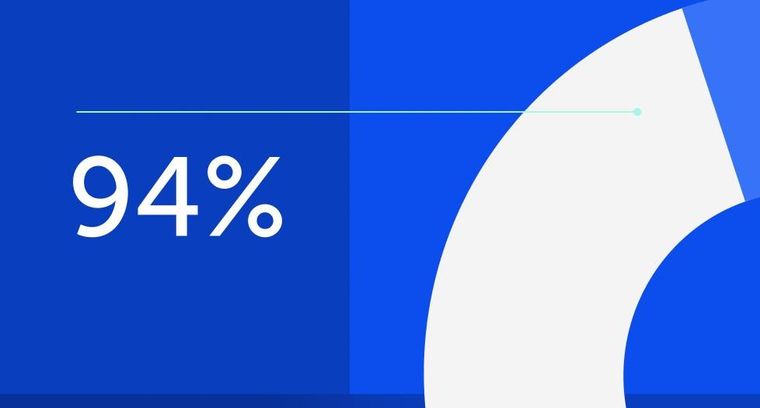
94% of researchers rate our articles as excellent or good
Learn more about the work of our research integrity team to safeguard the quality of each article we publish.
Find out more
HYPOTHESIS AND THEORY article
Front. Microbiol., 15 May 2023
Sec. Extreme Microbiology
Volume 14 - 2023 | https://doi.org/10.3389/fmicb.2023.1145915
This article is part of the Research Topic55th Anniversary of Ivan Barnes: Microbial Communities of Serpentinite-Hosted EcosystemsView all 10 articles
The demonstration by Ivan Barnes et al. that the serpentinization of fresh Alpine-type ultramafic rocks results in the exhalation of hot alkaline fluids is foundational to the submarine alkaline vent theory (AVT) for life’s emergence to its ‘improbable’ thermodynamic state. In AVT, such alkaline fluids ≤ 150°C, bearing H2 > CH4 > HS−—generated and driven convectively by a serpentinizing exothermic mega-engine operating in the ultramafic crust—exhale into the iron-rich, CO2> > > NO3−-bearing Hadean ocean to result in hydrothermal precipitate mounds comprising macromolecular ferroferric-carbonate oxyhydroxide and minor sulfide. As the nanocrystalline minerals fougerite/green rust and mackinawite (FeS), they compose the spontaneously precipitated inorganic membranes that keep the highly contrasting solutions apart, thereby maintaining redox and pH disequilibria. They do so in the form of fine chimneys and chemical gardens. The same disequilibria drive the reduction of CO2 to HCOO− or CO, and the oxidation of CH4 to a methyl group—the two products reacting to form acetate in a sequence antedating the ‘energy-producing’ acetyl coenzyme-A pathway. Fougerite is a 2D-layered mineral in which the hydrous interlayers themselves harbor 2D solutions, in effect constricted to ~ 1D by preferentially directed electron hopping/tunneling, and proton Gröthuss ‘bucket-brigading’ when subject to charge. As a redox-driven nanoengine or peristaltic pump, fougerite forces the ordered reduction of nitrate to ammonium, the amination of pyruvate and oxalate to alanine and glycine, and their condensation to short peptides. In turn, these peptides have the flexibility to sequester the founding inorganic iron oxyhydroxide, sulfide, and pyrophosphate clusters, to produce metal- and phosphate-dosed organic films and cells. As the feed to the hydrothermal mound fails, the only equivalent sustenance on offer to the first autotrophs is the still mildly serpentinizing upper crust beneath. While the conditions here are very much less bountiful, they do offer the similar feed and disequilibria the survivors are accustomed to. Sometime during this transition, a replicating non-ribosomal guidance system is discovered to provide the rules to take on the incrementally changing surroundings. The details of how these replicating apparatuses emerged are the hard problem, but by doing so the progenote archaea and bacteria could begin to colonize what would become the deep biosphere. Indeed, that the anaerobic nitrate-respiring methanotrophic archaea and the deep-branching Acetothermia presently comprise a portion of that microbiome occupying serpentinizing rocks offers circumstantial support for this notion. However, the inescapable, if jarring conclusion is drawn that, absent fougerite/green rust, there would be no structured channelway to life.
The three founding facts underpinning the submarine “alkaline vent theory” for the emergence of life are:
1. Barnes and O’Neil’s (1969) conclusion that: “convecting seawater at < 200°C would have serpentinized the crust, becoming alkaline by this process of hydrolysis, much as today, hot springs involving ground-water circulating in ophiolite, have a pH of between 11.5 and 12″;
2. Dudley Foster’s iconic photograph of the acidic ~ 380°C Black Smoker hydrothermal chimney with polychaete and tube worms on the East Pacific Rise (Ballard and Grassle, 1979; Spiess et al., 1980);
3. The smaller scale hydrothermal chimneys, chemical garden spires, and microbialites discovered in the ~ 340 million year old ore deposits in Ireland, inspired by the “Black Smoker” reports (Larter et al., 1981; Russell, 1996).
Jack Corliss et al. had calculated, on the basis of geochemical studies of basalts from the Mid-Atlantic Ridge and from the silica and magnesium chemistry of warm springs exhaling from the Galapagos submarine ridge, that 300°C metal-bearing hot springs should be found at ocean floor spreading centers (Corliss, 1971; Corliss et al., 1979). The discovery of acidic Black Smokers teaming with life met these predictions and led Corliss, John Baross and Sarah Hoffman to formulate a “hydrothermal origin-of-life hypothesis.” Rejected from Nature and Science, they resorted to the “grey literature” to present their manuscript (Corliss et al., 1980, 1981; Baross and Hoffman, 1985; Levitt, 2023). As reported in Corliss et al. (1980), the hypothesis maintains that “Submarine hydrothermal systems provide all of the conditions necessary for the abiotic synthesis of organic compounds, polymers, and simple cell-like organisms. The continuous flow of circulating fluids in a hydrothermal system provides the thermal and chemical gradients which create the variation in conditions necessary for the successive reactions to take place. Other models for the origin of life fail to fulfill one or more of these requirements.”
This “anaerobic chemoautotrophic” hypothesis riled those who had accultured to the Oparin–Haldane–Urey–Miller dogma of how life originated (Lahav, 1985; cf., Lane et al., 2010). Indeed, Stanley Miller himself, with his colleague Jeffrey Bada, took to print in 1988, opining “The high temperatures in the vents would not allow synthesis of organic compounds, but would decompose them, unless the exposure time at vent temperatures was short… Even if the essential organic molecules were available in the hot hydrothermal waters, the subsequent steps of polymerization and the conversion of these polymers into the first organisms would not occur as the vent waters were quenched to the colder temperatures of the primitive oceans” (Miller and Bada, 1988). Their criticism prompted a response, in which it was argued from the discovery of fossil chimneys at Silvermines and the Tynagh base-metal ore deposit in Ireland (Larter et al., 1981; Boyce et al., 1983; Banks, 1985) that “similar, less extreme environments are known and could have provided suitable sources of chemical energy and nutrients as well as stable ‘culture chambers’” (Russell et al., 1988).
