- Department of Biology, College of Science, University of Hafr Al Batin, Hafr Al-Batin, Saudi Arabia
The significance of nanoparticles (NPs) in technological advancements is due to their adaptable characteristics and enhanced performance over their parent material. They are frequently synthesized by reducing metal ions into uncharged nanoparticles using hazardous reducing agents. However, there have been several initiatives in recent years to create green technology that uses natural resources instead of dangerous chemicals to produce nanoparticles. In green synthesis, biological methods are used for the synthesis of NPs because biological methods are eco-friendly, clean, safe, cost-effective, uncomplicated, and highly productive. Numerous biological organisms, such as bacteria, actinomycetes, fungi, algae, yeast, and plants, are used for the green synthesis of NPs. Additionally, this paper will discuss nanoparticles, including their types, traits, synthesis methods, applications, and prospects.
1. Introduction
Nanotechnology evolved as the achievement of science in the 21st century. The synthesis, management, and application of those materials with a size smaller than 100 nm fall under the interdisciplinary umbrella of this field. Nanoparticles have significant applications in different sectors such as the environment, agriculture, food, biotechnology, biomedical, medicines, etc. like; for treatment of waste water (Zahra et al., 2020), environment monitoring (Rassaei et al., 2011), as a functional food additives (Chen et al., 2023), and as a antimicrobial agents (Islam et al., 2022). Cutting-edge properties of NPs such as; nature, biocompatibility, anti-inflammatory and antibacterial activity, effective drug delivery, bioactivity, bioavailability, tumor targeting, and bio-absorption have led to a growth in the biotechnological, and applied microbiological applications of NPs.
A particle of matter with a diameter of one to one hundred nanometers (nm) is commonly referred to as a nanoparticle or ultrafine particle. Nanoparticles frequently exhibit distinctive size-dependent features, mostly due to their tiny size and colossal surface area. The periodic boundary conditions of the crystalline particle are destroyed when the size of a particle approaches the nano-scale with the characteristic length scale close to or smaller than the de Broglie wavelength or the wavelength of light (Guo et al., 2013). Because of this, many of the physical characteristics of nanoparticles differ significantly from those of bulk materials, leading to a wide range of their novel uses (Hasan, 2015).
2. Emergence of nanotechnology
Nanotechnology emerged in the 1980s due to the convergence of experimental advances such as the invention of the scanning tunneling microscope in 1981 and the discovery of fullerenes in 1985 (Bayda et al., 2019), with the elucidation. The popularization of a conceptual framework for nanotechnology goals began with the publication of the book Engines of Creation in 1986 (Bayda et al., 2019).
2.1. Early stage of NPs
Carbon nanotubes have been discovered in pottery from Keeladi, India, dating from around 600–300 BC (Bayda et al., 2019; Kokarneswaran et al., 2020). Cementite nanowires have been discovered in Damascus steel, a material that dates back to around 900 AD; nevertheless, its origin and creation method are unclear (Kokarneswaran et al., 2020). However, it is unknown how they developed or whether the material containing them was used on purpose.
2.2. Discovery of C, Ag, Zn, Cu, and Au nanoparticles
Carbon NPs were found in 1991, and Iijima and Ichihashi announced the single-wall carbon nanotube synthesis with a diameter of 1 nanometer in 1993 (Chen et al., 2021). Carbon nanotubes (CNTs), also known as Bucky tubes, are a kind of nanomaterial made up of a two-dimensional hexagonal lattice of carbon atoms. They are bent one way and joined to produce a hollow cylindrical cylinder. Carbon nanotubes are carbon allotropes that fall between Fullerene (0 dimensional) and Grapheme (2 dimensional) (Chen et al., 2021).
In addition, M. C. Lea reported that the synthesis of citrate-stabilized silver colloid almost 120 years ago (Nowack et al., 2011). This process produces particles with an average diameter of 7 to 9 nm. Nanoscale size and citrate stabilization are analogous to recent findings on nanosilver production employing silver nitrate and citrate (Majeed Khan et al., 2011). The use of proteins to stabilize nanosilver has also been documented as early as 1902 (Nowack et al., 2011; Beyene et al., 2017). Since 1897, a nanosilver known as “Collargol” has been made commercially and used for medicinal purposes (Nowack et al., 2011). Collargol, a type of silver nanoparticle, has a particle size of about 10 nanometers (nm). This was determined as early as 1907, and it was found that the diameter of Collargol falls within the nanoscale range. In 1953, Moudry developed a different type of silver nanoparticle called gelatin-stabilized silver nanoparticles, with a diameter ranging from 2–20 nm. These nanoparticles were produced using another method than Collargol. The necessity of nanoscale silver was recognized by the creators of nanosilver formulations decades ago, as seen by the following remark from a patent: “for optimal efficiency, the silver must be disseminated as particles of colloidal size less than 25 nm in crystallite size”(Nowack et al., 2011).
Gold NPs (AuNPs) have a long history in chemistry, going back to the Roman era when they were used to decorate glassware by staining them. With the work of Michael Faraday, who may have been the first to notice that colloidal gold solutions have characteristics different from bulk gold, the contemporary age of AuNP synthesis began more than 170 years ago. Michael Faraday investigated the making and factors of colloidal suspensions of “Ruby” gold in 1857. They are among the magnetic nanoparticles due to their distinctive optical and electrical characteristics. Under specific illumination circumstances, Faraday showed how gold nanoparticles might create solutions of various colors (Bayda et al., 2019; Giljohann et al., 2020).
3. Classification of NPs
Nanoparticles (NPs) are categorized into the following classes based on their shape, size, and chemical characteristics
3.1. Carbon-based NPs
Fullerenes and carbon nanotubes (CNTs) are the two essential sub-categories of carbon-based NPs. NPs of globular hollow cages, like allotropic forms of carbon, are found in fullerenes. Due to their electrical conductivity, high strength, structure, electron affinity, and adaptability, they have sparked significant economic interest. These materials have organized pentagonal and hexagonal carbon units, each of which is sp2 hybridized. While CNTs are elongated and form 1–2 nm diameter tubular structures. These fundamentally resemble graphite sheets rolling on top of one another. Accordingly, they are referred to as single-walled (SWNTs), double-walled (DWNTs), or multi-walled carbon nanotubes (MWNTs) depending on how many walls are present in the rolled sheets (Elliott et al., 2013; Astefanei et al., 2015).
3.2. Metal NPs
Metal NPs are purely made of metals. These NPs have distinctive electrical properties due to well-known localized surface Plasmon resonance (LSPR) features. Cu, Ag, and Au nanoparticles exhibit a broad absorption band in the visible region of the solar electromagnetic spectrum. Metal NPs are used in several scientific fields because of their enhanced features like facet, size, and shape-controlled synthesis of metal NPs (Khan et al., 2019).
3.3. Ceramics NPs
Ceramic NPs are tiny particles made up of inorganic, non-metallic materials that are heat-treated and cooled in a specific way to give particular properties. They can come in various shapes, including amorphous, polycrystalline, dense, porous, and hollow, and they are known for heat resistance and durable properties. Ceramic NPs are used in various applications, including coating, catalysts, and batteries (Sigmund et al., 2006).
3.4. Lipid-based NPs
These NPs are helpful in several biological applications because they include lipid moieties. Lipid NPs typically have a diameter of 10–1,000 nm and are spherical. Lipid NPs, i.e., polymeric NPs, have a solid lipid core and a matrix consisting of soluble lipophilic molecules (Khan et al., 2019).
3.5. Semiconductor NPs
Semiconductor NPs have qualities similar to metals and non-metals. That is why Semiconductor NPs have unique physical and chemical properties that make them useful for various applications. For example, semiconductor NPs can absorb and emit light and can be used to make more efficient solar cells or brighter light-emitting diodes (LEDs). They can make smaller and faster electronic devices, such as transistors, and can be used in bio imaging and cancer therapy (Biju et al., 2008).
3.6. Polymeric NPs
Polymeric NPs with a size between 1 and 1,000 nm can have active substances surface-adsorbed onto the polymeric core or entrapped inside the polymeric body. These NPs are often organic, and the term polymer nanoparticle (PNP) is commonly used in the literature to refer to them. They resemble Nano spheres or Nano capsules for the most part (Khan et al., 2019; Zielińska et al., 2020).
4. Types of different metal-based NPs
Metal NPs are purely made of metal precursors. Due to well-known localized surface plasmon resonance (LSPR) characteristics, these NPs possess unique optoelectrical properties. NPs of the alkali and noble metals, i.e., Cu, Ag, and Au, have a broad absorption band in the visible zone of the solar electromagnetic spectrum. The facet, size, and shape-controlled synthesis of metal NPs are essential in present-day cutting-edge materials (Dreaden et al., 2012; Khan et al., 2019).
4.1. Silver nanoparticles (AgNPs)
AgNPs are particles with a size range of 1–100 nanometers made of silver. They have unique physical and chemical properties due to their small size, high surface area-to-volume ratio, and ability to absorb and scatter light in the visible and near-infrared range. Because of their relatively small size and high surface-to-volume ratios, which cause chemical and physical differences in their properties compared to their bulk counterparts, silver nanoparticles may exhibit additional antimicrobial capabilities not exerted by ionic silver (Shenashen et al., 2014).
Besides, AgNPs can be created in various sizes and forms depending on the manufacturing process, the most common of which is chemical reduction. The AgNPs were created by chemically reducing a 12 mM AgNO3 aqueous solution. The reaction was carried out in an argon environment using 70 mL of this solution containing PVP (keeping the molar ratio of the repeating unit of PVP and Ag equal to 34) and 21 mL of Aloe Vera. The mixture was agitated in ultrasonic for 45 min at ambient temperature, then heated 2°C/min to 80°C and left for 2 h to generate a transparent solution with tiny suspended particles that must be removed by simple filtering (Shenashen et al., 2014; Gloria et al., 2017).
4.2. Zinc nanoparticles (ZnONPs)
Zinc nanoparticles (ZnONPs) are particles with a size range of 1–100 nm made of zinc. Zinc oxide (ZnO) NPs are a wide band gap semiconductor with a room temperature energy gap of 3.37 eV. Its catalytic, electrical, optoelectronic, and photochemical capabilities have made it widely worthwhile (Kumar S.S. et al., 2013). ZnO nanostructures are ideal for catalytic reaction processes (Chen and Tang, 2007). Laser ablation, hydrothermal methods, electrochemical depositions, sol-gel method, chemical vapor deposition, thermal decomposition, combustion methods, ultrasound, microwave-assisted combustion method, two-step mechanochemical-thermal synthesis, anodization, co-precipitation, electrophoretic deposition, and precipitation processes are some methods for producing ZnO nanoparticles (Madathil et al., 2007; Moghaddam et al., 2009; Ghorbani et al., 2015).
4.3. Copper nanoparticles (CuNPs)
Copper nanoparticles (CuNPs) comprise a size range of 1–100 nm of copper-based particles (Khan et al., 2019). Cu and Au metal fluorescence have long been known to exist. For excitation at 488 nm, a fluorescence peak centering on the metals’ interband absorption edge has been noted. Additionally, it was noted that the fluorescence peaked at the same energy at two distinct excitation wavelengths (457.9–514.5 and 300–400 nm), and the high-energy tail somewhat grows with increased photon energy pumping. A unique, physical, top-down EEW approach has been used to create Cu nanoparticles. The EEW method involves sending a current of *1,010 A/m2 (1,010 A/m2) across a thin Cu wire, which explodes on a Cu plate for a duration of 10–6 s (Siwach and Sen, 2008).
4.4. Gold nanoparticles (AuNPs)
Gold nanoparticles(AuNPs) are nanometers made of gold. They have unique physical and chemical properties and can absorb and scatter light in the visible and near-infrared range (Rad et al., 2011; Compostella et al., 2017).
Scientists around the turn of the 20th century discovered anisotropic AuNPs. Zsigmond (Li et al., 2014) said that gold particles “are not always spherical when their size is 40 nm or lower” in his book, released in 1909. Additionally, he found anisotropic gold particles of various colors. Zsigmondy won the Nobel Prize in 1925 for “his demonstration of the heterogeneous character of colloidal solutions and the methods he utilized” and for developing the ultramicroscope, which allowed him to see the forms of Au particles. He noticed that gold frequently crystallized into a six-sided leaf shape (Li et al., 2014).
AuNPs are the topic of extensive investigation due to their optical, electrical, and molecular-recognition capabilities, with numerous prospective or promised uses in a wide range of fields, including electron microscopy, electronics, nanotechnology, materials science, and biomedicine (Rad et al., 2011).
4.5. Aluminum nanoparticles (AlNPs)
Aluminum nanoparticles (AlNPs) are nanoparticles made of aluminum. Aluminum nanoparticles’ strong reactivity makes them promising for application in high-energy compositions, hydrogen generation in water processes, and the synthesis of alumina 2D and 3D structures (Lerner et al., 2016).
4.6. Iron nanoparticles (FeNPs)
Iron nanoparticles(FeNPs) are particles with a size range of 1−100 nanometers (Khan et al., 2019) made of iron. FeNPs have several potential applications, including their use as catalysts, drug delivery systems, sensors, and energy storage and conversion. They have also been investigated for use in photovoltaic and solar cells and water purification and environmental remediation. FeNPs can also be used in magnetic resonance imaging (MRI) as contrast agents to improve the visibility of tissues and organs. They can also be used in magnetic recording media, such as hard disk drives (Zhuang and Gentry, 2011; Jamkhande et al., 2019).
