposure of intestinal flora to bile acid pools, eventually leading to an increase in the proportion of secondary bile acids (SBAs) (Wang et al., 2018). Postcholecystectomy may affect the long-term prognosis of patients. In addition to an increased risk of colorectal cancer (Goldacre et al., 2012), cholecystectomy is also associated with an increased risk of other gastrointestinal cancers and metabolic syndromes (such as insulin resistance, weight gain, dyslipidemia, hepatic steatosis, and hypertension) (Saklayen, 2018; Uldall Torp et al., 2020).
Colorectal cancer (CRC) is a relatively common cancer with a high degree of malignancy. Although its incidence is lower than lung cancer and stomach cancer, its mortality rate ranks second place. More and more clinical data show an increasing incidence in young and middle-aged people accompanied by a higher degree of malignancy (Saraste et al., 2020). The global estimated crude incidence of colon cancer in 2020 was 14.7 per 100,000, and the standardized incidence was 11.4 per 100,000. The crude mortality rate was 7.4 per 100,000, and the standard mortality rate was 5.4 per 100,000. The global estimated crude incidence of rectal cancer was 9.4 per 100,000, and the standard incidence was 7.6 per 100,000. The crude mortality rate was 4.3 per 100,000, and the standard mortality rate was 3.3 per 100,000. In 2020, the estimated incidence of colon cancer in our country was 26.6% of the world, with a crude incidence of 21.1/100,000 and a standard rate of 13.1/100,000. Colon cancer is estimated to account for 28.6% of global deaths, with a crude mortality rate of 11.4 per 100,000 and a standardized rate of 6.8 per 100,000. It is estimated that the incidence of rectal cancer accounts for 33.4% of the global population, with a crude incidence of 16.9 per 100,000 and a standardized rate of 10.6 per 100,000. Colorectal cancer is estimated to account for 35.1% of global deaths, with a crude mortality rate of 8.2 per 100,000 and a standardized rate of 5.0 per 100,000 (Sung et al., 2021).
Colorectal cancer is a complex disease, the cause and mechanism of which have not been fully elucidated so far. Known influencing factors include age, genetic factors, diet, metabolic diseases, and environment (Cho et al., 2019; De Almeida et al., 2019; Song and Chan, 2019). With the rise of molecular biology in recent years, through the application of gene sequencing technology, metabolomics, and other applications, more and more studies have found that intestinal flora disorders are closely related to the occurrence and development of CRC (Bundgaard-Nielsen et al., 2019). In recent years, several scholars have studied the intestinal flora after cholecystectomy, and the research consensus is that cholecystectomy changes the intestinal flora composition of patients and puts them at a high risk of colorectal cancer (Chen et al., 2014, 2020; Ábrahám et al., 2020).
At present, many scholars have conducted in-depth studies on the relationship and its mechanism between cholecystectomy and colorectal cancer through case–control studies, prospective and retrospective cohort studies, animal experimental studies, etc. Most scholars believe that cholecystectomy can increase the risk of colorectal cancer. However, its relationship with colorectal cancer after cholecystectomy and its mechanism remain unclear. In view of this, this article will combine the research progress at home and abroad in recent years, with the theme of “Relationship between Cholecystectomy and Colorectal Cancer” and make a review.
Most domestic and foreign studies suggest that cholecystectomy can increase the risk of colorectal cancer. A meta-analysis covering 33 case–control studies showed an association between cholecystectomy and risk of colorectal cancer (pooled relative risk [RR] = 1.34; 95% confidence interval [CI] = 1.14–1.57), particularly when limited to the proximal colon (RR = 1.88; 95% CI = 1.54–2.30) (Giovannucci et al., 1993). Another meta-analysis of nine cohort studies showed that an increased risk of CRC was found among the individuals who had undergone cholecystectomy [risk ratio (RR) 1.22; 95% confidence interval (CI) 1.08–1.38]. In addition, we also found a promising increased risk for colon cancer (CC) (RR 1.30, 95% CI 1.07–1.58), but no relationship between cholecystectomy and rectum cancer (RC) (RR 1.09; 95% CI 0.89–1.34) was observed. Furthermore, a positive relationship between the female gender and CRC was also found (RR 1.17; 95% CI 1.03–1.34) (Zhang et al., 2017a). Xu et al. (2009) and Chiong et al. (2012) confirmed an increased incidence of colorectal cancer in individuals who underwent cholecystectomy. Kim et al. (2020) studied the association between cholecystectomy and stomach or colorectal cancer in Korean individuals by collecting data about patients who underwent cholecystectomy at their institutions over an 8-year period (excluding patients with other gastrointestinal cancers and those diagnosed with stomach or colorectal cancer within 1 year of cholecystectomy). They found that the risk of CRC increased up to 108% after cholecystectomy in the patients compared with that in the general population with statistical significance. Moreover, the risk of CRC increased in female patients compared with that in the general population when analyzed by sex. The male and female participants had a 74 and 154% higher risk of CRC, respectively. In recent years, relevant literature has been updated continuously, but the analysis results still maintain a high consistency. These results suggest that the history of cholecystectomy is closely related to the occurrence and development of colorectal cancer.
There are also scholars who hold opposing views on this issue. A retrospective study in Hungary found that although a statistically higher risk of colon cancer was observed after gallbladder removal, the incubation period was long. Therefore, cholecystectomy may not be an independent risk factor for colorectal cancer (Mándi et al., 2021). A Japanese study revealed a negative association between cholecystectomy and colorectal cancer (Kaibara et al., 1986). Other studies have shown no relationship between cholecystectomy and colorectal cancer (Vinikoor et al., 2007; Zhao et al., 2012).
Most studies suggest that cholecystectomy is closely related to the occurrence of right colon cancer, but there is no obvious correlation with rectal cancer. Shao and Yang (2005) evaluated the relationship between cholecystectomy and colorectal cancer and found that cholecystectomy could promote the occurrence of colon cancer, especially right-sided colorectal cancer, and had nothing to do with the occurrence of rectal cancer and left-sided colorectal cancer. A small number of studies believe that cholecystectomy also has a certain impact on the occurrence of rectal cancer. Some studies have found that the increased risk of proximal colon cancer and rectal cancer after cholecystectomy is statistically significant (Schernhammer et al., 2003). Giovannucci et al. (1993) proposed that after cholecystectomy, CRC was more likely to occur in the proximal colon, which might be due to the difference in the origin of the proximal and distal colon embryos, so there was a difference in susceptibility to tumor formation.
Some scholars believe that the effect of cholecystectomy on colorectal cancer is a unimodal wave, and the risk of colorectal cancer after the operation gradually increases and gradually decreases after reaching the peak (Zhang et al., 2021). Some studies found that cholecystectomy has a positive effect on the occurrence of colorectal cancer, but the positive effect disappears after 10 years (Altieri et al., 2004). Chen et al. (2014) followed up patients after cholecystectomy and found that the incidence of colorectal cancer increased within 5 years after cholecystectomy, but no significant effect was observed 5 years later. Lee et al. (2018) also reached a consistent conclusion through their findings; a higher risk of colorectal cancer was observed 1–5 years after cholecystectomy.
In recent years, studies have shown that significant changes in bile acid metabolism after cholecystectomy may be the main cause of colorectal cancer induction, but the specific mechanism of colorectal cancer induction after cholecystectomy remains unclear. According to the research progress at home and abroad, the changes in bile acid metabolism after cholecystectomy and the mechanism of inducing colorectal cancer were briefly reviewed.
Studies have shown that the bile acid pool of patients undergoing cholecystectomy decreased 3 months after surgery, and the total amount of bile acids decreased by approximately 16%, while the total amount of bile acids remained basically unchanged 5~8 years after surgery (Kullak-Ublick et al., 1995). Another study found that the synthesis rate of anoxycholic acid and the capacity of the bile acid pool decreased 6 weeks after cholecystectomy, but the synthesis rate of anoxycholic acid and deoxycholic acid and the size of the bile acid pool did not change significantly 9 to 12 months after surgery (Zhao et al., 2022). These results suggest that the synthesis of bile acids is inhibited after cholecystectomy, and the size of the bile acid pool is reduced in the short term, but there is no significant effect in the long term.
After cholecystectomy, bile loses its storage place, and the internal pressure in the biliary tract is higher than the pressure of the Oddi sphincter, which leads to the long-term opening of the Oddi sphincter, resulting in the continuous discharge of bile produced by the liver into the intestine and an increase of the enterohepatic circulation of bile acids (Han et al., 2012). The amount of enterohepatic circulation of bile acids after cholecystectomy is twice that of healthy people (Sergeev et al., 2020). Clinical studies have shown that after cholecystectomy, the hepatoenteric circulation of bile acids will be accelerated, the content of cholic acid (CA) in intestinal bile acid will be decreased, the content of deoxycholic acid (DCA) and lithocholic acid (LCA) will be increased (Roda et al., 1978), and the content of SBAs in fecal bile acids will be increased (Zuccato et al., 1993). Studies have found that after cholecystectomy, bile acid circulation increases, the contact between primary bile acid and intestinal flora increases, the dehydroxylation of primary bile acid is enhanced, and the concentration of SBAs (especially stone cholic acid) in bile is significantly increased (Zhai et al., 2019). In addition, cholecystectomy increases bacterial decoupling and dehydroxylation of bile acids, thereby increasing the proportion of SBAs (Housset et al., 2016). Zhang et al. (2017b) results showed that deoxycholic acid, lithocholic acid, and their combined products with taurine significantly increased in ileal contents of mice after cholecystectomy, and fecal bile acids also increased accordingly.
