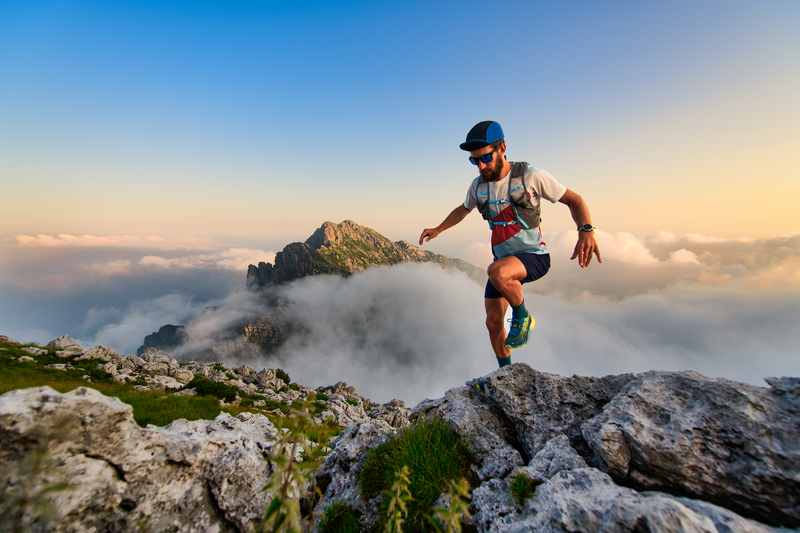
94% of researchers rate our articles as excellent or good
Learn more about the work of our research integrity team to safeguard the quality of each article we publish.
Find out more
ORIGINAL RESEARCH article
Front. Microbiol. , 10 August 2023
Sec. Microbial Symbioses
Volume 14 - 2023 | https://doi.org/10.3389/fmicb.2023.1183627
This article is part of the Research Topic Women in Microbial Symbioses: 2022/2023 View all 6 articles
Introduction: The associated diverse microbiome contributes to the overall fitness of Aurelia aurita, particularly to asexual reproduction. However, how A. aurita maintains this specific microbiome or reacts to manipulations is unknown.
Methods: In this report, the response of A. aurita to manipulations of its native microbiome was studied by a transcriptomics approach. Microbiome-manipulated polyps were generated by antibiotic treatment and challenging polyps with a non-native, native, and potentially pathogenic bacterium. Total RNA extraction followed by RNAseq resulted in over 155 million reads used for a de novo assembly.
Results: The transcriptome analysis showed that the antibiotic-induced change and resulting reduction of the microbiome significantly affected the host transcriptome, e.g., genes involved in processes related to immune response and defense mechanisms were highly upregulated. Similarly, manipulating the microbiome by challenging the polyp with a high load of bacteria (2 × 107 cells/polyp) resulted in induced transcription of apoptosis-, defense-, and immune response genes. A second focus was on host-derived quorum sensing interference as a potential defense strategy. Quorum Quenching (QQ) activities and the respective encoding QQ-ORFs of A. aurita were identified by functional screening a cDNA-based expression library generated in Escherichia coli. Corresponding sequences were identified in the transcriptome assembly. Moreover, gene expression analysis revealed differential expression of QQ genes depending on the treatment, strongly suggesting QQ as an additional defense strategy.
Discussion: Overall, this study allows first insights into A. aurita’s response to manipulating its microbiome, thus paving the way for an in-depth analysis of the basal immune system and additional fundamental defense strategies.
Cnidarians, such as the moon jellyfish Aurelia aurita, are distributed worldwide and play essential roles in shaping marine ecosystems (Brekhman et al., 2015). Cnidaria are dated back to about 700 million years and are considered a sister group to the Bilateria (Putnam et al., 2007; Park et al., 2012). Thus, they are among the simplest animals at the tissue level organization possessing two germ layers (ectoderm and endoderm) separated by the mesoglea (Ball et al., 2004). In addition to their morphological simplicity, many Cnidaria, particularly Scyphozoa, have a high level of developmental plasticity, allowing for an enormous tolerance, regeneration potential, and asexual proliferation during their life cycle (Richardson et al., 2009). Cnidaria have evolved and are constantly exposed to diverse microorganisms (Liu et al., 2019). This close association with microorganisms has profound effects on various host functions. Recent studies have demonstrated that specific host-associated microbiota can contribute to various host functions. Examples are host metabolism (Ochsenkühn et al., 2017), development (Rook et al., 2017), organ morphogenesis (Sommer and Bäckhed, 2013), pathogen protection and immunity (Moran and Yun, 2015), behavior (Ezenwa et al., 2012), environmental sensing and adaptation (Bang et al., 2018; Ziegler et al., 2019), developmental transitions (Webster and Reusch, 2017; Woznica et al., 2017), and reproduction (Chilton et al., 2015; Jacob et al., 2015).
Evidently, Cnidarians are constantly exposed to microbes in the environment; consequently, molecular analyses have revealed a variety of molecular pathways to respond to microbial exposure (Dierking and Pita, 2020). In the first step, extracellular surface receptors recognize microbe-associated molecular patterns (MAMPs) during microbial epithelium colonization (Chu and Mazmanian, 2013). MAMPs include lipopolysaccharides (LPSs), peptidoglycan (PGN), flagellin, and microbial nucleic acids (Rosenstiel et al., 2009). In the first line of defense, antimicrobial peptides (AMPs) regulate establishing and maintaining a specific microbiota (Bosch, 2013; Bosch and Zasloff, 2021). Toll-like receptors (TLRs) at the host cell surface further perceive the MAMP signal, initiating MAMP-triggered immunity (Augustin et al., 2010; Bosch, 2013). Downstream of those conserved signaling cascades are stress-responsive transcription factors, including eukaryotic transcription factors of the proteins’ NF-kappaB (NF-kB) family (Zheng et al., 2005). Recent studies revealed that eukaryotic hosts also use quorum quenching (QQ) as a strategy to respond to bacterial colonization (Grandclâment et al., 2016). The hosts interfere with the small molecule-dependent bacterial communication through enzymatic degradation of the autoinducer, blocking autoinducer production, or its reception to control population-dependent behaviors like colonization, biofilm formation, and pathogenesis (Kiran et al., 2017; Mukherjee and Bassler, 2019). In the Cnidarian Hydra, the autoinducer signaling molecule 3-oxo-homoserine lactone has been shown to be converted into the inactive 3-hydroxy counterpart by a host-derived oxidoreductase allowing host colonization of the main colonizer Curvibacter sp. (Pietschke et al., 2017).
The Cnidarian A. aurita harbors a highly diverse and dynamic microbiota specific to the animal, the different sub-populations, and life stages (Weiland-Bräuer et al., 2015a). In the absence of the specific microbial community, the fitness of A. aurita was significantly compromised, and notably, asexual reproduction was almost halted (Weiland-Bräuer et al., 2020a). This microbial impact is crucial at the polyp life stage before entering the process of asexual offspring production (strobilation) to ensure a normal progeny output (Jensen et al., 2023). Moreover, in A. aurita, three proteins interfering with bacterial QS were identified (Weiland-Bräuer et al., 2019). Incubation of native animals with potentially pathogenic bacteria induced the expression of the identified QQ-ORFs, strongly suggesting a host defense strategy.
