- 1Instituto de Ciencia y Tecnología Dr. César Milstein – Fundación Pablo Cassará – Consejo Nacional de Investigaciones Científicas y Técnicas (CONICET), Buenos Aires, Argentina
- 2Instituto de Investigación en Medicina y Ciencias de la Salud, Facultad de Medicina, Universidad del Salvador, Buenos Aires, Argentina
- 3Instituto Multidisciplinario de Investigación y Transferencia Agroalimentario y Biotecnológica (IMITAB, UNVM-CONICET), Instituto Académico Pedagógico de Ciencias Básicas y Aplicadas, Universidad Nacional de Villa María, Villa María, Argentina
- 4Asociación Nacional de Obtentores Vegetales (ANOVE), Madrid, Spain
- 5Facultad de Ciencias Agrarias, Instituto de Ciencias Agropecuarias del Litoral, CONICET, Universidad Nacional del Litoral, Esperanza, Argentina
- 6Departamento de Química, Facultad de Ciencias Exactas, Físico-Químicas y Naturales, Instituto de Investigaciones en Tecnologías Energéticas y Materiales Avanzados, Universidad Nacional de Rio Cuarto – CONICET, Rio Cuarto, Argentina
- 7Cátedra de Fitopatología, Departamento de Producción Vegetal, Facultad de Agronomía, Universidad de Buenos Aires, Buenos Aires, Argentina
- 8Laboratorio de Bacteriología, Departamento de Protección Vegetal, Instituto Nacional de Investigación y Tecnología Agraria/Consejo Superior de Investigaciones Científicas (INIA/CSIC), Madrid, Spain
Xanthomonas vesicatoria is one of the causal agents of bacterial spot, a disease that seriously affects the production of tomato (Solanum lycopersicum) and pepper (Capsicum annum) worldwide. In Argentina, bacterial spot is found in all tomato producing areas, with X. vesicatoria being one of the main species detected in the fields. Previously, we isolated three X. vesicatoria strains BNM 208, BNM 214, and BNM 216 from tomato plants with bacterial spot, and found they differed in their ability to form biofilm and in their degree of aggressiveness. Here, the likely causes of those differences were explored through genotypic and phenotypic studies. The genomes of the three strains were sequenced and assembled, and then compared with each other and also with 12 other publicly available X. vesicatoria genomes. Phenotypic characteristics (mainly linked to biofilm formation and virulence) were studied in vitro. Our results show that the differences observed earlier between BNM 208, BNM 214, and BNM 216 may be related to the structural characteristics of the xanthan gum produced by each strain, their repertoire of type III effectors (T3Es), the presence of certain genes associated with c-di-GMP metabolism and type IV pili (T4P). These findings on the pathogenicity mechanisms of X. vesicatoria could be useful for developing bacterial spot control strategies aimed at interfering with the infection processes.
1. Introduction
Bacterial spot is one of the most destructive diseases that affect tomato (Solanum lycopersicum L.) and pepper (Capsicum annuum L.) worldwide. The four lineages of Xanthomonas that cause bacterial spot are Xanthomonas euvesicatoria, Xanthomonas vesicatoria, Xanthomonas gardneri and Xanthomonas perforans (Jones et al., 2004; Potnis et al., 2015; Constantin et al., 2016). Strains belonging to different physiological races have been described within each of these four species, according to their pathogenic relationship with tomato and/or pepper plants (Sahin and Miller, 1998; Gore and O'Garro, 1999). More specifically, the races of xanthomonads that caused bacterial spot are recognised based on the hypersensitive response (HR) that they trigger on tomato and pepper lines (Jones et al., 2004). Different strains can infect tomato, pepper, or both. Strains that can infect tomato are classified into races T1-T5 and those that can infect pepper are classified into races P0-P10 (Astua-Monge et al., 2000; Jones et al., 2004; Stall et al., 2009). T1 strains correspond to X. euvesicatoria and carry the avrRxv effector (Whalen et al., 1993). T2 strains belong to X. vesicatoria given that they do not contain the avirulence genes rx1, rx2 and rx3, Xv3 or Xv4; they do not induce HR in any of the tomato indicator cultivars. Races T3, T4 and T5 tend to be X. perforans. T3 strains carry the avrXv3 effector which induces HR in the Xv3-containing genotype and T4 strains have avrXv4 which induces HR in the Xv4-containing genotype. Only S. pennellii x Hawaii 7998 provides resistance to race T5 (Thind, 2019). In pepper, differential genotypes carrying the Bs1, Bs2, Bs3 or Bs4 genes interact with the avirulence genes avrBs1, avrBs2, avrBs3, and avrBs4, respectively, that are present in bacterial strains (Cook and Guevara, 1984; Gaxiola, 2000). Recently, X. euvesicatoria and X. perforans were reclassified as two pathovars of the same species (X. euvesicatoria pv. euvesicatoria and X. euvesicatoria pv. perforans) and X. gardneri was reclassified as X. hortorum pv. gardneri, while the taxonomic classification of X. vesicatoria did not change (Osdaghi et al., 2021). X. vesicatoria frequently causes bacterial spot in field grown tomatoes in Argentina (Romero et al., 2003).
Previously, we found significant differences in the severity of bacterial spot caused by three X. vesicatoria strains (BNM 208, BNM 214, and BNM 216) isolated from diseased tomato plants in Argentina. These strains also showed differences in their motility, adhesion to surface, ability to form biofilm and in the viscosity of the xanthan they produce (Felipe et al., 2018). Although these factors are likely to play an essential role in the ability of X. vesicatoria to successfully colonise and infect tomato, identification of other critical virulence factors is needed for a deeper understanding of the pathogenesis.
The virulence factors that have been described for Xanthomonas so far include adhesins, flagella, fimbriae/pili, exopolysaccharides (EPSs), lipopolysaccharides (LPSs), different secretion systems and their effectors, extracellular degrading enzymes, biofilm formation, and a regulatory network involved in the coordination of these elements (An et al., 2020). Many of these virulence factors and bacterial structures have been widely studied in some phytopathogenic Xanthomonas spp., but little is known about some of them in X. vesicatoria. As in other xanthomonads, the type III secretion system (T3SS) in X. vesicatoria is particularly important for virulence, since it manipulates plant processes for the benefit of the pathogen by introducing type III effectors (T3Es) into the cytosol of the host cell (Thieme et al., 2005; Lorenz et al., 2008; Büttner, 2016). On the other hand, an important aspect of many pathogenic bacteria, including also Xanthomonas spp., is their ability to form biofilms to survive and interact with their hosts (Castiblanco and Sundin, 2016). The development of biofilms allows bacteria to resist biotic and abiotic stresses, supports the coordination of adaptive responses to environmental changes, and enables bacterial multiplication under stressful conditions (Picchi et al., 2021). A prerequisite for biofilm formation is the attachment to a surface through structures of a broad group of fimbrial and non-fimbrial adhesins (Felipe et al., 2018).
Flagella and T4P are multiprotein structures involved in bacterial motility, attachment, adhesion, biofilm formation and pathogenesis (Kearns, 2010; Burdman et al., 2011; Duan et al., 2013; Haiko and Westerlund-Wikström, 2013; Wood, 2013), whose importance has been demonstrated in some phytopathogenic Xanthomonas spp. (Rigano et al., 2007a; Malamud et al., 2011; Bianco et al., 2016; Dunger et al., 2016). T4P, in particular, are implicated in natural transformation, phage infection, and twitching motility (Caserta et al., 2010; Burrows, 2012; Dunger et al., 2014, 2016). There are different types of T4P, which are divided into two major subclasses, type IVa and IVb, though only type IVa pilins mediate twitching motility (Burrows, 2012). For its assembly and function, T4P require numerous structural and regulatory components, as major pilins, which are named PilA (main component of the filament), and minor pilins, which are present in lower quantities and prime the assembly of T4P (Nguyen et al., 2015; Ronish et al., 2019). PilA proteins belong to the type IVa pilins subclass, characterized by having a small prepilin leader sequence (5–6 amino acids) in the N-terminal region containing the G-1F + 1/E+5 motif. This motif is required for the cleavage of the prepilin leader sequence (between G-1 and F + 1) and the subsequent methylation of the phenylalanine located at the N-terminal end of the mature protein. Both modifications are essential to incorporate the mature pilin subunits into the nascent pilus (Dunger et al., 2014).
On the other hand, xanthan gum is the main EPS synthesised by Xanthomonas spp. and an essential component of the extracellular biofilm matrix. It contributes to epiphytic survival before plant colonisation and plays an important role in biofilm formation, being necessary for bacterial virulence and disease progression (Dow et al., 2003; Yun et al., 2006; Torres et al., 2007; Rigano et al., 2007a,b; Aslam et al., 2008; Darsonval et al., 2009; Yaryura et al., 2014; Bianco et al., 2016). Earlier, we reported the effects of xanthan composition, structure and viscosity on the characteristics of biofilm and virulence in Xanthomonas campestris pv. campestris (Xcc) (Bianco et al., 2016) and Xanthomonas citri subsp. citri strain 306 (Xac306) (Conforte et al., 2019). Moreover, we observed that xanthan-deficient mutants were unable to form biofilm (Rigano et al., 2007a; Bianco et al., 2016).
Xanthomonas genomes from all named Xanthomonas spp. are currently available in public databases and have been used in studies addressed to different purposes, including those aimed to elucidate virulence or host range factors in this bacterial genus (Timilsina et al., 2020). Since 2011 genome sequencing projects from bacterial spot of tomato and pepper xanthomonads have been generated and applied in studies addressed to explain the relationship among species and pathovars, their virulence and host range basis, the diversity of effectors and their epidemiology (Potnis et al., 2011; Schwartz et al., 2015; Barak et al., 2016). Our work is aimed to analyse bacterial virulence factors that might explain the differences in the aggressiveness between three X. vesicatoria strains isolated from Argentina (Felipe et al., 2018), on the basis of comparative phenotypic and genotypic analysis. Our goal is to increase our knowledge on virulence factors of X. vesicatoria that could be useful in other Xanthomonads models, as a preliminary step towards future new disease control methods.
2. Materials and methods
2.1. Bacterial strains and culture conditions
The three X. vesicatoria strains used in this work were BNM 208, BNM 214, and BNM 216. They had been isolated from leaves of tomato plants with symptoms of bacterial spot from commercial field crops and deposited at the culture collection of the “Banco Nacional de Microorganismos” (BNM, Argentina, WDCM number 938, GCM) as described previously (Felipe et al., 2018).
The strains were routinely grown on modified yeast dextrose calcium carbonate (YDC) agar plates (Ritchie and Dittapongpitch, 1991) or in peptone-yeast extract-malt extract (PYM) medium (Cadmus et al., 1976) and incubated at 28°C for 24–72 h, depending on the experiment and with shaking at 200 r.p.m. for liquid cultures.
2.2. Genomic sequencing and annotation
The DNA of the strains was extracted from 30 mL of a pure and fresh bacterial culture using the Wizard® Genomic DNA Purification Kit Promega (Madison, WI, United States) according to the manufacturer’s instructions. DNA quantity and quality were determined by Picodrop microlitre Spectrophotometer (Picodrop Ltd., Cambridge, United Kingdom) and through 0.8% agarose gel electrophoresis. The genomes of BNM 208, BNM 214, and BNM 216 strains were sequenced using the Illumina HiSeq 2,500 platform (2 × 100 bp paired-end reads) by an external sequencing service (Macrogen Inc., NGS Service, Seoul, Korea). The quality of the obtained reads was examined by FastQC v.0.11.8 (Andrews et al., 2010). Raw reads were quality filtered and trimmed with Trimmomatic v.0.38 (Bolger et al., 2014) (Parameters: ILLUMINACLIP:TruSeq3-PE.fa:2:30:10, LEADING:3 TRAILING:3 SLIDINGWINDOW:4:15 MINLEN:36). De novo genome assemblies of BNM 208, BNM 214, and BNM 216 were performed with MIRA v.4.0 (Chevreux et al., 1999) and some quality parameters (Table 1), such as the number of contigs, the finish quality (according to BUSCO completeness), N50, GC %, were verified by QUAST v.4.5 using its web version with default parameters (Gurevich et al., 2013). Automatic annotation of the draft genome sequences was conducted using the Prokka v.1.13.3 pipeline (Seemann, 2014) using a specific database constructed with all the complete reference genome sequences of the genus Xanthomonas available at NCBI, as well as using the Prokaryotic Genome Annotation Pipeline (PGAP) available at NCBI (Tatusova et al., 2016). The presence of signal peptides (leader sequences), transmembrane domains and the assignment of a potential function according to the Gene Ontology (GO) and the Clusters of Orhtologous Groups of proteins (COG) databases (Tatusov et al., 2001; The Gene Ontology Consortium, 2021) were performed for all the protein-coding sequences by using signalP v.5.0 (Almagro Armenteros et al., 2019), the TMHMM server v.2.0 (Krogh et al., 2001) and the functional annotation pipelines available in the OmicsBox (v. 1.3), the NCBI CD-Search online platform (Lu et al., 2020) and the Geneious (v. 7.1) software by using default parameters.