Studies have shown that there is an interaction between bile acid metabolism and intestinal microflora (Chunyang et al., 2019). After cholecystectomy, bile synthesis, secretion, concentration, excretion, and reabsorption are abnormal. A low concentration of bile acids reduces the antibacterial effect, which may cause excessive proliferation of intestinal bacteria. A low concentration of bile acids can reduce the transepithelial resistance of the colon tissue, increase the permeability of the intestinal epithelium, and increase the absorption of bacteria in the colon tissue. The reduced reabsorption of bile acids in the small intestine leads to the increase of bile acids discharged into the colon, which promotes the increase of the production of SBAs, enhances the inhibition of beneficial bacteria, and further leads to intestinal flora disorder.
High levels of SBAs and deoxycholic acid (DCA) in fecal matter and patient's serum correlate with increased adenomatous polyp recurrence and the development of higher grade, and more aggressive disease (Bayerdörffer et al., 1993). Animal experiments show that bile acids, especially DCA and LCA, can enhance the effect of cancer inducers on colorectal cancer (Zusman and Zimber, 1991). Bo Yang et al. conducted a study on the effect of cholecystectomy on colorectal tumors in mice caused by dimethylhydrazine (DMH) and found through a mice colon cancer induction experiment that cholecystectomy could increase the S-phase cells of the colon mucosal epithelium in mice, indicating that cholecystectomy can promote the occurrence of colon cancer, which may be related to the increased content of lithocholic acid in SBAs in the intestinal tract after operation (Bo et al., 2002). Kuniyasu et al. (1986) induced large intestinal carcinogenesis in hamsters using methylazoxymethanol (MAM), and the results indicate an enhancing effect of cholecystectomy on MAM acetate-induced large intestinal carcinogenesis in hamsters, which may be related to abnormal metabolism of secondary bile acid in the intestinal tract after operation.
At the cellular level, SBAs promote the proliferation of normal colorectal cells and tumor cells at the same time by changing the morphology and dynamics of colonic mucosa cells (Ajouz et al., 2014). Bile acids, including SBAs, are hydrophobic and therefore may alter the stability of the membrane lipid bilayer, and have the capacity to disturb the structure of, or partly digest, cell membranes (Sagawa et al., 1993). Secondary bile acids (DCA and LCA) also increased paracellular permeability in a dose-related manner, with LCA exerting more potent effects than DCA (Stenman et al., 2012). When present in high concentrations, SBAs cause unspecific cell membrane damage, resulting in focal destruction of intestinal epithelium (Payne et al., 2008). SBAs reduce immune function by inhibiting the proliferation of intestinal mucosa lamina propria lymphocytes (Collins et al., 2023).
At the molecular level, secondary bile acids can cause DNA damage, directly interfere with DNA metabolism, increase thymidine insertion and DNA synthesis outside normal sequence, improve ornithine decarboxylase activity in colonic mucosa cells, and induce multi-gene mutations of oncogenes, onco-suppressor genes, and mismatch repair genes (Ajouz et al., 2014; Niekamp and Kim, 2023). Secondary bile acids can induce genomic instability in colonic epithelial cells through multiple mechanisms, including the disruption of mitosis (leading to aneuploidy), defects in spindle assembly checkpoints, oxidative DNA damage, cell cycle arrest at G1 and/or G2 along with an improper alignment of chromosomes at the metaphase plate and multipolar divisions (Degirolamo et al., 2011). DCA stimulates extracellular signal-regulated kinases, which counteracts the tumor-suppressive activity of the tumor suppressor p53 (Qiao et al., 2001a). DCA impaired p53 signaling in a cancer cell line exposed to DNA-damaging reagents in vitro (Qiao et al., 2001b).
At present, it has become clear that bile acids can also act as versatile signaling molecules and even regulate gene expression. Bile acids have been discovered to activate specific nuclear receptors (farnesoid X receptor, preganane X receptor, and vitamin D receptor), G-protein-coupled receptor TGR5 (TGR5), and cell signaling pathways (c-jun N-terminal kinase 1/2, AKT, and ERK 1/2) in cells in the liver and gastrointestinal tract. Activation of nuclear receptors and cell signaling pathways alter the expression of numerous genes encoding enzymes/proteins involved in the regulation of bile acid, glucose, fatty acid, lipoprotein synthesis, metabolism, transport, and energy metabolism (Hylemon et al., 2009). Major pathways implicated in colon cancer development include WNT signaling, phosphatidylinositol 3-kinase (PI3K) signaling, MAPK signaling, p53 deregulation, and more recently prostaglandin E2 (PGE2) signaling (Centuori and Martinez, 2014). Secondary bile acids increase the risk of colorectal cancer through the epidermal growth factor receptor (EGFR), Wnt/β-catenin, and protein kinase C signaling pathways (Bingfeng et al., 2020). Yao (2018) identified the key role of secondary bile acids (especially deoxycholic acids) in the occurrence and development of colorectal cancer, namely, they mainly trigger colorectal cancer through the four pathways of NF Kb, Wnt/β-catenin, mitogen-activated protein kinase (MAPK), and miRNA.
Sustained exposure to deoxycholic acid (DCA) in the colon epithelium after cholecystectomy may have cytotoxic effects that promote colon cancer progression by inhibiting FXR expression and enhancing the Wnt-β-catenin pathway (Yao et al., 2022). The canonical WNT pathway is activated when a number of WNT ligands bind to Frizzled receptors. This interaction activates disheveled (DSH), which, then, inhibits the complex of proteins composed of axin, GSK-3β, and APC. This complex usually promotes the proteolytic degradation of β-catenin. By activating WNT signaling, this degradation is blocked, and β-catenin is able to accumulate in the cytoplasm and then translocate to the nucleus where it is able to interact with TCF and LEF transcription factors, resulting in upregulation of specific genes involved in cellular proliferation (Logan and Nusse, 2004).
β-Catenin is a key component of adherens junctions that link the actin cytoskeleton to members of the cadherin family of transmembrane cell–cell adhesion receptors. Tyrosine phosphorylation of β-catenin has been suggested to promote metastatic potential and tumor invasiveness by stabilizing β-catenin and promoting its binding of T cell factor (TCF)/lymphocyte enhancer factor 1 DNA transcription factors. The latter complex functions as a transcriptional activator and plays a key role in regulating cancer cell proliferation and metastasis. Transcriptional targets of β-catenin/TCF include cyclin D1, urokinase plasminogen activator receptor (uPAR), matrix metalloproteinase 7, cyclooxygenase-2, gastrin, and CD44 (Wong and Pignatelli, 2002). The experiment proved that physiologically relevant concentrations (5 or 50 μM) of DCA activate β-catenin signaling pathway and increase urokinase plasminogen activator (uPA), uPAR, and cyclin D1 expression in colon cancer cells. Inhibition of β-catenin expression by using siRNA targeted for β-catenin significantly suppressed DCA-induced uPAR and cyclin D1 expression. On the basis of these data, they proposed the following sequence of events. Deoxycholic acid-induced tyrosine phosphorylation stabilizes and translocates β-catenin into the nucleus and stimulates uPA, uPAR, and cyclin D1 expression. Tyrosine phosphorylation dissociates β-catenin from E-cadherin and thus induces loss of cell adhesion. uPA/uPAR–mediated proteolytic degradation of extracellular matrix accompanied by cyclin D1/uPA/uPAR-induced cell proliferation enhances colon cancer growth and progression (Pai et al., 2004).
Previous studies have shown that low concentrations of DCA (< 50 μM) can increase the proliferation of colon cancer cells, while high concentrations of DCA (>100 μM) can increase apoptosis (Peiffer et al., 1997). Colon cancer cells treated with higher concentrations of DCA are sufficient to induce strong activation of EGFR-MAPK signaling (Qiao et al., 2000). Activation of the EGFR signaling pathway by secondary bile acids is achieved mainly by disturbing the structure of the cell membrane (reduced membrane fluidity, altered membrane cholesterol distribution), binding to natural ligands (e.g., epidermal growth factor), or inducing calcium signaling-mediated non-dependent activation of ligands. Activation of EGFR activates downstream MAPK/RAS/RAF/MEX/extracellular signal-regulated kinase/proto-oncogene activator protein-1, which, in turn, mediates cell proliferation and activates RAS/RAF1/extracellular signal-regulated kinase signaling pathway, leading to upregulation of mucin 2 and also activates the phosphatidylinositol 3 kinase/Akt signaling pathway, which regulates downstream target molecules such as caspase-8, leading to apoptosis (Centuori et al., 2016).
Another molecule with tumor-promoting activities in colon cells is cyclooxygenase 2 (COX-2). DCA leads to COX-2 activation and increased expression. Stimulation of COX-2 leads to increased PGE2 levels, another molecule whose expression is enhanced by the presence of DCA. Once PGE2 production is induced in the colon cells, it is able feedback in an autocrine fashion that stimulates a complex signaling cascade. This stimulation of signaling leads to increased cell survival, proliferation, angiogenesis, and resistance to apoptosis (Khare et al., 2008).