As with any NPs, there are potential health and safety concerns associated with using FeNPs, e.g., FeNPs are used to deliver drugs to specific locations within the body, such as cancer cells and used in MRI, and used to remove contaminants from water (Farrell et al., 2003; Zhuang and Gentry, 2011). Tables 1, 2 show the characteristics of metal-based nanoparticles and the techniques to study their characteristics, respectively.
5. Approaches for the synthesis of metal NPs
There are mainly three types of approaches for the synthesis of NPs: the physical, chemical, and biological approaches. The physical approach is also called the top-down approach, while chemical and biological approaches are collectively called the bottom-up approach. The biological approach is also named green systems of NPs. All these approaches are further sub-categorized into various types based upon their method adopted. Figure 1 illustrates each approach’s reported methods for synthesizing NPs.
5.1. Top down/physical approach
Bulk materials are fragmented in top-down methods to create nano-structured materials (Figure 2). They are additionally known as physical approaches (Baig et al., 2021). The following techniques can achieve a top-down approach
5.1.1. Mechanical milling
The mechanical milling process uses balls inside containers and may be carried out in various mills, typically planetary and shaker mills, which is an impact process with high energy (Gorrasi and Sorrentino, 2015). Mechanical milling is a practical approach for creating materials at the nanoscale from bulk materials. Aluminum alloys that have been strengthened by oxide and carbide, spray coatings that are resistant to wear, nanoalloys based on aluminum, nickel, magnesium, and copper, and a variety of other nanocomposite materials may all be created mechanically. A unique class of nanoparticles known as ball-milled carbon nanomaterials has the potential to meet the needs for energy storage, energy conversion, and environmental remediation (Yadav et al., 2012; Lyu et al., 2017).
5.1.2. Electrospinning
Typically, it is used to create nanofibers from various materials, most often polymers (Ostermann et al., 2011). A technique for creating fibers called electrospinning draws charged threads from polymer melts or solutions up to fiber sizes of a few hundred nanometers (Chronakis, 2010). Coaxial electrospinning was a significant advancement in the field of electrospinning. The spinneret in coaxial electrospinning is made up of two coaxial capillaries. Core-shell nanoarchitectures may be created in these capillaries using two viscous liquids, a viscous liquid as the shell and a non-viscous liquid as the core (Du et al., 2012). Core-shell and hollow polymer, inorganic, organic, and hybrid materials have all been developed using this technique (Kumar R. et al., 2013).
5.1.3. Laser ablation
A microfeature can be made by employing a laser beam to vaporize a single material (Tran and Wen, 2014). Laser ablation synthesis produces nanoparticles by striking the target material with an intense laser beam. Due to the high intensity of the laser irradiation used in the laser ablation process, the source material or precursor vaporizes, causing the production of nanoparticles (Amendola and Meneghetti, 2009). Laser ablation is an environmentally friendly for producing noble metal nanoparticles (Baig et al., 2021). This method may be used to create a wide variety of nanomaterials, including metal nanoparticles, carbon nanomaterials, oxide composites, and ceramics (Su and Chang, 2018; Baig et al., 2021).
5.1.4. Sputtering
Microparticles of a solid material are expelled off its surface during the phenomenon known as sputtering, which occurs when the solid substance is assaulted by intense plasma or gas particles (Behrisch, 1981). According to the incident gaseous ion energy, energetic gaseous ions used in the sputtering deposition process physically expel tiny atom clusters off the target surface (Muñoz-García et al., 2009). The sputtering method is intriguing because it is more affordable than electron-beam lithography, and the composition of the sputtered nanomaterials is similar to the target material with fewer contaminants (Baig et al., 2021).
5.1.5. Electron explosion
In this technique, a thin metal wire is subjected to a high current pulse that causes an explosion, evaporation, and ionization. The metal becomes vaporized and ionized, expands, and cools by reacting with the nearby gas or liquid medium. The condensed vapor finally forms the nanoparticles (Joh et al., 2013). Electron explosion method because it produces plasma from the electrical explosion of a metallic wire, which may produce nanoparticles from a Pt solution without using a reducing agent (Joh et al., 2013).
5.1.6. Sonication
The most crucial step in the creation of nanofluids is sonication. After the mixture has been magnetically stirred in a magnetic stirrer, sonication is performed in an ultrasonication path, ultrasonic vibrator, and mechanical homogenizer. Sonicators have become the industry standard for Probe sonication and are noticeably more powerful and effective when compared to ultrasonic cleaner baths for nanoparticle applications. Probe sonication is highly effective for processing nanomaterials (carbon nanotubes, graphene, inks, metal oxides, etc.) (Zheng et al., 2010).
5.1.7. Pulsed wire discharge method
This is the most used method for creating metal nanoparticles. A pulsating current causes a metal wire to evaporate, producing a vapor that is subsequently cooled by an ambient gas to form nanoparticles. This plan may quickly produce large amounts of energy (Patil et al., 2021).
5.1.8. Arc discharge method
Two graphite rods are adjusted in a chamber with a constant helium pressure during the Arc Discharge procedure. It is crucial to fill the chamber with helium because oxygen or moisture prevents the synthesis of fullerenes. Arc discharge between the ends of the graphite rods drives the vaporization of carbon rods. Achieving new types of nanoparticles depends significantly on the circumstances in which arc discharge occurs. The creation of several nanostructured materials may be accomplished with this technique (Berkmans et al., 2014). It is well-recognized for creating carbon-based materials such as fullerenes, carbon nanohorns (CNHs), carbon nanotubes (Shi et al., 2000), few-layer graphene, and amorphous spherical carbon nanoparticles (Kumar R. et al., 2013).
5.1.9. Lithography
Lithography typically uses a concentrated beam of light or electrons to create nanoparticles, a helpful technique (Pimpin and Srituravanich, 2012). Masked and maskless lithography are the two primary categories of lithography. Without a mask, arbitrary nano-pattern printing is accomplished in maskless lithography. Additionally, it is affordable and easy to apply (Brady et al., 2019).
5.2. Bottom-up approach
Tiny atoms and molecules are combined in bottom-up methods to create nano-structured particles (Figure 2; Baig et al., 2021). These include chemical and biological approaches:
5.2.1. Chemical vapor deposition (CVD)
Through a chemical process involving vapor-phase precursors, a thin coating is created on the substrate surface during CVD (Dikusar et al., 2009). Precursors are deemed appropriate for CVD if they exhibit sufficient volatility, high chemical purity, strong evaporation stability, cheap cost, a non-hazardous nature, and long shelf life. Additionally, its breakdown should not leave behind any contaminants. Vapor phase epitaxy, metal-organic CVD, atomic layer epitaxy, and plasma-enhanced CVD are only a few CVD variations. This method’s benefits include producing very pure nanoparticles that are stiff, homogeneous, and strong (Ago, 2015). CVD is an excellent approach to creating high-quality nanomaterials (Machac et al., 2020). It is also well-known for creating two-dimensional nanoparticles (Baig et al., 2021).
5.2.2. Sol-gel process
A wet-chemical approach, called the sol-gel method, is widely utilized to create nanomaterials (Das and Srivasatava, 2016; Baig et al., 2021). Metal alkoxides or metal precursors in solution are condensed, hydrolyzed, and thermally decomposed. The result is a stable solution or sol. The gel gains greater viscosity as a result of hydrolysis or condensation. The particle size may be seen by adjusting the precursor concentration, temperature, and pH levels. It may take a few days for the solvent to be removed, for Ostwald ripening to occur, and for the phase to change during the mature stage, which is necessary to enable the growth of solid mass. To create nanoparticles, the unstable chemical ingredients are separated. The generated material is environmentally friendly and has many additional benefits thanks to the sol-gel technique (Patil et al., 2021). The uniform quality of the material generated, the low processing temperature, and the method’s ease in producing composites and complicated nanostructures are just a few of the sol-gel technique’s many advantages (Parashar et al., 2020).
5.2.3. Co-precipitation
It is a solvent displacement technique and is a wet chemical procedure. Ethanol, acetone, hexane, and non-solvent polymers are examples of solvents. Polymer phases can be either synthetic or natural. By mixing the polymer solution, fast diffusion of the polymer-solvent into the non-solvent phase of the polymer results. Interfacial stress at two phases results in the formation of nanoparticles (Das and Srivasatava, 2016). This method’s natural ability to produce high quantities of water-soluble nanoparticles through a straightforward process is one of its key benefits. This process is used to create many commercial iron oxide NP-based MRI contrast agents, including Feridex, Reservist, and Combidex (Baig et al., 2021; Patil et al., 2021).
5.2.4. Inert gas condensation/molecular condensation
Metal NPs are produced using this method in large quantities. Making fine NPs using the inactive gas compression approach has been widespread, which creates NPs by causing a metallic source to disappear in an inert gas. At an attainable temperature, metals evaporate at a tolerable pace. Copper metal nanoparticles are created by vaporizing copper metal inside a container containing argon, helium, or neon. The atom quickly loses its energy by cooling the vaporized atom with an inert gas after it boils out. Liquid nitrogen is used to cool the gases, forming nanoparticles in the range of 2–100 nm (Pérez-Tijerina et al., 2008; Patil et al., 2021).
5.2.5. Hydrothermal
In this method, for the production of nanoparticles, hydrothermal synthesis uses a wide temperature range from ambient temperature to extremely high temperatures. Comparing this strategy to physical and biological ones offers several benefits. At higher temperature ranges, the nanomaterials produced by hydrothermal synthesis could become unstable (Banerjee et al., 2008; Patil et al., 2021).
5.2.6. Green/biological synthesis
The synthesis of diverse metal nanoparticles utilizing bioactive agents, including plant materials, microbes, and various biowastes like vegetable waste, fruit peel waste, eggshell, agricultural waste, algae, and so on, is known as “green” or “biological” nanoparticle synthesis (Kumari et al., 2021). Developing dependable, sustainable green synthesis technologies is necessary to prevent the formation of undesirable or dangerous byproducts (Figure 3). The green synthesis of nanoparticles also has several advantages, including being straightforward, affordable, producing NPs with high stability, requiring little time, producing non-toxic byproducts, and being readily scaled up for large-scale synthesis (Malhotra and Alghuthaymi, 2022).
5.2.6.1. Biological synthesis using microorganisms
Microbes use metal capture, enzymatic reduction, and capping to create nanoparticles. Before being converted to nanoparticles by enzymes, metal ions are initially trapped on the surface or interior of microbial cells (Ghosh et al., 2021). Use of microorganisms (especially marine microbes) for synthesis of metalic NPs is environmental friendly, fast and economical (Patil and Kim, 2018). Several microorganisms are used in the synthesis of metal NPs, including:
Biosynthesis of NPs by bacteria: A possible biofactory for producing gold, silver, and cadmium sulfide nanoparticles is thought to be bacterial cells. It is known that bacteria may produce inorganic compounds either inside or outside of their cells (Hulkoti and Taranath, 2014). Desulforibrio caledoiensis (Qi et al., 2013), Enterococcus sp. (Rajeshkumar et al., 2014), Escherichia coli VM1 (Maharani et al., 2016), and Ochrobactrum anhtropi (Thomas et al., 2014) based metal NPs are reported previously for their potential photocatalytic properties (Qi et al., 2013), antimicrobial activity (Rajeshkumar et al., 2014), and anticancer activity (Maharani et al., 2016).
Extracellular synthesis of NPs by bacteria: The microorganisms’ extracellular reductase enzymes shrink the silver ions to the nanoscale range. According to protein analysis of microorganisms, the NADH-dependent reductase enzyme carries out the bio-reduction of silver ions to AgNPs. The electrons for the reductase enzyme come from NADH, which is subsequently converted to NAD+. The enzyme is also oxidized simultaneously when silver ions are reduced to nanosilver. It has been noted that bio-reduction can occasionally be caused by nitrate-dependent reductase. The decline occurs within a few minutes in the quick extracellular creation of nanoparticles (Mathew et al., 2010). At pH 7, the bacterium R. capsulata produced gold nanoparticles with sizes ranging from 10−20 nm. Numerous nanoplates and spherical gold nanoparticles were produced when the pH was changed to four (Sriram et al., 2012). By adjusting the pH, the gold nanoparticles’ form may be changed. Gold nanoparticle shape was controlled by regulating the proton content at various pH levels. The bacteria R. capsulata’s release cofactor NADH and NADH-dependent enzymes may cause the bioreduction of Au (3+) to Au (0) and the generation of gold nanoparticles. By using NADH-dependent reductase as an electron carrier, it is possible to start the reduction of gold ions (Sriram et al., 2012).
Intracellular synthesis of NPs by bacteria: Three processes are involved in the intracellular creation of NPs: trapping, bioreduction, and capping. The cell walls of microorganisms and ions charge contribute significantly to creating NPs in the intracellular route. This entails specific ion transit in the presence of enzymes, coenzymes, and other molecules in the microbial cell. Microbes have a range of polysaccharides and proteins in their cell walls, which function as active sites for the binding of metal ions (Slavin et al., 2017). Not all bacteria can produce metal and metal oxide nanoparticles. The only ions that pose a significant hazard to microorganisms are heavy metal ions, which, in response to a threat, cause the germs to react by grabbing or trapping the ions on the cell wall via electrostatic interactions. This occurs because a metal ion is drawn to the cell wall’s carboxylate groups, including cysteine and polypeptides, and certain enzymes with a negative charge (Zhang et al., 2011).
Additionally, the electron transfers from NADH via NADH-dependent educates, which serves as an electron carrier and is located inside the plasma membrane, causing the trapped ions to be reduced into the elemental atom. The nuclei eventually develop into NPs and build up in the cytoplasm or the pre-plasmic space. On the other hand, the stability of NPs is provided by proteins, peptides, and amino acids found inside cells, including cysteine, tyrosine, and tryptophan (Mohd Yusof et al., 2019).