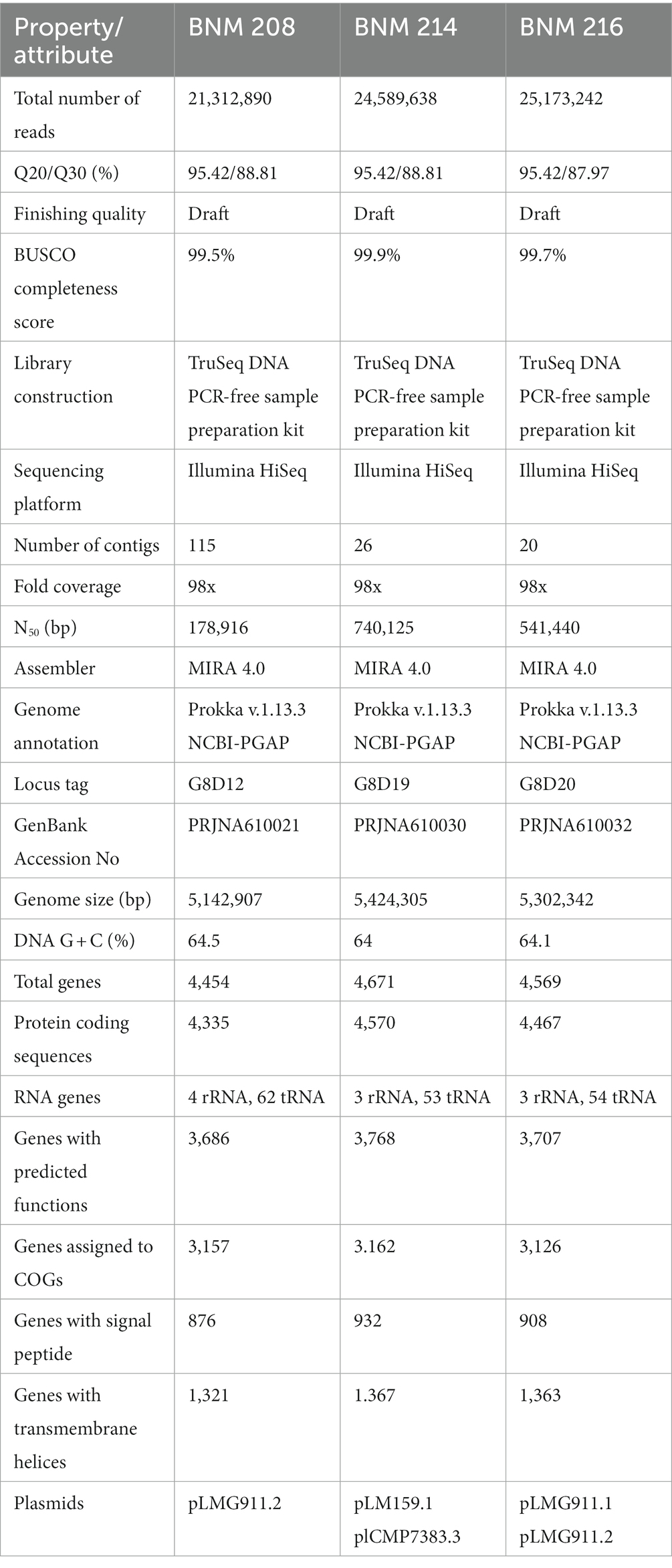
Table 1. Sequencing information and genome statistics of X. vesicatoria strains BNM 208, BNM 214 and BNM 216.
Genomic sequences of the three X. vesicatoria strains studied in this article were deposited in the GenBank database: GenBank Accession N° PRJNA610021 (BNM 208), PRJNA610030 (BNM 214) and PRJNA610032 (BNM 216).
2.3. Comparative genome analysis
Output data obtained from Prokka was used as input data to confirm the taxonomy of the sequenced strains by calculating the Average Nucleotide Identity (ANI) based on the BLAST algorithm compared to the reference genome sequences of the Xanthomonas species. These data were also used to perform the pangenomic analysis by comparing the genome of the three sequenced strains with other 12 X. vesicatoria genome sequences publicly available (Supplementary Table S1). These two analyses were conducted with the pipelines in Pyani v.0.2.7 (Pritchard et al., 2016) and Roary v.3.11.2 (Page et al., 2015).
A batch of 410 genes associated with pathogenesis in Xanthomonas (TBDTs, TonB dependent transporters; STCRs, sensors of the two-component regulatory system; MCPs, methyl accepting chemotaxis proteins; structure and regulation of flagella and T4P, production of xanthan gum, quorum sensing, cell wall degrading enzymes, secretion systems – types II, III, and IV – as well as their associated effectors) (Garita-Cambronero et al., 2019), was searched in all the 15 available genome sequences of X. vesicatoria with BLASTp by using an identity and coverage cutoff of 80%. Finally, the presence of plasmids was ascetained with the PLSDB plasmid database (Galata et al., 2019).
2.4. Bioinformatics analysis of genes associated with T4P
The identity of each sequence within the T4P cluster was confirmed with the protein databases InterPro (Blum et al., 2020) and BLASTp, and the online ORF Finder server (Open Reading Frame Finder; RRID:SCR_016643). In addition, the online server iPro54-PseKNC (Lin et al., 2014) was used to identify the presence of σ54 promoters as binding sites for the transcriptional regulator PilR. To identify the presence of the signal sequence characteristic of type IVa pilins, the protein sequences found were aligned using the ClustalW tool in MEGA11 software (Molecular Evolutionary Genetics Analysis version 11; Tamura et al., 2021).
2.5. Polymerase chain reaction (PCR) assays
The bacterial genomic DNA was prepared with Wizard® Genomic DNA Purification Kit (Promega Corporation, Madison, United States). PCR amplifications were carried out in a T-18 thermocycler (Ivema Desarrollos, Argentina). The samples contained PCR buffer 1X, 200 μM of each deoxynucleoside triphosphate (Inbio Highway, Tandil, Argentina), 100 mM of one primer set (see Supplementary Table S2), 1.5 mM of MgCl2, 1.25 U of Taq DNA polymerase (PB-L, Productos Bio-Lógicos SA, Buenos Aires, Argentina) and 50 ng of pure genomic DNA preparation in a total volume of 20 μL. The samples were heated as follows: 94°C for 3 min, 30 cycles at 94°C for 30 s, annealing temperature (of each primer set) for 30 s, 72°C for 1 min, and a final cycle at 72°C for 5 min. The PCR products were analysed by electrophoresis on 1.5% (w/vol) agarose gels.
2.6. Gene expression analysis
The strains were cultured in PYM medium until the stationary phase. The cells were harvested at OD600 1–1.2. Total RNA was extracted with TRIzol (Gibco-BRL, Burlington, ON, Canada) following the manufacturer’s instructions, and quantified by spectrometry. Its integrity was checked by agarose gel electrophoresis. Reverse transcription was conducted by using random primers and M-MLV RT Promega (Madison, WI, United States). All primers used in this work were designed with the software Primer Express 3.0 (Applied Biosystems, Foster City, CA, USA) (Supplementary Table S2). The reactions were performed using SybrGreen master mix (Roche, Mannheim, Germany) and a Step One Real Time-PCR system (qPCR) (Applied Biosystems), as described previously (Conforte et al., 2019). The protocol for the qPCR was as follows: 50°C for 2 min, initial denaturation at 95°C for 5 min, followed by 40 cycles of 10 s at 95°C and 30 s at 60°C. The qPCR data analysis and primer efficiencies were obtained using LinReg PCR software (Ramakers et al., 2003). The 16S rRNA gene was used to standardise the expression of a given target gene; then, a ratio between treatments was calculated using the algorithm developed by Pfaffl et al. (2002). The relative expression ratios and the statistical analyses were performed using the fgStatistics software interface (Di Rienzo, 2012). The cutoff for statistically significant differences was set at p < 0.05.
2.7. Motility assays
Swimming and swarming motility assays were performed as described by Picchi et al. (2016). In both cases, 2 μL of bacterial suspensions of BNM 208, BNM 214, and BNM 216 strains (OD600 0.1) were placed in the centre of Petri dishes with PYM medium with 0.5% (w/v) agar and NYGB medium with 0.25% (w/v) agar for swarming and swimming assays, respectively. After incubation at 28°C for 72 h, swimming and swarming motility of each strain was quantified by measuring the diameter of the bacterial growth halo with Image J1.4 software (Wayne Rasband, National Institutes of Health, United States).1
2.8. Examination of flagella
Flagellum staining for subsequent observation by optical microscopy was performed as previously described in Kodaka et al. (1982). Briefly, the staining solution was prepared by mixing 10 parts of the mordant solution [2 g tannic acid, 10 mL 5% (w/v) phenol, 10 mL saturated aqueous of AlKO8S2.12H2O] with one part of the crystal violet solution [12% (w/v) in ethanol]. A drop of an overnight culture of each strain was placed on a glass slide and air dried. Then, samples were stained with 10 mL of staining solution and observed at 1,000× with Leica DM1000 LED (Leica, Wetzlar, Germany) optical microscope. Images were taken with Leica MC170 HD camera.
2.9. Twitching motility: time-lapse imaging
T4P dependent movement (twitching motility) was studied by monitoring the position of bacterial cells under the microscope at different time intervals, following Llontop et al. (2021) with some modifications. Briefly, the different strains were grown on LB agar plates at 28°C, and then some colonies were sampled by scraping the plates. Bacterial suspensions were prepared with sterile water, and 2 μL of each were placed on a King B agar slab, left to dry, and covered with a coverslip. After 30 min at 28°C, they suspensions were observed under a Leica DM1000 LED (Leica, Wetzlar, Germany) microscope (1,000×), and images were taken every 30 s with a Leica MC170 HD camera.
The King-B agar plates were prepared as described by Bayer-Santos et al. (2019) with slight modifications. Briefly, the top of a glass slide was completely covered with double-sided tape (clear 3 M VHB, 19 mm wide, 1 mm thick). A rectangular space in the center was cut with a scalpel, removed and filled with melted King B medium containing 1% (w/v) agar and supplemented with 2 mM of CaCl2. To create a flat and smooth surface, a second glass slide was placed over it and was carefully slipped off when the medium solidified.
2.10. Xanthan analysis by atomic force microscopy (AFM)
AFM, which is based on the interaction between an exploratory tip and the surface of a sample (in our case, xanthan), makes it possible to visualize individual well separated macromolecules on a mica surface (Ikeda et al., 2012). Xanthan gum produced by the three X. vesicatoria strains was obtained as described previously described by Felipe et al. (2018). For the AFM analysis, xanthans were dispensed into type I grade water (Purelab Classic, Elga Veolia) at a concentration of ca. 5–15 μg mL−1. A drop of each sample was deposited onto a freshly cleaved mica (V-1 Grade, SPI Suppliers), kept in contact with the surface for 1–2 min, then the excess was removed by a gentle tilt of the substrate and left to dry in air.
The AFM experiments were performed with an Agilent Technologies SPM/AFM microscope model 5500, which was mounted into a vibration isolation chamber (Agilent Technologies Inc.) and operated in air at room temperature. The samples were analysed under intermittent contact (Acoustic AC Mode) using silicon cantilevers (MikroMasch, NSC15/Al BS) with a spring constant in the range of 20–75 N/m, oscillating at frequencies just below the resonance. The tip was scanned on the sample surface by A-type open-loop Multi-purpose Scanner (90 90 μm2) at a speed of 0.4–2 lines/s depending on the size of the observed area. The topographical images were created from raw data values treated and analysed using Gwyddion 2.602 software package, corrected only by using the 2D levelling (usually plane level) and scan line corrections (usually align rows) tools.
2.11. In planta assays
Bacterial suspensions of X. vesicatoria strains BNM 208, BNM 214, and BNM 216 [108 colony-forming unit (CFU) mL−1] were infiltrated into the abaxial leaflets and leaf surfaces of differential tomato differential plants using a needless syringe. The tomato differential lines used were S. lycopersicum Bonny Best (universal susceptible line), Hawaii 7998 (effective against avrRxv; race T1) (Whalen et al., 1993) and Solanum pimpinellifolium plant introduction (PI) 128216 (effective against avrXv3; race T3) (Scott et al., 1995).
2.12. Statistical analysis
Three replicate trials were carried out for each sample, and all the experiments were repeated three times. Data from experiments were analysed by one-way ANOVA and mean differences were determined by Tukey’s test at a significance level of p ≤ 0.05. For ANOVA, parametric assumptions were tested using the Shapiro–Wilk test for normality, and the Levene’s test for homogeneity of variance using the R functions of the R package stats. All statistical analyses were performed using the INFOSTAT 1.0 software (Di Rienzo et al., 2009). Data from the experiments were expressed as mean ± the standard deviation (SD).
3. Results
3.1. Genome sequencing and comparative analysis of Xanthomonas vesicatoria strains BNM 208, BNM 214, and BNM 216
3.1.1. General features
The whole-genomes of X.vesicatoria strains BNM 208, BNM 214, and BNM 216 were sequenced to explore genomic characteristics that might explain their different phenotypes. Table 1 shows information and statistics of the sequencing project, as well as the main general genomic features. The genome assemblies, based on 21,212,892 to 25,173,242 usable reads, resulted in 115 contigs for BNM 208, 26 for BNM 214, and only 20 for BNM 216, all with 98-fold coverage. Despite the difference in contig numbers among the strains, a similar number of genes and protein-coding sequences (CDS) was predicted (Table 1). Additionally, the potential plasmid sequences found in all three genomes were more than 99% identical to what had been previously described in X. vesicatoria and X. gardneri (Table 1).
When comparing the genome of BNM 208, BNM 214, and BNM 216 with those of 12 other representative X. vesicatoria strains (Supplementary Table S1), the average nucleotide identity (ANI) was more than 99% (Figure 1). This confirms that the three strains are members of X. vesicatoria. This analysis also showed that 3,577 core genes are shared by all 15 X. vesicatoria strains, that 3,147 accessory genes were identified (Figures 2A,B) and that a total of 68,196 CDSs were identified in the pangenome of X. vesicatoria (Figure 2C). BNM 208, BNM 214, and BNM 216 contained 119, 205, and 268 unique CDSs, respectively (Figure 2B).
