- Centro Andaluz de Biología del Desarrollo (CABD), Universidad Pablo de Olavide-CSIC-Junta de Andalucía, Seville, Spain
Phytopathogenic fungi must adapt to the different environmental conditions found during infection and avoid the immune response of the plant. For these adaptations, fungi must tightly control gene expression, allowing sequential changes in transcriptional programs. In addition to transcription factors, chromatin modification is used by eukaryotic cells as a different layer of transcriptional control. Specifically, the acetylation of histones is one of the chromatin modifications with a strong impact on gene expression. Hyperacetylated regions usually correlate with high transcription and hypoacetylated areas with low transcription. Thus, histone deacetylases (HDACs) commonly act as repressors of transcription. One member of the family of HDACs is represented by sirtuins, which are deacetylases dependent on NAD+, and, thus, their activity is considered to be related to the physiological stage of the cells. This property makes sirtuins good regulators during environmental changes. However, only a few examples exist, and with differences in the extent of the implication of the role of sirtuins during fungal phytopathogenesis. In this work, we have performed a systematic study of sirtuins in the maize pathogen Ustilago maydis, finding Sir2 to be involved in the dimorphic switch from yeast cell to filament and pathogenic development. Specifically, the deletion of sir2 promotes filamentation, whereas its overexpression highly reduces tumor formation in the plant. Moreover, transcriptomic analysis revealed that Sir2 represses genes that are expressed during biotrophism development. Interestingly, our results suggest that this repressive effect is not through histone deacetylation, indicating a different target of Sir2 in this fungus.
1. Introduction
Phytopathogenic fungi must sense many environmental host cues and respond with developmental changes in order to ensure proper plant infection progression. In the well-established model organism Ustilago maydis, a biotrophic pathogen infecting maize plants, the first step in the pathogenic program is the switch from yeast to filament on the surface of the plant, followed by the fusion of two sexually compatible filaments. This dikaryotic filament blocks the cell cycle and extends until it identifies the appropriate location for penetration, where it develops a specialized invasive structure, the appressoria. Upon plant penetration, the filament releases the cell cycle block and colonizes the plant until the development of teliospores inside plant tumors induced by the fungus (Brefort et al., 2009; Vollmeister et al., 2012; Castanheira and Pérez-Martín, 2015). All these sequential changes must be tightly controlled in order to ensure successful infection. Many advances have been achieved in determining the transcription factors involved in this control mechanism in many fungi. In U. maydis, environmental cues are transmitted by the MAP kinases and cAMP signaling pathways to the Prf1 transcription factor, which controls, among others, the bE/bW compatible heterodimer transcribed from the MAT b locus in the dikaryon. This heterodimer controls many downstream virulence genes, including other important transcription factors (Hartmann et al., 1996, 1999; Brachmann et al., 2001; Heimel et al., 2010; Lanver et al., 2017).
Another layer of control is chromatin modification, which plays crucial roles in transcriptional regulation in response to environmental cues (Mazzio and Soliman, 2012; Rando and Winston, 2012; Badeaux and Shi, 2013). Histone modifiers carry out different posttranslational modifications in the histone tails, such as acetylation, methylation or phosphorylation, among others, with potential alterations in the transcriptional stages of the surrounding area. One of the major and most well-described histone modifications, together with methylation, is the acetylation in a lysine residue (Allfrey et al., 1964). The enzymes responsible for this acetylation are called histone acetyltransferases (HATs), and those involved in the removal of this modification are the histone deacetylases (HDACs).
The role of these chromatin modifiers in pathogenesis has been explored in different fungi. Gcn5 is the main HAT studied in plant pathogenic fungi, with important roles in development and infection (de Ruijter et al., 2003; González-Prieto et al., 2014; Kong et al., 2018; Liu et al., 2022). In U. maydis, the deletion of Gcn5 causes constitutive filamentation and reduction of infection by, at least, the derepression of prf1 and bE1 genes (González-Prieto et al., 2014). On the other hand, the roles of HDACs during fungal plant pathogenesis, although poorly characterized, are better known than those for HATs. HDACs can be classified into three main different categories, Class I, II and III, based on their homology to the yeast orthologues Rpd3, Hda1 and Sir2, respectively (de Ruijter et al., 2003). Among Class I/II HDACs, the Set3 complex, comprising the HDAC Hos2, is one of the best studied in fungal plant pathogens, with conserved roles in pathogenesis (Elías-Villalobos et al., 2019). In U. maydis, Hos2 affects filamentation and pathogenesis through direct regulation of the MAT a locus (Elías-Villalobos et al., 2015). In addition to Hos2, other Class I/II HDACs, Hda1 and Hda2, have also been characterized in U. maydis. Hda1 is essential for teliospore production with a role in gene regulation, repressing the transcription of egl1 and mig1 during the non-pathogenic state of the fungus (Reichmann et al., 2002; Torreblanca et al., 2003). In contrast, deletion of hda2 did not alter the infection capability of U. maydis (González-Prieto et al., 2004; Elías-Villalobos et al., 2015). Class III HDACs constitute a particular group of histone deacetylases that are dependent on NAD+ for their catalytic activity (Tanner et al., 2000; Tanny and Moazed, 2001; Jackson and Denu, 2002; Zhao and Rusche, 2022). The founding member of this class, collectively named sirtuins, is Sir2 (Silent Information Regulator 2) from Saccharomyces cerevisiae. ScSir2 forms a complex with other SIR proteins and is involved in the silencing of heterochromatin-like regions in this yeast by deacetylating H4 lysine 16 residue (H4K16) (Robyr et al., 2002; Suka et al., 2002). The role of Sir2 in chromatin silencing is broadly observed, with examples also described in filamentous fungi, such as Neurospora crassa or Aspergillus nidulans (Smith et al., 2008; Shimizu et al., 2012; Itoh et al., 2017), which suggests an ancient role of this protein in silencing (Hickman et al., 2011). In addition to Sir2, other sirtuins have been characterized in different organisms. In S. cerevisiae and Schizosaccharomyces pombe, all the other sirtuins, Hst1 to 4 in S. cerevisiae and Hst2 and 4 in S. pombe, have been linked to chromatin silencing as well as direct gene regulation (Brachmann et al., 1995; Freeman-Cook et al., 1999; Sutton et al., 2001; Halme et al., 2004; Wilkins et al., 2014). Within the sirtuin family, Sir2 has been described to control pathogenesis in different fungi. For instance, in the human pathogen Candida glabrata, Sir2 represses the EPA adhesin genes, which are essential for infection (Domergue et al., 2005), and in Cryptococcus neoformans, Sir2 is essential for virulence, due to a mechanism not described so far (Arras et al., 2017). The main example to date for the role of Sir2 in plant pathogens is found in Magnaporthe oryzae. In this rice pathogen, Sir2 likely affects infection through inactivation by deacetylation of the MoJmjC repressor, which would lead to an increase in superoxide dismutase expression, allowing ROS detoxification (Fernandez et al., 2014).
In order to increase our knowledge regarding the role of sirtuins in fungal plant pathogens, we have performed a characterization of the sirtuin family in U. maydis. We have observed that two of the five sirtuins present in U. maydis, Sir2 and Hst4, display nuclear localization during the entire cell cycle. From them, Hst4 is essential and Sir2 negatively impacts the yeast to filament transition and virulence. While the deletion of sir2 slightly increases the virulence capacity, its overexpression significantly reduces virulence. A transcriptomic analysis of both deletion in filamentation conditions and overexpression during infection indicates that Sir2 avoids the proper activation of a group of genes induced during the biotrophic development. We have observed an increase in H4 acetylation in a Δsir2 mutant in the upregulated genes. However, this deacetylation is not detected in the typical residue observed in other organisms, lysine 16. As these effects may be the consequence of the increase in transcription observed, further analyses are required in order to detect the specific target of Sir2 in terms of its regulatory role in U. maydis.
2. Materials and methods
2.1. Strains and growth condition
Escherichia coli DH5α, pJET1.2/blunt (Thermo Scientific, Carlsbad, CA, United States) and pBluescript II SK (+) (Stratagene, San Diego, CA, United States) were used for cloning purposes. The growth conditions for E. coli were described in (Sambrook et al., 1989). All the strains used in this study are derived from the haploid pathogenic SG200 strain and are listed in Supplementary Table S1 and verified by Southern Blot (Supplementary Figure S1). As previously described in Gillissen et al. (1992), U. maydis cultures were performed in YEPSL (0.4% bactopeptone, 1% yeast extract and 0.4% saccharose) at 28°C, unless otherwise specified. For charcoal filamentation assays, exponential cultures were spotted onto PD–charcoal plates (2.4% potato dextrose broth, 1% charcoal, 2% agar) and grown for 18–20 h at 25°C. Pathogenicity assays were performed as described in Kämper et al. (2006). Ustilago maydis exponential cultures were concentrated to an OD600 of 0.5 or 1 and injected into 7-day-old maize (Zea mays) seedlings (Early Golden Bantam). Disease symptoms were quantified at 14 dpi. Data of individual infection experiments are listed in Supplementary Table S2. Statistical analyses were performed in the GraphPad Prism 8 software.
2.2. Plasmid and strain construction
Molecular biology techniques were used as previously described (Sambrook et al., 1989). Ustilago maydis DNA isolation and transformation procedures were carried out following the protocol described in Schulz et al. (1990). Deletion mutants for sir2 (UMAG_00963), hst2 (UMAG_05892), hst4 (UMAG_05758) and hst5 (UMAG_05239) were generated by homologous recombination, as described previously (Brachmann et al., 2004; Kämper, 2004). Deletion for hst6 (UMAG_12006) was performed using the NEBuilder HiFi DNA Assembly (New England Biolabs, Ipswich, MA, United States) system. The sir2 complementation mutant was generated by reintroducing the sir2 open reading frame (ORF) in the Δsir2 background in its endogenous loci, replacing the nat resistance cassette of the Δsir2 mutant with the sir2 ORF, followed by the geneticin resistance cassette by homologous recombination. For sir2 overexpression with the otef promoter, the p123-Potef:sir2 plasmid was generated by replacing the eGFP fragment from the p123 plasmid (Aichinger et al., 2003) with the sir2 ORF. The sir2 ORF was amplified by PCR using Q5 High-Fidelity DNA polymerase (New England Biolabs, Ipswich, MA, United States) and cloned into p123 within the NcoI and NotI restriction sites of p123. For sir2 overexpression with the pit2 promoter, we constructed the p123-Ppit2:sir2 plasmid. The sir2 ORF was amplified and cloned into p123-Ppit2 within the NcoI and XbaI restriction sites of p123-Ppit2. To generate SG200 Potef:sir2 or SG200 Ppit2:sir2, p123-Potef:sir2 or p123-Ppit2:sir2 was linearized with SspI and integrated into the ip locus by homologous recombination. For GFP endogenous sirtuin tagging, the plasmids pBSK-sir2:eGFP, pBSK-hst2:eGFP, pBSK-hst4:eGFP, pBSK-hst5:eGFP and pBSK-hst6:eGFP were generated using the NEBuilder HiFi DNA Assembly (New England Biolabs, Ipswich, MA, United States) system. A 1 Kb fragment containing the gene of interest (ORF without the STOP codon) and a 1 Kb fragment of the 3′ region were amplified using primers designed in the NEBuilder assembly tool. eGFP followed by hygromycin resistance cassettes were amplified from the pmf5-1 h plasmid (Becht et al., 2006). All the fragments were cloned into the pBluescript II SK (+) plasmid using the NEBuilder HiFi DNA Assembly (New England Biolabs, Ipswich, MA, United States). Constructs were amplified by PCR prior to their transformation in U. maydis. The primers used in this study are listed in Supplementary Table S3. All the strains and numbers of copies integrated into the ip locus were verified by PCR and Southern blotting.
2.3. Microscopy and image analysis
To analyze the filamentation capability of U. maydis in PD-charcoal plates, single colonies where visualized using the Leica M205 Stereoscope equipped with an ORCA-Flash4.0 LT Hamamatsu digital camera. The area of the colonies was measured by selecting the perimeter of each colony using the plugging convex hull of ImageJ software. To determine sirtuins’ localization, sir2:eGFP, hst2:eGFP, hst4:eGFP, hst5:eGFP and hst6:eGFP cells were visualized using a DeltaVision microscopy system comprising an Olympus IX71 microscope and CoolSnap HQ camera (Applied Precision, Issaquah WA, United States). To visualize mitochondria, 0.5 mM Mito-Tracker CM-H2Xros (Molecular Probes, Eugene, OR) was added to the U. maydis YEPSL cell culture and cells were incubated for 15 min at 25°C (Bortfeld et al., 2004). To analyze the U. maydis progression inside the maize plant, leaves samples from 3, 4 and 6 dpi infected plants were distained with ethanol, treated for 4 h at 60°C with 10% KOH, washed in phosphate buffer and then stained with propidium iodide (PI) to visualize plant tissues in red and wheat germ agglutinin (WGA)/AF488 to visualize the fungus in green. At least four leaves from two independent experiments were stained and visualized by fluorescence microscopy (Leica SPE DM2500, Leica, WZ, Germany). Image processing was carried out using the ImageJ software.