Biosynthesis of NPs by fungi: Because monodisperse nanoparticles with distinct dimensions, various chemical compositions, and sizes may be produced, the biosynthesis of nanoparticles utilizing fungus is frequently employed. Due to the existence of several enzymes in their cells and the ease of handling, fungi are thought to be great candidates for producing metal and metal sulfide nanoparticles (Mohanpuria et al., 2008).
The nanoparticles were created on the surface of the mycelia. After analyzing the results and noting the solution, it was determined that the Ag + ions are initially trapped on the surface of the fungal cells by an electrostatic interaction between gold ions and negatively charged carboxylate groups, which is facilitated by enzymes that are present in the mycelia’s cell wall. Later, the enzymes in the cell wall reduce the silver ions, causing the development of silver nuclei. These nuclei then increase as more Ag ions are reduced and accumulate on them.
The TEM data demonstrate the presence of some silver nanoparticles both on and inside the cytoplasmic membrane. The findings concluded that the Ag ions that permeate through the cell wall were decreased by enzymes found inside the cytoplasm and on the cytoplasmic membrane. Also possible is the diffusion of some silver nanoparticles over the cell wall and eventual cytoplasmic entrapment (Mukherjee et al., 2001; Hulkoti and Taranath, 2014).
It was observed that the culture’s age does not affect the shape of the synthesized gold nanoparticles. However, the number of particles decreased when older cells were used. The different pH levels produce a variety of shapes of gold nanoparticles, indicating that pH plays a vital role in determining the shape. The incubation temperature also played an essential role in the accumulation of the gold nanoparticles. It was observed that the particle growth rate was faster at increased temperature levels (Mukherjee et al., 2001; Ahmad et al., 2003). The form of the produced gold nanoparticles was shown to be unaffected by the age of the culture. However, when older cells were utilized, the particle count fell. The fact that gold nanoparticles take on various forms at different pH levels suggests that the pH is crucial in determining the shape. The incubation temperature significantly influenced the accumulation of the gold nanoparticles. It was found that higher temperatures caused the particle development rate to accelerate (Mukherjee et al., 2001; Ahmad et al., 2003). Verticillium luteoalbum is reported to synthesize gold nanoparticles of 20–40 nm in size (Erasmus et al., 2014). Aspergillus terreus and Penicillium brevicompactum KCCM 60390 based metal NPs are reported for their antimicrobial (Li G. et al., 2011) and cytotoxic activities (Mishra et al., 2011), respectively.
Biosynthesis of NPs using actinomycetes: Actinomycetes have been categorized as prokaryotes since they share significant traits with fungi. They are sometimes referred to as ray fungi (Mathew et al., 2010). Making NPs from actinomycetes is the same as that of fungi (Sowani et al., 2016). Thermomonospora sp., a new species of extremophilic actinomycete, was discovered to produce extracellular, monodispersed, spherical gold nanoparticles with an average size of 8 nm (Narayanan and Sakthivel, 2010). Metal NPs synthesized by Rhodococcus sp. (Ahmad et al., 2003) and Streptomyces sp. Al-Dhabi-87 (Al-Dhabi et al., 2018) are reported for their antimicrobial activities.
Biosynthesis of NPs using algae: Algae have a high concentration of polymeric molecules, and by reducing them, they may hyper-accumulate heavy metal ions and transform them into malleable forms. Algal extracts typically contain pigments, carbohydrates, proteins, minerals, polyunsaturated fatty acids, and other bioactive compounds like antioxidants that are used as stabilizing/capping and reducing agents (Khanna et al., 2019). NPs also have a faster rate of photosynthesis than their biosynthetic counterparts. Live or dead algae are used as model organisms for the environmentally friendly manufacturing process of bio-nanomaterials, such as metallic NPs (Hasan, 2015). Ag and Au are the most extensively researched noble metals to synthesized NPs by algae either intracellularly or extracellularly (Dahoumane et al., 2017). Chlorella vulgaris (Luangpipat et al., 2011), Chlorella pyrenoidosa (Eroglu et al., 2013), Nanochloropsis oculata (Xia et al., 2013), Scenedesmus sp. IMMTCC-25 (Jena et al., 2014) based metal NPs are reported for their potential catalytic (Luangpipat et al., 2011; Eroglu et al., 2013) and, antimicrobial (Eroglu et al., 2013; Jena et al., 2014) activities along with their use in Li-Ion batteries (Xia et al., 2013).
Intracellular synthesis of NPs using algae: In order to create intracellular NPs, algal biomass must first be gathered and thoroughly cleaned with distilled water. After that, the biomass (living algae) is treated with metallic solutions like AgNO3. The combination is then incubated at a specified pH and a specific temperature for a predetermined time. Finally, it is centrifuged and sonicated to produce the extracted stable NPs (Uzair et al., 2020).
Extracellular synthesis of NPs using algae: Algal biomass is first collected and cleaned with distilled water before being used to synthesize NPs extracellularly (Uzair et al., 2020). The following three techniques are frequently utilized for the subsequent procedure:
(i) A particular amount of time is spent drying the algal biomass (dead algae), after which the dried powder is treated with distilled water and filtered.
(ii) The algal biomass is sonicated with distilled water to get a cell-free extract.
(iii) The resultant product is filtered after the algal biomass has been rinsed with distilled water and incubated for a few hours (8–16 h).
5.2.6.2. Biological synthesis using plant extracts
The substance or active ingredient of the desired quality extracted from plant tissue by treatment for a particular purpose is a plant extract (Jadoun et al., 2021). Plant extracts are combined with a metal salt solution at room temperature to create nanoparticles. Within minutes, the response is finished. This method has been used to create nanoparticles of silver, gold, and many other metals (Li X. et al., 2011). Nanoparticles are biosynthesized using a variety of plants. It is known that the kind of plant extract, its concentration, the concentration of the metal salt, the pH, temperature, and the length of contact time all have an impact on how quickly nanoparticles are produced as well as their number and other properties (Mittal and Chisti, 2013). A leaf extract from Polyalthia longifolia was used to create silver nanoparticles, the average particle size was around 58 nm (Kumar and Yadav, 2009; Kumar et al., 2016).
Acacia auriculiformis (Saini et al., 2016), Anisomeles indica (Govindarajan et al., 2016), Azadirachta indica (Velusamy et al., 2015), Bergenia ciliate (Phull et al., 2016), Clitoria ternatea, Solanum nigrum (Krithiga et al., 2013), Coffea arabica (Dhand et al., 2016), Coleus forskohlii (Naraginti et al., 2016), Curculigo orchioides (Kayalvizhi et al., 2016), Digitaria radicosa (Kalaiyarasu et al., 2016), Dioscorea alata (Pugazhendhi et al., 2016), Diospyros paniculata (Rao et al., 2016), Elephantopus scaber (Kharat and Mendhulkar, 2016), Emblica officinalis (Ramesh et al., 2015), Euphorbia antiquorum L. (Rajkuberan et al., 2017), Ficus benghalensis (Nayak et al., 2016), Lantana camara (Ajitha et al., 2015), Cinnamomum zeylanicum (Soni and Sonam, 2014), and Parkia roxburghii (Paul et al., 2016) are the few examples of plants which are reported for the green synthesis of metal NPs (i.e., AgNPs). These were evaluated for their antifilaria activity (Saini et al., 2016), mosquitocidal activity (Govindarajan et al., 2016), antibacterial activity (Velusamy et al., 2015), catalytic activity (Edison et al., 2016), antioxidant activity (Phull et al., 2016), and Cytotoxicity (Patil et al., 2017).
5.2.6.3. Biological synthesis using biomimetic
“Biomimetic synthesis” typically refers to chemical processes that resemble biological synthesis carried out by living things (Dahoumane et al., 2017). In the biomimetic approach, proteins, enzymes, cells, viruses, pollen, and waste biomass are used to synthesize NPs. Two categories are used to classify biomimetic synthesis:
Functional biomimetic synthesis uses various materials and approaches to emulate particular characteristics of natural materials, structures, and systems (Zan and Wu, 2016).
Process biomimetic synthesis is a technique that aims to create different desirable nanomaterials/structures by imitating the synthesis pathways, processes, or procedures of natural chemicals and materials/structures. For instance, several distinctive nano-superstructures (such as satellite structures, dendrimer-like structures, pyramids, cubes, 2D nanoparticle arrays, 3D AuNP tubes, etc.) have been put together in vitro by simulating the protein manufacturing process (Zan and Wu, 2016).
6. Applications of NPs
6.1. Applications of NPs in environment industry
Due to their tiny size and distinctive physical and chemical characteristics, NPs appeal to various environmental applications. The properties of nanoparticals and their advantages are illustrated in Figure 4. The following are some possible NP uses in the environment.
6.1.1. Bioremediation
Nanoparticles (NPs) can remove environmental pollutants, such as heavy metals from water or organic contaminants from soil (Zhuang and Gentry, 2011). For example, silver nanoparticles (AgNPs) effectively degrade certain pollutants, such as organic dyes and compounds found in wastewater. Several nanomaterials have been considered for remediation purposes, such as nanoscale zeolites, metal oxides, and carbon nanotubes and fibers (Zhuang and Gentry, 2011). Nanoscale particles used in remediation can access areas that larger particles cannot. They can be coated to facilitate transport and prevent reaction with surrounding soil matrices before reacting with contaminants. One widely used nanomaterial for remediation is Nanoscale zerovalent iron (nZVI). It has been used at several hazardous waste sites to clean up chlorinated solvents that have contaminated groundwater (Elliott et al., 2013). Removing heavy metals such as mercury, lead, thallium, cadmium, and arsenic from natural water has attracted considerable attention because of their adverse effects on environmental and human health. Superparamagnetic iron oxide NPs are an effective sorbent material for this toxic soft material. So, no measurements of engineered NPs in the environment have been available due to the absence of analytical methods able to quantify the trace concentration of NPs (Elliott et al., 2013).
6.1.2. Sensors in environment
Nanotechnology/NPs are already being used to improve water quality and assist in environmental clean-up activities (Pradeep, 2009). Their potential use as environmental sensors to monitor pollutants is also becoming viable NPs can be used as sensors to detect the presence of certain compounds in the environment, such as heavy metals or pollutants. The nano-sensors small size and wide detection range provide great flexibility in practical applications. It has been reported that nanoscale sensors can be used to detect microbial pathogens and biological compounds, such as toxins, in aqueous environments (Yadav et al., 2010). NPS can be designed to selectively bind to specific types of pollutants, allowing them to be detected at low concentrations. For example, gold nanoparticles (AuNPs) have been used as sensors for the detection of mercury in water (Theron et al., 2010).
6.1.3. Catalysts in environment
Nanoparticles (NPs) are used as catalysts in chemical reactions, such as in the production of biofuels or environmental remediation processes, and to catalyze biomass conversion into fuels, such as ethanol or biodiesel. For example, platinum nanoparticles (PtNPs) have been explored for use in the production of biofuels due to their ability to catalyze the conversion of biomass into fuels (Lam and Luong, 2014). PtNPs also showed promising sensing properties; for example, Using Pt NPs, the Hg ions were quantified in the range of 50–500 nM in MilliQ, tap, and groundwater samples, and the limit of quantifications for Hg ions were 16.9, 26, and 47.3 nM. The biogenic PtNPs-based probe proved to be applicable for detecting and quantifying Hg ions (Kora and Rastogi, 2018).
Overall, NPs have significant potential for use in the environment and are being actively researched for a variety of applications.
6.2. Applications of NPs in medicine industry
Nanoparticles (NPs) have unique physical and chemical properties due to their small size, making them attractive for use in various applications, including the medicine industry. Some potential applications of NPs in medicine include:
6.2.1. Drug delivery
Technological interest has been given to AuNPs due to their unique optical properties, ease of synthesis, and chemical stability. The particles can be used in biomedical applications such as cancer treatment (Sun et al., 2014), biological imaging (Abdulle and Chow, 2019), chemical sensing, and drug delivery. Sun et al. (2014) mentioned in detail about two different methods of controlled release of drugs associated with NPs, which were (1) sustained (i.e., diffusion-controlled and erosion-controlled) and (2) stimuli-responsive (i.e., pH-sensitive, enzyme-sensitive, thermoresponsive, and photosensitive). Figure 5 illustrates that how NPs acts as targeted delivery of medicines to treat cancer cells (Figure 5A) and therapeutic gene delivery to synthesis proteins of interests in targeted cells (Figure 5B). NPs can deliver drugs to specific body areas, allowing for more targeted and effective treatment (Siddique and Chow, 2020). For example AgNPs have been explored for use in drug delivery due to their stability and ability to accumulate in certain types of cancerous tumors (Siddique and Chow, 2020). ZnONPs have also been explored for drug delivery due to their ability to selectively target cancer cells (Anjum et al., 2021). CuNPs have been shown to have antimicrobial properties and are being explored for drug delivery to treat bacterial infections (Yuan et al., 2018). AuNPs have unique optical, electrical, and catalytic properties and are being explored for drug delivery due to their ability to accumulate in certain cancerous tumors. Silver NPs (AgNPs) have been incorporated into wound dressings, bone cement, and implants (Schröfel et al., 2014).