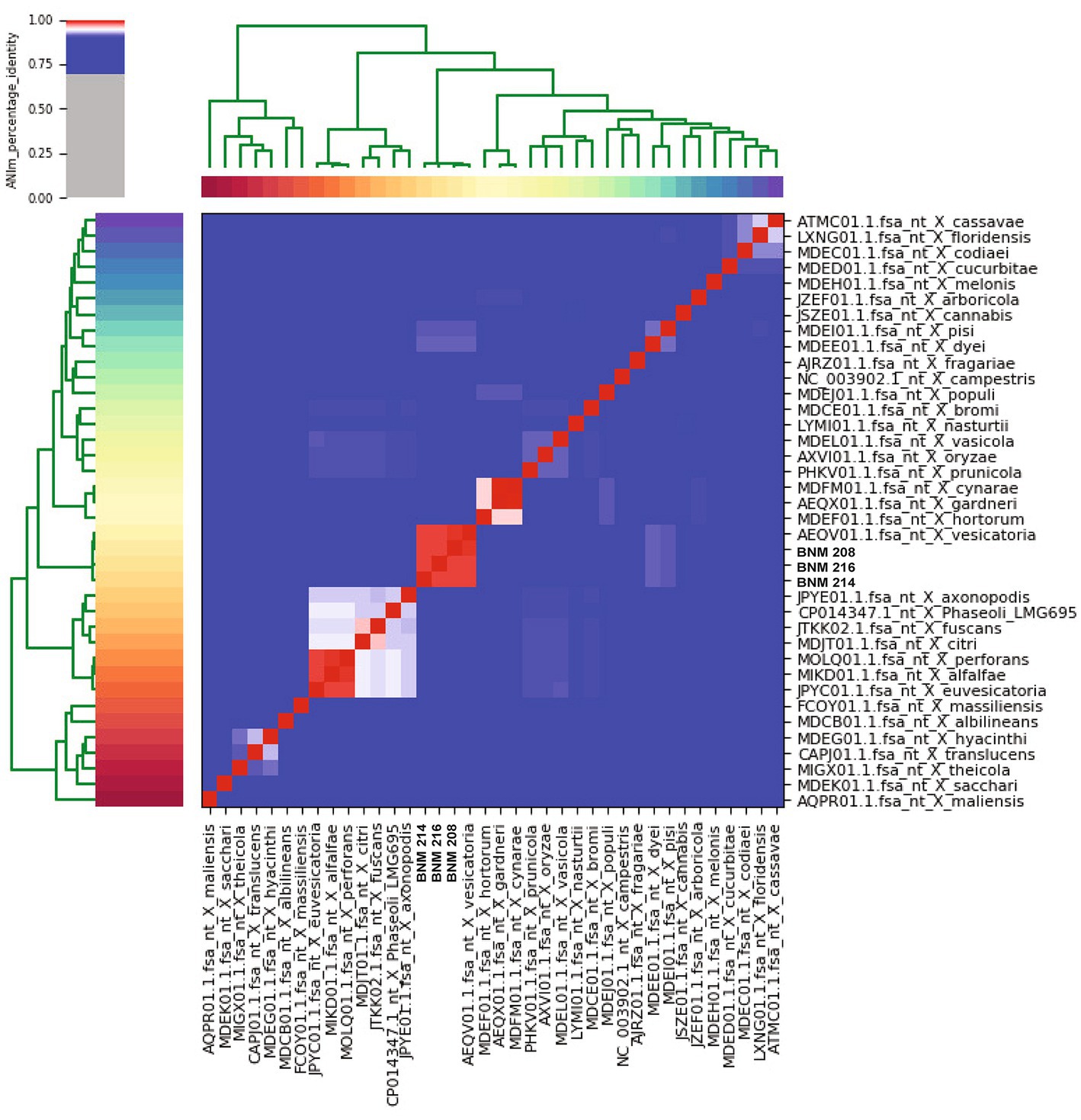
Figure 1. Average nucleotide identity (ANI) analysis. Confirmation of the taxonomy of BNM 208, BNM 214, and BNM 216 by calculating ANI compared to the reference genome sequences of the Xanthomonas species. The analysis shows ANI percentage over 99%.
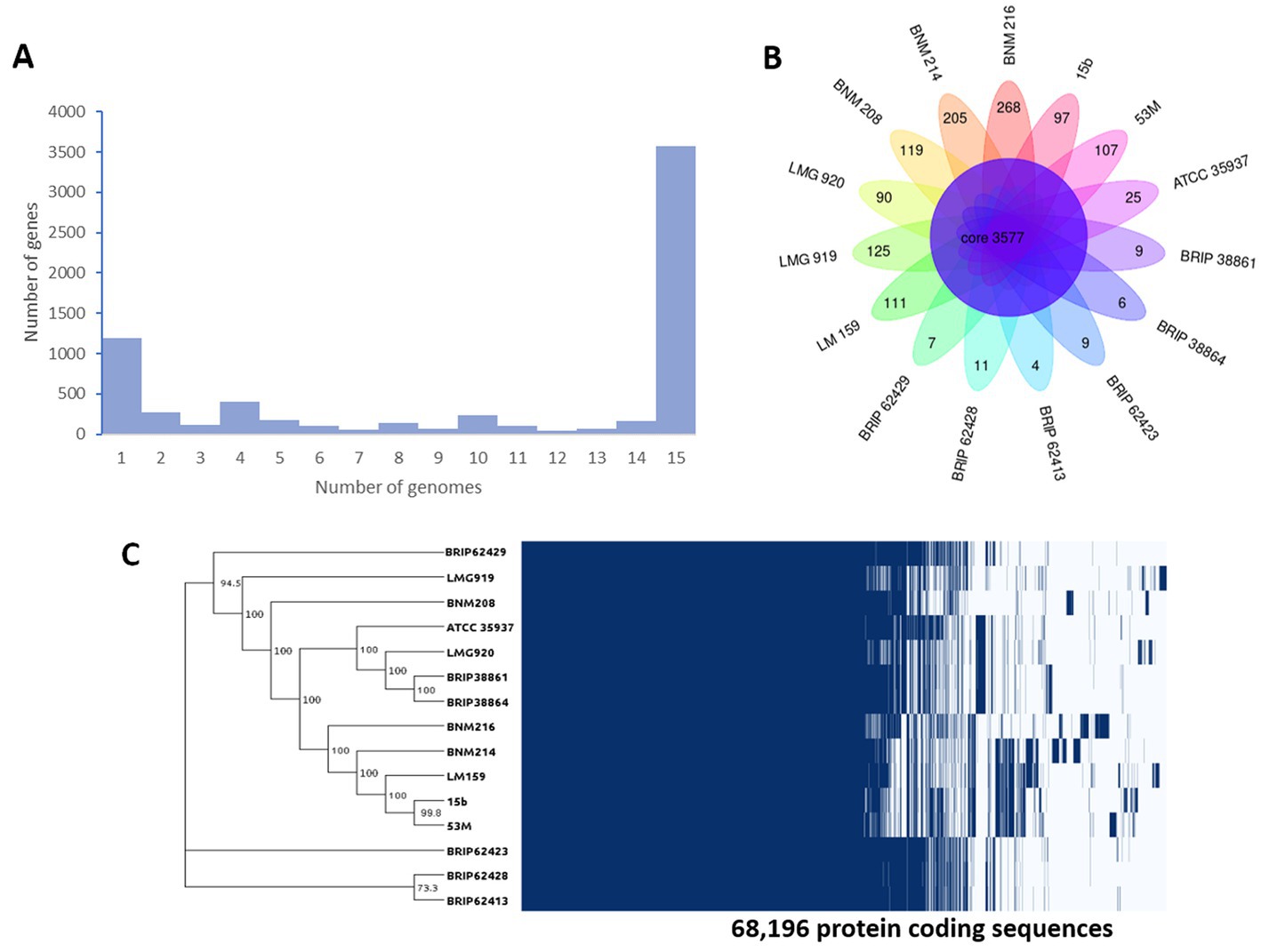
Figure 2. Genome comparative analysis between of X. vesicatoria strains BNM 208, BNM 214, BNM 216, and 12 X. vesicatoria strains. (A) Distribution of the 6,724 unique gene clusters per genome sequence. (B) Representation of genes shared among the 15 X. vesicatoria strains analysed in this work as well as BNM 208, BNM 214, and BNM 216 unique CDS. (C) Phylogenetic analysis of 15 strains of X. vesicatoria based on the core genome sequence (3,777 CDSs) and distribution of the 68,196 CDSs found in the pangenome of X. vesicatoria. Bootstrap values showed at the branch points.
3.1.2. Gene ontology
According to the functional analysis based on the Clusters of Orthologous Genes (COGs), the CDSs were similarly distributed in the genomes of the three strains, and 25 out of the 26 COG functional categories were represented (Table 2).
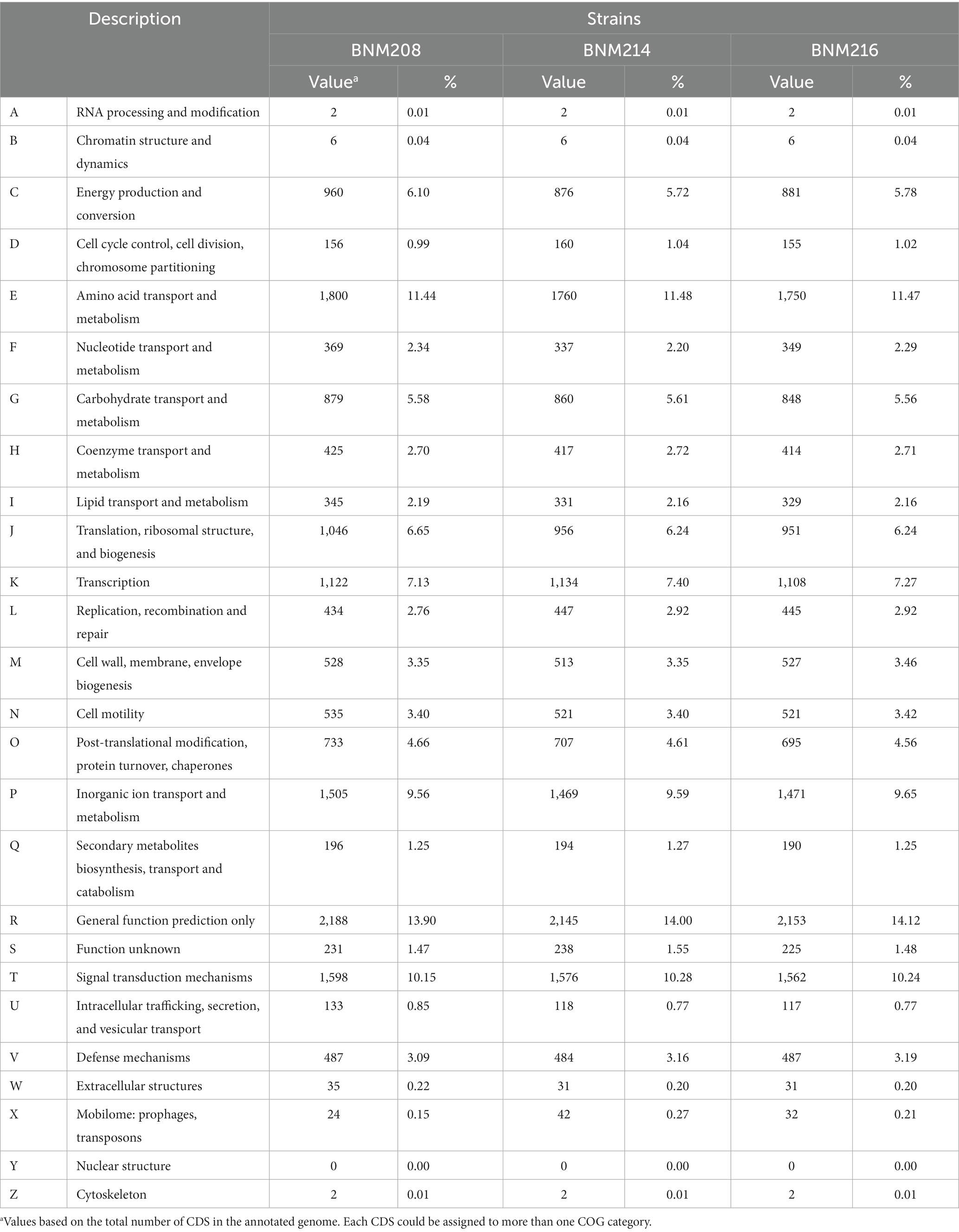
Table 2. CDSs distribution in COG functional categories for the three X. vesicatoria genomes sequenced.
A general functional analysis was performed using the Gene Ontology (GO) database. Out of 4,454 potential gene sequences in BNM 208, 4,400 were associated with 967 molecular functions (MFs); 3,413 with 651 biological processes (BPs), and 1,777 with 75 cellular components (CCs) (Figure 3). Out of 4,671 potential gene sequences in BNM 214, 4,316 were assigned to 970 MFs, 3,358 to 650 BPs, and 1,742 to 75 CCs. Finally, out of 4,569 potential gene sequences in BNM 216, 4,265 were associated with 972 MFs, 3,333 with 648 BPs and 1,724 with 75 CCs. The leading 20 MFs, BPs and CCs for each strain are shown in Figure 3. The most abundant BPs were the macromolecule metabolic process (GO:0043170), the cellular nitrogen compound metabolic process (GO:0034641), and the organonitrogen compound metabolic process (GO:1901564). In the case of MFs, the most abundant were nucleic acid binding (GO:0003676), nucleoside phosphate binding (GO:1901265), and the nucleotide binding function (GO:0000166). The most abundant CCs comprised the integral components of the membrane (GO:0016021) and plasma membrane (GO:0005886), as well as components of the non-membrane bound organelles (GO:0043228).