However, a reading of Ivan Barne’s studies and our field studies on Alpine-type ultramafic rocks in Southern Europe and Turkey (e.g., Fallick et al., 1991) led us to propose a substitution of the acidic hydrothermal spring models responsible for Black Smokers and exhalative orebodies, with a serpentinization-driven alkaline hydrothermal model that more appropriately explained the sources of fuel to feed life’s ‘origin’ (Russell et al., 1989). Further development of the model had the alkaline hydrothermal solutions precipitating iron sulfide bubbles on contact with “the mildly oxidized, acidic and iron-bearing Hadean ocean water” (Russell et al., 1993). While this model could demonstrate the analogy with CO2-based autotrophic metabolism—more fundamental was its explanation of the otherwise enigmatic origin of Peter Mitchell’s proton motive force (PMF). How life could have invented the PMF had been a puzzle, so a key insight of AVT was that no invention of the ‘force’ was necessary—the PMF had been there from the beginning, freely developed from one aspect of the initial conditions, i.e., as a proton gradient imposed across mineral precipitate membranes—itself a result of the acidulous ocean interfacing the alkaline hydrothermal fluid as it exhales from the Hadean ocean floor (Mitchell, 1961; Russell et al., 1994; Figure 1).
Figure 1. Initial conditions responsible for the emergence of life (EoL) according to the alkaline vent theory (AVT; Russell et al., 2013). Mantle-derived volcanic emanations (> 700°C) and high temperature acidic hot springs (~410°C) inject CO2, P4O10, some N2O, and the transition elements directly through the Hadean ocean floor into the cool carbonic Hadean ocean (Yamagata et al., 1991; Javoy and Pineau, 1991; Macleod et al., 1994; Kasting and Siefert 2001; Wong et al., 2017; Mandon et al., 2019; Ueda and Shibuya, 2021; Brady et al., 2022; Buessecker et al., 2022; Heays et al., 2022; Nishizawa, 2022; Tatzel et al., 2022). At the ocean bottom, and diffusing laterally and upward, these volatiles and ions remain as saturated or supersaturated until meeting with alkaline hydrothermal solutions (at ≤ 130°C) produced by the serpentinization engine (Barnes and O’Neil, 1969; Russell et al. 1989; Branscomb and Russell, 2018; Shibuya and Takai., 2022). Fougerite, along with subordinate amorphous silica, greenalite, and subsidiary mackinawite spontaneously precipitate at the interface between the alkaline solution and the ocean solvent (Russell, 2018; Tosca et al., 2016, 2019; Borrego-Sánchez et al., 2022). These inorganic barriers maintain the pH and redox disequilibria that drive the emergence of life (EoL; Russell and Hall, 1997), so focusing the electrochemical disequilibria as native electrons, cations, anions, and dissolved gasses across the fougerite exteriors of the mound. Thus, these nanocrysts, assisted by the electron-conducting mackinawite, are forced into acting as nanoengines to resolve the disequilibria and thereby bring embryonic life into being (Arrhenius, 2003; Mielke et al., 2010; Nitschke and Russell, 2012, 2013; Barge et al., 2015a; Halevy et al. 2017; Russell and Nitschke, 2017; Wong et al., 2017; Yamamoto et al., 2017, 2022; Kitadai et al., 2018; Duval et al. 2019; Ooka et al., 2019; Hudson et al., 2020; Nitschke et al., 2022; Buessecker et al. 2022). [NB., The electron acceptors and the H+ shown to be dissolved in the ocean are constantly delivered by the venturi effect to the outer barrier of the mound (Russell and Hall, 1997)]. Redrawn from Branscomb et al. (2017). An extensive aureole of the same minerals surround this and other vents, now altered to banded iron formation (BIF; Konhauser et al., 2007; Pons et al., 2011; Mloszewska et al., 2012). Not to scale. The serpentinite photograph was generously provided by Laura Barge.
Competing with AVT, though assuming only acidic conditions, is Günter Wächtershäuser’s theory that the origin of life involves the reduction of copious volcanic CO2 through the reductive acetyl coenzyme-A pathway to acetate (Wächtershäuser, 1988)—the pathway that is now broadly, but not universally, accepted for this role (Huber and Wächtershäuser, 1997; Peretó et al., 1999; Martin and Russell, 2003, 2007; Say and Fuchs, 2010; Martin, 2020; Boyd et al., 2023). That life was first engaged in the reduction of oceanic CO2 through the acetyl coenzyme-A pathway was incorporated in AVT and expressed in the (grossly) oversimplified empirical Equation 1 (Russell and Martin, 2004)
But more recently, we have argued for an alternative whereby the disequilibria across a ferrous–ferric oxyhydroxide membrane—with FeS now as a subordinate—are several and the potentials significantly greater, namely, the ‘denitrifying methanotrophic acetogenic pathway’ (DnMAP; Ducluzeau et al., 2009; Nitschke and Russell, 2013). This suggestion arises: (1) in consideration of methane emanations accompanying H2 in some serpentinizing systems—an overlooked otherwise ‘wasted’ fuel and source of organic carbon (Kelley, 1996); (2) the ‘energetic’ requirement for an electron acceptor with a higher potential than CO2 to order protometabolism (Russell and Hall, 1997; Nitschke and Russell, 2013); (3) in choosing the methanotrophic route, the steep uphill ‘free’ energy climb to the highly unstable formyl intermediate in the classic acetyl coenzyme-A pathway is avoided (Maden, 2000; Stojanowic and Hedderich, 2004); (4) to provide a source of ammonium, which otherwise is lacking (Nitschke and Russell, 2013); (5) and the ungainliness of the classic pathway’s two ‘legs’, i.e., the disproportionate numbers of the reductive steps involved, one to reduce CO2 to CO or formate, as against six to reduce it to a reactive methyl sulfide entity, is replaced by the more symmetrical, less complicated ‘CO2-reducing and denitrifying methanotrophic pathways’ that converge to acetyl coenzyme-A (DNitAP; Nitschke and Russell, 2013). This pathway is also highly simplified to
Apart from the addition of methane, this alternative takes into account the disequilibria focused at a submarine alkaline vent as outlined in Russell and Hall (1997): the natural proton motive force and a ‘respiratory’ redox mechanism with electrons (some of them bifurcated) that H2, via 2H•, provides, processing through a green rust (fougerite) nanoengine as electrons are conducted to high potential electron acceptors, e.g., nitrate (Nitschke and Russell, 2012, 2013; Barge et al., 2015a; Russell and Nitschke, 2017; Wong et al., 2017; Buessecker et al. 2022; Nitschke et al., 2022). We reiterate that this reductive mechanism also accounts for the required on-site source of ammonium for amino and nucleic acid synthesis, otherwise far from obvious (Barge et al., 2019).