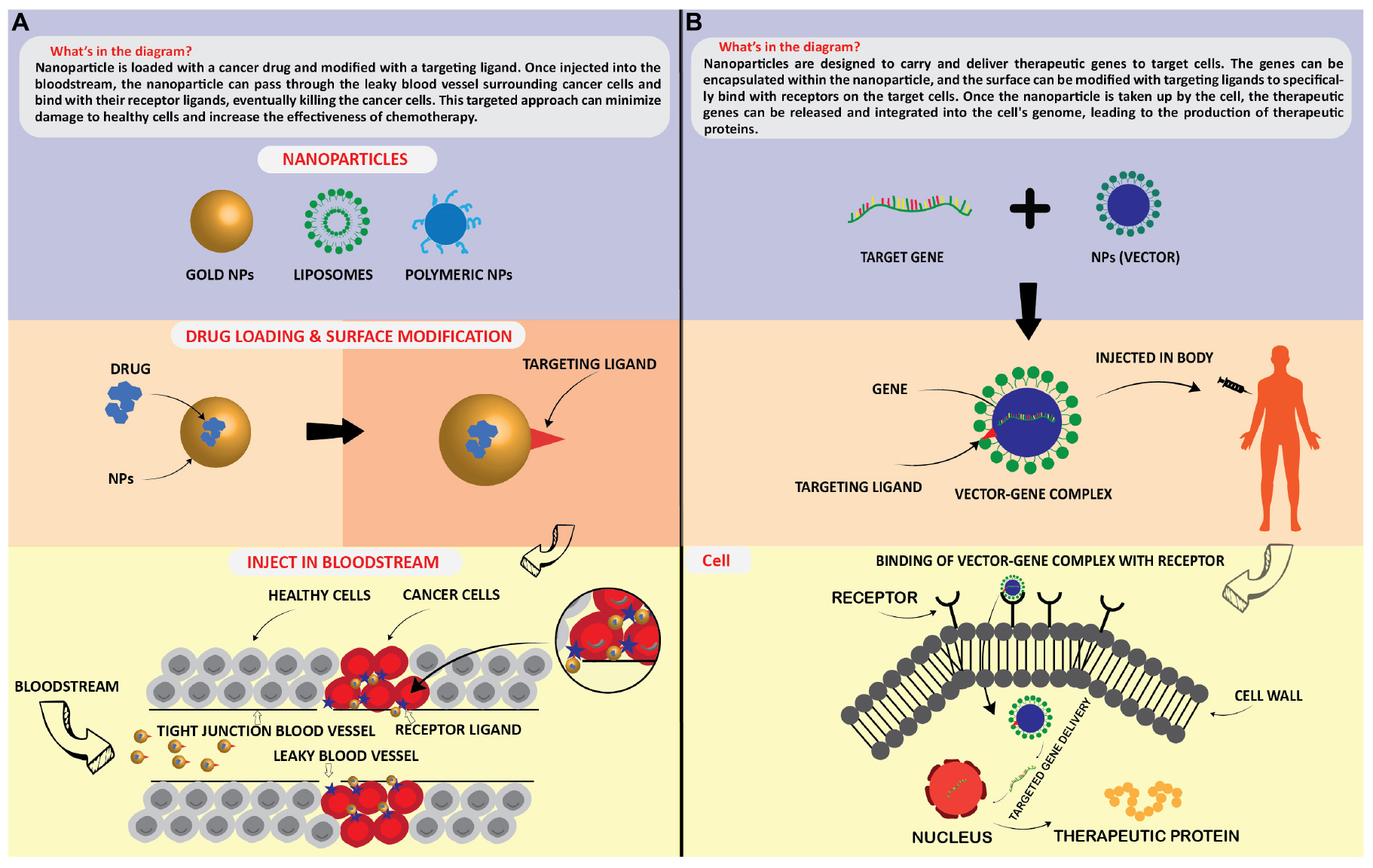
Figure 5. Application of nanoparticles as; targated drug delivery (A), and therapeutic protein generation in targated cells (B).
6.2.2. Diagnostics
Nanoparticles (NPs) can be used as imaging agents to help visualize specific body areas. For example, iron oxide nanoparticles (Fe3O4 NPs) have been used as magnetic resonance imaging (MRI) contrast agents to help visualize tissues and organs (Nguyen et al., 2013). AuNPs have unique optical, electrical, and catalytic properties and are being explored for diagnostics due to their ability to accumulate in certain cancerous tumors (Siddique and Chow, 2020).
6.2.3. Tissue engineering
Nanoparticles (NPs) can help stimulate the growth and repair of tissues and organs. For example, titanium dioxide nanoparticles (TiO2 NPs) have been explored for tissue engineering due to their ability to stimulate the growth of bone cells (Kim et al., 2014).
6.2.4. Antimicrobials
Some NPs, such as silver nanoparticles (AgNPs) and copper nanoparticles (CuNPs), have strong antimicrobial properties and are being explored for use in a variety of medical products, such as wound dressings and medical devices (Hoseinzadeh et al., 2017).
Overall, NPs have significant potential for use in the medical industry and are being actively researched for various applications. However, it is essential to carefully consider the potential risks and benefits of using NPs in medicine and ensure their safe and responsible use.
6.3. Applications of NPs in agriculture industry
There are several ways in which nanoparticles (NPs) have the potential to alter the agricultural sector. NPs may be used in agriculture for a variety of reasons, including:
6.3.1. Pesticides and herbicides
Nanoparticles (NPs) can be used to deliver pesticides and herbicides in a targeted manner, reducing the number of chemicals needed and minimizing the potential for environmental contamination (Khan et al., 2019). AgNPs and CuNPs have antimicrobial properties, making them potentially useful for controlling pests and diseases in crops. They can also be used as delivery systems for active ingredients, allowing for more targeted application and reducing the potential for environmental contamination (Hoseinzadeh et al., 2017; Dangi and Verma, 2021).
It is important to note that using metal NPs in pesticides and herbicides is still in the early stages of development. More research is needed to understand their potential impacts on human health and the environment (Dangi and Verma, 2021).
6.3.2. Fertilizers and plant growth
Nano fertilizers offer an opportunity for efficiently improving plant mineral nutrition. Some studies have shown that nanomaterials can be more effective than conventional fertilizers, with a controlled release of nutrients increasing the efficiency of plant uptake and potentially reducing adverse environmental outcomes associated with the loss of nutrients in the broader environment. However, other studies have found that nanomaterial has the same or even less effective effectiveness than conventional fertilizers. NPs used to deliver fertilizers to plants more efficiently, reducing the amount of fertilizer needed, and reducing the risk of nutrient runoff (Kopittke et al., 2019).
Ag (Jaskulski et al., 2022), Zn (Song and Kim, 2020), Cu, Au, Al, and Fe (Kopittke et al., 2019) based NPs have been shown to have fertilizing properties and plant growth-promoting properties, and may help provide essential nutrients to plants and improve plant growth and yield. It is important to note that the use of NPs in fertilizers is still in the early stages of development. More research is needed to understand their potential impacts on human health and the environment.
6.3.3. Food safety
Nanoparticles (NPs) can detect and eliminate pathogens in food products, improving food safety, and reducing the risk of foodborne illness (Zhuang and Gentry, 2011).
6.3.4. Water purification
Nanoparticles (NPs) can purify irrigation water, reducing the risk of crop contamination and improving crop yield (Zhuang and Gentry, 2011). Using NPs in agriculture can improve crop yields, reduce agriculture’s environmental impact, and improve food products’ safety and quality.
6.4. Applications of NPs in food industry
Numerous applications for nanoparticles (NPs) in the food sector are possible, including:
6.4.1. Food processing and food preservation/food packaging
Nanoparticles (NPs) can be used to improve the efficiency and performance of food processing operations, such as grinding, mixing, and drying, e.g., AgNPs have been used as a natural antimicrobial agent in food processing operations, helping to prevent the growth of bacteria and other microorganisms (Dangi and Verma, 2021) and also NPs are used to enhance the performance of materials used in food packaging, making them more resistant to pollutants like moisture and gases.
6.4.2. Food fortification
Nanoparticles (NPs) can deliver essential nutrients to food products, such as vitamins and minerals, more efficiently and effectively. e.g., Fe2O3, and CuNPs have been used to fortify food products with iron, and Cu is an essential nutrient necessary for the metabolism of iron and other nutrients. Iron is an essential nutrient often lacking in many people’s diets, particularly in developing countries (Kopittke et al., 2019).
6.4.3. Sensors
Nanoparticles (NPs) used to improve the sensitivity and specificity of food sensors, allowing them to detect a broader range of substances or signals (Yadav et al., 2010).
Overall, using NPs in the food industry can improve the performance, safety, and nutritional value of a wide range of food products and processes.
6.5. Applications of NPs in electronics industry and automotive industry
In many aspects, nanoparticles (NPs) can transform the electronics sector. NPs may be used in a variety of electrical applications, such as:
6.5.1. Display technologies/storage devices
Nanoparticles (NPs) can be used to improve the performance of displays (Park and Choi, 2019; Bahadur et al., 2021; Triana et al., 2022), such as LCD and OLED displays, by enhancing the brightness, color, and contrast of the image, such as silver NPs and gold NPs, have been explored for use in LCD and OLED displays as a means of improving the conductivity of the display (Gwynne, 2020). NPs improve the performance and durability of energy storage devices, such as batteries and supercapacitors, by increasing energy density and charging speed. Zinc oxide nanoparticles (ZnO NPs) have the potential to be used in energy storage devices, such as batteries and supercapacitors, due to their ability to store and release energy (Singh et al., 2011).
6.5.2. Data storage
Nanoparticles (NPs) can improve the capacity and speed of data storage devices, such as hard drives and flash drives. Magnetic NPs, such as iron oxide NPs, have been explored for use in data storage devices, such as hard drives, due to their ability to store, and retrieve data using magnetism. These NPs are often composed of a magnetic metal, such as iron, cobalt, or nickel. They can be magnetized and demagnetized, allowing them to store and retrieve data (Ahmad et al., 2021).
Overall, the use of NPs in electronics has the potential to improve the performance and efficiency of a wide range of electronic devices and systems.
Applications of NPs in chemical industry: The chemical industry might be entirely transformed by nanoparticles (NPs) in various ways. The following are potential uses for NPs in the chemical industry (Salem and Fouda, 2021).
6.5.3. Chemical processing/catalysis
Nanoparticles (NPs) can be used as catalysts in chemical reactions, allowing them to be carried out more efficiently and at lower temperatures. Some examples of metal NPs that have been used as catalysts in the chemical industry include: PtNPs have been used as catalysts in a variety of chemical reactions, including fuel cell reactions (Bhavani et al., 2021), hydrogenation reactions, and oxidation reactions (Lara and Philippot, 2014), PdNPs have been used as catalysts in a variety of chemical reactions, including hydrogenation reactions and cross-coupling reactions (Pérez-Lorenzo, 2012), FeNPs have been used as catalysts in a variety of chemical reactions, including hydrolysis reactions (Jiang and Xu, 2011), and oxygen reduction reactions, NiNPs have been used as catalysts in a variety of chemical reactions, including hydrogenation reactions, and hydrolysis reactions (Salem and Fouda, 2021).
6.5.4. Separation and purification
NPs are used to separate and purify chemicals and other substances, such as gases and liquids, by exploiting their size-based properties (Hollamby et al., 2010). Several types of metal nanoparticles (NPs) have been explored for use in separation and purification processes in the chemical industry, including Fe2O3 NPs have been used to separate and purify gases, liquids, and chemicals. They have also been used to remove contaminants from water (Pradeep, 2009; Siddique and Chow, 2020). AgNPs have been used to purify water and remove contaminants (Pradeep, 2009), such as bacteria and viruses. They have also been used to remove heavy metals from water and other substances (Zhuang and Gentry, 2011). AuNPs have been used to purify water and remove contaminants, such as bacteria and viruses (Siddique and Chow, 2020). They have also been used to separate and purify gases and liquids (Zhuang and Gentry, 2011). AlNPs have been used to remove contaminants from water and other substances, such as oils and fuels. They have also been used to purify gases (Zhuang and Gentry, 2011).
6.6. Applications of NPs in defense industry
Nanoparticles (NPs) can be used to improve the efficiency and performance of chemical processing operations, such as refining and synthesizing chemicals (Schröfel et al., 2014). Nanoparticles (NPs) have the potential to be used in the defense industry in several ways, including:
6.6.1. Sensors
Nanoparticles (NPs) can improve the sensitivity and specificity of sensors used in defense systems, such as sensors for detecting chemical, biological, or radiological threats (Zheng et al., 2010).
6.6.2. Protective coatings
Nanoparticles (NPs) can improve the performance and durability of protective coatings applied to defense equipment, such as coatings resistant to chemical or biological agents. For example, metal NPs can improve the mechanical properties and durability of the coating, making it more resistant to wear and corrosion. For example, adding Al or Zn based NPs to a polymer coating can improve its corrosion resistance. In contrast, adding Ni or Cr-based NPs can improve their wear resistance (Rangel-Olivares et al., 2021).
6.6.3. Weapons
Nanoparticles (NPs) are used as weapons against viruses, bacteria, etc, (Ye et al., 2020) and as well as in the development of armor and protective materials. There have been some reports of the potential use of NPs in military and defense applications, such as in the development of armor and protective materials. For example, adding nanoparticles, such as ceramic or metal NPs, to polymers or other materials can improve their mechanical properties and make them more resistant to damage. In addition, there have been reports of the use of NPs in developing sensors and detection systems for defense purposes.