Despite the growing knowledge about the impact of microbes on the host and the fundamental strategies of the host to respond, research to understand how microbiomes influence host gene expression is still in its infancy (Nichols and Davenport, 2021). Many studies of model organisms and humans demonstrated an interlinkage between the microbiome and the host’s gene expression. However, the direction of causality mainly remained unanswered (Nichols and Davenport, 2021). Comparing conventional (microbiome-containing) to germ-free systems is one way to assess whether the microbiome plays a causative role in regulating gene expression (Bäckhed et al., 2012; Al-Asmakh and Zadjali, 2015; Fu et al., 2017; Pierre, 2022). Genome-wide transcriptomic analyses are now routinely used to quantify the changing levels of each transcript under different conditions (Conesa et al., 2016).
In the present study, we aimed to determine the influence of the associated microbiota on A. aurita’s gene expression. After microbiome manipulation (by antibiotic treatment or bacterial challenge), RNA was extracted from polyps, followed by RNA-Seq and a de novo transcriptome assembly. Gene ontology categories and gene expression patterns were analyzed to elucidate how A. aurita recognizes and responds to the manipulation of its native microbiome and the presence of potential pathogens. A particular focus was on host-derived QQ activities as an additional potential defense strategy.
Husbandry is described in detail by Weiland-Bräuer et al. (2015a, 2020a). Briefly, polyps of the sub-population North Atlantic (Roscoff, France) were kept in the lab in 2-liter plastic tanks in 3% artificial seawater (ASW) (tropical sea salts; Tropic Marin). Polyps were fed twice a week with freshly hatched Artemia salina (HOBBY, Grafschaft-Gelsdorf, Germany).
Single native polyps were placed in 48 well multiwell plates in 1 mL ASW supplemented with an antibiotic mixture (Provasoli’s antibiotic mixture with final concentrations of 360,000 U/L penicillin G, 1.5 mg/L chloramphenicol, 1.8 mg/L neomycin, and 9,000 U/L polymyxin B; all components from Carl Roth, Karlsruhe, Germany). No food was provided during the antibiotic treatment. The reduction and consequent change of the microbiota were tested by plating a single homogenized polyp (10 replicates) on Marine Bouillon agar plates (Carl Roth, Karlsruhe, Germany). Plates were incubated for 5 days at 20°C. Colony forming units (cfu) were calculated, and an 87 ± 9% reduction per polyp was determined.
Bacteria (Pseudoalteromonas espejiana GenBank accession No. MK967174, and Vibrio anguillarum GenBank accession No. MK967055) for the microbial challenge were isolated from A. aurita polyps (Weiland-Bräuer et al., 2020b). Strains were grown in Marine Bouillon (MB; Carl Roth, Karlsruhe, Germany) at 30°C and 120 rpm to optical turbidity at 600 nm of 0.8. Klebsiella oxytoca M5aI (DSM No. 7342) was similarly grown in Luria-Bertani (LB) medium. Bacterial cell numbers were determined using a Neubauer count chamber (Assistant, Sondheim vor der Röhn, Germany). Pools of 20 native A. aurita polyps were separated in 6-well multiwell plates (Greiner, Kremsmünster, Austria) in 4 mL 3% ASW after washing them twice with sterile ASW. A pool of 20 native A. aurita polyps was incubated with 108 cells/mL (in 4 mL) of the respective strain at 20°C for 30 min. Next, polyps were washed twice with sterile ASW to remove the bacteria and used to isolate total RNA.
The North Atlantic sub-population polyps were generated from a single mother polyp by clonal budding. Pools of 20 daughter polyps were separated into 6-well plates in 4 mL 3% ASW. Polyps were kept in five conditions without food supply: 1. native polyps without treatment, 2. antibiotic (AB)-treated polyps, 3. native polyps challenged with 108 cells/mL Klebsiella oxytoca M5aI, 4. native polyps challenged with 108 cells/mL Pseudoalteromonas espejiana, and 5. native polyps challenged with 108 cells/mL Vibrio anguillarum (Figure 1). Three replicates (3 × 20 polyps) were used for each condition. For the bacterial challenges, 20 polyps in 4 mL ASW were supplemented with 4×108 cells and incubated for 30 min (Figure 1). The total RNA was extracted from each pool of polyps.
Figure 1. Experimental setup. RNA extraction with subsequent RNAseq and analysis was conducted on a pool of 20 polyps (3 biological replicates). Polyps were kept under native and microbiome-manipulated conditions. Created with BioRenders.
Total RNA of a pool of 20 A. aurita polyps was isolated with an adapted protocol of Gold et al. (2019). In more detail, polyps were washed three times with sterile ASW (to remove antibiotic residues) and homogenized with a motorized pestle. RiboLock RNase Inhibitor (40 U/μL, Thermo Fisher Scientific, Waltham/Massachusetts, United States), 200 μL lysis solution (100 mM Tris/HCl, pH 5.5, 10 mM disodium EDTA, 0.1 M NaCl, 1% SDS, 1% ß-mercaptoethanol), and 2 μL Proteinase K (25 mg/mL, Thermo Fisher Scientific, Waltham/Massachusetts, United States) were added to the homogenate and incubated for 10 min at 55°C. Chilled solutions were added with 5 μL of 3 M sodium acetate (pH 5.2) and 250 μL phenol-chloroform-isoamyl alcohol (25:24:1) and incubated for 15 min on ice prior to 15 min centrifugation at 12,000 x g at 4°C. The upper phase was mixed with 1 volume 2-propanol, and precipitation occurred at-80°C overnight. The precipitate was centrifuged for 15 min at 12,000 x g at 4°C. The pellet was washed twice with 70% ethanol before the air-dried pellet was dissolved in 25 μL RNase-free water. DNA contaminations were removed with Turbo DNA-free DNase (Thermo Fisher Scientific, Waltham/Massachusetts, United States). The RNA quality and quantity were assessed by NanoDrop1000 (Thermo Fisher Scientific, Waltham/Massachusetts, United States) and 1.5% agarose gel electrophoresis, and the cDNA library was prepared with DNA-free host RNA (500 ng) using the TruSeq Stranded mRNA Library Preparation kit. The library was sequenced with the NextSeq 500 System (Illumina, San Diego/ California, United States).
The raw reads were trimmed with Trimmomatic (Bolger et al., 2014) to remove bad-quality reads and the adapters. A de novo assembly with the trimmed sequences was conducted with the Trinity package v2.8.4 (Grabherr et al., 2011a,b). The quality of the de novo assembly was assessed with several parameters, such as the N50 value and the percentage of the assembly-mapped reads, which was calculated with Bowtie2. Finally, Benchmarking Universal Single-Copy Orthologs (BUSCO V2/3) against metazoan cassettes (Simão et al., 2015) was used to evaluate the completeness of the assembly regarding the core genes found in metazoans.
A Blastx of the transcriptome assembly was executed against the Swiss-Prot database for metazoans. For the gene expression analysis, reads were mapped to the reference assembly with Bowtie2 (Langmead and Salzberg, 2012). Transcript quantification was performed with RSEM (Li and Dewey, 2011). The differential gene expression (DGE) analysis was done with edgeR (Robinson et al., 2010; McCarthy et al., 2012). Pairwise comparisons between all experimental conditions were performed using FDR ≤ 0.001 and 2-fold changes as statistical parameters. Furthermore, GO enrichment analysis was conducted using a Fisher’s Exact Test in Blast2GOPRO (Conesa et al., 2005) with a value of p threshold of ≤0.05. Here, the total annotation file of the reference transcriptome was used as the “reference dataset,” whereas the upregulated genes in each condition served as the “test dataset.” Based on sequence depth and quality, only two out of three biological replicates were used for the gene expression analysis for the conditions of native polyps and native polyps under the bacterial challenges. Transcriptome data is deposited under the BioProject ID PRJNA938117. Gene expression patterns in the transcriptome data set were verified with qRT-PCRs of the housekeeping gene elongation factor 1 (EF1) (Weiland-Bräuer et al., 2019; Jensen et al., 2023). EF1 showed similar trends in gene expression for all treatments when comparing transcriptome data and qRT-PCR (Table 1). Moreover, the previously published QQ genes aaqq1, aaqq2, and aaqq3 of A. aurita (Weiland-Bräuer et al., 2019) were identified in the transcriptome data set. Their expression profiles have been studied using qRT-PCR in native, antibiotic-treated, and K. oxytoca-treated polyps [see study (Weiland-Bräuer et al., 2019)]. Gene expression profiles were similar in transcriptome data and qRT-PCR, which we rated and considered as internal control for our newly obtained data set and its validity (Table 1).
