- 1State Key Laboratory of Mycology, Institute of Microbiology, Chinese Academy of Sciences, Beijing, China
- 2Ecological Environment Planning and Environmental Protection Technology Center of Qinghai Province, Xining, China
- 3Institute of New Rural Development, Guizhou University, Guiyang, China
- 4Faculty of Veterinary and Agricultural Sciences, The University of Melbourne, Parkville, VIC, Australia
- 5Key Laboratory of Land Surface Pattern and Simulation, Beijing Key Laboratory of Environmental Damage Assessment and Remediation, Institute of Geographic Sciences and Natural Resources Research, Chinese Academy of Sciences, Beijing, China
- 6Shaanxi Academy of Forestry, Xi'an, China
Screening high-tolerant microorganisms to cadmium (Cd) and revealing their bio-obstruction mechanism could be significant for Cd regulation from farmland to the food chain. We examined the tolerance and bio-removal efficiency of Cd ions of two bacterial strains, Pseudomonas putida 23483 and Bacillus sp. GY16, and measured the accumulation of Cd ions in rice tissues and its different chemical forms in soil. The results showed that the two strains had high tolerance to Cd, but the removal efficiency was decreased successively with increasing Cd concentrations (0.05 to 5 mg kg−1). Cell-sorption accounted for the major proportion of Cd removal compared with excreta binding in both strains, which was conformed to the pseudo-second-order kinetics. At the subcellular level, Cd was mostly taken up by the cell mantle and cell wall, and only a small amount entered into the cytomembrane and cytoplasmic with time progressed (0 to 24 h) in each concentration. The cell mantle and cell wall sorption decreased with increasing Cd concentration, especially in the cytomembrane and cytoplasmic. The scanning electron microscope (SEM) and energy dispersive X-ray (EDS) analysis verified that Cd ions were attached to the cell surface, and the functional groups of C-H, C-N, C=O, N-H, and O-H in the cell surface may participate in cell-sorption process tested by the FTIR analysis. Furthermore, inoculation of the two strains significantly decreased Cd accumulation in rice straw and grain but increased in the root, increased Cd enrichment ratio in root from soil, decreased Cd translocation ratio from root to straw and grain, and increased the Cd concentrations of Fe–Mn binding form and residual form in rhizosphere soil. This study highlights that the two strains mainly removed Cd ions in solution through biosorption and passivated soil Cd as Fe–Mn combined form ascribe to its characteristics of manganese-oxidizing, eventually achieving bio-obstruction of Cd from soil to rice grain.
1. Introduction
Cadmium (Cd) resulting from industrial and mining activities can bring health risks such as liver injury or osteoporosis through the food chain (Jin et al., 2020; Peng et al., 2021). Conventional physical and chemical techniques for Cd contamination remediation may bring secondary pollution to soil structure and organisms (Li et al., 2017; Mukherjee et al., 2022). For example, physicochemical techniques with specialized equipment are often accompanied by a broken of soil properties and structures, and bring secondary pollution to soil fauna and microorganisms in farmland (Beleza et al., 2001). Thus, eco-friendly techniques of bioremediation are urgently needed in field remediation to mitigate the Cd bioavailability, and consequently to reduce Cd accumulation in food. As a low-cost and environmentally friendly approach, micro-remediation was advocated in practice by using resistant and functional microorganisms (Alluri et al., 2007; Su et al., 2020). In polluted farmland, Cd could be immobilized by highly tolerant microorganisms through bioaccumulation and precipitation, via exogenous addition, which could help in reducing its accumulation in crops (Farhadian et al., 2008; Mengual et al., 2016; Peng et al., 2021). Therefore, screening for highly tolerant and efficient microorganisms in Cd immobilization could be essential for Cd regulation from farmland to the food chain.
For toxic heavy metals, physisorption (Pabst et al., 2010), complexation, and chelation of microbial cells (Shim et al., 2015) are the primary micro-actions. Therefore, the exchanging of positive ions such as Ca2+, Mg2+, and H+ on the cell surface and the combining ability of bio-macromolecules are responsible for the efficiency of microbial cell adsorption (Wu et al., 2009; Peraferrer et al., 2012; Rukshana et al., 2012), while the active groups such as -SO3H, -COOH, and -NH in the cell surface participate in chelation (Blanco, 2000; Peraferrer et al., 2012). In addition, inorganic deposition such as phosphorus salt, sulfate, carbonate, and hydroxide formed with Cd in cells (Özdemir et al., 2009; Jafar Mazumder, 2020), and complex precipitated compounds induced by microbial metabolites (Mutiat et al., 2018; Jafar Mazumder, 2020) are efficient microbial passivating forms to reduce the bioactivity of Cd. Furthermore, for many highly tolerant microorganisms, Cd could be fixed inside the cells without causing serious damage or pumped out after transformation depending on various transport systems (Shim et al., 2015; Li et al., 2019). However, bio-removal efficiency and mechanisms of Cd by microbial species remain unclear (Sulaymon et al., 2013; Yin et al., 2019).
In situ immobilization of Cd2+ by efficient microbes is considerable in practice because of less effect on agricultural planning (Shi et al., 2021). High-tolerant microbes could mediate the mobility and availability of Cd by releasing chelating agents, redox changes, and phosphate solubilization (Idris et al., 2004; Manoj et al., 2020). For example, resistant microbes participated in solid-solution partitioning processes of soil Cd via absorption–desorption by creating organic compounds such as organic acids, biosurfactants, and siderophores, as well as oxidation and reduction reactions (Braud et al., 2009; Arwidsson et al., 2010; Venkatesh and Vedaraman, 2012). Moreover, in natural soil, manganese (Mn) oxides have a high ability to adsorb Cd ions due to their large negative charge, and Cd could even enter into the structure of Mn oxide to be fixed (Muller et al., 2002). Nature Mn oxidation often proceeds slowly (Nealson et al., 1988), while the rate could be accelerated when microorganisms are involved, consequently increasing the heavy metal adsorption of Mn oxides (Kim et al., 2003; Meng et al., 2009). Some functional microbes such as Pseudomonas putida MnB1, Leptothrix discophora SS-1 (Caspi et al., 1996), and Bacillus sp. SG-1 (Van Waasbergen et al., 1996) are said to participate in the Mn oxidation process in various ways (Villalobos et al., 2003; Saratovsky et al., 2006) and to synthesize high-activity biogenic nano-sized Mn oxides (Kim et al., 2003). Those biogenic Mn oxides are assumed to have high adsorption efficiency and sensitive reaction with heavy metal ions compared with nature ones (Sasaki et al., 2008; Meng et al., 2009). A previous study showed that the Cd maximum adsorption ability of biogenic Mn oxides is significantly stronger than natural todorokite (Meng et al., 2009). Therefore, the interactions among Cd, biogenic Mn oxides, and Cd-tolerant microbes could be potentially highly efficient for bioremediators to adsorb, degrade, convert, and neutralize Cd as a bioremediation method in Cd-contaminated environments. However, there is seldom research on bioremediation efficiency and mechanism of Cd using Mn oxide microbes in the soil.
Rice (Oryza sativa) is one of the world's most important crops (Hu et al., 2016; Negrini et al., 2020) with the strongest ability of Cd enrichment and absorption (Suksabye et al., 2016; Negrini et al., 2020). “Cadmium rice” has become the main source of Cd intake for rice-eating people in East Asia such as China and Japan (Tsukahara et al., 2003; Honma et al., 2016). In this study, two bacterial strains, Pseudomonas putida 23483 and Bacillus sp. GY16 with functions of Mn oxidation (Meng et al., 2009), were used for revealing the biosorption mechanism of Cd, and the bio-obstruction of Cd accumulation on rice plants, and its bio-immobilization in soil. The bio-removal pattern and subcellular accumulation of Cd were investigated under a gradient concentration. The scanning electron microscope (SEM) and the energy dispersive X-ray (EDS) spectra were used to test microbial cell surface sorption of Cd, and the Fourier transform infrared (FTIR) spectra were used to verify the functional groups that participated in cell-sorption. Eventually, the Cd accumulation on rice plant tissues and changes in chemical forms of Cd in soil were measured to reveal the bio-obstruction efficiency of the two strains based on a pot control experiment. We hope to provide theoretical guidance for field application and improve the bioremediation efficiencies under in situ conditions.
2. Experimental section
2.1. Preparation of bacterial strains
Microbial strains, Pseudomonas putida 23483 (P. putida) and Bacillus sp. GY16, were selected as the target microbial species, purchased from China General Microbiological Culture Collection Center (CGMCC), and isolated from Fe–Mn nodules from Hubei Province, Central China, respectively (He et al., 2008). The strains were cultured in LB medium (tryptone 10 g, yeast extract 5 g, NaCl per liter, 5 g, pH = 7.0).
2.2. Tolerance test of the two strains
To test Cd tolerance of the two strains, the growth performance was determined in LB liquid medium under Cd concentration gradients of 0, 0.05, 0.5, 5, 10, 50, and 100 mg L−1. The strains were incubated at 28°C for 72 h in 150-ml Erlenmeyer flasks, at 180 rpm using a thermostat shaker. The absorbance value of the solution was measured at 600 nm at the time point of 0, 6, 12, 24, 36, 48, 60, and 72 h using a microplate reader (BioTek Synergy H1), and the relative growth was calculated by the ratio of absorbance in each time point to that in point 0 h.