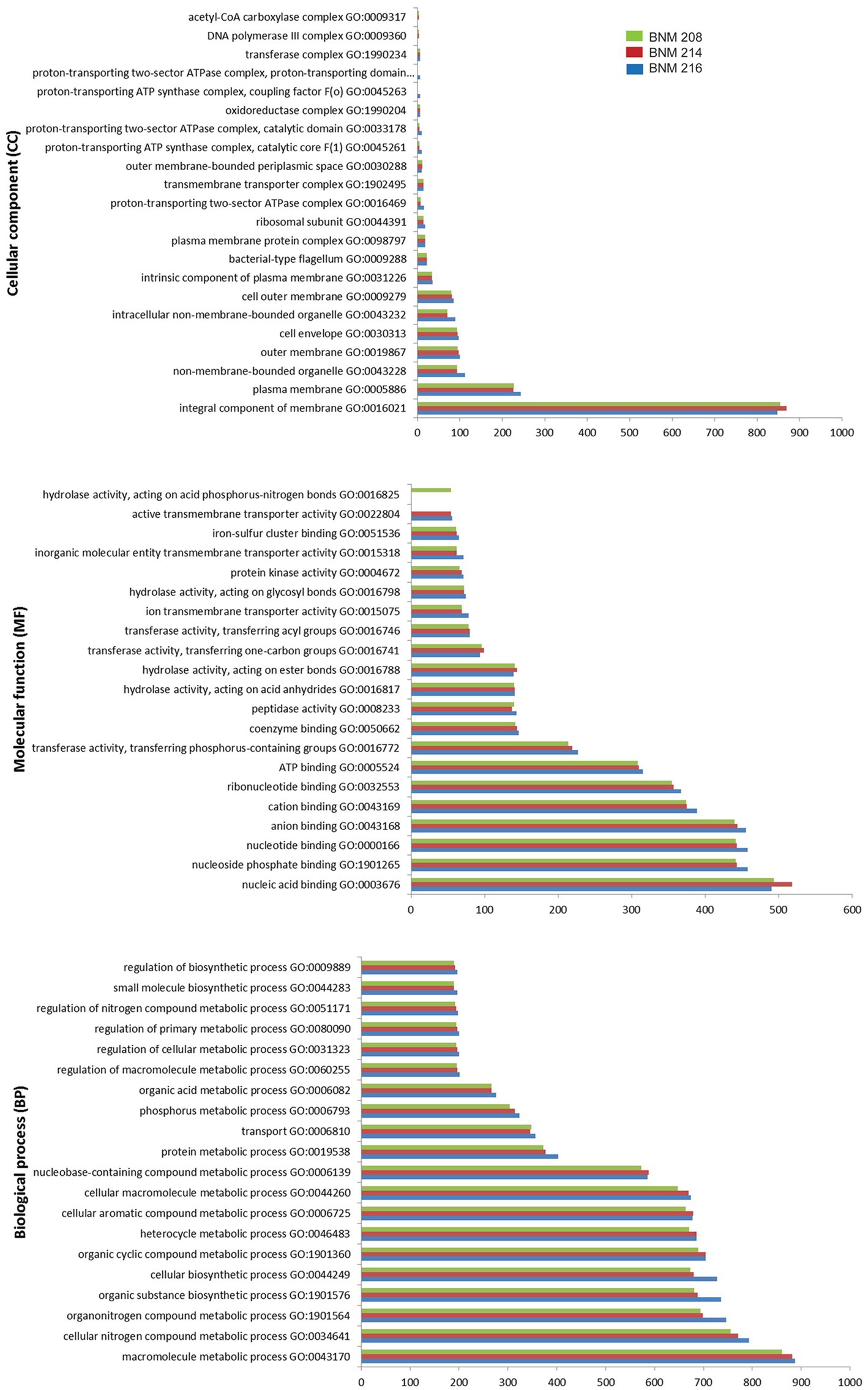
Figure 3. Number of gene coding sequences of X. vesicatoria strains BNM 208, BNM 214, and BNM 216 associated with the top 20 functions according to their cellular components (top panel), molecular functions (middle panel) and biological processes (bottom panel) in the GO database.
3.1.3. Genomic analysis of factors involved in virulence
The presence of more than 400 genes known to be functionally associated with pathogenesis in Xanthomonas, was determined in the genomes of the studied strains. As a whole, they showed a very similar repertoire. CDSs related to virulence enzymes were the same in BNM 208, BNM 214, and BNM 216. They consisted of at least 19 cellulolytic enzymes, 13 hemicellulolytic enzymes, 12 pectolytic enzymes and two lipases (Supplementary Table S3). Moreover, the strains had the same pattern for potential CDSs associated with chemotaxis, quorum sensing, flagellum components and regulators, xanthan gum production, and the components of secretion systems types II (T2SS) and III (T3SS) (Supplementary Table S3).
Slight variations were found in those genes that code for elements involved in sensing environmental changes, such as methyl-accepting chemotaxis proteins (MCPs), sensors of the two-component regulatory system (STCRs) and TonB-dependent transporters (TBDTs). Although the pattern of 23 MCPs-related genes was similar in the three strains, a CDS homologous to XCV1778 (NCBI RefSeq ID: WP_029819220) was absent in BM216. Out of the 70 genes that encode STCRs, xac0610 homologous (AAM35499.1) and xcv3267 (CAJ24998.1) were not present in BNM 216 and BNM 214, respectively. Finally, out of the 26 TBDT-encoding genes, only BNM 216 lacked sequenced homologous to xcc1340 (AAM40638.1) (Supplementary Table S3).
3.1.4. Pilin related in-silico analyses
The pilSRBACD gene cluster (Dunger et al., 2014), which encodes for major pilin PilA and minor pilins, was present in the three strains with some differences. Sequences homologous to pilS XAC3237, pilR XAC3238, pilB XAC3239 pilC XAC3242 and pilD XAC3243 in Xac306 (hereafter referred to as pilSRBCD) were found in our three X. vesicatoria strains (Supplementary Table S3 and Figure 4). In BNM 208, two genes (pilin-like 1 and pilin-like 2), were detected between pilB-like and pilC-like genes. The two were homologous to the fimbrillins pilAXac3241 (AAM38085.1) and pilAXac3240 (AAM38084.1) in Xac306, though the similarity/identity percentage was under 80% (Supplementary Table S3 and Figure 4). In BNM 214 and BNM 216, six genes were detected between pilB-like and pilC-like genes. The first encodes a hypothetical protein (hp), the second one is pilin-like 2, the third codes for a family transporter RarD (EamA), the next two for glycosyltransferases (gtf), and the last one for another hypothetical protein (Figure 5A). The amino acid sequences of the Pilin-like proteins in our strains were aligned for comparison with those of homologous major pilins in Xac306 (PilAXAC3240 and PilAXAC3241). Both Pilin-like 1 and Pilin-like 2 contain a G-1F + 1/E+5 motif, that is characteristic of type IVa pilins (Figure 5B). Besides, the in-silico analysis of the tertiary structures of Pilin-like 1 and Pilin-like 2 showed that their predicted structures are similar to PilAXAC3241 and PilAXAC3240 of Xac306 (Supplementary Figure S1).
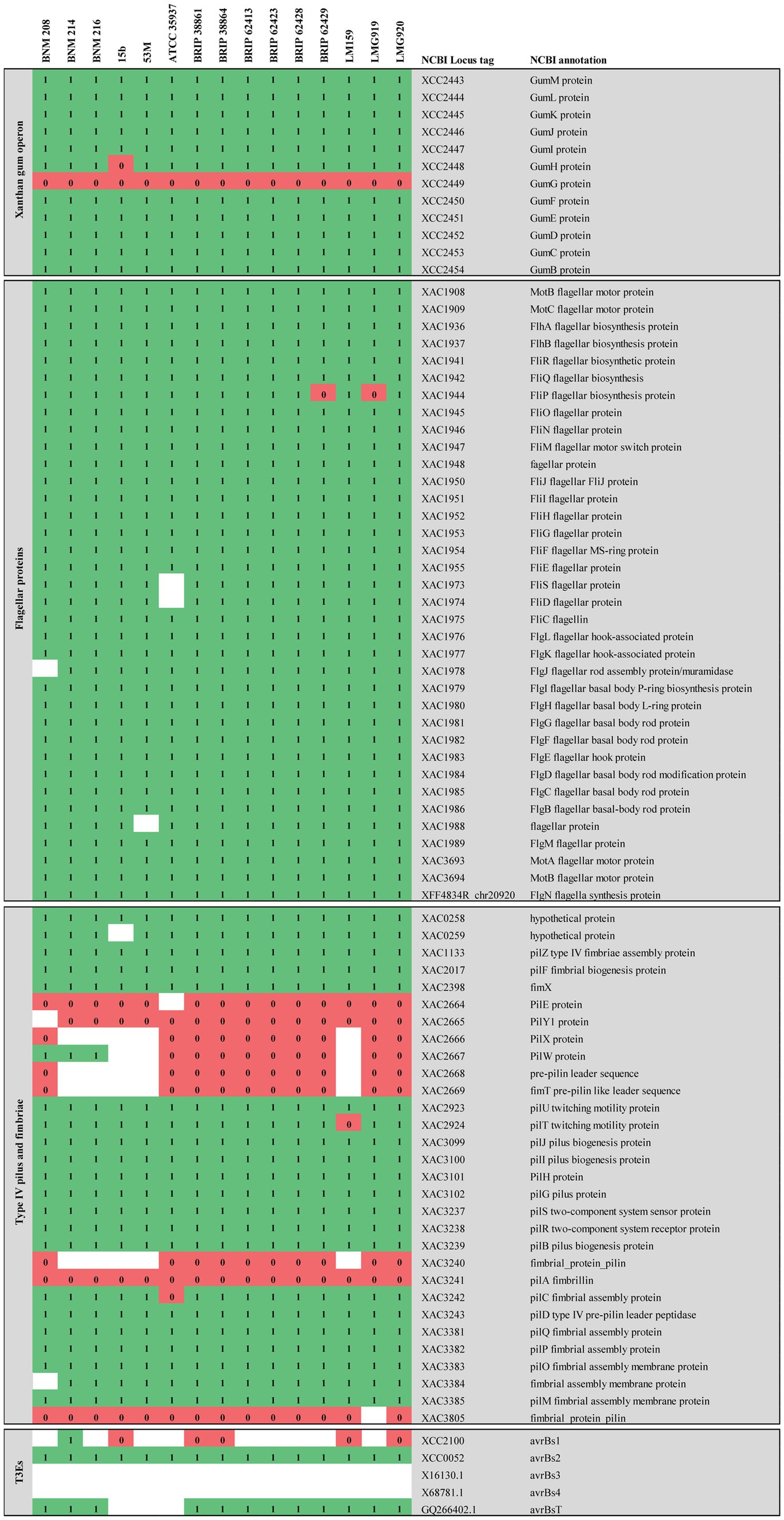
Figure 4. Comparative genomic analysis of CDSs associated with virulence-related genes described in Xanthomonas. The comparative analysis included strains BNM208, BNM214, BNM216, and 12 other X. vesicatoria strains whose genomes are available in databases. This figure shows only the results of the FIGURE 4 (Continued)analysis for clusters associated with xanthan, flagellum and type IV pili (T4P) and for genes encoding six effector proteins secreted by the type III secretion system (TE3s) associated with host specificity and genes encoding sensors of the two-component regulatory system (STCRs). Comparative genomic analysis for other genes associated with T3Es and STCRs is shown in the Supplementary Table S3. Each of the genes analysed is represented by its locus tag number at the National Center for Biotechnology Information (NCBI) database. Xac, X. citri subsp. citri; Xcc, X. campestris pv. campestris; Xff, Xanthomonas fuscans subsp. fuscans. The similarity/identity of homologous sequences showing a percentage higher than 80% (1) are shown in green, those showing a percentage lower than 80% (0) are shown in red and homologous sequences not found are shown in white.
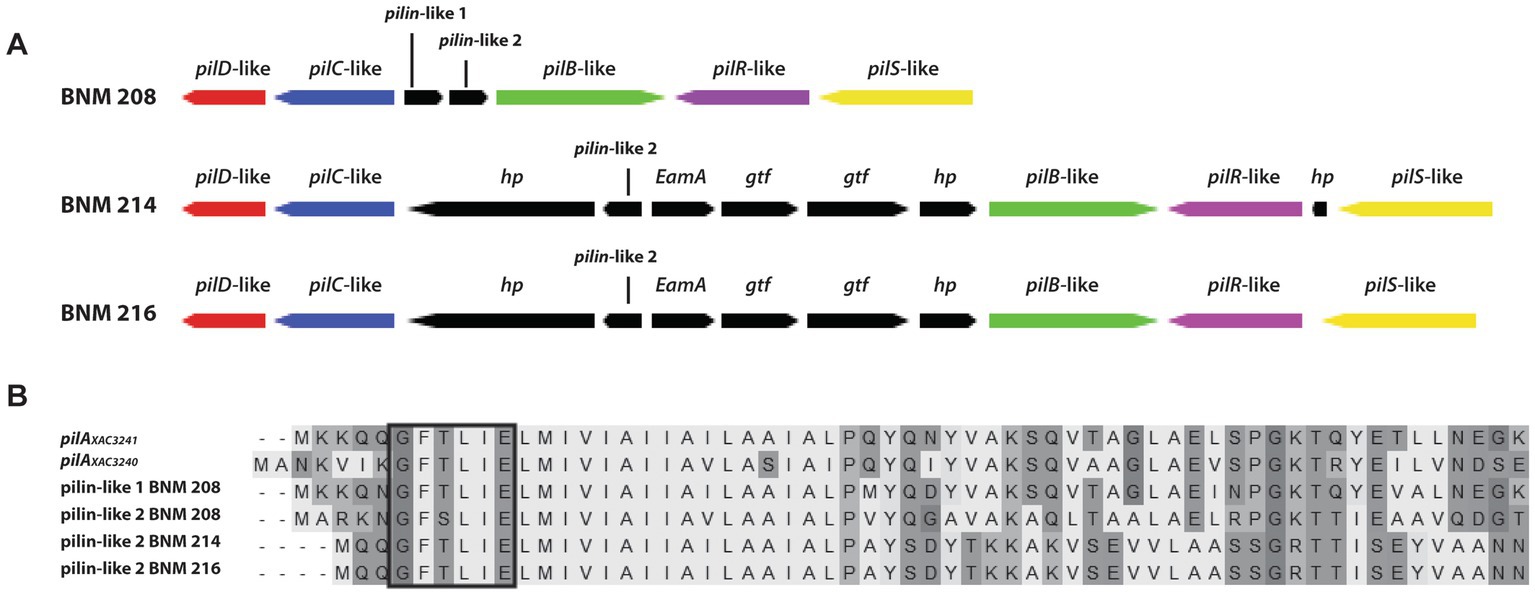
Figure 5. In silico analysis of some genes involved in the biosynthesis of T4P. (A) Schematic representation of the set of genes pilSRBCD found in the BNM 208, BNM 214, and BNM 216 genomes (hp, hypothetical protein; gtf, glycosyltransferase; EamA, family transporter RarD). (B) Amino acid alignment of proteins encoded by pilin-like 1 and pilin-like 2 genes of BNM 208, BNM 214, and BNM 216 with pilAXAC3241 and pilAXAC3240 of Xac306. The prepilin leader sequence (signal peptide) is marked with a box. The alignment was carried out using ClustalW.