6.6.4. Manufacturing
Nanoparticles (NPs) can improve the performance and durability of materials used in defense equipment, such as armor or structural materials. Metal NPs can be used in materials by adding them as a filler or reinforcement in polymers. For example, the addition of metal NPs such as aluminum (Al), copper (Cu), or nickel (Ni) to polymers can improve the mechanical properties, thermal stability, and electrical conductivity of the resulting composite material (Khan et al., 2019).
Metal NPs can also make functional materials, such as catalysts and sensors. For example, metal NPs, such as gold (Au), and platinum (Pt), can be used as catalysts in various chemical reactions due to their high surface area and ability to adsorb reactants (Zheng et al., 2010).
6.6.5. Energy storage
Nanoparticles (NPs) can improve the performance and efficiency of energy storage systems used in defense systems, such as batteries or fuel cells (Morsi et al., 2022). In batteries, nanoparticles can be used as a cathode material to increase the battery’s energy density, rate capability, and cycling stability. For example, lithium cobalt oxide (LiCoO2) nanoparticles have been used as cathode materials in lithium-ion batteries due to their high capacity and good rate performance. In addition, nanoparticles of transition metal oxides, such as iron oxide (Fe2O3), and manganese oxide (MnO2), have been used as cathode materials in rechargeable lithium batteries due to their high capacity and good rate performance. In supercapacitors, nanoparticles can be used as the active material in the electrodes to increase the specific surface area, leading to an increase in the device’s capacitance (Morsi et al., 2022). Using NPs in the defense industry can improve defense systems’ performance, efficiency, and safety.
7. Future perspectives
Metal nanoparticles (NPs) have many potential applications in various fields, including electronics, energy storage, catalysis, and medicine. However, there are also several challenges and potential future directions for developing and using metal NPs.
One major challenge is synthesizing and processing metal NPs with precise size and shape control. Many methods for synthesizing metal NPs involve high temperatures and harsh chemical conditions, which can be challenging to scale up for large-scale production. In addition, the size and shape of metal NPs can significantly impact their properties and potential applications, so it is essential to synthesize NPs with precise size and shape control.
Another challenge is the environmental impact of metal NPs. Some metal NPs, such as silver NPs, can be toxic to aquatic life and may have other environmental impacts. There is a need for more research on the environmental effects of metal NPs and the development of more environmentally friendly (Green) synthesis and processing methods.
In terms of future directions, one promising area is the use of metal NPs for energy storage, conversion, and protection of the environment. For example, metal NPs could be used to improve batteries’ performance or develop more efficient solar cells. In addition, metal NPs could be used in catalysis to improve the efficiency of chemical reactions. There is also ongoing research on metal NPs in medicine, including drug delivery and cancer therapy.
Author contributions
KAA: conceptualization, methodology, validation, formal analysis, investigation, writing – original draft, writing – review and editing, and visualization.
Acknowledgments
The author thanks Prof. Dr. Mona M. Sobhy, Department of Reproductive Diseases, Animal Reproduction Research Institute, ARC, Giza, Egypt, and Dr. Omar Hewedy, University of Guelph, Canada, for the critical reading of the manuscript.
Conflict of interest
The author declares that the research was conducted in the absence of any commercial or financial relationships that could be construed as a potential conflict of interest.
Publisher’s note
All claims expressed in this article are solely those of the authors and do not necessarily represent those of their affiliated organizations, or those of the publisher, the editors and the reviewers. Any product that may be evaluated in this article, or claim that may be made by its manufacturer, is not guaranteed or endorsed by the publisher.
References
Abdulle, A., and Chow, J. C. (2019). Contrast enhancement for portal imaging in nanoparticle-enhanced radiotherapy: A Monte Carlo phantom evaluation using flattening-filter-free photon beams. Nanomaterials 9:920. doi: 10.3390/nano9070920
Ago, H. (2015). “CVD growth of high-quality single-layer graphene,” in Frontiers of Graphene and Carbon Nanotubes, Ed. K. Matsumoto (Berlin: Springer), 3–20. doi: 10.1007/978-4-431-55372-4_1
Ahmad, A., Alsaad, A., Al-Bataineh, Q. M., Al-Akhras, M.-A. H., Albataineh, Z., Alizzy, K. A., et al. (2021). Synthesis and characterization of ZnO NPs-doped PMMA-BDK-MR polymer-coated thin films with UV curing for optical data storage applications. Polymer Bull. 78, 1189–1211. doi: 10.1007/s00289-020-03155-x
Ahmad, A., Senapati, S., Khan, M. I., Kumar, R., Ramani, R., Srinivas, V., et al. (2003). Intracellular synthesis of gold nanoparticles by a novel alkalotolerant actinomycete. Rhodococcus species. Nanotechnology 14:824. doi: 10.1088/0957-4484/14/7/323
Ahmad, T., Wani, I. A., Ahmed, J., and Al-Hartomy, O. A. (2014). Effect of gold ion concentration on size and properties of gold nanoparticles in TritonX-100 based inverse microemulsions. Appl. Nanosci. 4, 491–498. doi: 10.1007/s13204-013-0224-y
Ajitha, B., Reddy, Y. A. K., and Reddy, P. S. (2015). Green synthesis and characterization of silver nanoparticles using Lantana camara leaf extract. Mater. Sci. Eng. C 49, 373–381. doi: 10.1016/j.msec.2015.01.035
Al-Dhabi, N. A., Mohammed Ghilan, A.-K., and Arasu, M. V. (2018). Characterization of silver nanomaterials derived from marine Streptomyces sp. al-dhabi-87 and its in vitro application against multidrug resistant and extended-spectrum beta-lactamase clinical pathogens. Nanomaterials 8:279. doi: 10.3390/nano8050279
Amendola, V., and Meneghetti, M. (2009). Laser ablation synthesis in solution and size manipulation of noble metal nanoparticles. Phys. Chem. Chem. Phys. 11, 3805–3821. doi: 10.1039/b900654k
Anjum, S., Hashim, M., Malik, S. A., Khan, M., Lorenzo, J. M., Abbasi, B. H., et al. (2021). Recent advances in zinc oxide nanoparticles (Zno nps) for cancer diagnosis, target drug delivery, and treatment. Cancers 13:4570. doi: 10.3390/cancers13184570
Astefanei, A., Núñez, O., and Galceran, M. T. (2015). Characterisation and determination of fullerenes: a critical review. Anal. Chim. Acta 882, 1–21.
Bahadur, P. S., Jaiswal, S., Srivastava, R., and Kumar, A. (2021). “Advanced application of nanotechnology in engineering,” in Proceedings of the 2021 International Conference on Technological Advancements and Innovations (ICTAI), (Piscataway, NJ: IEEE), 92–95.
Baig, N., Kammakakam, I., and Falath, W. (2021). Nanomaterials: A review of synthesis methods, properties, recent progress, and challenges. Mater. Adv. 2, 1821–1871.
Banerjee, A., Krishna, R., and Das, B. (2008). Size controlled deposition of Cu and Si nano-clusters by an ultra-high vacuum sputtering gas aggregation technique. Appl. Phys. A 90, 299–303.
Bayda, S., Adeel, M., Tuccinardi, T., Cordani, M., and Rizzolio, F. (2019). The history of nanoscience and nanotechnology: from chemical–physical applications to nanomedicine. Molecules 25:112. doi: 10.3390/molecules25010112
Behrisch, R. (1981). Sputtering by Particle Bombardment Springer Verlag. Berlin-Heidelberg: Springer.
Berkmans, A. J., Jagannatham, M., Priyanka, S., and Haridoss, P. (2014). Synthesis of branched, nano channeled, ultrafine and nano carbon tubes from PET wastes using the arc discharge method. Waste Manag. 34, 2139–2145. doi: 10.1016/j.wasman.2014.07.004
Beyene, H. D., Werkneh, A. A., Bezabh, H. K., and Ambaye, T. G. (2017). Synthesis paradigm and applications of silver nanoparticles (AgNPs), a review. Sustain. Mater. Technol. 13, 18–23.
Bhattacharjee, S. (2016). DLS and zeta potential–what they are and what they are not? J. Control. Release 235, 337–351.
Bhavani, K. S., Anusha, T., and Brahman, P. K. (2021). Platinum nanoparticles decorated on graphitic carbon nitride-ZIF-67 composite support: An electrocatalyst for the oxidation of butanol in fuel cell applications. Int. J. Hydr. Energy 46, 9199–9214.
Biju, V., Itoh, T., Anas, A., Sujith, A., and Ishikawa, M. (2008). Semiconductor quantum dots and metal nanoparticles: syntheses, optical properties, and biological applications. Anal. Bioanal. Chem. 391, 2469–2495.
Brady, B., Wang, P. H., Steenhoff, V., and Brolo, A. G. (2019). “Nanostructuring solar cells using metallic nanoparticles,” in Metal Nanostructures for Photonics, eds L. R. P. Kassab, and C. B. De Araujo (Amsterdam: Elsevier), 197–221.
Cadene, A., Durand-Vidal, S., Turq, P., and Brendle, J. (2005). Study of individual Na-montmorillonite particles size, morphology, and apparent charge. J. Colloid Interf. Sci. 285, 719–730. doi: 10.1016/j.jcis.2004.12.016
Chen, J., and Zhu, X. (2016). Magnetic solid phase extraction using ionic liquid-coated core–shell magnetic nanoparticles followed by high-performance liquid chromatography for determination of Rhodamine B in food samples. Food Chem. 200, 10–15. doi: 10.1016/j.foodchem.2016.01.002
Chen, J., Guo, Y., Zhang, X., Liu, J., Gong, P., Su, Z., et al. (2023). Emerging nanoparticles in food: sources, application, and safety. J. Agricult. Food Chem. 71, 3564–3582.
Chen, J., Wei, S., and Xie, H. (2021). “A brief introduction of carbon nanotubes: history, synthesis, and properties,” in Proceedings of the Journal of Physics: Conference Series, (United Kingdom: IOP Publishing), 012184. doi: 10.1088/1742-6596/1948/1/012184
Chen, J.-C., and Tang, C.-T. (2007). Preparation and application of granular ZnO/Al2O3 catalyst for the removal of hazardous trichloroethylene. J. Hazardous Mater. 142, 88–96. doi: 10.1016/j.jhazmat.2006.07.061
Chronakis, I. S. (2010). Micro-/nano-fibers by electrospinning technology: processing, properties and applications. Micromanufact. Eng. Technol. 2010, 264–286. doi: 10.1016/B978-0-8155-1545-6.00016-8
Compostella, F., Pitirollo, O., Silvestri, A., and Polito, L. (2017). Glyco-gold nanoparticles: synthesis and applications. Beilstein J. Org. Chem. 13, 1008–1021. doi: 10.3762/bjoc.13.100
Dahoumane, S. A., Mechouet, M., Wijesekera, K., Filipe, C. D., Sicard, C., Bazylinski, D. A., et al. (2017). Algae-mediated biosynthesis of inorganic nanomaterials as a promising route in nanobiotechnology–a review. Green Chem. 19, 552–587. doi: 10.1039/C6GC02346K
Dangi, K., and Verma, A. K. (2021). Efficient & eco-friendly smart nano-pesticides: Emerging prospects for agriculture. Mater. Today Proc. 45, 3819–3824.
Das, S., and Srivasatava, V. C. (2016). Synthesis and characterization of ZnO–MgO nanocomposite by co-precipitation method. Smart Sci. 4, 190–195.
De La Calle, I., Menta, M., Klein, M., and Séby, F. (2018). Study of the presence of micro-and nanoparticles in drinks and foods by multiple analytical techniques. Food Chem. 266, 133–145. doi: 10.1016/j.foodchem.2018.05.107
Delvallée, A., Feltin, N., Ducourtieux, S., Trabelsi, M., and Hochepied, J. (2015). Direct comparison of AFM and SEM measurements on the same set of nanoparticles. Measur. Sci. Technol. 26:085601.
Dhand, V., Soumya, L., Bharadwaj, S., Chakra, S., Bhatt, D., and Sreedhar, B. (2016). Green synthesis of silver nanoparticles using Coffea arabica seed extract and its antibacterial activity. Mater. Sci. Eng. C 58, 36–43. doi: 10.1016/j.msec.2015.08.018
Dikusar, A., Globa, P., Belevskii, S., and Sidel’nikova, S. (2009). On limiting rate of dimensional electrodeposition at meso-and nanomaterial manufacturing by template synthesis. Surf. Eng. Appl. Electrochem. 45, 171–179.
Dragovic, R. A., Gardiner, C., Brooks, A. S., Tannetta, D. S., Ferguson, D. J., Hole, P., et al. (2011). Sizing and phenotyping of cellular vesicles using nanoparticle tracking analysis. Nanomedicine 7, 780–788.
Dreaden, E. C., Alkilany, A. M., Huang, X., Murphy, C. J., and El-Sayed, M. A. (2012). The golden age: gold nanoparticles for biomedicine. Chem. Soc. Rev. 41, 2740–2779. doi: 10.1039/c1cs15237h
Du, P., Song, L., Xiong, J., Li, N., Xi, Z., Wang, L., et al. (2012). Coaxial electrospun TiO2/ZnO core–sheath nanofibers film: Novel structure for photoanode of dye-sensitized solar cells. Electrochim. Acta 78, 392–397.
Edison, T. N. J. I., Lee, Y. R., and Sethuraman, M. G. (2016). Green synthesis of silver nanoparticles using Terminalia cuneata and its catalytic action in reduction of direct yellow-12 dye. Pectrochimica Acta Part A 161, 122–129. doi: 10.1016/j.saa.2016.02.044
Elliott, J. A., Shibuta, Y., Amara, H., Bichara, C., and Neyts, E. C. (2013). Atomistic modelling of CVD synthesis of carbon nanotubes and graphene. Nanoscale 5, 6662–6676. doi: 10.1039/c3nr01925j
Erasmus, M., Cason, E. D., Van Marwijk, J., Botes, E., Gericke, M., and Van Heerden, E. (2014). Gold nanoparticle synthesis using the thermophilic bacterium Thermus scotoductus SA-01 and the purification and characterization of its unusual gold reducing protein. Gold Bull. 47, 245–253.
