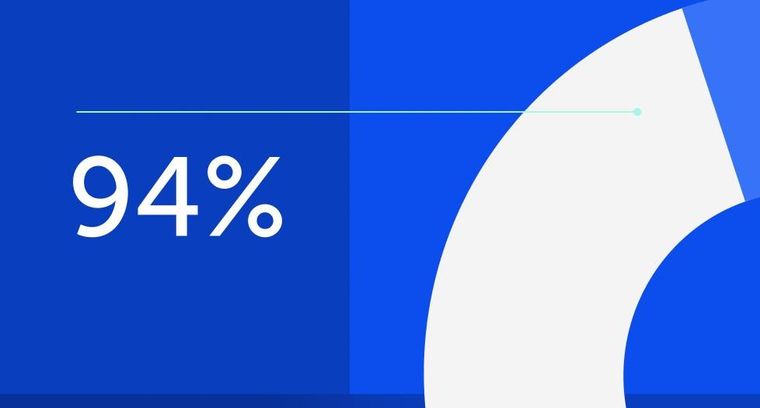
94% of researchers rate our articles as excellent or good
Learn more about the work of our research integrity team to safeguard the quality of each article we publish.
Find out more
PERSPECTIVE article
Front. Microbiol., 30 May 2023
Sec. Microbiotechnology
Volume 14 - 2023 | https://doi.org/10.3389/fmicb.2023.1166612
This article is part of the Research TopicInsights in Microbiotechnology: 2022View all 6 articles
Cyanobacteria are widespread phototrophic microorganisms that represent a promising biotechnological tool to satisfy current sustainability and circularity requirements. They are potential bio-factories of a wide range of compounds that can be exploited in several fields including bioremediation and nanotechnology sectors. This article aims to illustrate the most recent trends in the use of cyanobacteria for the bioremoval (i.e., cyanoremediation) of heavy metals and metal recovery and reuse. Heavy metal biosorption by cyanobacteria can be combined with the consecutive valorization of the obtained metal-organic materials to get added-value compounds, including metal nanoparticles, opening the field of phyconanotechnology. It is thus possible that the use of combined approaches could increase the environmental and economic feasibility of cyanobacteria-based processes, promoting the transition toward a circular economy.
In recent years environmental pollution has become one of the main concerns troubling societies due to its detrimental effect on human health, ecosystems, and the ways it can affect ecological balance and resource availability. Human activity is incessantly contributing to increasing concentrations of different polluting compounds in the environment. Within organic and inorganic pollutants, heavy metals, including cadmium, chromium, copper, lead, mercury, nickel, selenium, and zinc, are commonly found in all ecosystems. Despite some metals being essential for living organisms, all metals become toxic at high concentrations (US EPA, 2015). Their non-biodegradable nature causes their accumulation in the environment as well as their magnification through the food chain with a mutagenic and/or carcinogenic risk for humans.
Metal-based industries, such as those that involve mining and electroplating activity, produce effluents with high heavy metal content (Briffa et al., 2020), which become the main cause of redistribution and concentration of the metals in water, soil, and air ecosystems and generating a danger to aquatic life, water availability for rural and urban areas, and human beings. Thus, stringent regulations, efficient technologies, and long-term sustainable strategies are essential for reducing the accumulation of heavy metals in the environment. In addition, the possibility of recovering and reusing these compounds may represent an economic opportunity for the industry and could also sustain the development of “end of waste” processes through the implementation of a circular economy approach.
Cyanobacteria are cosmopolitan photoautotrophic bacteria that represent the largest and widest group of microorganisms. Their metabolic diversity represents a rich source of biotechnological instruments for sustainable development (Mona et al., 2020; Priyanka et al., 2020). Their ability to survive in extreme conditions, comprising environments containing pesticides, petroleum by-products, radioactive compounds, crude oils, xenobiotics, and heavy metals, has drawn increasing interest from the scientific community, shedding light on the cellular mechanisms involved as well as their possible exploitation as a clean green technology for degradation or detoxification of contaminants. Many studies have been carried out for soil and water bioremediation adopting cyanobacteria. Such a process is also named cyanoremediation (Mona et al., 2020; Rueda et al., 2020; Dutta et al., 2022; Zanganeh et al., 2022). The possibility of cultivating cyanobacteria on a large scale in large ponds, coupled with the ability to fix carbon dioxide as well as atmospheric nitrogen (for some genera), makes them self-sufficient in terms of adaptability, growth, and maintenance in controlled or contaminated environments (Gehlot et al., 2022). Furthermore, the use of cyanobacteria for bioremediation is enhanced also by their ability to tolerate environmental fluctuations (Gehlot et al., 2022). Additionally, the cyanobacterial biomass generated through this process can be exploited as a feedstock for the production of a wide range of biobased products with several applications (Encarnação et al., 2023). However, the valorization of cyanobacterial biomass obtained after heavy metal bioremediation is still poorly investigated (Blanco-Vieites et al., 2023; Encarnação et al., 2023; Thevarajah et al., 2023).
In recent years the field of nanotechnology has shown increasing scientific and economic interest in the possible application of cyanobacterial biomass (Selmani et al., 2022). Nanotechnology-based on phototrophic microorganisms is called phyconanotechnology (Chan et al., 2022; Pandey et al., 2022b), and represents an opportunity for cyanobacterial biomass valorization. Nevertheless, research studies in cyanoremediation and phyconanotechnology are still unlinked.
Considering that cyanobacteria may represent a useful biotechnological tool to promote societal transitions toward a circular economy, through waste recovery and valorization, this article aims to illustrate recent trends and future directions in cyanobacteria-based heavy metal bioremediation and recovery of the metals and/or the valorization of the biomasses with a particular focus on phyconanotechnology. The combination of both processes will be suggested within a circular concept to ensure higher economic and environmental feasibility.
Cyanobacteria exploit a variety of mechanisms to sequester and minimize the effect of heavy metals in contaminated environments, such as biosorption, bioaccumulation, and biotransformation. Metal-binding metallothionein (MT) proteins and phytochelatins (PCs), enzymatic and non-enzymatic antioxidants, and enzymes reducing heavy metals to less harmful forms play a pivotal role in cyanobacteria defense against heavy metals (Chakdar et al., 2022). A key role has been attributed to cyanobacteria exopolysaccharides (EPS), which are heteropolymers characterized by unique properties compared to other bacteria, including strong anionic nature due to the presence of one or two uronic acids and sulfate-containing sugars, and the presence of six or more different types of monosaccharides. The role of EPS in metal sequestration is mainly due to the presence of negatively charged groups, such as sulfate, phosphate, carboxyl, and hydroxyl, that may work as chelating agents for positively charged heavy metals (De Philippis and Micheletti, 2017; Cui et al., 2021; Potnis et al., 2021; Bhatt et al., 2022) and it has been widely demonstrated through molecular and biophysical techniques: mutagenesis, X-ray spectroscopy, Fourier transformed infrared spectroscopy (FTIR), and Scanning or Transmission Electron Microscopy (SEM–TEM) (Potnis et al., 2021).