Yet, a further change to AVT was the adoption of the term ‘emergence’, as we came to understand that the conventional ‘origin of life’ was an idiom devoid of evolutionary connotation (Russell et al., 1993)—this from the readings of Prigogine and Stengers’ (1984) book “Order out of Chaos” and Wicken’s (1987) volume “Evolution, Thermodynamics and Information.” Indeed, contemplating life’s ‘emergence’ forced a more serious consideration of the role of serpentinization in life’s onset (Russell et al., 2013).
In the present theoretical contribution, life’s emergence is traced from serpentinization to its fledging as a dynamic system that dramatically reduces entropy (thus substantially increasing the rate at which the driving disequilibria produce entropy). We conclude that the first proto-metabolic steps take place in the natural chemical garden spires that developed at a submarine alkaline hydrothermal vent sometime in the ~ 500 million year span of the Hadean era. Exothermic serpentinization is the mega-engine operating within the ultramafic oceanic crust that works to drive alkaline hydrothermal convective systems bearing H2 > CH4 fuels to exhale into the then carbonic, phosphate, nitrate, NO, and N2O as well as metal complex-, and proton-bearing acidulous ocean—the disequilibria resulting in autotrophic metabolisms involving quinone-dependent NO reductase and membrane-bound N2O reductase—supporting a primitive aerobic respiration (Ducluzeau et al., 2009, 2014; Nitschke and Russell, 2013; Russell et al., 2013; Brady et al., 2022; Buessecker et al., 2022; Farr et al., 2022).
This is where the structural and ionic complexity of the 2D green rust mineral fougerite (~Fe2+4Fe3+2(OH)12CO3.3H2O) moves to center stage in AVT. Initially precipitated as white rust (amakinite) under alkaline conditions, the oxidation by water to fougerite—with the concomitant evolution of H2—is rapid (Trolard et al., 2022; Helmbrecht et al., 2022). Though more ordered, i.e., of lower entropy than those of the contributing solutes, amakinite/fougerite precipitation is entropy-driven (Van Santen, 1984). The structure of fougerite (formerly green rust) is complex but not “pre-designed,” i.e., its growth is not algorithmic, it is merely self-ordered and requires no prescription (cf. Arrhenius, 2003; Abel and Trevors, 2006). It is the only macromolecular entity known to us with the chemical and physical flexibility and potential to respond to disequilibria at the vent. Thus, we propose that it has the wherewithal to act as the nanoengine to impel life into being. The iron sulfides mackinawite (FeS) and greigite (Fe3S4)—as subordinate components of the hydrothermal chimneys and spires—still hold vital support roles in their ‘free energy’ converting capacities and as conductors and semiconductors in AVT (Nitschke and Russell, 2009; Vasiliadou et al., 2019; Hudson et al., 2020).
The casting of exothermic serpentinization of ocean crust as a disequilibria- (“free energy”-) converting mega-engine (Russell et al., 2013) is based on an extensive literature (Barnes and O’Neil, 1969; Barnes et al., 1978; Neal and Stanger, 1983, 1984; Fallick et al., 1991; Kelley et al., 2001; Lowell and Rona, 2002; Russell and Arndt, 2005; Mielke et al., 2010; Paukert et al., 2012; Russell et al., 2013; Branscomb and Russell, 2018). Inspiration was rooted in the physics of materials, as considered by Cottrell (1979), whereby mechanical stress is converted through feedbacks, as in an engine, into physical and chemical disequilibria such as to result in “living things,” themselves engines. How might we see such engines developing in the early Earth?
With Hadean days so short, the moon so close, and the Earth’s mantle so soft, the mafic to ultramafic oceanic crust suffers pulses of incessant cracking, jointing, faulting, and brecciation that allow the invasion and gravitation of cool ocean water to depth (Miller et al., 2016; Heller et al., 2021; Tatzel et al., 2022). Once cracks form in a tensile stress regime near the surface, feedback ensures that smaller stresses are required to keep them ratcheting down through the crust as exothermic cracking engines (Cottrell, 1979; Lowell and Rona, 2002). Furthermore, the hydrostatic pressure so imposed increases the effective stress, though only after the crack has propagated at the nanoscale, feeding back to further cracking while the elasticity at the tip is converted to ‘free energy’—a counter-intuitive realization (Cottrell, 1979). Such an autocatalytic feedback is further augmented by the hydrostatic pressure imposed on the mafic to ultramafic wallrock by the ocean waters gravitating to depth—a pressure that increases both the effective tensile and related sheer stresses (Cathles, 1990; Russell and Skauli, 1991). These couplings eventuate in the cracks reaching brittle-to-ductile transition zones in ultramafic rocks estimated from the hydrogen isotope work of Proskurowski et al. (2006) to bottom out at ~ 150°C, corresponding to an eventual maximum crustal depth of around 8–10 km (Macleod et al., 1994). This self-ordering and self-healing process continues until much of the upper crust is hydrated and carbonated, causing a lowering of density (≳ 2.6 g cm−3) compared to the antecedent unaltered ultramafic crust (3.3 g cm−3), through expansion allowed for by crustal extension and/or domal uplift in processes leading to further cracking (Fujioka et al., 2002; Pons et al., 2011).
The exhaust from the serpentinizing system—as heat and solutes—is discharged in convective hydrothermal alkaline updrafts buffered to a pH of 10–11 units guided by approximately vertical fractures in a process that lasts a minimum of 30,000 years (~ 1021 nanoseconds; Früh-Green et al., 2003). The tectonic, thermal, and chemical disequilibria are resolved through hydrothermal convection to result in a hydrothermal fluid—initially carbonic ocean water—by being reduced to H2 and short carboxylic acids and sparse methane (Früh-Green et al., 2003; Proskurowski et al., 2006; Ludwig et al., 2011; Tutolo et al. 2020; White et al., 2020; Albers et al., 2021; Figure 1; Equation. 3):
Tidal and seismic pumping are additional inputs to the workings of this complex engine (Sibson et al., 1975; Davis and Becker, 1999; Glasby and Kasahara, 2001).
Spasmodic charges of methane, as well as of formate and acetate, are also recorded, both by a direct analysis and in laboratory experiments (Shock, 1992; Windman et al., 2007; White et al., 2020). However, it may be that much of the methane is derived through leaching from that generated in the lower crust and entrained in the same solutions (Shock, 1992; White et al., 2020). This thermal and chemical waste from serpentinization is now transported to the ocean floor in a hydrothermal solution that finds itself well out of thermal and electrochemical equilibrium with its new host, the iron-rich carbonic ocean water from which it first derives (Figure 1). Furthermore, the immediate effect of the meeting of the two contrasting solutions is the spontaneous precipitation of iron oxyhydroxides accompanied by silica and minor iron sulfides (Barge et al., 2015a,b, 2020; Helmbrecht et al., 2022). A portion of the precipitates makes up the hydrothermal chimneys and spires, while entrained flocs escape from this, and other alkaline springs, to disperse and lithify to banded iron formations comparable to those in Isua in the early Archean of western Greenland and in the Hadean Nuvvuagittuq greenstone belt in Canada (Appel, 1980; Papineau et al., 2011; Pons et al., 2011; Mloszewska et al., 2012; Halevy et al., 2017; Tosca et al., 2019; Bindeman and O’Neil, 2022).
