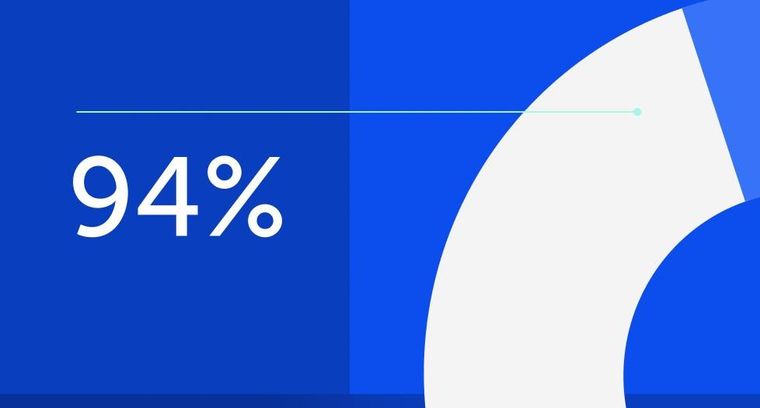
94% of researchers rate our articles as excellent or good
Learn more about the work of our research integrity team to safeguard the quality of each article we publish.
Find out more
ORIGINAL RESEARCH article
Front. Microbiol., 17 April 2023
Sec. Microbiological Chemistry and Geomicrobiology
Volume 14 - 2023 | https://doi.org/10.3389/fmicb.2023.1162788
Azoarcus sp. DN11 was previously isolated from gasoline-contaminated groundwater as an anaerobic benzene-degrading bacterium. Genome analysis of strain DN11 revealed that it contained a putative idr gene cluster (idrABP1P2), which was recently found to be involved in bacterial iodate (IO3−) respiration. In this study, we determined if strain DN11 performed iodate respiration and assessed its potential use to remove and sequester radioactive iodine (129I) from subsurface contaminated aquifers. Strain DN11 coupled acetate oxidation to iodate reduction and grew anaerobically with iodate as the sole electron acceptor. The respiratory iodate reductase (Idr) activity of strain DN11 was visualized on non-denaturing gel electrophoresis, and liquid chromatography–tandem mass spectrometry analysis of the active band suggested the involvement of IdrA, IdrP1, and IdrP2 in iodate respiration. The transcriptomic analysis also showed that idrA, idrP1, and idrP2 expression was upregulated under iodate-respiring conditions. After the growth of strain DN11 on iodate, silver-impregnated zeolite was added to the spent medium to remove iodide from the aqueous phase. In the presence of 200 μM iodate as the electron acceptor, more than 98% of iodine was successfully removed from the aqueous phase. These results suggest that strain DN11 is potentially helpful for bioaugmentation of 129I-contaminated subsurface aquifers.
Iodine is an essential trace element in humans and animals because it is a constituent of the thyroid hormones triiodothyronine (T3) and thyroxine (T4) (Dijck-Brouwer et al., 2022). Insufficient dietary iodine can cause deficiency disorders such as endemic goiter and congenital hypothyroidism (Chaker et al., 2022). Iodine deficiency often occurs in inland and mountainous regions, whereas it is less likely to occur in coastal areas where people consume iodine-rich seafood.
Iodine is primarily present in the ocean, and the average concentration of dissolved iodine in seawater is 0.45 μM (Wong, 1991; Fuge and Johnson, 2015). Inorganic iodate (IO3−) and iodide (I−) ions are the predominant chemical forms of iodine in seawater. Thermodynamically, the concentration ratio of iodate to iodide (IO3−/I−) in oxygenated seawater is 1013.5 (Sillen, 1961). This indicates that iodate is relatively more stable and that iodide should not be detectable in seawater. However, significant quantities of iodide are observed in certain surface waters (Wong, 1991). This apparent disequilibrium may be due to the biological reduction of iodate to iodide. Generally, it is difficult to convert iodate to volatile iodine species such as I2 and CH3I. However, iodide is easily converted to I2 and CH3I and released into the atmosphere (Amachi et al., 2001, 2005). Gaseous iodine compounds emitted from seawater are photolyzed and reach terrestrial environments through wet (rainwater) or dry deposition, where they accumulate in plants and animals (Fuge and Johnson, 2015). Therefore, iodate reduction in the ocean is vital in providing this essential trace element to humans and animals. Gaseous iodine compounds in the atmosphere also contribute to ozone (O3) destruction in the troposphere and the formation of cloud condensation nuclei and are thus involved in climate change on a global scale (O'Dowd et al., 2002; Carpenter et al., 2021).