DCA has also been documented to decrease the levels of p53, a tumor suppressor that regulates cell cycle progression. The loss of p53 is an integral requirement for the development of many tumors including adenocarcinomas of the colon. A study has investigated the effect of bile acids on the tumor suppressor p53 using the human colon tumor cell line HCT116. They found that exposure of the cells to elevated concentrations of DCA suppressed the accumulation of p53 protein as well as p53 transactivation and impaired the p53 response of the cells to DNA-damaging agents, such as ionizing radiation. The study suggested that DCA suppressed p53 by stimulating the process of proteasome-mediated degradation of p53, in part, by stimulating the ERK signaling pathway (Qiao et al., 2001b).
Intestinal flora is involved in physiological, biochemical, and pathological processes of the body. It has the function of promoting immune regulation and intestinal nervous system development, preventing pathogen invasion, maintaining normal intestinal movement, and inhibiting tumor occurrence and development. Intestinal flora changes after cholecystectomy, but the specific mechanism is still unclear and needs to be explored through a large number of basic and clinical experimental studies. Based on the research at home and abroad, this study summarizes the current research in order to provide a new target for the treatment of gallbladder diseases.
The study showed that the gut microbiome of patients undergoing cholecystectomy is significantly different from that of healthy individuals but similar to that of patients with colorectal cancer, suggesting that changes in the gut microbiome of patients undergoing cholecystectomy may activate the onset and progression of colorectal cancer (Ren et al., 2020). Cholecystectomy causes dramatic changes in the intestinal microecology, including the composition and function of the intestinal microbiome. Previous studies confirmed that after cholecystectomy, the number of Bifidobacteria and Lactobacillus were significantly decreased, while the number of Enterococcus, Oscillospira, Escherichia coli, Bacteroidaceae, and Bacteroidetes were significantly increased (Jiang et al., 2022). At the phylum level, the abundance of Fusobacteria increased, whereas that of Proteobacteria decreased. Other phyla, including Bacteroidetes, Firmicutes, and Actinobacteria showed distinct variations in different studies. Interestingly, the changes in bacterial abundance of Firmicutes and Actinobacteria were similar in all studies, in contrast to the alteration in the bacterial abundance of Bacteroidetes. At the genus level, existing research has not reached a consensus. Genera reported with increasing abundance mainly include Anaerostipes, Dorea, Clostridium, Mogibacterium, Flavonifractor, Shigella, and Escherichia, while those with reduced abundance include Paraprevotella, Prevotella, Barnesiella, Alistipes, Faecalibacterium, Haemophilus, and Desulfovibrio. Few studies have focused on the species level; Blautia obeum, Veillonella parvula, Bacteroides ovatus, Parabacteroides distasonis, and Fusobacterium varium were found to increase, and Eubacterium rectale, Roseburia faecis, and Bifidobacterium adolescentis were reported to decrease (Ma et al., 2022). Ren et al. (2020) described the overall structure of bacterial microbiota by 16S rRNA gene sequencing, proving that bacterial ecological disorders after cholecystectomy are characterized by unique microbial composition and changes in the relative abundance of species with specific functions. Wang et al. (2012) and Wu et al. (2013) conducted a comparative analysis of fecal flora in colorectal cancer patients and healthy people in China using 16SrRNA pyrosequencing. The results showed that colorectal patients have a higher number of pathogenic bacteria compared with healthy people, especially Enterococcus, Shigella, Klebsiella, Streptococcus, and Streptococcus. In addition, evidence from foreign studies shows that the fecal abundance of Clostridium, Porphyrophyria, and Strangella in patients with colorectal cancer increases (Ahn et al., 2013). At present, most domestic scholars believe that intestinal Bifidobacterium and Lactobacillus are significantly reduced after cholecystectomy, while Escherichia coli and Enterococcus are significantly increased (Hongguang and Deqing, 2015).
Fibroblast growth factor (FGF) is a cytokine synthesized by terminal ileal epithelial cells, which is involved in regulating bile acid metabolism. FGF19 or FGF15 produced in the ileum is transported to the liver via the portal system to inhibit bile acid synthesis. It has been found that the mRNA content of FGF19 in gallbladder epithelial tissue is 250 times more in terminal ileal epithelial tissue. After cholecystectomy, with the decrease of FGF19 expression, the metabolic balance of bile acids is broken, and the production of primary bile acid increases, which changes the two-way interaction between bile acid and intestinal flora (Barrera et al., 2015; Keren et al., 2015).
Elevated deoxycholic acid (DCA) in the gut inhibits the growth of most Gram-negative anaerobic bacteria, such as Bacillus fragilis, Clostridium perfringens, Lactobacillus, Bifidobacterium, and Enterococcus (Floch et al., 1972). In addition, Cao et al. (2017) found that DCA significantly upregulated the populations of opportunistic pathogens, including Ruminococcus, Escherichia-Shigella, Desulfovibrio, and Dorea. Moreover, they also confirmed that DCA significantly increased the levels of Clostridium and Escherichia-Shigella but markedly decreased the abundance of Lactobacillus_gasseri and mostly butyrate-producing bacteria, such as Clostridium leptum Lachnospiraceae bacterium, and Eubacterium coprostanoligenes (Cao et al., 2017).
The mucosal epithelium of the gallbladder synthesizes a surfactant protein D, which is discharged into the intestinal cavity along with the bile to promote the synthesis of intestinal T cells. Intestinal T cells are involved in regulating inflammation in the gut. After cholecystectomy, the number of intestinal T cells decreases sharply due to the lack of gallbladder surface protein D, which is easy to cause intestinal bacterial infection, resulting in intestinal flora imbalance. Gallbladder surface protein D can also inhibit the growth of intestinal Lactobacillus by directly binding to Lactobacillus and causing its cleavage (Boehm et al., 2012; Sarashina-Kida et al., 2017).
The small intestine fluid is weakly alkaline with a pH of 8.0~9.0. Normal bile is weakly acidic, and its impulsive secretion is conducive to shaping a good intestinal microecological environment and maintaining the stability of intestinal pH value. After cholecystectomy, alkaline hepatic bile secrets continuously, which affects the balance of pH in the intestine. The optimum pH values of Lactobacillus and Bifidobacterium are 5.5~6.0 and 6.5~7.0, respectively. Therefore, the rise of pH in the intestine inhibits the growth of Lactobacillus, Bifidobacterium, and other beneficial bacteria, resulting in the imbalance of intestinal flora (Lin et al., 2018).
Intestinal microbiota has the potential to form an inflammatory microenvironment, and inflammation is a well-established risk factor for colorectal cancer. A study showed that specific components of the gut microbiome promote the development and progression of colorectal cancer through the activation of inflammation (Sears et al., 2014).
Intestinal homeostasis is achieved through an ongoing interaction between the gut microbiome and the host immune system. Once this balance is upset, a variety of diseases, such as inflammatory bowel disease, can arise as a result of immune system dysfunction (Gophna et al., 2006).
Another oncogenic mechanism of the gut microbiome is the production of genotoxins, which can cascade with intracellular signals or cause mutations by binding to specific cell surface receptors, which can also damage DNA (Valguarnera and Wardenburg, 2020). Interestingly, although many genotoxins can cause tumors, recent research has shown their potential use in cancer treatment (Zahaf and Schmidt, 2017).
Bacteria in the gut produce a range of metabolites, including secondary bile acids, sulfides, ammonia, nitrosamines, and short-chain fatty acids (SCFAs), which are involved in the development and progression of colorectal cancer. Dietary fiber is metabolized and broken down into SCFAs in the colon, of which butyrate (the most widely studied SCFA) regulates cell proliferation, apoptosis, and differentiation to inhibit colorectal tumors. Cholecystectomy greatly reduces the number of gut bacteria responsible for metabolizing butyrate. Therefore, the expression level of butyrate is decreased, and the occurrence of colorectal tumors is promoted (Ren et al., 2020). Gut microbiota can also disrupt mucus barrier function by producing sulfides, thus enhancing intestinal cell stimulation (Ijssennagger et al., 2015).
Multiple signaling pathways, such as epithelial growth factor receptor (EGFR), Wnt/beta-catenin, NF-κB, and transforming growth factor-β pathways, are involved in the development and progression of colorectal cancer. Notably, the gut microbiome activates the host carcinogenic signaling pathway (Ma et al., 2022).
It was reported that Enterotoxigenic Bacteroides fragilis(ETBF) secrete a zinc-dependent metalloprotease toxin termed the B. fragilis toxin (BFT). BFT rapidly cleaves the extracellular domain of E-cadherin, leading to the complete degradation of the E-cadherin protein, triggering β-catenin nuclear signaling in intestinal epithelial cells, and inducing c-Myc expression and cellular proliferation (Wu et al., 2003). Chung et al. constructed ApcMin mice colonized with an enterotoxigenic B. fragilis strain, possessing an in-frame chromosomal deletion of the bft gene. The results showed that B. fragilis stimulated intracellular IL-17 secretion to activate the NF-κB pathway, which, in turn, induced the expression of chemokines (CXCL1, CXCL2, and CXCL5) that collectively contributed to colonic carcinogenesis (Chung et al., 2018). The cytokine IL-8, a key downstream target gene of NF-κB, was also significantly increased in intestinal epithelial cells treated with active enterotoxigenic B. fragilis (Wu et al., 2004). The β-catenin and GSK3β cellular signaling pathways are involved in NF-κB activity and IL-8 expression in B. fragilis-infected cells (Jeon et al., 2019).