Eroglu, E., Chen, X., Bradshaw, M., Agarwal, V., Zou, J., Stewart, S. G., et al. (2013). Biogenic production of palladium nanocrystals using microalgae and their immobilization on chitosan nanofibers for catalytic applications. RSC Adv. 3, 1009–1012.
Essajai, R., Benhouria, Y., Rachadi, A., Qjani, M., Mzerd, A., and Hassanain, N. (2019). Shape-dependent structural and magnetic properties of Fe nanoparticles studied through simulation methods. RSC Adv. 9, 22057–22063. doi: 10.1039/c9ra03047f
Falke, S., and Betzel, C. (2019). “Dynamic light scattering (DLS),” in Radiation in Bioanalysis, eds A. S. Pereira, P. Tavares, P. Limão-Vieira (Berlin: Springer), 173–193.
Farrell, D., Majetich, S. A., and Wilcoxon, J. P. (2003). Preparation and characterization of monodisperse Fe nanoparticles. J. Phys. Chem. B 107, 11022–11030.
Feng, L., Xuan, Z., Ma, J., Chen, J., Cui, D., Su, C., et al. (2015). Preparation of gold nanorods with different aspect ratio and the optical response to solution refractive index. J. Exp. Nanosci. 10, 258–267.
Ghorbani, H. R., Mehr, F. P., Pazoki, H., and Rahmani, B. M. (2015). Synthesis of ZnO nanoparticles by precipitation method. Orient. J. Chem. 31, 1219–1221.
Ghosh, S., Ahmad, R., Zeyaullah, M., and Khare, S. K. (2021). Microbial nano-factories: synthesis and biomedical applications. Front. Chem. 9:194. doi: 10.3389/fchem.2021.626834
Giljohann, D. A., Seferos, D. S., Daniel, W. L., Massich, M. D., Patel, P. C., and Mirkin, C. A. (2020). Gold nanoparticles for biology and medicine. Spherical Nucleic Acids 49, 3280–3294.
Giurlani, W., Innocenti, M., and Lavacchi, A. (2018). X-ray microanalysis of precious metal thin films: thickness and composition determination. Coatings 8:84.
Gloria, E. C., Ederley, V., Gladis, M., César, H., Jaime, O., Oscar, A., et al. (2017). “Synthesis of silver nanoparticles (AgNPs) with antibacterial activity,” in Proceedings of the Journal of Physics: Conference Series, (United Kingdom: IOP Publishing), 012023.
Gorrasi, G., and Sorrentino, A. (2015). Mechanical milling as a technology to produce structural and functional bio-nanocomposites. Green Chem. 17, 2610–2625.
Govindarajan, M., Rajeswary, M., Veerakumar, K., Muthukumaran, U., Hoti, S., and Benelli, G. (2016). Green synthesis and characterization of silver nanoparticles fabricated using Anisomeles indica: mosquitocidal potential against malaria, dengue and Japanese encephalitis vectors. Exp. Parasitol. 161, 40–47. doi: 10.1016/j.exppara.2015.12.011
Graf, C., Vossen, D. L., Imhof, A., and Van Blaaderen, A. (2003). A general method to coat colloidal particles with silica. Langmuir 19, 6693–6700.
Greczynski, G., and Hultman, L. (2020). X-ray photoelectron spectroscopy: towards reliable binding energy referencing. Progr. Mater. Sci. 107:100591.
Guo, D., Xie, G., and Luo, J. (2013). Mechanical properties of nanoparticles: basics and applications. J. Phys. D 47:013001.
Guo, W., Pleixats, R., and Shafir, A. (2015). Water-soluble gold nanoparticles: from catalytic selective Nitroarene reduction in water to refractive index sensing. Chem. An Asian J. 10, 2437–2443. doi: 10.1002/asia.201500290
Gwynne, K. (2020). Enhancement of the Photostability of Blue Phosphorescence Using Plasmonic Surfaces. New Brunswick, NJ: Rutgers University-School of Graduate Studies.
Haasch, R. T. (2014). “X-ray photoelectron spectroscopy (XPS) and auger electron spectroscopy (AES),” in Practical Materials Characterization, Ed. M. Sardela (Berlin: Springer), 93–132.
Hasan, S. (2015). A review on nanoparticles: their synthesis and types. Res. J. Recent Sci. 2277:2502.
Holder, C. F., and Schaak, R. E. (2019). Tutorial on Powder X-ray Diffraction for Characterizing Nanoscale Materials. Washington, DC: ACS Publications.
Hollamby, M. J., Eastoe, J., Chemelli, A., Glatter, O., Rogers, S., Heenan, R. K., et al. (2010). Separation and purification of nanoparticles in a single step. Langmuir 26, 6989–6994. doi: 10.1021/la904225k
Hoo, C. M., Starostin, N., West, P., and Mecartney, M. L. (2008). A comparison of atomic force microscopy (AFM) and dynamic light scattering (DLS) methods to characterize nanoparticle size distributions. J. Nanopart. Res. 10, 89–96.
Hortin, J., Anderson, A., Britt, D., Jacobson, A., and Mclean, J. (2020). Copper oxide nanoparticle dissolution at alkaline pH is controlled by dissolved organic matter: influence of soil-derived organic matter, wheat, bacteria, and nanoparticle coating. Environ. Sci. 7, 2618–2631.
Hoseinzadeh, E., Makhdoumi, P., Taha, P., Hossini, H., Stelling, J., and Amjad Kamal, M. (2017). A review on nano-antimicrobials: metal nanoparticles, methods and mechanisms. Curr. Drug Metab. 18, 120–128.
Hulkoti, N. I., and Taranath, T. (2014). Biosynthesis of nanoparticles using microbes—a review. Colloids Surf. B Biointerf. 121, 474–483. doi: 10.1016/j.colsurfb.2014.05.027
Islam, F., Shohag, S., Uddin, M. J., Islam, M. R., Nafady, M. H., Akter, A., et al. (2022). Exploring the journey of zinc oxide nanoparticles (ZnO-NPs) toward biomedical applications. Materials 15:2160. doi: 10.3390/ma15062160
Jadoun, S., Arif, R., Jangid, N. K., and Meena, R. K. (2021). Green synthesis of nanoparticles using plant extracts: A review. Environ. Chem. Lett. 19, 355–374.
Jamkhande, P. G., Ghule, N. W., Bamer, A. H., and Kalaskar, M. G. (2019). Metal nanoparticles synthesis: An overview on methods of preparation, advantages and disadvantages, and applications. J. Drug Deliv. Sci. Technol. 53, 101174.
Jana, N. R., Earhart, C., and Ying, J. Y. (2007). Synthesis of water-soluble and functionalized nanoparticles by silica coating. Chem. Mater. 19, 5074–5082.
Jaskulski, D., Jaskulska, I., Majewska, J., Radziemska, M., Bilgin, A., and Brtnicky, M. (2022). Silver Nanoparticles (AgNPs) in urea solution in laboratory tests and field experiments with crops and vegetables. Materials 15:870. doi: 10.3390/ma15030870
Jayaraman, V., Ghosh, S., Sengupta, A., Srivastava, S., Sonawat, H., and Narayan, P. K. (2014). Identification of biochemical differences between different forms of male infertility by nuclear magnetic resonance (NMR) spectroscopy. J. Assist. Reproduct. Genet. 31, 1195–1204. doi: 10.1007/s10815-014-0282-4
Jena, J., Pradhan, N., Nayak, R. R., Dash, B. P., Sukla, L. B., Panda, P. K., et al. (2014). Microalga Scenedesmus sp.: a potential low-cost green machine for silver nanoparticle synthesis. J. Microbiol. Biotechnol. Adv. 24, 522–533. doi: 10.4014/jmb.1306.06014
Jiang, H.-L., and Xu, Q. (2011). Catalytic hydrolysis of ammonia borane for chemical hydrogen storage. Catal. Today 170, 56–63.
Joh, D.-W., Jung, T.-K., Lee, H.-S., and Kim, D.-H. (2013). Synthesis of nanoparticles using electrical explosion of Ni wire in Pt solution. J. Nanosci. Nanotechnol. 13, 6092–6094. doi: 10.1166/jnn.2013.7677
Kahle, M., Kleber, M., and Jahn, R. (2002). Review of XRD-based quantitative analyses of clay minerals in soils: the suitability of mineral intensity factors. Geoderma 109, 191–205.
Kalaiyarasu, T., Karthi, N., Sharmila, G. V., and Manju, V. (2016). In vitro assessment of antioxidant and antibacterial activity of green synthesized silver nanoparticles from Digitaria radicosa leaves. Asian J. Pharm. Clin. Res. 9, 297–302.
Kayalvizhi, T., Ravikumar, S., and Venkatachalam, P. (2016). Green synthesis of metallic silver nanoparticles using Curculigo orchioides rhizome extracts and evaluation of its antibacterial, larvicidal, and anticancer activity. J. Environ. Eng. 142:C4016002.
Khan, A., Rashid, R., Murtaza, G., and Zahra, A. (2014). Gold nanoparticles: synthesis and applications in drug delivery. Trop. J. Pharm. Res. 13, 1169–1177.
Khan, I., Saeed, K., and Khan, I. (2019). Nanoparticles: Properties, applications and toxicities. Arab. J. Chem. 12, 908–931.
Khanna, P., Kaur, A., and Goyal, D. (2019). Algae-based metallic nanoparticles: Synthesis, characterization and applications. J. Microbiol Methods 163:105656. doi: 10.1016/j.mimet.2019.105656
Kharat, S. N., and Mendhulkar, V. D. (2016). Synthesis, characterization and studies on antioxidant activity of silver nanoparticles using Elephantopus scaber leaf extract. Mater. Sci. Eng. C 62, 719–724. doi: 10.1016/j.msec.2016.02.024
Kim, J.-H., Sheikh, F. A., Ju, H. W., Park, H. J., Moon, B. M., Lee, O. J., et al. (2014). 3D silk fibroin scaffold incorporating titanium dioxide (TiO2) nanoparticle (NPs) for tissue engineering. Int. J. Biol. Macromol. 68, 158–168. doi: 10.1016/j.ijbiomac.2014.04.045
Kohl, H., and Reimer, L. (2008). Transmission Electron Microscopy. Berlin: Springer Series in Optical Sciences, 36.
Kokarneswaran, M., Selvaraj, P., Ashokan, T., Perumal, S., Sellappan, P., Murugan, K. D., et al. (2020). Discovery of carbon nanotubes in sixth century BC potteries from Keeladi, India. Sci. Rep. 10, 1–6. doi: 10.1038/s41598-020-76720-z
Kopittke, P. M., Lombi, E., Wang, P., Schjoerring, J. K., and Husted, S. (2019). Nanomaterials as fertilizers for improving plant mineral nutrition and environmental outcomes. Environ. Sci. 6, 3513–3524. doi: 10.3390/biology10111123
Kora, A. J., and Rastogi, L. (2018). Peroxidase activity of biogenic platinum nanoparticles: A colorimetric probe towards selective detection of mercuric ions in water samples. Sens. Actuators B Chem. 254, 690–700.
Kreizer, M., Ratner, D., and Liberzon, A. (2010). Real-time image processing for particle tracking velocimetry. Exp. Fluids 48, 105–110.
Krithiga, N., Jayachitra, A., and Rajalakshmi, A. (2013). Synthesis, characterization and analysis of the effect of copper oxide nanoparticles in biological systems. Ind. J. Ns 1, 6–15.
Kumar, R., Singh, R. K., Dubey, P. K., Kumar, P., Tiwari, R. S., and Oh, I.-K. (2013). Pressure-dependent synthesis of high-quality few-layer graphene by plasma-enhanced arc discharge and their thermal stability. J. Nanopart. Res. 15, 1–10.
Kumar, S. S., Venkateswarlu, P., Rao, V. R., and Rao, G. N. (2013). Synthesis, characterization and optical properties of zinc oxide nanoparticles. Int. Nano Lett. 3, 1–6.
Kumar, V., and Yadav, S. K. (2009). Plant-mediated synthesis of silver and gold nanoparticles and their applications. J. Chem. Technol. Biotechnol. 84, 151–157.
Kumar, V., Bano, D., Mohan, S., Singh, D. K., and Hasan, S. H. (2016). Sunlight-induced green synthesis of silver nanoparticles using aqueous leaf extract of Polyalthia longifolia and its antioxidant activity. Mater. Lett. 181, 371–377.
Kumari, S. C., Dhand, V., and Padma, P. N. (2021). Green synthesis of metallic nanoparticles: a review. Nanomaterials 2021, 259–281.
Lam, E., and Luong, J. H. (2014). Carbon materials as catalyst supports and catalysts in the transformation of biomass to fuels and chemicals. ACS Catal. 4, 3393–3410.
Lara, P., and Philippot, K. (2014). The hydrogenation of nitroarenes mediated by platinum nanoparticles: an overview. Catal. Sci. Technol. 4, 2445–2465.
Lerner, M. I., Glazkova, E. A., Lozhkomoev, A. S., Svarovskaya, N. V., Bakina, O. V., Pervikov, A. V., et al. (2016). Synthesis of Al nanoparticles and Al/AlN composite nanoparticles by electrical explosion of aluminum wires in argon and nitrogen. Powder Technol. 295, 307–314.