2.3. Removal efficiency of the strains
Based on the Cd tolerance test, the biosorption experiment was arranged under Cd concentrations of 0.05, 0.5, and 5 mg L−1, and under this gradient, the strains were not cruelly restrained but grown well. First, the strains were cultured in the LB liquid medium for 24 h until the late exponential phase, and the cells were isolated after centrifugation at 6000 g for 10 min and then the adhered media were washed out with sterilized 0.05 M KNO3 solution (pH 7.0) three times. Second, we resuspended the cells in 0.05 M KNO3 solution (Huang et al., 2005) in three 150-ml triangular flasks with a biomass concentration of 8 × 109 cells ml−1, and adjusted Cd to three concentrations as 0.05, 0.5, and 5 mg L−1 by adding CdCl2 (Cd concentrations of 0.05, 0.5, and 5 mg L−1 in KNO3 solution without microbial cells as control). Third, the reaction solution of each concentration was sub-packaged into 2-ml tubes, with six replicates, and was placed in a thermostatted shaker at the conditions of 37°C, 180 rpm. We collected the solution at 0.5, 1, 1.5, 2.5, 3.5, 6, 12, and 24 h time nodes, the solution was centrifuged at 12,000 g for 5 min, and then, the supernatant was collected in clean tubes for Cd measurement (Microbial removal Cd). Finally, the leaving substrate precipitations were digested with 2 ml of concentrated nitric acid for 1 h (cell-sorption Cd) and analyzed for Cd concentrations by coupled plasma-mass spectrometry (ICP-MS). The excreta binding amount (excreta binding Cd) of the two microbial species = the amount of microbial removal Cd – the amount of cell-sorption of Cd.
2.4. Subcellular fractionation of the strains
The subcellular fractionation was isolated step by step according to the method of Kumar and Upretil (2000). First, the solution samples (six replicates) collected at each time node mentioned before were centrifuged for 5 min at 12,000 g, and the supernatant was discarded but the substrate precipitations were maintained. Then, the precipitations were washed three times with Tris buffer (1 mL, 0.03 mol L−1) containing 0.1 mol L−1 EDTA, adjusted pH to 8.0, and centrifuged three times at 12,000 g for 2 min. The centrifuged Tris buffer was collected together in clean tubes and the substrate precipitations were maintained. Second, the substrate precipitations were resuspended in the Tris buffer. We added lysozyme and adjusted it to 400 μg ml−1 with the precipitations, and incubated it at 25°C for 30 min to prepare spheroplasts. When finished, the spheroplasts were centrifuged at 2,576 g for 15 min under 0–4°C; the supernatant Tris buffer was a periplasmic fluid that consisted of a peptidoglycan layer and was obtained in clean tubes. The precipitations (spheroplasts) were resuspended in Tris buffer (pH = 8.1). Third, the spheroplasts were disrupted completely with the ultrasonic cell crusher by two 30 s bursts and then centrifuged at 12,000 g for 2 min to remove debris. The supernatants (cytoplasm) were collected into new test tubes, and the precipitations (crude membrane) consisting of both outer and inner membrane envelopes were maintained. Finally, the supernatants and the crude membrane in tubes were digested by concentrated nitric acid (2 ml) at 60°C for 1 h and analyzed for Cd concentrations by ICP-MS.
2.5. SEM, EDX, and FTIR spectra analysis
The strains were cultured in the LB medium at 28°C with Cd ions of 50 mg kg−1 and without Cd ions for 48 h. The medium was washed clearly with deionized water, and the microbial cells were resuspended and immobilized in 0.05 M KNO3 solution containing 2.5% glutaraldehyde for 4 h. When finished, the solution was centrifuged at 8,000 g for 5 min and maintained the precipitations, washed three times with 0.05 M KNO3 solution, and centrifuged at 8,000 g for 5 min. The precipitations were maintained and were ethanol gradient dehydrated in a sequence of 30, 50, 70, 85, and 90% once, and in 100% ethanol twice, 15 min per time, and centrifuged at 8,000 g for 5 min, and the supernatants were removed each time. The precipitations were immersed in a solution of isopentyl acetate twice to replace ethanol at 20 min per time. Then, the precipitations were gradiently frozen at −20°C (for 12 h), −40°C (for 12 h), and −80°C (for 24 h) step by step, and then freeze-dried at −40°C in a freeze drier for 24 h. Finally, the microbial cell powder was placed on SEM and EDS to observe the phenotypic changes and surface ion content, placed on an FTIR instrument (Bruker Tensor 27, Ettlingen, Germany) for in situ functional group analysis, and calculated the area and the height of the peaks using OPUS v5.5 software in FTIR analysis.
2.6. Greenhouse experiment arrangement
The soil was collected for rice plant cultivation from a Cd-contaminated area (E113°10′ 54″, N 27°30′ 45″), with a depth of 0–30 cm in Sima town, located in LiuYang county, Hunan Province. The air-dried soil was passed through a 2-mm sieve, mixed uniformly, and sterilized using ultraviolet. Soil total Cd concentration was 1.09 mg kg−1, available Cd concentration was 0.35 mg kg−1, cation exchange capacity was 12.16 cmol kg−1 (+), organic matter content was 50.44 g kg−1, the total carbon content was 2.6 %, the total nitrogen content was 0.3%, and the C and N ratio was 8.81. For pot experiments in the greenhouse, 3 kg of soil was poured into customized polyethylene plastic square pots with 40 cm height, 30 cm length, and 25 cm width. We arranged two treatments and six replicates for each treatment, including the bacterial inoculum of P. putida with 10 mM MnCl2 solution (P. putida) and the bacterial inoculum of Bacillus sp. GY16 with 10 mM MnCl2 solution (Bacillus sp. GY16). We also arranged soil with 10 mM MnCl2 solution (MnCl2) and without MnCl2 solution as control (control). The two bacterial strains were cultured in LB medium for 48 h and centrifuged to remove the adhered medium, washed, and dispersed in deionized water. Each single inoculum strain contains an amount of 5 × 108 colony-forming units ([cfu] ml−1). Afterward, rice seedlings (variety is Zhongjiazao 17) were germinated in sterilization vermiculite and transplanted into pots until grown to a 5 cm height, and one pot received two similar size seedlings. The pots with seedlings were placed in the greenhouse with 15–28°C and 16/8-h of light/dark period, and waterlogging growth was kept for 3 months.
After maturity, watering was stopped and lasted for 1 week, plants were harvested, and soil samples were collected. Roots, straw, and grain were separately oven-dried at 65°C for 72 h, and the biomass was weighed. According to Tessier et al. (1979) and Gleyzes et al. (2002), the fractional extraction method was adopted to extract and separate different forms of Cd in soil samples with a soil–water ratio of 1:2.5 (W/V), including exchangeable Cd (extracted by 1 M MgCl2, pH = 7.0), carbonate binding Cd (extracted by 1 M CH3COONa, pH = 5.0), iron manganese oxide binding Cd (extracted by 0.04 M NH2OH-HCl and 25% CH3COOH), organic binding Cd (extracted by 0.02 M HNO3 and 30% H2O2, 85 ± 3°C), and residue Cd (digested by HF-HClO4). The plant materials were ground into powder and digested with HNO3-HClO4 for Cd extraction (Wang et al., 2017). Eventually, the earlier processed samples were analyzed by ICP-MS for Cd concentrations in soil and plant tissues. We also calculated the translocation ratio and the enrichment ratio of Cd to trace that from soil to plant tissues. Taking the trace of soil Cd to plant root, for example, the translocation ratio was calculated by Cd accumulation in root/Cd accumulation in soil, while the enrichment ratio was calculated by Cd accumulation (in root–in soil)/soil Cd in control pots (Jankong and Visoottiviseth, 2008; Chen et al., 2016).
2.7. Statistical analysis
The statistical analyses were performed in R version 4.1.3. A one-way ANOVA was used to explore the difference in biosorption capacities among different treatments, and significant differences among treatments were compared using Tukey's honestly significant difference (HSD) test at p < 0.05, after the log transformation as the data did not satisfy the normality of distribution or homogeneity of variance.
3. Results and discussion
3.1. Cd tolerance and removal efficiency
Low concentration (0.05–0.5 mg kg−1) of Cd was not restrained even promoted microbial growth, and the two strains showed high tolerance to Cd in medium concentrations (5–10 mg kg−1), whereas high concentrations (50–100 mg kg−1) significantly inhabited the microbial growth (Figures 1A, B). This suggested that the two strains could be Cd-resistant microbes and good candidates for bioremediation, because the concentration of soil total Cd is 1.09 mg kg−1 and the available concentration is 0.35 mg kg−1 in nature farmland in our study sites. Therefore, we performed a biosorption experiment under the concentrations of 0.05, 0.5, and 5 mg kg−1, which, respectively, represented low, medium, and high levels of Cd stress. In line with the previous studies (Bai et al., 2008; Panwichian et al., 2010), we found that the removal efficiency was decreased successively with increasing Cd concentration in each strain (Figures 1C, D). This may be because of lacking sufficient-free sites on the cell surface to combine for more Cd ions (Foo and Hameed, 2010; Chakravarty and Banerjee, 2012), and the increased cell toxicity due to increased Cd concentration (Shafqat et al., 2008; Yu and Fein, 2015). Furthermore, the removal efficiency differs in P. putida (79.5–6.9%) and Bacillus sp. GY16 (90.3–18.8%), depending on different cellular structures and functions of different microbes (Guo et al., 2010; Shim et al., 2015). We also found that the cell-sorption responsible for Cd removed more than excreta binding in both strains (Figures 1C, D), suggesting the importance of cell wall structures and chemical composition in bio-adsorption (Peraferrer et al., 2012; Rukshana et al., 2012), and the cell-sorption pattern was conformed to the pseudo-second-order kinetics (Table 1). The cell-sorption efficiency increased rapidly in the first 30 min, which may result from rapid binding via electrostatic adsorption of metal ligands or via complex action of various polar functional groups that existed on the cell wall surface, rather than internalized in the microbial cells (Wu et al., 2009; Ali et al., 2022). After 30/60 min, it reached a steady state for the maximum capacity of biosorption. This may be ascribed to the active transport process of Cd accompanied by energy consumption through microbial metabolism, which was slower than cell wall binding (Bai et al., 2008). It should be pointed out that the experiment was performed in simplex KNO3 solution without supplementary nutrients, the microbial secretions were relatively limited, and their reactions of ions or compounds to immobilize Cd was lacking. Therefore, compared with soil conditions, the Cd removal efficiency by microbial-secreted extracellular precipitation may be somehow diminished (Achal et al., 2009).