2.4. RNA extraction and RT-qPCR
Total RNA was extracted from U. maydis cells grown in YEPSL medium, PD–charcoal plates and from infected leaves by excising 2–3 cm segments from below the injection holes. All the samples were ground into a powder using a mortar/pestle under liquid nitrogen. Total RNA was purified using TRIzol reagent (Invitrogen, Carlsbad, CA, United States) and the Direct-zol RNA Miniprep Plus Kit (Zymo Research, Irvine, CA, United States). RNA was retrotranscribed from 3 μg of total RNA using the RevertAid H Minus First Strand cDNA Synthesis Kit (Thermo Scientific, Carlsbad, CA, United States). RT-qPCR was performed using a Real-Time CFX Connect (Bio-Rad, Hercules, CA, United States) and SYBR Premix Ex Taq II (Tli RNase H Plus) (Takara Bio INC, Kusatsu, Japan). All reactions were performed in at least three biological replicates, and gene expression levels were calculated relative to the expression levels of the constitutively expressed fungal ppi1 gene. Primers used for RT-qPCR are listed in Supplementary Table S3. The quantification of relative fungal biomass in infected maize leaves was performed as previously described (Brefort et al., 2014). Ustilago maydis biomass was quantified measuring the signal of the ppi1 fungal gene relative to the plant gene GAPDH. Statistical analyses were performed in the GraphPad Prism 8 software.
2.5. RNA-Seq analysis
Total RNA extracted from axenic cultures and PD–charcoal plates from U. maydis wild-type and Δsir2 strains was submitted to BGI TECH SOLUTIONS (HONGKONG) CO., LIMITED, in a 200–500 ng/μL concentration, with a total RNA quantity of 5–8 μg and quality parameters of OD260/280 = 1.8–2.1 and OD260/230 > 1.5. The BGI company prepared all libraries and performed the single-end sequencing via the BGISEQ-500 RNA-Seq service. Two replicates of each strain and condition were processed. To determine Sir2-regulated genes during pathogenesis, 7-day-old maize seedlings were infected with wild-type and Ppit2:sir2 > 1c strains and total RNA was purified at 3 dpi. RNA samples were submitted to BGI TECH SOLUTIONS (HONGKONG) CO., LIMITED, and paired-end sequenced via the DNBseq PE100 service. Three replicates of each strain were processed. Reads were mapped to the U. maydis genome using HISAT2 and reads from infected plant tissues were previously filtered against the annotated maize genome. Reads were counted for U. maydis using the HTseq tool in the Galaxy platform, and, for expression analysis, only uniquely mapping exon read counts were considered. Pairwise comparisons were performed using the R package DESeq2 (Love et al., 2014). Genes with log2 fold change >0.5 or < −0.5 and adjusted value of p <0.05 were considered differentially regulated.
2.6. Western blot analysis
For total protein extraction, cells from exponential culture were collected by centrifugation and washed twice with 20 mM Tris–HCl pH 8.8. Pellets were ground to powder with a mortar under liquid nitrogen and resuspended in RIPA buffer (50 mM Tris–HCl, pH 8, 150 mM NaCl, 1% Nonidet P-40, 0.5% sodium deoxycholate, 0.1% SDS) supplemented with 1 μg/mL Pepstatin A (PanReac AppliChem, Barcelona, Spain), 1 μg/mL Bestatin (Thermo Scientific, Carlsbad, CA, United States), 1 mM PMSF (PanReac AppliChem, Barcelona, Spain) and EDTA-free protease inhibitor complex (cOmplete Tablets EDTA-free, Roche, Mannheim, BW, Germany). After cell lysis, samples were centrifuged and the supernatant was collected. For protein extraction in PD–charcoal plates, cells were scraped off and ground to powder in liquid nitrogen and resuspended in 12% TCA solution to precipitate proteins. Pellets were washed 4 times with ice-cold acetone and dissolved in extraction buffer (100 mM Tris–HCl pH 8, 50 mM NaCl, 1% SDS, 1 mM EDTA) supplemented with the protease inhibitors listed above. Protein concentration was measured by the BCA protein assay. Here, 40 μg of protein extract was loaded into a 10% TGX Stain-Free FastCast Acrylamide Gel (Bio-Rad, Hercules, CA, United States) or SDS polyacrylamide 15% running gel in the case of histone analysis. Separated proteins were transferred into a nitrocellulose membrane using the Trans-Blot Turbo transfer system (Bio-Rad, Hercules, CA, United States). The membrane was incubated with mouse polyclonal anti-GFP antibody (Roche, Mannheim, BW, Germany) (1:1000 in PBST 5% fat-free milk) and anti-mouse IgG HRP conjugated (Invitrogen, Carlsbad, CA, United States) (1:5000) was used as a secondary antibody. Histone modifications were detected with primary antibodies specific to H3 (Sigma-Aldrich, Darmstadt, Germany), H3ac (Sigma-Aldrich, DA, Germany), H3K9ac (abcam, Cambridge, UK) (1:5000 in PBST 5% fat-free milk), H4 (Sigma-Aldrich, Darmstadt, Germany), H4ac (Sigma-Aldrich, Darmstadt, Germany) (1:5000 in PBST 3% BSA) and H4K16ac (Sigma-Aldrich, Darmstadt, Germany) (1:5000 in PBST 5% fat-free milk) and anti-rabbit HRP conjugated as a secondary antibody (Sigma-Aldrich, Darmstadt, Germany) (1:5000). Chemiluminescence was detected with SuperSignal™ West Femto Maximum Sensitivity Substrate (Thermo Scientific, Carlsbad, CA, United States). Image gel and membrane acquisition was carried out with the ChemiDoc XRS (Bio-Rad, Hercules, CA, United States). All the Western blot assays were performed with at least three biological replicates and quantified using the Image Lab software.
2.7. Chromatin immunoprecipitation
Exponential culture of U. maydis cells were cross-linked by incubating with 1% formaldehyde for 10 min and reaction stopped by adding glycine to a final concentration of 250 mM for 10 min at room temperature. Cells were collected by centrifugation and washed twice with cold PBS. 2× 250 mg of pellets were ground to powder with a mortar under liquid nitrogen and resuspended in ChIP lysis Buffer (50 mM HEPES-KOH pH 7.5, 140 mM NaCl, 1 mM EDTA pH 8, 1% Triton X-100, 0.1% Na-deoxycholate, 0.1% SDS) supplemented with 1 μg/mL Pepstatin A (PanReac AppliChem, Barcelona, Spain), 1 μg/mL Bestatin (Thermo Scientific, Carlsbad, CA, United States), 1 mM PMSF (PanReac AppliChem, Barcelona, Spain) and EDTA-free protease inhibitor complex (cOmplete Tablets EDTA-free, Roche, Mannheim, BW, Germany). Samples were then sonicated in a Bioruptor® sonication device (Diagenode) for 20 min, with 2 min pulses separated by 1 min rest periods at maximum power. 100 μL of the chromatin extract was kept as input and a total of 10 O.D. of chromatin extract was used for IP. Samples were incubated with 3 μL of antibodies against H3ac (Sigma-Aldrich, DA, Germany), H4 (Sigma-Aldrich, Darmstadt, Germany), H4ac (Sigma-Aldrich, Darmstadt, Germany) and H4K16ac (Sigma-Aldrich, Darmstadt, Germany) at 4°C overnight on a rotary shaker. Precipitation of the protein-antibody conjugate was performed incubating with Dynabeads® Protein A (Thermo Scientific, Carlsbad, CA, United States) 40 min at 4°C in a rotary shaker. Beads were washed twice with WB150 (20 mM Tris–HCl pH 8, 150 mM NaCl, 2 mM EDTA pH 8, 1% Triton X-100), once with WB500 (20 mM Tris–HCl pH 8, 500 mM NaCl, 2 mM EDTA pH 8, 1% Triton X-100) and eluted in TES buffer (50 mM Tris–HCl pH 8, 10 mM EDTA, 1% SDS). To reverse the crosslink, both input and IP chromatin extracts were incubated at 65°C for 16 h. Histone-bound DNA was treated with Proteinase K (Thermo Scientific, Carlsbad, CA, United States) and DNA purification was done using the ChIP DNA Clean & Concentrator™ (Zymo Research, Irvine, CA, United States). For RT-qPCR a 20-fold dilution of each immunoprecipitated sample and a 200-fold dilution of input samples were used. Primers used for each amplicon were listed in Supplementary Table S3. All experiments were performed with three biological replicates.
2.8. Immunoprecipitation and mass spectrometry
Cells grown in YEPSL to exponential phase were collected and washed twice with 20 mM Tris–HCl pH 8.8. Pellets were then resuspended, and cells lysed in RIPA buffer (50 mM Tris/HCl, pH 8, 150 mM NaCl, 1% Nonidet P-40, 0.5% sodium deoxycholate, 0.1% SDS) supplemented 1 μg/mL Pepstatin A (PanReac AppliChem, Barcelona, Spain), 1 μg/mL Bestatin (Thermo Scientific, Carlsbad, CA, United States), 1 mM PMSF (PanReac AppliChem, Barcelona, Spain) and EDTA-free protease inhibitor complex (cOmplete Tablets EDTA-free, Roche, Mannheim, BW, Germany). After cell lysis, samples were centrifuged at 14000 rpm for 30 min at 4°C and the supernatant was collected. Sir2:eGFP was immunoprecipitated with ChromoTek GFP-Trap® Magnetic Agarose (ChromoTek, Planegg, Germany). Elution was performed by boiling the samples in LaemmLi buffer supplemented 200 mM DTT and proteins precipitated by addition of 4 volumes 1:1 methanol:acetone. Protein pellets were resuspended in urea 6 M, 50 mM ammonium bicarbonate. Disulfide bonds were reduced adding DTT 10 Mm and for carbamidometylation of cysteine –SH groups, IAA 30 mM was added. Samples were digested overnight at 37°C using trypsin bovine (Sequencing Grade Modified Trypsin, Promega) in a ratio 1:12 enzyme-substrate. Reaction was stopped using formic acid to 0.5%. OMIX C18 tips (Agilent Technologies) were used for concentrating and desalting peptide extracts. Samples were dried and resuspended in 0.1% trifluoroacetic acid. 1ug of each sample was injected in nano-HPLC system. LC–MS data were analyzed using the SEQUEST® HT search engine in Thermo Scientific™ Proteome Discoverer™ 2.2 software. Data were searched against the Uniprot U. maydis protein database and results were filtered using a 1% protein FDR threshold. Two replicates of each strain were processed.
3. Results
3.1. The systematic characterization of sirtuins in Ustilago maydis shows Sir2 as a nuclear sirtuin controlling cell-to-filament transition
In a previous phylogenetic analysis (Elías-Villalobos et al., 2019), five sirtuin homologs were described in U. maydis: UMAG_00963 (Sir2), UMAG_05892 (Hst2), UMAG_05758 (Hst4), UMAG_05239 (Hst5) and UMAG_12006 (Hst6). As expected, all these proteins contained the conserved sirtuin catalytic domain (PROSITE:PS50305) involved in protein deacetylation (Figure 1A). Additionally, we detected nuclear localization signals in only Sir2 and Hst4 (Figure 1A). As we were interested in studying the possible role of sirtuins in the control of the transcriptional pathogenic program, we examined the cellular localization of these proteins in order to focus on the nuclear ones. Consistent with their localization motifs, we observed that Sir2 and Hst4 displayed nuclear localization (Figure 1B). Additionally, Hst2 showed a nuclear signal only in mitotic cells (Figure 1B). By contrast, Hst5 and 6 were localized in the mitochondria, as verified by Mito-tracker colocalization (Figure 1B; Supplementary Figure S2A). This is consistent with the similarity described for these sirtuins to mitochondrial ones (Elías-Villalobos et al., 2019). In addition to the cellular localization study, we deleted all sirtuin genes in the solopathogenic strain SG200, except for the hst4 gene, whose deletion we found to be lethal (Supplementary Figure S2B). We performed plant infection assays with these mutants and found no significant changes in the symptoms of plants infected with ∆hst2, ∆hst5 or ∆hst6 mutants compared to the wild-type strain (Supplementary Figure S1C). However, we detected a slight increase in the symptoms of plants infected with the ∆sir2 mutant (Figure 1C). A more significant difference was observed when we studied the yeast to filament transition in solid PD–charcoal plates, which mimicked the hydrophobic conditions of the plant surface. Here, we observed an increase in the filamentation capability of the ∆sir2 mutant (Figure 1D; Supplementary Figure S2D), which was restored to its normal level after the reintroduction of sir2 in the endogenous locus (Supplementary Figure S2D). We quantified this increase in filamentation by growing individual colonies on PD-charcoal plates and measuring filaments length of the single colonies, observing that ∆sir2 colonies exhibit longer filaments compared to wild-type (Figure 1D). No changes in filamentation were observed for the rest of the sirtuin mutants (Supplementary Figure S2D).