Heavy metal remediation by cyanobacteria can be carried out through two main processes: bioaccumulation and biosorption (Bloch and Ghosh, 2022). The former is a metabolically driven active process that requires living cells, whereas the latter is a passive process that can be performed by both dead or living cells (Pandey et al., 2022a).
Biosorption, which is considered the major mechanism for the removal of heavy metals from wastewater, involves several mechanisms, such as ion exchange, adsorption, surface complexation, precipitation, and chelation (Bhatt et al., 2022). Since the cell wall of cyanobacteria is generally rich in negatively charged groups, which represent potential binding sites for heavy metals (De Philippis and Micheletti, 2017; Mota et al., 2022), in the first stage, metal ions can be rapidly sorbed to the surface of the cells. Consequently, metal ions can be translocated inside the cells through active transporters and carriers which are converted into less toxic forms and/or stored in vacuoles.
Several cyanobacteria genera, such as Anabaena, Cyanobium, Nostoc, Cyanothece, Arthrospira, Microcystis, Synechocystis, and Leptolyngbya, have shown promising results on Cu, Cd, Zn, Cr, Pb, Ni, Co or Hg removal with initial concentration ranging from some mg/L to 150–200 mg/L (Mota et al., 2016; Zinicovscaia et al., 2018; Yadav et al., 2021; Bloch and Ghosh, 2022; Pandey et al., 2022a). Maximum uptake is typically in the range of 15–80 mg/g dry weight, but some works have presented values even higher than 300 mg/g dry weight (Cui et al., 2021). Also, the use of consortia of different cyanobacteria species or microalgae/other microbes and cyanobacteria may help to attain higher metal tolerance as well as higher metabolite synthesis, positively contributing to metal removal (Cui et al., 2021). Nevertheless, the stability of the consortia should be monitored to ensure constant bioactivity. Generally, since the removal efficiency is maximized with a lower initial metal concentration, biosorption or bioaccumulation by cyanobacteria can be adopted after conventional methods that are characterized by low efficiency at a low heavy metal concentration (Agarwal et al., 2020).
Biosorption is considered a more feasible approach for heavy metal removal from wastewater compared to bioaccumulation, as it is characterized by faster kinetics, and the cells are not affected by heavy metal concentration which may be toxic at a high value. Nevertheless, several parameters are known to influence the biosorption process, such as pH, temperature, biosorbent dosage, and pretreatment, which require attention for improving the adsorption ability (Al-Amin et al., 2021). Another advantage of biosorption is represented by the possibility to exploit the cells for several desorption/adsorption cycles increasing their shelf-life and thus their economic value. During desorption, several solutions may be adopted, including strong acid or base, EDTA, or water, depending on the strength of the binding between metal ions and binding groups as well as the mechanical and physical strength of the biosorbents (Chatterjee and Abraham, 2019; Agarwal et al., 2020; Satya et al., 2021). Once eluted, the metal ions can be recovered to enter again inside the productive cycle of the industries, while the metal-free biomass can be used in a further adsorption cycle. When the adsorption capacity of the biomass is exhausted, the cells can be harvested for the last heavy metal recovery or their valorization.
It is worth mentioning cyanobacterial biomass management options. If cyanobacteria can be directly grown in open ponds or closed photobioreactors containing heavy metals contaminated wastewater exploiting active removal processes, other approaches should be implemented for biosorption since it doesn’t require metabolically active cells. The biomass obtained after their cultivation can be confined in closed systems with low porosity, such as dialysis membrane devices, or immobilized in polymeric matrices or filter-columns or filter-press (De Philippis et al., 2011; Ramírez Calderón et al., 2020). These systems are also advantageous to carry out adsorption/desorption cycles and/or for the recovery of metal-contaminated biomass that can be disposed for the following valorization. Since the materials remain confined or entrapped for the entire duration of the process, no risks due to biomass or metal contamination exist. Batch systems, which consist of metal-containing solutions and biosorbents, are commonly used in lab trials due to their simplicity. Nevertheless, the use of continuous reactors (e.g., fixed-bed column with continuous liquid flow) is favored at the industrial scale (Ramírez Calderón et al., 2020). Immobilization of cyanobacteria as biofilms has been recently developed as a feasible cultivation strategy to reduce water use and simplify the harvesting process (Cui et al., 2021). Immobilized cells or EPS onto a suitable carrier can be also used for the metal biosorption process, due to the increase in mechanical strength and chemical resistance of the biosorbents. Thus, multiple adsorption/desorption cycles can be easily carried out, and the exhausted biosorbents can be harvested at the end of the process for their valorization. In this context, adsorption, covalent bonds in vector compounds such as silica gel, entrapment/encapsulation in polymeric matrices, and cross-linking, can be exploited for immobilization. Nevertheless, the diffusion rate of metal ions into the polymeric matrices should be carefully checked (Velkova et al., 2018; Ajao et al., 2020; Cui et al., 2021). Nostoc muscorum immobilized on a glass surface through the formation of biofilm has been used for Cd removal from water solutions. The cultures exhibited higher cell resistance compared to the cell suspension and higher Cd tolerance (Raghavan et al., 2020). Velu et al. (2020) cultivated Tolypothrix sp. in outdoor cultures in simulated ash dam wastewater adopting 500 L vertical bag photobioreactor and as biofilms in algal-turf scrubbers. They found similar metal removal efficiency between the two cultivation systems.
Despite cyanobacteria biosorption has been widely recognized as an effective, fast, low-cost, and eco-friendly treatment method due to the large surface-to-volume ratio, the strong anionic character of EPS, and the possibility to regenerate, reuse, and easily recover the biosorbents (De Philippis and Micheletti, 2017; Singh, 2020; Priya et al., 2022), there are still economic and technical concerns that need to be managed for the process optimization, such as the economic cost and environmental footprint of biomass production, and metal recovery and reuse, that will be further faced in the following sections.
During the last decades, the necessity to minimize resource overexploitation and maximize waste prevention while generating economic gains has developed the circular economy concept (Velenturf and Purnell, 2021). This topic has been addressed by many research areas, including phycology (i.e., the study of algae). Many recent works investigated the cultivation of microalgae and cyanobacteria in conjunction with nutrient recycling from agro-industrial wastewater for biomass production and valorization (Abinandan et al., 2018; Gorain et al., 2019; Bhatt et al., 2022). However, research studies on heavy metal removal coupled with biomass application are still missing.
Cyanobacteria are commonly considered a potential source of bio-control agents, bio-fertilizers, soil amendments, food supplements, biofuels, high-value products, and biopolymers (Gomes Gradíssimo et al., 2020; Mona et al., 2020; Kholssi et al., 2021). Thus, the cyanobacteria biomass obtained after biosorption and/or bioaccumulation of heavy metal can be harvested and potentially converted into various economically significant by-products. Additionally, heavy metals can be recovered through desorption from metal-enriched biomasses or can be immobilized on biomass-derived carbons as metal-loading materials (Chai et al., 2022).