In terms of minor pilins involved in virulence in other species, the three strains in this study had a similar sequence to xac3805 (AAM38647.1) in Xac306 (named DUF4339 domain containing protein gene, henceforth referred to as DUF4339). They also presented a CDS homologous to pilEXac2664 (AAM37511.1). On the other hand, a CDS homologous to pilY1Xac2665 was detected in BNM 214 and BNM 216 although with less than 80% similarity/identity (Supplementary Table S3 and Figure 4). Potential CDSs homologous to pilXxac2666 (AAM37513.1) and fimTxac2669 (AAM37516.1) in Xac306 were absent in BNM 214 and BNM 216. However, BNM 208 did harbour potentially homologous sequences to these genes, although the similarity/identity percentage was once again under 80% (Supplementary Table S3 and Figure 4).
3.1.5. In-silico analyses for T3Es
Differences between the three strains were also noted in the repertoire of potentially homologous T3E-encoding sequences. Out of 38 CDSs associated with these effectors, 26 were found in BNM 208, the most virulent strain (10 with a similarity/identity percentage over 80% and 16 with a similarity/identity percentage under 80%), 29 in BNM 214 (13 with a similarity/identity percentage over 80% and 16 with a similarity/identity percentage under 80%), and 25 in the least virulent strain, BNM 216 (8 with a similarity/identity percentage over 80% and 17 with a similarity/identity percentage under 80%). Supplementary Table S3 shows the presence/absence of each of these 38 T3E-asociated CDs in the 15 genomes analysed, with the main differences pertaining to our strains mentioned below. Potentially homologous sequences to xopR (KT873949.1), xopD (CAJ22068.1), xopE4 (EFF46466.1), xopAG (EGD09323.1), xac0277 (AAM35169), xac0543 (AAM35432.1) and xcc3600 (AAM42870.1) were not detected in any of our three strains (Supplementary Table S3).
Only BNM 214 has a complete sequence of avrBs1 (AAM41388.1) with over 80% similarity to that in Xac306. In the three strains, analysis of amino acid sequences corresponding to avrBs3 (CAA34257.1) and avrBs4 (CAA48680.1) showed a partial nucleotide sequence that mapped about 2,000 bp out of a total of 4,000 bp for the described genes. There were no potentially homologous sequence to xopE2 (AAM39257.1), xopH1 (CAJ19917.1), or xopE3 (AAM38068.1) in BNM 216, or to xopN (AAM37631.1) in BNM 214 and BNM 216. Similarly, potentially homologous sequences to xopX (EGD08143.1) and xac0543 (AAM35432.1) were undetected in BNM 208 (Supplementary Table S3).
3.2. In vitro analysis of virulence factors that contribute to biofilm formation and/or virulence
3.2.1. Fimbrial adhesins involved in bacterial adhesion and motility: flagella and T4P
Previously, we reported significant differences in swarming (flagellum-dependent movement) between BNM 208, BNM 214, and BNM 216 (Felipe et al., 2018). Considering that swarming also depends on other factors than flagella, in this work, we studied swimming motility, which is mainly dependent on the flagella. Our results showed that the three strains were able to swim at similar levels (Figure 6A). In addition, we observed by optical microscopy a single polar flagellum in BNM 208, BNM 214, and BNM 216 cells (Figure 6B).
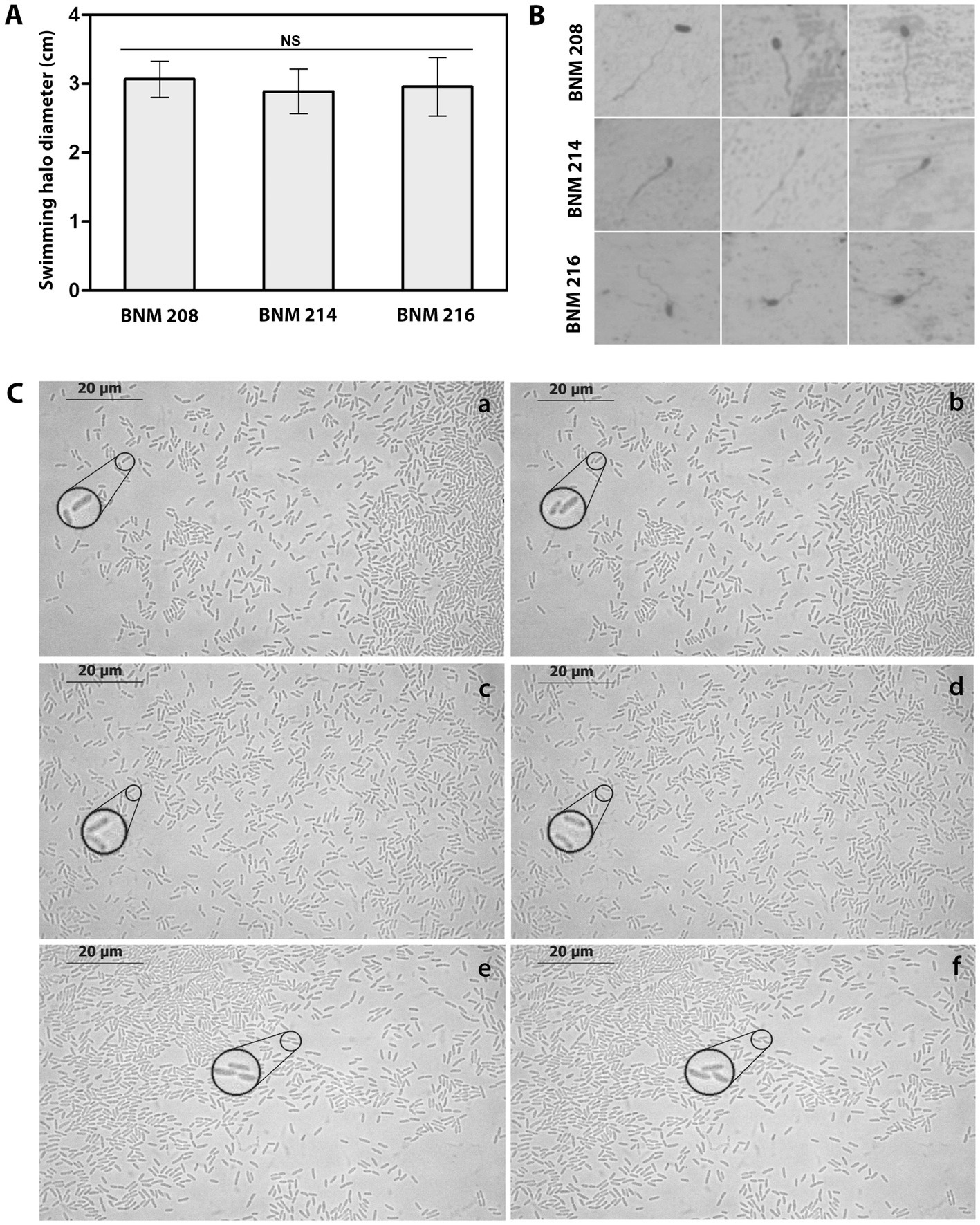
Figure 6. Functional analysis of flagella and T4P. (A) Swimming halo diameter of BNM 208, BNM 214, and BNM 216 cultured in PYM agar. Letters “NS” means no significant difference according to one-way ANOVA followed by Tukey’s test at a significance level of p ≤ 0.05. (B) Microscopic observation (1,000×) of the single polar flagellum of BNM 208, BNM 214, and BNM 216. (C) Twitching motility of BNM 208 (panels a and b), BNM 214 (panels c and d) and BNM 216 (panels e and f) analysed by optical microscopy (1,000×) by time-lapse observations. The right panels (b, d, and f) show images taken 30 s later than the images from the left panels (a, c and e). To easily visualize the typical twitching motility zoom was placed in regions of the observed fields, for each strain, in the reported time lapse.
Twitching motility, which depends on T4P was observed by time-lapse microscopy as characteristic sudden movements by the individual cells of all three strains. Changes in position corresponding to this bacterial movement are shown in Figure 6C and Supplementary Figure 1.
3.2.2. Xanthan
As mentioned in Section 3.1.3, the genomes of BNM 208, BNM 214, and BNM 216 were no different in their gum genes. However, in previous work, we reported that xanthans produced by these strains showed significant different viscosities (Felipe et al., 2018). Since the chain-length of xanthan molecules has been reported to affect xanthan viscosity (Galvan et al., 2013), the EPS molecules synthesised by the three strains were analysed by AFM. According to the images, BNM 208 produced longer xanthan molecules than the other two strains. The shortest xanthan molecules were produced by BNM 216 (Figures 7A–C).
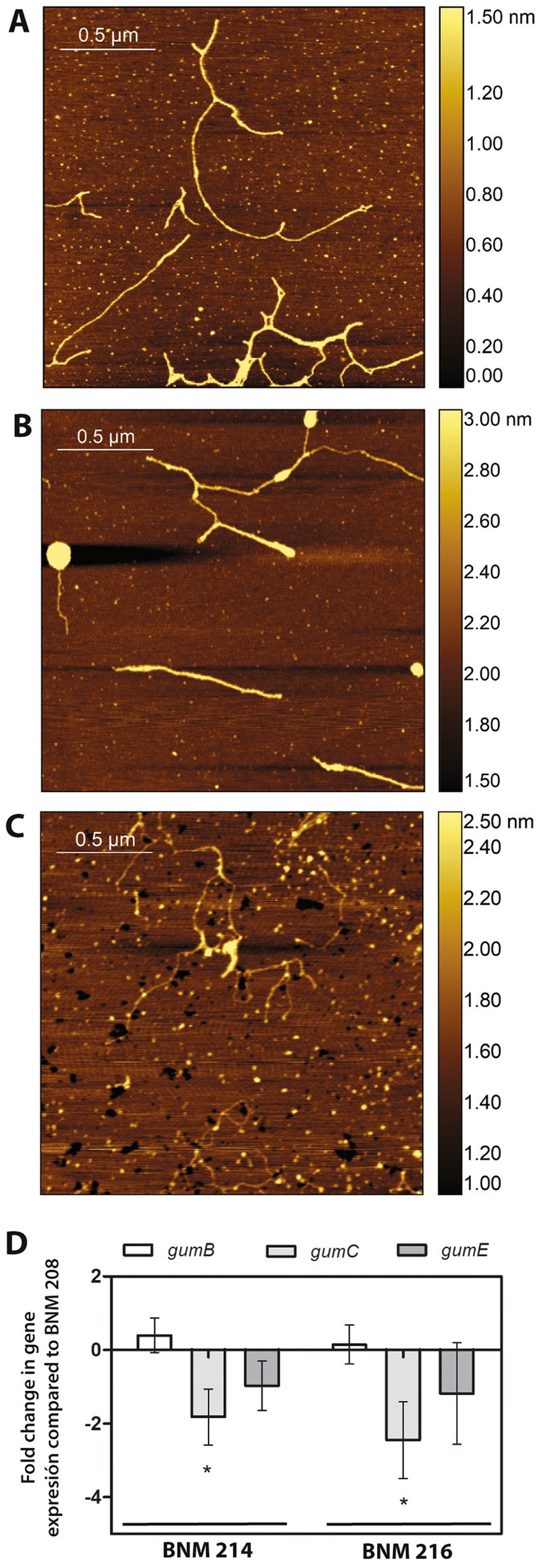
Figure 7. Xanthan analysis. (A–C) Chains of xanthans produced by BNM 208, BNM 214, and BNM 216, respectively. Representative AFM images were obtained from over 200 observed chains/strands. (D) Expression of gumB, gumC, and gumE in BNM 214 and BNM 216 relativised to the respective genes in BNM 208.
Next, qPCR was performed to explore the expression of gumB, gumC and gumE genes, which are involved in xanthan polymerization and export. These genes are related to xanthan chain-length (Galvan et al., 2013). The expression of gumC was approximately 2 and 2.5 times less in BNM 214 and BNM 216 than in BNM 208, respectively. No significant differences were registered in the expression levels of gumB or gumE between the three strains (Figure 7D).
3.3. In planta analysis to verify the host specificity in tomato
Differential tomato genotypes are used to identify races based on HR expression (Jones et al., 2004). The three differential lines used in this study (S. lycopersicum lines Bonny Best and Hawaii 7998 and S. pimpellifolium PI 128216) resulted in susceptible reactions following inoculation with BNM 208, BNM 214, and BNM 216, indicating the three strains belong to race T2 (data not shown).