A growing body of evidence suggests a potential link between the Fusobacterium nucleatum (F. nucleatum) and colorectal carcinogenesis. Fusobacterium nucleatum is enriched in human colorectal adenomas and carcinomas compared with adjacent normal tissue (Kostic et al., 2013; Ito et al., 2015). A marked accumulation of Fusobacterium nucleatum, which expresses FadA adhesin on its surface, was found in patients with CRC. Fusobacterium nucleatum adheres to and invades the endothelial and epithelial cells through its unique FadA adhesin. FadA modulates E-cadherin and activates β-catenin signaling, leading to increased expression of transcription factors, oncogenes, Wnt genes, and inflammatory genes, as well as growth stimulation of CRC cells (Rubinstein et al., 2013). Fusobacterium nucleatum can also directly activate Toll-like receptor signaling to promote tumor development (To et al., 2015). It has been shown that F. nucleatum-mediated infection leads to a strong induction of TLR4/MYD88 and results in the sustained activation of NFκB. The hyperactive NFκB binds to the promoter of miR21 and increases its transcription level. MiR21 is a downstream target of F. nucleatum, and F. nucleatum regulates the expression of miR21 novel target RASA1 and activates the MAPK signaling pathway, consequently leading to the initiation of CRC (Yang et al., 2017).
The NF-κB family of transcriptional factors regulates a large number of genes involved in different cellular processes, such as cell proliferation, differentiation, genome stability, and innate immune and adaptive immune responses (Liu et al., 2017). The NF-κB signaling pathway can be activated to modulate host cellular events after exposure to different microbial pathogens or microbial products, such as lipopolysaccharide (LPS) and pathogen-associated molecular patterns (PAMPs) (Rahman and McFadden, 2011). Cytoplasmic NF-κB is transferred to the nucleus, where it induces antimicrobial inflammatory cytokine expression, which functions as a rapid defense mechanism against microbes, including infectious bacteria. However, prolonged chronic inflammation due to the activation of NF-κB proteins may result in tissue damage, further contributing to tumorigenesis by changing the genetic and epigenetic states of damaged tissues and the host microenvironment (Taniguchi and Karin, 2018). Fusobacterium nucleatum (F. nucleatum), Escherichia coli (E. coli), Peptostreptococcus anaerobius (P. anaerobius), and Enterotoxigenic Bacteroides fragilis (ETBF), which are enriched in patients with CRC, are involved in the modulation of this pathway (Peng et al., 2020). Enteropathogenic and enterohemorrhagic E. coli use a type III secretion system (T3SS) to transport dozens of effector proteins into host cells; these effector proteins, in turn, manipulate the host inflammatory response through activation of the NF-κB pathway (Litvak et al., 2017). Mechanistically, internalization of E. coli leads to the activation of NF-κB through increased phosphorylation of the NF-κB subunit RelA/p65 and IKKα, inactivation of IκBα, and induction of the Wnt/β-catenin pathway through the upregulation of β-catenin and its downstream genes. Then, NF-κB and Wnt/β-catenin synergistically promote tumorigenic stemness traits (Sahu et al., 2017). P. anaerobius interacts with TLR2 and TLR4 on colon cells to increase the levels of reactive oxidative species, which promotes cholesterol synthesis and cell proliferation (Tsoi et al., 2017). P. anaerobius adheres to the CRC mucosa and accelerates CRC development. A study identified a P. anaerobius surface protein, putative cell wall binding repeat 2 (PCWBR2), which directly interacts with colonic cell lines via α2/β1 integrin. Interaction between PCWBR2 and integrin α2/β1 induces the activation of the PI3K-Akt pathway in CRC cells via phospho-focal adhesion kinase, leading to increased cell proliferation and nuclear factor kappa-light-chain-enhancer of activated B cells (NF-κB) activation. NF-κB, in turn, triggers a pro-inflammatory response as indicated by increased levels of cytokines (Long et al., 2019).
Several specific and cumulative genetic changes occur during colorectal tumorigenesis, including mutation and activation of the cellular proto-oncogene Ki-ras and inactivation of tumor suppressor genes such as P53, hMSH2, APC, and DCC. If the above genetic alterations occur in colorectal epithelial cells (EC) in patients with cholelithiasis, the correlation between cholelithiasis and CRC can be evaluated in comparison with the healthy population without cholelithiasis (Werner et al., 1977).
The expression of Ki-67 in intestinal mucosa after cholecystectomy was significantly higher than that in non-cholecystectomy, indicating that intestinal mucosa was in a state of high proliferation after cholecystectomy (Jager et al., 2016).
The study showed that the probability of developing dMMR colorectal cancer after cholecystectomy was not significantly different from that of non-dMMR colorectal cancer. Therefore, the theory that cholecystectomy causes DNA mismatch repair dysfunction in colorectal cells still needs to be further confirmed by more studies (Skagen et al., 2009).
Gastrin increased significantly in blood circulation after cholecystectomy. Gastrin is a trophic growth factor of colon epithelial cells, which can activate a variety of signaling pathways, such as the HedgeHog signaling pathway, the Wnt signaling pathway, and the MAPK signaling pathway, and promote the occurrence and development of colorectal cancer (Redaelli et al., 2002; Wang et al., 2011).
Studies have shown that the concentration of cholecystokinin increases after cholecystectomy, which can be used as a ligand to activate the cholecystokinin II receptor (CCK2R). The upregulation of CCK2R can cause the transformation of human non-tumorigenic NCM356 colon epithelial cells into tumor-germinating cells (Chao et al., 2010).
Since gallstones are the main reason for cholecystectomy, some scholars believe that gallstones are a risk factor for colorectal cancer. A study which to systematically review and meta-analyze the association between the presence of gallstone disease (GD) or cholecystectomy (CE) and the incidence of colorectal cancer (CRC) showed that the overall association of GD and/or CE with CRC was RR = 1.15 (1.08; 1.24), primarily driven by hospital-based case-control studies [RR = 1.61 (1.29; 2.01)]. whereas a more modest association was found in population-based case-control and cohort studies [RR = 1.10 (1.02; 1.19)]. This study showed that gallstones were associated with a moderately increased risk of colon cancer (Polychronidis et al., 2023). Pinlian et al. (2012) believed that gallstones had adverse effects on the intestinal microenvironment and even led to precancerous lesions before cholecystectomy. Gallstones can cause inflammation of the gallbladder, bile ducts, liver, and pancreas, so the link between inflammation and cancer is well established, and as a result, gallstones can increase the risk of many different digestive cancers (Gosavi et al., 2017). Some scholars believe that the factors that can promote the formation of gallstones may also be the inducement of colorectal cancer, such as genetic predisposition, female sex, obesity, high fat, and low fiber diet (Völzke et al., 2005).
Recently, several studies have attempted to explore the causal relationship between cholecystectomy and colorectal cancer (CRC) from the genetic perspective. Studies have confirmed that gallstone disease is associated with an increased risk of CRC (Chen et al., 2023; Tsai et al., 2023). Chen et al. (2023) obtained genetic variants associated with cholecystectomy at a genome-wide significant level (P-value < 5 × 10–8) as instrumental variables (IVs) and performed Mendelian randomization (MR) to identify the complications of cholecystectomy. Furthermore, cholelithiasis was also treated as the exposure to compare its causal effects with that of cholecystectomy, and multivariable MR analysis was carried out to judge whether the effect of cholecystectomy was independent of cholelithiasis. They found that cholelithiasis could increase the risk of CRC in the largest population (OR = 1.041, 95% CI: 1.010–1.073). The multivariable MR analysis suggested that genetic liability to cholelithiasis could increase the risk of CRC in the largest population (OR = 1.061, 95% CI: 1.002–1.125), after adjustment of cholecystectomy. Another study came to a consistent conclusion, showing that cholelithiasis is associated with an increased risk of CRC, especially in the right-sided colon and among female patients. Cholecystitis and cholecystectomy may shift cancer to the distal part of the large bowel (Tsai et al., 2023).
However, research has found that increased production of bilirubin, the metabolic by-product of hemoglobin degradation, is also associated with gallstone disease (Kühn et al., 2017). This is intriguing as bilirubin has antioxidant and anti-inflammatory attributes, with experimental studies reporting mildly elevated levels being associated with decreased oxidative stress-related disease, including cancer (Vítek, 2012).
At present, the cholecystolithotomy technique of natural orifice transluminal endoscopic surgery is evolving, with some experts advocating gallbladder stone removal without gallbladder excision in order to preserve gallbladder function and eliminate post-cholecystectomy syndromes, including complications of the surgical incision, bile duct injury, functional gastrointestinal, and psychological conditions, and possibly an increase in colon cancer. In addition, transluminal endoscopic cholecystolithotomy is an option for elderly patients who are not suitable candidates for open surgery and those who desire scar-free minimally invasive surgery with organ preservation (Shang et al., 2022).