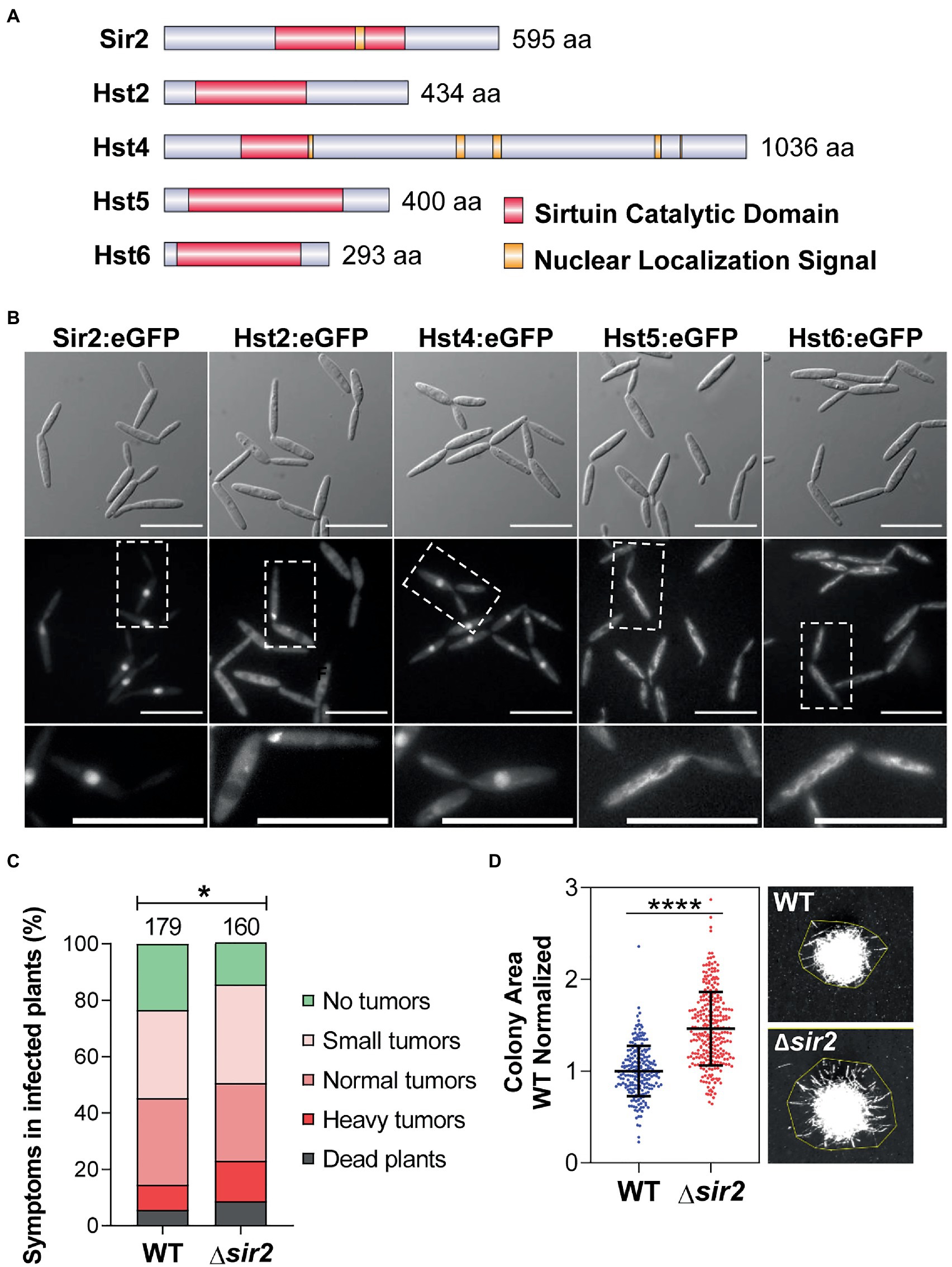
Figure 1. The nuclear sirtuin Sir2 is involved in the virulence and filamentation of Ustilago maydis. (A) Schematic representation of all the sirtuins identified in U. maydis. (B) Subcellular localization of the indicated U. maydis sirtuins tagged with eGFP in its endogenous loci. Scale bar represents 20 μm. (C) Quantification of symptoms for plants infected with the wild-type and Δsir2 mutant at 14 dpi. Total number of infected plants is indicated above each column. Three biological replicates were analyzed. Mann–Whitney statistical test was performed (*p-value < 0.05). (D) Quantification of the area of the wild-type and Δsir2 mutant single colonies grown on PD–charcoal plates for 48 h at 25°C. The colony area was measured as indicated in the stereoscopic images. Data was normalized with the mean of the area of the wild-type colonies. Three biological replicates were analyzed. Student’s t-test statistical analysis was performed (****p-value < 0.0001).
3.2. Sir2 affects the transcription of some genes during filamentation
In order to disregard a possible pleiotropic effect of sir2 deletion, we performed growth assays in rich (YEPSL), complete (CMD) and minimal (MMD) media (Supplementary Figure S3A), flow cytometry analysis of DNA-stained cells (Supplementary Figure S3B) and a stress assay with sorbitol and NaCl as osmotic stressors, H2O2 as an oxidant, SDS as a membrane-perturbing drug, calcofluor white (CFW) and Congo red as cell wall integrity sensors and Tunicamycin and DTT as endoplasmic reticulum stressors (Supplementary Figure S3C). We were not able to detect any significant differences with respect to wild-type cells, suggesting no pleiotropy. Thus, we focused on the role of Sir2 in cell to filament transition. We analyzed Sir2 protein levels and observed a drastic reduction in Sir2 during filamentation (Figure 2A). Although Sir2 is mainly present in axenic conditions, and its deletion caused an increase in filamentation on PD–charcoal plates, no filaments were found in the ∆sir2 mutant in axenic conditions (Figure 2B). In addition, almost no differences in gene expression were found by RNA sequencing (RNA-seq) analysis in a ∆sir2 mutant in axenic conditions (Figure 2C; Supplementary Table S4). The most upregulated gene was eff1-9 (Figure 2C; Supplementary Table S4), a member of the eff family of effector proteins important for virulence (Khrunyk et al., 2010; Schuster et al., 2018). eff1-9 upregulation was confirmed by RT-qPCR in the ∆sir2 mutant and the transcription levels were restored in the complementation strain (Supplementary Figure S3D). The other upregulated genes were two subtelomeric genes, UMAG_04104 and UMAG_05781, and three genes involved in metabolism, UMAG_01476, UMAG_04922 and UMAG_01656 (Figure 2C; Supplementary Table S4). We then analyzed transcription changes in a ∆sir2 mutant in filament-induced PD–charcoal plates by RNA-seq. We obtained 11 downregulated and 31 upregulated genes (Figure 2D; Supplementary Table S5). Many of the upregulated genes, 58%, encoded for predicted effector proteins, including many of the previously characterized ones: Mig2-6 (Farfsing et al., 2005), Pit1 and 2 (Doehlemann et al., 2011; Mueller et al., 2013), Eff1-7 (Khrunyk et al., 2010), Cmu1 (Djamei et al., 2011), Rsp3 (Ma et al., 2018), Erc1 (Ökmen et al., 2022) and Egl1 and 3 (Schauwecker et al., 1995; Doehlemann et al., 2008). In order to know if the ∆sir2 upregulated genes are genes which have to be expressed when filamentation is induced in PD-charcoal plates, we studied the distribution of the significantly different log2 fold changes in expression of all these genes during charcoal growth, in comparison with axenic conditions, in a wild-type strain (Supplementary Table S6). We observed that when all genes of a wild-type strain were analyzed, there was a general distribution in which log2 fold changes expanded from negative to positive values (Figure 2E). However, the group of genes that were upregulated in ∆sir2 corresponded to genes upregulated during charcoal growth (Figure 2E; Supplementary Tables S5, S6). As comparison between growth on charcoal plates versus liquid rich media imply other changes different than filamentation, we crossed our data with other datasets more specific for filamentation. Interestingly, we found that 42% of the upregulated genes in the Δsir2 mutant are genes upregulated when filamentation and appressoria formation is induced by an hydrophobic surface and hydroxy fatty acids (Lanver et al., 2014; Supplementary Table S5). Interestingly, egl1 has been previously identified as a gene specifically expressed in filaments (Schauwecker et al., 1995). As many of the described effectors found to be upregulated in a Δsir2 mutant have their effect during infection, we also studied the possibility of these genes being activated during the infection process. In a previous study of the transcriptional changes observed during infection performed by RNA-seq, Lanver et al. (2018) described different modules of coexpressed genes during infection. We observed the strong enrichment of genes belonging to the magenta (52%) and the cyan (26%) modules in the group of genes upregulated in ∆sir2 in comparison to all the upregulated genes during filamentation (Figure 2F; Supplementary Tables S5, S6). The magenta module includes genes induced at the beginning of infection, at 0.5 to 2 days post inoculation (dpi) and expression is maintained during most of the pathogenic program, thus correlates with the establishment and maintenance of biotrophy. The genes present in the cyan module are induced at 2 to 4 dpi, just at the onset of tumor formation, and stay expressed up to the last moments of the infection process, thus representing a tumor module (Lanver et al., 2018). All these data may indicate a role of Sir2 in avoiding the proper activation of a group of genes induced during filamentation and probably during infection.
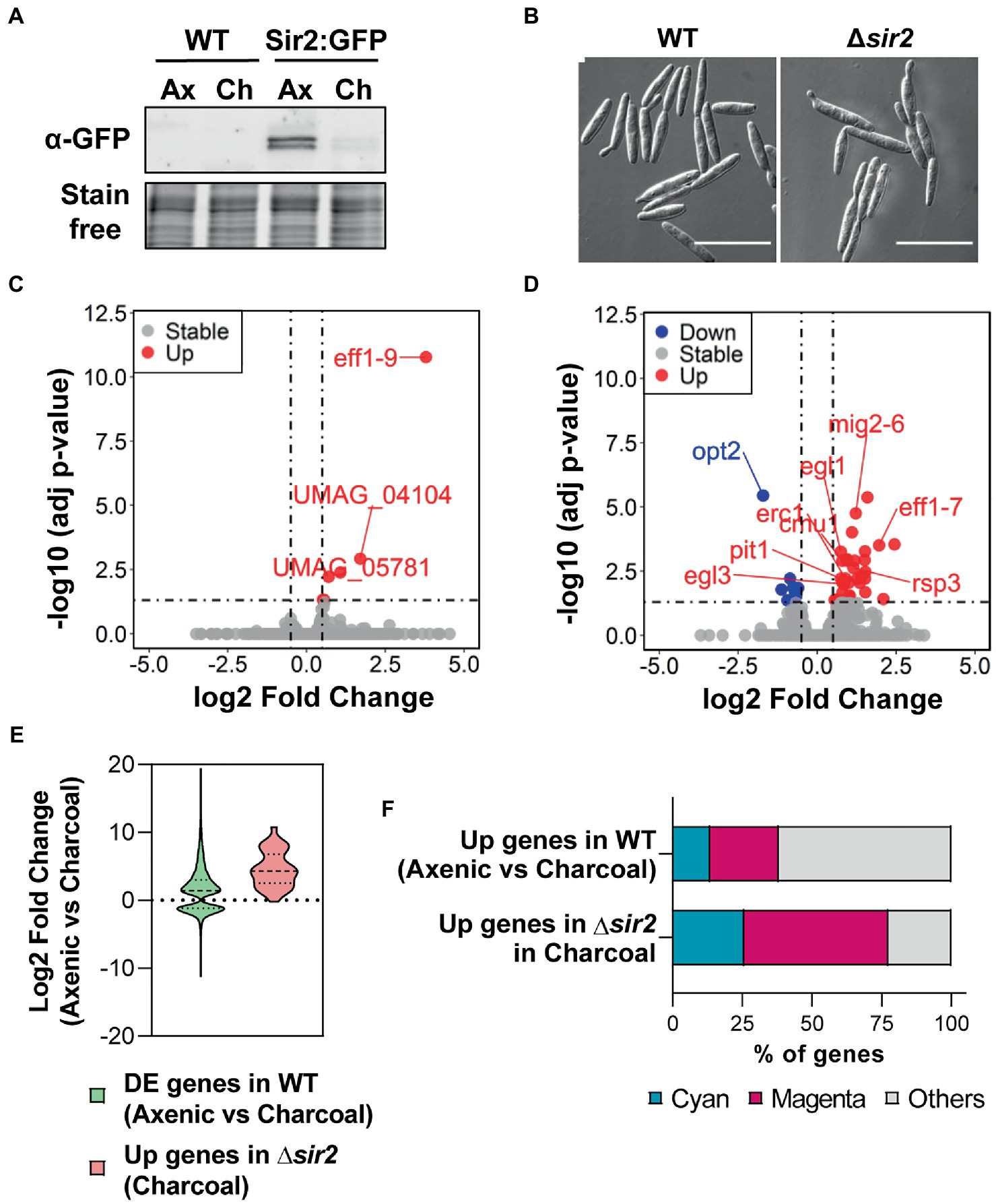
Figure 2. Sir2 is degraded during filamentation and is involved in the repression of a group of filamentation-induced genes. (A) Western blot showing Sir2:eGFP protein levels of wild-type strain. Total proteins were extracted from cells growing in axenic culture (Ax) and PD–charcoal plates (Ch) for 18 h at 25°C. Stain-free gel is shown as a loading control. (B) Images of wild-type and Δsir2 mutant growing in axenic culture. Scale bar represents 20 μm. (C,D) Volcano plot showing the log2 fold change in gene expression and the statistical significance of the differential expression analysis from RNA-seq data obtained for the Δsir2 mutant compared to wild-type in axenic culture (C) and PD–charcoal plates (D). Red, blue and grey dots represent the upregulated genes (log2 fold change ≥0.5, adjusted p-value < 0.05), downregulated genes (log2 fold change ≤ −0.5 adjusted p-value < 0.05) and genes without changes in the Δsir2 mutant, respectively. sir2 data has been removed for plotting purpose. (E) Log2 fold change distribution of the differentially expressed genes (adjusted p-value < 0.05) for wild-type in PD–charcoal plates compared to axenic culture and for the upregulated genes in the Δsir2 mutant compared to wild-type in PD–charcoal plates. (F) Percentage of genes belonging to the magenta (genes induced during the establishment and maintenance of biotrophism) or cyan modules (genes induced during tumor formation) of coexpressed genes throughout infection for the upregulated genes of wild-type in PD–charcoal plates compared to axenic culture and for the upregulated genes in the Δsir2 mutant compared to wild-type in PD–charcoal plates.
