- Institute of Animal Husbandry and Veterinary Science, Shanghai Academy of Agricultural Sciences, Shanghai, China
Bile acids(BAs) are important components of bile and play a significant role in fat metabolism. However, there is currently no systematic evaluation of the use of BAs as feed additives for geese.This study aimed to investigate the effects of adding BAs to goose feed on growth performance, lipid metabolism, intestinal morphology, mucosal barrier function, and cecal microbiota. A total of 168 28-day-old geese were randomly assigned to four treatment groups and fed diets supplemented with 0, 75, 150, or 300 mg/kg of BAs for 28 days. The addition of 75 and 150 mg/kg of BAs significantly improved the feed/gain (F/G) (p < 0.05).The addition of BAs decreased abdominal fat percentage and serum total cholesterol (TC) levels, with 150 mg/kg of BAs significantly reducing serum triglyceride levels and increased expression of Farnesoid X Receptor (FXR) mRNA in the liver(p < 0.05), 300 mg/kg of BAs significantly increasing the expression level of liver peroxisome proliferator-activated receptor α (PPARα) (p < 0.05). In terms of intestinal morphology and mucosal barrier function, 150 mg/kg of BAs significantly increased villus height (VH) and VH/crypt depth (CD) in the jejunum (p < 0.05). The addition of 150 and 300 mg/kg of BAs significantly reduced the CD in the ileum, while increasing VH and VH/CD (p<0.05). Additionally, the addition of 150 and 300 mg/kg of BAs significantly increased the expression levels of zonula occludens-1 (ZO-1) and occludin in the jejunum. Simultaneously 150mg/kg and 300mg/kg BAs increased the total short-chain fatty acids (SCFA) concentrations in the jejunum and cecum(p < 0.05).Supplementation with BAs resulted in a significant increase in the ɑ-diversity of cecal microbiota and a decrease in the abundance of Proteobacteria in the cecum. The addition of 150 mg/kg of BAs significantly reduced the abundance of Bacteroidetes and increased the abundance of Firmicutes. Moreover,Linear discriminant analysis Effect Size analysis (LEfSe) showed that the abundances of bacteria producing SCFA and bile salt hydrolases (BSH) were increased in the BAs-treated group. Furthermore, Spearman’s analysis showed that the genus Balutia, which is negatively correlated with visceral fat area, was positively correlated with serum high-density lipoprotein cholesterol (HDL-C), while Clostridium was positively correlated with intestinal VH and VH/CD. In conclusion, BAs can be considered an effective feed additive for geese, as they increased SCFA concentration, improve lipid metabolism and intestinal health by enhancing the intestinal mucosal barrier, improving intestinal morphology, and altering the cecal microbiota structure.
Introduction
In intensive poultry farming, it is common to add fat to feed to increase its energy density, improve palatability, and enhance poultry’s feed intake, thereby improving production performance (Ma et al., 2022; Saminathan et al., 2022). However, during the early stages of development, poultry have underdeveloped digestive systems, which limits their ability to digest and utilize fats (Iji et al., 2001; Vertiprakhov et al., 2022). When they consume more energy than they need, it can lead to increased fat accumulation in their abdominal area, as well as health problems such as fatty liver (Sanz, 1999; Crespo and Esteve-Garcia, 2001). This places a significant burden on their liver and intestines. Therefore, it is feasible to develop additives that can help poultry digest fats while ensuring their production performance. For example, adding probiotics or prebiotics can improve gut health and enhance the digestibility of fats, while antibiotics or antibiotic alternatives can also be used as growth promoters to improve poultry digestive performance (Duskaev et al., 2021; Wang et al., 2022).
Bile acids, which are the primary component of bile, are synthesized by the liver and stored in the gallbladder (Hofmann and Hagey, 2008). As a fat emulsifier, BAs effectively emulsify lipids into minute chylomicron particles, which augment lipid metabolism by promoting contact with lipases (Lefebvre et al., 2009). Furthermore, BAs act as signaling molecules that bind to the farnesoid X receptor (FXR), which plays a role in regulating lipid metabolism (Arthur et al., 2019; Li et al., 2019). These mechanisms promote the absorption of lipids and fat-soluble vitamins, while also mitigating the negative impact of a high-fat diet on lipid deposition (Saeed et al., 2017; Adachi, 2018), resulting in improved growth performance (Watanabe et al., 2006). Additionally, BAs have been found to play a critical role in physiological functions such as inflammation and energy homeostasis (Reschly et al., 2008; Wu et al., 2019). Recent studies have emphasized the importance of studying how BAs and the gut microbiota of poultry interact with each other. BAs have a significant impact on the composition and ratio of intestinal microbiota, which can affect their metabolism and ultimately influence the metabolism, immune function, and overall health of the host. This interaction between BAs and intestinal microbiota is a crucial factor that influences the health of the host (Liu et al., 2022a,b). Additionally, the intestinal microbiota can produce beneficial secondary metabolites, such as BSH, through the metabolism of BAs, which can further contribute to the health of the host (Burns et al., 2021). There is evidence to suggest that BAs can stimulate the growth of beneficial bacteria and hinder the growth of harmful bacteria in the gut, which may reduce the risk of enteric infections (Inagaki et al., 2006; Gao et al., 2022). Research on the impact of BAS on intestinal microbiota can help to understand the interaction mechanism between intestinal microbiota and the host, and provide important theoretical support for the development of new health management methods and disease treatment strategies.
In 2014, BAs were granted approval as a feed additive and can now be utilized in broiler chickens, weaned piglets, and freshwater fish. However, no research has been conducted on the effectiveness and safety of adding BAs to geese.
Materials and methods
Animal ethics
Animal handling and treatment were carried out in accordance with the Chinese Animal Welfare Guidelines. This research was approved by the Laboratory Animal Ethics Committee of the Shanghai Academy of Agricultural Sciences (SAASPZ0522046).
Animals and experimental design
BAs used in this experiment were purchased from Zhengzhou Shangshui Biotechnology Co., Ltd., Zhengzhou City, Henan Province, China. with a purity of 99.5%. The BAs used in this experiment were synthesized artificially and the content of each component in the BAs was detected using liquid chromatography–tandem mass spectrometry. The detection results showed that the BAs contained 15.00% of cholanic acid, 44.00% of hyodesoxycholic acid, and 40.50% of chenodeoxycholic acid. A total of 168 28-day-old male Holldobagy geese were selected for the experiment, which were purchased from Xiangtian Ge Family Farm in He County, Ma’anshan City, Anhui Province, China. All geese were randomly divided into four groups (with dietary BAs concentrations of 0, 75, 150, and 300 mg/kg), with six replicates per group and 7 geese per replicate. The diet used in this study was formulated to meet the nutrient requirements of geese in China, based on the recommendations of NRC 1994 (Table 1). All geese were raised in a closed house with a density of 0.5m2 per goose, with temperature maintained at around 15°C. They were exposed to natural light during the day and provided with artificial light at night. Throughout the experiment, the geese had unrestricted access to feed or water, and their health status and vaccination were monitored regularly.
Growth performance
During the experimental period, daily feed intake of the geese in each treatment group was carefully recorded. In addition, the geese were subjected to a 10-h fast and a 2-h water withholding period before their morning feeding at 35, 49, and 63 days of age, followed by an accurate weight measurement to determine their body weight (BW) on an empty stomach. These measures were conducted to calculate the average daily gain (ADG), average daily feed intake (ADFI), and F/G at the ages of 35–49, 49–63, and 35–63 days, respectively.
ADG = (weight at time 2 - weight at time 1)/(number of days between time 1 and time 2)
ADFI = total feed intake/number of days× test geese
F/G = total feed intake/total weight gain
Serum lipid
At the conclusion of the study, a single goose was chosen from each trial whose body weight closely approximated the replicate average. Blood collection of 4 mL was taken from the vein of each chosen goose. Subsequently, the blood samples were left at 37°C for 4 h and centrifuged at 4000 rpm to separate the serum. The serum samples were analyzed for levels of total cholesterol (TC), triglycerides (TG), HDL-C, and low-density lipoprotein cholesterol (LDL-C) using an automated biochemical analyzer (Mindray BS200, China).
Liver lipids and gut mucosal barrier
For each replicate (n = 7), a goose with a weight close to the mean was selected and euthanized by bleeding from the neck with efforts made to minimize the suffering of the geese during the procedure. All samples were obtained from 24 geese. The liver was completely removed and all abdominal fat was peeled off, weighed to calculate the organ index (organ weight, g/body weight, kg). A small quantity of liver and jejunal mucosa were collected and stored in a 2 ml cryotube, which was then placed in liquid nitrogen for preservation.
The liver and jejunal mucosa RNA was extracted using Trizol (Invitrogen, Carlsbad, CA, USA), and a reverse transcription kit (TransScript, TransGen, Beijing, China) was used to transcribe the total RNA with a concentration and purity measured using Nano-drop 1,000 Spectrophotometer (Thermo Fisher Scientific, Waltham, MA, USA) into cDNA. The β-actin gene was selected as a reference gene. Real-time PCR was conducted to determine the expression levels of target genes using the TransGen TB Green kit (TransGen Bio Inc., Beijing, China) and a fluorescence quantitative reaction instrument (QuantStudio 5, Thermo Fisher Scientific, MA, USA), including sterol-regulatory element binding proteins (SREBP-1), PPARα, fatty acid synthase (FAS), Acetyl CoA carboxylase (ACCA), Farnesoid X Receptor (FXR), zona occludens 1 (ZO-1), Occludin and Claudin-1 with primer sequences provided in Table 2, the β-actin gene was a reference gene. For data analysis, a relative quantitative method (2^-ΔΔCT) was used.
Intestinal morphology
Jejunum and ileum intestinal segments were collected and immediately placed on ice. The segments were washed with 4°C pre-cooled PBS for 20 s to remove intestinal contents. Three-centimeter long complete middle segments were collected, fixed in 4% paraformaldehyde solution (Aladdin, Shanghai, China) for 24 h, and then subjected to treatments such as water washing, dehydration, transparency, paraffin embedding (Electron Microscopy Sciences, Hatfield, USA), and sectioning using Leica RM2016 microtome (Leica, Germany). After staining with hematoxylin and eosin, the sections were observed under an optical microscope (Olympus BX53, Olympus Optical Co. Ltd., Shenzhen, China) to examine the morphological parameters of the jejunum, and ileum. CD, VH and the VH/CD were calculated after morphological measurements.
Cecal microbiota
Approximately 1 g of cecal chyme was collected and transferred into sterile cryovials, stored in liquid nitrogen, and transferred to a −80°C ultra-low temperature freezer after sampling. DNA was extracted from cecal contents using a DNA extraction kit. The purity and quality of DNA were determined by 1.0% agarose gel electrophoresis. The V3-V4 region of the 16S rDNA was amplified using specific primers (338F, 5′-ACTCCTACGGGAGGCAGCA-3′, 806R: 5′-GGACTACHVGGGTWTCTAAT-3′). The PCR products were purified and recovered after being analyzed by lipopolysaccharide agarose gel electrophoresis. MiSeq library was constructed and Illumina sequencing was performed by Shanghai Personal Biotechnology Co., Ltd. (Shanghai, China).
Intestine short-chain fatty acids
Aseptically collect 1 g of chyme from the cecum and colon, and add an appropriate amount of PBS (pH = 7.4) to a 2 mL Eppendorf tube. Quickly freeze and store the sample in liquid nitrogen. After thawing the chyme, homogenize it thoroughly using a homogenizer. Centrifuge the homogenate for 20 min at 3000 rpm, and collect the supernatant. The concentration of total SCFA in the cecum and colon samples of each group was detected using an Infinite F50 microplate reader (Tecan, Switzerland) and an enzyme-linked immunosorbent assay (ELISA) kit (Jiangsu Jingmei Biotechnology Co., Ltd., Yancheng, China) for goose SCFA.