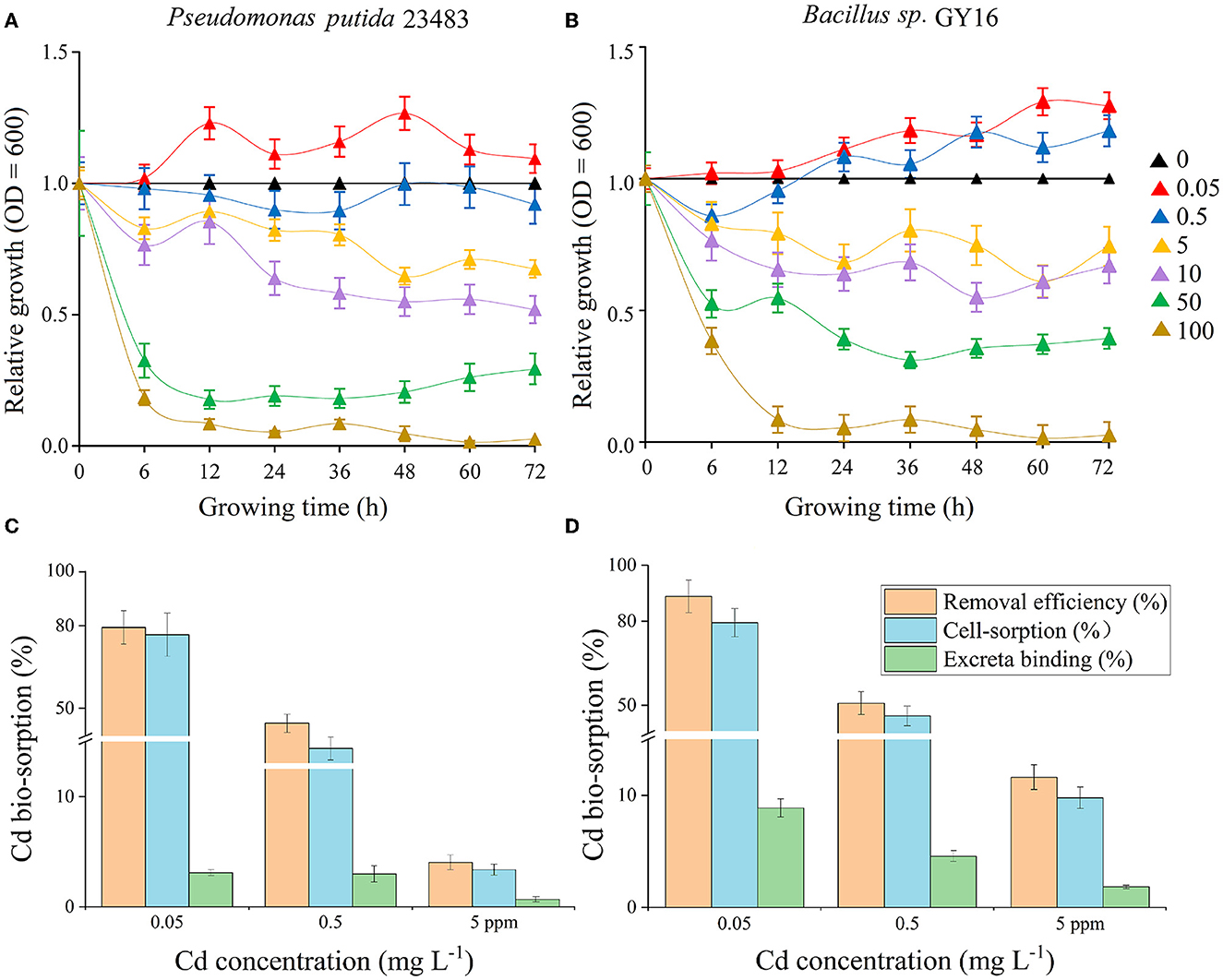
Figure 1. (A, B) Tolerance test of Cd concentrations of 0, 0.05, 0.5, 5, 10, 50, and 100 mg L−1 on the growth of microbial strains Pseudomonas putida 23483 (A) and Bacillus sp. GY16 (B). The relative growth indicates the growth efficiency in the presence of Cd of different concentrations (0.05–100 mg L−1) compared with the growth in the medium without Cd (0 mg L−1). (C, D) The removal efficiency, cell-sorption, and excreta binding of Cd during the adsorption equilibrium phase (24 h) by Pseudomonas putida 23483 (C) and Bacillus sp. GY16 (D) under different Cd concentrations (0.05, 0.5, and 5 mg L−1).
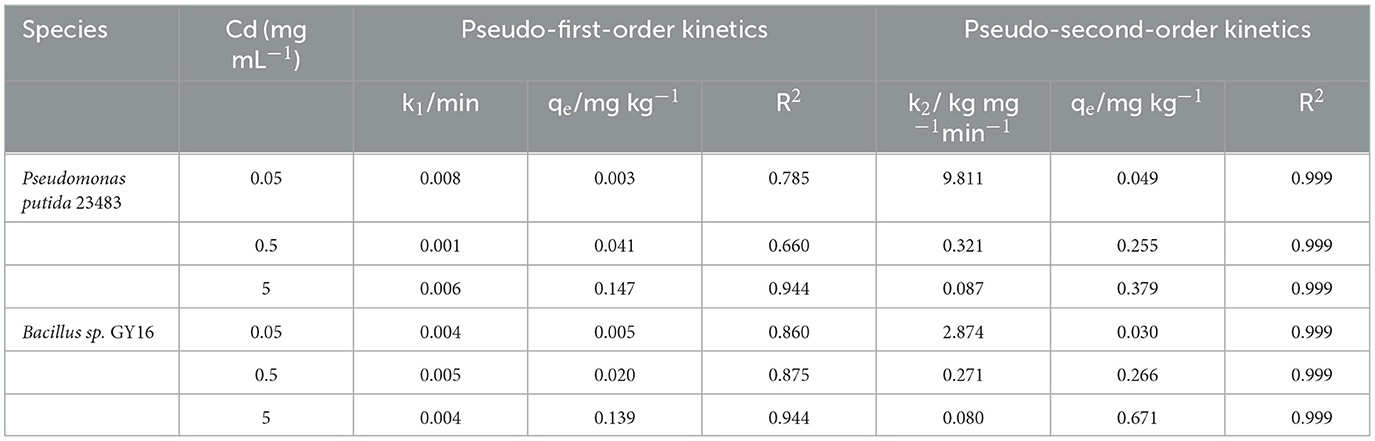
Table 1. Biosorption kinetic models for Cd of Pseudomonas putida 23483 and Bacillus sp. GY16 under different concentrations of Cd ions.
3.2. Subcellular sorption of Cd by the strains
We measured Cd concentration in different subcellular fractionations including cell mantle, cell wall, cell cytomembrane, and cytoplasm to ascertain the sorption mechanism of microbial cells. The results showed that Cd accumulation on subcellular fractionations was decreased with increasing concentration (Figure 2). Cd was mainly taken up by microbial cell mantle with 55.2–45.1% (Cd = 0.05 mg mL−1), 48.8–40.7% (Cd = 0.5 mg mL−1), and 3.7–2.4% (Cd = 5 mg mL−1) in P. putida (Figures 2A–C) and 53.6–45.2% (Cd = 0.05 mg mL−1), 42.1–33.6% (Cd = 0.5 mg mL−1), and 6.3–5.2% (Cd = 5 mg mL−1) in Bacillus sp. GY16 at different concentrations within 24 h (Figures 2D–F). This was in line with previous studies which suggested that most of the Cd was taken up by a cell wall (Kumar and Upretil, 2000; Bai et al., 2008; Ali et al., 2022). Furthermore, the Cd resistance and fixation patterns were adjusted by microorganisms in different Cd stress degrees. For example, at low concentration (0.05 mg L−1), Cd was mainly allocated in the cell wall system but barely entered into the cytomembrane (< 3%) and cytoplasmic (< 1%), but less allocated even in the cell wall (< 8%) at the higher concentrations of 0.5 and 5 mg L−1. This may be a self-protected mechanism to avoid high concentrations of Cd ions from entering cells and poisoning microbial membrane systems (Zouboulis et al., 2004; Yin et al., 2019).
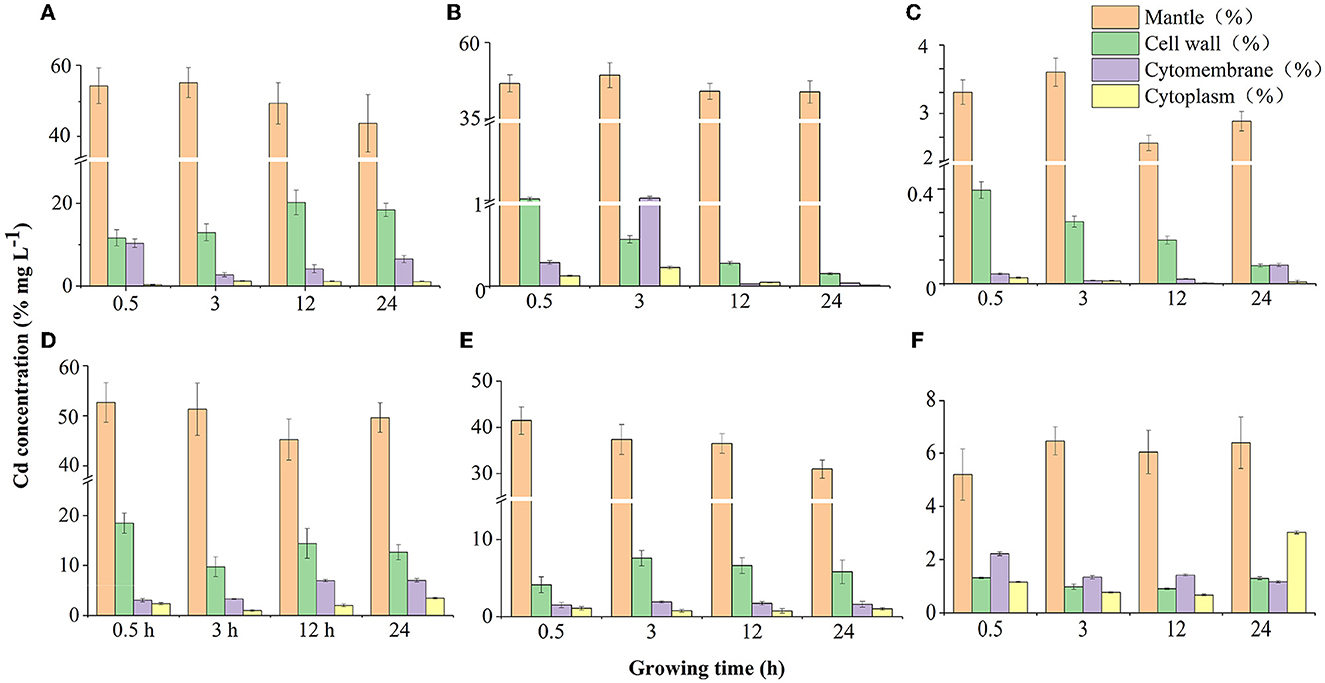
Figure 2. Cd distribution on substructures (cell mantle, cell wall, cytomembrane, and cytoplasm) of two microbial strains in different Cd concentrations during sorption time (0.5, 3, 12, and 24 h). (A–C) Cd sorption by Pseudomonas putida 23483 in concentrations of 0.05, 0.5, and 5 mg L−1, respectively. (D–F) Cd sorption by Bacillus sp.GY16 in concentrations of 0.05, 0.5, and 5 mg L−1, respectively.