Biological iodate reduction has been studied in marine microalgae (phytoplankton) and bacteria (Amachi, 2008; Yeager et al., 2017). Among these organisms, the molecular mechanism of iodate reduction has recently become clear in dissimilatory iodate-reducing bacteria (DIRB), such as Pseudomonas sp. SCT and Denitromonas sp. IR-12 (Yamazaki et al., 2020; Reyes-Umana et al., 2022a). DIRB possesses an idrABP1P2 gene cluster in its genome. The idrA and idrB genes encode the large catalytic subunit and electron-transferring small subunit of novel dimethylsulfoxide (DMSO) reductase family protein, Idr, respectively (Yamazaki et al., 2020). Although the function of idrP1P2 gene products is still unclear, they may be involved in detoxifying hydrogen peroxide (H2O2), which is supposed to be formed during iodate reduction (Yamazaki et al., 2020; Reyes-Umana et al., 2022a). The idrABP1P2 gene cluster is distributed not only in marine bacteria but also in terrestrial bacteria, including subsurface and groundwater metagenome libraries, suggesting the possibility that DIRB plays a crucial role in the transformation of iodate in terrestrial environments (Yamazaki et al., 2020).
A long-lived radioactive iodine 129I (half-life: 1.6 × 107 years) is naturally produced in the atmosphere by cosmic ray interactions with xenon, and by the spontaneous fission of 238U in the earth’s crust (Fabryka-Martin et al., 1985). However, in the post nuclear era, the amount of anthropogenic 129I released from nuclear weapon tests and nuclear facilities is higher than that produced naturally (Kaplan et al., 2014). 129I was also released into the environment from two major nuclear accidents, Chernobyl in 1986 and Fukushima in 2011 (Yeager et al., 2017). Due to its possible accumulation in the human thyroid gland and rapid mobility in the subsurface environment, 129I is one of the three key risk drivers at several US Department of Energy (DOE) sites, such as the Hanford Site and the Savannah River Site (SRS) (Kaplan et al., 2014). Both sites have contaminant plumes containing 129I at concentrations well above the drinking water standard of 0.04 Bq L−1 (Neeway et al., 2019). Iodine speciation analysis in groundwater samples recovered from the Hanford site indicated that iodate was the predominant species (Zhang et al., 2013). Similarly, in groundwater samples collected from the SRS, iodate accounted for 7 to 100% of the 129I (Otosaka et al., 2011). Radioactive iodine can be removed and sequestered by silver-impregnated sorbents such as zeolite and granular activated carbon, but they are ineffective at removing iodate (Kaplan et al., 2019; Li et al., 2019). Therefore, bacterial iodate reduction to form iodide could be a promising way to help the restoration of 129I-contaminated subsurface aquifers. However, to date, there are only scarce information on iodate reduction by terrestrial bacteria.
In this study, we focused on Azoarcus sp. DN11, a denitrifying benzene-degrading bacterium previously isolated from a gasoline-contaminated subsurface aquifer at Kumamoto-city, Japan (Kasai et al., 2006, 2007). Because strain DN11 is a terrestrial bacterium possessing a putative idrABP1P2 gene cluster in its genome, we first determined whether this strain could perform iodate respiration. The involvement of the Idr proteins in iodate respiration was confirmed using proteomic and transcriptomic techniques. Finally, silver-impregnated zeolite was added to the spent medium of strain DN11 to assess its potential use in the removal and sequestration of 129I from contaminated aquifers.
Azoarcus sp. DN11 was grown anaerobically using 2 mM acetate and 2 mM iodate as the electron donor and acceptor. As shown in Figure 1, strain DN11 coupled acetate oxidation to iodate reduction, and iodate was reduced entirely to an equivalent amount of iodide. The growth of strain DN11 coincided with the reduction of iodate, but the strain did not grow when iodate or acetate was omitted from the growth medium. These results strongly suggest that acetate and iodate are required for the growth of strain DN11 and that iodate reduction by this strain is a respiratory process. The following equation can express acetate oxidation coupled with iodate reduction:
Figure 1. Growth of strain DN11 with 2 mM acetate as the electron donor and 2 mM iodate as the electron acceptor. Growth in the absence of acetate (white diamonds) and in the absence of iodate (crosses) is also shown. All experiments were performed in triplicate, and error bars represent standard deviations. The absence of bars indicates that the error is smaller than the symbols.
3C2H3O2− + 4IO3− + 3H+ → 4I− + 6CO2 + 6H2O (ΔG0′ = −743 kJ/mol acetate).
This indicates that 1.5 mM of acetate is required for the respiratory reduction of 2 mM iodate. However, as shown in Figure 1, nearly equal amounts of acetate and iodate were consumed by DN11. This can be explained by the incorporation of some carbon into the cell biomass.