For instance, Serrà et al. (2020) proposed a circular zero-residue process adopting living cells of Arthrospira platensis for heavy metal bioremediation and the generated biomass for the production of bioethanol, biogas, and Fenton-like catalysts adopted for the degradation of persistent organic pollutants. Besides, the remaining low-activity ashes were used for the preparation of an ash-based medium for microalgae cultivation.
A further potentially interesting application that may be coupled with heavy metal removal is suggested by a recent work that investigated the use of hydrogels composed of sulphated polysaccharides from red microalgae and enriched with Zn as antimicrobial wound-dressing materials (Netanel Liberman et al., 2021).
The valorization of metallic-organic materials obtained through metal biosorption in nanotechnology may represent a significant opportunity to increase the economic value of cyanobacteria. Nanotechnology is an emerging field concerning the synthesis, characterization, and application of nanomaterials (1–100 nm size) characterized by high surface area-to-volume ratio enhancing their physico-chemical properties (Hamida et al., 2020). The global nanotechnology market was valued at USD 9.39 billion in 2021 and is expected to register a CAGR of 14.9% by 2030 with increased application in electronics, followed by the medical industry (Market Analysis Report, 2021).
Among nanotechnologies, metal nanoparticles (NPs) are applicable in several fields, including diagnostic, biosensing, imaging, antimicrobials, catalysis, electronics, optics, biofuel cells, anticancer, and drug delivery (Hamida et al., 2020). Nevertheless, conventional methods for NP synthesis are often expensive and produce toxic by-products. To avoid these drawbacks, eco-compatible systems for the production of NPs are challenging: intra- and extracellular green synthesis of NPs adopting biological systems, including algae and cyanobacteria are receiving increasing attention (Ijaz et al., 2022; Mandhata et al., 2022; Barciela et al., 2023).
Microbes, and in particular prokaryotic organisms, have been demonstrated to be effective nano-factories, thanks to their ability to accumulate and detoxify heavy metals and to the presence of a wide range of reductase enzymes, microbial cells are able to immobilize and reduce heavy metals, through a dose-dependent process (Mandhata et al., 2022). Metal NPs synthesis can be carried out through a metabolism-dependent or independent process, where proteins and polysaccharides work as reducing, stabilizing, and capping agents (Mandhata et al., 2022). Generally, metal ions are entrapped into the cell surface thanks to the presence of negatively charged groups in the biosorption process, where they are reduced by enzymes, proteins, lipids, and pigments. Metal ions can also enter into the cell through internal absorption, to be reduced intracellularly by enzymes (nitrate reductase, nitrogenase) and then stabilized.
The term phyconanotechnology is referred to nanotechnology based on biobased material produced or constituted by photosynthetic microorganisms (Chan et al., 2022; Pandey et al., 2022b). For example, polysaccharides from brown algae (e.g., alginate, fucoidan, and laminaran) can be used as reducing and stabilizing agents for eco-friendly synthesis of silver NPs with cytotoxicity and antibacterial activity (Yugay et al., 2020). Recent studies have been carried out to obtain Cu, Zn, Cd, Ti, Au, and Ag NPs of different sizes (5–266 nm) and shapes (mainly spherical) adopting cyanobacteria EPS, cells, or cellular extracts as summarized in Table 1. These metal NPs have been tested for their application in medical, antimicrobial, and bioremediation fields (Saran et al., 2017; Ebadi et al., 2019; Ismail et al., 2021; Hanna et al., 2022; Mandhata et al., 2022; Pandey et al., 2022b). Most studies are based on AuNPs and AgNPs biosynthesis (Table 1) due to their non-toxicity, bio-compatibility, and for their high-potential therapeutic applications (Aziz et al., 2021; Mandhata et al., 2022). Although therapeutic and anti-microbial applications are commonly studied, the use of bio-based metal NPs in the industrial sector needs deeper investigation.
For instance, the development of functional textiles adopting metal NPs has triggered the interest of the industrial sector in recent years. The incorporation of metal NPs provides textiles with antimicrobial, ultraviolet-resistance, self-cleaning capabilities, and flame-retardant properties. Polysaccharides can reduce and stabilize metal NPs and promote their adhesion to fabrics. In addition, polysaccharides can improve the properties of textiles due to their physico-chemical characteristics (Fernandes et al., 2022). In this frame, cyanobacterial EPS, with metal chelating properties and a high amount that can be synthesized, could constitute an interesting tool for NPs stabilization and functional textiles improvement.
Additionally, metal NPs offer numerous benefits as green catalysts due to their high reactivity, selectivity, low cost, and easy preparation, and the fact that they can be widely applied for the production of pharmaceuticals and some commodity chemicals (Sheldon and Woodley, 2018). For instance, simple Pd complexes can be heterogenized into red algae-derived polysaccharide supports to improve conversion rates in Suzuki cross-coupling reaction (Wolfson et al., 2018), while CuNPs can be stabilized in chitosan-based hydrogel and used as a catalyst for the synthesis of 1,2,3-triazoles (Souza et al., 2019). Sulfated polysaccharides of algal origin have been also used as bio-matrix and capping agent for BaFe12O19 NPs. These heterogeneous materials were characterized by high catalytic activity in the one-pot synthesis of 2-amino-4H-pyrans and pyrans annulated heterocyclic compounds, effective reusability, and antibacterial activity (Amirnejat et al., 2022). The differences in the catalytic effectiveness between commercial polysaccharides and extracted microbial exopolysaccharides are probably ascribable to their different composition, for example to the presence of peculiar monomers in the polysaccharidic backbone of microbial EPS (Sutherland, 1990).
According to Sheldon and Woodley (2018), new developments in the biobased economy will further enhance broader applications of biocatalysis. The study of microbial cells or soluble chelating EPS for obtaining metal-bearing biocatalysts can be a new opportunity for developing innovative and eco-sustainable high value industrial products even in a circular economy concept.
For example, microbial biomass and EPS can be recovered after metal biosorption and used as biocatalysts for many organic transformations. In this context, Gandolfi et al. (2022) successfully valorized the use of two EPS-producing bacterial strains, using their biomass after Cu biosorption as hybrid catalysts in the asymmetric boron addition on α,β-unsaturated chalcones for the synthesis of valuable pharmaceutical intermediates.
Therefore, as a perspective, since many cyanobacteria species are excellent EPS producers in terms of quality and quantity (Cruz et al., 2020; Morais et al., 2022), and their monosaccharidic composition is highly heterogeneous, their application in the field of green catalysis may represent a huge opportunity.
Despite ongoing research in phyconanotechnology, the field is still at the beginning, this area of study is considered an option for increasing the market value and potential applications of EPS (Morais et al., 2022). Additionally, coupling heavy metal removal with metal NPs production may promote the development of a circular system to get high-added value products from waste as suggested in Figure 1. Nevertheless, new studies based on the elucidation of the mechanisms for biosynthesis of NPs and screening of different strains are needed to develop standardized protocols.