Moreover, Cd in microbial subcellular fractionations fluctuated as time progressed (Figure 2). At the beginning, Cd ions adhered to the cell mantle ascribed to the cell-intrinsic surface properties, a few were transmitted to cytomembrane cross-inner cell wall with contact time passed, and some even entered into and were stored in cytoplasmic of the cells under low concentration (0.05 mg mL−1, Figure 2A). This may be an active transportation accompanied by water/nutrient uptake processes for barely poisoning in low Cd concentration (Marques et al., 2009), and depended on different import mechanisms of sorption, diffusion, complexation, chelation, and micro-precipitation (Franke et al., 2003; Srivastava and Gupta, 2021; Verma et al., 2021). In medium (0.5 mg kg−1) and high (5 mg L−1) concentrations, as contact time progresses, Cd ions in the inner cell wall first access into cytomembrane, resulting in an increase of Cd accumulated in the cytomembrane (earlier 0.5–3 h). Then, Cd ions in the cytomembrane and cytoplasmic were flowed out and nearly without retention. However, the cell mantle kept the most amount of Cd during the contact time for P. putida (Figures 2B, C). The export mechanisms such as CDF race efflux system (Blanco, 2000), CBA, and P type-ATP enzyme (Leedjärv et al., 2008) may play the leading role in this process (Perron et al., 2004; Srivastava and Gupta, 2021; Verma et al., 2021), yet the precise mechanism remains to be further studied. In comparison, Cd ions were stably stocked in the cell mantle, cell wall, cytomembrane, and cytoplasmic of Bacillus sp. GY16 with an extension of the contact time in the medium and high concentration (Figures 2E, F), confirmed its high Cd tolerance and import mechanisms (Franke et al., 2003). Therefore, our study confirmed that Cd was not only adsorbed in microbial cell surface (Pabst et al., 2010) but also inside the cells (Shim et al., 2015) via two biochemical stages including microbial biosorption and microbial bioaccumulation (Sprocati et al., 2006; Verma et al., 2021).
3.3. SEM, EDX, and FTIR spectra analysis
The SEM image and EDS analysis confirmed that Cd ions were attached to the cell surface (Figures 3A–D). The cell surface was smooth and the microbial cells were plumped without Cd stress (control) (Figures 3A, C), which was rough, atrophic, and with shiny bulky particles facing the stress of Cd of 50 mg L−1 (Figures 3B, D). This suggested that the damage to the cells and surface-active components were caused by Cd ions. The Cd ions exhibited in microbial cells (exposed to 50 mg L−1 of Cd) proved by the EDS atlas also supported the cell-sorption (Figure 3). The FTIR analysis showed changes in absorption peak areas of the cells exposed to 50 mg L−1 of Cd compared with that without Cd (Figures 3E, F), suggesting specific functional groups in the cell surface participated in cell-sorption (Pabst et al., 2010). For P. putida, the functional groups of C-H (CH3 symmetrical angle changed in 1371 cm−1, CH2 asymmetrical stretching in 2960 cm−1, and vibration as a move-left of 3423 to 3287 cm−1), C=O (such as stretching in 1239 and 2960 cm−1), N-H (such as stretching in 1540 cm−1, move-left of 3423 to 3287 cm−1), and O-H (stretching in 1239 and 3423 cm−1) (Zamil et al., 2008; Özdemir et al., 2009) were involved in biosorption of Cd (Figure 3E). For Bacillus sp. GY16, the functional groups of C-N, C-O, O-H (vibration as move-left in 1245 to 1230 cm−1, stretching in 1059 cm−1), C-H (vibration as move-left of protein amide I in 1400 to CH3 in 1375 cm−1, stretching in 3287 cm−1), and C=O (peaks in 1722 cm−1) (Figure 3F) were involved in biosorption of Cd (Zamil et al., 2008; Özdemir et al., 2009). Therefore, the number and type of these functional groups may determine the capacity and efficiency of biosorption (Figure 3), which could help reveal the chemical mechanism of bio-sorption of microorganisms.
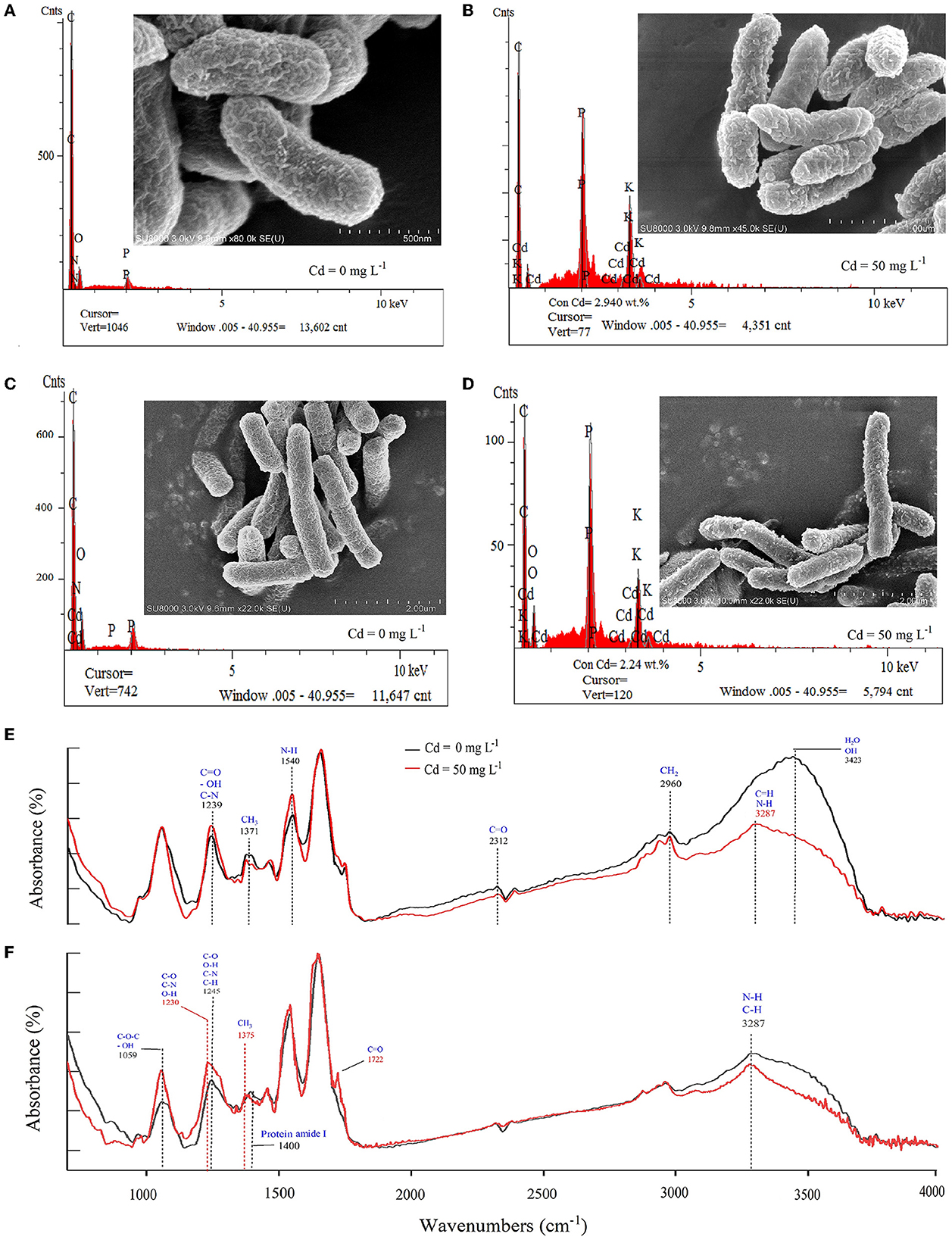
Figure 3. (A–D) Appearance and Cd elements on the cell surface of the two bacterial strains using SEM combined with the EDS analysis. (A) Pseudomonas putida 23483 without Cd solution. (B) Pseudomonas putida 23483 exposure to Cd solution (50 mg L−1) for 48 h. (C) Bacillus sp. GY16 without Cd solution. (D) Bacillus sp. GY16 exposure to Cd solution (50 mg L−1) for 48 h. (E, F) Functional groups on the cell surface of the microbial strains using the FTIR analysis. (E) Pseudomonas putida 23483. (F) Bacillus sp. GY16. The red lines represent strains without Cd solution, and the black lines represent strains exposed to Cd solution (50 mg L−1) for 48 h.