Statistical analysis
Use Microsoft Excel 2007 to organize various data. Perform one-way ANOVA analysis and Duncan multiple comparison of variance using SPSS 25.0 software (IBM, New York, USA). Polynomial contrasts were used to determine the linear and quadratic effects of dietary BAs supplementation levels. Results will be presented as mean ± standard deviation and significant differences will be indicated as p < 0.05.
Raw cecal microbiota sequencing data were quality-controlled using QIIME 2.0 software. Optimized sequences were obtained through sequence splicing, screening, and elimination of chimeras. USEARCH 7.0 software was used for cluster analysis of amplicon sequence variants (ASV). Greengenes data were used for the taxonomic analysis of ASV sequences, and the community composition of each sample was counted at the levels of phylum, class, order, family, genus, and species. Principal coordinate analysis (PCoA) of the cecal microbiota was performed using R language, and data visualization was conducted using STAMP 2.1.3 software. ɑ-diversity indices were calculated using Mothur 1.2 software. Using Phylogenetic Investigation of Communities by Reconstruction of Unobserved States (PICRUSt2) to predict the metabolic capabilities of the cecal microbiota.
Results
Growth performance
During the experiment, the geese remained in good health, and there were no eliminations or deaths due to stress or disease. The growth performance during the experimental period is presented in Table 3. No significant differences (p > 0.05) in BW were observed among the groups at 35, 49, and 63d. No significant differences (p > 0.05) were found in ADFI among the groups at 35–49, 49–63, and 35–63d. Regarding ADG, there were no significant differences (p > 0.05) among the groups at 49–63 and 35–63d. However, the addition of 150 mg/kg BAs increased ADG by 12.16% (p < 0.05) at 35–49d. In terms of F/G, the addition of 75, 150, and 300 mg/kg significantly increased F/G at 35–49d (p < 0.05). No significant differences (p > 0.05) were found among the groups at 49–63d. The addition of 75 and 150 mg/kg significantly improved F/G at 35–63d (p < 0.05).
Bile acid receptor expression
We examined the expression of bile acid receptor-FXR mRNA in both the liver and jejunum. According to the results presented in Figure 1, the addition of bile acid to the diet led to an increase in the expression of FXR in the liver. Specifically, both the 150 mg/kg significantly elevated the levels of FXR mRNA expression in the liver (p < 0.05), whereas there were no significant differences among the groups in the jejunum. Furthermore, the expression of FXR mRNA in the liver was found to be higher than that in the jejunum, suggesting that FXR may play a more crucial role or have a broader range of physiological effects in the liver.
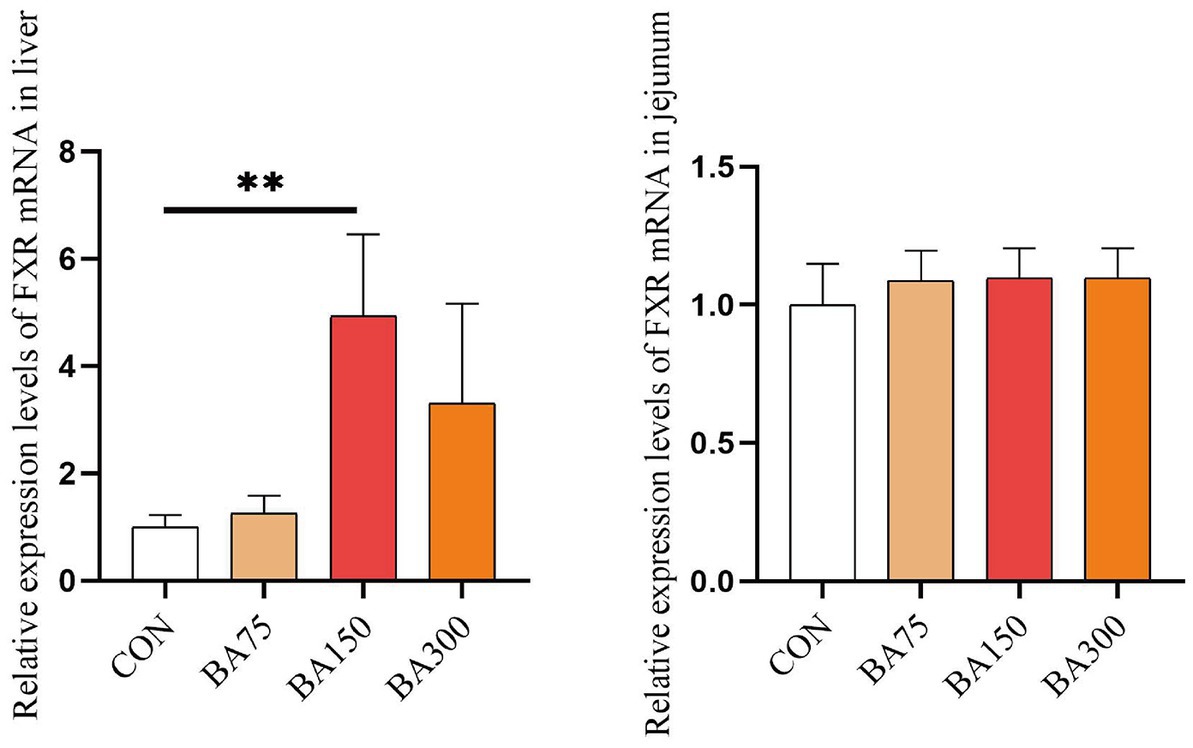
Figure 1. Effects of different levels of BAs on the expression of goose bile acid receptor. FXR, Farnesoid X Receptor. CON, 0 mg/kg BAs; BA75, 75 mg/kg BAs; BA150, 150 mg/kg BAs; BA300, 300 mg/kg BAs. *p < 0.05, **p < 0.01.
Lipid metabolism
In order to investigate the impact of different levels of BAs on the metabolism of goose fat, we first examined their effects on liver and abdominal fat indices (Figure 2A). We found no significant differences in liver index between the groups (p > 0.05). As the level of BAs increased, the abdominal fat index gradually decreased. At 300 mg/kg, there was a trend toward lower abdominal fat (p = 0.058).
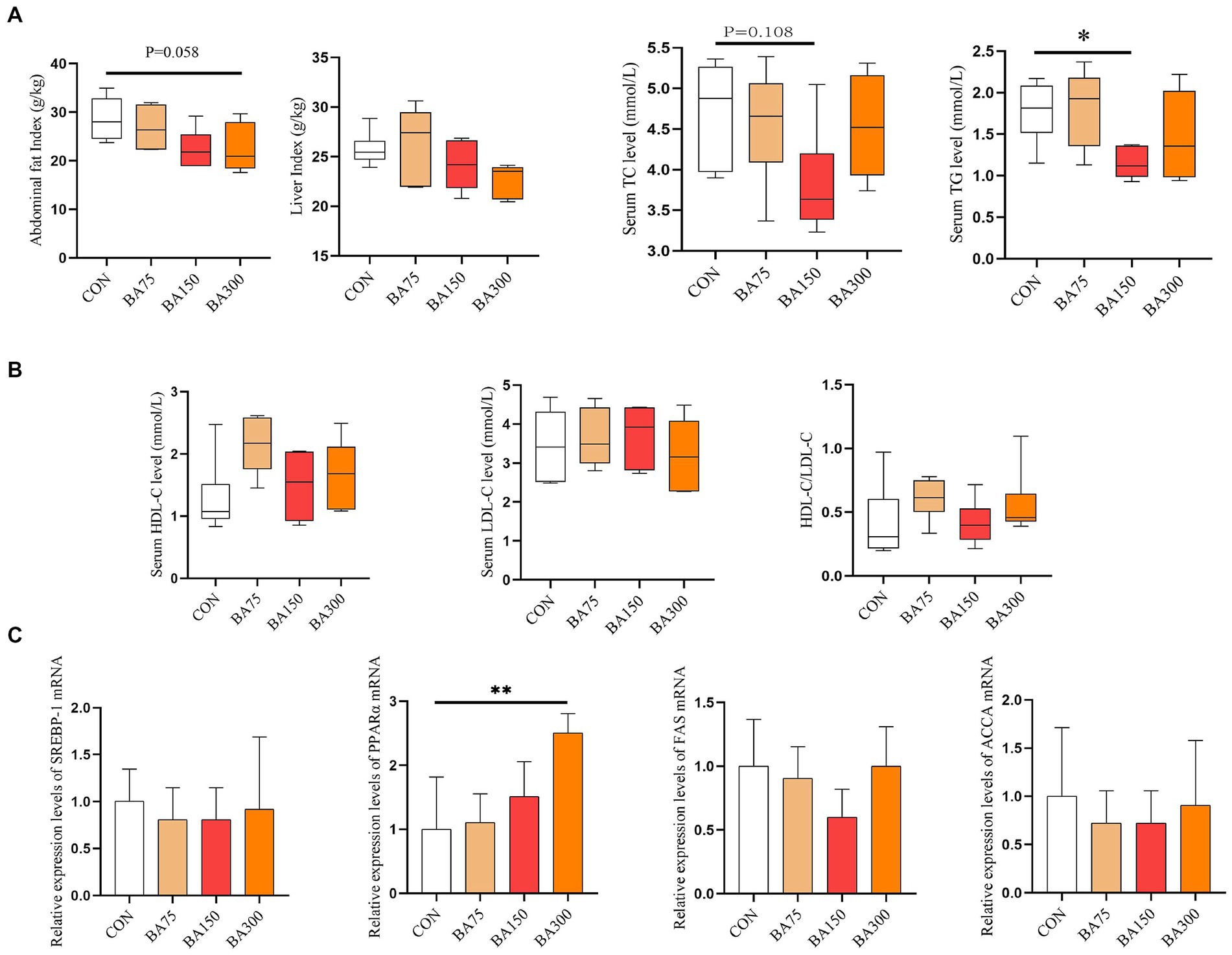
Figure 2. Effects of different levels of BAs on fat metabolism in geese. (A) Effects of BAs on liver index and visceral fat index. (B) Effects of BAs on serum total cholesterol (TC), triglycerides (TG), high-density lipoprotein cholesterol (HDL-C), and low-density lipoprotein cholesterol (LDL-C). (C) Effects of BAs on mRNA expression of liver lipid metabolism genes, sterol-regulatory element binding proteins (SREBP-1), peroxisome proliferators-activated receptor α (PPARα), fatty acid synthase (FAS), Acetyl CoA carboxylase (ACCA). *p < 0.05, **p < 0.01.
We determined the differences in serum lipid metabolism among different treatment groups at the same time. As shown in Figure 2B, the addition of 75 mg/kg of BAs had no significant effect on serum TG levels (p > 0.05), while the addition of 150 mg/kg of BAs significantly reduced the levels (p < 0.05). As the level of dietary BAs increased, HDL-C and HDL-C/LDL-C levels also increased, but there was no statistically significant difference among the groups (p > 0.05).
To further investigate its impact on lipid metabolism, we measured the mRNA expression levels of liver SREBP-1, PPARα, FAS, and ACCA (Figure 2C). The addition of 75, 150, and 300/kg decreased the expression of SREBP-1, FAS, and ACCA on the liver, but there was no significant difference among the groups (p > 0.05). However, adding BAs increased the expression levels of PPARα, and at a level of 300 mg/kg, PPARα reached a significant difference (p < 0.05).