Figure 1. Schematic representation of heavy metal biosorption adopting cyanobacteria coupled with metal recovery and phyconanotechnology through a circular process. Created with BioRender.com.
Cyanobacteria advanced cultivation systems for higher target molecules and biomass productivity or improved downstream processing may be adopted to enhance the bioremoval process. Molecular engineering strategies targeting the ability, specificity, and robustness of cyanobacteria strains can also improve their properties. Even if general risks for cyanobacteria-based remediation are not illustrated in the literature, biosafety issues and contamination risk need to be considered for engineered cyanobacteria (Cui et al., 2021).
Another target to achieve is the reduction of the costs associated with biomass cultivation while increasing the environmental benefits of the process (Wan Mahari et al., 2022). Cyanobacteria can be cultivated by replacing conventional fertilizers with nutrient-rich wastewater (Figure 1), leading to a double outcome: the recovery of carbon, nitrogen, phosphorus, and other nutrients through their assimilation, together with cyanobacteria biomass production (Sachdeva et al., 2018; Gomes Gradíssimo et al., 2020; Kholssi et al., 2021; Prabha et al., 2022). Thus, the costs associated with artificial salts and water requirements are reduced together with the environmental footprint of the process (Sachdeva et al., 2018). The produced biomass can be harvested and pre-treated to be repeatedly used as biosorbent in multiple adsorption/desorption cycles for the continuous recovery of metals from wastewater. Moreover, when used at the end of the cycle, the obtained metal-organic materials can be valorized, exploiting the biochemical properties of cyanobacteria to achieve high-value products through phyconanotechnologies (Figure 1). In this context, the effect of biomass-specific properties, or metal type and quantity on the performance of the obtained materials needs to be explored together with their physical-chemical characterization. This approach may help to reach circularity and sustainability requirements, maximizing the valorization of wastes.
To date, a limited number of works have evaluated metal biosorption from industrial wastewater, despite their composition and pH being known to strongly influence the heavy metal removal process, including the selectivity of the biosorbent toward metals (Zinicovscaia et al., 2019; Li et al., 2022). Specific studies with metal-rich wastewater are needed to implement this approach at pilot and industrial scales. For instance, the recovery of water and metals from electroplating effluents is particularly challenging due to the high costs associated with the metal-coating process and the treatment of the generated effluents (Li et al., 2022). Thus, the cyanobacteria-based approach suggested in this article may be implemented to reduce metal concentration from these effluents, while obtaining a metal-rich organic material.
The environmental and economic benefits as well as the potential risks of converting and recycling heavy metal-contaminated biomass into value-added materials should be carefully evaluated. The valorization of these materials (cells or EPS) in different application fields, including nanotechnology, encompasses several safety concerns, which hinder their applicability. The increasing worldwide production of NPs and their low size may lead to their release into the atmosphere, aquatic, and terrestrial environments, through aggregation or biological transportation, reaching long distances. Due to their small size, NPs can easily cross biological barriers reaching any organ in living beings. Their bioaccumulation has been associated with many toxic effects, including alteration of the immune system, oxidation stress, carcinogenesis, and DNA damage (Solano et al., 2021; Liu et al., 2022). Size, shape, surface charge, and agglomeration state define several toxicological aspects of metal NPs (Fernandes et al., 2022). The risk assessment of these materials should be taken into account, even more accurately if the synthesis is coupled with heavy metal bioremoval from wastewater. Risk assessment and management process must be evaluated through the identification of the physic-chemical properties of the material, hazard identification, and dose-response assessment (Solano et al., 2021).
Cyanobacteria represent a potential tool for heavy metal bioremediation exploiting biosorption or bioaccumulation processes. Cyanobacteria cultivation, harvesting, pre-treatment, and exploitation in heavy metal bioremoval can be manipulated to get efficient metal recovery together with a reduced environmental footprint and the economic cost of the process. Nevertheless, considering cyanobacteria exclusively for their use in the bioremediation sector is economically disadvantageous. The metal-rich biomass obtained through this system can be converted into high-value products, such as metal nanoparticles, to be valorized into pharmaceutical and industrial fields, exploiting a closed-loop system with no waste production. However, more studies are needed to optimize heavy metal bioremoval at an industrial scale and also to explore a feasible and safe valorization of the obtained materials.
The original contributions presented in this study are included in the article/supplementary material, further inquiries can be directed to the corresponding author/s.
MC and AA developed the concept of the manuscript and have critically read, and advised on improvements concerning the science and general outline of the manuscript. MC wrote the initial draft. Both authors contributed to manuscript revision, read, and approved the submitted version.
This work was supported by Fondazione CariplO-Circular Economy 2020 project number: 1069-2020 “Heavy Metal Bio-recovery and Valorization-HMBV”.
The authors declare that the research was conducted in the absence of any commercial or financial relationships that could be construed as a potential conflict of interest.
All claims expressed in this article are solely those of the authors and do not necessarily represent those of their affiliated organizations, or those of the publisher, the editors and the reviewers. Any product that may be evaluated in this article, or claim that may be made by its manufacturer, is not guaranteed or endorsed by the publisher.