Lewczuk, B., and Szyryńska, N. (2021). Field-emission scanning electron microscope as a tool for large-area and large-volume ultrastructural studies. Animals 11:3390. doi: 10.3390/ani11123390
Li, G., He, D., Qian, Y., Guan, B., Gao, S., Cui, Y., et al. (2011). Fungus-mediated green synthesis of silver nanoparticles using Aspergillus terreus. Int. J. Mol. Sci. 13, 466–476. doi: 10.3390/ijms13010466
Li, N., Zhao, P., and Astruc, D. (2014). Anisotropic gold nanoparticles: synthesis, properties, applications, and toxicity. Angewand. Chem. Int. Edn. 53, 1756–1789.
Li, T., Senesi, A. J., and Lee, B. (2016). Small angle X-ray scattering for nanoparticle research. Chem. Rev. 116, 11128–11180. doi: 10.1021/acs.chemrev.5b00690
Li, X., Xu, H., Chen, Z.-S., and Chen, G. (2011). Biosynthesis of nanoparticles by microorganisms and their applications. J. Nanomater. 2011:270974.
Luangpipat, T., Beattie, I. R., Chisti, Y., and Haverkamp, R. G. (2011). Gold nanoparticles produced in a microalga. J. Nanopart. Res. 13, 6439–6445.
Lyon, L. A., Keating, C. D., Fox, A. P., Baker, B. E., He, L., Nicewarner, S. R., et al. (1998). Raman spectroscopy. Anal. Chem. 70, 341–362.
Lyu, H., Gao, B., He, F., Ding, C., Tang, J., and Crittenden, J. C. (2017). Ball-milled carbon nanomaterials for energy and environmental applications. ACS Sust. Chem. Eng. 5, 9568–9585. doi: 10.1016/j.biortech.2020.123613
Machac, P., Cichon, S., Lapcak, L., and Fekete, L. (2020). Graphene prepared by chemical vapour deposition process. Graph. Technol. 5, 9–17.
Madathil, A. N. P., Vanaja, K., and Jayaraj, M. (2007). “Synthesis of ZnO nanoparticles by hydrothermal method,” in Nanophotonic materials IV, eds Z. Gaburro and S. Cabrini (Bellingham, WA: SPIE), 47–55.
Maharani, V., Sundaramanickam, A., and Balasubramanian, T. J. E. (2016). In vitro anticancer activity of silver nanoparticle synthesized by Escherichia coli VM1 isolated from marine sediments of Ennore southeast coast of India. Enzyme Microb. Technol. 95, 146–154. doi: 10.1016/j.enzmictec.2016.09.008
Majeed Khan, M. A., Kumar, S., Ahamed, M., Alrokayan, S. A., and Alsalhi, M. S. (2011). Structural and thermal studies of silver nanoparticles and electrical transport study of their thin films. Nanosc. Res. Lett. 6, 1–8.
Malhotra, S. P. K., and Alghuthaymi, M. A. (2022). Biomolecule-assisted biogenic synthesis of metallic nanoparticles. Agri-Waste Microb. Product. Sust. Nanomater. 2022, 139–163.
Mathew, L., Chandrasekaran, N., and Mukherjee, A. (2010). “Biomimetic synthesis of nanoparticles: science, technology & applicability,” Biomimetics learning from nature, Ed. A. Mukherjee (Norderstedt: Books on Demand).
Mishra, A., Tripathy, S. K., Wahab, R., Jeong, S.-H., Hwang, I., Yang, Y.-B., et al. (2011). Microbial synthesis of gold nanoparticles using the fungus Penicillium brevicompactum and their cytotoxic effects against mouse mayo blast cancer C 2 C 12 cells. Appl. Microbiol. Biotechnol. Adv. 92, 617–630. doi: 10.1007/s00253-011-3556-0
Mittal, A., and Chisti, Y. (2013). Synthesis of metallic nanoparticles using plant extracts. Biotechnol. Adv. 31, 346–356.
Moghaddam, A. B., Nazari, T., Badraghi, J., and Kazemzad, M. (2009). Synthesis of ZnO nanoparticles and electrodeposition of polypyrrole/ZnO nanocomposite film. Int. J. Electrochem. Sci. 4, 247–257.
Mohanpuria, P., Rana, N. K., and Yadav, S. K. (2008). Biosynthesis of nanoparticles: technological concepts and future applications. J. Nanopart. Res. 10, 507–517.
Mohd Yusof, H., Mohamad, R., Zaidan, U. H., and Rahman, A. (2019). Microbial synthesis of zinc oxide nanoparticles and their potential application as an antimicrobial agent and a feed supplement in animal industry: a review. J. Anim. Sci. Biotechnol. 10, 1–22. doi: 10.1186/s40104-019-0368-z
Morsi, M., Abdelrazek, E., Ramadan, R., Elashmawi, I., and Rajeh, A. (2022). Structural, optical, mechanical, and dielectric properties studies of carboxymethyl cellulose/polyacrylamide/lithium titanate nanocomposites films as an application in energy storage devices. Polymer Test. 114, 107705.
Mott, D., Galkowski, J., Wang, L., Luo, J., and Zhong, C.-J. (2007). Synthesis of size-controlled and shaped copper nanoparticles. Langmuir 23, 5740–5745.
Mukherjee, P., Ahmad, A., Mandal, D., Senapati, S., Sainkar, S. R., Khan, M. I., et al. (2001). Fungus-mediated synthesis of silver nanoparticles and their immobilization in the mycelial matrix: a novel biological approach to nanoparticle synthesis. Nano Lett. 1, 515–519.
Muñoz-García, J., Vázquez, L., Cuerno, R., Sánchez-García, J. A., Castro, M., and Gago, R. (2009). “Self-organized surface nanopatterning by ion beam sputtering,” in Toward Functional Nanomaterials, Ed. Z. M. Wang (Berlin: Springer), 323–398.
Naraginti, S., Kumari, P. L., Das, R. K., Sivakumar, A., Patil, S. H., and Andhalkar, V. V. (2016). Amelioration of excision wounds by topical application of green synthesized, formulated silver and gold nanoparticles in albino Wistar rats. Mater. Sci. Eng. C 62, 293–300. doi: 10.1016/j.msec.2016.01.069
Narayanan, K. B., and Sakthivel, N. (2010). Biological synthesis of metal nanoparticles by microbes. Adv. Colloid Interf. Sci. 156, 1–13.
Nayak, D., Ashe, S., Rauta, P. R., Kumari, M., and Nayak, B. (2016). Bark extract mediated green synthesis of silver nanoparticles: evaluation of antimicrobial activity and antiproliferative response against osteosarcoma. Mater. Sci. Eng. C 58, 44–52. doi: 10.1016/j.msec.2015.08.022
Ndolomingo, M. J., and Meijboom, R. (2016). Determination of the surface area and sizes of supported copper nanoparticles through organothiol adsorption—Chemisorption. Appl. Surf. Sci. 390, 224–235.
Newbury, D. E., and Ritchie, N. W. (2013). Is scanning electron microscopy/energy dispersive X-ray spectrometry (SEM/EDS) quantitative? Scanning 35, 141–168. doi: 10.1002/sca.21041
Nguyen, K. T., Menon, J. U., Jadeja, P. V., Tambe, P. P., Vu, K., and Yuan, B. (2013). Nanomaterials for photo-based diagnostic and therapeutic applications. Theranostics 3, 152–166.
Nowack, B., Krug, H. F., and Height, M. (2011). 120 Years of Nanosilver History: Implications for Policy Makers. Washington, DC: ACS Publications.
Önal, E. S., Yatkın, T., Aslanov, T., Ergüt, M., and Özer, A. (2019). Biosynthesis and characterization of iron nanoparticles for effective adsorption of Cr (VI). Int. J. Chem. Eng. 2019:2716423.
Ostermann, R., Cravillon, J., Weidmann, C., Wiebcke, M., and Smarsly, B. M. (2011). Metal–organic framework nanofibers via electrospinning. Chem. Commun. 47, 442–444.
Parashar, M., Shukla, V. K., and Singh, R. (2020). Metal oxides nanoparticles via sol–gel method: a review on synthesis, characterization and applications. J. Mater. Sci. 31, 3729–3749.
Park, C. Y., and Choi, B. (2019). Enhanced light extraction from bottom emission oleds by high refractive index nanoparticle scattering layer. Nanomaterials 9:1241. doi: 10.3390/nano9091241
Patil, M. P., and Kim, G.-D. (2018). Marine microorganisms for synthesis of metallic nanoparticles and their biomedical applications. Colloids Surf. B Biointerf. 172, 487–495.
Patil, M. P., Ngabire, D., Thi, H. H. P., Kim, M.-D., and Kim, G.-D. (2017). Eco-friendly synthesis of gold nanoparticles and evaluation of their cytotoxic activity on cancer cells. J. Clust. Sci. 28, 119–132.
Patil, N., Bhaskar, R., Vyavhare, V., Dhadge, R., Khaire, V., and Patil, Y. (2021). Overview on methods of synthesis of nanoparticles. Int. J. Curr. Pharm. Res. 13, 11–16.
Patois, E., Capelle, M., Palais, C., Gurny, R., and Arvinte, T. (2012). Evaluation of nanoparticle tracking analysis (NTA) in the characterization of therapeutic antibodies and seasonal influenza vaccines: pros and cons. J. Drug Deliv. Sci. Technol. 22, 427–433.
Paul, B., Bhuyan, B., Purkayastha, D. D., and Dhar, S. S. (2016). Photocatalytic and antibacterial activities of gold and silver nanoparticles synthesized using biomass of Parkia roxburghii leaf. J. Photochem. Photobiol. B Biol. 154, 1–7. doi: 10.1016/j.jphotobiol.2015.11.004
Pérez-Lorenzo, M. (2012). Palladium nanoparticles as efficient catalysts for Suzuki cross-coupling reactions. J. Phys. Chem. Lett. 3, 167–174.
Pérez-Tijerina, E., Pinilla, M. G., Mejía-Rosales, S., Ortiz-Méndez, U., Torres, A., and José-Yacamán, M. (2008). Highly size-controlled synthesis of Au/Pd nanoparticles by inert-gas condensation. Faraday Discuss. 138, 353–362. doi: 10.1039/b705913m
Phull, A.-R., Abbas, Q., Ali, A., Raza, H., Zia, M., and Haq, I.-U. (2016). Antioxidant, cytotoxic and antimicrobial activities of green synthesized silver nanoparticles from crude extract of Bergenia ciliata. Fut. J. Pharm. Sci. 2, 31–36.
Pimpin, A., and Srituravanich, W. (2012). Review on micro-and nanolithography techniques and their applications. Eng. J. 16, 37–56.
Pradeep, T. (2009). Noble metal nanoparticles for water purification: a critical review. Thin Solid Films 517, 6441–6478.
Praseptiangga, D., Zahara, H. L., Widjanarko, P. I., Joni, I. M., and Panatarani, C. (2020). Preparation and FTIR spectroscopic studies of SiO2-ZnO nanoparticles suspension for the development of carrageenan-based bio-nanocomposite film. 100005.
Pugazhendhi, S., Sathya, P., Palanisamy, P., and Gopalakrishnan, R. (2016). Synthesis of silver nanoparticles through green approach using Dioscorea alata and their characterization on antibacterial activities and optical limiting behavior. J. Photochem. Photobiol. B Biol. 159, 155–160. doi: 10.1016/j.jphotobiol.2016.03.043
Qi, P., Zhang, D., and Wan, Y. (2013). Sulfate-reducing bacteria detection based on the photocatalytic property of microbial synthesized ZnS nanoparticles. Anal. Chim. Acta 800, 65–70. doi: 10.1016/j.aca.2013.09.015
Rad, A. G., Abbasi, H., and Afzali, M. H. (2011). Gold nanoparticles: synthesising, characterizing and reviewing novel application in recent years. Phys. Proc. 22, 203–208.
Rahmati-Abkenar, M., and Manteghian, M. (2020). Effect of silver nanoparticles on the solubility of methane and ethane in water. J. Nat. Gas Sci. Eng. 82:103505.
Rajeshkumar, S., Ponnanikajamideen, M., Malarkodi, C., Malini, M., and Annadurai, G. (2014). Microbe-mediated synthesis of antimicrobial semiconductor nanoparticles by marine bacteria. J. Nanostruct. Chem. 4, 1–7.
Rajkuberan, C., Prabukumar, S., Sathishkumar, G., Wilson, A., Ravindran, K., and Sivaramakrishnan, S. (2017). Facile synthesis of silver nanoparticles using Euphorbia antiquorum L. latex extract and evaluation of their biomedical perspectives as anticancer agents. J. Saudi Chem. Soc. 21, 911–919.
Ramesh, P., Kokila, T., and Geetha, D. (2015). Plant mediated green synthesis and antibacterial activity of silver nanoparticles using Emblica officinalis fruit extract. Spectrochim. Acta Part A 142, 339–343. doi: 10.1016/j.saa.2015.01.062
Rangel-Olivares, F. R., Arce-Estrada, E. M., and Cabrera-Sierra, R. (2021). Synthesis and characterization of polyaniline-based polymer nanocomposites as anti-corrosion coatings. Coatings 11:653.