Intestinal morphology and mucosal barrier
Figure 3A shows the changes in the morphology of the cecum following the addition of 0, 75, 150, and 300 mg/kg of dietary BAs. We found that the addition of BAs to the diet did not have a significant effect on CD in jejunum (p > 0.05), but 150 mg/kg of BAs significantly increased VH and VH/CD (p < 0.05) (Figure 3B). Figure 3C shows the changes in the morphology of the ileum in each treatment group. Unlike jejunum, the addition of 150 and 300 mg/kg significantly decreased CD in the ileum, increased VH and VH/CD (p < 0.05) (Figure 3D).
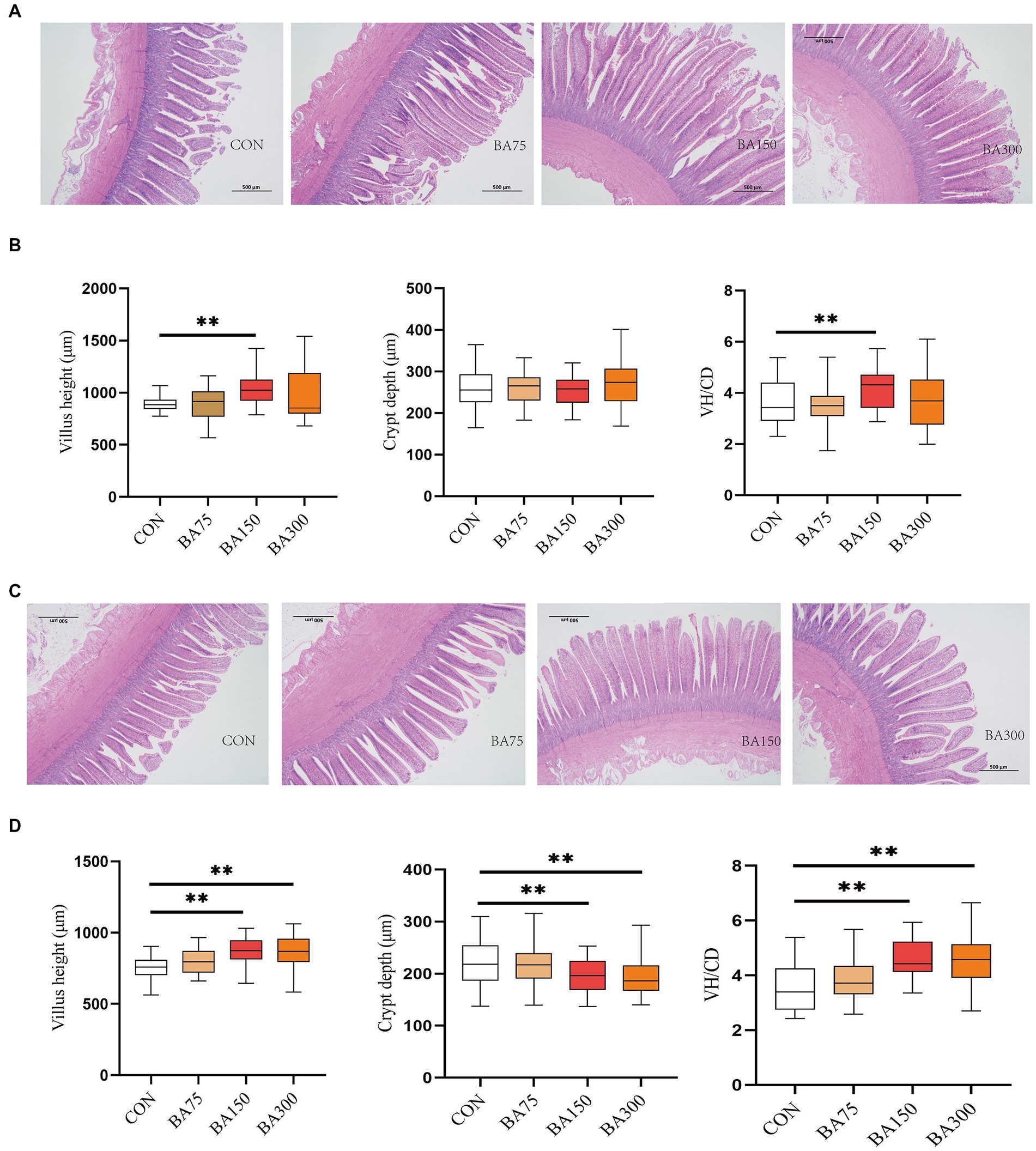
Figure 3. Effects of different levels of BAs on the morphology of the jejunum and ileum in geese. (A) Effects of BAs on the morphology of the jejunum after Hematoxylin and Erosion staining. Scale bar, 500 μm. (B) Villus height (VH), crypt depth (CD), and VH/CD ratio in the jejunum of geese fed a basal diet with different levels of BAs. (C) Effects of BAs on the morphology of the ileum after Hematoxylin and Erosion staining. Scale bar, 500 μm. (D) Villus height (VH), crypt depth (CD), and VH/CD ratio in the ileum of geese fed a basal diet with different levels of BAs. *p < 0.05, **p < 0.01.
To further investigate the impact of BAs on intestinal barrier integrity, we measured the relative expression levels of Occludin, ZO-1 and Claudin-1 mRNA in the jejunum (Figure 4). Our findings revealed that the addition of BAs increased the expression levels of all three genes. Notably, the expression of ZO-1 was significantly increased with the addition of 150 mg and 300 mg/kg of BAs (p < 0.05), with the highest expression observed with the addition of 150 mg/kg. Although there was no significant difference in Claudin-1 expression levels among the groups (p > 0.05), the expression of Occludin was increased with the addition of 75, 150, and 300 mg/kg of BAs (p < 0.05).
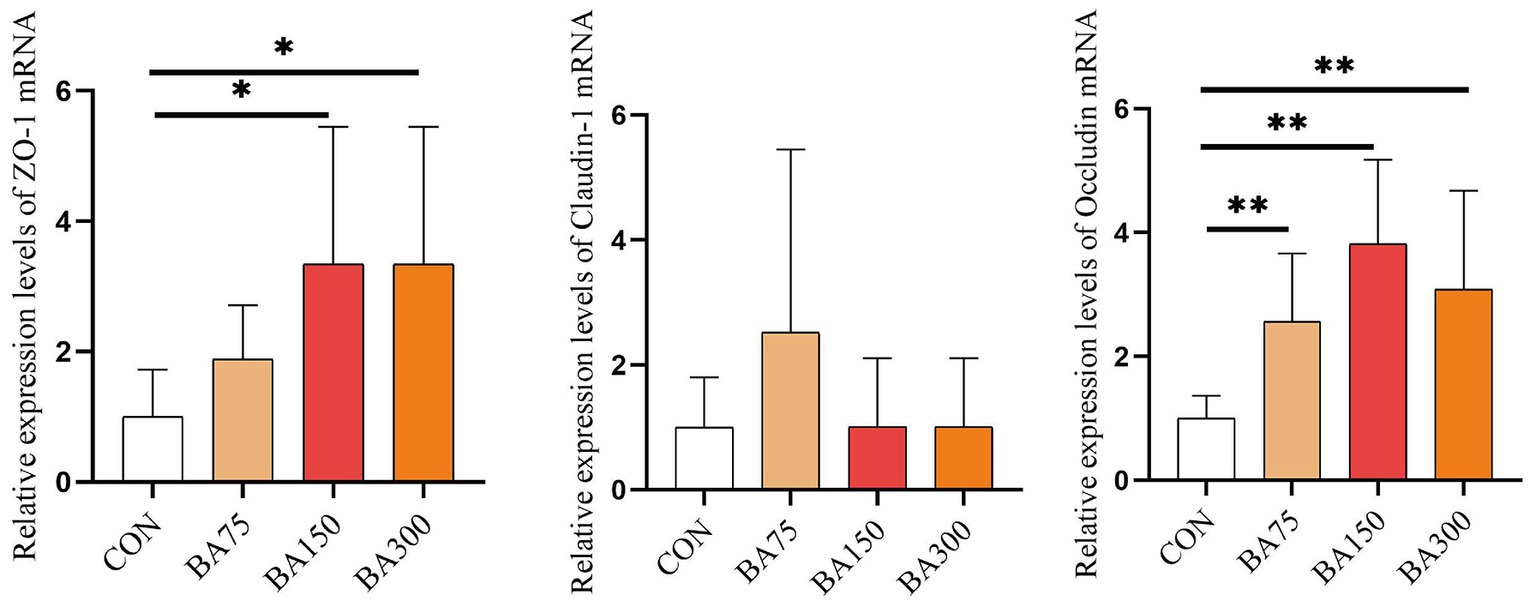
Figure 4. Effects of different levels of BAs on the integrity of the jejunal barrier in geese. Effects of BAs on jejunal expression of zona occludens 1 (ZO-1), Occludin, and Claudin-1 mRNA in geese. *p < 0.05, **p < 0.01.
Cecal microbiota diversity
To evaluate the effect of BAs on the microbiota in the cecum of geese, we analyzed the α-diversity and β-diversity between groups based on sequencing data. In terms of α-diversity (Figure 5A), we measured the indices of Chao1, Observed species, Shannon, Simpson, Faith’s PD, and Pielou’s evenness, where Chao1 and Observed species represent richness, Shannon and Simpson represent diversity, Faith’s PD represents evolutionary diversity, and Pielou’s evenness represents evenness. The results showed no significant differences in Shannon, Simpson, and Pielou’s indices between groups (p > 0.05). However, adding 150 mg/kg of BAs significantly increased Chao1 (p < 0.05), and adding 300 mg/kg of BAs significantly increased Chao1, Observed species, and Faith’s PD indices (p < 0.05). The β-diversity is showed in Figure 5B. The PCoA based on Bray-Curtis distance showed the β-diversity between groups. We found that there was some overlap between the samples treated with 0 mg/kg and 75 mg/kg of BAs, while those treated with 150 mg/kg and 300 mg/kg of BAs showed a more obvious clustering, although the difference was not significant. Therefore, further analysis is needed to explore the differences in microbial composition between groups.