Strain DN11 was grown in the presence of 2 mM iodate or 2 mM nitrate as the electron acceptor, and Idr activity and respiratory nitrate reductase (Nar) activity in the crude extracts were determined using reduced methyl viologen (MV) as an electron donor. Under iodate-respiring conditions, significant Idr activity (2.98 U mg protein−1) was observed, while no significant Nar activity (less than 0.1 U mg protein−1) was detected in the crude extracts (Supplementary Table S1). Under denitrification conditions, no significant Idr activity was observed, while 0.96 U mg protein−1 of Nar activity was detected (Supplementary Table S1). These results suggest that Idr activity is induced under iodate-respiring conditions in DN11.
After crude extracts were subjected to SDS-polyacrylamide gel electrophoresis (SDS-PAGE) under non-denaturing conditions, the gel was stained for Idr activity. A single clear band was observed on the gel (Figure 2A), and it was then excised and run again on SDS-PAGE under fully denaturing conditions. As shown in Figure 2B, three bands (designated A, B, and C) with apparent molecular weights of 100, 80, and 50 kDa were observed after Coomassie brilliant blue (CBB) staining. All bands were excised, trypsin-digested, and subjected to liquid chromatography–tandem mass spectrometry (LC–MS/MS) analysis. From band A, 49 proteins with apparent molecular weights of 16–171 kDa were identified (Supplementary Table S2A). Among these, a protein with accession number AYH44092.1 showed the highest sequence coverage of 77%. In addition, this protein had the highest exclusive unique peptide count of 72. BLASTP analysis of this protein revealed that it is a homolog of IdrA in Pseudomonas sp. SCT. Other proteins included homologs of IdrP1 (AYH44094.1) and IdrP2 (AYH44095.1), with a sequence coverage of 15 and 20%, respectively. From band B, 56 proteins were identified, among which the homologs of IdrA, IdrP1, and IdrP2 were detected with sequence coverages of 31, 41, and 39%, respectively (Supplementary Table S2B). Similarly, 88 proteins were identified from band C, from which the homologs of IdrA, IdrP1 and IdrP2 were detected with sequence coverage of 45, 19, and 29%, respectively (Supplementary Table S2C). The fact that homologs of IdrA, IdrP1 and IdrP2 were detected repeatedly from bands A, B, and C with relatively high sequence coverage strongly suggests that these proteins are involved in iodate respiration by strain DN11.
Figure 2. (A) Activity staining of Idr protein. The crude extracts were run on sodium dodecyl sulfate (SDS)-polyacrylamide gel electrophoresis at 4°C under non-denatured conditions (without SDS and reducing agents). After the electrophoresis, the gel was stained anaerobically with methyl viologen and iodate as the electron donor and acceptor, respectively. A clear band represents the active band. (B) Separation of the excised active band under completely denatured conditions (100°C, 5 min with SDS and 2-mercaptoethanol). The gel was stained with Coomassie brilliant blue R-250. Lane 1 represents the standard marker proteins. Three bands with molecular weights of approximately 100 kDa (band A), 80 kDa (band B), and 50 kDa (band C) were detected.
In the genome of DN11, the idrABP1P2 gene cluster formed an operon-like structure (Supplementary Figure S1A). Upstream of the idr gene cluster, a putative sigma-54 dependent transcriptional regulator gene (atoC) and a two-component system-sensor histidine kinase gene (atoS) were located. However, c-type cytochrome gene as well as molybdenum cofactor biosynthesis genes, both of which are present in the vicinity of the idr gene cluster in Pseudomonas sp. SCT (Yamazaki et al., 2020), were absent. One exception was that the moeB gene was upstream of the atoS gene. As shown in Supplementary Figure S1B, the amino acid sequence of IdrA (AYH44092.1) predicted that it included a [3Fe-4S]-binding motif (CX2CX3CX70S) and molybdopterin-guanine dinucleotide coordination motif. In addition, IdrB (AYH44093.1) appears to include a [2Fe-2S]-binding motif (CXHX18CX2H) and a twin-arginine translocation (TAT) signal sequence (SRRSFL) at the N-terminal region. Both IdrP1 (AYH44094.1) and IdrP2 (AYH44095.1) are diheme proteins containing two heme-binding motifs (CX2CH). The SignalP 5.0 server predicted that both IdrP1 and IdrP2 possessed a signal peptide sequence for the Sec translocation system. These features were similar to those observed for IdrABP1P2 of Pseudomonas sp. SCT (Yamazaki et al., 2020), and suggest that these Idr proteins are secreted and function in the bacterial periplasmic space.