The membranes precipitated at the hydrothermal mound have the effect of frustrating the release of the pent-up disequilibria, until a weakness can be found to guide interaction of the contrasting fluids. In the case of serpentinization and convection—the mega-engines just described—this was via chance cracks in the crust. However, at the nanoscale, the dissipative system finds a way to partial relaxation by the forced exploitation of nanochannels prized from hydrous cleavage cracks constituting fougerite interlayers (Figure 2). Furthermore, the redox- and pH-active nanochannels within the interlayers might impose a vectorial two-way ordering—a primitive guidance system—along the reductive and oxidative synthetic steps of the denitrifying methanotrophic acetogenic pathway toward an incomplete reverse tricarboxylic acid cycle (TCA) (Hartman, 1975; Wander et al., 2007; Nitschke and Russell, 2013; cf., Gatenby and Frieden, 2017; Figure 2).
Figure 2. Fougerite modeled as a ready-made multifunctional motor enzyme/pump precursor set in the inorganic membrane, wherein it reduces nitrate drawn from the ocean (curved blue arrow to the left) to aminogen or ammonium, or nitrite to NO, N2O, and N2, vectored from ‘left’ to ‘right’ within the hydrate galleries (Hansen et al., 2001; Génin et al., 2005, 2006; Trolard et al., 2007, 2022; Trolard and Bourrié, 2012; Gerbois et al., 2014; Russell, 2018; Duval et al., 2019, 2020). At the same time and in theory, methane would be converted to a methyl group by NO (Kampschreur et al., 2011; Nitschke and Russell, 2013). Barge et al. (2019, 2020) show that in the same circumstances, pyruvate can be aminated to alanine and oxalate to glycine. Hydrazine is another speculative product (Duval et al., 2020). Note that an anion-binding pocket forms by the oxidation of the opposed iron molecules as they are confronted with nitrate which is thereby reduced (Nitschke and Russell, 2013). However, transmission (‘escape’) of a product to the interior is only permitted (ungated) when the nitrite is itself reduced to neutral NO and can be driven by the ionic gradients further into the interlayer. Note too that the flows (and counterflows) are vectorial, controlled by electron hopping rates (Wander et al., 2007). There is an expectation that short peptides will be produced within the interlayers (Muñoz-Santiburcio and Marx, 2017; Erastova et al., 2017; Grégoire et al., 2018; Holden et al. 2022) and partially extruded into the spire’s interior. Further H2 and organic molecules can be released to the hydrothermal flow by delamination and/or diagenetic alteration of fougerite to magnetite at depth in the mound (Asimakidou et al., 2020; Farr et al., 2022). The upward-directed arrows to the right signify the alkaline hydrothermal updraft, and the smaller arrows either side of the fougerite representing the inorganic membrane denote the direction of electron and proton flow. Not to scale.
Thus, a way is open, even at the nanoscale for a specialized dissipative (entropy-generating metabolizing) engine to materialize in obeyance to the Universe’s predilection to ‘produce’ ever more disorder in its blind bid to continue its relaxation, independent of scale, from its initial excruciating disequilibrium at the origin of space–time (Nitschke and Russell, 2010; Russell et al., 2013; Carroll, 2016).
The series of orderly convection engines governed by physical transitions in the body of our planet, brought to a head by the serpentinization cracking engine, ultimately results in a long-lasting flow of reduced alkaline fluids into a highly contrasting relatively oxidized and mildly acidic Hadean seawater. Furthermore, the interactions of two solutions at the spontaneously precipitated membrane provide just the electrochemical disequilibria required to drive entropy-reducing metabolic pathways and reproductive cycles (Hitchcock and Lovelock, 1967; Russell and Hall, 1997; Russell and Arndt, 2005; Nitschke et al., 2022). However to bring these factors into play, disequilibria-converting engines are again required. Yet, the building components for construction are necessarily restricted to any inorganic materials at hand. Of course, at every step, engines must locally disproportionate entropy in such a ratio as to ensure its decrease in the driving of anabolic metabolism by an overall larger increase in entropy. This is achieved by the transportation of uncooperative molecules as waste from the partially open protometabolizing system.
Glaringly obvious is the requirement for the coupled hydrolysis of adenosine triphosphate, ATP, in present-day life—and thereby the need for its synthesis (Whicher et al., 2018; Pinna et al., 2022). To achieve such biosynthesis, Mitchell (1961) showed a pH gradient—a proton motive force—to be capable of driving the condensation of adenosine diphosphate (ADP) and inorganic phosphate (Pi) to adenine triphosphate (ATP) via the complex enzyme ATP synthetase situated in life’s membranes. Thus, Mitchell apparently dispensed with the long-favored view that a ‘high energy’ intermediate molecule was responsible, calling his process ‘chemiosmosis’. However, Boyer (1997) first made mechanical sense of Mitchell’s finding, demonstrating that ATP synthetase is actually a rotating nanoengine involving ‘binding-change’ and gated escapement mechanisms driven by the proton gradient. In life, protons are delivered by the machinations of complex 1 and the like (Hedderich, 2004; Kaila, 2021). Boyer mapped out the stages the ATP synthetase rotatory enzyme took to complete the cycle, realizations now fundamental to an understanding of how life works and indicating how some, albeit simpler engine must have worked from the very beginning (Boyer, 1979, 1997; Astumian, 2012; Astumian et al., 2016; Anashkin, et al., 2021). For reasons of pedagogy, Yoshida et al. (2001) compare the ATPase to a Wankel engine, while Carter (2020) likens the binding change and reciprocally coupled gating mechanism to escapements in the workings of a mechanical clock.
Important though ATP is, clearly ATP synthetase itself is much too complicated to have been available at life’s onset. Indeed, the discovery by Baltscheffsky et al. (1966) that inorganic pyrophosphate (PPi), situated in the membrane, can act as ‘energy donor’ in an electron-transport phosphorylation system introduced the hypothesis that a proton pyrophosphatase (H+-PPase) was a precursor to the ATP (Russell et al. 1994; Baltscheffsky et al., 1999). These reversible vacuolar pyrophosphatases are stochastic nanopumps (Lin et al., 2012, Li et al., 2016), in which a highly restricted number of water molecules within the axial region have the effect of dampening the flow of protons or cations. The protons make their way one-by-one through the gating mechanism, either inward or outward, depending on the ambient disequilibria (Branscomb and Russell, 2013, 2019; Scholz-Starke et al., 2019; Astumian et al., 2020).