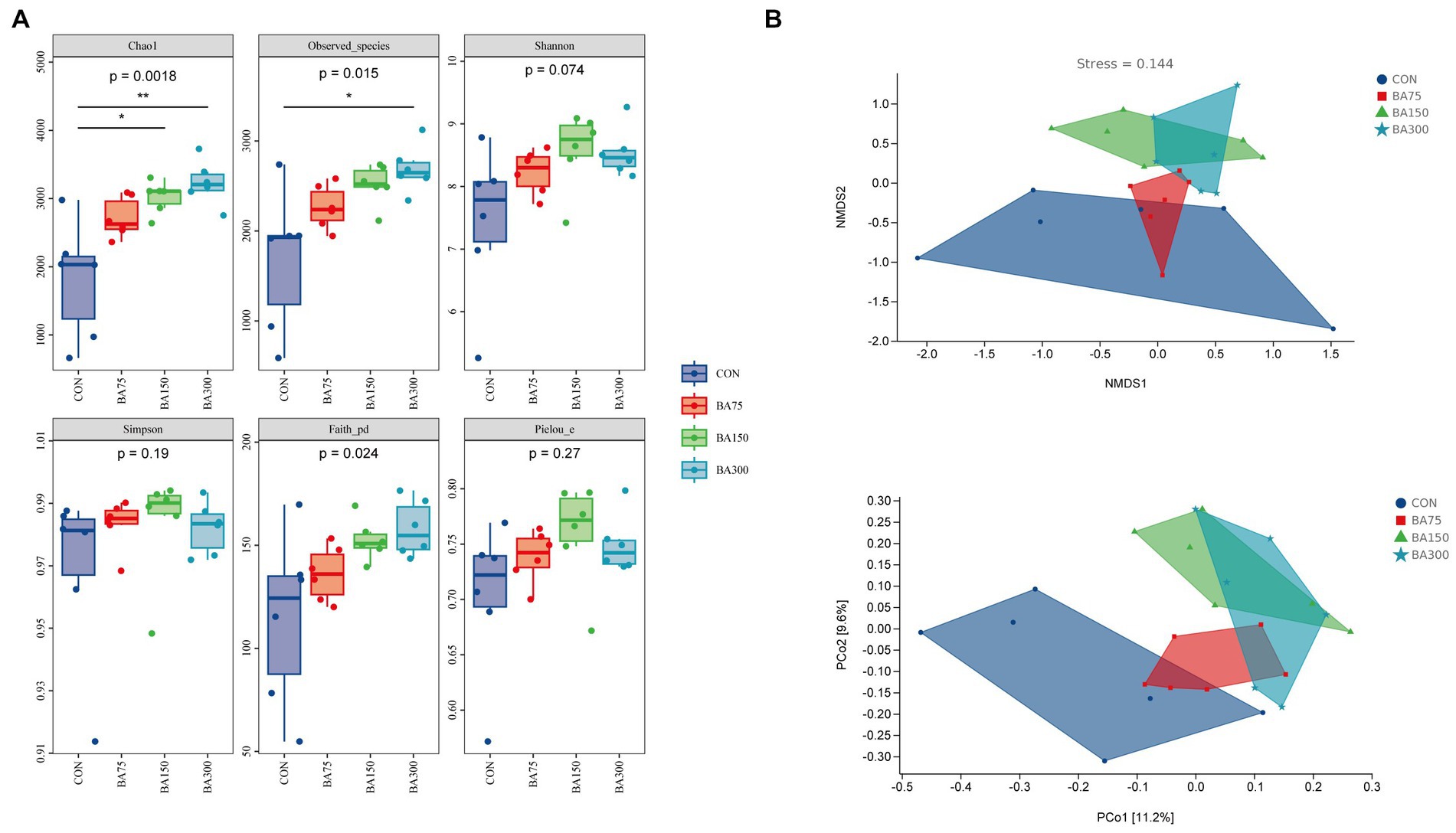
Figure 5. Effects of different levels of BAs on the diversity of cecum microbiota in geese. (A) Effects of adding BAs to the basal diet on the ɑ-diversity of fecal bacteria in geese. Boxplots show the differences in Chao1, Observed species, Shannon, Simpson, Faith’s PD, and Pielou’s evenness indices between groups. (B) The β-diversity analysis of cecum microbiota among groups. PCoA and NMDS analyses were performed based on Bray–Curtis distance, and different colors and shapes represent samples from different groups. *p < 0.05, **p < 0.01.
Microbial composition
To investigate the changes in gut bacteria abundance among different groups, we calculated the impact of different levels of BAs on the composition of cecal microbiota in geese. Subsequently, we constructed a bar graph to represent the relative abundance of cecal bacteria in each sample. To further elucidate the abundance levels and proportions of different phyla within each sample, we generated a column accumulation chart based on the relative abundance of bacteria (Figure 6A).
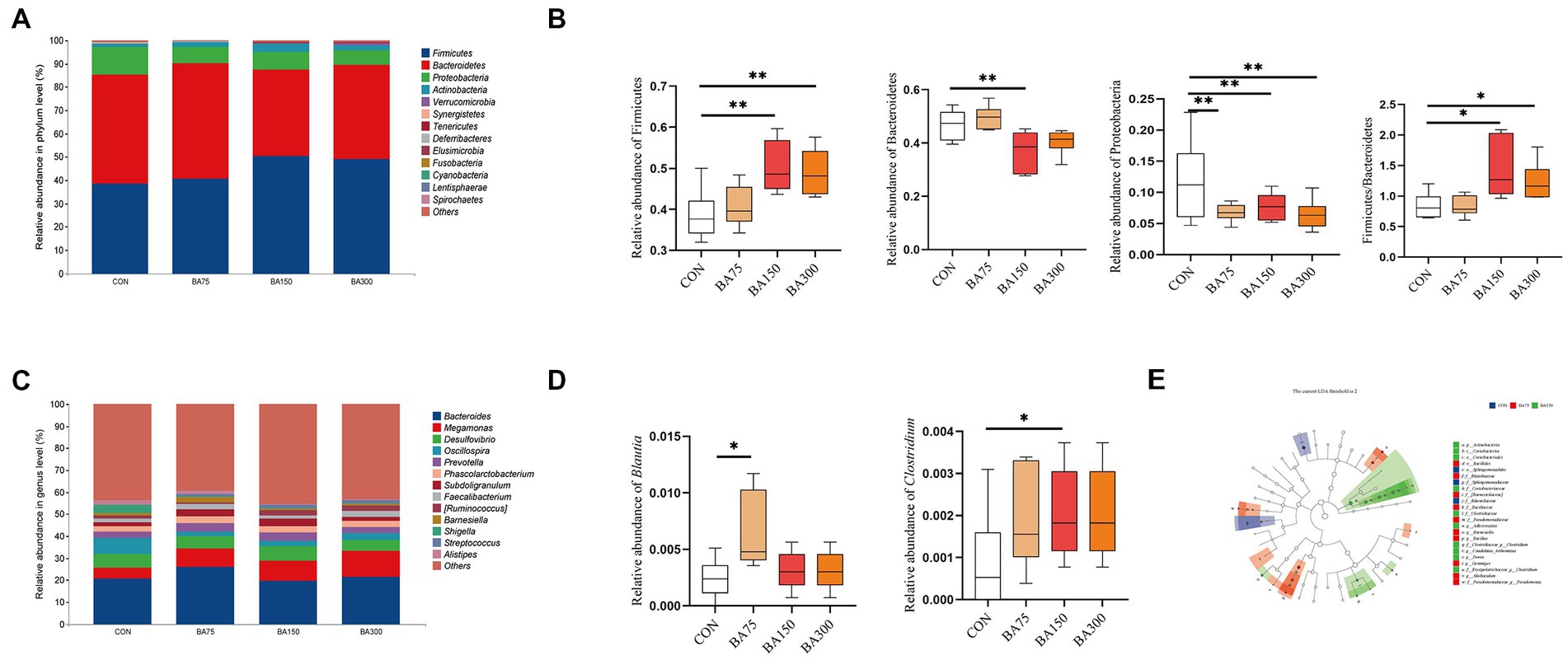
Figure 6. Effects of different BAs on changes in fecal microbiota of geese. At the phylum (A) and genus (B) levels, the classification composition and relative abundance of fecal microbiota in each group. The abundance of Firmicutes, Bacteroidetes, and Proteobacteria and the ratio of Firmicutes to Bacteroidetes at the phylum level (C). The relative abundance of Blautia and Clostridium at the genus level (D). Linear discriminant analysis (LDA) (LDA > 2.0, p < 0.05) of cecal microbiomes (E). *p < 0.05, **p < 0.01.
The primary microbial phyla in the cecum are Firmicutes, Bacteroides, Proteobacteria, and Actinobacteria, which together account for over 98% of total abundance in each group with different dosages of BAs supplementation—namely 98.46% for 0 mg/kg, 99.19% for 75 mg/kg, 98.45% for 150 mg/kg, and 97.81% for 300 mg/kg. Notably, the phylum Bacteroidetes exhibited the highest relative abundance in samples supplemented with 0 mg/kg and 75 mg/kg, reaching 46.83 and 49.60%, respectively. By contrast, the addition of 150 mg/kg caused a 26.50% decrease in the abundance of this phylum (p < 0.05) (Figure 6C). In contrast, the highest abundance of Firmicutes was observed in samples supplemented with 150 mg/kg and 300 mg/kg, with relative abundances of 50.28 and 48.97%, respectively, representing increases of 30.29 and 26.90% compared with 0 mg/kg (p < 0.05). In addition, the Firmicutes to Bacteroidetes (F/B) ratio increased significantly with the addition of 150 and 300 mg/kg BAs (p < 0.05). Moreover, there was a significant decrease in Proteobacteria abundance with the supplementation of 75, 150, and 300 mg/kg BAs (p < 0.05). The dominant microbial genera in the cecum were found to be Bacteroides, Megamonas, Desulfovibrio, and Oscillospira, which together accounted for 39.41, 43.33, 37.84, and 41.48%, respectively (Figures 6B). One-way ANOVA analysis of the top 50 abundant genera in each sample showed significant increases in the abundance of Blautia with the addition of 75 mg/kg BAs and the abundance of with 150 mg/kg BAs (p < 0.05) (Figure 6D). Using Linear Discriminant Analysis Effect Size (LEfSe) analysis, biomarkers for each group were identified, including f__Rikenellaceae, f__Sphingomonadaceae and o_Sphingomonadales for the group that received 0 mg/kg BAs, f_[Barnesiellaceae], g_Barnesiella, f_Rhizobiaceae, g_Allobaculum, o_Bacillales, g_Gemmiger, f_Bacillaceae, g__Bacillus, f_Pseudomonadaceae, and f_Pseudomonadaceae_g__Pseudomonas for the group that received 75 mg/kg BAs, and p_Actinobacteria, o_Coriobacteriales, f__Coriobacteriaceae, c_Coriobacteriia, f__Erysipelotrichaceae_g__Clostridium, f__Clostridiaceae, f_Clostridiaceae_g__Clostridium, g__Dorea, g_Adlercreutzia, and g_Adlercreutzia Candidatus Arthromitus for the group that received 150 mg/kg BAs (Figure 6E).
Intestine short-chain fatty acids
We found an increase in the abundance of SCFA-producing bacteria in the cecum by 16S sequencing. Therefore, we detected the total concentration of SCFA in the jejunum and cecum using ELISA. As shown in Figure 7, we observed that the addition of 75 mg/kg of BAs had no significant effect on the SCFA concentration in the jejunum and ileum (p > 0.05), but the addition of 150 mg/kg and 300 mg/kg of bile acid significantly increased the SCFA concentration in the cecum and jejunum (p < 0.05).
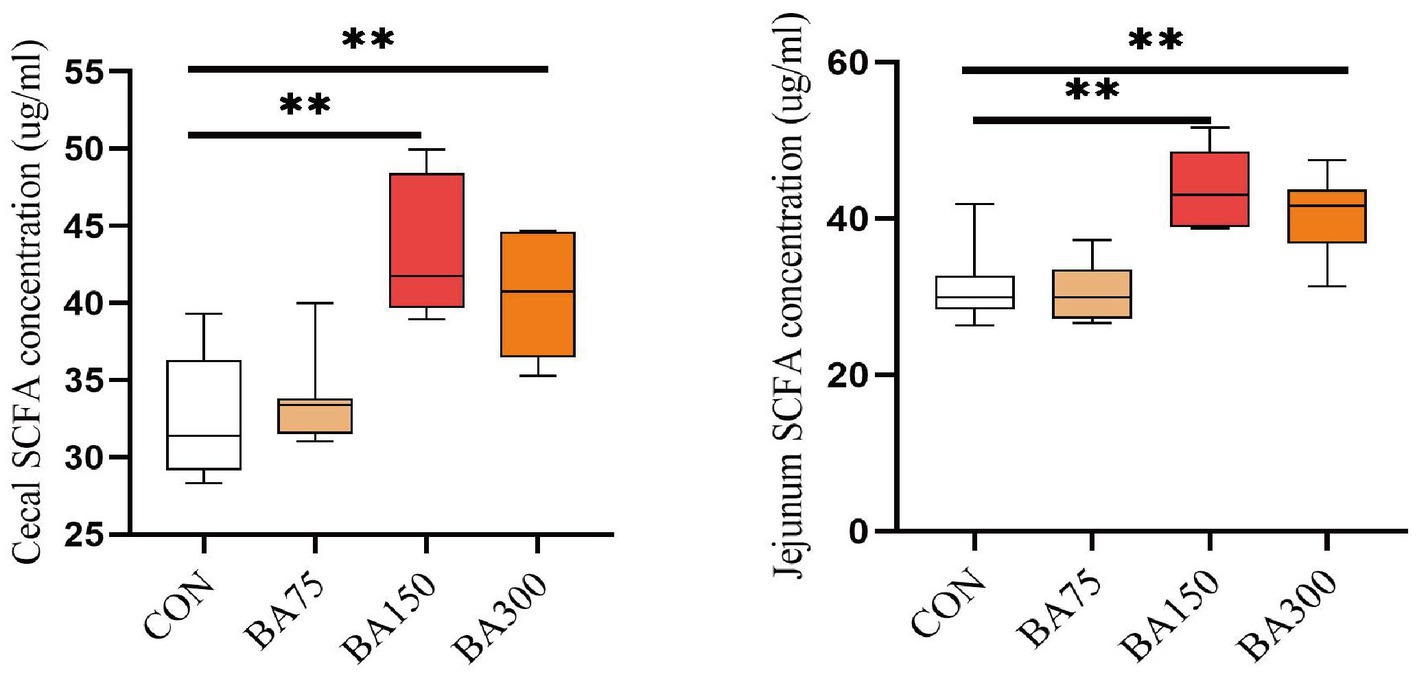
Figure 7. Effects of different levels of BAs on the content of SCFA in goose jejunum and cecum. SCFA, short chain fatty acids. *p < 0.05, **p < 0.01.