3.4. Cd accumulation in rice plants and the chemical forms in soil affected by the strains
The root, straw, and grain biomass were neither affected (F= 15.856, p = 0.231) by the inoculation of P. putida and Bacillus sp. GY16 nor by the addition of MnCl2 (Figure 4A). This suggested that P. putida and Bacillus sp. GY16 accompanied by MnCl2 did not influence the growth and production of rice plants. Therefore, we suggested that the two Cd-resistant strains have no exceptional ability to promote the growth of the host plant in this concentration, not like other reported plant growth–promoting bacteria such as Bradyrhizobium sp. 750 (Dary et al., 2010), Bacillus megaterium H3, and Neorhizobium huautlense T1-17 (Li et al., 2017). Gratefully, Cd concentrations significantly decreased in rice straw and grain when inoculated with P. putida (p = 0.033, 0.014) and Bacillus sp. GY16 (p = 0.019, 0.030; Figure 4B), although Cd concentrations increased in roots when inoculated with P. putida (p = 0.016) and Bacillus sp. GY16 (p = 0.021; Figure 4B). This suggested microbial obstruction effect for Cd migration from soil to aboveground tissues. The reduced Cd uptake of rice in aboveground tissue may ascribe to IAA, siderophores, and ACC deaminase produced by bacteria evidenced by previous studies (Babu et al., 2015; Chen et al., 2016).
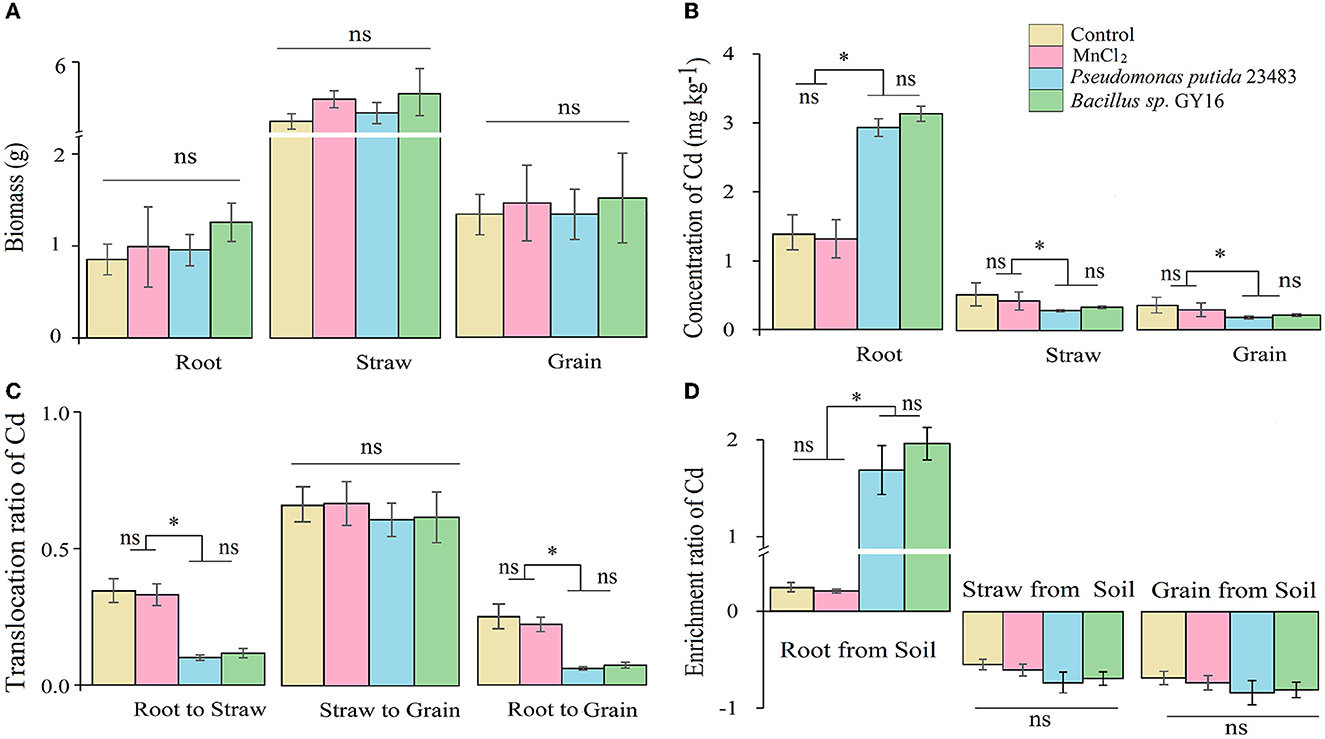
Figure 4. Biomass (A), concentrations of Cd in root, straw, and grain (B), the transmission ratio of Cd from root to straw and grain, (C) and enrichment ratio (D) without or with inoculation of microbial strains Pseudomonas putida 23483 and Bacillus sp. GY16. Bars with an asterisk (*) indicate significant differences among treatments determined by Tukey's HSD test at P < 0.05, and “ns” means not significant.
To reveal the effect of the two strains on the Cd accumulation in rice plants, we calculated the translocation ratio and the enrichment ratio along with the transport route of Cd in rice plants, such as from root to straw and to grain. The results showed that the enrichment ratio in root from soil (uptake ability) was significantly increased, whereas the translocation ratio of Cd from root to straw was significantly decreased when inoculated with P. putida (p = 0.018, 0.020) and Bacillus sp. GY16 (p = 0.011, 0.009) compared with the control (Figures 4C, D). This may be because microbes in the rhizosphere can develop a thick mucus barrier on the root tip and bind Cd ions inside microbial cell walls or on the root surface to limit its migration from root to plant aboveground tissues (Pál et al., 2006). As a result, the translocation ratio of Cd from root to grain was decreased when inoculated with P. putida (p = 0.034) and Bacillus sp. GY16 (p = 0.042) (Figure 4C), and the translocation ratio of Cd from rice straw to grain was not significantly different among the groups because of root binding (Figure 4C). This suggested that the inoculation of P. putida and Bacillus sp. GY16 allows soil Cd ions to mainly enrich and fix in plant roots and reduce their translocation in straw and grain from the root. Therefore, the microbial inhibition of Cd in rice mainly occurred in the root and was achieved by preventing Cd transmission and enrichment in the straw and grain from the soil.
Furthermore, the immobilization of Cd in soil by functional microbes is crucial to reduce its uptake by plants (Kuffner et al., 2010; Dourado et al., 2013; Ahmad et al., 2014). The transformation of chemical forms of soil Cd could directly affect its accumulation in rice plants because only the exchangeable Cd in the soil can be uptaken by plants. Our results showed that the external addition of MnCl2 of this amount in soil did not affect the chemical forms of Cd, but the concentration of soil exchangeable Cd was significantly decreased when inoculated with P. putida (p = 0.007) and Bacillus sp. GY16 (p = 0.004) accompanied by MnCl2 addition (Figure 5). This was consistent with a previous study that suggested that resistant bacteria reduced Cd accumulation in grains of rice by reducing the available Cd in the soil (Lin et al., 2016). Notably, the inoculation of P. putida and Bacillus sp. GY16 accompanied by MnCl2 significantly increased the Cd concentrations of the Fe–Mn binding form (pP.putida = 0.018; pGY16 = 0.023) and the residual form (pP.putida = 0.034; pGY16 = 0.028) compared with the control (Figure 5). This suggested that the strains play important roles in preventing soil Cd from converting to a soluble form but existing as combined forms such as iron–manganese oxide binding form and residue form, ascribed to its characteristics of manganese-oxidizing (He et al., 2008). It has been demonstrated that microorganisms can speed up the rate of Mn oxidization up to 105 times faster than abiotic Mn oxidization. We inoculated the strains accompanied by MnCl2 in soil because the process of Mn bio-oxidization needs a supplement of active Mn2+, but most of the Mn2+ in the soil exists in the form of compounds (Kim et al., 2003; Tebo et al., 2004). However, the concentrations of carbonate binding and organic binding forms of Cd were not significantly different among treatments (Figure 5). Nevertheless, the interaction mechanism between Cd-contaminated soil, rice plants, and the functional microbes needs further study.
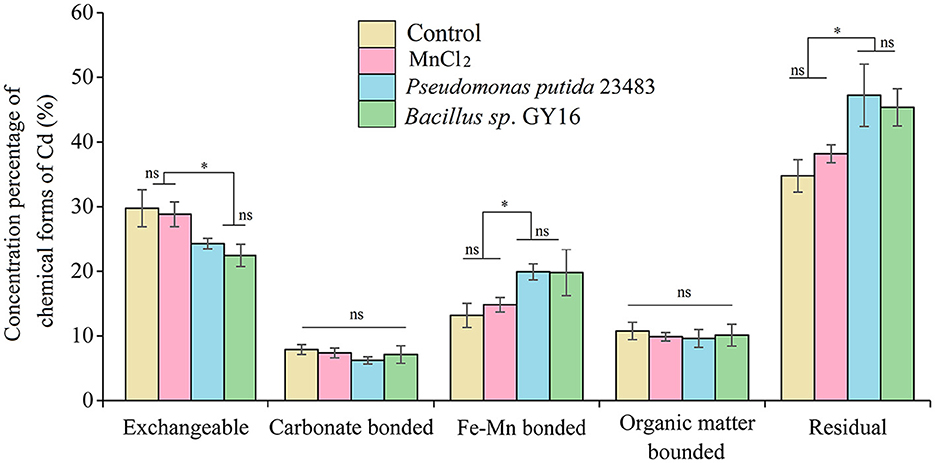
Figure 5. Fractionation of Cd in soil without or with inoculation of microbial strains Pseudomonas putida 23483 and Bacillus sp. GY16. Bars with an asterisk (*) indicate significant differences among treatments determined by Tukey's HSD test at P < 0.05, and “ns” means not significant.