However, PPi has strong competitors as a phosphorylating agent, e.g., acetyl phosphate [AcP]. Acetyl phosphate itself is readily generated from thioacetate and disodium phosphate under alkaline conditions (Heinen and Lauwers 1996; Huber and Wächtershäuser, 1997; Whicher et al., 2018). AcP is especially attractive as a precursor candidate of ATP (Pinna et al., 2022). Furthermore, Whicher et al. (2018) demonstrate the phosphorylation of ribose to ribose-5-phosphate and the phosphorylation of ADP to ATP also under alkaline conditions—the only nucleoside diphosphate to be thus phosphorylated—so explaining the primacy of ATP in bioenergetics (Pinna et al., 2022). Given that Wang et al. (2019) produce PPi from AcP and Pi, but not from 2Pi across an Fe-rich membrane in a microfluidic rig, would leave AcP as, perhaps, the major phosphate player in early metabolism. However, there are still other compounds such as the linear oligophosphates, glycolaldehyde phosphate (Arrhenius et al., 1993; Pitsch et al., 1995; Krishnamurthy et al., 1999), and the cyclic trimetaphosphate (TMP) shown by Etaix and Orgel (1978) to be capable of phosphorylating nucleosides in water—a discovery transferred into the 2D interlayers of the double-layer oxyhydroxides (DLHs; Yamanaka, 1988; Kuma et al., 1989; Yamagata et al., 1997; Kolb et al., 1997). Kolb et al. (1997), using TMP, induce phosphorylation of the glycolate ion in the interlayers of DLHs to glycolophosphate and diphosphate at a rate which is independent of the external concentration of glycolate ion in the range of 1–100 mM as measured—a remarkable and highly significant finding as we shall see. The phosphorylation can be followed by measuring the height of the interlayer; the initially absorbed hydroxyl height measures 0.29 nm, the glycolate replacement is 0.49 nm, and the trimetaphosphate (TMP) on its own is 0.68 nm, while adding TMP to glycolate to produce glycolophosphate plus the diphosphate generates a height of only 0.64 nm (Adam and Delbrück, 1968; Kolb et al., 1997).
Distinct from, and additional to the pyrophosphatase argument, and following Wächtershäuser (1990) and Peretó et al. (1999), the direct reduction of carbon dioxide via the reductive acetyl coenzyme-A pathway was next considered, though in terms of an inorganic FeS membrane rather than through the pyrite reaction (Russell and Martin, 2004). Branscomb and Russell (2013) modeled such a reduction involving the hydrogenation of CO2 dissolved in, and sourced from, the most ancient ocean, combined with that ambient proton force in a membrane comprising iron monosulfide and fougerite. Several authors calculate that the proton motive force summing to 2 pH units or more (equivalent to ~ 120 + mV) will facilitate the reduction of CO2 in alkaline waters (Russell and Hall, 1997; Schoepp-Cothenet et al., 2013; Sojo et al., 2016). Since then, and following Vasiliadou et al. (2019), Reuben Hudson and his coworkers have tested the latter hypothesis which is of direct relevance to the AVT using a microfluidic technique involving an iron sulfide membrane, duly demonstrating the requirement for “proticity” in such a reduction (Hudson et al., 2020). Whether fougerite, lightly dosed with sulfide, could achieve a similar result awaits experimental testing. However, fougerite’s propensity to enforce redox reactions as well as its ability to interconvert redox and pH gradients is now well known (Hansen et al., 2001; Génin et al., 2005, 2006; Figure 2). Left hanging is fougerite’s possible role as a precellular inorganic non-ribosomal peptide synthetase (Bernhardt, 2019).
Our realization that prebiotic chemistry could not explain the extreme reduction of entropy involved in the emergence of life focused attention on the ferroferric DLH, green rust—now named by its discoverer, Fabienne Trolard, ‘fougerite’ (Trolard et al., 2007). Arrhenius (1984, 2003) and Arrhenius et al. (1997) were the first to see the benefits of considering green rust/fougerite (and comparable non-redox but positively charged DLHs) in this precellular context, owing to, (1) its premetamorphic abundance in Archaean banded iron formations (Arrhenius et al., 1997; Halevy et al., 2017), (2) its propensity to selectively absorb anions where, in the 2D interlayers, the effective concentrations are increased up to a million-fold (Delbrück, 1970; Arrhenius et al., 1993; Pitsch et al., 1995), (3) its “structures”; capable of dynamic agency while limiting degrees of freedom (Pitsch et al., 1995; Kolb et al., 1997), (4) its potential, sited within and as a membrane separating two strongly contrasting solutions, to respond to environmental perturbations for the sake of continued growth, cf., Mitchell, 1959), and (5) such arrangements might provide the governance required for the emergence of ordered reproduction (Popov, 1999; Arrhenius, 2003; Greenwell and Coveney, 2006; Galimov, 2014; Endres, 2017; Branscomb et al., 2017; Cartwright and Russell, 2019; Gribov et al., 2021). We might add the speculation, derived from other DLHs, that its variable pattern of cations could affect the configuration of any nucleic acids produced in the system, eventually resulting in a functional polymeric sequence of nucleic acids to govern the established metabolisms (Erastova et al., 2017; Grégoire et al., 2018).
That fougerite is conformationally flexible and responds reversibly and interactively to environmental pH and redox conditions as Génin et al. (2005, 2006), Ruby et al. (2010) demonstrate also supports the hypothesis that fougerite is the precursor to the ‘free energy’/disequilibria-converting enzymes involved in conformational cycling (Nitschke et al., 2022; Equation 4):
a view warranted in consideration of the remarkable experiments of Hansen et al. who demonstrate the power of fougerite to effect the ready reduction of nitrate to ammonia—a process involving the addition of eight separate electrons to the initial nitrate as fougerite is reduced to magnetite (Hansen et al., 1996; Génin et al. 2008; Russell, 2018; Asimakidou et al., 2020). Moreover using similar conditions, Gerbois et al. (2014) demonstrate the reduction of nitrite to NO, N2O, and N2. These capabilities demonstrate an enzyme-like agency of fougerite in out-of-equilibrium geochemical systems—engineering conversions not only comparable to the nitrate and nitrite reductases, but also comparable to the enzymes such as methane monooxygenase, aminotransferase (transaminase), and acetyl phosphatase, an inorganic phosphoesterase, and, perhaps, a non-ribosomal peptide synthetase (Russell, 2018; Barge et al. 2019; Bernhardt, 2019; Branscomb and Russell, 2019; Duval et al., 2019; Huang, 2019, 2022; Wang et al., 2019).