The expression of idrA, idrP1, and idrP2 relative to the expression of recA was quantified and compared using RNA extracted from iodate-, nitrate-, or aerobically (O2)-grown cells of strain DN11. The respiratory nitrate reductase gene (narG) expression was also quantified. As shown in Table 1, the idrA gene in iodate-grown cells showed 104- and 59-fold upregulation compared to that in nitrate- and O2-grown cells, respectively (0.71 versus 0.0068 versus 0.012). Similarly, idrP1 expression in iodate-grown cells was 53- and 67-fold higher than in nitrate- and O2-grown cells. The expression patterns of idrP2 were similar to those of idrA and idrP1; 26- and 12-fold upregulation was observed in iodate-grown cells. In contrast, the expression of narG was highest in nitrate-grown cells, which was 1,260-fold higher than that in iodate- and O2-grown cells (2.4 versus 0.0019).
Table 1. Expression of idr genes and narG gene relative to that of recA gene in iodate-, nitrate-, or aerobically grown cells.
Strain DN11 was grown anaerobically using 200 μM iodate as the electron acceptor. After growth, the liquid culture was mixed vigorously with silver-impregnated zeolite (AgIZ), and the iodine (iodate and iodide) in the supernatant was determined. Before the inoculation of strain DN11, the iodate concentration in the medium was constant (192–193 μM), regardless of AgIZ addition (Table 2). This indicates that AgIZ did not remove iodate under our experimental conditions. However, after the growth of strain DN11, the iodide produced by strain DN11 (198 μM) was removed completely from the supernatant by AgIZ. Consequently, neither iodate nor iodide was detected in the supernatant. Because the detection limit of iodide under our experimental conditions was 5 μM, the efficacy of iodine removal was calculated to be more than 97.5%.
Table 2. Removal of iodine from the growth medium of strain DN11 by silver-impregnated zeolitea.
The first DIRB, Pseudomonas sp. SCT, was isolated from the marine sediment of Sagami Bay (Amachi et al., 2005), and proteomic and transcriptomic analyses of this strain have suggested the involvement of IdrABP1P2 proteins in iodate respiration (Yamazaki et al., 2020). Reyes-Umana et al. (2022a) isolated another DIRB, Denitromonas sp. IR-12, from San Francisco Bay estuarine sediment and demonstrated that the idrA gene is responsible for iodate respiration by constructing a gene knockout mutant. Very recently, Reyes-Umana et al. (2022b) isolated a third DIRB from freshwater creek sediment located in the vicinity of the Northern Pacific Ocean. This bacterium, Aromatoleum toluclasticum TC-10, is phylogenetically closely related to Azoarcus sp. DN11 and coupled acetate oxidation to iodate reduction to conserve the energy for growth. In this study, we found that Azoarcus sp. DN11 can also grow anaerobically in the presence of acetate and iodate as the electron donor and acceptor, respectively. Strain DN11 was previously isolated from gasoline-contaminated groundwater in Higashino, Kumamoto-city, Japan (Takahata et al., 2006), approximately 15 km inland from the coastal area. To our knowledge, this is the first report of a DIRB isolated from a terrestrial groundwater system. Such bacteria could potentially be helpful for the removal and sequestration of 129I, which contaminates underground aquifers at relatively low concentrations (Kaplan et al., 2014).
Strain DN11 can utilize nitrate as the sole electron acceptor via nar genes encoding respiratory nitrate reductase (Kasai et al., 2006, 2007). However, DN11 cells grown under denitrification conditions showed no Idr activity, whereas cells grown under iodate-respiring conditions showed no Nar activity (Supplementary Table S1). Similarly, the expression of idr genes was downregulated under denitrification conditions, whereas narG was downregulated under iodate-respiring conditions (Table 1). These results were in good agreement with those observed for Pseudomonas sp. SCT (Yamazaki et al., 2020), and support the idea that nitrate reductase is not involved in iodate reduction by DIRB (Amachi et al., 2005; Mok et al., 2018; Reyes-Umana et al., 2022b). If strain DN11 is used for the bioaugmentation of 129I-contaminated groundwater in the future, pre-cultivation of this strain in the presence of stable iodate (127IO3−) may be necessary for sufficient induction of the idr gene cluster.
Previously, the active Idr band of Pseudomonas sp. SCT was excised and subjected to SDS-PAGE (Yamazaki et al., 2020). Three bands with molecular weights of 100, 40, and 35 kDa were observed, and IdrA, IdrP1, and IdrP2 were detected from these bands with the highest sequence coverage. This was reasonable because the predicted molecular weights of IdrA, IdrP1, and IdrP2 are approximately 100, 40, and 40 kDa, respectively. In addition, multiple Idr proteins were detected in these bands, with relatively lower sequence coverage. For example, the 100- and 35-kDa bands included all Idr proteins (IdrA, IdrB, IdrP1, and IdrP2), whereas no other proteins (non-Idr proteins) were recovered from these bands. In this study, three bands were observed after SDS-PAGE, with molecular weights of 100, 80, and 50 kDa (Figure 2). All these bands included IdrA, IdrP1, and IdrP2 proteins, but a wide variety of non-Idr proteins that are irrelevant to iodate respiration were also recovered (Supplementary Table S2). Although active bands often include proteins that are not targeted or differ from the predicted molecular weight, the exact reason for this is still unclear (Tsuchiya et al., 2019; Muramatsu et al., 2020). Nevertheless, the fact that active bands recovered from both strains SCT and DN11 included multiple Idr proteins strongly suggests that IdrABP1P2 forms a protein complex possibly in the bacterial periplasmic space. Further biochemical studies are required to understand the molecular weight and subunit composition of the Idr protein complex.