4. Conclusion
Our study revealed that Cd ions could be efficiently removed by P. putida and Bacillus sp. GY16 through biosorption of the cell wall. The two strains do not significantly affect the rice plant growth and production but have microbial obstruction effect on Cd enrichment from soil to grain via root binding of bioactive Cd and soil passivation as Fe–Mn compound and residual combination forms (Figure 6). Therefore, we advocate the application of those high Cd-resistant strains for Cd pollution remediation in soil or water as an efficient and environmentally friendly approach and to prove the bioremediation consequence in field conditions. Furthermore, we advocate some microbial composite eco-materials such as biological Mn oxide to be fabricated to improve the biosorption efficiency in complicated soil or water conditions.
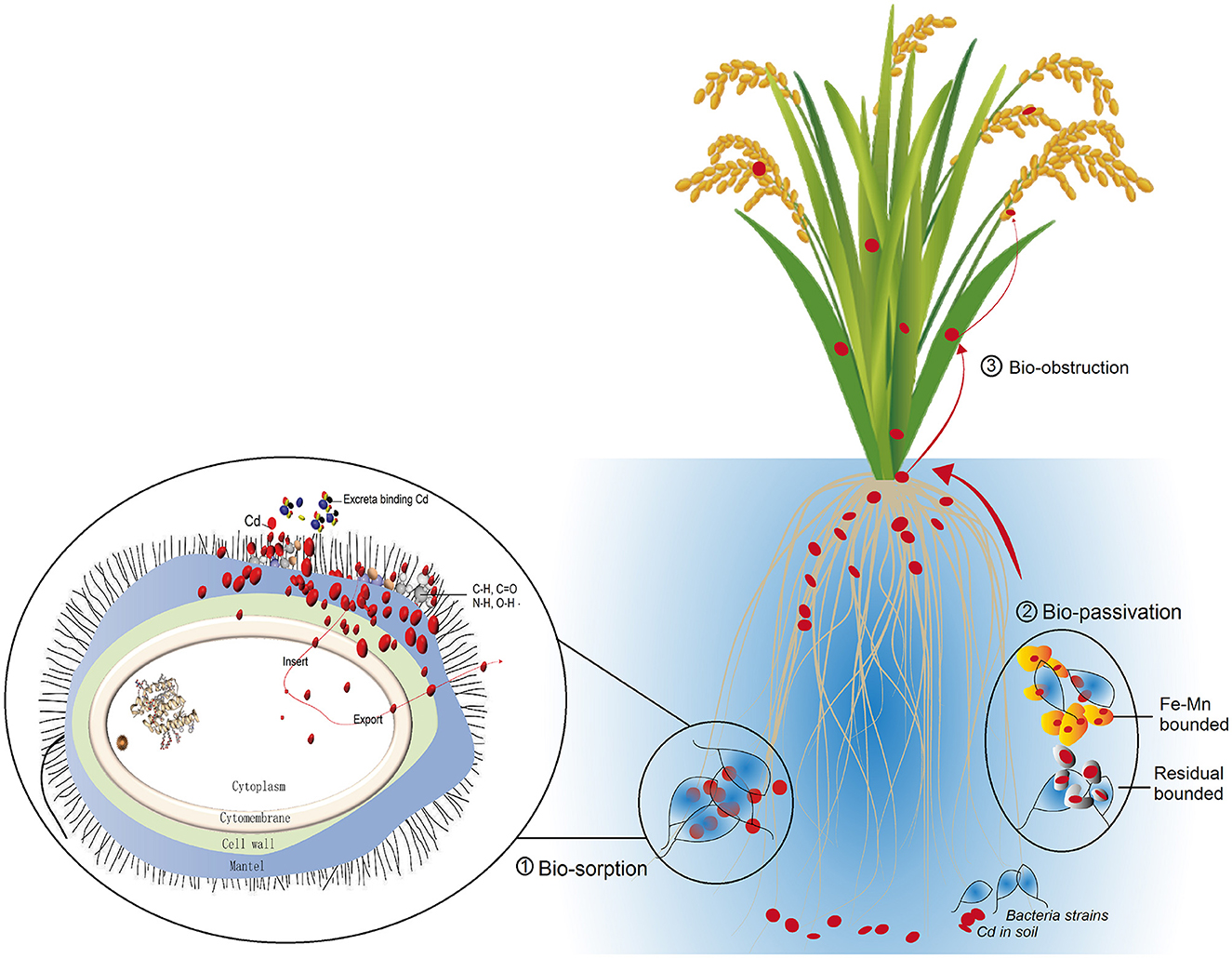
Figure 6. Conceptual graph summarizes the Cd dispose mechanism of the two microbial strains, including biosorption mainly via cell mantel and cell wall (Bio-sorption), bio-passivation of soil Cd as Fe–Mn combined form (Bio-passivation), and the bio-obstruction (Bio-obstruction) achieved by transmission from root to straw and grains.
4.1. Environmental implication
The two high-tolerant microbial strains can efficiently remove Cd ions and play an important role in preventing soil Cd from converting to bioactive form but passivating to Fe–Mn combined form, eventually decreasing the accumulation of Cd on rice grain, and achieving bio-obstruction of Cd from soil to rice grain. We advocate that those high Cd-resistant strains be used in soil or water remediation of Cd pollution in an efficient and eco-friendly way, and some microbial composite eco-materials such as biological Mn oxide be fabricated to improve the biosorption efficiency in complicated soil or water conditions.
Data availability statement
The original contributions presented in the study are included in the article/supplementary material.
Author contributions
JL, H-YC, and J-ZH conceived and designed the experiments. JL, Y-KG, and C-QL performed the experiments, analyzed the data, prepared figures and/or tables, and wrote the manuscript. QZ helped to determine the samples. All authors approved the final draft.
Funding
This study was supported financially by the Strategic Priority Research Program of the Chinese Academy of Sciences (XDA23010400), the Forestry Science and Technology Innovation Project of Shaanxi Province (SXLK2021-0101), and the National Key Research and Development Plan (No. 2017YFD0801502).
Conflict of interest
The authors declare that the research was conducted in the absence of any commercial or financial relationships that could be construed as a potential conflict of interest.
Publisher's note
All claims expressed in this article are solely those of the authors and do not necessarily represent those of their affiliated organizations, or those of the publisher, the editors and the reviewers. Any product that may be evaluated in this article, or claim that may be made by its manufacturer, is not guaranteed or endorsed by the publisher.
References
Achal, V., Mukherjee, A., Basu, P. C., and Reddy, M. S. (2009). Strain improvement of Sporosarcina pasteurii for enhanced urease and calcite production. J. Ind. Microbiol. Biotechnol. 36, 981–988. doi: 10.1007/s10295-009-0578-z
Ahmad, I., Akhtar, M. J., Zahir, Z. A., Naveed, M., Mitter, B., Sessitsch, A., et al. (2014). Cadmium-tolerant bacteria induce metal stress tolerance in cereals. Environ. Sci. Pollut. Res. 21, 11054–11065. doi: 10.1007/s11356-014-3010-9
Ali, Q., Ayaz, M., Yu, C., Wang, Y., Gu, Q., Wu, H., et al. (2022). Cadmium tolerant microbial strains possess different mechanisms for cadmium biosorption and immobilization in rice seedlings. Chemosphere 303, 135206. doi: 10.1016/j.chemosphere.2022.135206
Alluri, H. K., Ronda, S. R., Settalluri, V. S., Singh, J., Bondili, S. V., Venkateshwar, P., et al. (2007). Biosorption: an eco-friendly alternative for heavy metal removal. Afr J Biotechno l 6, 2924–2931. doi: 10.5897/AJB2007.000-2461
Arwidsson, Z., Johansson, E., von Kronhelm, T., Allard, B., and van Hees, P. (2010). Remediation of metal contaminated soil by organic metabolites from fungi I - production of organic acids. Water Air Soil Pollut. 205, 215–226. doi: 10.1007/s11270-009-0067-z
Babu, A. G., Shea, P. J., Sudhakar, D., Jung, I. B., and Oh, B. T. (2015). Potential use of Pseudomonas koreensis AGB-1 in association with Miscanthus sinensis to remediate heavy metal(loid)-contaminated mining site soil. J. Environ. Manag. 151, 160–166. doi: 10.1016/j.jenvman.2014.12.045
Bai, H. J., Zhang, Z. M., Yang, G. E., and Li, B. Z. (2008). Bioremediation of cadmium by growing Rhodobacter sphaeroides: kinetic characteristic and mechanism studies. Bioresour. Technol. 99, 7716–7722. doi: 10.1016/j.biortech.2008.01.071
Beleza, V. M., Boaventura, R. A., and Almeida, M. F. (2001). Kinetics of chromium removal from spent tanning liquors using acetylene production sludge. Environ. Sci. Technol. 35, 4383. doi: 10.1021/es0108786
Blanco, A. (2000). Immobilization of Nonviable Cyanobacteria and Their Use for Heavy Metal Adsorption From Water in Environmental Biotechnology and Cleaner Bioprocesses. Philadelphia, PA: Taylor and Francis, 135.