An accompanying nickel-bearing iron sulfide mineral, mackinawite, is also planar conducting and can act as a hydrogenase (H2 → 2ē), ferredoxin, carbon-monoxide dehydrogenase, and acetyl coenzyme-A synthase (Hudson et al., 2020). Together, these are the conversions required to make the first ordered steps to autogenic life fed by H2, CO2, CH4, HNO3−, HNO2−, NO, and PO43− with an ambient steep proton gradient (Russell and Martin, 2004; Schoepp-Cothenet et al., 2013). Experiments grounded in the submarine alkaline vent model for life’s emergence have largely demonstrated that these conversions—this “sucking of order” from the environment (Schrödinger, 1944)—had the capacity to get autotrophic (self-ordering, self-sufficient, self-sustaining, though not self-referencing) metabolism started (Russell et al., 2003; Nitschke et al., 2022).
Having some similar properties to enzymes, these minerals or their macromolecular precursors should give us a better understanding of biological phenomena (Smith, 1986; Branscomb and Russell, 2019). Indeed, the jarring conclusion is forced that only these two minerals together can execute most of the disequilibria conversions required by the first ordered steps to autogenic life fed by H2, CO2, CH4, HNO3−, HNO2−, NO, and PO43− with an ambient steep proton gradient (Barge et al. 2019; Hudson et al., 2020). In this view, the rates of synthesis would be governed independently of variations and fluctuations in chemical concentrations and pressure through the viscosity of water ‘trapped’ in the confined spaces of the interlayers, which consequentially severely restrict the degrees of freedom of the system (Kolb et al., 1997; Astumian, 2007; Muñoz-Santiburcio and Marx, 2017; Branscomb and Russell, 2019). Furthermore, although fougerite is a 2D mineral, motions within the interlayers would be generally restricted to 1D as the 0.56-nm iron-to-iron hopping rate to next-nearest neighbors is ~ 1010 s−1 at standard temperature and pressure (STP), 3 orders of magnitude faster than those of the other two symmetry-unique hops, thus imposing vectorial flow, as in modern cells, but through the ‘green rust’ interlayers (Wander et al. 2007). This electron tunneling activity would tend to pull the more laggardly protons in single file in the hydrous interlayers by the Grötthuss mechanism (Muñoz-Santiburcio and Marx, 2017).
Fougerite, acting as the first nanoengine driving emergent metabolism, has to be mounted and secured in the inorganic membrane such as to cater for, and feed, order-for-order exchanges while concomitantly allowing for an entropy increase via waste disposal. In an attempt to resolve how this might turn out to be, we return to consider the natural chemical garden membranes comprising this ferroferric-carbonate DLH and subordinate iron sulfides, further buttressed, perhaps, by silica and/or greenalite (Mielke et al., 2011; Russell et al., 2013; McMahon, 2019; Tosca et al., 2019; Barge et al., 2020, 2015a; Rasmussen et al. 2021). These are the minerals comprising the Hadean to Archaean banded iron formations (Halevy et al., 2017; Tosca et al., 2019), which are presumed to be the overspill of hydrothermal exhalations (Russell, 1975; Pons et al., 2011; Mloszewska et al., 2012).
As usually understood, crystal-hydrate gardens are self-ordered structures driven by the osmotic flow of the external alkaline water across a spontaneously precipitated semipermeable barrier (inorganic membrane) drawn inward by the high concentrations of a hydrous acidic salt as it dissolves in its water of crystallization (Leduc, 1911; Cartwright et al., 2002; Barge et al. 2012, 2015b). Growth is generally limited to tens of minutes to a few hours by the initial crystal’s mass as a result of the time taken to approach equalization of the ionic (and thereby the hydrostatic) pressures as inhibited by water stiction (Ding et al., 2016). Perret (1960) suggests that the spontaneous occurrence of expanding systems in a non-living environment such as a chemical garden might mark the first step toward the evolution of living organisms. However, in the case of the hydrothermal gardens, the expansion would mostly be the result of injection of alkaline into an ambient acidulous solution rather than osmosis (Russell et al., 1989, 1994; Russell, Hall, 1997; Mielke et al., 2011; Barge et al., 2015a). The chemical garden-like spires would continue to develop incrementally as the internal fluid perforates or breaks through, mostly at the top where the membrane is the thinnest, and reacts with the ambient fluid to produce a further segment (Barge et al., 2015b). The flow in this case is not osmotic, but ‘chemiosmotic’, where the inward transmission of individual protons via the Gröthuss mechanism (Muñoz-Santiburcio and Marx, 2017) is ~ 6 orders of magnitude faster than the outward velocity of hydroxyl ions as calculated for iron monosulfide membranes in the microfluidic experiments of Vasiliadou et al. (2019).
Extrapolation from similar microfluidic experiments involving chemical garden-like membranes comprising fougerite, as well as subsidiary mackinawite nanocrysts, is expected to reduce these external protons to hydrogen, and reduce carbonate to carbon monoxide and carboxylic acids; nitrate and nitrite to nitric oxide and ammonium; and furthermore, that the ammonium ion would aminate the carboxylic ions to the ‘short’ amino acids such as glycine, alanine, aspartate, serine, ornithine, and lysine (Hafenbradl et al., 1995; Huber and Wächtershäuser, 1998; Grégoire et al., 2016; Barge et al., 2019). Furthermore, there is some evidence to suggest that such amino acids would condense to short peptides within the confines of the interlayers of the fougerite and other DLHs where most of the water is not free, but is bound to the interior walls or even on their outer surfaces (Huber and Wächtershäuser, 1998; Rode, 1999; Huber et al., 2003; Grégoire et al., 2016, 2018; Erastova et al., 2017; Muñoz-Santiburcio and Marx, 2017; Branscomb and Russell, 2019; Rimola et al., 2022). In broad support of this view, Holden et al. (2022) show, using the electrospray mass spectrometry, that a substantial reduction in water activity does drive the condensation of glycine and alanine to dipeptide and, in droplet fusion reactions, protonated tri- and tetra-glycines. Additions of single glycines thereafter produced Gly6, an introduction to a peptide ‘world’ (Holden et al. 2022). Moreover, Boigenzahn and Yin (2022) demonstrate the condensation of glycylglycine to oligoglycines driven by trimetaphosphate at low water activities (cf. Yamagata et al., 1997).
The formation of peptide isomers on the microsecond timescale within the interlayers on fougerite could further support the potential role of confined-volume systems in abiogenesis. That is, by membrane, cell-wall, and biofilm-like structures built from materials generated on site rather than from random organic molecules supposedly delivered haphazardly to the mound from Fischer–Tropsch reactions and the like, generated remotely at depth in the crust (Muñoz-Santiburcio and Marx, 2017; Holden et al., 2022; Römling, 2022). The addition of amino acids and short peptides to the inorganic membranes renders the chemical gardens significantly more durable (Russell et al. 1994; McGlynn et al. 2012; Barge et al., 2019; Hooks et al., 2020; Flores et al., 2021; Borrego-Sánchez et al., 2022). Moreover, as so generated, the backbone amides in short peptides would prove irresistible to inorganic ions and complexes through hydrogen bonding in the membrane—sequestering them to make enzyme-like structures such as hydrogenase and ferredoxin analogs (Nitschke and Russell 2013; Nitschke et al. 2022). Furthermore, being attractive to each other, they can produce robust peptide membranes involving the same ions—the beginning of the organic takeover (Zhang et al., 1993; Milner-White and Russell, 2005, 2008, 2011; Maury, 2009; Bianchi et al., 2012; Zhang, 2012; Kandemir et al., 2016).