In summary, epidemiological studies on colorectal cancer and cholecystectomy have demonstrated the correlation between the two parameters, and most scholars support that cholecystectomy increases the risk of colorectal cancer. Therefore, patients after cholecystectomy should be followed up regularly to detect colorectal tumors early. In addition, since cholecystectomy may induce colorectal cancer, the pros and cons should be weighed when performing cholecystectomy, to reduce unnecessary cholecystectomy. The authors believe that with continuous research on the mechanism of the occurrence and development of colorectal cancer induced by cholecystectomy, new methods for targeted treatment of colorectal cancer may appear in the future.
ZY, JW, and XS conceived and designed the study. ZD, RS, and PL selected the studies, collected the data, and drafted the manuscript. FD, JZ, RW, ZL, and MD analyzed the data. All authors interpreted the results and revised the draft manuscript. All authors contributed to the article and approved the submitted version.
The authors declare that the research was conducted in the absence of any commercial or financial relationships that could be construed as a potential conflict of interest.
All claims expressed in this article are solely those of the authors and do not necessarily represent those of their affiliated organizations, or those of the publisher, the editors and the reviewers. Any product that may be evaluated in this article, or claim that may be made by its manufacturer, is not guaranteed or endorsed by the publisher.
Ábrahám, S., Németh, T., Benko, R., Matuz, M., Ottlakán, A., Váczi, D., et al. (2020). Evaluating the distribution of the locations of colorectal cancer after appendectomy and cholecystectomy. World J. Surg. Oncol. 18, 94. doi: 10.1186/s12957-020-01861-4
Ahn, J., Sinha, R., Pei, Z., Dominianni, C., Wu, J., Shi, J., et al. (2013). Human gut microbiome and risk for colorectal cancer. J. Natl. Cancer Inst. 105, 1907–1911. doi: 10.1093/jnci/djt300
Ajouz, H., Mukherji, D., and Shamseddine, A. (2014). Secondary bile acids: an underrecognized cause of colon cancer. World J. Surg. Oncol. 12, 164. doi: 10.1186/1477-7819-12-164
Altieri, A., Pelucchi, C., Talamini, R., Bosetti, C., Franceschi, S., La Vecchia, C., et al. (2004). Cholecystectomy and the risk of colorectal cancer in Italy. Br. J. Cancer. 90, 1753–1755. doi: 10.1038/sj.bjc.6601721
Barrera, F., Azócar, L., Molina, H., Schalper, K. A., Ocares, M., Liberona, J., et al. (2015). Effect of cholecystectomy on bile acid synthesis and circulating levels of fibroblast growth factor 19. Ann. Hepatol. 14, 710–721. doi: 10.1016/S1665-2681(19)30766-5
Bayerdörffer, E., Mannes, G. A., Richter, W. O., Ochsenkühn, T., Wiebecke, B., Köpcke, W., et al. (1993). Increased serum deoxycholic acid levels in men with colorectal adenomas. Gastroenterology.104, 145–151. doi: 10.1016/0016-5085(93)90846-5
Bingfeng, Z., Zexu, C., Qi, Z., and Jiansheng, L. (2020). Research advances in bile acid metabolism after cholecystectomy and its mechanism of inducing colorectal cancer. Chinese J. Digestive Surg. 19, 559–562. doi: 10.3760/cma.j.cn115610-20200419-00264
Bo, Y., Yanyong, J., Yinqi, X., and Yongdong, P. (2002). Effect of cholecystectomy on the induction of large intestine cancer by dimethylhydrazine in mice. Chinese J. Bases Clinics General Surg. 9, 19–22. doi: 10.3969/j.issn.1007-9424.2002.01.008
Boehm, F., Martin, M., Kesselring, R., Schiechl, G., Geissler, E. K., Schlitt, H. J., et al. (2012). Deletion of Foxp3+ regulatory T cells in genetically targeted mice supports development of intestinal inflammation. BMC Gastroenterol. 12, 97. doi: 10.1186/1471-230X-12-97
Bundgaard-Nielsen, C., Baandrup, U. T., Nielsen, L. P., and Sørensen, S. (2019). The presence of bacteria varies between colorectal adenocarcinomas, precursor lesions and non-malignant tissue. BMC Cancer. 19, 399. doi: 10.1186/s12885-019-5571-y
Cao, H., Xu, M., Dong, W., Deng, B., Wang, S., Zhang, Y., et al. (2017). Secondary bile acid-induced dysbiosis promotes intestinal carcinogenesis. Int. J. Cancer. 140, 2545–2556. doi: 10.1002/ijc.30643
Centuori, S. M., Gomes, C. J., Trujillo, J., Borg, J., Brownlee, J., Putnam, C. W., et al. (2016). Deoxycholic acid mediates non-canonical EGFR-MAPK activation through the induction of calcium signaling in colon cancer cells. Biochim. Biophys. Acta. 1861, 663–670. doi: 10.1016/j.bbalip.2016.04.006
Centuori, S. M., and Martinez, J. D. (2014). Differential regulation of EGFR-MAPK signaling by deoxycholic acid (DCA) and ursodeoxycholic acid (UDCA) in colon cancer. Dig. Dis. Sci. 59, 2367–2380. doi: 10.1007/s10620-014-3190-7
Chao, C., Han, X., Ives, K., Park, J., Kolokoltsov, A. A., Davey, R. A., et al. (2010). CCK2 receptor expression transforms non-tumorigenic human NCM356 colonic epithelial cells into tumor forming cells. Int. J. Cancer. 126, 864–875. doi: 10.1002/ijc.24845
Chattopadhyay, K., and Das, R. (2020). Laparoscopic and open cholecystectomy: A comparative study. Int. J. Surg Sci. 4, 427–430. doi: 10.33545/surgery.2020.v4.i1h.375
Chen, C. H., Lin, C. L., and Kao, C. H. (2020). The effect of cholecystectomy on the risk of colorectal cancer in patients with gallbladder stones. Cancers (Basel). 12, 550. doi: 10.3390/cancers12030550
Chen, L., Fan, Z., Sun, X., Qiu, W., Mu, W., Chai, K., et al. (2023). Associations of cholecystectomy with the risk of colorectal cancer: a Mendelian randomization study. Chin. Med. J. (Engl). 136, 840–847. doi: 10.1097/CM9.0000000000002612
Chen, Y. K., Yeh, J. H., Lin, C. L., Peng, C. L., Sung, F. C., Hwang, I. M., et al. (2014). Cancer risk in patients with cholelithiasis and after cholecystectomy: a nationwide cohort study. J. Gastroenterol. 49, 923–931. doi: 10.1007/s00535-013-0846-6
Chiong, C., Cox, M. R., and Eslick, G. D. (2012). Gallstones are associated with colonic adenoma: a meta-analysis. World J. Surg. 36, 2202–2209. doi: 10.1007/s00268-012-1646-5
Cho, Y. A., Lee, J., Oh, J. H., Chang, H. J., Sohn, D. K., Shin, A., et al. (2019). Genetic risk score, combined lifestyle factors and risk of colorectal cancer. Cancer Res. Treat. 51, 1033–1040. doi: 10.4143/crt.2018.447
Chung, L., Thiele Orberg, E., Geis, A. L., Chan, J. L., Fu, K., DeStefano Shields, C. E., et al. (2018). Bacteroides fragilis toxin coordinates a pro-carcinogenic inflammatory cascade via targeting of colonic epithelial cells. Cell Host. Microbe. 23, 203–214.e5. doi: 10.1016/j.chom.2018.01.007
Chunyang, H., Jie, C., Yanmin, L., and Jin, S. (2019). Role of intestinal flora in the development/progression and treatment of autoimmune liver diseases. J. Clin. Hepatol. 35, 205–207. doi: 10.3969/j.issn.1001-5256.2019.01.045
Collins, S. L., Stine, J. G., Bisanz, J. E., Okafor, C. D., and Patterson, A. D. (2023). Bile acids and the gut microbiota: metabolic interactions and impacts on disease. Nat. Rev. Microbiol. 21, 236–247. doi: 10.1038/s41579-022-00805-x
De Almeida, C. V., de Camargo, M. R., Russo, E., and Amedei, A. (2019). Role of diet and gut microbiota on colorectal cancer immunomodulation. World J. Gastroenterol. 25, 151–162. doi: 10.3748/wjg.v25.i2.151
Degirolamo, C., Modica, S., Palasciano, G., and Moschetta, A. (2011). Bile acids and colon cancer: Solving the puzzle with nuclear receptors. Trends Mol. Med. 17, 564–572. doi: 10.1016/j.molmed.2011.05.010
Floch, M. H., Binder, H. J., Filburn, B., and Gershengoren, W. (1972). The effect of bile acids on intestinal microflora. Am. J. Clin. Nutr. 25, 1418–1426. doi: 10.1093/ajcn/25.12.1418