Quorum quenching (QQ) assays using the reporter strains AI1-QQ.1 and AI2-QQ.1 were performed with cell-free supernatants and cell extracts of EST clones from the A. aurita EST library as described (Weiland-Bräuer et al., 2015b). Briefly, QQ screening plates were prepared with LB agar containing 0.8% agar at 50°C supplemented with final concentrations of 100 μM N-(β-ketocaproyl)-l-homoserine lactone (3-oxo-C6-HSL) (Sigma-Aldrich, Munich, Germany), 100 μg/mL ampicillin, 30 μg/mL kanamycin, and 10% (vol/vol) exponentially growing culture of the reporter strain AI1-QQ.1. Similarly, AI-2 QQ screening plates were prepared with final concentrations of 50 mM 4-hydroxy-5-methyl-3-furanone (Sigma-Aldrich, Munich, Germany), 100 μg/mL ampicillin, 30 μg/mL kanamycin, and 5% (vol/vol) exponentially growing culture of the reporter strain AI2-QQ.1. LB agar plates were coated with the agar mixture. After 10 min, 5 μL of the test substances were applied, followed by overnight incubation at 37°C. QQ activities were visualized by the growth of the respective reporter strain. Preparation of cell extracts and cell-free culture supernatants was conducted with 5 mL overnight cultures of EST clones grown at 37°C and 120 rpm. Cells were harvested by centrifugation at 7,000 × g, and the culture supernatant was subsequently filtered using 0.2-μm centrifugal filter units (Carl Roth, Karlsruhe, Germany). The cell extract was prepared from the cell pellet using the Geno/Grinder 2000 (BT&C/OPS Diagnostics, Bridgewater, NJ). Cell extracts were filtered through 0.2-μm filter units.
Following the manufacturer’s protocol, the plasmids of identified QQ-active single EST clones were purified using the Presto Mini Plasmid kit (GeneAid, New Taipeh City, Taiwan). The respective inserts were Sanger sequenced at the Institute of Clinical Molecular Biology in Kiel with the primer set T7_Promoter (5′-TAATACGACTCACTATAGGG-3′) and T7_Reverse (5’-TAGTTATTGCTCAGCGGTGG-3′). Sequences are deposited at NCBI GenBank under Accession Nos. OQ581004 - OQ581041.
A transcriptomics approach was applied to gain insights into the response of A. aurita polyps to manipulation of its native, associated microbiota, with a particular focus on quorum sensing interference as a potential host defense strategy. In general, pools of 20 polyps were treated and processed together in the experiments, each with three biological replicates using the following treatments: native, antibiotic-treated polyps resulting in 87 ± 9% reduced microbial cells per polyp (cfu/polyp), and native polyps challenged with Klebsiella oxytoca M5aI, Pseudoalteromonas espejiana, or Vibrio anguillarum (2 × 107 cells/polyp, Figure 1). The respective pools were used for total RNA extraction followed by RNAseq and transcriptome analysis.
The RNAseq approach overall resulted in 167,269,257 raw reads. After quality trimming, 145,746,530 reads (87.1% of the total reads) remained for further analysis and were used for the de novo transcriptome assembly (Supplementary Table S1A). The de novo assembly resulted in 213,897 transcripts and 160,700 genes (Supplementary Table S1A) with an N50 value of 1,170 (Supplementary Table S1B). 94.25% of reads were successfully aligned back to the assembly, while the completeness of genes was 96.42% according to the BUSCO score for metazoans (Supplementary Table S1B). 30% (597,796 transcripts) of the assembly revealed an annotation against the Swiss-Prot database for metazoans (Supplementary Table S2).
Transcriptome analysis identified five separated clusters corresponding to the different conditions, while biological replicates of a condition cluster together (Figure 2). It should be noted that three replicates were only analyzed for antibiotic (AB)-treated polyps, as all other conditions resulted in an unacceptable low sequence depth for one replicate. Notably, two clusters were identified within the hierarchical clustering of transcripts. Here, native and AB-treated conditions yielded a tremendous difference. AB-treated polyps showed a massive reduction of the microbial load (by 87 ± 9%), assuming a rigorous change in the abundance and diversity of microbial colonizers. Bacteria-challenged polyps showed a mixture of native and AB-treated expression patterns (Figure 2). In more detail, the comparison of native and AB-treated polyps resulted in the highest number of differentially expressed genes (22,073 genes), with 10,451 upregulated and 11,622 down-regulated genes in AB-treated compared to native polyps (Supplementary Table S3A). According to the GO enrichment analysis (Conesa et al., 2005), the 10,451 upregulated genes were overrepresented in processes related to inflammation and immune response (e.g., acute inflammatory response to antigenic stimuli, MAP-kinase activity, cytokine production, and neutrophil cell activation) (Figure 3A; Supplementary Table S4A). Genes involved in immune response and apoptosis included, e.g., Caspases, Apoptosis regulators, Interferones, and Toll-like receptors (Figure 3B; Supplementary Tables S3A, S4A). On the contrary, down-regulated genes in AB-treated polyps were enriched for processes related to development, morphogenesis, reproduction, stimuli response, and signaling (Figures 3C,D; Supplementary Tables S3A, S4A).
Figure 2. Holistic expression patterns of A. aurita polyps. Heatmap including the Differential Expressed (DE) genes in all the pairwise comparisons of the native, AB-treated, and bacteria-challenged polyps, including replicates.
Figure 3. GO enrichment analysis of differentially expressed genes of native and AB-treated A. aurita polyps. (A,C) Barplots with GO-enriched gene categories when comparing native and Ab-treated polyps. Barplots indicate the proportion (%) of DE gene sequences involved in (A) upregulated and (C) down-regulated GO-enriched categories compared to the reference (de novo assembly). (B,D) Heatmap of upregulated and down-regulated genes with the comparison of native and AB-treated polyps related to (B) immune response and apoptosis (D) and common physiological processes. Relative expression level increase from blue to red.