Abinandan, S., Subashchandrabose, S. R., Venkateswarlu, K., and Megharaj, M. (2018). Nutrient removal and biomass production: Advances in microalgal biotechnology for wastewater treatment. Crit. Rev. Biotechnol. 38, 1244–1260. doi: 10.1080/07388551.2018.1472066
Agarwal, A., Upadhyay, U., Sreedhar, I., Singh, S. A., and Patel, C. M. (2020). A review on valorization of biomass in heavy metal removal from wastewater. J. Water Proc. Eng. 38:101602. doi: 10.1016/j.jwpe.2020.101602
Ajao, V., Nam, K., Chatzopoulos, P., Spruijt, E., Bruning, H., Rijnaarts, H., et al. (2020). Regeneration and reuse of microbial extracellular polymers immobilised on a bed column for heavy metal recovery. Water Res. 171:115472. doi: 10.1016/j.watres.2020.115472
Al-Amin, A., Parvin, F., Chakraborty, J., and Kim, Y.-I. (2021). Cyanobacteria mediated heavy metal removal: A review on mechanism, biosynthesis, and removal capability. Environ. Technol. Rev. 10, 44–57. doi: 10.1080/21622515.2020.1869323
Alsamhary, K., Al-Enazi, N. M., Alhomaidi, E., and Alwakeel, S. (2022). Spirulina platensis mediated biosynthesis of Cuo Nps and photocatalytic degradation of toxic azo dye Congo red and kinetic studies. Environ. Res. 207:112172. doi: 10.1016/j.envres.2021.112172
Amirnejat, S., Nosrati, A., Peymanfar, R., and Javanshir, S. (2022). Correction to: Synthesis and antibacterial study of 2-amino-4H-pyrans and pyrans annulated heterocycles catalyzed by sulfated polysaccharide-coated BaFe12O19 nanoparticles. Res. Chem. Intermed. 48, 2765–2765. doi: 10.1007/s11164-021-04593-6
Asif, N., Fatima, S., Aziz, M., Shehzadi, Zaki, A., and Fatma, T. (2021). Biofabrication and characterization of cyanobacteria derived ZnO NPs for their bioactivity comparison with commercial chemically synthesized nanoparticles. Bioorg. Chem. 113:104999. doi: 10.1016/j.bioorg.2021.104999
Aziz, N., Zaki, A., Ahamad, I., and Fatma, T. (2021). “Silver nanoparticle synthesis from cyanobacteria: Environmental and biomedical applications,” in Emerging technologies for nanoparticle manufacturing, eds J. K. Patel and Y. V. Pathak (Cham: Springer International Publishing), 461–472. doi: 10.1007/978-3-030-50703-9_21
Barciela, P., Carpena, M., Li, N.-Y., Liu, C., Jafari, S. M., Simal-Gandara, J., et al. (2023). Macroalgae as biofactories of metal nanoparticles; biosynthesis and food applications. Adv. Colloid Interface Sci. 311:102829. doi: 10.1016/j.cis.2022.102829
Bhatt, P., Bhandari, G., Bhatt, K., and Simsek, H. (2022). Microalgae-based removal of pollutants from wastewaters: Occurrence, toxicity and circular economy. Chemosphere 306:135576. doi: 10.1016/j.chemosphere.2022.135576
Blanco-Vieites, M., Casado, V., Battez, A. H., and Rodríguez, E. (2023). Culturing Arthrospira maxima in mining wastewater: Pilot-scale culturing and biomass valorisation into C-phycocyanin and crude lipid extract. Environ. Technol. Innov. 29:102978. doi: 10.1016/j.eti.2022.102978
Bloch, K., and Ghosh, S. (2022). “Chapter 23—Cyanobacteria mediated toxic metal removal as complementary and alternative wastewater treatment strategy,” in Integrated environmental technologies for wastewater treatment and sustainable development, eds V. Kumar and M. Kumar (Amsterdam: Elsevier), 533–548. doi: 10.1016/B978-0-323-91180-1.00002-8
Briffa, J., Sinagra, E., and Blundell, R. (2020). Heavy metal pollution in the environment and their toxicological effects on humans. Heliyon 6:e04691. doi: 10.1016/j.heliyon.2020.e04691
Chai, Y., Chen, A., Bai, M., Peng, L., Shao, J., Yuan, J., et al. (2022). Valorization of heavy metal contaminated biomass: Recycling and expanding to functional materials. J. Clean. Prod. 366:132771. doi: 10.1016/j.jclepro.2022.132771
Chakdar, H., Thapa, S., Srivastava, A., and Shukla, P. (2022). Genomic and proteomic insights into the heavy metal bioremediation by cyanobacteria. J. Hazard. Mater. 424:127609. doi: 10.1016/j.jhazmat.2021.127609
Chan, S. S., Low, S. S., Chew, K. W., Ling, T. C., Rinklebe, J., Juan, J. C., et al. (2022). Prospects and environmental sustainability of phyconanotechnology: A review on algae-mediated metal nanoparticles synthesis and mechanism. Environ. Res. 212:113140. doi: 10.1016/j.envres.2022.113140
Chatterjee, A., and Abraham, J. (2019). Desorption of heavy metals from metal loaded sorbents and e-wastes: A review. Biotechnol. Lett. 41, 319–333. doi: 10.1007/s10529-019-02650-0
Cruz, D., Vasconcelos, V., Pierre, G., Michaud, P., and Delattre, C. (2020). Exopolysaccharides from cyanobacteria: Strategies for bioprocess development. Appl. Sci. 10:11. doi: 10.3390/app10113763
Cui, J., Xie, Y., Sun, T., Chen, L., and Zhang, W. (2021). Deciphering and engineering photosynthetic cyanobacteria for heavy metal bioremediation. Sci. Total Environ. 761:144111. doi: 10.1016/j.scitotenv.2020.144111
De Philippis, R., and Micheletti, E. (2017). “Heavy metal removal with exopolysaccharide-producing cyanobacteria,” in Handbook of advanced industrial and hazardous wastes management, eds L. K. Wang, M.-H. S. Wang, Y.-T. Hung, N. K. Shammas, and J. P. Chen (Boca Raton, FL: CRC Press). doi: 10.1111/j.1365-2672.2008.03728.x
De Philippis, R., Colica, G., and Micheletti, E. (2011). Exopolysaccharide-producing cyanobacteria in heavy metal removal from water: Molecular basis and practical applicability of the biosorption process. Appl. Microbiol. Biotechnol. 92, 697–708. doi: 10.1007/s00253-011-3601-z
Dutta, S., Ghosh, D., Lahiri, A., Chakraborty, S., and Pandit, S. (2022). “Chapter 15 - Cyanoremediation: A clean and green approach toward the sustainable environment,” in Development in wastewater treatment research and processes, eds S. Rodriguez-Couto and M. P. Shah (Amsterdam: Elsevier), 335–354. doi: 10.1016/B978-0-323-85839-7.00017-7
Ebadi, M., Reza Zolfaghari, M., Soheil Aghaei, S., Zargar, M., Shafiei, M., Shahbani Zahiri, H., et al. (2019). A bio-inspired strategy for the synthesis of zinc oxide nanoparticles (ZnO NPs) using the cell extract of cyanobacterium Nostoc sp. EA03: From biological function to toxicity evaluation. RSC Adv. 9, 23508–23525. doi: 10.1039/C9RA03962G