Giovannucci, E., Colditz, G. A., and Stampfer, M. J. (1993). A meta-analysis of cholecystectomy and risk of colorectal cancer. Gastroenterology. 105, 130–141. doi: 10.1016/0016-5085(93)90018-8
Goldacre, M. J., Wotton, C. J., Abisgold, J., Yeates, D. G., and Collins, J. (2012). Association between cholecystectomy and intestinal cancer: a national record linkage study. Ann. Surg. 256, 1068–1072. doi: 10.1097/SLA.0b013e3182759efb
Gophna, U., Sommerfeld, K., Gophna, S., Doolittle, W. F., and Veldhuyzen van Zanten, S. J. (2006). Differences between tissue-associated intestinal microfloras of patients with Crohn's disease and ulcerative colitis. J. Clin. Microbiol. 44, 4136–4141. doi: 10.1128/JCM.01004-06
Gosavi, S., Mishra, R. R., and Kumar, V. P. (2017). Study on the relation between colorectal cancer and gall bladder disease. J. Clin. Diagn Res. 11, OC25–OC27. doi: 10.7860/JCDR/2017/22954.9485
Han, Y., Liu, Y., and Qi, F. (2012). Meta-Analysis on Correlation of Cholecystolithiasis or Cholecystectomy with Colorectal Neoplasm. Chinese J. Surg. Integr. Tradit. Western Med. 18, 17–20. doi: 10.3969/j.issn.1007-6948.2012.01.005
Harrison, E. M., O'Neill, S., Meurs, T. S., Wong, P. L., and Duxbury, M. (2012). Paterson-Brown S, et al. Hospital volume and patient outcomes after cholecystectomy in Scotland: retrospective, national population based study. BMJ. 344, e3330. doi: 10.1136/bmj.e3330
Hongguang, Q., and Deqing, Y. (2015). Clinical observation of cholecystectomy patients with intestinal microflora changes. Chinese J. Gastroesophageal Reflux Dis. 2, 99–101. doi: 10.3877/cma.j.issn.1674-6899.2015.02.011
Housset, C., Chrétien, Y., Debray, D., and Chignard, N. (2016). Functions of the Gallbladder. Compr. Physiol. 6, 1549–1577. doi: 10.1002/cphy.c150050
Hylemon, P. B., Zhou, H., Pandak, W. M., Ren, S., Gil, G., Dent, P., et al. (2009). Bile acids as regulatory molecules. J. Lipid Res. 50, 1509–1520. doi: 10.1194/jlr.R900007-JLR200
Ijssennagger, N., Belzer, C., Hooiveld, G. J., Dekker, J., van Mil, S. W., Müller, M., et al. (2015). Gut microbiota facilitates dietary heme-induced epithelial hyperproliferation by opening the mucus barrier in colon. Proc. Natl. Acad. Sci. U S A. 112, 10038–10043. doi: 10.1073/pnas.1507645112
Islam, M. R., Ali, M. S., Azam, S. G., and Islam, M. R. (2021). Comparative study between laparoscopic and open cholecystectomy: complications and management. Med. Today. 52, 19–21. doi: 10.3329/medtoday.v33i01.52152
Ito, M., Kanno, S., Nosho, K., Sukawa, Y., Mitsuhashi, K., Kurihara, H., et al. (2015). Association of Fusobacterium nucleatum with clinical and molecular features in colorectal serrated pathway. Int. J. Cancer. 137, 1258–1268. doi: 10.1002/ijc.29488
Jager, J., Aparicio-Vergara, M., and Aouadi, M. (2016). Liver innate immune cells and insulin resistance: the multiple facets of Kupffer cells. J. Intern. Med. 280, 209–220. doi: 10.1111/joim.12483
Jeon, J. I., Ko, S. H., and Kim, J. M. (2019). Intestinal epithelial cells exposed to bacteroides fragilis enterotoxin regulate Nf-κb activation and inflammatory responses through β-catenin expression. Infect. Immun. 87, e00312–e00319. doi: 10.1128/IAI.00312-19
Jiang, X., Jiang, Z., Cheng, Q., Sun, W., Jiang, M., Sun, Y., et al. (2022). Cholecystectomy promotes the development of colorectal cancer by the alternation of bile acid metabolism and the gut microbiota. Front. Med. 9, 1000563. doi: 10.3389/fmed.2022.1000563
Kaibara, N., Wakatsuki, T., Mizusawa, K., Sugesawa, A., Kimura, O., Koga, S., et al. (1986). Negative correlation between cholecystectomy and the subsequent development of large bowel carcinoma in a low-risk Japanese population. Dis. Colon. Rectum. 29, 644–646. doi: 10.1007/BF02560328
Keren, N., Konikoff, F. M., Paitan, Y., Gabay, G., Reshef, L., Naftali, T., et al. (2015). Interactions between the intestinal microbiota and bile acids in gallstones patients. Environ. Microbiol. Rep. 7, 874–880. doi: 10.1111/1758-2229.12319
Khare, S., Mustafi, R., Cerda, S., Yuan, W., Jagadeeswaran, S., Dougherty, U., et al. (2008). Ursodeoxycholic acid suppresses Cox-2 expression in colon cancer: roles of Ras, p38, and CCAAT/enhancer-binding protein. Nutr. Cancer. 60, 389–400. doi: 10.1080/01635580701883003
Kim, S. B., Kim, K. O., and Kim, T. N. (2020). Prevalence and risk factors of gastric and colorectal cancer after cholecystectomy. J. Korean Med. Sci. 35, e354. doi: 10.3346/jkms.2020.35.e354
Kostic, A. D., Chun, E., Robertson, L., Glickman, J. N., Gallini, C. A., Michaud, M., et al. (2013). Fusobacterium nucleatum potentiates intestinal tumorigenesis and modulates the tumor-immune microenvironment. Cell Host Microbe. 14, 207–215. doi: 10.1016/j.chom.2013.07.007
Kühn, T., Sookthai, D., Graf, M. E., Schübel, R., Freisling, H., Johnson, T., et al. (2017). Albumin, bilirubin, uric acid and cancer risk: results from a prospective population-based study. Br. J. Cancer. 117, 1572–1579. doi: 10.1038/bjc.2017.313
Kullak-Ublick, G. A., Paumgartner, G., and Berr, F. (1995). Long-term effects of cholecystectomy on bile acid metabolism. Hepatology. 21, 41–45. doi: 10.1002/hep.1840210109
Kuniyasu, T., Tanaka, T., Shima, H., Sugie, S., Mori, H., Takahashi, M., et al. (1986). Enhancing effect of cholecystectomy on colon carcinogenesis induced by methylazoxymethanol acetate in hamsters. Dis. Colon. Rectum. 29, 492–494. doi: 10.1007/BF02562600
Lee, J., Choe, S., Park, J. W., Jeong, S. Y., and Shin, A. (2018). The risk of colorectal cancer after cholecystectomy or appendectomy: a population-based cohort study in Korea. J. Prev Med. Public Health. 51, 281–288. doi: 10.3961/jpmph.18.105
Lin, W., Feng, Z., Changsheng, Y., Dongxiao, L., Yongwei, W., and Xiangsong, W. (2018). Research progress of gut microbiota dysbiosis after cholecystectomy. Chinese J. Digestive Surg. 17, 321–324. doi: 10.3760/cma.j.issn.1673-9752.2018.03.019
Litvak, Y., Sharon, S., Hyams, M., Zhang, L., Kobi, S., Katsowich, N., et al. (2017). Epithelial cells detect functional type III secretion system of enteropathogenic Escherichia coli through a novel NF-κB signaling pathway. PLoS Pathog. 13, e1006472. doi: 10.1371/journal.ppat.1006472
Liu, T., Zhang, L., Joo, D., and Sun, S. C. (2017). NF-κB signaling in inflammation. Signal Transduct. Target Ther. 2, 17023. doi: 10.1038/sigtrans.2017.23
Logan, C. Y., and Nusse, R. (2004). The Wnt signaling pathway in development and disease. Annu. Rev. Cell Dev. Biol. 20, 781–810. doi: 10.1146/annurev.cellbio.20.010403.113126
Long, X., Wong, C. C., Tong, L., Chu, E. S. H., Ho Szeto, C., Go, M. Y. Y., et al. (2019). Peptostreptococcus anaerobius promotes colorectal carcinogenesis and modulates tumour immunity. Nat. Microbiol. 4, 2319–2330. doi: 10.1038/s41564-019-0541-3
Ma, Y., Qu, R., Zhang, Y., Jiang, C., Zhang, Z., Fu, W., et al. (2022). Progress in the Study of Colorectal Cancer Caused by Altered Gut Microbiota After Cholecystectomy. Front. Endocrinol. 13, 815999. doi: 10.3389/fendo.2022.815999
Mándi, M., Keleti, G., and Juhász, M. (2021). The role of appendectomy and cholecystectomy in the pathogenesis of colorectal carcinomas. Ann. Med. Surg. 72, 102991. doi: 10.1016/j.amsu.2021.102991
Niekamp, P., and Kim, C. H. (2023). Microbial metabolite dysbiosis and colorectal cancer. Gut Liver. 17, 190–203. doi: 10.5009/gnl220260
Pai, R., Tarnawski, A. S., and Tran, T. (2004). Deoxycholic acid activates beta-catenin signaling pathway and increases colon cell cancer growth and invasiveness. Mol. Biol. Cell. 15, 2156–2163. doi: 10.1091/mbc.e03-12-0894
Payne, C. M., Bernstein, C., Dvorak, K., and Bernstein, H. (2008). Hydrophobic bile acids, genomic instability, Darwinian selection, and colon carcinogenesis. Clin. Exp. Gastroenterol. 1, 19–47. doi: 10.2147/CEG.S4343