Function prediction
We utilized PICRUSt2 to predict the KEGG pathways associated with the cecal microbiota involved in lipid metabolism (Figure 8). The results showed that the abundance of certain metabolic pathways was increased with 75 mg/kg of BAs, including Fructose and mannose metabolism, Purine metabolism, and Galactose metabolism (p < 0.05). Fatty acid metabolism and Oxidative phosphorylation were increased with 150 and 300 mg/kg of BAs (p < 0.05). In contrast, Secondary bile acid biosynthesis, TCA cycle, and Fatty acid biosynthesis were decreased with 150 and 300 mg/kg of BAs, and Primary bile acid biosynthesis was decreased with 300 mg/kg of BAs (p < 0.05). However, 75 mg/kg of BAs had no significant effect on the above pathways (p > 0.05).
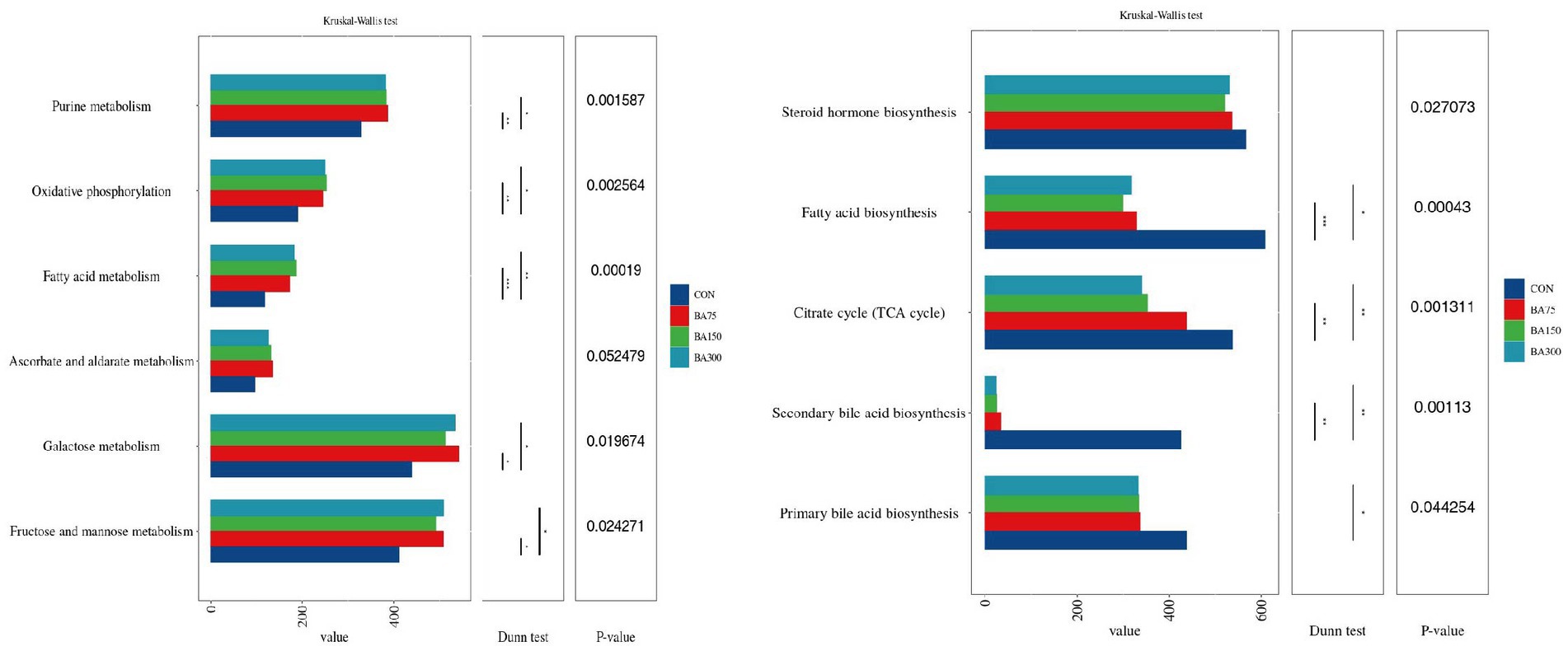
Figure 8. Effect of different levels of BAs on the KEGG pathways of cecal microbiota in geese, and *p < 0.05 and, **p <0.01 or ***p <0.001 indicate significant correlations. The Y-axis represents metabolic pathways, and the X-axis represents the relative abundance under each pathway.
Main parameters and cecum microbiota
In order to gain a deeper understanding of the relationship between the cecal microbiota and lipid metabolism and intestinal function, the Spearman correlation analysis was conducted based on the relative abundance of the top 30 bacterial genera’s main species. This was done to determine the relationships between them. Figure 9A presents the relationship between lipid metabolism and genera. The findings revealed that [Ruminococcus], Erysipelotrichaceae Clostridium, and Clostridiaceae Clostridium were negatively correlated with TG levels (p < 0.05), whereas Bacteroides, Slackia, and LDL-C levels were positively correlated (p < 0.05). Additionally, Blautia was positively associated with HDL-C levels (p < 0.05). Regarding liver lipid metabolism, Bacteroides was found to be negatively correlated with the expression level of liver PPARα mRNA (p < 0.05). In contrast, [Ruminococcus], Ruminococcaceae Ruminococcus, Clostridiaceae Clostridium, and Dorea were positively correlated with PPARα expression level (p < 0.05). Similarly, Desulfovibrio, and Slackia exhibited a negative correlation with FAS expression level (p < 0.05).
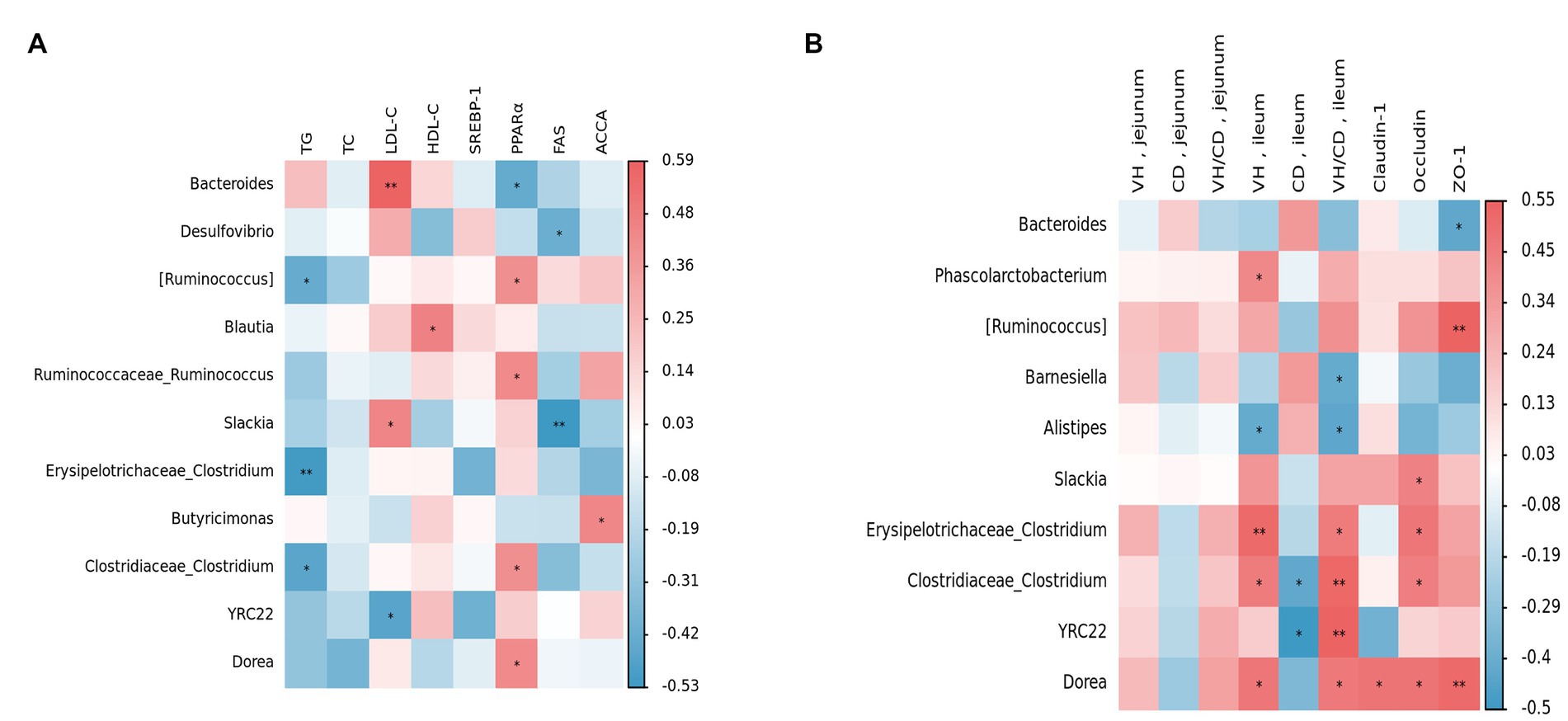
Figure 9. Relationships between main parameters and the top 30 genera in the cecum. Red represents positive correlation, blue represents negative correlation, and *p < 0.05 and **p < 0.01 represent significant correlations. (A) Relationships between fat metabolism and gut microbiota. (B) Relationships between gut morphology and gut microbiota.
Figure 9B shows the relationship between bacterial genera, VH, CD, and mucosal barriers. The results indicated that there were no significant differences between the parameters of the jejunum and the genera. In the ileum, Phascolarctobacterium, Erysipelotrichaceae Clostridium, Clostridiaceae Clostridium, and Dorea were positively correlated with VH (p < 0.05). Additionally, Clostridiaceae Clostridium and YRC22 were negatively correlated with CD (p < 0.05). Furthermore, Barnesiella and Alistipes exhibited a negative correlation with VH/CD (p < 0.05). In terms of gene expression related to intestinal mucosal barriers, the results showed that Slackia, Erysipelotrichaceae Clostridium, Clostridiaceae Clostridium, Dorea, and Occludin were positively correlated (p < 0.05). Conversely, Bacteroides was found to be negatively correlated with the expression of ZO-1 (p < 0.05) but positively correlated with [Ruminococcus] and Dorea expression levels (p < 0.05).