Baranov et al. (2016) note that flexible linear peptides would have more structural uses and functions than cyclic molecules in the first stages of life. Moreover, Popov (1999) emphasizes that the peptide folding itself is a nonlinear non-equilibrium thermodynamic process. Intriguingly, H+-PPases boast of a phosphate-binding site, a protein loop (P-loop) homologous with that of ATPases, that sequesters phosphate. And just such a peptide has since been assembled in the laboratory from a mixture of simple amino acids rich in glycine, whereby its flexible backbone is shown to cosset and sequester phosphate with two of the three main chain NH groups comprising the glycine-rich peptide backbone, that is, through hydrogen bonds to the phosphate ion which thereby bridges it to take the concave form (Milner-White and Russell, 2008; Bianchi et al., 2012).
The discoveries that both bacteria and archaea have prion-like domains allow the consideration of prions being pre-LUCA (Prusiner, 1998; Zajkowski et al., 2021). It is notable that uncoded peptides are self-recognizing and tend to arrange themselves as parallel 𝛼-sheets that can spontaneously convert into the more stable and insoluble amyloid 𝛽-sheet by plane flipping (Armen et al., 2004; Milner-White et al., 2006; Hayward and Milner-White, 2008, 2021; Milner-White, 2019). Such self-propagating and temperature-resistant sheets are much stronger than lipids and have the potential to act at the emergence of life in such roles as cell membrane/cell walls and biofilm analogs (Kosolapova et al., 2020). Furthermore, comprising membranes their backbones can sequester metals and phosphate without reliance on the side-chain order (Zhang et al., 1993; Milner-White and Russell, 2005; Childers et al., 2009, 2010; Maury, 2009; Greenwald and Riek, 2010; Li et al., 2010; Goodwin et al., 2012; Milner-White, 2019). Other short peptides that do involve side chains have shown similar or superior mastery over metal-ion chelation and, thereby, agency (Aithal et al., 2023; Timm et al. 2023).
Of course, the popular view is that lipids constituted the first organic membranes but there is no theoretical or experimental evidence to suggest how they would be produced in the protometabolic system autotrophically at plausible rates and temperature. What use would they have beyond acting, as they do today, as membrane fillers and lubricants? After all, in contrast to lipids, peptides and amyloids are, (i) interactively cooperative with other ions, (ii) stronger as in their involvement in a web-like role in cell walls, strong enough even to contain turgor pressures (Kandler and König, 1998; Desvaux et al., 2006), (iii) can be seen to have roles extending to the enzyme structure, proteins, and cofactors, (iv) as well as much of the membrane, and (v) as prions they seemingly offer autonomous and ‘intentional exploration’ of space and time for similar disequilibria (Chernoff, 2004; Lupi et al., 2006; Maury, 2009; Coca et al., 2021; Jheeta et al., 2021; Zajkowski et al., 2021).
Under alkaline vent conditions, amyloid peptides and amyloid fibrils would be expected to exude from the interlayers to produce organic molecular webs adhering to the spire’s inner walls as metal-dosed organic films (Takahashi and Mihara, 2004; Larsen et al. 2007; Römling, 2022; Figure 3). Subject to entrainment in the hydrothermal updrafts, some of this amalgam is likely to spall off in the general direction of flow, eventually crowding and necking to form offspring capable of interacting and sharing the peptide film (Milner-White and Russell, 2005; Larsen et al. 2007; Greenwald and Riek, 2010; Greenwald et al., 2018; Römling, 2022). Yet, it is admitted that this speculation falls well short of how prions in membrane or cell-wall microbes could segue to a peptide–nucleotide world governing metabolic pathways and the reverse Krebs cycle (Gallardo and Rodhe, 1997; Schumann and Huntrieser, 2007; Carey et al. 2016; Weiss et al. 2016; Bromberg et al., 2022; Harrison et al., 2022; Lane, 2022; Palmeira et al., 2022). For this, we are forced to consider the emergence of an albeit imperfect genetic governance to get life into its historical and present role.
Figure 3. (A) Sketch of chemical garden spires comprising ferroferric oxyhydroxide and minor iron sulfide that spontaneously precipitate and continue to grow through injection at alkaline/acidic interfaces (Barge et al., 2015a, b; Helmbrecht et al., 2022; Akbari and Palsson, 2022b; see Figure 1). Short peptides produced in the fougerite interlayers are presumed to be gradient-driven vectorially through the fougerite nanocrysts from outside to the spire’s inner surface where they are hypothesized to form metal-dosed organic films signified here by brown color (cf., Oda and Fukuyoshi, 2015; Römling, 2022). Within certain limits of externally applied disequilibria, the fougerite or similar DLHs act like an enzyme, in that organic production rates remain constant in spite of fluctuations in supply (Pitsch et al., 1995; Hansen et al., 1996; Kolb et al., 1997; Branscomb et al., 2017). Continuation of the process leads to spalling and entrainment and eventual crowding at the growing spire’s tip where necking-off produces organic/inorganic cells that gravitate to depth in a geode (also brown; Russell and Martin, 2004): (B) natural chemical garden sulfide bubbles by comparison in the 340 Ma Tynagh orebody (Russell and Hall, 1997).
Furthermore, Abel and Trevors (2006) provide a cogent argument against the assumption that the complexification of peptide- and prion-assisted metabolisms is enough to initiate vertical evolution without the insertion of a rule-based physicochemical program. In other words, we have to face up to the introduction of a material program, which makes life distinct in being able to defend itself by exerting an autonomous choice, if surprised by external alterations beyond itself (Kordium, 2021). This is in contrast to ALL other simple self-ordering phenomena driven by the many varieties of known disequilibria (Russell et al., 2013; Ramstead et al., 2019).
However, there is at present no clearcut path from a putative fougerite–mackinawite–peptide reproducing system to a nucleotide-based replicative one.
Noting the main dilemma in the origin-of-life research, Freeman Dyson famously suggests that life must have originated twice “with two separate kinds of creatures, one kind capable of metabolism without exact replication and the other kind capable of replication without metabolism” (Dyson, 1986). Metabolism’s creature seems to emerge through the synthesis and reproduction of amino acids and even peptides but has nowhere to go, while the replication creature is supposedly born whole in an age of information, yet cannot find the wherewithal to ‘be’. These two creatures each have their champions and the regrettable outcome is the erection of an intellectual wall built between the ‘computing replicationists/geneticists’ and the ‘engineers of metabolism’. What to do?