4. Discussion
X. vesicatoria and X. euvesicatoria were historically considered the predominant bacterial spot lineages worldwide, although X. perforans and X. gardneri are increasingly isolated from areas in America, Africa, the Middle East, and the Indian Ocean (Vancheva et al., 2021). However, the disease caused by X. vesicatoria continues to be a worldwide problem due to the seedborne nature of this pathogen (Kebede et al., 2014; Potnis et al., 2015; Berrueta et al., 2016; Roach et al., 2018; Osdaghi et al., 2021; Potnis, 2021; Ahmad and Ahmad, 2022).
This study aimed to elucidate the factors that could be associated with the development of bacterial spot on tomato plants caused by X. vesicatoria. To achieve this goal, we analysed three strains of X. vesicatoria, BNM 208, BNM 214, and BNM 216, which had shown significant differences in aggressiveness in a previous study (Felipe et al., 2018). The data collected at that time suggested that those differences might be connected to variations in the ability of these strains to form biofilm, which in turn may respond (at least in part) to differences in bacterial motility and in the rheological properties of the xanthan produced by each strain. The most virulent strain, BNM 208, was able to form a mature, homogeneous and well-structured biofilm, while the least virulent one, BNM 216, formed a biofilm that was practically detached and had no characteristics indicating maturity (Felipe et al., 2018).
Since biofilm formation is a complex process involving numerous microbial components and produced/secreted compounds, such as EPSs (An et al., 2020), here, we studied some microbial features associated with aggregation mechanisms. Little is known about the processes that allow X. vesicatoria to survive, multiply and establish itself in its hosts. The same can be said for virulence factors related to host colonization or the evasion of the host’s defense response. Understanding which of these factors are key in pathogenesis and how their repertoire varies from one strain to another could go a long way in explaining differences in aggressiveness. For this reason, we compared the genomes of BNM 208, BNM 214, BNM 216, not only with each other but also with those of 12 other X. vesicatoria strains, which are available in public databases (Supplementary Table S1). Our three strains were confirmed to be X. vesicatoria. Despite their different degrees of aggressiveness and phenotypic characteristics (e.g., motility, adhesion, biofilm-forming ability, relative viscosity of xanthan), their genomes had similar functional patterns in terms of GO and COG categories (Figure 3 and Table 2).
An important virulence factor related to biofilm formation is xanthan. BNM 208, BNM 214 and, BNM 216 contain all the potential CDSs encoding gum proteins, which are involved in the synthesis of xanthan repetitive units, its decoration with non-glycosidic groups (except gumG), polymerization, translocation to the periplasm, and export to the extracellular medium. Therefore, the differences in the viscosity of the xanthans synthesised by these strains (Felipe et al., 2018) cannot be attributed to differences in gum genes was. However, a molecular chain-length analysis showed that the xanthan strands produced by BNM 208 are the longest, while those produced by BNM 216 are the shortest (Figure 7). Moreover, the gumC gene was expressed significantly more in BNM 208 than in the other two strains. These results are consistent with those reported by Galvan et al. (2013), according to whom xanthan with longer molecules results in a more viscous EPS than xanthan with shorter ones.
Xanthan is also important as a surfactant for swarming motility: its amphipathic characteristics reduce the tension between the substrate and the bacterial cells, and thus enhance their spread on solid surfaces (Kearns, 2010). Since we did not observe differences in the CDSs encoding flagellum components (Supplementary Table S3), we cannot attribute the differences observed earlier in swarming motility between BNM 208, BNM 214, and BNM 216 (Felipe et al., 2018) to genetic differences between the three strains. Furthermore, we observed by light microscopy that all three strains have a unique flagellum, the functionality of which was verified by swimming assays (Figures 6A,B). Their differing ability to swarm might be due to the characteristics of the xanthans produced by BNM 208, BNM 214, and BNM 216.
T4P is another factor that influences virulence, the initial stages of biofilm formation and maturation and other bacterial functions in many bacteria species (Jarrell and McBride, 2008; Dunger et al., 2016; Dubnau and Blokesch, 2019; Ellison et al., 2022). Its role in the pathogenesis of Xanthomonas is not the same across all species (Dunger et al., 2016; Shah et al., 2021). The gene(s) that encode(s) PilA remain unknown in X. vesicatoria. Two genes named pilAXAC3241 and pilAXAC3240 have been reported to code for this protein in Xac306 (Dunger et al., 2014). The presence/absence of these genes in the genomes of our strains was studied by genomic and PCR analyses. Homologs of pilAXAC3240 were not found in any of the three strains, and a homologous sequence to pilAXAC3241 was only found in BNM 208 (Supplementary Table S4).
The ability of BNM 208, BNM 214, and BNM 216 to move by twitching was assessed as well, since it depends exclusively on the presence of a functional T4P. Instead of examining the edges of the colonies formed by each strain, as in our previous work (Felipe et al., 2018), we now tracked the movement of individual bacterial cells at very short time intervals (Figure 6C). This technique revealed that BNM 208, BNM 214, and BNM 216 are all capable to move by twitching, whereas earlier results (Felipe et al., 2018) shown this type of movement only in BNM 208 and BNM 214, probably due to the technique used in that previous work had not allowed us to observe movement of individual bacterial cells.
We delve into the analysis of the cluster where the gene(s) encoding pilA should be found according to the literature (Dunger et al., 2014). As in most Xanthomonas genomes, in our strains the genes that code for major pilins are part of a cluster that also contains pilS, pilR, pilB, pilC and pilD. According to Dunger et al. (2014), pilAXAC3241 and pilAXAC3240 are located between pilB and pilC in the pilSRBACD gene cluster. In BNM 208, BNM 214, and BNM 216, we also found a pilSRBCD cluster with some differences between the three strains. BNM 208 has two genes between the pilB-like and pilC-like genes, named pilin-like 1 and pilin-like 2, which might, respectively, correspond to pilAXAC3241 and pilAXAC3240. In contrast, BNM 214 and BNM 216 have six genes between the pilB-like and pilC-like genes, including pilin-like 2. A protein alignment and structure analysis revealed that Pilin-like 1 and Pilin-like 2 proteins in the three strains contain the motif G-1F + 1/E+5 (Figure 5B), and that their predicted tertiary structures are similar to those of PilAXAC3241 and PilAXAC3240 in Xac306. These results could serve as the basis for more accurate identification and functional characterisation of T4P pilins in X. vesicatoria.
The DUF4339 gene, which is homologous to xac3805 of Xac306, is present in the genomes of BNM 208, BNM 214, and BNM 216. Its interest lies in the fact that xac3805 encodes a pilin-like protein that was initially annotated as pilA (da Silva et al., 2002). However, Dunger et al. (2014) later noted that it did not feature the characteristic prepilin leader sequence of type IVa pilins, and it is located far from the pilSRBACD genes. Since the same observations were made for DUF4339 in our X. vesicatoria strains, it might actually be a pilin-encoding paralogue of pilA. Still, further studies should determine whether it encodes a minor or major pilin, and the significance of that pilin for T4P functionality.
Functional studies with Xac306 mutants defective in PilA, PilB, PilZ, or FimX showed that they were impaired in their ability to adhere to the leaf surface, which highlights the importance of these proteins for the formation of stable biofilms (Dunger et al., 2014). Likewise, the involvement of PilA in bacterial adhesion was demonstrated with PilA-defective mutants of Xanthomonas fuscans subsp. fuscans (Darsonval et al., 2009). Other examples are FimA mutants of X. campestris pv. vesicatoria, which exhibited dramatically reduced cell aggregation in vitro and on tomato leaves, and decreased tolerance to ultraviolet light (Ojanen-Reuhs et al., 1997). In BNM 214 and BNM 216, the absence of minor pilin-encoding genes homologous to pilEXac2664, pilXxac2666 and fimTxac2669, and pilAxac3241 (which was confirmed by PCR analysis; see Supplementary Tables S3, S4) could explain why the biofilms formed by these two strains are not as mature and well-structured as the one developed by BNM 208.
There are a few differences in the genomes of the three strains as far as STCRs are concerned. BNM 208 and BNM 214 have a sequence homologous to xac0610 (AAM35499.1). In Xac306, this gene encodes a protein with diguanylate cyclase (DGC) activity, associated with the presence of a GGDEF domain that leads to the production of cyclic diguanosine monophosphate (c-di-GMP) (Oliveira et al., 2015). In addition, the three strains have a sequence homologous to xac1345 (AAM36216.1), which encodes another protein with a GGDEF domain in Xac306 (Andrade et al., 2006). However, its similarity/identity percentage was under 80% in BNM 216 (Supplementary Table S3 and Figure 4). Cyclic-di-GMP is a ubiquitous second messenger in bacteria and a signaling molecule whose intracellular levels are decisive in the transition from a planktonic to a sessile lifestyle that lead to biofilm formation, among other functions (Valentini and Filloux, 2019). In many bacteria, an increase in c-di-GMP levels raises the expression of several factors necessary for the establishment and maintenance of biofilm. Our genomic findings on xac0610 and xac1345 (Supplementary Table S3 and Figure 4) were verified by PCR. The sequence homologous to xac0610 was only amplified in BNM 208, while that homologous to xac1345 was only amplified in BNM 208 and BNM 214. Again, more in-depth research should elucidate the role of these genes in biofilm formation by X. vesicatoria.
On the other hand, since T3Es are associated with host specificity and pathogenicity and play an essential role in the early steps of bacterial interaction with host and non-host plants (Alavi et al., 2008), we analysed the repertoire of CDSs encoding them in BNM 208, BNM 214, and BNM 216 and found several differences between the three strains (Supplementary Table S3 and Figure 4).
Finally, since BNM 208, BNM 214, and BNM 216 are X. vesicatoria and caused symptoms in S. lycopersicum lines Bonny Best and Hawaii 7,998 and in S. pimpellifolium PI 128216, we concluded that all three strains belong to race T2.
The novel findings presented in this paper contribute to our comprehension of the mechanisms underlying biofilm formation and pathogenicity in X. vesicatoria. To our knowledge, this is the first report that studied genotype and phenotype parameters of key virulence factors in this species, including xanthan, flagella and T4P. In conclusion, this study provides information on the biology and pathogenicity of X. vesicatoria, which is still scarce, and contributes to the knowledge of this important plant pathogen. While this work represents a crucial starting point, deeper analyses, such as the construction and examination of mutant strains in various virulence factors or transcriptomic analyses, are needed to further elucidate the underlying mechanisms involved. The final goal of our study is to use this knowledge for designing disease control tools addressed to interfere or attenuate bacterial pathogenicity mitigating the devastating impact of X. vesicatoria infections on crops.
Data availability statement
The datasets presented in this study can be found in online repositories. The names of the repository/repositories and accession number(s) can be found below: https://www.ncbi.nlm.nih.gov/genbank/, PRJNA610021 (BNM 208), PRJNA610030 (BNM 214) and PRJNA610032 (BNM 216).
Author contributions
MB: conceptualisation, research and formal analysis, writing of the original draft, and revision and editing of the manuscript. MP: research and formal analysis, preparation and editing of some figures, contribution to the writing of the original draft, and revision of the final manuscript. JG-C: assembly and analysis of X. vesicatoria genomes, preparation and editing of some figures, contribution to the writing of the original draft, and revision of the final manuscript. VC: gene expression assays. TG: contribution to the analysis of X. vesicatoria genomes and revision of the original and final draft. GD: methodological and analytical support for some experiments and revision of the original and final draft. GM: AFM tests, formal analysis of the images, and review of the final manuscript. AV: research support and review of original and final drafts. AR: trials on tomato plants and revision of the original and final draft. JC: conceptualisation support and assembly and analysis of X. vesicatoria genomes, contribution to the writing of the original draft, and revision of the final manuscript. PY: fund acquisition and project management, conceptualisation, research supervision and formal analysis, and contribution to the correction of the original draft and revision of the final manuscript. All authors contributed to the article and approved the submitted version.
Funding
This work was funding by the Fondo para la Investigación Científica y Tecnológica (FONCyT) of the Agencia Nacional de Promoción de la Investigación, el Desarrollo Tecnológico y la Innovación (Argentina): PICT 2019 N° 3274.
Acknowledgments
MB, VC, GD, GM, AV, and PY are Career Investigators of the Consejo Nacional de Investigaciones Científicas y Técnicas (CONICET), Argentina. MP was supported by a doctoral fellowship from CONICET, Argentina. TG was supported by a doctoral fellowship from Agencia Nacional de Promoción Científica y Tecnológica, Argentina. JC is Investigator of Consejo Superior de Investigaciones Científicas (CSIC), España. JG-C and JC participated in this work under PID2021-123600OR-C41 project by MCIN/AEI/10.13039/501100011033. The authors thank Natalia Mielnichuk for their contribution in reviewing this manuscript.
Conflict of interest
The authors declare that the research was conducted in the absence of any commercial or financial relationships that could be construed as a potential conflict of interest.
Publisher’s note
All claims expressed in this article are solely those of the authors and do not necessarily represent those of their affiliated organizations, or those of the publisher, the editors and the reviewers. Any product that may be evaluated in this article, or claim that may be made by its manufacturer, is not guaranteed or endorsed by the publisher.