3.3. Sir2 overexpression drastically reduces infection capability
The observation that Sir2 affects the expression of genes that are induced during infection led us to study the role of Sir2 during plant infection. As the deletion of sir2 showed a slight increase in infected plant symptoms (Figure 1C), and Sir2 seemed to repress genes activated during infection (Figure 2F), we decided to study the effect of sir2 overexpression. First, we integrated one or more than one copy of the sir2 gene under the control of the constitutive otef promoter in the ip locus. No growth defects were detected in the overexpression mutant (Supplementary Figure S4A), however, as it can be observed in Supplementary Figures S4B–D, the filamentation capability was reduced according to the sir2 expression level found in both mutants. These data confirm the role of Sir2 in avoiding the proper induction of the filamentation process. Additionally, we overexpressed sir2 during pathogenesis by using the pit2 promoter, which reaches its expression peak at 2 dpi (Figure 4B). We infected maize plants with mutants harboring one or more than one copy of Ppit2:sir2, which did not show any significant defect in growth in different tested media (Figure 3A), and quantified the symptoms in infected plants. The size of tumors was clearly reduced when sir2 was significantly induced at 3 dpi (Figures 3B–D), indicating that Sir2 affects the infection process during plant colonization. When we analyzed the pathogenic defects of this mutant, we did not detect any alteration in fungi morphology during plant colonization (Supplementary Figure 5A). However, after 3 dpi, we detected a gradual reduction in fungal biomass during the progression of infection (Figure 4A). It is interesting to note that the reduction in fungal biomass was detected several days after the main overexpression of sir2, obtained at 2 dpi (Figure 4B). A possible explanation is that the overexpression of sir2 during the biotrophy establishment (2 dpi), affects the transcription of effector genes essential for this process or genes with roles from 4 dpi onward. Another alternative may be that the reduction in biomass observed (Figure 4A) is through an expression-independent effect of Sir2.
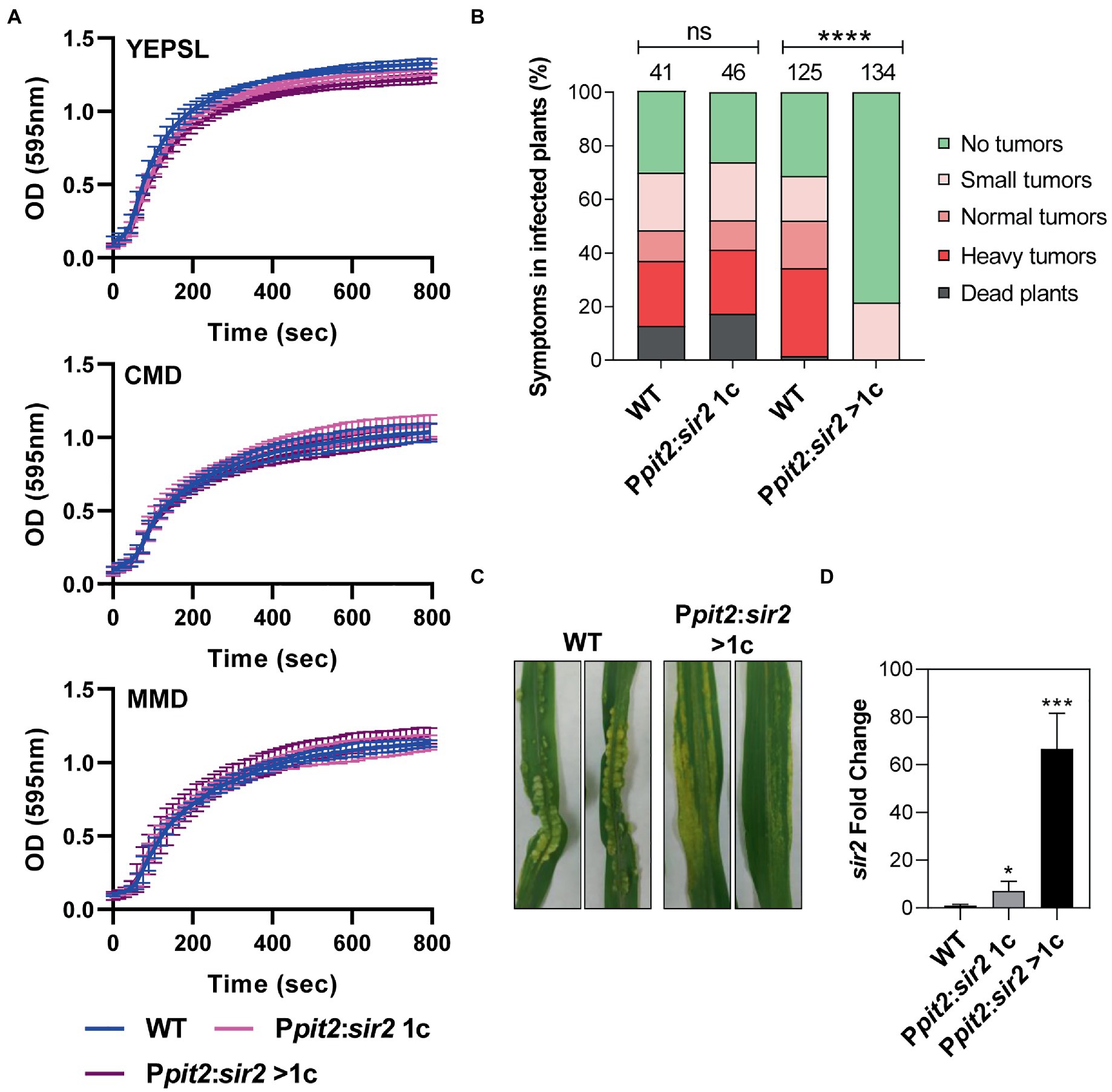
Figure 3. Sir2 overexpression reduces filamentation and virulence in U. maydis. (A) Growth curve of wild-type, Ppit2:sir2 1c and Ppit2:sir2 > 1c mutants growing in YEPSL, CMD or MMD media. Error bars represent the standard deviation from three independent replicates. (B) sir2 expression levels in axenic culture of wild-type and the sir2 overexpression mutants measured by RT-qPCR. U. maydis ppi1 was used as reference gene. Values were normalized to wild-type. Error bars represent the standard deviation from at least three independent replicates. Student’s t-test statistical analysis was performed (**p-value < 0.005). (C) Photograph of maize leaves infected with the indicated strains (D) sir2 expression levels of wild-type and the sir2 overexpression mutants containing one (1c) or more copies (>1c) of the Ppit2:sir2 construct infecting maize leaves at 3 dpi, measured by qPCR. U. maydis ppi1 was used as reference gene. Values were normalized to wild-type. Error bars represent the standard deviation from at least three independent replicates. Student’s t-test statistical analysis was performed (**p-value < 0.005).
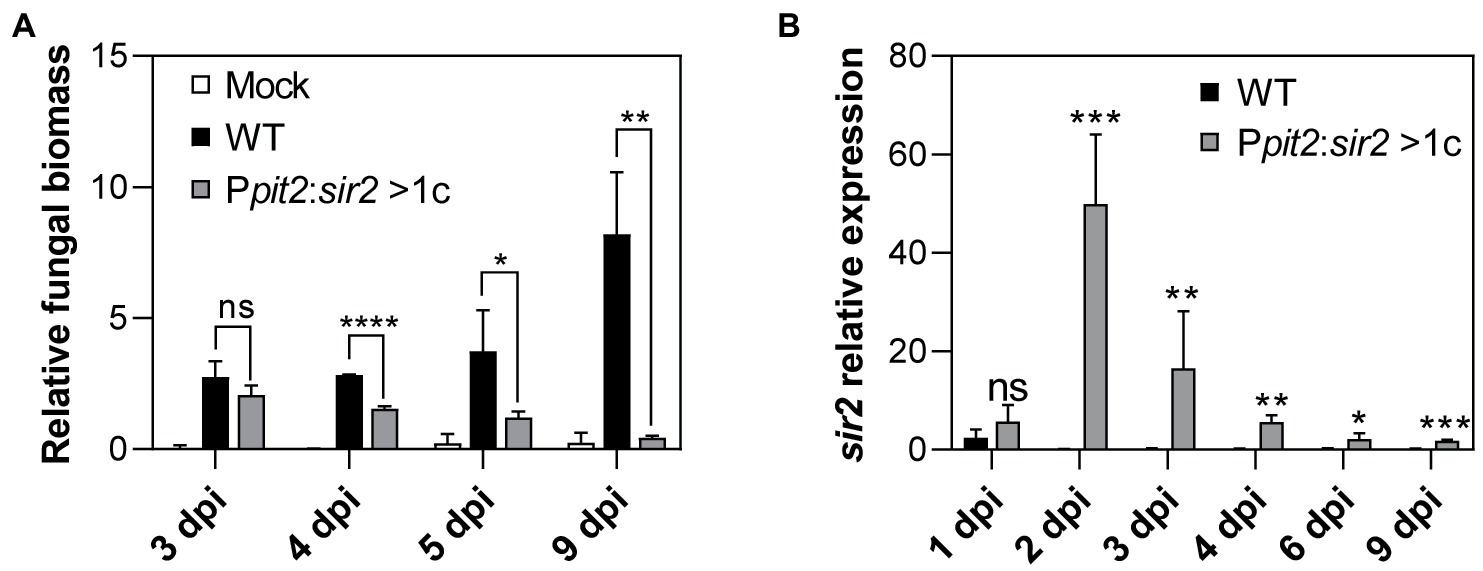
Figure 4. Progression inside the plant is impaired by the overexpression of sir2. (A) Relative fungal biomass was calculated by comparison between U. maydis ppi1 gene and Z. mays glyceraldehyde 3-phosphate dehydrogenase gene (GAPDH), measured by RT-qPCR of genomic DNA extracted from leaves infected with wild-type and Ppit2:sir2 > 1c mutant at 3, 4, 5, and 9 dpi. Error bars represent the standard deviation from three independent replicates. Student’s t-test statistical analysis was performed (ns, not significant, *p-value < 0.05, **p-value < 0.005, ****p-value < 0.0001). (B) sir2 expression of U. maydis wild-type and Ppit2:sir2 > 1c infecting maize leaves at 1, 2, 3, 4, 6, and 9 dpi, measured by RT-qPCR. U. maydis ppi1 was used as reference gene. Values were normalized to wild-type. Error bars represent the standard deviation from at least three independent replicates. Student’s t-test statistical analysis was performed (ns, not significant, *p-value < 0.05, **p-value < 0.005, ***p-value < 0.0005).
3.4. Sir2 prevents induction of a pool of virulence genes
To study the effect of Sir2 overexpression on gene transcription during infection, we carried out RNA-seq analysis of maize leaves infected with the wild-type strain or the mutant with more than one copy of Ppit2:sir2 at 3 dpi, when there was no significant change in fungal biomass and sir2 had been highly induced. We identified 51 genes downregulated and 39 upregulated in the mutant compared to the wild-type strain (Figure 5A; Supplementary Table S7), with the downregulated genes showing a stronger change in terms of differential expression. When we considered the distribution of transcriptional fold changes in a wild-type strain at 3 days post-infection in comparison to axenic conditions, the downregulated genes in the sir2-overexpressed mutant represented a small group of all genes induced during infection (Figure 5B; Supplementary Tables S7, S8). Interestingly, as observed in filamentation conditions (Figure 2F), we detected strong enrichment for genes belonging to the cyan (50%) and the magenta (40%) modules in this group of downregulated genes (Figure 5C; Supplementary Tables S7, S8). Although Sir2 avoided the full activation of mainly cyan and magenta genes, they were only a small subgroup of the entire modules (19 out of 558 magenta genes showed upregulation in our experiment at 3 dpi, and 26 out of 444 total cyan genes) (Supplementary Tables S7, S8). We wished to determine whether this group of genes has some specific expression profile during infection; therefore, we performed clustering analysis using the expression level of the cyan and magenta gene modules obtained from the RNA-seq data from Lanver et al. (2018). In the resulting heatmap, we marked the Sir2-repressed genes, observing that many of them were clustered together (Figure 5D), indicating that they share a similar expression profile. Specifically, they are genes repressed during the first few days of infection and are strongly induced at 4 dpi, several days after sir2 overexpression. These data may suggest that the overexpression of sir2 avoids the subsequent induction of a group of genes induced at 4 dpi, which could explain the decrease in fungal biomass that we observed from this day onwards (Figure 4A). However, it is necessary to exercise caution regarding our timing interpretation, as the data obtained by Lanver et al. (2018) were obtained in a different genetic background (FB1xFB2), and the timing of the infection process may not be the same as the one that we observed in an SG200 background, where we conducted the sir2 overexpression.