Discussion
Growth performance is an indicator reflecting the growth and development of animals. For breeding enterprises, improving the growth performance of animals is of great significance to enhance economic benefits. Among them, F/G is a representative index for evaluating growth performance, and a lower F/G indicates better growth performance (Chen et al., 2022). Based on their common usage in fisheries, BAs have been found to be effective supplements. Specifically, adding 600 mg/kg of BAs to a low fishmeal diet significantly improved the final body weight of Litopenaeus vannamei (Li et al., 2023), while a feeding trial using 1.25 g/kg of BAs significantly improved the feed efficiency ratio of striped catfish (Adam et al., 2023). Currently, the application of BAs in livestock and poultry is limited. However, it has been found that supplementing BAs can improve the feed efficiency ratio of broiler chickens. Yang et al. (2022) discovered that supplementing 60 mg/kg of BAs could improve the egg-laying performance of laying hens in the later stages. It was also found that supplementing BAs did not affect the production performance of weaned piglets (Chiang and Ferrell, 2020).
The growth performance of animals is closely related to their intestinal morphology (Yousefi et al., 2023; Zahid et al., 2023). The VH, CD and VH/CD are important indicators for measuring intestinal digestion and absorption function (Gu et al., 2022; Martel et al., 2022). BAs have different effects on intestinal cells in various animal species. Supplementation of BAs in the diets of intrauterine growth-restricted piglets can increase the VH/CD of the ileum (Jain et al., 2012). In a mouse model of lipopolysaccharide-induced intestinal injury, adding BAs can improve intestinal cell proliferation and increase VH, thereby protecting the intestinal mucosa. Therefore, BAs supplementation may potentially protect the intestine from injury or infection (Perrone et al., 2010). However, some studies have found that adding BAs to the diet can decrease the VH of the intestine (Parsaie et al., 2007; Liu et al., 2022a,b), which may be related to the concentration and method of use. Furthermore, it is known that BAs participate in lipid metabolism by activating FXR (Wang et al., 1999). This experiment also found that adding BAs can increase the expression levels of FXR in the liver. Studies have shown that FXR plays an important role in the formation and maintenance of the intestinal mucosal barrier. In intestinal epithelial cells, FXR can promote normal intestinal cell proliferation while inhibiting the occurrence of intestinal developmental abnormalities, tumors, and other related diseases. In addition, FXR can also regulate the permeability of the intestinal mucosal barrier and the secretion of the intestinal mucus layer, thereby enhancing the protective function of the intestinal mucosa (Stojancevic et al., 2012).
ZO-1 is a protein that enhances the stability and integrity of intercellular connections (Gilani et al., 2018). Claudin-1 and Occludin are the main proteins that make up tight junctions between cells. They directly act on the tight connections between epithelial cells and thereby control the permeability of molecules between cells (Song et al., 2018). According to previous studies, dietary supplementation with BAs can increase the abundance of beneficial bacteria and reduce harmful bacteria (Yang et al., 2022). Pathogenic bacteria attach to host cells through adhesion and subsequently use nutrients in host cells to proliferate and release enzymes and toxins, causing damage to the body (Glasser et al., 2001). Some beneficial bacteria have high adhesion capabilities and can inhibit the attachment of pathogenic bacteria to the intestine (Burgain et al., 2013). In this experiment, we observed that the addition of BAs to the diet had no significant impact on jejunum claudin-1 expression, but enhanced the expression of both Occludin and ZO-1. Therefore, our findings suggest that supplementing the diet with BAs can significantly improve the F/G of geese during the pre-trial and entire trial period, likely due to the promotion of intestinal health through exogenous BAs supplementation.
Bile acids are synthesized from cholesterol in the liver and stored in the gallbladder in the form of bile salts, which combine with taurine in poultry (Chiang and Ferrell, 2020). When the gallbladder contracts during feeding, it promotes the release of bile salts into the small intestine. The hydroxyl and carboxyl groups of BAs are oriented toward one side as a hydrophilic surface, while the other side consists of an alkyl chain and is hydrophobic, making BAs both hydrophilic and hydrophobic in nature (Li and Chiang, 2014). Lipid is composed of various fatty acids and glycerol molecules, and the interactions between these molecules cause fat to form insoluble droplets that are difficult to break down. Due to its amphiphilic nature, BAs can form a mixture of water and fat, increasing the contact area between lipase and fat, thereby increasing lipolysis (Fuchs, 2003). Additionally, BAs can also enhance the catalytic activity of lipase (Nguyen and Van Do, 2021), leading to the absorption and transportation of fats and fat-soluble microbes (Li and Apte, 2015). Therefore, BAs can help to maintain the steady state of TG and regulate lipid deposition, thereby playing a significant role in lipid metabolism (Hu et al., 2019).
SREBP-1 is a transcription factor involved in lipid synthesis. It promotes lipid synthesis by regulating the expression of multiple enzymes (Horton et al., 2002). FAS, an important enzyme responsible for fatty acid synthesis, plays a crucial role in lipid metabolism by synthesizing triglycerides and other fatty acid esters (Smith, 1994). SREBP-1 and FAS are closely related in lipid metabolism and exhibit positive feedback regulation (Dihingia et al., 2018). ACCA is a key limiting enzyme in the fatty acid synthesis pathway. Inhibition of ACCA can lead to impaired fatty acid synthesis and stimulation of fatty acid oxidation, thereby altering the rate of fatty acid synthesis (Harwood, 2004). PPARα is a transcription factor that can promote lipid metabolism and plays an important regulatory role in the processes of lipid oxidation and β-oxidation in the body (Chinetti et al., 2000). Consumption of a high-fat diet can increase the expression of FAS and SREBP-1, while decreasing the expression of PPARα in grouper (Xu et al., 2023). Nevertheless, this expression can be ameliorated by BAs, which can facilitate the breakdown of lipids. Meanwhile, adding a small amount of BAs can reduce the lipid content in the whole body and liver of Nile tilapia and tiger pufferfish (El-Shenawy et al., 2020; Liao et al., 2020). In this experiment, we found a trend of decreasing expression of liver SREBP-1 and FAS with the addition of BAs in all groups, but there was no significant difference between the groups, which is consistent with previous findings (Liu et al., 2022a,b). However, the expression level of PPARα was significantly increased with the addition of 300 mg/kg BAs. Therefore, we believe that the addition of BAs in the diet can promote hepatic lipid metabolism, reduce serum TC and TG levels, and thereby improve abdominal fat deposition in geese.
Bile acids can not only provide feedback inhibition on cholesterol synthesis (Wang et al., 2003), but also enhance the expression of low-density lipoprotein receptor genes on the cell membrane, promoting the absorption and conversion of LDL-C, thereby lowering serum LDL-C levels (Langhi et al., 2008). Furthermore, an increasing number of studies have shown that BAs can also lower serum TG levels (Watanabe et al., 2011). BAs promote the degradation and utilization of TG by regulating the expression of lipid metabolism enzymes in the liver and adipose tissue, thereby lowering serum TG levels. This effect may help reduce animal fat weight, which was also observed in this study.
The gut microbiota contains trillions of microorganisms. The gut microbiota is not only beneficial for extracting nutrients and energy from ingested food, but also produces a large number of metabolites to regulate host metabolism (Ramírez et al., 2017). In this experiment, we analyzed the diversity of each group to determine the preliminary effect of BAs on the gut microbiota of geese. We found that the addition of BAs increased both richness and evenness, which differs from previous studies (Yang et al., 2022). The addition of BAs to the diets of piglets and laying hens did not significantly affect ɑ-diversity, which could be due to differences in BAs concentration or different gut segments. However, BAs had a similar effect on β-diversity (Liu et al., 2022a,b). The PCoA results showed that the addition of 150 and 300 mg/kg significantly altered the community structure of the gut microbiota. The diversity of gut microbiota is a dynamic process that changes over time. It is generally believed that a high degree of diversity and evenness are signs of mature gut microbiota (Micah et al., 2007).
Bile acids can affect the phylum-level composition of gut microbiota. Similar to our experiment, it has been reported that the supplementation of BAs in rats increased the abundance of Firmicutes and decreased the abundance of Bacteroidetes (Islam et al., 2011; Zheng et al., 2017). A similar trend was also observed in laying hens during the late laying period after supplementation with BAs (Yang et al., 2022). Research has shown a positive correlation between the abundance of Firmicutes and the absorption of energy and nutrients, while an increase in Bacteroidetes may lead to a decrease in nutrient digestibility (Jumpertz et al., 2011). Our study also found that the addition of BAs significantly reduced the abundance of Proteobacteria in the cecum. The acidic nature of BAs may have inhibited the growth of Proteobacteria, which is considered beneficial as bacteria in this phylum can often cause gut inflammation (Mukhopadhya et al., 2012).
The relationship between BAs and gut microbiota is bidirectional. Gut microbiota can affect the metabolism of BAs by modulating the activity of BSH and by influencing a range of enzymes and pathways involved in BAs synthesis (Sun et al.,2023). Most studies have found that Lactobacillus and Bifidobacterium have higher abundance in the gut of animals supplemented with BAs. This may be due to their strong BSH activity and ability to remove cholesterol, as these bacteria can deconjugate BAs to form free BAs (Fiorucci and Distrutti, 2015; Song et al., 2019). In the present study, dominant genera in the cecum of geese, such as Bacteroides, Prevotella, and Faecalibacterium, were found to produce BSH (Labbé et al., 2014; Song et al., 2019; Burns et al., 2021). We noticed that they are all bacteria that can produce SCFA. Studies have found that there is a mechanism of mutual regulation between SCFA and BAs (Zeng et al., 2019). Through LEfSe analysis, we found that Blautia and Clostridium were significantly enriched in the 150 mg/kg BAs group. Blautia is a type of probiotic bacteria that can participate in carbohydrate metabolism and fatty acid production. Previous study have demonstrated a negative correlation between Blautia and visceral fat area (Liu et al., 2021). Additionally, Clostridium can utilize BAs as a substrate for growth and replication (Burns et al., 2010). Furthermore, BAs can also inhibit the growth of other competing bacterial populations (Sannasiddappa et al., 2017), which may provide more favorable conditions for the growth of Clostridium. According to Chen et al. (2020)‘s research, Clostridium are crucial in the cecal metabolism of BAs in mice, resulting in a decrease in the number of other gut bacteria and the amount of BAs in feces. Furthermore, our study found that supplementing BAs in the cecum resulted in a significant change in the amount of SCFA in the gut, This is correlated with the total concentration of SCFA detected in the cecum and jejunum. It is generally recognized that a higher concentration of SCFA is beneficial for animals, as they play a crucial role in maintaining intestinal mucosal health, regulating intestinal pH, modulating energy metabolism by promoting glucose metabolism and fatty acid oxidation (Den et al., 2013), and enhancing intestinal immunity (Xu et al., 2020). These findings suggest that the relationship between BAs, intestinal microbiota, and SCFA is bidirectional, and that BAs can elevate SCFA levels, thereby promoting the health of geese.
In summary, dietary supplementation with 150 mg/kg BAs was found to reduce the F/G in Holdobagy geese at 35–63 days. BAs, as an effective dietary additive, can improve lipid metabolism and gut health of geese by improving intestinal morphology, mucosal barrier, intestinal SCFA concentration and change the microbial community structure in the cecum.
Data availability statement
The datasets presented in this study can be found in online repositories. The names of the repository/repositories and accession number(s) can be found in the article/supplementary material.
Ethics statement
The animal study was reviewed and approved by Laboratory Animal Ethics Committee of the Shanghai Academy of Agricultural Sciences.