Supplementary material
The Supplementary material for this article can be found online at: https://www.frontiersin.org/articles/10.3389/fmicb.2023.1185368/full#supplementary-material
Footnotes
References
Ahmad, I., and Ahmad, M. (2022). Bacterial spot caused by Xanthomonas vesicatoria devastated tomato production of Khyber Pakhtunkhwa-Pakistan. Int. J. Plant Pathol. 11, 77–95. doi: 10.33687/phytopath.011.01.4071
Alavi, S. M., Sanjari, S., Durand, F., Brin, C., Manceau, C., and Poussier, S. (2008). Assessment of the genetic diversity of Xanthomonas axonopodis pv. Phaseoli and Xanthomonas fuscans subsp. fuscans as a basis to identify putative pathogenicity genes and a type III secretion system of the SPI-1 family by multiple suppression subtractive hybridizations. Appl. Environ. Microbiol. 74, 3295–3301. doi: 10.1128/AEM.02507-07
Almagro Armenteros, J. J., Tsirigos, K. D., Sønderby, C. K., Petersen, T. N., Winther, O., Brunak, S., et al. (2019). SignalP 5.0 improves signal peptide predictions using deep neural networks. Nat. Biotechnol. 37, 420–423. doi: 10.1038/s41587-019-0036-z
An, S.-Q., Potnis, N., Dow, M., Vorhölter, F.-J., He, Y.-Q., Becker, A., et al. (2020). Mechanistic insights into host adaptation, virulence and epidemiology of the phytopathogen Xanthomonas. FEMS Microbiol. Rev. 44, 1–32. doi: 10.1093/femsre/fuz024
Andrade, M. O., Alegria, M. C., Guzzo, C. R., Docena, C., Pareda Rosa, M. C., Ramos, C. H., et al. (2006). The HD-GYP domain of RpfG mediates a direct linkage between the Rpf quorum-sensing pathway and a subset of diguanylate cyclase proteins in the phytopathogen Xanthomonas axonopodis pv citri. Mol. Microbiol. 62, 537–551. doi: 10.1111/j.1365-2958.2006.05386.x
Andrews, S., Krueger, F., Seconds-Pichon, A., Biggins, F., and Wingett, S. (2010). FastQC: a quality control tool for high throughput sequence data. Available at: https://www.bioinformatics.babraham.ac.uk/projects/fastqc/ (Accessed April 21, 2022).
Aslam, S. N., Newman, M. A., Erbs, G., Morrissey, K. L., Chinchilla, D., Boller, T., et al. (2008). Bacterial polysaccharides suppress induced innate immunity by calcium chelation. Curr. Biol. 18, 1078–1083. doi: 10.1016/j.cub.2008.06.061
Astua-Monge, G., Minsavage, G. V., Stall, R. E., Vallejos, C. E., Davis, M. J., and Jones, J. B. (2000). Xv4-vrxv4: a new gene-for-gene interaction identified between Xanthomonas campestris pv. Vesicatoria race T3 and the wild tomato relative Lycopersicon pennellii. Mol. Plant-Microbe Interact. 13, 1346–1355. doi: 10.1094/MPMI.2000.13.12.1346
Barak, J. D., Vancheva, T., Lefeuvre, P., Jones, J. B., Timilsina, S., Minsavage, G. V., et al. (2016). Whole-genome sequences of Xanthomonas euvesicatoria strains clarify taxonomy and reveal a stepwise erosion of type 3 effectors. Front. Plant Sci. 7:1805. doi: 10.3389/fpls.2016.01805
Bayer-Santos, E., Ceseti, L. D. M., Farah, C. S., and Alvarez-Martinez, C. E. (2019). Distribution, function and regulation of type 6 secretion systems of Xanthomonadales. Front. Microbiol. 10:1635. doi: 10.3389/fmicb.2019.01635
Berrueta, M. C., Giménez, G., Galván, G. A., and Borges, A. (2016). New sources of partial resistance to bacterial spot race T2 in processing tomatoes. Hortic. Bras. 34, 326–332. doi: 10.1590/S0102-05362016003004
Bianco, M. I., Toum, L., Yaryura, P. M., Mielnichuk, N., Gudesblat, G. E., Roeschlin, R., et al. (2016). Xanthan pyruvilation is essential for the virulence of Xanthomonas campestris pv. Campestris. Mol. Plant-Microbe Interact. 29, 688–699. doi: 10.1094/MPMI-06-16-0106-R
Blum, M., Chang, H.-Y., Chuguransky, S., Grego, T., Kandasaamy, S., Mitchell, A., et al. (2020). The InterPro protein families and domains database: 20 years on. Nucleic Acids Res. 49(D1), D344-D354. doi: 10.1093/nar/gku1019
Bolger, A. M., Lohse, M., and Usadel, B. (2014). Trimmomatic: a flexible trimmer for illumina sequence data. Bioinformatics 30, 2114–2120. doi: 10.1093/bioinformatics/btu170
Burdman, S., Bahar, O., Parker, J. K., and De La Fuente, L. (2011). Involvement of type IV pili in pathogenicity of plant pathogenic bacteria. Genes 2, 706–735. doi: 10.3390/genes2040706
Burrows, L. L. (2012). Pseudomonas aeruginosa twitching motility: type IV pili in action. Annu. Rev. Microbiol. 66, 493–520. doi: 10.1146/annurev-micro-092611-150055
Büttner, D. (2016). Behind the lines–actions of bacterial type III effector proteins in plant cells. FEMS Microbiol. Rev. 40, 894–937. doi: 10.1093/femsre/fuw026
Cadmus, M. C., Rogovin, S. P., Burton, K. A., Pittsley, J. E., Knutson, C. A., and Jeanes, A. (1976). Colonial variation in Xanthomonas campestris NRRL B-1459 and characterization of the polysaccharide from a variant strain. Can. J. Microbiol. 22, 942–948. doi: 10.1139/m76-136
Caserta, R., Takita, M. A., Targon, M., Rosselli-Murai, L., De Souza, A., Peroni, L., et al. (2010). Expression of Xylella fastidiosa fimbrial and afimbrial proteins during biofilm formation. Appl. Environ. Microbiol. 76, 4250–4259. doi: 10.1128/AEM.02114-09
Castiblanco, L. F., and Sundin, G. W. (2016). New insights on molecular regulation of biofilm formation in plant-associated bacteria. J. Integr. Plant Biol. 58, 362–372. doi: 10.1111/jipb.12428
Chevreux, B., Wetter, T., and Suhai, S. (1999). “Genome sequence assembly using trace signals and additional sequence information,” in Proceedings of the German Conference on Bioinformatics, Hannover, 99, 45–56.
Conforte, V. P., Yaryura, P. M., Bianco, M. I., Rodríguez, M. C., Daglio, Y., Prieto, E., et al. (2019). Changes in the physico-chemical properties of the xanthan produced by Xanthomonas citri subsp. citri in grapefruit leaf extract. Glycobiology 29, 269–278. doi: 10.1093/glycob/cwy114
Constantin, E., Cleenwerck, I., Maes, M., Baeyen, S., Van Malderghem, C., De Vos, P., et al. (2016). Genetic characterization of strains named as Xanthomonas axonopodis pv. Dieffenbachiae leads to a taxonomic revision of the X. axonopodis species complex. Plant Pathol. 65, 792–806. doi: 10.1111/ppa.12461
Cook, A., and Guevara, Y. (1984). Hypersensitivity in Capsicum chacoense to race 1 of the bacterial spot pathogen of pepper. Plant Dis. 68, 329–330. doi: 10.1094/PD-68-329
da Silva, A. R., Ferro, J. A., Reinach, F., Farah, C., Furlan, L., Quaggio, R., et al. (2002). Comparison of the genomes of two Xanthomonas pathogens with differing host specificities. Nature 417, 459–463. doi: 10.1038/417459a
Darsonval, A., Darrasse, A., Durand, K., Bureau, C., Cesbron, S., and Jacques, M.-A. (2009). Adhesion and fitness in the bean phyllosphere and transmission to seed of Xanthomonas fuscans subsp. fuscans. Mol. Plant-Microbe Interact. 22, 747–757. doi: 10.1094/MPMI-22-6-0747
Di Rienzo, J. A. (2009). InfoStat versión 2009. Grupo InfoStat, FCA, Universidad Nacional de Córdoba, Argentina. Available at: http://www.infostat.com.ar.
Di Rienzo, J.A. (2012). fgStatistics. Statistical software for the analysis of experiments of functional genomics. Available at: http://sites.google.com/site/fgStatistics/.
Dow, J. M., Crossman, L., Findlay, K., He, Y. Q., Feng, J. X., and Tang, J. L. (2003). Biofilm dispersal in Xanthomonas campestris is controlled by cell-cell signaling and is required for full virulence to plants. Proc. Natl. Acad. Sci. 100, 10995–11000. doi: 10.1073/pnas.1833360100
Duan, Q., Zhou, M., Zhu, L., and Zhu, G. (2013). Flagella and bacterial pathogenicity. J. Basic Microbiol. 53, 1–8. doi: 10.1002/jobm.201100335
Dubnau, D., and Blokesch, M. (2019). Mechanisms of DNA uptake by naturally competent bacteria. Annu. Rev. Genet. 53, 217–237. doi: 10.1146/annurev-genet-112618-043641
Dunger, G., Guzzo, C. R., Andrade, M. O., Jones, J. B., and Farah, C. S. (2014). Xanthomonas citri subsp. citri type IV pilus is required for twitching motility, biofilm development, and adherence. Mol. Plant-Microbe Interact. 27, 1132–1147. doi: 10.1094/MPMI-06-14-0184-R
Dunger, G., Llontop, E., Guzzo, C. R., and Farah, C. S. (2016). The Xanthomonas type IV pilus. Curr. Opin. Microbiol. 30, 88–97. doi: 10.1016/j.mib.2016.01.007
Ellison, C. K., Whitfield, G. B., and Brun, Y. V. (2022). Type IV pili: dynamic bacterial nanomachines. FEMS Microbiol. Rev. 46:fuab053. doi: 10.1093/femsre/fuab053
Felipe, V., Romero, A., Montecchia, M. S., Vojnov, A. A., Bianco, M. I., and Yaryura, P. M. (2018). Xanthomonas vesicatoria virulence factors involved in early stages of bacterial spot development in tomato. Plant Pathol. 67, 1936–1943. doi: 10.1111/ppa.12905
Galata, V., Fehlmann, T., Backes, C., and Keller, A. (2019). PLSDB: a resource of complete bacterial plasmids. Nucleic Acids Res. 47, D195–D202. doi: 10.1093/nar/gky1050
Galvan, E. M., Ielmini, M. V., Patel, Y. N., Bianco, M. I., Franceschini, E. A., Schneider, J. C., et al. (2013). Xanthan chain length is modulated by increasing the availability of the polysaccharide copolymerase protein GumC and the outer membrane polysaccharide export protein GumB. Glycobiology 23, 259–272. doi: 10.1093/glycob/cws146
Garita-Cambronero, J., Sena-Velez, M., Ferragud, E., Sabuquillo, P., Redondo, C., and Cubero, J. (2019). Xanthomonas citri subsp. citri and Xanthomonas arboricola pv. Pruni: comparative analysis of two pathogens producing similar symptoms in different host plants. PLoS One 14:e0219797. doi: 10.1371/journal.pone.0219797
Gaxiola, E. G. (2000). Distribución de la mancha bacteriana (Xanthomonas campestris pv. vesicatoria) (Doidge) Dye. y su detección de resistencia a los bactericidas (Doctoral dissertation, Tesis de Licenciatura, Universidad Autónoma de Sinaloa, Culiacán, Sinaloa).
Gore, J., and O'Garro, L. (1999). Xanthomonas campestris pv. Vesicatoria from bell pepper and tomato in Barbados undergoes changes in race structure, virulence and sensitivity to chemical control agents. J. Phytopathol. 147, 397–402. doi: 10.1111/j.1439-0434.1999.tb03840.x
Gurevich, A., Saveliev, V., Vyahhi, N., and Tesler, G. (2013). QUAST: quality assessment tool for genome assemblies. Bioinformatics 29, 1072–1075. doi: 10.1093/bioinformatics/btt086
Haiko, J., and Westerlund-Wikström, B. (2013). The role of the bacterial flagellum in adhesion and virulence. Biology 2, 1242–1267. doi: 10.3390/biology2041242
Ikeda, S., Gohtani, S., Nishinari, K., and Zhong, Q. (2012). Single molecules and networks of xanthan gum probed by atomic force microscopy. Food Sci. Technol. Res. 18, 741–745. doi: 10.3136/fstr.18.741
Jarrell, K. F., and McBride, M. J. (2008). The surprisingly diverse ways that prokaryotes move. Nat. Rev. Microbiol. 6, 466–476. doi: 10.1038/nrmicro1900
Jones, J. B., Lacy, G. H., Bouzar, H., Stall, R. E., and Schaad, N. W. (2004). Reclassification of the Xanthomonads associated with bacterial spot disease of tomato and pepper. Syst. Appl. Microbiol. 27, 755–762. doi: 10.1078/0723202042369884
Kearns, D. B. (2010). A field guide to bacterial swarming motility. Nat. Rev. Microbiol. 8, 634–644. doi: 10.1038/nrmicro2405
Kebede, M., Timilsina, S., Ayalew, A., Admassu, B., Potnis, N., Minsavage, G. V., et al. (2014). Molecular characterization of Xanthomonas strains responsible for bacterial spot of tomato in Ethiopia. Eur. J. Plant Pathol. 140, 677–688. doi: 10.1007/s10658-014-0497-3
Kodaka, H., Armfield, A. Y., Lombard, G. L., and Dowell, V. R. Jr. (1982). Practical procedure for demonstrating bacterial flagella. J. Clin. Microbiol. 16, 948–952. doi: 10.1128/jcm.16.5.948-952.1982
Krogh, A., Larsson, B., Von Heijne, G., and Sonnhammer, E. L. (2001). Predicting transmembrane protein topology with a hidden Markov model: application to complete genomes. J. Mol. Biol. 305, 567–580. doi: 10.1006/jmbi.2000.4315
Lin, H., Deng, E.-Z., Ding, H., Chen, W., and Chou, K.-C. (2014). iPro54-PseKNC: a sequence-based predictor for identifying sigma-54 promoters in prokaryote with pseudo k-tuple nucleotide composition. Nucleic Acids Res 42(21):12961–12972.