Peiffer, L. P., Peters, D. J., and McGarrity, T. J. (1997). Differential effects of deoxycholic acid on proliferation of neoplastic and differentiated colonocytes in vitro. Dig. Dis. Sci. 42, 2234–2240. doi: 10.1023/A:1018806431866
Peng, C., Ouyang, Y., Lu, N., and Li, N. (2020). The NF-κB Signaling pathway, the microbiota, and gastrointestinal tumorigenesis: recent advances. Front. Immunol. 11, 1387. doi: 10.3389/fimmu.2020.01387
Pinlian, H., Xizhi, L., and Zhaosen, C. (2012). Correlation analysis of cholecystolithiasis and cholecystectomy with colorectal Carcinoma. Nat. Med. Front. China. 7, 31–32. doi: 10.3969/j.issn.1673-5552.2012.10.0022
Polychronidis, G., Siddiqi, H., Ali Ahmed, F., Papatheodorou, S., Giovannucci, E. L., Song, M., et al. (2023). Association of gallstone disease with risk of colorectal cancer: a systematic review and meta-analysis of observational studies. Int. J. Epidemiol. 18, dyad042. doi: 10.1093/ije/dyad042
Qi, L., Tian, Y., and Chen, Y. (2019). Gall bladder: The metabolic orchestrator. Diab. Metab. Res. Rev. 35, e3140. doi: 10.1002/dmrr.3140
Qiao, D., Chen, W., Stratagoules, E. D., and Martinez, J. D. (2000). Bile acid-induced activation of activator protein-1 requires both extracellular signal-regulated kinase and protein kinase C signaling. J. Biol. Chem. 275, 15090–15098. doi: 10.1074/jbc.M908890199
Qiao, D., Gaitonde, S. V., Qi, W., and Martinez, J. D. (2001b). Deoxycholic acid suppresses p53 by stimulating proteasome-mediated p53 protein degradation. Carcinogenesis. 22, 957–964. doi: 10.1093/carcin/22.6.957
Qiao, D., Stratagouleas, E. D., and Martinez, J. D. (2001a). Activation and role of mitogen-activated protein kinases in deoxycholic acid-induced apoptosis. Carcinogenesis. 22, 35–41. doi: 10.1093/carcin/22.1.35
Rahman, M. M., and McFadden, G. (2011). Modulation of NF-κB signalling by microbial pathogens. Nat. Rev. Microbiol. 9, 291–306. doi: 10.1038/nrmicro2539
Redaelli, C. A., Tian, Y. H., Schaffner, T., Ledermann, M., Baer, H. U., Dufour, J. F., et al. (2002). Extended preservation of rat liver graft by induction of heme oxygenase-1. Hepatology. 35, 1082–1092. doi: 10.1053/jhep.2002.33067
Ren, X., Xu, J., Zhang, Y., Chen, G., Zhang, Y., Huang, Q., et al. (2020). Bacterial alterations in post-cholecystectomy patients are associated with colorectal cancer. Front. Oncol. 10, 1418. doi: 10.3389/fonc.2020.01418
Roda, E., Aldini, R., Mazzella, G., Roda, A., Sama, C., Festi, D., et al. (1978). Enterohepatic circulation of bile acids after cholecystectomy. Gut. 19, 640–649. doi: 10.1136/gut.19.7.640
Rubinstein, M. R., Wang, X., Liu, W., Hao, Y., Cai, G., Han, Y. W., et al. (2013). Fusobacterium nucleatum promotes colorectal carcinogenesis by modulating E-cadherin/β-catenin signaling via its FadA adhesin. Cell Host Microbe. 14, 195–206. doi: 10.1016/j.chom.2013.07.012
Sagawa, H., Tazuma, S., and Kajiyama, G. (1993). Protection against hydrophobic bile salt-induced cell membrane damage by liposomes and hydrophilic bile salts. Am. J. Physiol. 264, G835–G839. doi: 10.1152/ajpgi.1993.264.5.G835
Sahu, U., Choudhury, A., Parvez, S., Biswas, S., and Kar, S. (2017). Induction of intestinal stemness and tumorigenicity by aberrant internalization of commensal non-pathogenic E. coli. Cell Death Dis. 8, e2667. doi: 10.1038/cddis.2017.27
Saklayen, M. G. (2018). The global epidemic of the metabolic syndrome. Curr. Hypertens Rep. 20, 12. doi: 10.1007/s11906-018-0812-z
Sakorafas, G. H., Milingos, D., and Peros, G. (2007). Asymptomatic cholelithiasis: is cholecystectomy really needed? A critical reappraisal 15 years after the introduction of laparoscopic cholecystectomy. Dig. Dis. Sci. 52, 1313–1325. doi: 10.1007/s10620-006-9107-3
Sarashina-Kida, H., Negishi, H., Nishio, J., Suda, W., Nakajima, Y., Yasui-Kato, M., et al. (2017). Gallbladder-derived surfactant protein D regulates gut commensal bacteria for maintaining intestinal homeostasis. Proc. Natl. Acad. Sci. U S A. 114, 10178–10183. doi: 10.1073/pnas.1712837114
Saraste, D., Järås, J., and Martling, A. (2020). Population-based analysis of outcomes with early-age colorectal cancer. Br. J. Surg. 107, 301–309. doi: 10.1002/bjs.11333
Schernhammer, E. S., Leitzmann, M. F., Michaud, D. S., Speizer, F. E., Giovannucci, E., Colditz, G. A., et al. (2003). Cholecystectomy and the risk for developing colorectal cancer and distal colorectal adenomas. Br. J. Cancer. 88, 79–83. doi: 10.1038/sj.bjc.6600661
Sears, C. L., Geis, A. L., and Housseau, F. (2014). Bacteroides fragilis subverts mucosal biology: from symbiont to colon carcinogenesis. J. Clin. Invest. 124, 4166–4172. doi: 10.1172/JCI72334
Sergeev, I. N., Aljutaily, T., Walton, G., and Huarte, E. (2020). Effects of synbiotic supplement on human gut microbiota, body composition and weight loss in obesity. Nutrients. 12, 222. doi: 10.3390/nu12010222
Shabanzadeh, D. M. (2018). Incidence of gallstone disease and complications. Curr. Opin. Gastroenterol. 34, 81–89. doi: 10.1097/MOG.0000000000000418
Shang, L., Shen, X., Niu, W., Zhang, Y., Han, J., Liu, H., et al. (2022). Update on the natural orifice transluminal endoscopic surgery for gallbladder preserving gallstones therapy: A review. Medicine. 101, e31810. doi: 10.1097/MD.0000000000031810
Shao, T., and Yang, Y. X. (2005). Cholecystectomy and the risk of colorectal cancer. Am. J. Gastroenterol. 100, 1813–1820. doi: 10.1111/j.1572-0241.2005.41610.x
Sicklick, J. K., Camp, M. S., Lillemoe, K. D., Melton, G. B., Yeo, C. J., Campbell, K. A., et al. (2005). Surgical management of bile duct injuries sustained during laparoscopic cholecystectomy: perioperative results in 200 patients. Ann. Surg. 241, 786–792. doi: 10.1097/01.sla.0000161029.27410.71
Skagen, C., Lucey, M., and Said, A. (2009). Liver transplantation: an update 2009. Curr. Opin. Gastroenterol. 25, 202–208. doi: 10.1097/MOG.0b013e3283299652
Song, M., and Chan, A. T. (2019). Environmental factors, gut microbiota, and colorectal cancer prevention. Clin. Gastroenterol. Hepatol. 17, 275–289. doi: 10.1016/j.cgh.2018.07.012
Stenman, L. K., Holma, R., and Korpela, R. (2012). High-fat-induced intestinal permeability dysfunction associated with altered fecal bile acids. World J. Gastroenterol. 18, 923–929. doi: 10.3748/wjg.v18.i9.923
Sung, H., Ferlay, J., Siegel, R. L., Laversanne, M., Soerjomataram, I., Jemal, A., et al. (2021). Global Cancer statistics 2020: globocan estimates of incidence and mortality worldwide for 36 cancers in 185 countries. CA Cancer J. Clin. 71, 209–249. doi: 10.3322/caac.21660
Taniguchi, K., and Karin, M. (2018). NF-κB, inflammation, immunity and cancer: coming of age. Nat. Rev. Immunol. 18, 309–324. doi: 10.1038/nri.2017.142
Tiderington, E., Lee, S. P., and Ko, C. W. (2016). Gallstones: new insights into an old story. F1000Res. 5, F1000. doi: 10.12688/f1000research.8874.1
To, T. T., Güm,üş, P., Nizam, N., Buduneli, N., and Darveau, R. P. (2015). Subgingival plaque in periodontal health antagonizes at toll-like receptor 4 and inhibits e-selectin expression on endothelial cells. Infect. Immun. 84, 120–126. doi: 10.1128/IAI.00693-15
Tsai, C. J., Leitzmann, M. F., Willett, W. C., and Giovannucci, E. L. (2008). Macronutrients and insulin resistance in cholesterol gallstone disease. Am. J. Gastroenterol. 103, 2932–2939. doi: 10.1111/j.1572-0241.2008.02189.x
Tsai, P., Lin, Y., Chen, J., Wu, S., Chiang, M., and Chen, M. (2023). Cholelithiasis, cholecystitis, cholecystectomy and the associated risk of colorectal cancer. Formosan J. Surg. 9, 47. doi: 10.1097/FS9.0000000000000047