El-Belely, E. F., Farag, M. M. S., Said, H. A., Amin, A. S., Azab, E., Gobouri, A. A., et al. (2021). Green synthesis of Zinc Oxide Nanoparticles (ZnO-NPs) Using Arthrospira platensis (Class: Cyanophyceae) and evaluation of their biomedical activities. Nanomaterials 11:95. doi: 10.3390/nano11010095
El-Naggar, N. E.-A., Hussein, M. H., and El-Sawah, A. A. (2018). Phycobiliprotein-mediated synthesis of biogenic silver nanoparticles, characterization, in vitro and in vivo assessment of anticancer activities. Sci. Rep. 8:8925. doi: 10.1038/s41598-018-27276-6
Encarnação, T., Ramos, P., Mohammed, D., McDonald, J., Lizzul, M., Nicolau, N., et al. (2023). “Bioremediation using microalgae and cyanobacteria and biomass valorisation,” in Marine Organisms: A solution to environmental pollution? Uses in bioremediation and in biorefinery, eds T. Encarnação and A. Canelas Pais (Cham: Springer International Publishing), 5–28. doi: 10.1007/978-3-031-17226-7_2
Fernandes, M., Padrão, J., Ribeiro, A. I., Fernandes, R. D. V., Melro, L., Nicolau, T., et al. (2022). Polysaccharides and metal nanoparticles for functional textiles: A review. Nanomaterials 12:6. doi: 10.3390/nano12061006
Gandolfi, R., Facchetti, G., Cavalca, L., Mazzini, S., Colombo, M., Coffetti, G., et al. (2022). Hybrid catalysts from copper biosorbing bacterial strains and their recycling for catalytic application in the asymmetric addition reaction of B2(pin)2 on α,β-unsaturated chalcones. Catalysts 12:433. doi: 10.3390/catal12040433
Gehlot, P., Pareek, N., and Vivekanand, V. (2022). “Cyanobacterial and microalgal bioremediation: an efficient and eco-friendly approach toward industrial wastewater treatment and value-addition,” in Microbial biodegradation and bioremediation: techniques and case studies for environmental pollution, eds S. Das, and H. R. Dash (Hilversum: Scopus), 343–362. doi: 10.1016/B978-0-323-85455-9.00002-3
Gomes Gradíssimo, D., Pereira Xavier, L., and Valadares Santos, A. (2020). Cyanobacterial polyhydroxyalkanoates: A sustainable alternative in circular economy. Molecules 25:18. doi: 10.3390/molecules25184331
Gorain, P. C., Paul, I., Bhadoria, P. S., and Pal, R. (2019). An integrated approach towards agricultural wastewater remediation with fatty acid production by two cyanobacteria in bubble column photobioreactors. Algal Res. 42:101594. doi: 10.1016/j.algal.2019.101594
Hamida, R. S., Ali, M. A., Redhwan, A., and Bin-Meferij, M. M. (2020). Cyanobacteria – A promising platform in green nanotechnology: A review on nanoparticles fabrication and their prospective applications. Int. J. Nanomed. 15, 6033–6066. doi: 10.2147/IJN.S256134
Hamouda, R. A., Hussein, M. H., Abo-elmagd, R. A., and Bawazir, S. S. (2019). Synthesis and biological characterization of silver nanoparticles derived from the cyanobacterium Oscillatoria limnetica. Sci. Rep. 9:13071. doi: 10.1038/s41598-019-49444-y
Hanna, A. L., Hamouda, H. M., Goda, H. A., Sadik, M. W., Moghanm, F. S., Ghoneim, A. M., et al. (2022). Biosynthesis and characterization of silver nanoparticles produced by Phormidium ambiguum and Desertifilum tharense cyanobacteria. Bioinorg. Chem. Appl. 2022:e9072508. doi: 10.1155/2022/9072508
Husain, S., Afreen, S., Hemlata, Yasin, D., Afzal, B., and Fatma, T. (2019). Cyanobacteria as a bioreactor for synthesis of silver nanoparticles-an effect of different reaction conditions on the size of nanoparticles and their dye decolorization ability. J. Microbiol. Methods 162, 77–82. doi: 10.1016/j.mimet.2019.05.011
Ijaz, I., Bukhari, A., Gilani, E., Nazir, A., Zain, H., Saeed, R., et al. (2022). Green synthesis of silver nanoparticles using different plants parts and biological organisms, characterization and antibacterial activity. Environ. Nanotechnol. Monit. Manag. 18:100704. doi: 10.1016/j.enmm.2022.100704
Ismail, G. A., Allam, N. G., El-Gemizy, W. M., and Salem, M. A. (2021). The role of silver nanoparticles biosynthesized by Anabaena variabilis and Spirulina platensis cyanobacteria for malachite green removal from wastewater. Environ. Technol. 42, 4475–4489. doi: 10.1080/09593330.2020.1766576
Keskin, N. O. S., Kılıç, N. K., Dönmez, G., and Tekinay, T. (2016). Green synthesis of silver nanoparticles using cyanobacteria and evaluation of their photocatalytic and antimicrobial activity. J. Nano Res. 40, 120–127. doi: 10.4028/www.scientific.net/JNanoR.40.120
Kholssi, R., Ramos, P. V., Marks, E. A. N., Montero, O., and Rad, C. (2021). 2Biotechnological uses of microalgae: A review on the state of the art and challenges for the circular economy. Biocatal. Agric. Biotechnol. 36:102114. doi: 10.1016/j.bcab.2021.102114
Li, S., Dai, M., Wu, Y., Fu, H., Hou, X., Peng, C., et al. (2022). Resource utilization of electroplating wastewater: Obstacles and solutions. Environ. Sci. Water Res. Technol. 8, 484–509. doi: 10.1039/D1EW00712B
Liu, Z., Malinowski, C. R., and Sepúlveda, M. S. (2022). Emerging trends in nanoparticle toxicity and the significance of using Daphnia as a model organism. Chemosphere 291:132941. doi: 10.1016/j.chemosphere.2021.132941
Mandhata, C. P., Sahoo, C. R., and Padhy, R. N. (2022). Biomedical applications of biosynthesized gold nanoparticles from cyanobacteria: An overview. Biol. Trace Elem. Res. 200, 5307–5327. doi: 10.1007/s12011-021-03078-2
Mandhata, C. P., Sahoo, C. R., Mahanta, C. S., and Padhy, R. N. (2021). Isolation, biosynthesis and antimicrobial activity of gold nanoparticles produced with extracts of Anabaena spiroides. Bioproc. Biosyst. Eng. 44, 1617–1626. doi: 10.1007/s00449-021-02544-4
Market Analysis Report (2021). Nanomaterials Market Size, Share and Growth. Available online at: https://www.grandviewresearch.com/industry-analysis/nanotechnology-and-nanomaterials-market (accessed December 15, 2022).