Braud, A., Jézéquel, K., Vieille, E., Tritter, A., and Lebeau, T. (2009). Changes in extractability of Cr and Pb in a polycontaminated soil after bioaugmentation with microbial producers of biosurfactants, organic acids and siderophores. Water Air. Soil. Pollut. 6, 261–279. doi: 10.1007/s11267-005-9022-1
Caspi, R., Haygood, M. G., and Tebo, B. M. (1996). Unusual ribulose-1,5-bisphosphate carboxylase/oxygenase genes from a marine manganese-oxidizing bacterium. Microbiology 142, 2549–2559. doi: 10.1099/00221287-142-9-2549
Chakravarty, R., and Banerjee, P. C. (2012). Mechanism of cadmium binding on the cell wall of an acidophilic bacterium. Bioresour. Technol. 108, 176–183. doi: 10.1016/j.biortech.2011.12.100
Chen, L., He, L. Y., Wang, Q., and Sheng, X. F. (2016). Synergistic effects of plant growth promoting Neorhizobium huautlense T1−17 and immobilizers on the growth and heavy metal accumulation of edible tissues of hot pepper. J. Hazard. Mat. 312, 123–131. doi: 10.1016/j.jhazmat.2016.03.042
Dary, M., Chamber-Perez, M., Palomares, A., and Pajuelo, E. (2010). In situ phytostabilisation of heavy metal polluted soils using Lupinus luteus inoculated with metal resistant plant-growth promoting rhizobacteria. J. Hazard. Mater 177, 323–330. doi: 10.1016/j.jhazmat.2009.12.035
Dourado, M. N., Martins, P. F., Quecine, M. C., Piotto, F. A., Souza, L. A., Franco, M. R., et al. (2013). Burkholderia sp SCMS54 reduces cadmium toxicity and promotes growth in tomato. Ann. Appl. Biol. 163, 494–507. doi: 10.1111/aab.12066
Farhadian, M., Vachelard, C., Duchez, D., and Larroche, C. (2008). In situ bioremediation of monoaromatic pollutants in groundwater: a review. Bioresour. Technol. 99, 5296–5308. doi: 10.1016/j.biortech.2007.10.025
Foo, K., and Hameed, B. (2010). Insights into the modeling of adsorption isotherm systems. Chem. Eng. J. 156, 2–10. doi: 10.1016/j.cej.2009.09.013
Franke, S., Grass, G., Rensing, C., and Nies, D. H. (2003). Molecular analysis of the copper transporting efflux system Cus CFBA of Escherichia coli. J. Bacteriol. 185, 3804–3812. doi: 10.1128/JB.185.13.3804-3812.2003
Gleyzes, C., Tellier, S., and Astruc, M. (2002). Fractionation studies of trace elements in contaminated soils and sediments: a review of sequential extraction procedures. Trend. Anal. Chem. 21, 451–467. doi: 10.1016/S0165-9936(02)00603-9
Guo, H., Luo, S., Chen, L., Xiao, X., Xi, Q., Wei, W., et al. (2010). Bioremediation of heavy metals by growing hyperaccumulaor endophytic bacterium Bacillus sp L14. Bioresour. Technol. 101, 8599–8605. doi: 10.1016/j.biortech.2010.06.085
He, J. Z., Zhang, L. M., Jin, S. S., Zhu, Y. G., and Liu, F. (2008). Bacterial communities inside and surrounding soil iron–manganese nodules. Geomicrobiol. J. 25, 14–24. doi: 10.1080/01490450701829014
Honma, T., Ohba, H., and Kaneko-Kadokura, A. (2016). Optimal soil Eh, pH, and water management for simultaneously minimizing arsenic and cadmium concentrations in rice grains. Environ. Sci. Tech. 50, 4178–4185. doi: 10.1021/acs.est.5b05424
Hu, Y. N., Cheng, H. F., and Tao, S. (2016). The challenges and solutions for cadmium-contaminated rice in China: a critical review. Environ. Int. 93, 515–532. doi: 10.1016/j.envint.2016.04.042
Huang, Q., Chen, W., and Xu, L. (2005). Adsorption of copper and cadmium by Cu- and Cd-resistant bacteria and their composites with soil colloids and kaolinite. Geomicrobiol. J. 22, 227–236. doi: 10.1080/01490450590947779
Idris, R., Trifonova, R., Puschenreiter, M., Wenzel, W. W., and Sessitsch, A. (2004). Bacterial communities associated with flowering plants of the Ni hyperaccumulator Thaspi goesingense. Appl. Environ. Microbiol. 70, 2667–2677. doi: 10.1128/AEM.70.5.2667-2677.2004
Jafar Mazumder, M. A. (2020). A review of green scale inhibitors: process, types, mechanism and properties. Coatings 10, 928. doi: 10.3390/coatings10100928
Jankong, P., and Visoottiviseth, P. (2008). Effects of arbuscular mycorrhizal inoculation on plants growing on arsenic contaminated soil. Chemosphere 72, 1092–1097. doi: 10.1016/j.chemosphere.2008.03.040
Jin, S., Jin, W., Bai, Y., Dong, C., Jin, D., Hu, Z., et al. (2020). Response of rice and bacterial community to phosphorus-containing materials in soil-plant ecosystem of rare earth mining area. J. Hazard Mater 381, 121004. doi: 10.1016/j.jhazmat.2019.121004
Kim, H. S., Pasten, P. A., Gaillard, J. F., and Stair, P. C. (2003). Nanocrystalline todorokite-like manganese oxide produced by bacterial catalysis. J. Am. Chem. Soc. 125, 14284–14285. doi: 10.1021/ja0375784
Kuffner, M., De Maria, S., Puschenreiter, M., Fallmann, K., Wieshammer, G., Gorfer, M., et al. (2010). Culturable bacteria from Zn- and Cd accumulating Salix caprea with differential effects on plant growth and heavy metal availability. J. Appl. Microbiol. 108, 1471–1484. doi: 10.1111/j.1365-2672.2010.04670.x
Kumar, M., and Upretil, RK. (2000). Impact of lead stress and adaptation in Escherichia coli. Environ Res. 47, 246–252. doi: 10.1006/eesa.2000.1960
Leedjärv, A., Ivask, A., and Virta, M. (2008). The interplay of different transporters in the mediation of divalent heavy metal resistance in Pseudomonas putida KT2440. J. Bacteriol. 190, 2680–2689. doi: 10.1128/JB.01494-07
Li, J., Liu, Y. R., Zhang, L. M., and He, J. Z. (2019). Sorption mechanism and distribution of cadmium by different microbial species. J. Environ. Manage. 237, 552–559. doi: 10.1016/j.jenvman.2019.02.057
Li, Y., Pang, H. D., He, L. Y., Wang, Q., and Sheng, X. F. (2017). Cd immobilization and reduced tissue Cd accumulation of rice (Oryza sativa wuyun-23) in the presence of heavy metal-resistant bacteria. Ecotox. Environ. Safe 138, 56–63. doi: 10.1016/j.ecoenv.2016.12.024
Lin, X., Mou, R., Cao, Z., Xu, P., Wu, X., Zhu, Z., et al. (2016). Characterization of cadmium-resistant bacteria and their potential for reducing accumulation of cadmium in rice grains. Sci. Total Environ. 97, 570. doi: 10.1016/j.scitotenv.2016.06.121
Manoj, S. R., Karthik, C., Kadirvelu, K., Arulselvi, P. I., Shanmugasundaram, T., Bruno, B., et al. (2020). Understanding the molecular mechanisms for the enhanced phytoremediation of heavy metals through plant growth promoting rhizobacteria: a review. J. Environ. Manage. 254, 109779. doi: 10.1016/j.jenvman.2019.109779
Marques, A. P. G. C., Rangel, A. O. S. S., and Castro, P. M. L. (2009). Remediation of heavy metal contaminated soils: phytoremediation as a potentially promising clean-up technology. Crit. Rev. Environ. Sci. Technol. 39, 622–654. doi: 10.1080/10643380701798272
Meng, Y. T., Zheng, Y. M., Zhang, L. M., and He, J. Z. (2009). Biogenic Mn oxides for effective adsorption of Cd from aquatic environment. Environ. Pollut. 157, 2577–2583. doi: 10.1016/j.envpol.2009.02.035
Mengual, C., Schoebitz, M., Caravaca, F., and Roldán, A. (2016). Assessment of the potential role of Streptomyces strains in the revegetation of semiarid sites: the relative incidence of strain origin and plantation site on plant performance and soil quality indicators. Biol. Fertil. Soils 52, 53–64. doi: 10.1007/s00374-015-1052-x
Mukherjee, S., Sarkar B,Aralappanavar, V. K., Mukhopadhyay, R., Basak, B. B., Srivastava, P., et al. (2022). Biochar-microorganism interactions for organic pollutant remediation: challenges and perspectives. Environ. Pollut. 308, 119609. doi: 10.1016/j.envpol.2022.119609
Muller, B., Granina, L., Schaller, T., Ulrich, A., and Wehrli, B. P. (2002). As, Sb, Mo, and other elements in sedimentary Fe/Mn layers of lake Baikal. Environ. Sci. Tech. 36, 411–420. doi: 10.1021/es010940z
Mutiat, F. B. Y., Gbolahan, B., and Olu, O. (2018). A comparative study of the wild and mutated heavy metal resistant Klebsiella variicola generated for cadmium bioremediation. Ann. Financ. 22, 1–15. doi: 10.1080/10889868.2018.1445695
Nealson, K. H., Tebo, B. M., Rosson, R. A., and Allen, I. L. (1988). Occurrence and mechanisms of microbial oxidation of manganese. Adv. Appl. Microbiol. 33, 279–318. doi: 10.1016/S0065-2164(08)70209-0
Negrini, M., Fidelis, E. G., Picanço, M. C., and Ramos, R. S. (2020). Mapping of the Steneotarsonemus spinki invasion risk in suitable areas for rice (Oryza sativa) cultivation using MaxEnt. Exp. Appl. Acarol. 80, 445–461. doi: 10.1007/s10493-020-00474-6
Özdemir, S., Kilinc, E., Poli, A., Nicolaus, B., and Güven, K. (2009). Biosorption of Cd, Cu, Ni, Mn and Zn from aqueous solutions by thermophilic bacteria, Geobacillus toebii sub sp decanicus and Geobacillus thermoleovorans sub sp stromboliensis: equilibrium, kinetic and thermodynamic studies. Chem. Eng. J. 152, 195–206. doi: 10.1016/j.cej.2009.04.041