When comparing expression patterns between native and bacteria-challenged polyps, differences based on the bacterial species used in each challenge were revealed (Figure 4). The comparison of native polyps compared to those challenged with V. anguillarum gave the highest number of differentially expressed (DE) genes (7,193 genes, Figure 4A; Supplementary Table S3B), followed by polyps challenged with K. oxytoca (6,974 DE genes, Figure 4A; Supplementary Table S3C), and P. espejiana (2,721 genes, Figures 4A; Supplementary Table S3D). We observed 2,210 genes were commonly upregulated among the treatments compared to native polyps (Figure 4A; Supplementary Table S3E). At the same time, 52 genes were jointly downregulated (Figure 4B; Supplementary Table S3E). Genes related to biological processes, such as defense, immune and inflammatory responses, and the regulation of apoptotic processes, were upregulated in bacteria-challenged polyps (Figure 4B; Supplementary Table S3E). In contrast, genes related to organism development, cell cycle processes, and cytoskeleton organization were down-regulated in challenged polyps (Figure 4B; Supplementary Table S3E). Moreover, exclusively upregulated and down-regulated genes were identified for each bacterial challenge (Figure 4). 1,319 genes were exclusively upregulated in V. anguillarum-challenged polyps (Figure 4A left panel; Supplementary Table S3F), 1,020 genes were exclusively upregulated in polyps challenged with K. oxytoca (Figure 4A left panel; Supplementary Table S3G), and 160 genes exclusively upregulated in P. espejiana-challenged polyps (Figure 4A left panel; Supplementary Table S3H). Similarly, exclusively down-regulated genes were identified (Figure 4A, right panel). Analyzing the GO-enriched categories for each species (Supplementary Table S4B–D), we found categories common among all treatments (Supplementary Table S4E) as well as categories present in two different treatments or only in one (Figure 4B; Supplementary Table S4B–D). Focusing on genes exclusively upregulated after each species-specific challenge, we indeed observed that the same GO categories, e.g., immune response, MAPK signal transduction, autophagy, and DNA repair, were enriched to different degrees, though represented by various genes (depicted in Figure 5).
Figure 4. GO enrichment and differential expression analysis of genes divergently transcribed in native compared to bacteria-challenged polyps. (A) Venn diagram indicating the genes that are commonly and exclusively upregulated (left panel) or down-regulated (right panel) in the different bacteria-challenged conditions. (B) Heatmap of up-and downregulated genes in native compared to bacteria-challenged polyps. Relative expression level increases from blue to red.
Figure 5. Exclusively upregulated genes in native vs. bacteria-challenged polyps. Bubble plot showing the degree of enrichment per category of exclusively upregulated genes in each bacteria-challenged compared to native conditions.
Recent studies revealed that interfering with Qurom sensing, so-called quorum quenching (QQ), might be a fundamental, additional inter-phylum interaction to maintain metaorganismal homeostasis. Consequently, we aimed to identify host-derived QQ activities. An expressed sequence tag (EST) library from A. aurita polyps-derived mRNA was constructed in E. coli SoluBL21 (Ladewig et al., 2023). The library consisted of 29,952 clones with an insertion efficiency of approx. 98% and an average insert size of 1.46 kbp, resulting in 43 Mbps cloned host transcriptome, corresponding to an estimated 11.4% coverage [calculated A. aurita genome size 376 Mbps (Gold et al., 2019)]. The A. aurita EST library was successively screened for QQ activities toward acyl-homoserine lactones (AHL) and autoinducer-2 (AI-2) in cell-free cell extracts and culture supernatants of the EST clones using established E. coli-based reporter systems (Weiland-Bräuer et al., 2015b). Overall, 37 out of 29,952 EST clones were identified as QQ active (Table 2). Predominantly, QQ activities against the Gram-negative signaling molecule AHL were detected. Although those host-derived QQ activities were identified in a functional screen, their biological activities in vivo and their functions in A. aurita have to be explored. In a first attempt, plasmid insertions of QQ-active single EST clones were sequenced, and sequence data subsequently checked for homologies in the A. aurita transcriptome assembly. All QQ-conferring sequences were identified in the transcriptome assembly with homologies ranging from 83 to 100% (Supplementary Table S5). Furthermore, public databases (NCBI, UniProt, PFAM) were used for homology predictions and annotation (Supplementary Table S5). Unexpectedly, several QQ-ORFs showed homologies to highly conserved ribosomal proteins, suggesting a moonlighting function of those proteins (Jeffery, 2003; Singh and Bhalla, 2020). Secondly, analyzing transcription levels of those identified QQ-ORFs in the generated RNAseq data set (see above) allowed first insights into their transcriptional regulation in response to the respective treatments, which might support predicting their biological role. Relative expression of the 37 QQ-conferring genes was calculated for microbiome-manipulated versus native polyps. Hierarchical clustering observed four clusters of expression profiles. The first cluster represents QQ genes similarly expressed in microbiome-manipulated polyps compared to native ones (Figure 6, right column highlighted in grey). The second cluster of genes showed decreased expression in all treatments, except when challenged with P. espejiana (Figure 6, right column light orange cluster). The third cluster summarizes those genes with increased expression regardless of the type of manipulation (Figure 6, right column dark orange cluster). The fourth cluster includes those genes with an increased expression after adding potential pathogens but reduced expression when polyps were AB-treated (Figure 6, right column green cluster). Notably, P. espejiana primarily showed increased expression of QQ-ORFs; thus, hierarchal clustering showed the highest dissimilarity compared to the other treatments.
Table 2. Aurelia aurita-derived quorum quenching activities derived from an expressed sequence tag (EST) library.
Figure 6. Differential expression of functionally identified A. aurita QQ-ORFs. Heatmap visualizes the expression patterns of 37 identified host-derived QQ-ORFs per condition as a mean of 2 to 3 replicates in combination with hierarchical clustering of conditions and expression profiles of ORFs.
In the present study, we obtained the first insights into the transcriptomic response of A. aurita to microbiome manipulation. The manipulations of the polyp microbiome included a massive reduction of viable bacterial cells (87% reduction) due to applying a broad-spectrum antibiotics mixture. The mixture contained Penicillin, Polymyxin B, Chloramphenicol, and Neomycin (Provasoli and Pintner, 1980; Liu et al., 2017a; KleinJan et al., 2022), and has been shown to only partially eliminate an entire bacterial community (Azma et al., 2010; Liu et al., 2017a). We assume that by drastically reducing the colony-forming units by 87%, the diversity and abundance of community members on the polyp are crucially changed. Consequently, the polyp was confronted with the loss of certain community members and a drastic change in the relative abundance of remaining ones. This extreme change in microbiome composition resulted in the up-regulation of various immune response mechanisms. Pattern recognition receptors (PRRs) like Toll-like receptors and Ficolins were upregulated, inducing the first line of defense (Figure 3). Further, Caspases were enriched in AB-treated polyps, potentially maintaining homeostasis through regulating cell death and inflammation (McIlwain et al., 2013). Contrarily, all processes involved in morphogenesis and development, thus not essential for survival, were down-regulated in AB-treated polyps (Figure 3).
Furthermore, the native microbiome was affected by challenging the polyps with three different bacteria in high cell numbers: (i) K. oxytoca has not been detected in any life stage of A. aurita and thus represents a non-native bacterium (Weiland-Bräuer et al., 2015a, 2019); (ii) V. anguillarum, an opportunistic pathogen of various invertebrates and vertebrates, has been isolated from an A. aurita polyp (Austin, 2010; Frans et al., 2011; Weiland-Bräuer et al., 2015a,b); and (iii) P. espejiana was initially isolated from seawater but was also found in high abundance associated with all life stages of A. aurita, thus representing a native bacterium (Isnansetyo and Kamei, 2009; Weiland-Bräuer et al., 2015a,b). Due to bacterial challenges, we observed the up-regulation of defense, immune, and inflammatory responses, and apoptosis regardless of the bacterial species. Based on this finding, we hypothesize that the host recognized the non-native and native bacteria as a potential threat, at least when present in such high cell numbers. The immune system of A. aurita likely responded with the four primary innate immune system functions as demonstrated for other Cnidarians and already partly observed for AB-treated polyps (Miller et al., 2007; Parisi et al., 2020). First, immune recognition occurred by PRRs, like Fucolectins, Ficolins, and NACHT-containing domain proteins, binding to bacterial MAMPs/PAMPs (Figures 4, 5). Subsequently, various transcription factors from the NF-κB family were activated (Figures 4, 5). Intracellular signaling cascades (MAPK, interleukin, cytokines) led to target gene transcription to eliminate the threat and mitigate self-harm (Figures 4, 5). Lastly, autophagy, DNA repair, and programmed cell death were upregulated after bacterial challenge (Figures 4, 5). Our observations are consistent with previous results showing that A. aurita’s general fitness and, in particular, its asexual reproduction were drastically affected in the absence of microbes and due to the manipulation of the microbiota by challenging with non-native colonizers, e.g., P. espejiana and V. anguillarum (Weiland-Bräuer et al., 2020a).