Mona, S., Kumar, V., Deepak, B., and Kaushik, A. (2020). “Cyanobacteria: The eco-friendly tool for the treatment of industrial wastewaters,” in Bioremediation of industrial waste for environmental safety: Volume II: Biological agents and methods for industrial waste management, eds R. N. Bharagava and G. Saxena (Cham: Springer), 389–413. doi: 10.1007/978-981-13-3426-9_16
Morais, M. G., Santos, T. D., Moraes, L., Vaz, B. S., Morais, E. G., and Costa, J. A. V. (2022). Exopolysaccharides from microalgae: Production in a biorefinery framework and potential applications. Bioresour. Technol. Rep. 18:101006. doi: 10.1016/j.biteb.2022.101006
Morsy, F. M., Nafady, N. A., Abd-Alla, M. H., and Elhady, D. A. (2014). Green synthesis of silver nanoparticles by water soluble fraction of the extracellular polysaccharides/matrix of the cyanobacterium Nostoc commune and its application as a potent fungal surface sterilizing agent of seed crops. Univ. J. Microbiol. Res. 2, 36–43. doi: 10.13189/ujmr.2014.020303
Mota, R., Flores, C., and Tamagnini, P. (2022). “Cyanobacterial extracellular polymeric substances (EPS),” in Polysaccharides of microbial origin, eds J. M. Oliveira, H. Radhouani, and R. L. Reis (Cham: Springer International Publishing), 139–165. doi: 10.1007/978-3-030-42215-8_11
Mota, R., Rossi, F., Andrenelli, L., Pereira, S. B., De Philippis, R., and Tamagnini, P. (2016). Released polysaccharides (RPS) from Cyanothece sp. CCY 0110 as biosorbent for heavy metals bioremediation: Interactions between metals and RPS binding sites. Appl. Microbiol. Biotechnol. 100, 7765–7775. doi: 10.1007/s00253-016-7602-9
MubarakAli, D., Gopinath, V., Rameshbabu, N., and Thajuddin, N. (2012). Synthesis and characterization of CdS nanoparticles using C-phycoerythrin from the marine cyanobacteria. Mater. Lett. 74, 8–11. doi: 10.1016/j.matlet.2012.01.026
Netanel Liberman, G., Ochbaum, G., Bitton, R., and Arad, S. (2021). Antimicrobial hydrogels composed of chitosan and sulfated polysaccharides of red microalgae. Polymer 215:123353. doi: 10.1016/j.polymer.2020.123353
Pandey, S., Dubey, S. K., Kashyap, A. K., and Jain, B. P. (2022a). “Cyanobacteriamediated heavy metal and xenobiotics bioremediation,” in Cyanobacterial lifestyle and its applications in biotechnology, eds P. Singh, M. Fillat, and A. Kumar (Cambridge, MA: Academic Press), 335–350. doi: 10.1016/B978-0-323-90634-0.00001-9
Pandey, S., Rai, L. C., and Dubey, S. K. (2022b). Cyanobacteria: Miniature factories for green synthesis of metallic nanomaterials: A review. BioMetals 35, 653–674. doi: 10.1007/s10534-022-00405-5
Patel, V., Berthold, D., Puranik, P., and Gantar, M. (2015). Screening of cyanobacteria and microalgae for their ability to synthesize silver nanoparticles with antibacterial activity. Biotechnol. Rep. 5, 112–119. doi: 10.1016/j.btre.2014.12.001
Potnis, A. A., Raghavan, P. S., and Rajaram, H. (2021). Overview on cyanobacterial exopolysaccharides and biofilms: Role in bioremediation. Rev. Environ. Sci. Biotechnol. 20, 781–794. doi: 10.1007/s11157-021-09586-w
Prabha, S., Vijay, A. K., Paul, R. R., and George, B. (2022). Cyanobacterial biorefinery: Towards economic feasibility through the maximum valorization of biomass. Sci. Total Environ. 814:152795. doi: 10.1016/j.scitotenv.2021.152795
Priya, A. K., Gnanasekaran, L., Dutta, K., Rajendran, S., Balakrishnan, D., and Soto-Moscoso, M. (2022). Biosorption of heavy metals by microorganisms: Evaluation of different underlying mechanisms. Chemosphere 307:135957. doi: 10.1016/j.chemosphere.2022.135957
Priyanka, Kumar, C., Chatterjee, A., Wenjing, W., Yadav, D., and Singh, P. K. (2020). “Chapter 10 - Cyanobacteria: Potential and role for environmental remediation,” in Abatement of environmental pollutants, eds P. Singh, A. Kumar, and A. Borthakur (Amsterdam: Elsevier), 193–202. doi: 10.1016/B978-0-12-818095-2.00010-2
Raghavan, P. S., Potnis, A. A., Bhattacharyya, K., Salaskar, D. A., and Rajaram, H. (2020). Axenic cyanobacterial (Nostoc muscorum) biofilm as a platform for Cd(II) sequestration from aqueous solutions. Algal Res. 46:101778. doi: 10.1016/j.algal.2019.101778
Ramírez Calderón, O. A., Abdeldayem, O. M., Pugazhendhi, A., and Rene, E. R. (2020). Current updates and perspectives of biosorption technology: An alternative for the removal of heavy metals from wastewater. Current Pollut. Rep. 6, 8–27. doi: 10.1007/s40726-020-00135-7
Rueda, E., García-Galán, M. J., Ortiz, A., Uggetti, E., Carretero, J., García, J., et al. (2020). Bioremediation of agricultural runoff and biopolymers production from cyanobacteria cultured in demonstrative full-scale photobioreactors. Proc. Saf. Environ. Protect. 139, 241–250. doi: 10.1016/j.psep.2020.03.035
Sachdeva, N., Mascolo, C., Wattiez, R., and Leroy, B. (2018). Embedding photosynthetic biorefineries with circular economies: Exploring the waste recycling potential of Arthrospira sp. to produce high quality by-products. Bioresour. Technol. 268, 237–246. doi: 10.1016/j.biortech.2018.07.101
Saran, S., Sharma, G., Kumar, M., and Ali, M. I. (2017). Biosynthesis of copper oxide nanoparticles using cyanobacteria Spirulina platensis and its antibacterial activity. Int. J. Pharm. Sci. Res. 8, 3887–3892. doi: 10.13040/IJPSR.0975-8232.8(9).3887-92
Satya, A., Harimawan, A., Sri Haryani, G., Johir, M. A. H., Nguyen, L. N., Nghiem, L. D., et al. (2021). Fixed-bed adsorption performance and empirical modelingof cadmium removal using adsorbent prepared from the cyanobacterium Aphanothece sp cultivar. Environ. Technol. Innov. 21:101194. doi: 10.1016/j.eti.2020.101194
Selmani, A., Kovaèeviæ, D., and Bohinc, K. (2022). Nanoparticles: From synthesis to applications and beyond. Adv. Colloid Interface Sci. 303:102640. doi: 10.1016/j.cis.2022.102640
Serrà, A., Artal, R., García-Amorós, J., Gómez, E., and Philippe, L. (2020). Circular zero-residue process using microalgae for efficient water decontamination, biofuel production, and carbon dioxide fixation. Chem. Eng. J. 388:124278. doi: 10.1016/j.cej.2020.124278
Sheldon, R. A., and Woodley, J. M. (2018). Role of biocatalysis in sustainable chemistry. Chem. Rev. 118, 801–838. doi: 10.1021/acs.chemrev.7b00203
Siddiqui, T., Khan, N. J., Asif, N., Ahamad, I., Yasin, D., and Fatma, T. (2022). Screening, characterisation and bioactivities of green fabricated TiO2 NP via cyanobacterial extract. Environ. Sci. Pollut. Res. 29, 39052–39066. doi: 10.1007/s11356-021-17639-4
Singh, S. (2020). “Biosorption of heavy metals by cyanobacteria: Potential of live and dead cells in bioremediation,” in Microbial bioremediation and biodegradation, ed. M. P. Shah (Cham: Springer), 409–423. doi: 10.1007/978-981-15-1812-6_15
Singh, Y., Kaushal, S., and Sodhi, R. S. (2020). Biogenic synthesis of silver nanoparticles using cyanobacterium Leptolyngbya sp. WUC 59 cell-free extract and their effects on bacterial growth and seed germination. Nanoscale Adv. 2, 3972–3982. doi: 10.1039/D0NA00357C
Solano, R., Patiño-Ruiz, D., Tejeda-Benitez, L., and Herrera, A. (2021). Metal- and metal/oxide-based engineered nanoparticles and nanostructures: A review on the applications, nanotoxicological effects, and risk control strategies. Environ. Sci. Pollut. Res. 28, 16962–16981. doi: 10.1007/s11356-021-12996-6
Souza, J., Costa, G. P., Luque, R., Alves, D., and Fajardo, A. R. (2019). Polysaccharide-based superporous hydrogel embedded with copper nanoparticles: A green and versatile catalyst for the synthesis of 1,2,3-triazoles. Catal. Sci. Technol. 9, 136–145. doi: 10.1039/C8CY01796D
Sutherland, I. W. (1990). Biotechnology of microbial exopolysaccharides. Cambridge: Cambridge University Press.