Yamazaki et al. (2020) proposed a hypothetical model for iodate respiration by Pseudomonas sp. SCT, in which iodate is first reduced to an intermediate, hypoiodous acid (HIO), by IdrAB with concomitant hydrogen peroxide (H2O2) production. H2O2 is then reduced to H2O by IdrP1 and (or) IdrP2 using reduced cytochrome c as the electron donor. HIO, a potentially toxic compound, is assumed to be detoxified by a chlorite dismutase (Cld)-like protein, which is abundantly expressed in iodate-respiring cells of strain SCT, to form iodide and molecular oxygen.
Reyes-Umana et al. (2022a) proposed an alternative model of iodate respiration in which HIO is chemically disproportionated to yield iodide and iodate in a 2:1 ratio since HIO is thermodynamically unstable, especially at pH > 5.0.
Although whether HIO is degraded biochemically or abiotically in DIRB is controversial, we could not find a gene encoding a Cld-like protein in the draft genome of strain DN11. This might indicate that HIO is degraded abiotically or that a yet-identified protein is involved in HIO degradation in strain DN11.
Combining DN11 and AgIZ, iodate was removed completely from the aqueous culture medium as solid silver iodide (AgI) (Table 2). Due to ion chromatography’s high detection limits (3 μM for iodate and 5 μM for iodide), we applied 200 μM iodate, which is several orders of magnitude higher than the environmentally relevant concentration. The average total iodine concentration in groundwater collected from the Hanford Site was approximately 0.2 μM, 45–84% as iodate (Zhang et al., 2013). In SRS F-area, on the other hand, iodate concentration was reported to be ranging from 0 to 0.075 μM (Otosaka et al., 2011). Since the solubility product of AgI is very low (Ksp = 1 × 10−16), more than 3 × 10−5 μM of iodide can theoretically be removed by AgIZ as AgI. Thus, the possible limiting factor for the restoration of 129I in subsurface aquifers might be the affinity of Idr for its substrate iodate but not the effectiveness of AgIZ. A detailed biochemical study of Idr is required to elucidate its kinetics (Km, Vmax, and kcat) toward iodate. Moreover, further study is needed to understand the effect of other competitive electron acceptors such as nitrate on iodate reduction by strain DN11. Adsorption of iodate to Fe- and Al-containing minerals (Yoshida et al., 1992) may also affect bacterial iodate reduction in terrestrial environments.
Azoarcus sp. DN11 was previously isolated from gasoline-contaminated groundwater in Kumamoto City, Japan (Kasai et al., 2006, 2007). The strain was routinely grown anaerobically at 30°C in the basal medium, as described previously (Yamazaki et al., 2020). One liter of the medium contained NH4Cl (0.54 g), KH2PO4 (0.14 g), MgCl2·6H2O (0.20 g), CaCl2·2H2O (0.15 g), Na2SO4 (0.14 g), casamino acids (0.10 g), NaHCO3 (2.5 g), a vitamin solution (1.0 mL), and a trace metal solution (1.0 mL). Acetate (2 mM) and iodate (2 mM) were added to the medium from the sterile anaerobic stock solutions. The medium (20 mL) was dispensed into a 60 mL serum bottle under an N2-CO2 (80:20) gas stream, and the bottle was sealed with a thick butyl rubber stopper and an aluminum cap. In some cases, nitrate (2 mM) or oxygen was used as the electron acceptor, as previously described (Yamazaki et al., 2020).
To prepare crude extracts, cells grown on iodate or nitrate for 48 h were collected, washed, and resuspended in 10 mM potassium phosphate buffer (pH 7.0). The cells were disrupted by sonication (Q500 Sonicator; Qsonica, Newtown, CT, United States), followed by centrifugation (17,000 × g, 30 min, 4°C) to remove cell debris, and the supernatant was used as the crude enzyme. As described previously, the reductase activity was assayed by a spectrophotometer (Yamazaki et al., 2020). The reaction mixture contained 20 mM Tris–HCl (pH 6.8), 0.3 mM MV (ε578 = 9.7 mM−1·cm−1), an appropriate amount of enzyme, and an electron acceptor (iodate or nitrate). After the reaction mixture was degassed and sparged with N2 gas, the reaction was initiated by adding sodium dithionite. One unit (U) of reductase activity was defined as the enzyme required to oxidize 1 μmol of reduced MV per minute. Protein concentration was determined using a protein assay kit (Bio-Rad, Hercules, CA, United States), with bovine serum albumin as a standard protein.