The acquisition, establishment, and maintenance of a specific microbiota are advantageous for the host, and its disturbance can contribute to developing diseases (Zheng et al., 2020). The host strives to maintain this homeostasis and presumably uses other defense mechanisms besides the innate immune system (Nichols and Davenport, 2021). The use of bacterial communication and its interference by the host has been recently regarded as an additional interaction mechanism within the complex interplay of the host and its microbiome (White et al., 2020; Weiland-Bräuer, 2021). Several studies evaluated the implication of highly conserved paraoxonases (PON1, PON2, and PON3) as QQ enzymes of the host defense against the pathogen Pseudomonas aeruginosa (Mochizuki et al., 1998; Draganov et al., 2005; Stoltz et al., 2007). In the present study, we functionally detected QQ activities of A. aurita in a cDNA expression library and verified the respective transcripts within the de novo assembled host transcriptome. Their native expression in response to microbiome manipulation resulted in four different expression profiles (Figure 6). A first cluster represents QQ-ORFs similarly low expressed in treated and native polyps. Those findings argue against a biological function in the defense against pathogens. The second cluster showed decreased expression in all treatments except when challenged with P. espejiana. Here, a specific defense against P. espejiana can be assumed. This assumption aligns with previous studies showing massive declined survival rates in the presence of this bacterium (Weiland-Bräuer et al., 2020a). The multitude of initiated defense mechanisms in the presence of the ubiquitous, native P. espejiana (various QQ-ORFs and acute inflammatory response, Figures 5, 6) might indicate that this bacterium represents a threat to A. aurita, at least in the high cell numbers, and must be rapidly defended. The third type of expression profile showed increased expression regardless of the type of microbiome manipulation, assuming a general defense. Among those is Ferritin, generally regarded as an intracellular iron storage protein and suggested to be involved in response to infection in different organisms, including fish and marine invertebrates (Moreira et al., 2020). The expression of Ferritin or Ferritin-homologs was upregulated in different tissues in response to bacterial infections or stimulation with LPS (Neves et al., 2009; He et al., 2013; Ren et al., 2014; Chen et al., 2016; Sun et al., 2016; Martínez et al., 2017; Liu et al., 2017b). Furthermore, Ferritin was shown to protect shrimp and fish from viral infections (Ye et al., 2015; Chen et al., 2018). The fourth cluster was expressed in the presence of the non-native and native bacteria, regarded as potential pathogens, but not after antibiotic treatment, assuming a fast response to potential pathogens’ colonization possessing similar PAMPs. Homologies to already described genes within the NCBI database of those QQ-ORFs to predict their function were rare. QQ-ORFs Aa_QQ_15 and Aa_QQ_30, belonging to the fourth cluster, showed non-significant homologies to a chitinase and acetyl-transferase, respectively. Here, enzymatic modification (hydrolysis or acetylation) of the bacterial signaling molecule can be speculated. The four other QQ-ORFs within this expression profile showed the best homologies with highly conserved proteins, including actin, heat shock, and ribosomal proteins. Particularly, ribosomal proteins crucially involved in translation have been shown to comprise other functions (Hurtado-Rios et al., 2022), thus recognized as moonlighting proteins. Moonlighting proteins are capable of performing more than one biochemical function within the same polypeptide chain, including inhibiting infectious bacteria, viruses, parasites, fungi, and tumor cells (Jeffery, 2003; Gao and Hardwidge, 2011; Wang et al., 2015; Singh and Bhalla, 2020). Thus, they have been considered antimicrobial peptides (AMPs) (Hurtado-Rios et al., 2022). Consequently, we hypothesize that the identified QQ activity, besides their canonical function, is promiscuous. Future studies have to be conducted to verify the biological function of the identified A. aurita QQ-ORFs, e.g., by genetic and biochemical approaches.
In conclusion, this study provides the first A. aurita transcriptome analysis focusing on the impact of microbiome disturbance on host gene expression. Overall it highlights the importance of the native microbiome since processes like morphogenesis, development, and response reactions are down-regulated when it is disturbed due to antibiotics. Microbiome disturbance further induced apoptosis and immune responses, indicating the microbiome’s protective function. Similarly, challenging A. aurita with high loads of bacteria resulted in the up-regulation of defense, immune, and inflammatory responses to maintain metaorganismal homeostasis. We have further received indications that quorum quenching is likely an additional mechanism for maintaining the specific microbiota besides the innate immune system, particularly acting as a fast response to potential pathogens.
The datasets presented in this study can be found in online repositories. The names of the repository/repositories and accession number(s) can be found below: PRJNA938117 (SRA), OQ581004-OQ581041 in the NCBI database.
RS and NW-B: conceptualization and supervision. DL: methodology. DL and NW-B: investigation. NW-B: formal analysis. VK: bioinformatics analysis and data curation. NW-B, VK, and RS: writing—original draft preparation, writing—review, and editing. RS: project administration and funding acquisition. All authors have read and agreed to the published version of the manuscript.
This work was conducted with the financial support of the DFG-funded Collaborative Research Center CRC1182 “Origin and Function of Metaorganisms” (B2) and the DFG-funded ImmuBase project. Project number 261376515 to the CRC1182 fund, and Project number 417981041 to the Immubase fund.
The authors would like to thank colleagues in the Department of Rheumatology and Clinical Immunology for their diligent work and participation in this study.
The authors declare that the research was conducted in the absence of any commercial or financial relationships that could be construed as a potential conflict of interest.
All claims expressed in this article are solely those of the authors and do not necessarily represent those of their affiliated organizations, or those of the publisher, the editors and the reviewers. Any product that may be evaluated in this article, or claim that may be made by its manufacturer, is not guaranteed or endorsed by the publisher.