Thevarajah, B., Nishshanka, G. K. S. H., Premaratne, M., Wasath, W. A. J., Nimarshana, P. H. V., Malik, A., et al. (2023). Cyanobacterial pigment production in wastewaters treated for heavy metal removal: Current status and perspectives. J. Environ. Chem. Eng. 11:108999. doi: 10.1016/j.jece.2022.108999
Uma Suganya, K. S., Govindaraju, K., Ganesh Kumar, V., Stalin Dhas, T., Karthick, V., Singaravelu, G., et al. (2015). Blue green alga mediated synthesis of gold nanoparticles and its antibacterial efficacy against Gram positive organisms. Mater. Sci. Eng. C 47, 351–356. doi: 10.1016/j.msec.2014.11.043
US EPA (2015). Metals [Data and Tools]. Available online at: https://www.epa.gov/caddis-vol2/metals (accessed November 5, 2022).
Velenturf, A. P. M., and Purnell, P. (2021). Principles for a sustainable circular economy. Sustain. Prod. Consum. 27, 1437–1457. doi: 10.1016/j.spc.2021.02.018
Velkova, Z., Kirova, G., Stoytcheva, M., Kostadinova, S., Todorova, K., and Gochev, V. (2018). Immobilized microbial biosorbents for heavy metals removal. Eng. Life Sci. 18, 871–881. doi: 10.1002/elsc.201800017
Velu, C., Cirés, S., Brinkman, D. L., and Heimann, K. (2020). Bioproduct potential of outdoor cultures of Tolypothrix sp.: Effect of carbon dioxide and metal-rich wastewater. Front. Bioeng. Biotechnol. 8:51. doi: 10.3389/fbioe.2020.00051
Wan Mahari, W. A., Wan Razali, W. A., Manan, H., Hersi, M. A., Ishak, S. D., Cheah, W., et al. (2022). Recent advances on microalgae cultivation for simultaneous biomass production and removal of wastewater pollutants to achieve circular economy. Bioresour. Technol. 364:128085. doi: 10.1016/j.biortech.2022.128085
Wolfson, A., Biton, S., and Levy-Ontman, O. (2018). Study of Pd-based catalysts within red algae-derived polysaccharide supports in a Suzuki cross-coupling reaction. RSC Adv. 8, 37939–37948. doi: 10.1039/C8RA08408D
Yadav, A. P. S., Dwivedi, V., Kumar, S., Kushwaha, A., Goswami, L., and Reddy, B. S. (2021). Cyanobacterial extracellular polymeric substances for heavy metal removal: A mini review. J. Compos. Sci. 5:1. doi: 10.3390/jcs5010001
Younis, N. S., Bakir, E. M., Mohamed, M. E., and El Semary, N. A. (2019). Cyanobacteria as nanogold factories II: Chemical reactivity and anti-myocardial infraction properties of customized gold nanoparticles biosynthesized by Cyanothece sp. Mar. Drugs 17:402. doi: 10.3390/md17070402
Younis, N. S., Mohamed, M. E., and El Semary, N. A. (2022). Green synthesis of silver nanoparticles by the cyanobacteria Synechocystis sp.: Characterization, antimicrobial and diabetic wound-healing actions. Mar. Drugs 20:56. doi: 10.3390/md20010056
Yugay, Y. A., Usoltseva, R. V., Silant’ev, V. E., Egorova, A. E., Karabtsov, A. A., Kumeiko, V. V., et al. (2020). Synthesis of bioactive silver nanoparticles using alginate, fucoidan and laminaran from brown algae as a reducing and stabilizing agent. Carbohydr. Polym. 245:116547. doi: 10.1016/j.carbpol.2020.116547
Zanganeh, F., Heidari, A., Sepehr, A., and Rohani, A. (2022). Bioaugmentation and bioaugmentation–assisted phytoremediation of heavy metal contaminated soil by a synergistic effect of cyanobacteria inoculation, biochar, and purslane (Portulaca oleracea L.). Environ. Sci. Pollut. Res. 29, 6040–6059. doi: 10.1007/s11356-021-16061-0
Zayadi, R. A., and Abu Bakar, F. (2020). Comparative study on stability, antioxidant and catalytic activities of bio-stabilized colloidal gold nanoparticles using microalgae and cyanobacteria. J. Environ. Chem. Eng. 8:103843. doi: 10.1016/j.jece.2020.103843
Zinicovscaia, I., Safonov, A., Ostalkevich, S., Gundorina, S., Nekhoroshkov, P., and Grozdov, D. (2019). Metal ions removal from different type of industrial effluents using Spirulina platensis biomass. Int. J. Phytoremediation 21, 1442–1448. doi: 10.1080/15226514.2019.1633264
Keywords: cyanobacteria, bioremediation, heavy metal biosorption, metal nanoparticles, sustainability
Citation: Ciani M and Adessi A (2023) Cyanoremediation and phyconanotechnology: cyanobacteria for metal biosorption toward a circular economy. Front. Microbiol. 14:1166612. doi: 10.3389/fmicb.2023.1166612
Received: 15 February 2023; Accepted: 09 May 2023;
Published: 30 May 2023.
Edited by:
Obulisamy Parthiba Karthikeyan, South Dakota School of Mines and Technology, United StatesReviewed by:
Riti Thapar Kapoor, Amity University Noida, IndiaCopyright © 2023 Ciani and Adessi. This is an open-access article distributed under the terms of the Creative Commons Attribution License (CC BY). The use, distribution or reproduction in other forums is permitted, provided the original author(s) and the copyright owner(s) are credited and that the original publication in this journal is cited, in accordance with accepted academic practice. No use, distribution or reproduction is permitted which does not comply with these terms.
*Correspondence: Alessandra Adessi, YWxlc3NhbmRyYS5hZGVzc2lAdW5pZmkuaXQ=
Disclaimer: All claims expressed in this article are solely those of the authors and do not necessarily represent those of their affiliated organizations, or those of the publisher, the editors and the reviewers. Any product that may be evaluated in this article or claim that may be made by its manufacturer is not guaranteed or endorsed by the publisher.
Research integrity at Frontiers
Learn more about the work of our research integrity team to safeguard the quality of each article we publish.