Tsoi, H., Chu, E. S. H., Zhang, X., Sheng, J., Nakatsu, G., Ng, S. C., et al. (2017). Peptostreptococcus anaerobius induces intracellular cholesterol biosynthesis in colon cells to induce proliferation and causes dysplasia in mice. Gastroenterology. 152, 1419–1433.e5. doi: 10.1053/j.gastro.2017.01.009
Uldall Torp, N. M., Kristensen, S. B., Mortensen, F. V., and Kirkegård, J. (2020). Cholecystitis and risk of pancreatic, liver, and biliary tract cancer in patients undergoing cholecystectomy. HPB. 22, 1258–1264. doi: 10.1016/j.hpb.2019.11.012
Valguarnera, E., and Wardenburg, J. B. (2020). Good gone bad: one toxin away from disease for bacteroides fragilis. J. Mol Biol. 432, 765–785. doi: 10.1016/j.jmb.2019.12.003
Vinikoor, L. C., Robertson, D. J., Baron, J. A., Silverman, W. B., and Sandler, R. S. (2007). Cholecystectomy and the risk of recurrent colorectal adenomas. Cancer Epidemiol. Biomarkers Prev. 16, 1523–1525. doi: 10.1158/1055-9965.EPI-07-0243
Vítek, L. (2012). The role of bilirubin in diabetes, metabolic syndrome, and cardiovascular diseases. Front. Pharmacol. 3, 55. doi: 10.3389/fphar.2012.00055
Völzke, H., Baumeister, S. E., Alte, D., Hoffmann, W., Schwahn, C., Simon, P., et al. (2005). Independent risk factors for gallstone formation in a region with high cholelithiasis prevalence. Digestion. 71, 97–105. doi: 10.1159/000084525
Wang, C. F., Wang, Z. Y., and Li, J. Y. (2011). Dual protective role of HO-1 in transplanted liver grafts: a review of experimental and clinical studies. World J. Gastroenterol. 17, 3101–3108. doi: 10.3748/wjg.v17.i26.3101
Wang, T., Cai, G., Qiu, Y., Fei, N., Zhang, M., Pang, X., et al. (2012). Structural segregation of gut microbiota between colorectal cancer patients and healthy volunteers. ISME J. 6, 320–329. doi: 10.1038/ismej.2011.109
Wang, W., Wang, J., Li, J., Yan, P., Jin, Y., Zhang, R., et al. (2018). Cholecystectomy Damages Aging-Associated Intestinal Microbiota Construction. Front. Microbiol. 9, 1402. doi: 10.3389/fmicb.2018.01402
Werner, B., de Heer, K., and Mitschke, H. (1977). Cholecystectomy and carcinoma of the colon. An experimental study. Z Krebsforsch Klin Onkol. Cancer Res. Clin. Oncol. 88, 223–230. doi: 10.1007/BF00305360
Wong, N. A., and Pignatelli, M. (2002). Beta-catenin-a linchpin in colorectal carcinogenesis? Am. J. Pathol. 160, 389–401. doi: 10.1016/S0002-9440(10)64856-0
Wu, N., Yang, X., Zhang, R., Li, J., Xiao, X., Hu, Y., et al. (2013). Dysbiosis signature of fecal microbiota in colorectal cancer patients. Microb. Ecol. 66, 462–470. doi: 10.1007/s00248-013-0245-9
Wu, S., Morin, P. J., Maouyo, D., and Sears, C. L. (2003). Bacteroides fragilis enterotoxin induces c-Myc expression and cellular proliferation. Gastroenterology. 124, 392–400. doi: 10.1053/gast.2003.50047
Wu, S., Powell, J., Mathioudakis, N., Kane, S., Fernandez, E., Sears, C. L., et al. (2004). Bacteroides fragilis enterotoxin induces intestinal epithelial cell secretion of interleukin-8 through mitogen-activated protein kinases and a tyrosine kinase-regulated nuclear factor-kappaB pathway. Infect. Immun. 72, 5832–5839. doi: 10.1128/IAI.72.10.5832-5839.2004
Xu, Y. K., Zhang, F. L., Feng, T., Li, J., and Wang, Y. H. (2009). Meta-analysis on the correlation of cholecystectomy or cholecystolithiasis to risk of colorectal cancer in Chinese population. Ai Zheng. 28, 749–755. doi: 10.5732/cjc.008.10829
Yang, Y., Weng, W., Peng, J., Hong, L., Yang, L., Toiyama, Y., et al. (2017). Fusobacterium nucleatum increases proliferation of colorectal cancer cells and tumor development in mice by activating toll-like receptor 4 signaling to nuclear factor-κB, and Up-regulating Expression of MicroRNA-21. Gastroenterology. 152, 851–866.e24. doi: 10.1053/j.gastro.2016.11.018
Yao, Y., Li, X., Xu, B., Luo, L., Guo, Q., Wang, X., et al. (2022). Cholecystectomy promotes colon carcinogenesis by activating the Wnt signaling pathway by increasing the deoxycholic acid level. Cell Commun. Signal. 20, 71. doi: 10.1186/s12964-022-00890-8
Yao, Z. (2018). Mechanisms of deoxycholic acid to promote the initiation and progression of colorectal cancer. J. Med. Postgraduates.31, 878–881. doi: 10.16571/j.cnki.1008-8199.2018.08.020
Zahaf, N. I., and Schmidt, G. (2017). Bacterial Toxins for Cancer Therapy. Toxins (Basel). 9, 236. doi: 10.3390/toxins9080236
Zhai, X., Yang, Y., Wang, W., and Geng, J. (2019). Relationship between cholecystectomy and colorectal cancer. Chinese J. Digest. 39, 715–717. doi: 10.3760/cma.j.issn.0254-1432.2019.10.019
Zhang, F., Qin, H., Zhao, Y., Wei, Y., Xi, L., Rao, Z., et al. (2017b). Effect of cholecystectomy on bile acids as well as relevant enzymes and transporters in mice: Implication for pharmacokinetic changes of rifampicin. Eur. J. Pharm Sci. 96, 141–153. doi: 10.1016/j.ejps.2016.09.006
Zhang, W., Sun, Y., and Tian, Z. (2021). Research progress on the relationship between cholecystectomy and colorectal cancer. J. Hepatopancreatobiliary Surg. 33, 53–55. doi: 10.11952/j.issn.1007-1954.2021.01.013
Zhang, Y., Liu, H., Li, L., Ai, M., Gong, Z., He, Y., et al. (2017a). Cholecystectomy can increase the risk of colorectal cancer: A meta-analysis of 10 cohort studies. PLoS ONE. 12, e0181852. doi: 10.1371/journal.pone.0181852
Zhao, C., Ge, Z., Wang, Y., and Qian, J. (2012). Meta-analysis of observational studies on cholecystectomy and the risk of colorectal adenoma. Eur. J. Gastroenterol. Hepatol. 24, 375–381. doi: 10.1097/MEG.0b013e328350f86b
Zhao, Y., Zhang, S., Su, T., and Cui, N. (2022). Research progress on the incidence of colorectal cancer and changes in intestinal environment after cholecystectomy. Chin. J. Surg. Integr. Tradit. Western Med. 28, 269–272. doi: 10.3969/j.issn.1007-6948.2022.02.024
Zuccato, E., Venturi, M., Di Leo, G., Colombo, L., Bertolo, C., Doldi, S. B., et al. (1993). Role of bile acids and metabolic activity of colonic bacteria in increased risk of colon cancer after cholecystectomy. Dig. Dis. Sci. 38, 514–519. doi: 10.1007/BF01316508
Keywords: cholecystectomy, colorectal cancer, bile acid, intestinal flora, microbiome
Citation: Dong Z, Shi R, Li P, Song X, Dong F, Zhu J, Wu R, Liang Z, Du M, Wang J and Yang Z (2023) Does postcholecystectomy increase the risk of colorectal cancer? Front. Microbiol. 14:1194419. doi: 10.3389/fmicb.2023.1194419
Received: 27 March 2023; Accepted: 31 May 2023;
Published: 22 June 2023.
Edited by:
Khalid Mehmood, Islamia University of Bahawalpur, PakistanReviewed by:
Qiuhe Lu, Cleveland Clinic, United StatesCopyright © 2023 Dong, Shi, Li, Song, Dong, Zhu, Wu, Liang, Du, Wang and Yang. This is an open-access article distributed under the terms of the Creative Commons Attribution License (CC BY). The use, distribution or reproduction in other forums is permitted, provided the original author(s) and the copyright owner(s) are credited and that the original publication in this journal is cited, in accordance with accepted academic practice. No use, distribution or reproduction is permitted which does not comply with these terms.
*Correspondence: Jijun Wang, d2FuZ2ppanVuMjAwNEBzaW5hLmNvbQ==; Zhigang Yang, MTk3MXlhbmd6aGlnYW5nQHNpbmEuY29t
†These authors have contributed equally to this work
Disclaimer: All claims expressed in this article are solely those of the authors and do not necessarily represent those of their affiliated organizations, or those of the publisher, the editors and the reviewers. Any product that may be evaluated in this article or claim that may be made by its manufacturer is not guaranteed or endorsed by the publisher.
Research integrity at Frontiers
Learn more about the work of our research integrity team to safeguard the quality of each article we publish.