The Supplementary material for this article can be found online at: https://www.frontiersin.org/articles/10.3389/fmicb.2023.1183627/full#supplementary-material
Al-Asmakh, M., and Zadjali, F. (2015). Use of germ-free animal models in microbiota-related research. J. Microbiol. Biotechnol. 25, 1583–1588. doi: 10.4014/jmb.1501.01039
Augustin, R., Fraune, S., and Bosch, T. C. (2010). “How Hydra senses and destroys microbes” Semin. Immunol, 22, 54–58. doi: 10.1016/j.smim.2009.11.002
Austin, B. (2010). Vibrios as causal agents of zoonoses. Vet. Microbiol. 140, 310–317. doi: 10.1016/j.vetmic.2009.03.015
Azma, M., Mohamad, R., Rahim, R. A., and Ariff, A. B. (2010). Improved protocol for the preparation of axenic culture and adaptation to heterotrophic cultivation. Open Biotechnol. J. 4, 36–46. doi: 10.2174/1874070701004010036
Bäckhed, F., Fraser, C. M., Ringel, Y., Sanders, M. E., Sartor, R. B., Sherman, P. M., et al. (2012). Defining a healthy human gut microbiome: current concepts, future directions, and clinical applications. Cell Host Microbe 12, 611–622. doi: 10.1016/j.chom.2012.10.012
Ball, E. E., Hayward, D. C., Saint, R., and Miller, D. J. (2004). A simple plan—cnidarians and the origins of developmental mechanisms. Nat. Rev. Genet. 5, 567–577. doi: 10.1038/nrg1402
Bang, C., Dagan, T., Deines, P., Dubilier, N., Duschl, W. J., Fraune, S., et al. (2018). Metaorganisms in extreme environments: do microbes play a role in organismal adaptation? Zoology 127, 1–19. doi: 10.1016/j.zool.2018.02.004
Bolger, A. M., Lohse, M., and Usadel, B. (2014). Trimmomatic: a flexible trimmer for Illumina sequence data. Bioinformatics 30, 2114–2120. doi: 10.1093/bioinformatics/btu170
Bosch, T. C. (2013). Cnidarian-microbe interactions and the origin of innate immunity in metazoans. Annu. Rev. Microbiol. 67, 499–518. doi: 10.1146/annurev-micro-092412-155626
Bosch, T. C., and Zasloff, M. (2021). Antimicrobial peptides—or how our ancestors learned to control the microbiome. MBio 12, e01847–e01821. doi: 10.1128/mBio.01847-21
Brekhman, V., Malik, A., Haas, B., Sher, N., and Lotan, T. (2015). Transcriptome profiling of the dynamic life cycle of the scypohozoan jellyfish Aurelia aurita. BMC Genomics 16:74. doi: 10.1186/s12864-015-1320-z
Chen, X.-X., Li, Y.-Y., Chang, X.-J., Xie, X.-L., Liang, Y.-T., Wang, K.-J., et al. (2018). A CqFerritin protein inhibits white spot syndrome virus infection via regulating iron ions in red claw crayfish Cherax quadricarinatus. Dev. Comp. Immunol. 82, 104–112. doi: 10.1016/j.dci.2018.01.008
Chen, G., Zhang, C., Wang, Y., Guo, C., Sang, F., and Wang, C. (2016). Identification and characterization of a ferritin gene involved in the immune defense response of scallop Chlamys farreri. Fish Shellfish Immunol. 55, 1–9. doi: 10.1016/j.fsi.2016.04.128
Chilton, S. N., Enos, M. K., Burton, J. P., and Reid, G. (2015). The effects of diet and the microbiome on reproduction and longevity: a comparative review across 5 continents. J. Nutr. Food Sci. 5: 364. doi: 10.4172/2155-9600.1000364
Chu, H., and Mazmanian, S. K. (2013). Innate immune recognition of the microbiota promotes host-microbial symbiosis. Nat. Immunol. 14, 668–675. doi: 10.1038/ni.2635
Conesa, A., Götz, S., García-Gómez, J. M., Terol, J., Talón, M., and Robles, M. (2005). Blast2GO: a universal tool for annotation, visualization and analysis in functional genomics research. Bioinformatics 21, 3674–3676. doi: 10.1093/bioinformatics/bti610
Conesa, A., Madrigal, P., Tarazona, S., Gomez-Cabrero, D., Cervera, A., McPherson, A., et al. (2016). A survey of best practices for RNA-seq data analysis. Genome Biol. 17, 1–19. doi: 10.1186/s13059-016-0881-8
Dierking, K., and Pita, L. (2020). Receptors mediating host-microbiota communication in the metaorganism: the invertebrate perspective. Front. Immunol. 11:1251. doi: 10.3389/fimmu.2020.01251
Draganov, D. I., Teiber, J. F., Speelman, A., Osawa, Y., Sunahara, R., and La Du, B. N. (2005). Human paraoxonases (PON1, PON2, and PON3) are lactonases with overlapping and distinct substrate specificities. J. Lipid Res. 46, 1239–1247. doi: 10.1194/jlr.M400511-JLR200
Ezenwa, V. O., Gerardo, N. M., Inouye, D. W., Medina, M., and Xavier, J. B. (2012). Animal behavior and the microbiome. Science 338, 198–199. doi: 10.1126/science.1227412
Frans, I., Michiels, C. W., Bossier, P., Willems, K., Lievens, B., and Rediers, H. (2011). Vibrio anguillarum as a fish pathogen: virulence factors, diagnosis and prevention. J. Fish Dis. 34, 643–661. doi: 10.1111/j.1365-2761.2011.01279.x
Fu, Z. D., Selwyn, F. P., Cui, J. Y., and Klaassen, C. D. (2017). RNA-Seq profiling of intestinal expression of xenobiotic processing genes in germ-free mice. Drug Metab. Dispos. 45, 1225–1238. doi: 10.1124/dmd.117.077313
Gao, X., and Hardwidge, P. R. (2011). Ribosomal protein s3: a multifunctional target of attaching/effacing bacterial pathogens. Front. Microbiol. 2:137. doi: 10.3389/fmicb.2011.00137
Gold, D. A., Katsuki, T., Li, Y., Yan, X., Regulski, M., Ibberson, D., et al. (2019). The genome of the jellyfish Aurelia and the evolution of animal complexity. Nat. Ecol. Evol. 3, 96–104. doi: 10.1038/s41559-018-0719-8
Grabherr, M. G., Haas, B. J., Yassour, M., Levin, J. Z., Thompson, D. A., Amit, I., et al. (2011a). Full-length transcriptome assembly from RNA-Seq data without a reference genome. Nat. Biotechnol. 29, 644–652. doi: 10.1038/nbt.1883
Grabherr, M. G., Haas, B. J., Yassour, M., Levin, J. Z., Thompson, D. A., Amit, I., et al. (2011b). Trinity: reconstructing a full-length transcriptome without a genome from RNA-Seq data. Nat. Biotechnol. 29, 644–652. doi: 10.1038/nbt.1883
Grandclâment, C., Tannières, M., Morâra, S., Dessaux, Y., and Faure, D. D. (2016). Quorum quenching: role in nature and applied developments. FEMS Microbiol. Rev. 40, 86–116. doi: 10.1093/femsre/fuv038
He, S., Peng, K., Hong, Y., Wang, J., Sheng, J., and Gu, Q. (2013). Molecular properties and immune defense of two ferritin subunits from freshwater pearl mussel. Fish Shellfish Immunol. 34, 865–874. doi: 10.1016/j.fsi.2012.12.021
Hurtado-Rios, J. J., Carrasco-Navarro, U., Almanza-Pérez, J. C., and Ponce-Alquicira, E. (2022). Ribosomes: the new role of ribosomal proteins as natural antimicrobials. Int. J. Mol. Sci. 23:9123. doi: 10.3390/ijms23169123
Isnansetyo, A., and Kamei, Y. (2009). Bioactive substances produced by marine isolates of Pseudomonas. J. Ind. Microbiol. Biotechnol. 36, 1239–1248. doi: 10.1007/s10295-009-0611-2
Jacob, S., Parthuisot, N., Vallat, A., Ramon-Portugal, F., Helfenstein, F., and Heeb, P. (2015). Microbiome affects egg carotenoid investment, nestling development and adult oxidative costs of reproduction in great tits. Funct. Ecol. 29, 1048–1058. doi: 10.1111/1365-2435.12404
Jeffery, C. J. (2003). Moonlighting proteins: old proteins learning new tricks. Trends Genet. 19, 415–417. doi: 10.1016/S0168-9525(03)00167-7
Jensen, N., Weiland-Bräuer, N., Joel, S., Chibani, C. M., and Schmitz, R. A. (2023). The Life Cycle of Aurelia aurita Depends on the Presence of a Microbiome in Polyps Prior to Onset of Strobilation. Microbiology Spectrum, e00262–23.