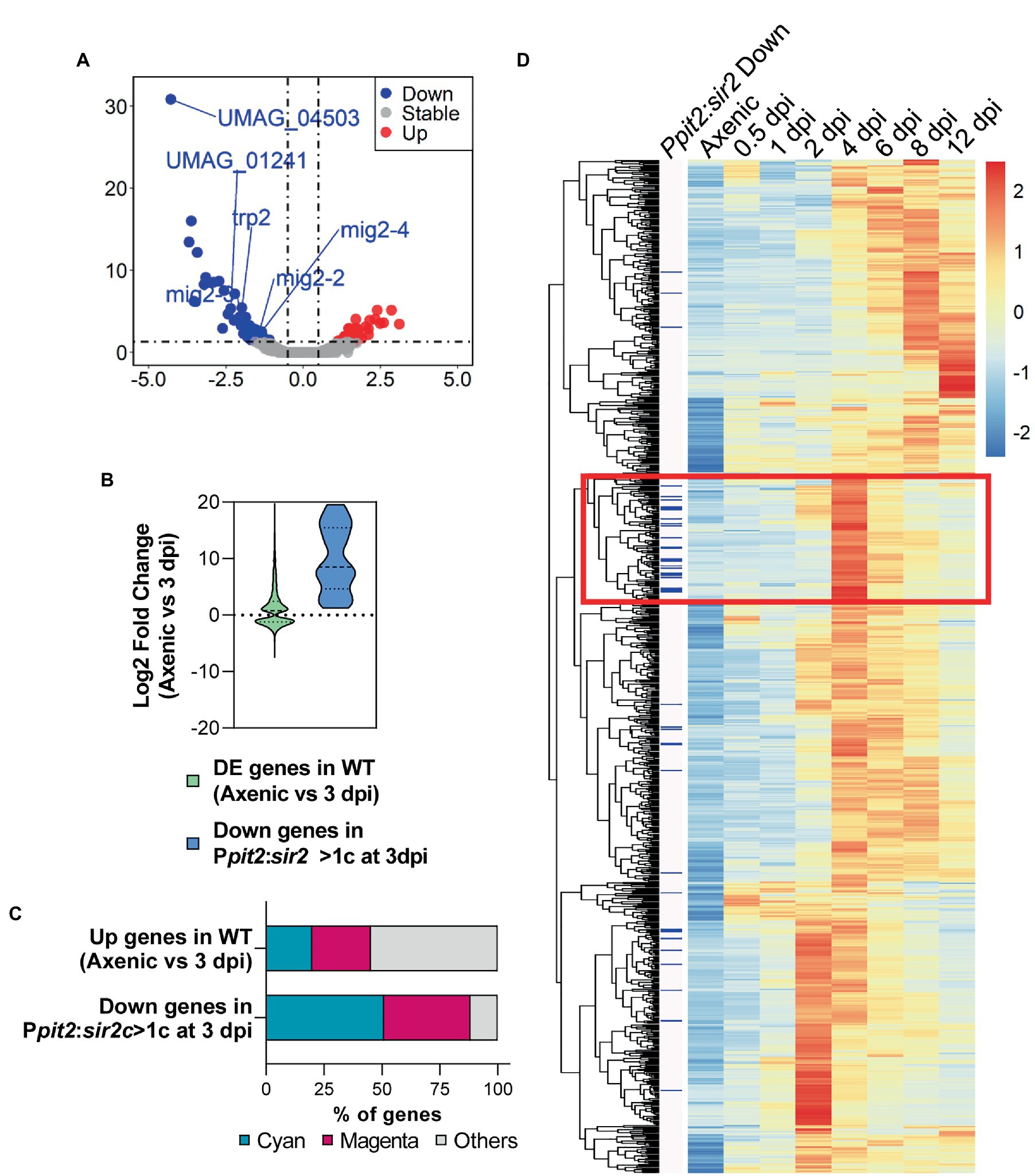
Figure 5. Overexpression of sir2 during pathogenesis avoids the proper activation of a subpopulation of genes related to biotrophism establishment and tumorigenesis. (A) Volcano plot showing the log2 fold change in gene expression and the statistical significance of the differential expression analysis from RNA-seq data obtained in the Ppit2:sir2 > 1c mutant compared to wild-type at 3 dpi. Red, blue and grey dots represent the upregulated genes (log2 fold change ≥0.5, adj p-value < 0.05), downregulated genes (log2 fold change ≤ −0.5 adj p-value < 0.05) and genes without changes in the Ppit2:sir2 > 1c mutant, respectively. sir2 and sdh2 (integration resistance cassette used for over expression mutant) data have been removed for plotting purpose. (B) Log2 fold change distribution of the differentially expressed genes (adj p-value < 0.05) at 3 dpi compared to axenic conditions in wild-type and the downregulated genes in the Ppit2:sir2 > 1c mutant compared to wild-type at 3dpi. (C) Percentage of genes belonging to the magenta or cyan modules of coexpressed genes during infection for the upregulated genes of wild-type at 3 dpi compared to axenic culture and for the downregulated genes in the Ppit2:sir2 > 1c mutant compared to wild-type at 3 dpi. (D) Clustering analysis of the expression profile of genes belonging to the cyan and magenta modules in axenic cultures and the indicated dpi for the wild-type strain (normalized counts obtained from the published RNA-seq data (Lanver et al., 2018). Heatmap color-scale values correspond to the Z-score transformation of the expression data, with +2 (red) being the highest expression and-2 (blue) being the lowest expression. Blue bars on the left indicate the downregulated genes in the Ppit2:sir2 > 1c mutant at 3 dpi compared to wild-type.
3.5. Δsir2 mutant shows increased acetylation of histone H4 at regulated genes
In order to check whether Sir2 controls filamentation and gene expression through histone deacetylation, we performed Western blot analysis using antibodies against acetylated histone 3 (H3ac) and histone 4 (H4ac), the canonical histone targets of Sir2 (Robyr et al., 2002; Suka et al., 2002; Vaquero et al., 2006; Shimizu et al., 2012; Cai et al., 2021; Zhao and Rusche, 2022), from total proteins extracted after growth in rich media (Figure 6A) and in filamentation induction media (Figure 6B). We did not detect any significant change for these two modifications in either a sir2 deletion or overexpression mutant (Figures 6A,B). Due to the effect observed in the sir2 mutants for specific loci rather than broad chromatin regions, we studied the acetylation state of different Sir2 regulated genes. To this aim, we carried out Chromatin Immunoprecipitation (ChIP) experiments using antibodies against H3ac and H4ac followed by RT-qPCR of the promoter region and the ORF of some of the genes that change their expression in the Δsir2 mutant in axenic culture (eff1-9) (Figure 2C; Supplementary Figure S3D; Supplementary Table S4), PD-charcoal plates (UMAG_06128, mig2-6, rsp3) (Figure 2D; Supplementary Table S5) and at 3 dpi in the sir2 overexpressed mutant (mig2-3, UMAG_01241) (Figure 5A; Supplementary Table S7). As observed in Figure 6C, there was an enrichment of acetylated H4 in eff1-9 and UMAG_06128 and a slight increase of acetylated H3 in the promoter region of eff1-9 in the Δsir2 mutant. As Sir2 commonly deacetylase lysine 16 in histone 4 (H4K16) (Robyr et al., 2002; Suka et al., 2002; Vaquero et al., 2006; Shimizu et al., 2012; Cai et al., 2021; Zhao and Rusche, 2022), we checked the H4K16 acetylation level by ChIP and RT-qPCR of the genes with enriched H4 acetylation, but no differences were observed in the acetylation of this residue (Figure 6D). Although lysine 14 is the main target of Sir2 in histone H4, other lysine has been observed to be deacetylated by Sir2 in different fungi (Lai et al., 2022). Thus, we cannot discard a different lysine residue as the histone target of Sir2 in U. maydis. Alternatively, as histone acetylation has been described to be dependent on transcription level (Martin et al., 2021), the increase in histone acetylation may be consequence and not the cause of the gene upregulation and the defects observed in filamentation and gene regulation in this work may be due to a deacetylation-independent function of Sir2 or the deacetylation of a different target. As a first approach to this idea, we have performed mass spectrometry analysis of a functional Sir2:eGFP (Figure 7A) pull down and found, among others, a transcription factor involved in virulence, Med1 (Chacko and Gold, 2012), to interact with Sir2. Additional interesting interactors found in our analysis are the mitogen-activated protein kinase (MAPK) Mkk1 involved in cell wall integrity signaling (Carbó and Pérez-Martín, 2010) and five uncharacterized proteins (Figure 7B).
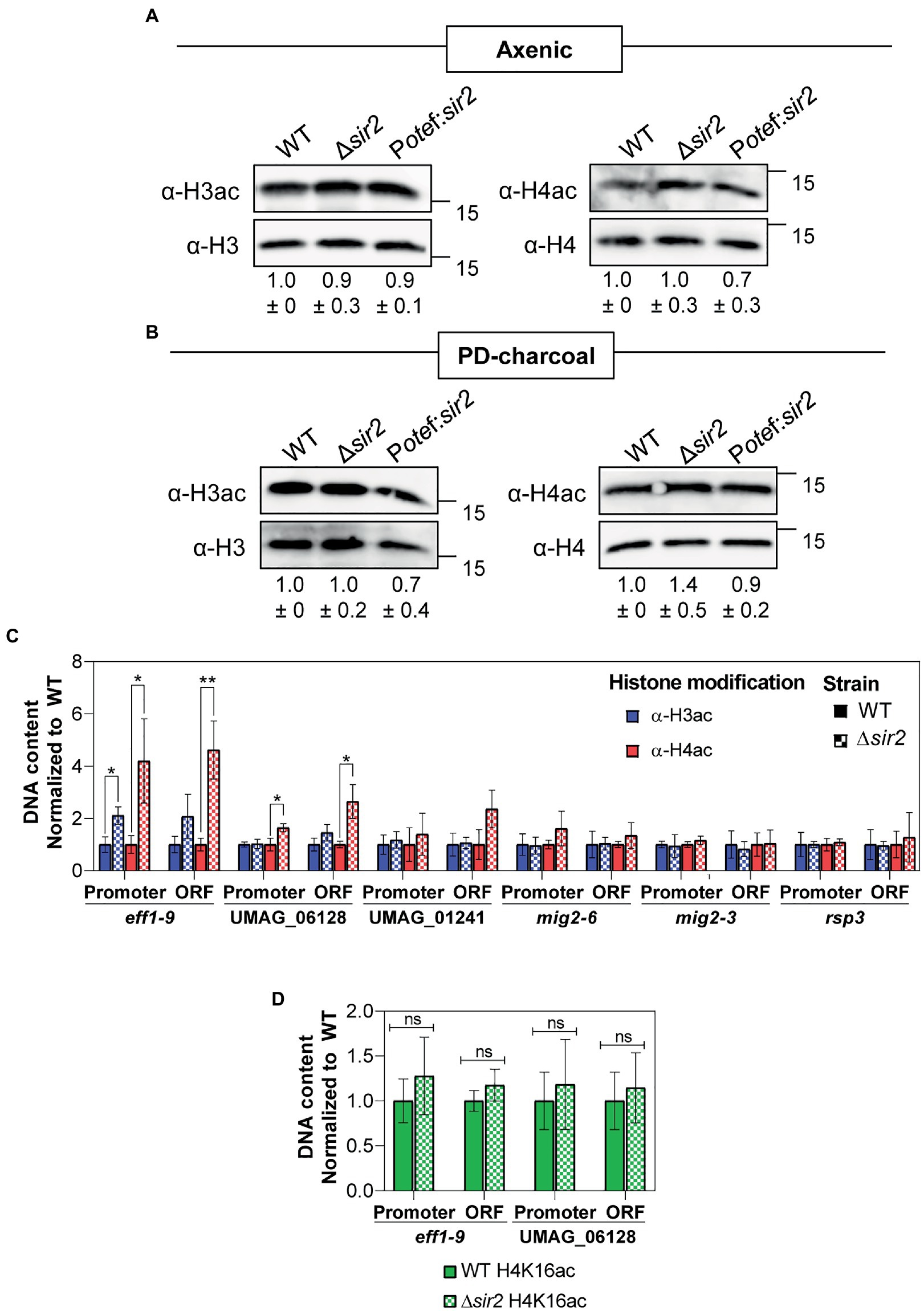
Figure 6. Effect of sir2 mutants in histone acetylation. (A,B) Total proteins extracted from axenic culture (A) or PD-charcoal plates (B) of wild-type, Δsir2 and the Potef:sir2 > 1c strains were used for Western blotting. The H3ac and H4ac antibodies were used to detect histone acetylation and H3 and H4 antibodies were used as loading controls. H3ac and H4ac signals were normalized to H3 and H4 levels, respectively, and compared to wild-type from three independent replicates. (C,D) ChIP analysis using H3ac and H4ac antibodies (C) or H4K16ac antibody (D) on chromatin extracts from axenic culture of wild-type and Δsir2 strains. Immunoprecipitated DNA was analyzed by RT-qPCR, amplifying regions within the promoter and the open reading frame (ORF) of the indicated gene. Values correspond to the amount of DNA recovered in the IP relative to ppi1 gene. Values were normalized to wild-type. Error bars represent the standard deviation from at least three independent replicates. Student’s t-test statistical analysis was performed (ns, no significant, *p-value < 0.05, **p-value < 0.005).