Llontop, E. E., Cenens, W., Favaro, D. C., Sgro, G. G., Salinas, R. K., Guzzo, C. R., et al. (2021). The PilB-PilZ-FimX regulatory complex of the type IV pilus from Xanthomonas citri. PLoS Pathog. 17:e1009808. doi: 10.1371/journal.ppat.1009808
Lorenz, C., Kirchner, O., Egler, M., Stuttmann, J., Bonas, U., and Buttner, D. (2008). HpaA from Xanthomonas is a regulator of type III secretion. Mol. Microbiol. 69, 344–360. doi: 10.1111/j.1365-2958.2008.06280.x
Lu, S., Wang, J., Chitsaz, F., Derbyshire, M. K., Geer, R. C., Gonzales, N. R., et al. (2020). CDD/SPARCLE: the conserved domain database in 2020. Nucleic Acids Res. 48, D265–D268. doi: 10.1093/nar/gkz991
Malamud, F., Torres, P. S., Roeschlin, R., Rigano, L. A., Enrique, R., Bonomi, H. R., et al. (2011). The Xanthomonas axonopodis pv. Citri flagellum is required for mature biofilm and canker development. Microbiology 157, 819–829. doi: 10.1099/mic.0.044255-0
Nguyen, Y., Sugiman-Marangos, S., Harvey, H., Bell, S. D., Charlton, C. L., Junop, M. S., et al. (2015). Pseudomonas aeruginosa minor pilins prime type IVa pilus assembly and promote surface display of the PilY1 adhesin. J. Biol. Chem. 290, 601–611. doi: 10.1074/jbc.M114.616904
Ojanen-Reuhs, T., Kalkkinen, N., Westerlund-Wikström, B., Van Doorn, J., Haahtela, K., Nurmiaho-Lassila, E.-L., et al. (1997). Characterization of the fimA gene encoding bundle-forming fimbriae of the plant pathogen Xanthomonas campestris pv. Vesicatoria. J. Bacteriol. 179, 1280–1290. doi: 10.1128/jb.179.4.1280-1290.1997
Oliveira, M. C., Teixeira, R. D., Andrade, M. O., Pinheiro, G. M., Ramos, C. H., and Farah, C. S. (2015). Cooperative substrate binding by a diguanylate cyclase. J. Mol. Biol. 427, 415–432. doi: 10.1016/j.jmb.2014.11.012
Osdaghi, E., Jones, J. B., Sharma, A., Goss, E. M., Abrahamian, P., Newberry, E. A., et al. (2021). A centenary for bacterial spot of tomato and pepper. Mol. Plant Pathol. 22, 1500–1519. doi: 10.1111/mpp.13125
Page, A. J., Cummins, C. A., Hunt, M., Wong, V. K., Reuter, S., Holden, M. T., et al. (2015). Roary: rapid large-scale prokaryote pan genome analysis. Bioinformatics 31, 3691–3693. doi: 10.1093/bioinformatics/btv421
Pfaffl, M. W., Horgan, G. W., and Dempfle, L. (2002). Relative expression software tool (REST©) for group-wise comparison and statistical analysis of relative expression results in real-time PCR. Nucleic Acids Res. 30, e36–e336. doi: 10.1093/nar/30.9.e36
Picchi, S. C., Granato, L. M., Franzini, M. J. F., Andrade, M. O., Takita, M. A., Machado, M. A., et al. (2021). Modified monosaccharides content of xanthan gum impairs Citrus canker disease by affecting the epiphytic lifestyle of Xanthomonas citri subsp. citri. Microorganisms 9:1176. doi: 10.3390/microorganisms9061176
Picchi, S., Takita, M., Coletta-Filho, H., Machado, M., and de Souza, A. (2016). N-acetylcysteine interferes with the biofilm formation, motility and epiphytic behaviour of Xanthomonas citri subsp. citri. Plant Pathol. 65, 561–569. doi: 10.1111/ppa.12430
Pritchard, L., Glover, R. H., Humphris, S., Elphinstone, J. G., and Toth, I. K. (2016). CGenomics and taxonomy in diagnostics for food security: soft-rotting enterobacterial plant pathogens. Analytical Methods 8(1):12–24.
Potnis, N. (2021). Harnessing eco-evolutionary dynamics of Xanthomonads on tomato and pepper to tackle new problems of an old disease. Annu. Rev. Phytopathol. 59, 289–310. doi: 10.1146/annurev-phyto-020620-101612
Potnis, N., Krasileva, K., Chow, V., Almeida, N. F., Patil, P. B., Ryan, R. P., et al. (2011). Comparative genomics reveals diversity among xanthomonads infecting tomato and pepper. BMC Genomics 12:146. doi: 10.1186/1471-2164-12-146
Potnis, N., Timilsina, S., Strayer, A., Shantharaj, D., Barak, J. D., Paret, M. L., et al. (2015). Bacterial spot of tomato and pepper: diverse Xanthomonas species with a wide variety of virulence factors posing a worldwide challenge. Mol. Plant Pathol. 16, 907–920. doi: 10.1111/mpp.12244
Ramakers, C., Ruijter, J. M., Deprez, R. H. L., and Moorman, A. F. (2003). Assumption-free analysis of quantitative real-time polymerase chain reaction (PCR) data. Neurosci. Lett. 339, 62–66. doi: 10.1016/s0304-3940(02)01423-4
Rigano, L. A., Payette, C., Brouillard, G., Marano, M. R., Abramowicz, L., Torres, P. S., et al. (2007b). Bacterial cyclic beta-(1,2)-glucan acts in systemic suppression of plant immune responses. Plant Cell 19, 2077–2089. doi: 10.1105/tpc.106.047944
Rigano, L. A., Siciliano, F., Enrique, R., Sendin, L., Filippone, P., Torres, P. S., et al. (2007a). Biofilm formation, epiphytic fitness, and canker development in Xanthomonas axonopodis pv. Citri. Mol. Plant-Microbe Interact. 20, 1222–1230. doi: 10.1094/MPMI-20-10-1222
Ritchie, D. F., and Dittapongpitch, V. (1991). Copper-and streptomycin-resistant strains and host differentiated races of Xanthomonas campestris pv. Vesicatoria in North Carolina. Plant Dis. 75, 733–736. doi: 10.1094/PD-75-0733
Roach, R., Mann, R., Gambley, C. G., Shivas, R. G., and Rodoni, B. (2018). Identification of Xanthomonas species associated with bacterial leaf spot of tomato, capsicum and chilli crops in eastern Australia. Eur. J. Plant Pathol. 150, 595–608. doi: 10.1007/s10658-017-1303-9
Romero, A. M., Correa, O. S., Moccia, S., and Rivas, J. G. (2003). Effect of Azospirillum-mediated plant growth promotion on the development of bacterial diseases on fresh-market and cherry tomato. J. Appl. Microbiol. 95, 832–838. doi: 10.1046/j.1365-2672.2003.02053.x
Ronish, L. A., Lillehoj, E., Fields, J. K., Sundberg, E. J., and Piepenbrink, K. H. (2019). The structure of PilA from Acinetobacter baumannii AB5075 suggests a mechanism for functional specialization in Acinetobacter type IV pili. J. Biol. Chem. 294, 218–230. doi: 10.1074/jbc.RA118.005814
Sahin, F., and Miller, S. (1998). Resistance in Capsicum pubescens to Xanthomonas campestris pv. Vesicatoria pepper race 6. Plant Dis. 82, 794–799. doi: 10.1094/PDIS.1998.82.7.794
Schwartz, A. R., Potnis, N., Timilsina, S., Wilson, M., Patane, J., Martins, J. J., et al. (2015). Phylogenomics of Xanthomonas field strains infecting pepper and tomato reveals diversity in effector repertoires and identifies determinants of host specificity. Front. Microbiol. 6:535. doi: 10.3389/fmicb.2015.00535
Scott, J. W., Jones, J. B., Somodi, G. C., and Stall, R. E. (1995). Screening tomato accessions for resistance to Xanthomonas campestris pv. Vesicatoria, race T3. HortScience 30, 579–581. doi: 10.21273/HORTSCI.30.3.579
Seemann, T. (2014). Prokka: rapid prokaryotic genome annotation. Bioinformatics 30, 2068–2069. doi: 10.1093/bioinformatics/btu153
Shah, S. M. A., Khojasteh, M., Wang, Q., Taghavi, S. M., Xu, Z., Khodaygan, P., et al. (2021). Genomics-enabled novel insight into the pathovar-specific population structure of the bacterial leaf streak pathogen Xanthomonas translucens in small grain cereals. Front. Microbiol. 12:674952. doi: 10.3389/fmicb.2021.674952
Stall, R. E., Jones, J. B., and Minsavage, G. V. (2009). Durability of resistance in tomato and pepper to xanthomonads causing bacterial spot. Annu. Rev. Phytopathol. 47, 265–284. doi: 10.1146/annurev-phyto-080508-081752
Tamura, K., Stecher, G., and Kumar, S. (2021). MEGA11: molecular evolutionary genetics analysis version 11. Mol. Biol. Evol. 38, 3022–3027. doi: 10.1093/molbev/msab120
Tatusov, R. L., Natale, D. A., Garkavtsev, I. V., Tatusova, T. A., Shankavaram, U. T., Rao, B. S., et al. (2001). The COG database: new developments in phylogenetic classification of proteins from complete genomes. Nucleic Acids Res. 29, 22–28. doi: 10.1093/nar/29.1.22
Tatusova, T., DiCuccio, M., Badretdin, A., Chetvernin, V., Nawrocki, E. P., Zaslavsky, L., et al. (2016). NCBI prokaryotic genome annotation pipeline. Nucleic Acids Res. 44, 6614–6624. doi: 10.1093/nar/gkw569
The Gene Ontology Consortium (2021). The Gene Ontology resource: enriching a gold mine. Nucleic Acids Res. 49, D325–D334. doi: 10.1093/nar/gkaa1113
Thieme, F., Koebnik, R., Bekel, T., Berger, C., Boch, J., Buttner, D., et al. (2005). Insights into genome plasticity and pathogenicity of the plant pathogenic bacterium Xanthomonas campestris pv. Vesicatoria revealed by the complete genome sequence. J. Bacteriol. 187, 7254–7266. doi: 10.1128/JB.187.21.7254-7266.2005
Timilsina, S., Potnis, N., Newberry, E. A., Liyanapathiranage, P., Iruegas-Bocardo, F., White, F. F., et al. (2020). Xanthomonas diversity, virulence and plant–pathogen interactions. Nat. Rev. Microbiol. 18, 415–427. doi: 10.1038/s41579-020-0361-8
Torres, P. S., Malamud, F., Rigano, L. A., Russo, D. M., Marano, M. R., Castagnaro, A. P., et al. (2007). Controlled synthesis of the DSF cell-cell signal is required for biofilm formation and virulence in Xanthomonas campestris. Environ. Microbiol. 9, 2101–2109. doi: 10.1111/j.1462-2920.2007.01332.x
Valentini, M., and Filloux, A. (2019). Multiple roles of c-di-GMP signaling in bacterial pathogenesis. Annu. Rev. Microbiol. 73, 387–406. doi: 10.1146/annurev-micro-020518-115555
Vancheva, T., Bogatzevska, N., Moncheva, P., Mitrev, S., Vernière, C., and Koebnik, R. (2021). Molecular epidemiology of Xanthomonas euvesicatoria strains from the Balkan Peninsula revealed by a new multiple-locus variable-number tandem-repeat analysis scheme. Microorganisms 9:536. doi: 10.3390/microorganisms9030536
Whalen, M. C., Wang, J. F., Carland, F. M., Heiskell, M. E., Dahlbeck, D., Minsavage, G. V., et al. (1993). Avirulence gene avrRxv from Xanthomonas campestris pv. Vesicatoria specifies resistance on tomato line Hawaii 7998. Mol. Plant-Microbe Interact. 6, 616–627. doi: 10.1094/MPMI-6-616
Wood, T. K. (2013). Precedence for the structural role of flagella in biofilms. MBio 4, e00225–e00213. doi: 10.1128/mBio.00225-13
Yaryura, P. M., Conforte, V. P., Malamud, F., Roeschlin, R., de Pino, V., Castagnaro, A. P., et al. (2014). XbmR, a new transcription factor involved in the regulation of chemotaxis, biofilm formation and virulence in Xanthomonas citri subsp. citri. Environ. Microbiol. 17, 4164–4176. doi: 10.1111/1462-2920.12684
Keywords: Xanthomonas vesicatoria , bacterial spot, xanthan, bacterial motility, type IV pili, flagellum, tomato
Citation: Bianco MI, Ponso MA, Garita-Cambronero J, Conforte VP, Galván TE, Dunger G, Morales GM, Vojnov AA, Romero AM, Cubero J and Yaryura PM (2023) Genomic and phenotypic insight into Xanthomonas vesicatoria strains with different aggressiveness on tomato. Front. Microbiol. 14:1185368. doi: 10.3389/fmicb.2023.1185368
Edited by:
Jeffrey Jones, University of Florida, United StatesReviewed by:
Jinyun Li, University of Florida, United StatesAnuj Sharma, University of Florida, United States
Copyright © 2023 Bianco, Ponso, Garita-Cambronero, Conforte, Galván, Dunger, Morales, Vojnov, Romero, Cubero and Yaryura. This is an open-access article distributed under the terms of the Creative Commons Attribution License (CC BY). The use, distribution or reproduction in other forums is permitted, provided the original author(s) and the copyright owner(s) are credited and that the original publication in this journal is cited, in accordance with accepted academic practice. No use, distribution or reproduction is permitted which does not comply with these terms.
*Correspondence: Pablo Marcelo Yaryura, cHlhcnl1cmFAdW52bS5lZHUuYXI=; María Isabel Bianco, aWJpYW5jb0BjZW50cm9taWxzdGVpbi5vcmcuYXI=
†These authors share first authorship
‡These authors share senior and last authorship