Kiran, G. S., Hassan, S., Sajayan, A., and Selvin, J. (2017). “Quorum quenching compounds from natural sources” in Bioresources and bioprocess in biotechnology, eds. Sugathan, S., Pradeep, N., Abdulhameed, S. (Singapore: Springer).
KleinJan, H., Frioux, C., Califano, G., Aite, M., Fremy, E., Karimi, E., et al. (2022). Insights into the potential for mutualistic and harmful host–microbe interactions affecting brown alga freshwater acclimation. Mol. Ecol. doi: 10.1111/mec.16766
Ladewig, L., Gloy, L., Langfeldt, D., Pinnow, N., Weiland-Bräuer, N., and Schmitz, R. A. (2023). Antimicrobial peptides originating from expression libraries of Aurelia aurita and Mnemiopsis leidyi prevent biofilm formation of opportunistic pathogens. bioRix. doi: 10.1101/2023.03.02.530746
Langmead, B., and Salzberg, S. L. (2012). Fast gapped-read alignment with bowtie 2. Nat. Methods 9, 357–359. doi: 10.1038/nmeth.1923
Li, B., and Dewey, C. N. (2011). RSEM: accurate transcript quantification from RNA-Seq data with or without a reference genome. BMC Bioinform. 12, 1–16. doi: 10.1186/1471-2105-12-323
Liu, J., Meng, Z., Liu, X., and Zhang, X.-H. (2019). Microbial assembly, interaction, functioning, activity and diversification: a review derived from community compositional data. Mar. Life Sci. Technol. 1, 112–128. doi: 10.1007/s42995-019-00004-3
Liu, C.-L., Place, A. R., and Jagus, R. (2017a). Use of antibiotics for maintenance of axenic cultures of Amphidinium carterae for the analysis of translation. Mar. Drugs 15:242. doi: 10.3390/md15080242
Liu, Q.-N., Xin, Z.-Z., Liu, Y., Wang, Z.-F., Chen, Y.-J., Zhang, D.-Z., et al. (2017b). A ferritin gene from Procambarus clarkii, molecular characterization and in response to heavy metal stress and lipopolysaccharide challenge. Fish Shellfish Immunol. 63, 297–303. doi: 10.1016/j.fsi.2017.02.025
Martínez, D., Oyarzún, R., Vargas-Lagos, C., Pontigo, J., Soto-Dávila, M., Saravia, J., et al. (2017). Identification, characterization and modulation of ferritin-H in the sub-Antarctic Notothenioid Eleginops maclovinus challenged with Piscirickettsia salmonis. Dev. Comp. Immunol. 73, 88–96. doi: 10.1016/j.dci.2017.03.015
McCarthy, D. J., Chen, Y., and Smyth, G. K. (2012). Differential expression analysis of multifactor RNA-Seq experiments with respect to biological variation. Nucleic Acids Res. 40, 4288–4297. doi: 10.1093/nar/gks042
McIlwain, D. R., Berger, T., and Mak, T. W. (2013). Caspase functions in cell death and disease. Cold Spring Harb. Perspect. Biol. 5:a008656. doi: 10.1101/cshperspect.a008656
Miller, D., Hemmrich, G., Ball, E., Hayward, D., Khalturin, K., Funayama, N., et al. (2007). The innate immune repertoire in Cnidaria - ancestral complexity and stochastic gene loss. Genome Biol. 8:R59. doi: 10.1186/gb-2007-8-4-r59
Mochizuki, H., Scherer, S. W., Xi, T., Nickle, D. C., Majer, M., Huizenga, J. J., et al. (1998). Human PON2 gene at 7q21.3: cloning, multiple mRNA forms, and missense polymorphisms in the coding sequence. Gene 213, 149–157. doi: 10.1016/s0378-1119(98)00193-0
Moran, N. A., and Yun, Y. (2015). Experimental replacement of an obligate insect symbiont. Proc. Natl. Acad. Sci. 112, 2093–2096. doi: 10.1073/pnas.1420037112
Moreira, A. C., Mesquita, G., and Gomes, M. S. (2020). Ferritin: an inflammatory player keeping iron at the core of pathogen-host interactions. Microorganisms 8:589. doi: 10.3390/microorganisms8040589
Mukherjee, S., and Bassler, B. L. (2019). Bacterial quorum sensing in complex and dynamically changing environments. Nat. Rev. Microbiol. 17, 371–382. doi: 10.1038/s41579-019-0186-5
Neves, J. V., Wilson, J. M., and Rodrigues, P. N. (2009). Transferrin and ferritin response to bacterial infection: the role of the liver and brain in fish. Dev. Comp. Immunol. 33, 848–857. doi: 10.1016/j.dci.2009.02.001
Nichols, R. G., and Davenport, E. R. (2021). The relationship between the gut microbiome and host gene expression: a review. Hum. Genet. 140, 747–760. doi: 10.1007/s00439-020-02237-0
Ochsenkühn, M. A., Röthig, T., D’Angelo, C., Wiedenmann, J., and Voolstra, C. R. (2017). The role of floridoside in osmoadaptation of coral-associated algal endosymbionts to high-salinity conditions. Sci. Adv. 3:e1602047. doi: 10.1126/sciadv.1602047
Parisi, M. G., Parrinello, D., Stabili, L., and Cammarata, M. (2020). Cnidarian immunity and the repertoire of defense mechanisms in anthozoans. Biology 9:283. doi: 10.3390/biology9090283
Park, E., Hwang, D.-S., Lee, J.-S., Song, J.-I., Seo, T.-K., and Won, Y.-J. (2012). Estimation of divergence times in cnidarian evolution based on mitochondrial protein-coding genes and the fossil record. Mol. Phylogenet. Evol. 62, 329–345. doi: 10.1016/j.ympev.2011.10.008
Pierre, J.F. (2022). Metabolism of Nutrients by Gut Microbiota, The Royal Society of Chemistry, 1–17.