Pabst, M. W., Miller, C. D., Dimkpa, C. O., Anderson, A. J., and McLean, J. E. (2010). Defining the surface adsorption and internalization of copper and cadmium in a soil bacterium, Pseudomonas putida. Chemosphere 81, 904–910. doi: 10.1016/j.chemosphere.2010.07.069
Pál, M., Horváth, E., and Janda, T. (2006). Physiological changes and defense mechanisms induced by cadmium stress in maize. J. Plant Nutr. Soil Sci. 169, 239246. doi: 10.1002/jpln.200520573
Panwichian, S., Kantachote, D., Wittayaweerasak, B., and Mallavarapu, M. (2010). Factors affecting immobilization of heavy metals by purple nonsulfur bacteria isolated from contaminated shrimp ponds. World J. Microbiol. Biotechnol. 26, 2199–2210. doi: 10.1007/s11274-010-0405-8
Peng, G., Sun, J., Peng, B., Tan, Y., Wu, Y., Bai, X., et al. (2021). Assessment of essential element accumulation in red swamp crayfish (Procambarus clarkii) and the highly efficient selenium enrichment in freshwater animals. J. Food Compos. Anal. 101, 103953. doi: 10.1016/j.jfca.2021.103953
Peraferrer, C., Martínez, M., Poch, J., and Villaescusa, I. (2012). Toxicity of metal–ethylenediaminetetraacetic acid solution as a function of chemical speciation: an approach for toxicity assessment. Arch. Environ. Contam. Toxicol. 63, 484–494. doi: 10.1007/s00244-012-9788-x
Perron, K., Caille, O., Rossier, C., Van Delden, C., Dumas, J. L., Kohler, T., et al. (2004). CzcRCzcS, a two-component system involved in heavy metal and carbapenem resistance in Pseudomonas aeruginosa. J. Biol. Chem. 279, 8761–8768. doi: 10.1074/jbc.M312080200
Rukshana, F., Butterly, C. R., Baldock, J. A., Xu, J. M., and Tang, C. (2012). Model organic compounds differ in priming effects on alkalinity release in soils through carbon and nitrogen mineralization. Soil. Biol. Biochem. 51, 35–43. doi: 10.1016/j.soilbio.2012.03.022
Saratovsky, I., Wightman, P. G., Pasten, P. A., Gaillard, J. F., and Poeppelmeier, K. R. (2006). Manganese oxides: parallels between abiotic and biotic structures. J. Am. Chem. Soc.128, 11188–11198. doi: 10.1021/ja062097g
Sasaki, K., Matsuda, M., Urata, T., Hirajima, T., and Konno, H. (2008). Sorption of Co ions on the biogenic Mn oxide produced by a Mn-oxidizing fungus, Paraconiothyrium sp WL-2. Mater Trans. 49, 605–611. doi: 10.2320/matertrans.M-MRA2007888
Shafqat, F., Bhatt, H. N., Hanif, M. A., and Zubair, A. (2008). Kinetic and equilibrium studies of Cr (III) and Cr (VI) sorption from aqueous solution using Rosa Gruss an Teplitz (red rose) waste biomass. J. Chil. Chem. Soc. 53, 1667–1672. doi: 10.4067/S0717-97072008000400006
Shi, Z., Qi, X., Zeng, X., Lu, Y., Zhou, J., Cui, K., et al. (2021). A newly isolated bacterium Comamonas sp. XL8 alleviates the toxicity of cadmium exposure in rice seedlings by accumulating cadmium. J. Hazard Mater. 403, 123824. doi: 10.1016/j.jhazmat.2020.123824
Shim, J., Kim, J., Shea, P. J., and Oh, B. T. (2015). Biosorption of cadmium by Citrobacter sp JH 11-2 isolated from mining site soil. Sep. Sci. Technol. 50, 2134–2141. doi: 10.1080/01496395.2015.1041978
Sprocati, A. R., Alisi, C., Segre, L., Tasso, F., Galletti, M., Cremisini, C., et al. (2006). Investigating heavy metal resistance, bioaccumulation and metabolic profile of a metallophile microbial consortium native to an abandoned mine. Sci. Total Environ. 366, 649–658. doi: 10.1016/j.scitotenv.2006.01.025
Srivastava, S., and Gupta, B. (2021). Application of immobilization techniques in heavy metal and metalloid remediation. Immobilization Strat. 2021, 581–595. doi: 10.1007/978-981-15-7998-1_17
Su, Y. Q., Zhao, Y. J., Zhang, W. J., Chen, G. C., Qin, H., Qiao, D. R., et al. (2020). Removal of mercury (II), lead (II) and cadmium (II) from aqueous solutions using Rhodobacter sphaeroides SC01. Chemosphere 243, 125166. doi: 10.1016/j.chemosphere.2019.125166
Suksabye, P., Pimthong, A., Dhurakit, P., Mekvichitsaeng, P., and Thiravetyan, P. (2016). Effect of biochars and microorganisms on cadmium accumulation in rice grains grown in Cd-contaminated soil. Environ. Sci. Pollut. Res. 23, 962–973. doi: 10.1007/s11356-015-4590-8
Sulaymon, A. H., Ebrahim, S. E., and Mohammed-Ridha, M. J. (2013). Equilibrium, kinetic, and thermodynamic biosorption of Pb (II), Cr (III), and Cd (II) ions by dead anaerobic biomass from synthetic wastewater. Environ. Sci. Pollut. Res. 20, 175–187. doi: 10.1007/s11356-012-0854-8
Tebo, B. M., Bargar, J. R., Clement, B. G., Dick, G. J., Murray, K. J., Parker, D., et al. (2004). Biogenic manganese oxides: properties and mechanisms of formation. Ann Rev Earth Pl Sc 32, 287–328. doi: 10.1146/annurev.earth.32.101802.120213
Tessier, A., Campbell, P. G. C., and Bisson, M. (1979). Sequential extraction procedure for the speciation of particulate trace metals. Anal. Chem. 51, 844–851. doi: 10.1021/ac50043a017
Tsukahara, T., Ezaki, T., Moriguchi, J., Furuki, K., Shimbo, S., Matsuda-Inoguchi, N., et al. (2003). Rice as the most influential source of cadmium intake among general Japanese population. Sci. Total Environ. 305, 41–51. doi: 10.1016/S0048-9697(02)00475-8
Van Waasbergen, L. G., Hildebrand, M., and Tebo, B. M. (1996). Identification and characterization of a gene cluster involved in manganese oxidation by spores of the marine Bacillus sp strain SG-1. J. Bacteriol. 178, 3517–3530. doi: 10.1128/jb.178.12.3517-3530.1996
Venkatesh, N. M., and Vedaraman, N. (2012). Remediation of soil contaminated with copper using rhamnolipids produced from Pseudomonas aeruginosa MTCC 2297 using waste frying rice bran oil. Ann. Microbiol. 62, 85–91. doi: 10.1007/s13213-011-0230-9
Verma, S., Bhatt, P., Verma, A., Mudila, H., Prasher, P., Rene, E. R., et al. (2021). Microbial technologies for heavy metal remediation: effect of process conditions and current practices. Clean. Technol. Environ. Policy 14, 1–23. doi: 10.1007/s10098-021-02029-8
Villalobos, M., Toner, B., Bargar, J., and Sposito, G. (2003). Characterization of the manganese oxide produced by Pseudomonas putida strain MnB1. Geochimica. et Cosmochimica Acta. 67, 2649–2662. doi: 10.1016/S0016-7037(03)00217-5
Wang, X. H., Nie, Z. W., and He, L. Y. (2017). Isolation of As-tolerant bacteria and their potentials of reducing As and Cd accumulation of edible tissues of vegetables in metal (loid) contaminated soils. Sci. Total Environ. 579, 179–189. doi: 10.1016/j.scitotenv.2016.10.239
Wu, S. C., Peng, X. L., Cheung, K. C., Liu, S. L., and Wong, M. H. (2009). Adsorption kinetics of Pb and Cd by two plant growth promoting rhizobacteria. Bioresour. Technol. 100, 4559–4563. doi: 10.1016/j.biortech.2009.04.037
Yin, K., Wang, Q., Lv, M., and Chen, L. (2019). Microorganism remediation strategies towards heavy metals. Chem. Eng. J. 360, 1553–1563. doi: 10.1016/j.cej.2018.10.226
Yu, Q., and Fein, J. B. (2015). The effect of metal loading on Cd adsorption onto Shewanella oneidensis bacterial cell envelopes: the role of sulfhydryl sites. Geochem. Cosmochim. Acta. 167, 1–10. doi: 10.1016/j.gca.2015.06.036
Zamil, S., Choi, M., Song, J., Park, H., Xu, J., Chi, K. W., et al. (2008). Enhanced biosorption of mercury (II) and cadmium (II) by coldinduced hydrophobic exobiopolymer secreted from the psychrotroph Pseudomonas fluorescens BM07. Appl. Microbiol. Biotechnol. 80, 531–544. doi: 10.1007/s00253-008-1621-0
Keywords: cadmium, bio-sorption, tolerant species, Cd accumulation, rice
Citation: Li J, Guo Y-K, Zhao Q-X, He J-Z, Zhang Q, Cao H-Y and Liang C-Q (2023) Microbial cell wall sorption and Fe–Mn binding in rhizosphere contribute to the obstruction of cadmium from soil to rice . Front. Microbiol. 14:1162119. doi: 10.3389/fmicb.2023.1162119
Received: 09 February 2023; Accepted: 07 March 2023;
Published: 17 April 2023.
Edited by:
Yong Jiang, Fujian Agriculture and Forestry University, ChinaReviewed by:
Linlin Dong, Suzhou Academy of Agricultural Sciences, ChinaXiaohong Zhao, Chang'an University, China
Copyright © 2023 Li, Guo, Zhao, He, Zhang, Cao and Liang. This is an open-access article distributed under the terms of the Creative Commons Attribution License (CC BY). The use, distribution or reproduction in other forums is permitted, provided the original author(s) and the copyright owner(s) are credited and that the original publication in this journal is cited, in accordance with accepted academic practice. No use, distribution or reproduction is permitted which does not comply with these terms.
*Correspondence: Hong-Ying Cao, Y2FvaHkmI3gwMDA0MDtpZ3NucnIuYWMuY24=; Chao-Qiong Liang, bGNxMTk4ODAzMDUmI3gwMDA0MDsxMjYuY29t
†ORCID: Jie Li orcid.org/0000-0002-6691-5882