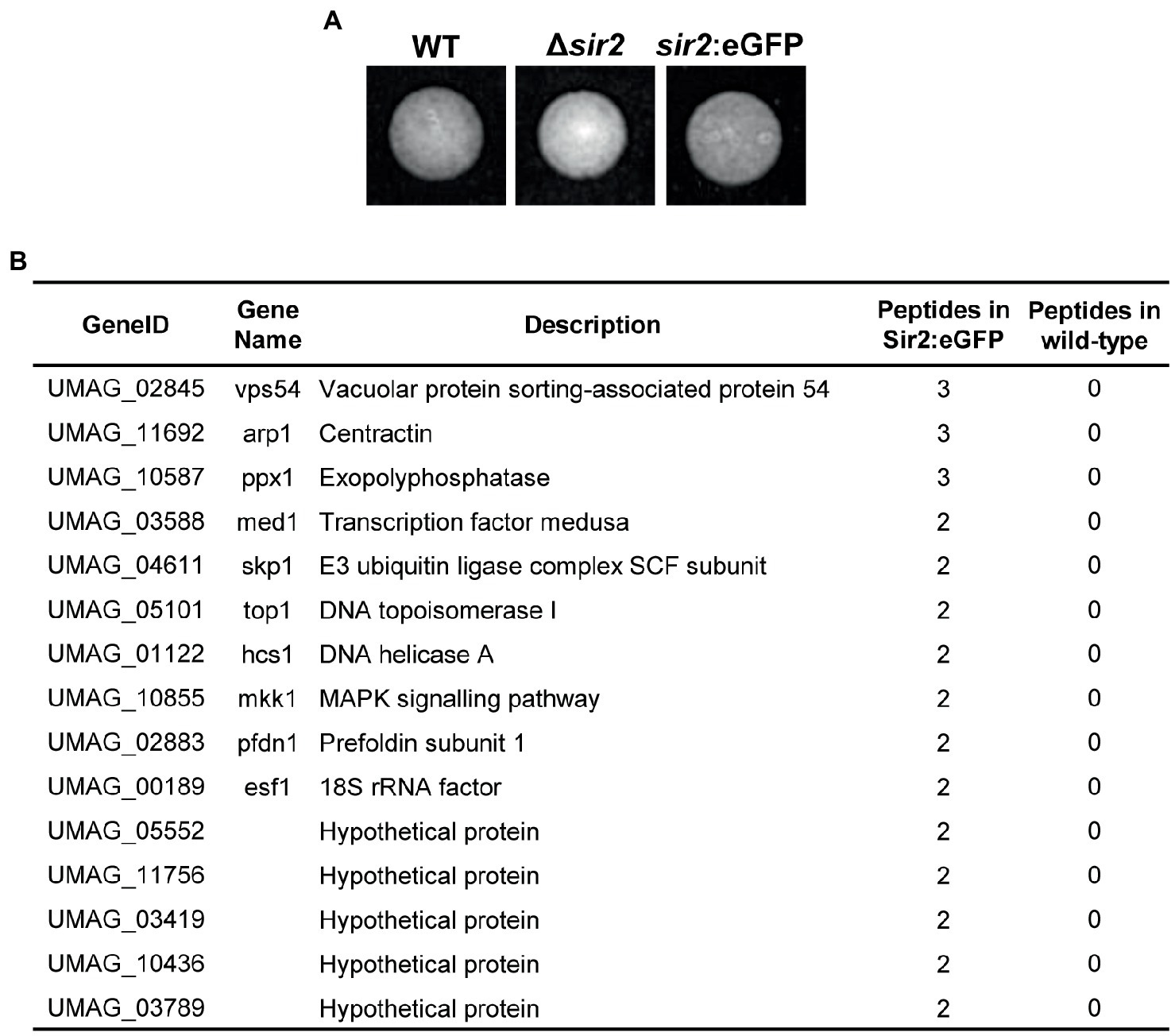
Figure 7. Sir2 interacting proteins. (A) Sir2 fussed to eGFP does not affect its function. Images showing the filamentation of wild-type, and the indicated mutants on PD-charcoal plates for 18 h at 25°C. (B) Proteins interacting with Sir2. Identified proteins by Sir2 immunoprecipitation followed by mass spectrometry analysis. Proteins with log2 > 6 and, at least, 2 peptide in all replicates of the sir2:eGFP mutant and none in the wild-type are shown.
4. Discussion
Sirtuins are NAD+-dependent deacetylases with important regulatory roles in processes such as lifespan, metabolic control or pathogenesis in fungi. As they require the NAD+ cofactor for their activity, sirtuins may serve as regulators of many of these processes in response to metabolic stages. Here, we have performed a systematic analysis of all sirtuins present in U. maydis and focused on the nuclear sirtuin Sir2, demonstrating its role in the control of part of the pathogenic program.
4.1. The sirtuin repertoire of Ustilago maydis
Fungal sirtuins can be classified into five principal subfamilies according to their orthologs in other organisms: Sir2/Hst1, Hst2, Hst3/4, SirT4 and SirT5 (Zhao and Rusche, 2022). Interestingly, U. maydis harbors a sirtuin member for each subfamily (Elías-Villalobos et al., 2019). The SirT4 and SirT5 subfamilies are mitochondrial sirtuins with not many examples described in fungi. We have demonstrated here that the two members of these families in U. maydis, Hst5 and Hst6, display mitochondrial localization. However, we have not detected any essential role for them during pathogenesis. On the other hand, Hst2 has been described to show a primary cytoplasmatic localization, although it is involved in locus-specific silencing (Halme et al., 2004; Durand-Dubief et al., 2007) and has a prominent function in chromosome condensation during mitosis (Vaquero et al., 2006; Wilkins et al., 2014; Kruitwagen et al., 2018; Jain et al., 2021). This conflict between location and function may be explained in mammalian cells since Hst2 can move from the cytoplasm to the nucleus, mainly in premitotic cells (Vaquero et al., 2006; Wilson et al., 2006). Similarly to mammalian cells, we have observed that U. maydis Hst2 is mainly cytoplasmic, but it is transported to the nucleus in premitotic cells and it remains bound to chromatin during mitosis. This observation strongly supports the idea that in U. maydis, Hst2 conserves its role in chromatin condensation during mitosis, probably via the deacetylation of H4K16, as in yeast and mammals (Vaquero et al., 2006; Wilkins et al., 2014). Finally, we found Hst4 and Sir2, members of the Hst3/4 and Sir2/Hst1 subfamilies, respectively, both with constitutive nuclear localization. Although Hst4, as well as the other member of the subfamily, Hst3, may be involved in the transcriptional silencing of a specific locus and heterochromatin (Freeman-Cook et al., 1999; Durand-Dubief et al., 2007), the main function described is their role in chromosome integrity through H3K56 deacetylation. Consequently, cells with a misregulation of these sirtuins show genome stability-related phenotypes such as spontaneous DNA damage, chromosome loss or sensitivity to DNA damage (Celic et al., 2006; Maas et al., 2006; Haldar and Kamakaka, 2008). Although mutants for hst3 and hst4 are viable in S. cerevisiae and S. pombe, the deletion of the single member of this subfamily in Candida albicans, hst3, which has been demonstrated to be involved in H3K56 deacetylation, has not been possible (Wurtele et al., 2010), suggesting that this gene is essential in this yeast. Here, we have demonstrated that the deletion of hst4 in U. maydis is lethal, which supports again a conserved role of Hst4 in this fungus in genome stability through the acetylation of H3K56. The other nuclear sirtuin in U. maydis is Sir2. This is the founding member of the family and has been extensively characterized in different organisms. The main described role for this sirtuin is transcriptional silencing through histone deacetylation, particularly of H4K16 and H3K9 (Robyr et al., 2002; Suka et al., 2002; Vaquero et al., 2006; Shimizu et al., 2012; Cai et al., 2021; Zhao and Rusche, 2022). The most recognized biological function for this sirtuin is the regulation of aging (Kaeberlein et al., 1999; Fabrizio et al., 2005; Fu et al., 2008; Bouklas et al., 2017). However, in the human pathogens Candida glabrata (Domergue et al., 2005) and Cryptococcus neoformans (Arras et al., 2017), in the insect pathogen Beauveria bassiana (Cai et al., 2021) and in the plant pathogen M. oryzae (Fernandez et al., 2014), it has been demonstrated to have implications for the virulence process. In M. oryzae, thus far, the only phytopathogen with an in-depth characterization of Sir2, this sirtuin controls virulence through deacetylation of the transcriptional repressor Jmjc, which allows the expression of the superoxide dismutase Sod1, with important roles in ROS detoxification during the first few steps of infection (Fernandez et al., 2014). Here, we provide another example of the role of Sir2 in a phytopathogen, now a basidiomycete, where this sirtuin affects the regulation of a subset of the virulence genes. We have observed that the overexpression of sir2 reduces the filamentation capability and inhibits the correct induction of virulence genes, affecting proper tumor formation. In contrast, the deletion of sir2 causes an increase in the filamentation and infection capabilities and allows a better induction of filamentation and virulence genes. Interestingly, Sir2 affects filamentation as well in the human pathogen C. albicans (Zhao and Rusche, 2021). However, the effect is the reverse, as Sir2 is essential for proper filamentation in this yeast. Further examples of the role of Sir2 in filamentation in different fungi would be useful to verify the possible conserved role of Sir2 during this process.
4.2. The role of UmSir2 in transcriptional regulation
Sir2 has a transcriptional silencing role conserved through evolution (Hickman et al., 2011). In high eukaryotes and yeast such as S. pombe, with the hallmarks of high eukaryotes and heterochromatin (methylation of H3K9, Heterochromatin Protein 1 (HP1) and RNA interference (RNAi) to produce the heterochromatin silencing platform), Sir2 has been described to aid in the silencing of this region through the deacetylation of histones, mainly H4K16 and H3K9 (Shankaranarayana et al., 2003). However, U. maydis lacks all these hallmarks of heterochromatin formation. A similar situation is found in S. cerevisiae, where RNAi, H3K9 methylation and HP1 proteins are not present (Hickman et al., 2011). In this organism, Sir2, as part of the SIR complex, together with Sir3 and 4, is the main factor involved in the formation of heterochromatin-like regions (Robyr et al., 2002; Suka et al., 2002). It is tempting to believe that in U. maydis, with a similar chromatin scenario, Sir2 would have a similar central role in the silencing of heterochromatin-like regions. However, in U. maydis, we did not find homologs of the other members of the SIR complex, which are required for the spreading effect required for heterochromatin formation (Elías-Villalobos et al., 2019). In addition, in our mass spectrometry analysis we have not detected any factor belonging to a putative SIR complex. Thus, we observe a different scenario in U. maydis, with no hallmarks of heterochromatin and no apparent SIR complex. Nevertheless, the low evolutionary conservation of Sir3 and Sir4 proteins (Hickman et al., 2011), keeps the door open to some of the four hypothetical proteins found on the mass spectrometry analysis to be members of a possible SIR complex in U. maydis. Our RNA-seq studies reveal that Sir2 seems to not be an essential factor for heterochromatin-like silencing in this organism, as we have not found a clear effect in the characteristic heterochromatin regions, such as telomeres or centromeres, or in clusters of coregulated genes, which would be putative chromatin-silenced areas. However, we have found a direct or indirect regulatory effect of Sir2, mainly in silencing, in specific loci. This is consistent with the regulatory effect observed for different sirtuins, including Sir2, and in the general silencing effect observed through evolution (Zhao and Rusche, 2022).
An interesting observation is that Sir2 is degraded during filamentation, and we were able to detect two bands of this protein by Western blot (Figure 2A). A tentative speculation is that a posttranslational modification (ptm) of Sir2 during the pathogenic program leads to its degradation and/or inactivation, allowing the proper expression of a pool of virulence genes. Sir2 has been described in yeast to suffer phosphorylation and sumoylation, altering its function (Hannan et al., 2015; Kang et al., 2015). In M. oryzae, Sir2 accumulation during infection is controlled through ubiquitination by the E3 ubiquitin ligase Upl3, and basal Sir2 levels are controlled by the Grr1 (Patton et al., 1998) and Ptr1 ones (Li et al., 2020). Interestingly, we found UMAG_04611, a homolog of the E3 ubiquitin ligase Skp1, to interact with Sir2 in our mass spectrometry analysis. Further analysis would be interesting to confirm the biological significance of this interaction and the possible role of ubiquitination or other Sir2 modifications in U. maydis filamentation and virulence.
4.3. Possible substrates of Sir2
As mentioned previously, Sir2 represses the transcription of specific substrates or mediates the silencing of heterochromatin regions by the deacetylation of histones, typically H4K16 and H3K9 (Robyr et al., 2002; Suka et al., 2002; Vaquero et al., 2006; Shimizu et al., 2012; Cai et al., 2021; Zhao and Rusche, 2022). In U. maydis, in agreement with the observed repressive effect in specific loci for Sir2, we have not detected any significant change in acetylation for either H3 or H4 by Western blot. However, there is a significant increase of mainly H4 acetylation when specific regulation targets of Sir2 were studied by ChIP and RT-qPCR. Interestingly, we have not observed an increase in acetylation for the specific lysine 16. As Sir2 is able to deacetylate other lysine residues in different fungi (Lai et al., 2022), we cannot discard the possibility of a different lysine residue as the target for Sir2. However, other possibility is that Sir2 has a non-histone target in U. maydis. If this is the case, the increase in histone acetylation observed in the Δsir2 mutant should be a consequence of the upregulation of the genes and not the cause. In agreement with this possibility, it has been recently described that RNA polymerase II promotes the recruitment and activity of histone acetyltransferases (Martin et al., 2021), which explain an increase of histone acetylation as a consequence of a transcriptional de-repression. Sir2 has been already demonstrated to have non-histone targets in different fungi. In the insect pathogen B. bassiana, acetylation analysis has revealed that, besides H4 and H3, hundreds of proteins show an altered acetylation pattern in a sir2 mutant (Cai et al., 2021). More specifically, in plant pathogens, Sir2 has been demonstrated to affect the virulence of M. orizae by deacetylation of a transcription factor (Fernandez et al., 2014). In our mass spectrometry analysis, we found the transcription factor Med1 to interact with Sir2. This transcription factor acts upstream of the virulence master regulator Prf1, affecting its expression and virulence (Chacko and Gold, 2012). Although we have not found any significant defect in prf1 expression on a sir2 mutant, further investigation would be necessary to study a possible regulatory effect of Sir2 over the Med1 transcription factor and other possible substrates that could explain more in depth the filamentation and transcriptional defects observed on the sir2 mutant in U. maydis. Lastly, we cannot discard the possibility of a deacetylase independent function of Sir2 in U. maydis. Future experiments mutating the catalytic domain should clarify this possibility.