Electrophoresis was performed in two steps to separate the proteins included in the crude extracts. In the first step, non-denaturing gel electrophoresis was performed at 4°C using a 10% polyacrylamide gel in 25 mM Tris-glycine buffer (pH 8.3), omitting both reducing agents and SDS. Following non-denaturing electrophoresis, the gel was incubated in a nitrogen atmosphere with 20 mM Tris–HCl (pH 6.8) containing 0.3 mM MV, 12 mM iodate, and 10 mM dithionite. In the second step, the clear band (active band) that appeared on the gel was excised, boiled for complete denaturation (with 2% SDS and 5% 2-mercaptoethanol for 5 min), and then subjected to SDS-PAGE again. The Protein Molecular Weight Marker (Takara, Otsu, Japan) was used as the standard marker. Proteins were visualized by staining with CBB R-250, and the selected bands were excised, trypsin-digested, and subjected to LC–MS/MS analysis, as described previously (Shevchenko et al., 1996).
All manipulations were performed as previously described (Yamazaki et al., 2020). RNA was prepared from cultures under all growth conditions, i.e., on iodate, nitrate, or oxygen as the terminal electron acceptor. Cells in the late exponential growth phase were collected, and pellets were stored at −80°C. Total RNA was extracted using the RNeasy Miniprep kit (QIAGEN, Hilden, Germany), followed by treatment with a TURBO DNA-free kit (Ambion, Carlsbad, CA, USA) to remove residual DNA. cDNA was synthesized using a SuperScript First-Strand Synthesis System for RT-PCR (Invitrogen, Carlsbad, CA, USA). Quantifying idrA, idrP1, idrP2, and narG in the cDNA samples was performed using quantitative PCR (qPCR). The recA gene was quantified in the cDNA samples to normalize the qRT-PCR data. As shown in Supplementary Table S3, new primers were designed for qPCR analysis. SYBR green detection chemistry was used for qPCR assays using a Step One Plus instrument (Applied Biosystems). Standard curves were constructed using serial dilutions of cDNA prepared from cells grown on iodate. The slopes of the standard curves were used to calculate PCR efficiencies according to the following equation:
The E values obtained for all genes and primer pairs ranged from 90 to 110%, with R2 values greater than 0.98.
Strain DN11 was grown anaerobically in a basal medium containing 200 μM iodate as the electron acceptor. After 3 days of growth, 1 mL of the liquid culture was removed and mixed with 0.2 g of silver-impregnated zeolite (NE-AG/ZEO-KUR, Nuclear Engineering Co., Ltd., Ibaraki, Japan). After vigorous shaking, the mixture of liquid culture and zeolite was centrifuged (15,000 × g, 3 min, 4°C) to remove the cells and zeolite. Iodate and iodide in the supernatant were determined using ion chromatography (see below).
The concentrations of iodate, iodide, and acetate were determined using an ion chromatograph IC-2010 (Tosoh, Tokyo, Japan), as described previously (Yamazaki et al., 2020). The detection limits of iodate and iodide were 3 μM and 5 μM, respectively.
The datasets presented in this study can be found in online repositories. The names of the repository/repositories and accession number(s) can be found at: https://www.ncbi.nlm.nih.gov/genbank/, CP021731.1.
SA designed the research and acquired funding. SS and SA performed the experiments, wrote the draft manuscript, and created the figures and tables with guidance from TO and NK. All authors contributed to the data analysis, reviewed the manuscript, and approved the submitted version.
This study was financially supported by JSPS KAKENHI (grant number 20H02896).
SA would like to thank H. Nojiri (The University of Tokyo), C. Suzuki-Minakuchi (The University of Tokyo), and Y. Takahata (Taisei Corporation) for providing us with Azoarcus sp. DN11.
The authors declare that the research was conducted in the absence of any commercial or financial relationships that could be construed as a potential conflict of interest.
All claims expressed in this article are solely those of the authors and do not necessarily represent those of their affiliated organizations, or those of the publisher, the editors and the reviewers. Any product that may be evaluated in this article, or claim that may be made by its manufacturer, is not guaranteed or endorsed by the publisher.