Author contributions
GL and XW contributed equally to this study, covering conceptualization, methodology, investigation, and original draft preparation. YL, SG, YY, and CW assisted with data collecting, formal analysis, and review and editing. HW and DH co-supervised the project and provided the funding. All authors have read and approved the final manuscript.
Funding
This research was funded by the Climbing Plan of Shanghai Academy of Agricultural Sciences (PG21171), the SAAS Program for Excellent Research Team (2022–021), and the China Agriculture Research System of MOF and MARA (CARS-42-35).
Conflict of interest
The authors declare that the research was conducted in the absence of any commercial or financial relationships that could be construed as a potential conflict of interest.
Publisher’s note
All claims expressed in this article are solely those of the authors and do not necessarily represent those of their affiliated organizations, or those of the publisher, the editors and the reviewers. Any product that may be evaluated in this article, or claim that may be made by its manufacturer, is not guaranteed or endorsed by the publisher.
References
Adachi, R. (2018). Bile acids and their derivatives as vitamin D receptor agonists: molecular mechanism and biological actions. Doctoral dissertation, University of Tsukuba.
Adam, A. H., Verdegem, M., Soliman, A. A., Zaki, M., Khalil, R. H., Nour, A. E. M., et al. (2023). Effect of dietary bile acids: growth performance, immune response, genes expression of fatty acid metabolism, intestinal, and liver morphology of striped catfish (Pangasianodon hypophthalmus). Aquacul. Rep. 29:101510. doi: 10.1016/j.aqrep.2023.101510
Arthur, S., Chaffins, S., Palaniappan, B., and Sundaram, U. (2019). Effect of Farnesoid X receptor activation on bile acid absorption associated proteins in intestinal epithelial cells. Gastroenterology 156:S-95. doi: 10.1016/S0016-5085(19)37023-4
Burgain, J., Gaiani, C., Francius, G., Revol-Junelles, A. M., Cailliez-Grimal, C., Lebeer, S., et al. (2013). In vitro interactions between probiotic bacteria and milk proteins probed by atomic force microscopy. Colloid Surface B 104, 153–162. doi: 10.1016/j.colsurfb.2012.11.032
Burns, D. A., Heap, J. T., and Minton, N. P. (2010). SleC is essential for germination of Clostridium difficile spores in nutrient-rich medium supplemented with the bile salt taurocholate. J. Bacteriol. 192, 657–664. doi: 10.1128/JB.01209-09
Burns, G. L., Hoedt, E. C., Walker, M. M., Talley, N. J., and Keely, S. (2021). Physiological mechanisms of unexplained (functional) gastrointestinal disorders. J. Physiol. 599, 5141–5161. doi: 10.1113/JP281620
Chen, J., Chen, Z., Wang, Z., Zheng, A., Chang, W., Cai, H., et al. (2022). Dietary 5-aminolevulinic acid supplementation improves growth performance, nutrient utilisation, iron status and antioxidant capacity of broilers. Ital. J. Anim. Sci. 21, 445–454. doi: 10.1080/1828051X.2022.2034541
Chen, D., Jin, D., Huang, S., Wu, J., Xu, M., Liu, T., et al. (2020). Clostridium butyricum, a butyrate-producing probiotic, inhibits intestinal tumor development through modulating Wnt signaling and gut microbiota. Cancer Lett. 469, 456–467. doi: 10.1016/j.canlet.2019.11.019
Chiang, J. Y. L., and Ferrell, J. M. (2020). Bile acid biology, pathophysiology, and therapeutics. Clin. Liver Dis. 15, 91–94. doi: 10.1002/cld.861
Chinetti, G., Fruchart, J. C., and Staels, B. (2000). Peroxisome proliferator-activated receptors (PPARs): nuclear receptors at the crossroads between lipid metabolism and inflammation. Inflam Res 49, 497–505. doi: 10.1007/s000110050622
Crespo, N., and Esteve-Garcia, E. (2001). Dietary fatty acid profile modifies abdominal fat deposition in broiler chickens. Poult. Sci. 80, 71–78. doi: 10.1093/ps/80.1.71
Den, B. G., Van, E. K., Groen, A. K., Venema, K., Reijngoud, D. J., and Bakker, B. M. (2013). The role of short-chain fatty acids in the interplay between diet, gut microbiota, and host energy metabolism. J. Lipid Res. 54, 2325–2340. doi: 10.1194/jlr.R036012
Dihingia, A., Bordoloi, J., Dutta, P., Kalita, J., and Manna, P. (2018). Hexane-isopropanolic extract of Tungrymbai, a north-east Indian fermented soybean food prevents hepatic steatosis via regulating AMPK-mediated SREBP/FAS/ACC/HMGCR and PPARα/CPT1A/UCP2 pathways. Sci. Rep. 8:10021. doi: 10.1038/s41598-018-27607-7
Duskaev, G., Kvan, O., Vershinina, I., and Rakhmatullin, S. (2021). Assessment of lipid metabolism in broilers against plant extract and probiotic substance or their combined use. Iran. J. Appl. Anim. Sci. 11, 161–168.
El-Shenawy, A. M., Abeer, E. K. M., Alsokary, E. T., and Gad, D. M. (2020). Impact of carbohydrate to lipid ratio and bile salts supplementation on performance, body gain and body composition of Nile tilapia fish. Int. J. Fish. Aquat. Stud. 8, 88–97.
Fiorucci, S., and Distrutti, E. (2015). Bile acid-activated receptors, intestinal microbiota, and the treatment of metabolic disorders. Trends Mol. Med. 21, 702–714. doi: 10.1016/j.molmed.2015.09.001
Fuchs, M. (2003). III. Regulation of bile acid synthesis: past progress and future challenges. Am. J. Physiol.-Gastr L. 284, G551–G557. doi: 10.1152/ajpgi.00468.2002
Gao, R., Meng, X., Xue, Y., Mao, M., Liu, Y., Tian, X., et al. (2022). Bile acids-gut microbiota crosstalk contributes to the improvement of type 2 diabetes mellitus. Front. Pharmacol. 13:1027212. doi: 10.3389/fphar.2022.1027212
Gilani, S., Howarth, G. S., Nattrass, G., Kitessa, S. M., Barekatain, R., Forder, R. E. A., et al. (2018). Gene expression and morphological changes in the intestinal mucosa associated with increased permeability induced by short-term fasting in chickens. J. Anim. Physiol. Anim. Nutr. (Berl) 102, e653–e661. doi: 10.1111/jpn.12808
Glasser, A. L., Boudeau, J., Barnich, N., Perruchot, M. H., Colombel, J. F., and Darfeuille-Michaud, A. (2001). Adherent invasive Escherichia coli strains from patients with Crohn's disease survive and replicate within macrophages without inducing host cell death. Infect. Immun. 69, 5529–5537. doi: 10.1128/IAI.69.9.5529-5537.2001
Gu, Y. F., Chen, Y. P., Jin, R., Wang, C., Wen, C., and Zhou, Y. M. (2022). Dietary chitooligosaccharide supplementation alleviates intestinal barrier damage, and oxidative and immunological stress in lipopolysaccharide-challenged laying hens. Poultry Sci. 101:101701. doi: 10.1016/j.psj.2022.101701
Harwood, H. J. (2004). Acetyl-CoA carboxylase inhibition for the treatment of metabolic syndrome. Curr Opini Investigational Dr 5, 283–289.
Hofmann, A. F., and Hagey, L. R. (2008). Bile acids: chemistry, pathochemistry, biology, pathobiology, and therapeutics. Cell. Mol. Life Sci. 65, 2461–2483. doi: 10.1007/s00018-008-7568-6
Horton, J. D., Goldstein, J. L., and Brown, M. S. (2002). SREBPs: activators of the complete program of cholesterol and fatty acid synthesis in the liver. J. Clin. Invest. 109, 1125–1131. doi: 10.1172/JCI0215593
Hu, J., Hong, W., Yao, K. N., Zhu, X. H., Chen, Z. Y., and Ye, L. (2019). Ursodeoxycholic acid ameliorates hepatic lipid metabolism in LO2 cells by regulating the AKT/mTOR/SREBP-1 signaling pathway. World J. Gastroenterol. 25, 1492–1501. doi: 10.3748/wjg.v25.i12.1492
Iji, P. A., Saki, A., and Tivey, D. R. (2001). Body and intestinal growth of broiler chicks on a commercial starter diet. 2. Development and characteristics of intestinal enzymes. Br. Poult. Sci. 42, 514–522. doi: 10.1080/00071660120073142
Inagaki, T., Moschetta, A., Lee, Y. K., Peng, L., Zhao, G., Downes, M., et al. (2006). Regulation of antibacterial defense in the small intestine by the nuclear bile acid receptor. Proc. Natl. Acad. Sci. U. S. A. 103, 3920–3925. doi: 10.1073/pnas.0509592103
Islam, K. S., Fukiya, S., Hagio, M., Fujii, N., Ishizuka, S., Ooka, T., et al. (2011). Bile acid is a host factor that regulates the composition of the cecal microbiota in rats. Gastroenterology 141, 1773–1781. doi: 10.1053/j.gastro.2011.07.046
Jain, A. K., Stoll, B., Burrin, D. G., Holst, J. J., and Moore, D. D. (2012). Enteral bile acid treatment improves parenteral nutrition-related liver disease and intestinal mucosal atrophy in neonatal pigs. Am. J. Physiol.-Gastr L. 302, G218–G224. doi: 10.1152/ajpgi.00280.2011
Jumpertz, R., Le, D. S., Turnbaugh, P. J., Trinidad, C., Bogardus, C., Gordon, J. I., et al. (2011). Energy-balance studies reveal associations between gut microbes, caloric load, and nutrient absorption in humans. Am. J. Clin. Nutr. 94, 58–65. doi: 10.3945/ajcn.110.010132
Labbé, A., Ganopolsky, J. G., Martoni, C. J., Prakash, S., and Jones, M. L. (2014). Bacterial bile metabolising gene abundance in Crohn's, ulcerative colitis and type 2 diabetes metagenomes. PLoS One 9:e115175. doi: 10.1371/journal.pone.0115175
Langhi, C., Le May, C., Kourimate, S., Caron, S., Staels, B., Krempf, M., et al. (2008). Activation of the farnesoid X receptor represses PCSK9 expression in human hepatocytes. FEBS Lett. 582, 949–955. doi: 10.1016/j.febslet.2008.02.038
Lefebvre, P., Cariou, B., Lien, F., Kuipers, F., and Staels, B. (2009). Role of bile acids and bile acid receptors in metabolic regulation. Physiol. Rev. 89, 147–191. doi: 10.1152/physrev.00010.2008
Li, T., and Apte, U. (2015). Bile acid metabolism and signaling in cholestasis, inflammation, and cancer. Adv. Pharmacol. 74, 263–302. doi: 10.1016/bs.apha.2015.04.003
Li, T., and Chiang, J. Y. (2014). Bile acid signaling in metabolic disease and drug therapy. Pharmacol. Rev. 66, 948–983. doi: 10.1124/pr.113.008201
Li, S., Li, C., and Wang, W. (2019). Bile acid signaling in renal water regulation. Am. J. Physiol. Renal Physiol. 317, F73–F76. doi: 10.1152/ajprenal.00563.2018
Li, X., Shi, M., Chen, L., Zhang, S., Chi, S., Dong, X., et al. (2023). Effects of bile acids supplemented into low fishmeal diet on growth, molting, and intestinal health of Pacific white shrimp. Aquacul Rep 29:101491. doi: 10.1016/j.aqrep.2023.101491