Data availability statement
The datasets presented in this study can be found in online repositories. The names of the repository/repositories and accession number(s) can be found at: https://www.ncbi.nlm.nih.gov/genbank/, GSE226596.
Author contributions
RB and JI designed the concept of the study and supervised the project. BN performed the experiment. RB and BN analyzed the data and drafted the manuscript. All authors contributed to the article and approved the submitted version.
Funding
This research was supported by MCIN/AEI/10.13039/501100011033/FEDER, grant numbers BIO2016-80180-P and PID2019-110477GB-I00 to JII. BN was awarded by grant BES-2017-079765 MCIN/AEI/FEDER.
Acknowledgments
We thank the Genetics Department for their useful discussions and comments; Víctor Manuel Carranco, Sandra Romero, Laura Tomás, and Ismael Fernández Portillo for technical support.
Conflict of interest
The authors declare that the research was conducted in the absence of any commercial or financial relationships that could be construed as a potential conflict of interest.
Publisher’s note
All claims expressed in this article are solely those of the authors and do not necessarily represent those of their affiliated organizations, or those of the publisher, the editors and the reviewers. Any product that may be evaluated in this article, or claim that may be made by its manufacturer, is not guaranteed or endorsed by the publisher.
Supplementary material
The Supplementary material for this article can be found online at: https://www.frontiersin.org/articles/10.3389/fmicb.2023.1157990/full#supplementary-material
References
Aichinger, C., Hansson, K., Eichhorn, H., Lessing, F., Mannhaupt, G., Mewes, W., et al. (2003). Identification of plant-regulated genes in Ustilago maydis by enhancer-trapping mutagenesis. Mol. Gen. Genomics. 270, 303–314. doi: 10.1007/s00438-003-0926-z
Allfrey, V. G., Faulkner, R., and Mirsky, A. E. (1964). Acetylation and methylation of histones and their role in the regulation of RNA synthesis. Proc. Natl. Acad. Sci. U. S. A. 51, 786–794. doi: 10.1073/pnas.51.5.786
Arras, S. D. M., Chitty, J. L., Wizrah, M. S. I., Erpf, P. E., Schulz, B. L., Tanurdzic, M., et al. (2017). Sirtuins in the phylum Basidiomycota: a role in virulence in Cryptococcus neoformans. Sci. Rep. 7:46567. doi: 10.1038/srep46567
Badeaux, A. I., and Shi, Y. (2013). Emerging roles for chromatin as a signal integration and storage platform. Nat. Rev. Mol. Cell Biol. 14, 211–224. doi: 10.1038/nrm3545
Becht, P., König, J., and Feldbrügge, M. (2006). The RNA-binding protein Rrm4 is essential for polarity in Ustilago maydis and shuttles along microtubules. J. Cell Sci. 119, 4964–4973. doi: 10.1242/jcs.03287
Bortfeld, M., Auffarth, K., Kahmann, R., and Basse, C. W. (2004). The Ustilago maydis a2 mating-type locus genes lga2 and rga2 compromise pathogenicity in the absence of the mitochondrial p32 family protein Mrb1. Plant Cell 16, 2233–2248. doi: 10.1105/tpc.104.022657
Bouklas, T., Jain, N., and Fries, B. C. (2017). Modulation of replicative lifespan in Cryptococcus neoformans: implications for virulence. Front. Microbiol. 8:98. doi: 10.3389/fmicb.2017.00098
Brachmann, A., König, J., Julius, C., and Feldbrügge, M. (2004). A reverse genetic approach for generating gene replacement mutants in Ustilago maydis. Mol. Gen. Genomics. 272, 216–226. doi: 10.1007/S00438-004-1047-Z/FIGURES/4
Brachmann, C. B., Sherman, J. M., Devine, S. E., Cameron, E. E., Pillus, L., and Boeke, J. D. (1995). The SIR2 gene family, conserved from bacteria to humans, functions in silencing, cell cycle progression, and chromosome stability. Genes Dev. 9, 2888–2902. doi: 10.1101/gad.9.23.2888
Brachmann, A., Weinzierl, G., Kamper, J., and Kahmann, R. (2001). Identification of genes in the bW/bE regulatory cascade in Ustilago maydis. Mol. Microbiol. 42, 1047–1063. doi: 10.1046/j.1365-2958.2001.02699.x
Brefort, T., Doehlemann, G., Mendoza-Mendoza, A., Reissmann, S., Djamei, A., and Kahmann, R. (2009). Ustilago maydis as a pathogen. Annu. Rev. Phytopathol. 47, 423–445. doi: 10.1146/annurev-phyto-080508-081923
Brefort, T., Tanaka, S., Neidig, N., Doehlemann, G., Vincon, V., and Kahmann, R. (2014). Characterization of the largest effector gene cluster of Ustilago maydis. PLoS Pathog. 10:e1003866. doi: 10.1371/journal.ppat.1003866
Cai, Q., Tian, L., Xie, J., Huang, Q., Feng, M., and Keyhani, N. O. (2021). A fungal sirtuin modulates development and virulence in the insect pathogen, Beauveria bassiana. Environ. Microbiol. 23, 5164–5183. doi: 10.1111/1462-2920.15497
Carbó, N., and Pérez-Martín, J. (2010). Activation of the cell wall integrity pathway promotes escape from G2 in the fungus Ustilago maydis. PLoS Genet. 6:e1001009. doi: 10.1371/journal.pgen.1001009
Castanheira, S., and Pérez-Martín, J. (2015). Appressorium formation in the corn smut fungus Ustilago maydis requires a G2 cell cycle arrest. Plant Signal. Behav. 10:e1001227. doi: 10.1080/15592324.2014.1001227
Celic, I., Masumoto, H., Griffith, W. P., Meluh, P., Cotter, R. J., Boeke, J. D., et al. (2006). The Sirtuins Hst3 and Hst4p preserve genome integrity by controlling histone H3 lysine 56 deacetylation. Curr. Biol. 16, 1280–1289. doi: 10.1016/j.cub.2006.06.023
Chacko, N., and Gold, S. (2012). Deletion of the Ustilago maydis ortholog of the aspergillus sporulation regulator medA affects mating and virulence through pheromone response. Fungal Genet. Biol. 49, 426–432. doi: 10.1016/j.fgb.2012.04.002
de Ruijter, A. J. M, Gennip, A. H.Van, Caron, H. N., Kemp, S., and Kuilenburg, A. B. P. Van (2003). Histone deacetylases (HDACs): characterization of the classical HDAC family. Biochem. J. 370, 737–749. doi: 10.1042/bj20021321
Djamei, A., Schipper, K., Rabe, F., Ghosh, A., Vincon, V., Kahnt, J., et al. (2011). Metabolic priming by a secreted fungal effector. Nature 478, 395–398. doi: 10.1038/nature10454
Doehlemann, G., Reissmann, S., Aßmann, D., Fleckenstein, M., and Kahmann, R. (2011). Two linked genes encoding a secreted effector and a membrane protein are essential for Ustilago maydis-induced tumour formation. Mol. Microbiol. 81, 751–766. doi: 10.1111/j.1365-2958.2011.07728.x
Doehlemann, G., Wahl, R., Vranes, M., de Vries, R. P., Kämper, J., and Kahmann, R. (2008). Establishment of compatibility in the Ustilago maydis/maize pathosystem. J. Plant Physiol. 165, 29–40. doi: 10.1016/j.jplph.2007.05.016
Domergue, R., Castaño, I., De Las Peñas, A., Zupancic, M., Lockatell, V., Hebel, J. R., et al. (2005). Nicotinic acid limitation regulates silencing of Candida adhesins during UTI. Science 308, 866–870. doi: 10.1126/science.1108640
Durand-Dubief, M., Sinha, I., Fagerström-Billai, F., Bonilla, C., Wright, A., Grunstein, M., et al. (2007). Specific functions for the fission yeast Sirtuins Hst2 and Hst4 in gene regulation and retrotransposon silencing. EMBO J. 26, 2477–2488. doi: 10.1038/sj.emboj.7601690
Elías-Villalobos, A., Barrales, R. R., and Ibeas, J. I. (2019). Chromatin modification factors in plant pathogenic fungi: insights from Ustilago maydis. Fungal Genet. Biol. 129, 52–64. doi: 10.1016/j.fgb.2019.04.006
Elías-Villalobos, A., Fernández-Álvarez, A., Moreno-Sánchez, I., HelmLinger, D., and Ibeas, J. I. (2015). The Hos2 histone deacetylase controls Ustilago maydis virulence through direct regulation of mating-type genes. PLoS Pathog. 11:e1005134. doi: 10.1371/journal.ppat.1005134
Fabrizio, P., Gattazzo, C., Battistella, L., Wei, M., Cheng, C., McGrew, K., et al. (2005). Sir2 blocks extreme life-span extension. Cells 123, 655–667. doi: 10.1016/j.cell.2005.08.042
Farfsing, J. W., Auffarth, K., and Basse, C. W. (2005). Identification of cis-active elements in Ustilago maydis mig2 promoters conferring high-level activity during pathogenic growth in maize. Mol. Plant-Microbe Interact. 18, 75–87. doi: 10.1094/MPMI-18-0075
Fernandez, J., Marroquin-Guzman, M., Nandakumar, R., Shijo, S., Cornwell, K. M., Li, G., et al. (2014). Plant defence suppression is mediated by a fungal sirtuin during rice infection by Magnaporthe oryzae. Mol. Microbiol. 94, 70–88. doi: 10.1111/mmi.12743
Freeman-Cook, L. L., Sherman, J. M., Brachmann, C. B., Allshire, R. C., Boeke, J. D., and Pillus, L. (1999). The Schizosaccharomyces pombe hst4+ gene is a SIR2 homologue with silencing and centromeric functions. Mol. Biol. Cell 10, 3171–3186. doi: 10.1091/mbc.10.10.3171
Fu, X.-H., Meng, F.-L., Hu, Y., and Zhou, J.-Q. (2008). Candida albicans, a distinctive fungal model for cellular aging study. Aging Cell 7, 746–757. doi: 10.1111/j.1474-9726.2008.00424.x
Gillissen, B., Bergemann, J., Sandmann, C., Schroeer, B., Bölker, M., and Kahmann, R. (1992). A two-component regulatory system for self/non-self recognition in Ustilago maydis. Cells 68, 647–657. doi: 10.1016/0092-8674(92)90141-X
González-Prieto, J. M., Domínguez, A., Rosas-Quijano, R., Cervantes-Chávez, J. A., and Ruiz-Herrera, J. (2004). Isolation and molecular analysis of Umhda2 a gene encoding a histone deacetylase from Ustilago maydis. DNA Seq. 15, 44–50. doi: 10.1080/10425170310001652192
González-Prieto, J. M., Rosas-Quijano, R., Domínguez, A., and Ruiz-Herrera, J. (2014). The UmGcn5 gene encoding histone acetyltransferase from Ustilago maydis is involved in dimorphism and virulence. Fungal Genet. Biol. 71, 86–95. doi: 10.1016/j.fgb.2014.09.002
Haldar, D., and Kamakaka, R. T. (2008). Schizosaccharomyces pombe Hst4 functions in DNA damage response by regulating histone H3 K56 acetylation. Eukaryot. Cell 7, 800–813. doi: 10.1128/EC.00379-07
Halme, A., Bumgarner, S., Styles, C., and Fink, G. R. (2004). Genetic and epigenetic regulation of the FLO gene family generates cell-surface variation in yeast. Cells 116, 405–415. doi: 10.1016/S0092-8674(04)00118-7
Hannan, A., Abraham, N. M., Goyal, S., Jamir, I., Priyakumar, U. D., and Mishra, K. (2015). Sumoylation of Sir2 differentially regulates transcriptional silencing in yeast. Nucleic Acids Res. 43, gkv842–g10226. doi: 10.1093/nar/gkv842
Hartmann, H. A., Kahmann, R., and Bölker, M. (1996). The pheromone response factor coordinates filamentous growth and pathogenicity in Ustilago maydis. EMBO J. 15, 1632–1641. doi: 10.1002/j.1460-2075.1996.tb00508.x
Hartmann, H. A., Krüger, J., Lottspeich, F., and Kahmann, R. (1999). Environmental signals controlling sexual development of the corn smut fungus Ustilago maydis through the transcriptional regulator Prf1. Plant Cell 11, 1293–1305. doi: 10.1105/tpc.11.7.1293
Heimel, K., Scherer, M., Vranes, M., Wahl, R., Pothiratana, C., Schuler, D., et al. (2010). The transcription factor Rbf1 is the master regulator for b-mating type controlled pathogenic development in Ustilago maydis. PLoS Pathog. 6:e1001035. doi: 10.1371/journal.ppat.1001035
Hickman, M. A., Froyd, C. A., and Rusche, L. N. (2011). Reinventing heterochromatin in budding yeasts: Sir2 and the origin recognition complex take center stage. Eukaryot. Cell 10, 1183–1192. doi: 10.1128/EC.05123-11
Itoh, E., Shigemoto, R., Oinuma, K.-I., Shimizu, M., Masuo, S., and Takaya, N. (2017). Sirtuin a regulates secondary metabolite production by aspergillus nidulans. J. Gen. Appl. Microbiol. 63, 228–235. doi: 10.2323/jgam.2016.11.002