The Supplementary material for this article can be found online at: https://www.frontiersin.org/articles/10.3389/fmicb.2023.1162788/full#supplementary-material
Amachi, S. (2008). Microbial contribution to global iodine cycling: volatilization, accumulation, reduction, oxidation, and sorption of iodine. Microbes Environ. 23, 269–276. doi: 10.1264/jsme2.ME08548
Amachi, S., Kamagata, Y., Kanagawa, T., and Muramatsu, Y. (2001). Bacteria mediate methylation of iodine in marine and terrestrial environments. Appl. Environ. Microbiol. 67, 2718–2722. doi: 10.1128/AEM.67.6.2718-2722.2001
Amachi, S., Muramatsu, Y., Akiyama, Y., Miyazaki, K., Yoshiki, S., Hanada, S., et al. (2005). Isolation of iodide-oxidizing bacteria from iodine-rich natural gas brines and seawaters. Microbial Ecol. 49, 547–557. doi: 10.1007/s00248-004-0056-0
Carpenter, L. J., Chance, R. J., Sherwen, T., Adams, T. J., Ball, S. M., Evans, M. J., et al. (2021). Marine iodine emissions in a changing world. Proc. R. Soc. A 477:20200824. doi: 10.1098/rspa.2020.0824
Chaker, L., Razvi, S., Bensenor, I. M., Azizi, F., Pearce, E. N., and Peeters, R. P. (2022). Hypothyroidism. Nat. Rev. Dis. Primers. 8:30. doi: 10.1038/s41572-022-00357-7
Dijck-Brouwer, D. A. J., Muskiet, F. A. J., Verheesen, R. H., Schaafsma, G., Schaafsma, A., and Geurts, J. M. W. (2022). Thyroidal and extrathyroidal requirements for iodine and selenium: a combined evolutionary and (patho)physiological approach. Nutrients 14:3886. doi: 10.3390/nu14193886
Fabryka-Martin, J., Bentley, H., Elmore, D., and Airey, P. L. (1985). Natural iodine-129 as an environmental tracer. Geochim. Cosmochim. Acta 49, 337–347. doi: 10.1016/0016-7037(85)90027-4
Fuge, R., and Johnson, C. C. (2015). Iodine and human health, the role of environmental geochemistry and diet, a review. Appl. Geochem. 63, 282–302. doi: 10.1016/j.apgeochem.2015.09.013
Kaplan, D. I., Denham, M. E., Zhang, S., Yeager, C., Xu, C., Schwehr, K. A., et al. (2014). Radioiodine biogeochemistry and prevalence in groundwater. Crit. Rev. Environ. Sci. Technol. 44, 2287–2335. doi: 10.1080/10643389.2013.828273
Kaplan, D. I., Price, K. A., Xu, C., Li, D., Lin, P., Xing, W., et al. (2019). Iodine speciation in a silver-amended cementitious system. Environ. Int. 126, 576–584. doi: 10.1016/j.envint.2019.02.070
Kasai, Y., Kodama, Y., Takahata, Y., Hoaki, T., and Watanabe, K. (2007). Degradative capacities and bioaugmentation potential of an anaerobic benzene-degrading bacterium strain DN11. Environ. Sci. Technol. 41, 6222–6227. doi: 10.1021/es062842p
Kasai, Y., Takahata, Y., Manefield, M., and Watanabe, K. (2006). RNA-based stable isotope probing and isolation of anaerobic benzene-degrading bacteria from gasoline-contaminated groundwater. Appl. Environ. Microbiol. 72, 3586–3592. doi: 10.1128/AEM.72.5.3586-3592.2006
Li, D., Kaplan, D. I., Price, K. A., Seaman, J. C., Roberts, K., Xu, C., et al. (2019). Iodine immobilization by silver-impregnated granular activated carbon in cementitious systems. J. Environ. Radioact. 208-209:106017. doi: 10.1016/j.jenvrad.2019.106017
Mok, J. K., Toporek, Y. J., Shin, H.-D., Lee, B. D., Lee, M. H., and DiChristina, T. J. (2018). Iodate reduction by Shewanella oneidensis does not involve nitrate reductase. Geomicrobiol J. 35, 570–579. doi: 10.1080/01490451.2018.1430189
Muramatsu, F., Tonomura, M., Yamada, M., Kasahara, Y., Yamamura, S., Iino, T., et al. (2020). Possible involvement of a tetrathionate reductase homolog in dissimilatory arsenate reduction by Anaeromyxobacter sp. PSR-1. Appl. Environ. Microbiol. 86:e00829-20. doi: 10.1128/AEM.00829-20
Neeway, J. J., Kaplan, D. I., Bagwell, C. E., Rockhold, M. L., Szecsody, J. E., Truex, M. J., et al. (2019). A review of the behavior of radioiodine in the subsurface at two DOE sites. Sci. Total Environ. 691, 466–475. doi: 10.1016/j.scitotenv.2019.07.146
O'Dowd, C. D., Jimenez, J. L., Bahreini, R., Flagan, R. C., Seinfeld, J. H., Hämeri, K., et al. (2002). Marine aerosol formation from biogenic iodine emissions. Nature 417, 632–636. doi: 10.1038/nature00775