Liao, Z. B., Sun, B., Zhang, Q. G., Jia, L. L., Wei, Y. L., Liang, M. Q., et al. (2020). Dietary bile acids regulate the hepatic lipid homeostasis in tiger puffer fed normal or high-lipid diets. Aquaculture 519:734935. doi: 10.1016/j.aquaculture.2020.734935
Liu, Y., Azad, M. A. K., Kong, X., Zhu, Q., and Yu, Z. (2022a). Dietary bile acids supplementation modulates immune response, antioxidant capacity, glucose, and lipid metabolism in normal and intrauterine growth retardation piglets. Front. Nutr. 9:991812. doi: 10.3389/fnut.2022.991812
Liu, Y., Azad, M. A. K., Zhu, Q., Yu, Z., and Kong, X. (2022b). Dietary bile acid supplementation alters plasma biochemical and hormone indicators, intestinal digestive capacity, and microbiota of piglets with normal birth weight and intrauterine growth retardation. Front. Microbiol. 13:1053128. doi: 10.3389/fmicb.2022.1053128
Liu, X., Mao, B., Gu, J., Wu, J., Cui, S., Wang, G., et al. (2021). Blautia—a new functional genus with potential probiotic properties? Gut Microbes 13, 1–21. doi: 10.1080/19490976.2021.1875796
Ma, H., Liang, S., Wu, H., Du, C., Ren, Z., Yang, X., et al. (2022). Effects of in ovo feeding and dietary addition oils on growth performance and immune function of broiler chickens. Poult. Sci. 101:101815. doi: 10.1016/j.psj.2022.101815
Martel, J., Chang, S. H., Ko, Y. F., Hwang, T. L., Young, J. D., and Ojcius, D. M. (2022). Gut barrier disruption and chronic disease. Trends Endocrinol. Metab. 33, 247–265. doi: 10.1016/j.tem.2022.01.002
Micah, H., Claire, F.-L., Rob, K., and Gordon Jeffrey, I. (2007). The human microbiome project: exploring the microbial part of ourselves in a changing world. Nature 449, 804–810. doi: 10.1038/nature06244
Mukhopadhya, I., Hansen, R., El-Omar, E. M., and Hold, G. L. (2012). IBD- what role Proteobacteria play? Nat. Rev. Gastroenterol. Hepatol. 9, 219–230. doi: 10.1038/nrgastro.2012.14
Nguyen, H. P., and Van Do, T. (2021). Digested soybean protein and taurine influence bile acid level, lipase activity, lipid digestibility, and growth performance of pompano (Trachinotus blochii). Fish Physiol. Biochem. 47, 1199–1209. doi: 10.1007/s10695-021-00972-3
Parsaie, S., Shariatmadari, F., Zamiri, M. J., and Khajeh, K. (2007). Influence of wheat-based diets supplemented with xylanase, bile acid and antibiotics on performance, digestive tract measurements and gut morphology of broilers compared with a maize-based diet. Br. Poult. Sci. 48, 594–600. doi: 10.1080/00071660701615788
Perrone, E. E., Chen, C., Longshore, S. W., Okezie, O., Warner, B. W., Sun, C. C., et al. (2010). Dietary bile acid supplementation improves intestinal integrity and survival in a murine model. J. Pediatr. Surg. 45, 1256–1265. doi: 10.1016/j.jpedsurg.2010.02.094
Ramírez, P. O., Cruz, R. V., Chinchilla, L. P., and Méndez, S. N. (2017). The role of the gut microbiota in bile acid metabolism. Ann. Hepatol. 16, S21–S26. doi: 10.5604/01.3001.0010.5672
Reschly, E. J., Ai, N., Ekins, S., Welsh, W. J., Hagey, L. R., Hofmann, A. F., et al. (2008). Evolution of the bile salt nuclear receptor FXR in vertebrates. J. Lipid Res. 49, 1577–1587. doi: 10.1194/jlr.M800138-JLR200
Saeed, A., Hoekstra, M., Hoeke, M. O., Heegsma, J., and Faber, K. N. (2017). The interrelationship between bile acid and vitamin a homeostasis. BBA-Mol. Cell Biol. L. 1862, 496–512. doi: 10.1016/j.bbalip.2017.01.007
Saminathan, M., Mohamed, W. N. W., Noh, A. M., Ibrahim, N. A., Fuat, M. A., and Ramiah, S. K. (2022). Effects of dietary palm oil on broiler chicken productive performance and carcass characteristics: a comprehensive review. Trop. Anim. Health Prod. 54:64. doi: 10.1007/s11250-022-03046-5
Sannasiddappa, T. H., Lund, P. A., and Clarke, S. R. (2017). In vitro antibacterial activity of unconjugated and conjugated bile salts on Staphylococcus aureus. Front. Microbiol. 8:1581. doi: 10.3389/fmicb.2017.01581
Sanz, M. (1999). Higher lipid accumulation in broilers fed on saturated fats than in those fed on unsaturated fats. Br. Poult. Sci. 40, 95–101. doi: 10.1080/00071669987908
Smith, S. (1994). The animal fatty acid synthase: one gene, one polypeptide, seven enzymes. FASEB J. 8, 1248–1259. doi: 10.1096/fasebj.8.15.8001737
Song, Z., Cai, Y., Lao, X., Wang, X., Lin, X., Cui, Y., et al. (2019). Taxonomic profiling and populational patterns of bacterial bile salt hydrolase (BSH) genes based on worldwide human gut microbiome. Microbiome 7, 9–16. doi: 10.1186/s40168-019-0628-3
Song, J., Li, Q., Li, P., Liu, R., Cui, H., Zheng, M., et al. (2018). The effects of inulin on the mucosal morphology and immune status of specific pathogen-free chickens. Poult. Sci. 97, 3938–3946. doi: 10.3382/ps/pey260
Stojancevic, M., Stankov, K., and Mikov, M. (2012). The impact of farnesoid X receptor activation on intestinal permeability in inflammatory bowel disease. Can. J. Gastroenterol. 26, 631–637. doi: 10.1155/2012/538452
Sun, L., Zhang, Y., Cai, J., Rimal, B., Rocha, E. R., Coleman, J. P., et al. (2023). Bile salt hydrolase in non-enterotoxigenic Bacteroides potentiates colorectal cancer. Nat. Commun. 14:755. doi: 10.1038/s41467-023-36089-9
Vertiprakhov, V. G., Grozina, A. A., Ovchinnikova, N. V., and Kislova, I. V. (2022). Age-related changes in enzyme activity in pancreas, intestines, and blood plasma of broiler chickens of the Smena 9 breed. Russ. Agric. Sci. 48, 302–308. doi: 10.3103/S1068367422040140
Wang, R. L., Chen, J. W., Gooneratne, R., He, X. P., Huang, J. Z., and Zhao, Z. H. (2022). Effects of varied molecular weight of chitosan oligosaccharides on growth performance, carcass trait, meat quality, and fat metabolism in indigenous yellow-feathered chickens. J. Appl. Poult. Res. 31:100221. doi: 10.1016/j.japr.2021.100221
Wang, H., Chen, J., Hollister, K., Sowers, L. C., and Forman, B. M. (1999). Endogenous bile acids are ligands for the nuclear receptor FXR/BAR. Mol. Cell 3, 543–553. doi: 10.1016/S1097-2765(00)80348-2
Wang, D. Q. H., Tazuma, S., Cohen, D. E., and Carey, M. C. (2003). Feeding natural hydrophilic bile acids inhibits intestinal cholesterol absorption: studies in the gallstone-susceptible mouse. Am J Physiol Gastr L 285, G494–G502. doi: 10.1152/ajpgi.00156.2003
Watanabe, M., Horai, Y., Houten, S. M., Morimoto, K., Sugizaki, T., Arita, E., et al. (2011). Lowering bile acid pool size with a synthetic farnesoid X receptor (FXR) agonist induces obesity and diabetes through reduced energy expenditure. J. Biol. Chem. 286, 26913–26920. doi: 10.1074/jbc.M111.248203
Watanabe, M., Houten, S. M., Mataki, C., Christoffolete, M. A., Kim, B. W., Sato, H., et al. (2006). Bile acids induce energy expenditure by promoting intracellular thyroid hormone activation. Nature 439, 484–489. doi: 10.1038/nature04330
Wu, H., Liu, G., He, Y., Da, J., and Xie, B. (2019). Obeticholic acid protects against diabetic cardiomyopathy by activation of FXR/Nrf2 signaling in db/db mice. Eur. J. Pharmacol. 858:172393. doi: 10.1016/j.ejphar.2019.05.022
Xu, W., Lin, L., Liu, A., Zhang, T., Zhang, S., Li, Y. H., et al. (2020). L-theanine affects intestinal mucosal immunity by regulating short-chain fatty acid metabolism under dietary fiber feeding. Food Funct. 11, 8369–8379. doi: 10.1039/D0FO01069C
Xu, J., Shi, M., Chen, L., Chi, S., Zhang, S., Cao, J., et al. (2023). Muscular lipidomics and transcriptomics reveal the effects of bile acids on lipid metabolism in high-fat diet-fed grouper. Fish Physiol. Biochem. 23, 1–17. doi: 10.1007/s10695-023-01176-7
Yang, B., Huang, S., Zhao, G., and Ma, Q. (2022). Dietary supplementation of porcine bile acids improves laying performance, serum lipid metabolism and cecal microbiota in late-phase laying hens. Anim Nutr 11, 283–292. doi: 10.1016/j.aninu.2022.08.003
Yousefi, J., Taherpour, K., Ghasemi, H. A., Akbari Gharaei, M., Mohammadi, Y., and Rostami, F. (2023). Effects of emulsifier, betaine, and L-carnitine on growth performance, immune response, gut morphology, and nutrient digestibility in broiler chickens exposed to cyclic heat stress. Br. Poult. Sci. 1-14, 1–14. doi: 10.1080/00071668.2022.2160626
Zahid, M. U., Khalique, A., Qaisrani, S. N., Ashraf, M., Sheikh, A. A., and Yaqoob, M. U. (2023). The effect of Acacia nilotica bark extract on growth performance, carcass characteristics, immune response, and intestinal morphology in broilers as an alternative to antibiotic growth promoter. Anim. Biosci. doi: 10.5713/ab.22.0284
Zeng, H., Umar, S., Rust, B., Lazarova, D., and Bordonaro, M. (2019). Secondary bile acids and short chain fatty acids in the colon: a focus on colonic microbiome, cell proliferation, inflammation, and cancer. In J Mol Sci 20:1214. doi: 10.3390/ijms20051214
Keywords: bile acids, growth, lipid, gut, cecal microbiota
Citation: Li G, Wang X, Liu Y, Gong S, Yang Y, Wang C, Wang H and He D (2023) Bile acids supplementation modulates lipid metabolism, intestinal function, and cecal microbiota in geese. Front. Microbiol. 14:1185218. doi: 10.3389/fmicb.2023.1185218
Edited by:
Michael Kogut, United States Department of Agriculture, United StatesReviewed by:
Yu Pi, Chinese Academy of Agricultural Sciences, ChinaHao Bai, Yangzhou University, China
Mariano Enrique Fernández-Miyakawa, Instituto Nacional de Tecnología Agropecuaria, Argentina
Copyright © 2023 Li, Wang, Liu, Gong, Yang, Wang, Wang and He. This is an open-access article distributed under the terms of the Creative Commons Attribution License (CC BY). The use, distribution or reproduction in other forums is permitted, provided the original author(s) and the copyright owner(s) are credited and that the original publication in this journal is cited, in accordance with accepted academic practice. No use, distribution or reproduction is permitted which does not comply with these terms.
*Correspondence: Huiying Wang, eWpzaHl3YW5nQHNpbmEuY29t; Daqian He, ZGFxaWFuaGVAYWxpeXVuLmNvbQ==