Pietschke, C., Treitz, C., Foret, S., Schultze, A., Kunzel, S., Tholey, A., et al. (2017). Host modification of a bacterial quorum-sensing signal induces a phenotypic switch in bacterial symbionts. Proc. Natl. Acad. Sci. 114, E8488–E8497. doi: 10.1073/pnas.1706879114
Provasoli, L., and Pintner, I. J. (1980). Bacteria induced polymorphism in an axenic laboratory strain of Ulva lactuca (Chlorophyceae) 1. J. Phycol. 16, 196–201. doi: 10.1111/j.1529-8817.1980.tb03019.x
Putnam, N. H., Srivastava, M., Hellsten, U., Dirks, B., Chapman, J., Salamov, A., et al. (2007). Sea anemone genome reveals ancestral eumetazoan gene repertoire and genomic organization. Science 317, 86–94. doi: 10.1126/science.1139158
Ren, C., Chen, T., Jiang, X., Wang, Y., and Hu, C. (2014). Identification and functional characterization of a novel ferritin subunit from the tropical sea cucumber. Fish Shellfish Immunol. 38, 265–274. doi: 10.1016/j.fsi.2014.03.022
Richardson, A. J., Bakun, A., Hays, G. C., and Gibbons, M. J. (2009). The jellyfish joyride: causes, consequences and management responses to a more gelatinous future. Trends Ecol. Evol. 24, 312–322. doi: 10.1016/j.tree.2009.01.010
Robinson, M. D., McCarthy, D. J., and Smyth, G. K. (2010). edgeR: a Bioconductor package for differential expression analysis of digital gene expression data. Bioinformatics 26, 139–140. doi: 10.1093/bioinformatics/btp616
Rook, G., Bäckhed, F., Levin, B. R., McFall-Ngai, M. J., and McLean, A. R. (2017). Evolution, human-microbe interactions, and life history plasticity. Lancet 390, 521–530. doi: 10.1016/S0140-6736(17)30566-4
Rosenstiel, P., Philipp, E. E. R., Schreiber, S., and Bosch, T. C. G. (2009). Evolution and function of innate immune receptors – insights from marine invertebrates. J. Innate Immun. 1, 291–300. doi: 10.1159/000211193
Simão, F. A., Waterhouse, R. M., Ioannidis, P., Kriventseva, E. V., and Zdobnov, E. M. (2015). BUSCO: assessing genome assembly and annotation completeness with single-copy orthologs. Bioinformatics 31, 3210–3212. doi: 10.1093/bioinformatics/btv351
Singh, N., and Bhalla, N. (2020). Moonlighting proteins. Annu. Rev. Genet. 54, 265–285. doi: 10.1146/annurev-genet-030620-102906
Sommer, F., and Bäckhed, F. (2013). The gut microbiota—masters of host development and physiology. Nat. Rev. Microbiol. 11, 227–238. doi: 10.1038/nrmicro2974
Stoltz, D. A., Ozer, E. A., Ng, C. J., Yu, J. M., Reddy, S. T., Lusis, A. J., et al. (2007). Paraoxonase-2 deficiency enhances Pseudomonas aeruginosa quorum sensing in murine tracheal epithelia. Am. J. Phys. Lung Cell. Mol. Phys. 292, L852–L860. doi: 10.1152/ajplung.00370.2006
Sun, S., Zhu, J., Ge, X., and Zhang, W. (2016). Molecular characterization and gene expression of ferritin in blunt snout bream (Megalobrama amblycephala). Fish Shellfish Immunol. 57, 87–95. doi: 10.1016/j.fsi.2016.08.029
Wang, W., Nag, S., Zhang, X., Wang, M. H., Wang, H., Zhou, J., et al. (2015). Ribosomal proteins and human diseases: pathogenesis, molecular mechanisms, and therapeutic implications. Med. Res. Rev. 35, 225–285. doi: 10.1002/med.21327
Webster, N. S., and Reusch, T. B. (2017). Microbial contributions to the persistence of coral reefs. ISME J. 11, 2167–2174. doi: 10.1038/ismej.2017.66
Weiland-Bräuer, N. (2021). Friends or foes—microbial interactions in nature. Biology 10:496. doi: 10.3390/biology10060496
Weiland-Bräuer, N., Fischer, M. A., Pinnow, N., and Schmitz, R. A. (2019). Potential role of host-derived quorum quenching in modulating bacterial colonization in the moon jellyfish Aurelia aurita. Sci. Rep. 9:34. doi: 10.1038/s41598-018-37321-z
Weiland-Bräuer, N., Neulinger, S. C., Pinnow, N., Kunzel, S., Baines, J. F., and Schmitz, R. A. (2015a). Composition of bacterial communities associated with Aurelia aurita changes with compartment, life stage, and population. Appl. Environ. Microbiol. 81, 6038–6052. doi: 10.1128/AEM.01601-15
Weiland-Bräuer, N., Pinnow, N., Langfeldt, D., Roik, A., Güllert, S., Chibani, C. M., et al. (2020a). The native microbiome is crucial for offspring generation and fitness of Aurelia aurita. MBio 11, e02336–e02320. doi: 10.1128/mBio.02336-20
Weiland-Bräuer, N., Pinnow, N., and Schmitz, R. A. (2015b). Novel reporter for identification of interference with acyl homoserine lactone and autoinducer-2 quorum sensing. Appl. Environ. Microbiol. 81, 1477–1489. doi: 10.1128/AEM.03290-14
Weiland-Bräuer, N., Prasse, D., Brauer, A., Jaspers, C., Reusch, T. B. H., and Schmitz, R. A. (2020b). Cultivable microbiota associated with Aurelia aurita and Mnemiopsis leidyi. MicrobiologyOpen 9:e1094. doi: 10.1002/mbo3.1094
White, J. R., Dauros-Singorenko, P., Hong, J., Vanholsbeeck, F., Phillips, A., and Swift, S. (2020). The role of host molecules in communication with the resident and pathogenic microbiota: a review. Med. Microecol. 4:100005. doi: 10.1016/j.medmic.2020.100005
Woznica, A., Gerdt, J. P., Hulett, R. E., Clardy, J., and King, N. (2017). Mating in the closest living relatives of animals is induced by a bacterial chondroitinase. Cells 170, 1175–1183.e11. e1111. doi: 10.1016/j.cell.2017.08.005
Ye, T., Wu, X., Wu, W., Dai, C., and Yuan, J. (2015). Ferritin protect shrimp Litopenaeus vannamei from WSSV infection by inhibiting virus replication. Fish Shellfish Immunol. 42, 138–143. doi: 10.1016/j.fsi.2014.10.039
Zheng, D., Liwinski, T., and Elinav, E. (2020). Interaction between microbiota and immunity in health and disease. Cell Res. 30, 492–506. doi: 10.1038/s41422-020-0332-7
Zheng, L., Zhang, L., Lin, H., McIntosh, M., and Malacrida, A. (2005). Toll-like receptors in invertebrate innate immunity. Invertebr. Surviv. J. 2, 105–113.
Keywords: Aurelia aurita , microbiome, transcriptome, immune system, defense mechanisms, quorum quenching
Citation: Weiland-Bräuer N, Koutsouveli V, Langfeldt D and Schmitz RA (2023) First insights into the Aurelia aurita transcriptome response upon manipulation of its microbiome. Front. Microbiol. 14:1183627. doi: 10.3389/fmicb.2023.1183627
Received: 10 March 2023; Accepted: 18 July 2023;
Published: 10 August 2023.
Edited by:
Alejandra Prieto-Davó, National Autonomous University of Mexico, MexicoReviewed by:
Ming Guo, Ningbo University, ChinaCopyright © 2023 Weiland-Bräuer, Koutsouveli, Langfeldt and Schmitz. This is an open-access article distributed under the terms of the Creative Commons Attribution License (CC BY). The use, distribution or reproduction in other forums is permitted, provided the original author(s) and the copyright owner(s) are credited and that the original publication in this journal is cited, in accordance with accepted academic practice. No use, distribution or reproduction is permitted which does not comply with these terms.
*Correspondence: Ruth A. Schmitz, cnNjaG1pdHpAaWZhbS51bmkta2llbC5kZQ==
†Present address: Daniela Langfeldt, Institute of Clinical Molecular Biology (IKMB), Kiel University, Kiel, Germany
‡These authors have contributed equally to this work and share first authorship
Disclaimer: All claims expressed in this article are solely those of the authors and do not necessarily represent those of their affiliated organizations, or those of the publisher, the editors and the reviewers. Any product that may be evaluated in this article or claim that may be made by its manufacturer is not guaranteed or endorsed by the publisher.
Research integrity at Frontiers
Learn more about the work of our research integrity team to safeguard the quality of each article we publish.