Otosaka, S., Schwehr, K. A., Kaplan, D. I., Roberts, K. A., Zhang, S., Xu, C., et al. (2011). Factors controlling mobility of 127I and 129I species in an acidic groundwater plume at the Savannah River site. Sci. Total Environ. 409, 3857–3865. doi: 10.1016/j.scitotenv.2011.05.018
Reyes-Umana, V., Henning, Z., Lee, K., Barnum, T. P., and Coates, J. D. (2022a). Genetic and phylogenetic analysis of dissimilatory iodate-reducing bacteria identifies potential niches across theworld's oceans. ISME J. 16, 38–49. doi: 10.1038/s41396-021-01034-5
Reyes-Umana, V., Kretschmer, J., and Coates, J. D. (2022b). Isolation of a dissimilatory iodate-reducing Aromatoleum sp. from a freshwater creek in the San Francisco Bay Area. Front. Microbiol. 12:804181. doi: 10.3389/fmicb.2021.804181
Shevchenko, A., Wilm, M., Vorm, O., and Mann, M. (1996). Mass spectrometric sequencing of proteins from silver-stained polyacrylamide gels. Anal. Chem. 68, 850–858. doi: 10.1021/ac950914h
Sillen, L. G. (1961). “The physical chemistry of seawater” in Oceanography. ed. M. Sears (Washington, DC: American Association for the Advancement of Science)
Takahata, Y., Kasai, Y., Hoaki, T., and Watanabe, K. (2006). Rapid intrinsic biodegradation of benzene, toluene, and xylenes at the boundary of a gasoline-contaminated plume under natural attenuation. Appl. Microbiol. Biotechnol. 73, 713–722. doi: 10.1007/s00253-006-0522-3
Tsuchiya, T., Ehara, A., Kasahara, Y., and Amachi, S. (2019). Expression of genes and proteins involved in arsenic respiration and resistance in dissimilatory arsenate-reducing Geobacter sp. OR-1. Appl. Environ. Microbiol. 85:e00763-19. doi: 10.1128/AEM.00763-19
Yamazaki, C., Kashiwa, S., Horiuchi, A., Kasahara, Y., Yamamura, S., and Amachi, S. (2020). A novel dimethylsulfoxide reductase family of molybdoenzyme, Idr, is involved in iodate respiration by pseudomonas sp. SCT. Environ. Microbiol. 22, 2196–2212. doi: 10.1111/1462-2920.14988
Yeager, C. M., Amachi, S., Grandbois, R., Kaplan, D. I., Xu, C., Schwehr, K. A., et al. (2017). Microbial transformation of iodine: from radioisotopes to iodine deficiency. Adv. Appl. Microbiol. 101, 83–136. doi: 10.1016/bs.aambs.2017.07.002
Yoshida, S., Muramatsu, Y., and Uchida, S. (1992). Studies on the sorption of I− (iodide) and IO3− (iodate) onto andosols. Water Air Soil Pollut. 63, 321–329. doi: 10.1007/BF00475499
Keywords: iodate respiration, Azoarcus sp. DN11, IdrABP1P2, radioiodine, 129I
Citation: Sasamura S, Ohnuki T, Kozai N and Amachi S (2023) Iodate respiration by Azoarcus sp. DN11 and its potential use for removal of radioiodine from contaminated aquifers. Front. Microbiol. 14:1162788. doi: 10.3389/fmicb.2023.1162788
Received: 10 February 2023; Accepted: 22 March 2023;
Published: 17 April 2023.
Edited by:
Chris Yeager, Los Alamos National Laboratory (DOE), United StatesReviewed by:
Gabriela Alejandra Vázquez-Rodríguez, Autonomous University of the State of Hidalgo, MexicoCopyright © 2023 Sasamura, Ohnuki, Kozai and Amachi. This is an open-access article distributed under the terms of the Creative Commons Attribution License (CC BY). The use, distribution or reproduction in other forums is permitted, provided the original author(s) and the copyright owner(s) are credited and that the original publication in this journal is cited, in accordance with accepted academic practice. No use, distribution or reproduction is permitted which does not comply with these terms.
*Correspondence: Seigo Amachi, YW1hY2hpQGZhY3VsdHkuY2hpYmEtdS5qcA==
Disclaimer: All claims expressed in this article are solely those of the authors and do not necessarily represent those of their affiliated organizations, or those of the publisher, the editors and the reviewers. Any product that may be evaluated in this article or claim that may be made by its manufacturer is not guaranteed or endorsed by the publisher.
Research integrity at Frontiers
Learn more about the work of our research integrity team to safeguard the quality of each article we publish.