Rao, N. H., Lakshmidevi, N., Pammi, S., Kollu, P., Ganapaty, S., and Lakshmi, P. (2016). Green synthesis of silver nanoparticles using methanolic root extracts of Diospyros paniculata and their antimicrobial activities. Mater. Sci. Eng. C 62, 553–557. doi: 10.1016/j.msec.2016.01.072
Rassaei, L., Marken, F., Sillanpää, M., Amiri, M., Cirtiu, C. M., and Sillanpää, M. (2011). Nanoparticles in electrochemical sensors for environmental monitoring. TrAC Trends Anal. Chem. 30, 1704–1715.
Rocha, F. S., Gomes, A. J., Lunardi, C. N., Kaliaguine, S., and Patience, G. S. (2018). Experimental methods in chemical engineering: Ultraviolet visible spectroscopy—UV-Vis. Can. J. Chem. Eng. 96, 2512–2517.
Saini, P., Saha, S. K., Roy, P., Chowdhury, P., and Babu, S. P. S. (2016). Evidence of reactive oxygen species (ROS) mediated apoptosis in Setaria cervi induced by green silver nanoparticles from Acacia auriculiformis at a very low dose. Exp. Parasitol. 160, 39–48. doi: 10.1016/j.exppara.2015.11.004
Saldarriaga, J. F., Aguado, R., Pablos, A., Amutio, M., Olazar, M., and Bilbao, J. (2015). Fast characterization of biomass fuels by thermogravimetric analysis (TGA). Fuel 140, 744–751.
Salem, S. S., and Fouda, A. (2021). Green synthesis of metallic nanoparticles and their prospective biotechnological applications: an overview. Biol. Trace Element Res. 199, 344–370. doi: 10.1007/s12011-020-02138-3
Salopek, B., Krasic, D., and Filipovic, S. (1992). Measurement and application of zeta-potential. Rudarsko-Geolosko-Naftni Zbornik 4:147.
Saw, M. J., Ghosh, B., Nguyen, M. T., Jirasattayaporn, K., Kheawhom, S., Shirahata, N., et al. (2019). High aspect ratio and post-processing free silver nanowires as top electrodes for inverted-structured photodiodes. ACS Omega 4, 13303–13308. doi: 10.1021/acsomega.9b01479
Schröfel, A., Kratošová, G., Šafar̄ík, I., Šafar̄íková, M., Raška, I., and Shor, L. M. (2014). Applications of biosynthesized metallic nanoparticles–a review. Acta Biomater. 10, 4023–4042.
Shenashen, M. A., El-Safty, S. A., and Elshehy, E. A. (2014). Synthesis, morphological control, and properties of silver nanoparticles in potential applications. Part. Part. Syst. Char. 31, 293–316.
Shi, Z., Lian, Y., Liao, F. H., Zhou, X., Gu, Z., Zhang, Y., et al. (2000). Large scale synthesis of single-wall carbon nanotubes by arc-discharge method. J. Phys. Chem. Solids 61, 1031–1036. doi: 10.1166/jnn.2001.012
Siddiqi, K. S., and Husen, A. (2016). Green synthesis, characterization and uses of palladium/platinum nanoparticles. Nanosc. Res. Lett. 11, 1–13. doi: 10.1186/s11671-016-1695-z
Siddique, S., and Chow, J. C. (2020). Gold nanoparticles for drug delivery and cancer therapy. Appl. Sci. 10:3824.
Sigmund, W., Yuh, J., Park, H., Maneeratana, V., Pyrgiotakis, G., and Daga, A. (2006). Processing and structure relationships in electrospinning of ceramic fiber systems. J. Am. Ceramic Soc. 89, 395–407.
Singh, R. P., Shukla, V. K., Yadav, R. S., Sharma, P. K., Singh, P. K., and Pandey, A. C. (2011). Biological approach of zinc oxide nanoparticles formation and its characterization. Adv. Mater. Lett. 2, 313–317.
Siwach, O. P., and Sen, P. (2008). Synthesis and study of fluorescence properties of Cu nanoparticles. J. Nanopart. Res. 10, 107–114.
Slavin, Y. N., Asnis, J., Häfeli, U. O., and Bach, H. (2017). Metal nanoparticles: understanding the mechanisms behind antibacterial activity. J. Nanobiotechnol. 15, 1–20.
Song, U., and Kim, J. (2020). Zinc oxide nanoparticles: a potential micronutrient fertilizer for horticultural crops with little toxicity. Horticult. Environ. Biotechnol. 61, 625–631.
Sowani, H., Mohite, P., Munot, H., Shouche, Y., Bapat, T., Kumar, A. R., et al. (2016). Green synthesis of gold and silver nanoparticles by an actinomycete Gordonia amicalis HS-11: mechanistic aspects and biological application. Process Biochem. 51, 374–383.
Sriram, M. I., Kalishwaralal, K., Barathmanikanth, S., and Gurunathani, S. (2012). Size-based cytotoxicity of silver nanoparticles in bovine retinal endothelial cells. Nanosci. Methods 1, 56–77.
Stepanov, A. L., Nuzhdin, V. I., Valeev, V. F., and Kreibig, U. (2011). “Optical properties of metal nanoparticles,” in Proceedings of the ICONO 2010: International Conference on Coherent and Nonlinear Optics, (Bellingham, WA: SPIE), 543–552.
Su, S. S., and Chang, I. (2018). “Review of production routes of nanomaterials,” in Commercialization of nanotechnologies–a case study approach, eds D. Brabazon, E. Pellicer, F. Zivic, J. Sort, M. D. Baró, N. Grujovic, K.-L. Choy (Berlin: Springer), 15–29.
Sugihartono, I., Dianisya, D., and Isnaeni, I. (2018). “Crystal structure analyses of ZnO nanoparticles growth by simple wet chemical method,” in Proceedings of the IOP Conference Series: Materials Science and Engineering, (Bristol: IOP Publishing), 012077.
Sun, T., Zhang, Y. S., Pang, B., Hyun, D. C., Yang, M., and Xia, Y. J. A. C. I. E. (2014). Engineered nanoparticles for drug delivery in cancer therapy. Angew. Chem. Int. Ed. Engl. 53, 12320–12364.
Tavakoli, A. H., Maram, P. S., Widgeon, S. J., Rufner, J., Van Benthem, K., Ushakov, S., et al. (2013). Amorphous alumina nanoparticles: structure, surface energy, and thermodynamic phase stability. J. Phys. Chem. C 117, 17123–17130. doi: 10.1021/jp405820g
Theron, J., Eugene Cloete, T., and De Kwaadsteniet, M. (2010). Current molecular and emerging nanobiotechnology approaches for the detection of microbial pathogens. Crit. Rev. Microbiol. 36, 318–339. doi: 10.3109/1040841X.2010.489892
Thomas, R., Janardhanan, A., Varghese, R. T., Soniya, E., Mathew, J., and Radhakrishnan, E. (2014). Antibacterial properties of silver nanoparticles synthesized by marine Ochrobactrum sp. Braz. J. Microbiol. 45, 1221–1227. doi: 10.1590/s1517-83822014000400012
Titus, D., Samuel, E. J. J., and Roopan, S. M. (2019). “Nanoparticle characterization techniques,” in Green synthesis, characterization and applications of nanoparticles, eds A. Shukla and S. Iravani (Amsterdam: Elsevier), 303–319. doi: 10.1016/B978-0-08-102579-6.00012-5
Tran, V., and Wen, X. (2014). “Rapid prototyping technologies for tissue regeneration,” in Rapid prototyping of biomaterials, Ed. R. Narayan (Sawston: Woodhead Publishing), 97–155. doi: 10.1533/9780857097217.97
Triana, M. A., Hsiang, E.-L., Zhang, C., Dong, Y., and Wu, S.-T. (2022). Luminescent nanomaterials for energy-efficient display and healthcare. ACS Energy Lett. 7, 1001–1020.
Uzair, B., Liaqat, A., Iqbal, H., Menaa, B., Razzaq, A., Thiripuranathar, G., et al. (2020). Green and cost-effective synthesis of metallic nanoparticles by algae: Safe methods for translational medicine. Bioengineering 7:129. doi: 10.3390/bioengineering7040129
Van Thai, P., Abe, S., Kosugi, K., Saito, N., Takahashi, K., Sasaki, T., et al. (2019). Size/shape control of gold nanoparticles synthesized by alternating current glow discharge over liquid: The role of pH. Mater. Res. Expr. 6:095074.
Velusamy, P., Das, J., Pachaiappan, R., Vaseeharan, B., and Pandian, K. (2015). Greener approach for synthesis of antibacterial silver nanoparticles using aqueous solution of neem gum (Azadirachta indica L.). Indus. Crops Products 66, 103–109.
Wang, P., Menzies, N. W., Lombi, E., Sekine, R., Blamey, F. P. C., Hernandez-Soriano, M. C., et al. (2015). Silver sulfide nanoparticles (Ag2S-NPs) are taken up by plants and are phytotoxic. Nanotoxicology 9, 1041–1049. doi: 10.3109/17435390.2014.999139
Wang, Z., Li, H., Tang, F., Ma, J., and Zhou, X. (2018). A facile approach for the preparation of nano-size zinc oxide in water/glycerol with extremely concentrated zinc sources. Nanosc. Res. Lett. 13, 1–9. doi: 10.1186/s11671-018-2616-0
Wiesendanger, R., and Güntherodt, H.-J. (2013). Scanning tunneling microscopy III: theory of STM and related scanning probe methods. Berlin: Springer Science & Business Media.
Xia, Y., Xiao, Z., Dou, X., Huang, H., Lu, X., Yan, R., et al. (2013). Green and facile fabrication of hollow porous MnO/C microspheres from microalgaes for lithium-ion batteries. ACS Nano 7, 7083–7092. doi: 10.1021/nn4023894
Yadav, R., Dwivedi, S., Kumar, S., and Chaudhury, A. (2010). Trends and perspectives of biosensors for food and environmental virology. Food Environ. Virol. 2, 53–63.
Yadav, T. P., Yadav, R. M., and Singh, D. P. (2012). Mechanical milling: a top down approach for the synthesis of nanomaterials and nanocomposites. Nanosci. Nanotechnol. 2, 22–48.
Yang, W., Wang, L., Mettenbrink, E. M., Deangelis, P. L., and Wilhelm, S. (2021). Nanoparticle toxicology. Annu. Rev. Pharmacol. Toxicol. 61, 269–289.
Ye, Q., Chen, W., Huang, H., Tang, Y., Wang, W., Meng, F., et al. (2020). Iron and zinc ions, potent weapons against multidrug-resistant bacteria. Appl. Microbiol. Biotechnol. 104, 5213–5227. doi: 10.1007/s00253-020-10600-4
Yuan, P., Ding, X., Yang, Y. Y., and Xu, Q. H. (2018). Metal nanoparticles for diagnosis and therapy of bacterial infection. Adv. Healthc. Mater. 7:1701392.
Zahra, Z., Habib, Z., Chung, S., and Badshah, M. A. (2020). Exposure route of TiO2 NPs from industrial applications to wastewater treatment and their impacts on the agro-environment. Nanomaterials 10:1469. doi: 10.3390/nano10081469
Zan, G., and Wu, Q. (2016). Biomimetic and bioinspired synthesis of nanomaterials/nanostructures. Adv. Mater. 28, 2099–2147.
Zhang, X., Yan, S., Tyagi, R., and Surampalli, R. (2011). Synthesis of nanoparticles by microorganisms and their application in enhancing microbiological reaction rates. Chemosphere 82, 489–494.
Zheng, Z., Zhang, X., Carbo, D., Clark, C., Nathan, C.-A., and Lvov, Y. (2010). Sonication-assisted synthesis of polyelectrolyte-coated curcumin nanoparticles. Langmuir 26, 7679–7681. doi: 10.1021/la101246a
Zhou, C., Wang, Y., Du, L., Yao, H., Wang, J., and Luo, G. (2016). Precipitation preparation of high surface area and porous nanosized ZnO by continuous gas-based impinging streams in unconfined space. Indus. Eng. Chem. Res. 55, 11943–11949.
Zhou, M., Wei, Z., Qiao, H., Zhu, L., Yang, H., and Xia, T. (2009). Particle size and pore structure characterization of silver nanoparticles prepared by confined arc plasma. J. Nanomater. 2009:968058.
Zhuang, J., and Gentry, R. W. (2011). “Environmental application and risks of nanotechnology: a balanced view,” in Biotechnology and Nanotechnology Risk Assessment: Minding and Managing the Potential Threats around Us, eds S. Ripp and T. Henry (Washington, DC: ACS Publications), 41–67. doi: 10.3390/ijerph16234848
Keywords: green synthesis, nanoparticles, nanotechnology, biological synthesis, microbial nanotechnology, bionanotechnology
Citation: Altammar KA (2023) A review on nanoparticles: characteristics, synthesis, applications, and challenges. Front. Microbiol. 14:1155622. doi: 10.3389/fmicb.2023.1155622
Received: 31 January 2023; Accepted: 21 March 2023;
Published: 17 April 2023.
Edited by:
Madan L. Verma, Indian Institute of Information Technology, IndiaReviewed by:
Maheshkumar Prakash Patil, Pukyong National University, Republic of KoreaSri Ramkumar Vijayan, Technical University of Denmark, Denmark
Copyright © 2023 Altammar. This is an open-access article distributed under the terms of the Creative Commons Attribution License (CC BY). The use, distribution or reproduction in other forums is permitted, provided the original author(s) and the copyright owner(s) are credited and that the original publication in this journal is cited, in accordance with accepted academic practice. No use, distribution or reproduction is permitted which does not comply with these terms.
*Correspondence: Khadijah A. Altammar, a2hhZGlqYWhhYUB1aGIuZWR1LnNh; orcid.org/0000-0002-8691-086X