Jackson, M. D., and Denu, J. M. (2002). Structural identification of 2′- and 3′-O-acetyl-ADP-ribose as novel metabolites derived from the Sir2 family of β-NAD+-dependent histone/protein deacetylases. J. Biol. Chem. 277, 18535–18544. doi: 10.1074/jbc.M200671200
Jain, N., Janning, P., and Neumann, H. (2021). 14-3-3 protein Bmh1 triggers short-range compaction of mitotic chromosomes by recruiting sirtuin deacetylase Hst2. J. Biol. Chem. 296:100078. doi: 10.1074/jbc.AC120.014758
Kaeberlein, M., McVey, M., and Guarente, L. (1999). The SIR2/3/4 complex and SIR2 alone promote longevity in Saccharomyces cerevisiae by two different mechanisms. Genes Dev. 13, 2570–2580. doi: 10.1101/gad.13.19.2570
Kämper, J. (2004). A PCR-based system for highly efficient generation of gene replacement mutants in Ustilago maydis. Mol. Gen. Genomics. 271, 103–110. doi: 10.1007/s00438-003-0962-8
Kämper, J., Kahmann, R., Bölker, M., Ma, L.-J., Brefort, T., Saville, B. J., et al. (2006). Insights from the genome of the biotrophic fungal plant pathogen Ustilago maydis. Nature 444, 97–101. doi: 10.1038/nature05248
Kang, W. K., Kim, Y. H., Kang, H. A., Kwon, K.-S., and Kim, J.-Y. (2015). Sir2 phosphorylation through cAMP-PKA and CK2 signaling inhibits the lifespan extension activity of Sir2 in yeast. Elife 4:e09709. doi: 10.7554/eLife.09709
Khrunyk, Y., Münch, K., Schipper, K., Lupas, A. N., and Kahmann, R. (2010). The use of FLP-mediated recombination for the functional analysis of an effector gene family in the biotrophic smut fungus Ustilago maydis. New Phytol. 187, 957–968. doi: 10.1111/j.1469-8137.2010.03413.x
Kong, X., van Diepeningen, A. D., van der Lee, T. A. J., Waalwijk, C., Xu, J., Xu, J., et al. (2018). The fusarium graminearum histone acetyltransferases are important for morphogenesis, DON biosynthesis, and pathogenicity. Front. Microbiol. 9:654. doi: 10.3389/fmicb.2018.00654
Kruitwagen, T., Chymkowitch, P., Denoth-Lippuner, A., Enserink, J., and Barral, Y. (2018). Centromeres license the mitotic condensation of yeast chromosome arms. Cells 175, 780–795.e15. doi: 10.1016/j.cell.2018.09.012
Lai, Y., Wang, L., Zheng, W., and Wang, S. (2022). Regulatory roles of histone modifications in filamentous fungal pathogens. J. Fungi 8:565. doi: 10.3390/jof8060565
Lanver, D., Berndt, P., Tollot, M., Naik, V., Vranes, M., Warmann, T., et al. (2014). Plant surface cues prime Ustilago maydis for biotrophic development. PLoS Pathog. 10:e1004272. doi: 10.1371/journal.ppat.1004272
Lanver, D., Müller, A. N., Happel, P., Schweizer, G., Haas, F. B., Franitza, M., et al. (2018). The biotrophic development of Ustilago maydis studied by RNA-Seq analysis. Plant Cell 30, 300–323. doi: 10.1105/tpc.17.00764
Lanver, D., Tollot, M., Schweizer, G., Lo Presti, L., Reissmann, S., Ma, L.-S., et al. (2017). Ustilago maydis effectors and their impact on virulence. Nat. Rev. Microbiol. 15, 409–421. doi: 10.1038/nrmicro.2017.33
Li, G., Qi, X., Sun, G., Rocha, R. O., Segal, L. M., Downey, K. S., et al. (2020). Terminating rice innate immunity induction requires a network of antagonistic and redox-responsive E3 ubiquitin ligases targeting a fungal sirtuin. New Phytol. 226, 523–540. doi: 10.1111/nph.16365
Liu, J., An, B., Luo, H., He, C., and Wang, Q. (2022). The histone acetyltransferase FocGCN5 regulates growth, conidiation, and pathogenicity of the banana wilt disease causal agent fusarium oxysporum f.sp. cubense tropical race 4. Res. Microbiol. 173:103902. doi: 10.1016/j.resmic.2021.103902
Love, M. I., Huber, W., and Anders, S. (2014). Moderated estimation of fold change and dispersion for RNA-seq data with DESeq2. Genome Biol. 15:550. doi: 10.1186/s13059-014-0550-8
Ma, L.-S., Wang, L., Trippel, C., Mendoza-Mendoza, A., Ullmann, S., Moretti, M., et al. (2018). The Ustilago maydis repetitive effector Rsp3 blocks the antifungal activity of mannose-binding maize proteins. Nat. Commun. 9:1711. doi: 10.1038/s41467-018-04149-0
Maas, N. L., Miller, K. M., DeFazio, L. G., and Toczyski, D. P. (2006). Cell cycle and checkpoint regulation of histone H3 K56 acetylation by Hst3 and Hst4. Mol. Cell 23, 109–119. doi: 10.1016/j.molcel.2006.06.006
Martin, B. J. E., Brind’Amour, J., Kuzmin, A., Jensen, K. N., Liu, Z. C., Lorincz, M., et al. (2021). Transcription shapes genome-wide histone acetylation patterns. Nat. Commun. 12:210. doi: 10.1038/s41467-020-20543-z
Mazzio, E. A., and Soliman, K. F. A. (2012). Basic concepts of epigenetics. Epigenetics 7, 119–130. doi: 10.4161/epi.7.2.18764
Mueller, A. N., Ziemann, S., Treitschke, S., Aßmann, D., and Doehlemann, G. (2013). Compatibility in the Ustilago maydis–maize interaction requires inhibition of host cysteine proteases by the fungal effector Pit2. PLoS Pathog. 9:e1003177. doi: 10.1371/journal.ppat.1003177
Ökmen, B., Jaeger, E., Schilling, L., Finke, N., Klemd, A., Lee, Y. J., et al. (2022). A conserved enzyme of smut fungi facilitates cell-to-cell extension in the plant bundle sheath. Nat. Commun. 13:6003. doi: 10.1038/s41467-022-33815-7
Patton, E. E., Willems, A. R., and Tyers, M. (1998). Combinatorial control in ubiquitin-dependent proteolysis: don’t Skp the F-box hypothesis. Trends Genet. 14, 236–243. doi: 10.1016/S0168-9525(98)01473-5
Rando, O. J., and Winston, F. (2012). Chromatin and transcription in yeast. Genetics 190, 351–387. doi: 10.1534/genetics.111.132266
Reichmann, M., Jamnischek, A., Weinzierl, G., Ladendorf, O., Huber, S., Kahmann, R., et al. (2002). The histone deacetylase Hda1 from Ustilago maydis is essential for teliospore development. Mol. Microbiol. 46, 1169–1182. doi: 10.1046/j.1365-2958.2002.03238.x
Robyr, D., Suka, Y., Xenarios, I., Kurdistani, S. K., Wang, A., Suka, N., et al. (2002). Microarray deacetylation maps determine genome-wide functions for yeast histone deacetylases. Cells 109, 437–446. doi: 10.1016/S0092-8674(02)00746-8
Sambrook, J., Frisch, E. R., and Maniatis, T. (1989). Molecular cloning: A laboratory manual. 2nd ed.. Cold Spring Harbor NY: Cold Spring Harbor Laboratory Press.
Schauwecker, F., Wanner, G., and Kahmann, R. (1995). Filament-specific expression of a cellulase gene in the dimorphic fungus Ustilago maydis. Biol. Chem. Hoppe Seyler 376, 617–626. doi: 10.1515/bchm3.1995.376.10.617
Schulz, B., Banuett, F., Dahl, M., Schlesinger, R., Schäfer, W., Martin, T., et al. (1990). The b alleles of U. maydis, whose combinations program pathogenic development, code for polypeptides containing a homeodomain-related motif. Cells 60, 295–306. doi: 10.1016/0092-8674(90)90744-Y
Schuster, M., Schweizer, G., and Kahmann, R. (2018). Comparative analyses of secreted proteins in plant pathogenic smut fungi and related basidiomycetes. Fungal Genet. Biol. 112, 21–30. doi: 10.1016/j.fgb.2016.12.003
Shankaranarayana, G. D., Motamedi, M. R., Moazed, D., and Grewal, S. I. S. (2003). Sir2 regulates histone H3 lysine 9 methylation and heterochromatin assembly in fission yeast. Curr. Biol. 13, 1240–1246. doi: 10.1016/S0960-9822(03)00489-5
Shimizu, M., Masuo, S., Fujita, T., Doi, Y., Kamimura, Y., and Takaya, N. (2012). Hydrolase controls cellular NAD, sirtuin, and secondary metabolites. Mol. Cell. Biol. 32, 3743–3755. doi: 10.1128/MCB.00032-12
Smith, K. M., Kothe, G. O., Matsen, C. B., Khlafallah, T. K., Adhvaryu, K. K., Hemphill, M., et al. (2008). The fungus Neurospora crassa displays telomeric silencing mediated by multiple sirtuins and by methylation of histone H3 lysine 9. Epigenetics Chromatin 1:5. doi: 10.1186/1756-8935-1-5
Suka, N., Luo, K., and Grunstein, M. (2002). Sir2p and Sas2p opposingly regulate acetylation of yeast histone H4 lysine16 and spreading of heterochromatin. Nat. Genet. 32, 378–383. doi: 10.1038/ng1017
Sutton, A., Heller, R. C., Landry, J., Choy, J. S., Sirko, A., and Sternglanz, R. (2001). A novel form of transcriptional silencing by Sum1-1 requires Hst1 and the origin recognition complex. Mol. Cell. Biol. 21, 3514–3522. doi: 10.1128/MCB.21.10.3514-3522.2001
Tanner, K. G., Landry, J., Sternglanz, R., and Denu, J. M. (2000). Silent information regulator 2 family of NAD- dependent histone/protein deacetylases generates a unique product, 1- O- acetyl-ADP-ribose. Proc. Natl. Acad. Sci. U. S. A. 97, 14178–14182. doi: 10.1073/pnas.250422697
Tanny, J. C., and Moazed, D. (2001). Coupling of histone deacetylation to NAD breakdown by the yeast silencing protein Sir2: evidence for acetyl transfer from substrate to an NAD breakdown product. Proc. Natl. Acad. Sci. U. S. A. 98, 415–420. doi: 10.1073/pnas.98.2.415
Torreblanca, J., Stumpferl, S., and Basse, C. W. (2003). Histone deacetylase Hda1 acts as repressor of the Ustilago maydis biotrophic marker gene mig1. Fungal Genet. Biol. 38, 22–32. doi: 10.1016/S1087-1845(02)00505-4
Vaquero, A., Scher, M. B., Lee, D. H., Sutton, A., Cheng, H.-L., Alt, F. W., et al. (2006). SirT2 is a histone deacetylase with preference for histone H4 Lys 16 during mitosis. Genes Dev. 20, 1256–1261. doi: 10.1101/gad.1412706
Vollmeister, E., Schipper, K., Baumann, S., Haag, C., Pohlmann, T., Stock, J., et al. (2012). Fungal development of the plant pathogen Ustilago maydis. FEMS Microbiol. Rev. 36, 59–77. doi: 10.1111/j.1574-6976.2011.00296.x
Wilkins, B. J., Rall, N. A., Ostwal, Y., Kruitwagen, T., Hiragami-Hamada, K., Winkler, M., et al. (2014). A cascade of histone modifications induces chromatin condensation in mitosis. Science 343, 77–80. doi: 10.1126/science.1244508
Wilson, J. M., Le, V. Q., Zimmerman, C., Marmorstein, R., and Pillus, L. (2006). Nuclear export modulates the cytoplasmic Sir2 homologue Hst2. EMBO Rep. 7, 1247–1251. doi: 10.1038/sj.embor.7400829
Wurtele, H., Tsao, S., Lépine, G., Mullick, A., Tremblay, J., Drogaris, P., et al. (2010). Modulation of histone H3 lysine 56 acetylation as an antifungal therapeutic strategy. Nat. Med. 16, 774–780. doi: 10.1038/nm.2175
Zhao, G., and Rusche, L. N. (2021). Genetic analysis of Sirtuin deacetylases in hyphal growth of Candida albicans. mSphere 6:6. doi: 10.1128/mSphere.00053-21
Keywords: sirtuins, phytopathogenesis, HDAC, histone deacetylases, gene regulation, plant infection, filamentation
Citation: Navarrete B, Ibeas JI and Barrales RR (2023) Systematic characterization of Ustilago maydis sirtuins shows Sir2 as a modulator of pathogenic gene expression. Front. Microbiol. 14:1157990. doi: 10.3389/fmicb.2023.1157990
Edited by:
Christian Koch, Friedrich-Alexander-Universität Erlangen-Nürnberg, GermanyReviewed by:
Tong-Bao Liu, Southwest University, ChinaIrene Castano, Instituto Potosino de Investigación Científica y Tecnológica (IPICYT), Mexico
Copyright © 2023 Navarrete, Ibeas and Barrales. This is an open-access article distributed under the terms of the Creative Commons Attribution License (CC BY). The use, distribution or reproduction in other forums is permitted, provided the original author(s) and the copyright owner(s) are credited and that the original publication in this journal is cited, in accordance with accepted academic practice. No use, distribution or reproduction is permitted which does not comply with these terms.
*Correspondence: José I. Ibeas, am9pYmVjb3JAdXBvLmVz; Ramón R. Barrales, cnJhbWJhckB1cG8uZXM=
†These authors share senior authorship