The ‘metabolists’ do need to find geologically informed ways to synthesize the nucleotides indispensable to making a code. The ball is in our court. Just how information was introduced early into the engines of metabolism is the ‘hard problem of life’ (Walker and Davies, 2017; Wong and Prabhu, 2023). From a crystallographic perspective, we might start with the size and shape, recalling Erwin Schrödinger’s classification of the gene as an aperiodic crystal (Schrödinger 1944). Moreover 2 years later, Linus Pauling (1946) promulgated his views on the importance of complementarity of molecular shape as determinants of their interactions (Pauling, 1946), originally considered as the “lock and key” requirement for molecular interactions (Fischer, 1894) and the “side chain theory” of Ehrlich (1901). These articles set the scene for the self-assembly hypotheses of: (1) Dounce’s (1953) nucleic acid template hypothesis, whereby the ribonucleic acids synthesized on the gene templates would, in turn, become templates for protein synthesis, (2) Gamow’s (1954 and Gamow and Yčas, 1955) double coding hypothesis, in which “amino acid residues in proteins are selected by independent triplets of nucleotides,” and (3) Nirenberg and Leder’s (1964) “affinity method.”
Woese et al. (1966) picked up on these ideas, framing the issue in terms of “whether or not amino acid-oligonucleotide steric interactions play or have played a role in determining these assignments, and if so, to what extent?” Their resounding and “essentially unavoidable conclusion” is that “codon assignments manifest an underlying codon-amino acid pairing”; a conclusion still resonating today (Woese et al., 1966; Russell et al., 2003; Yarus et al., 2005, 2009; Yarus, 2021; Harrison et al. 2022). Moreover, it leads to Massimo Di Giulio’s (2008) hypothesis of an extension of the coevolution theory for the origin of the genetic code.
While these theories have been widely entertained, experimental exploration is limited. One notable success is due to Mellersh and Wilkinson (2000) who demonstrated, for example, how “polyadenylic acid immobilized on silica gel stereoselectively binds L-lysine from dilute aqueous solution”… and so facilitates “subsequent amide bond formation” (Russell et al., 2003). At the same time, Levy and Ellington (2003) expand upon their ideas regarding peptide-templated nucleic acid ligations. Mike Yarus (2017) confirms these affinities by demonstrating that the RNA–amino-acid interface logically relates triplets to the side chains of particular amino acids, concluding that “peptides may have been produced directly on an instructive amino acid binding RNA” (Yarus, 2017). We should also note the possibility that, given the degeneracy in the genetic code, the progenitors of the earliest genetic code were codons with four bases (or more)—the tessera codes of Baranov et al. (2009) and Gonzalez et al. (2012, 2019). Yarus (2011) also sees a way of “getting past the RNA world”—a world that never was according to Yockey (1995), Kurland (2010), and Wills and Carter (2018).
Wächtershäuser (1990), Martin and Russell (2007), and Harrison and Lane (2018) have made attempts at a “progression” but it might be argued, to use Stanley Miller’s apothegm, that these erections are nothing more than “paper chemistry” (Hagmann, 2002, but see Polanyi (1962, p. 165) for a thoughtful defense of such ‘speculations’). Abel and Trevors (2006) attempt to discipline the “metabolists,” by pointing out that, absent a program, metabolic cycling is doomed to docile repetition as long as their particular driving disequilibria last, as per the laws of chemistry and physics. To animate metabolism and make it reflexive, we cannot expect complexification per se to answer the conundrum (Abel, 2011). The workings of life have to be understood in terms of their entirety and as Abel (2011) emphasizes, work itself “entails more than spontaneous phase transitions.” Once metabolism’s disequilibria-converting engines are up and running, to allow the system as a whole to progress and evolve they all must be algorithmically directed, and continually replaced (Trixler, 2021). Moreover, to last, any product stemming from the alkaline mound has to have its use as a component part of each metabolic engine; has to pay its way or be discarded (Branscomb et al., 2017). There seems nothing for it but to seek a non-ribosomal peptide synthetase that includes nucleotides in its structure (Kleinkauf and von Döhren, 1996; von Döhren et al., 1999; Fischbach and Walsh, 2006).
Attempts to assail this conceptual wall have been less than successful. However, just this year some ‘cracks’ have appeared on the metabolist-cum-chemical side. The Nick Lane–Stuart Harrison group at the Department of Genetics, Evolution and Environment, University College London, look to how randomly synthesized nuclear monomers could become involved in the very basis of metabolism—namely, as nucleotide catalysts in CO2 hydrogenation and in amination of carboxylates to amino acids (Harrison et al. 2022; Palmeira et al., 2022; Pinna et al. 2022). This forward-looking approach can explain why ATP is universally conserved across life (Pinna et al., 2022). Moreover on this side, in a series of experiments, Joseph Moran’s group at the Institut de Science et d’Ingénierie Supramoléculaires, Strasbourg, France, demonstrates the likely networks followed by the earliest non-enzymatic metabolic pathways, for example, metal-ion transaminations (Mayer et al., 2021), the centrality of iron in catabolic as well as anabolic processes (Muchowska et al., 2019, 2020), and the abiotic conversion of aspartate to orotate and further reactions to produce all three of the pyrimidine nucleobases in water at 60°C catalyzed by a variety of metal ions along with an oxidant (Yi et al., 2022). Furthermore, Müller et al. (2022) explore ‘palaeochemistry’ in their search for a plausible scenario of an RNA–peptide world, while Akbari and Palsson (2022a) formulate how metabolic homeostasis and cellular growth might arise in the acetyl coenzyme-A pathway and the reductive TCA cycle, and Helmbrecht et al. (2022) demonstrate the accumulation of RNA in amakinite/fougerite chemical gardens.
How much of this might progress in the precellular DLH world? Pitsch et al. (1995) demonstrate how a weakly alkaline 20 μM solution of glycolaldehyde phosphate can, once absorbed within M2+/M3+ oxyhydroxide interlayers of green rust, be transformed to hexose- and pentose-phosphates—the latter structurally related to the sugar phosphate units in RNA. Moreover, Krishnamurthy et al. (1996) demonstrate that the ‘alternative’ nucleic acid pyranosyl ribose-2,4-phosphate, the near-planar sugar phosphate structure formed in similar conditions, is a nucleic acid with exceptional base-pairing properties (Eschenmoser, 1994). Krishnamurthy et al. (1996) show that, on introducing formaldehyde and glyceraldehyde phosphate into the DLH interlayer, 40% of the product consists of pyranosyl ribose.
Perhaps, the secret of “The First Cell,” the title of Azra Raza’s book, which refers to the first cancer cell in an oncology case, is also the secret of the very first cell (Raza, 2019; Marshall, 2021; see Szent-Györgyi, 1968). According to Bernhardt and Patrick (2014), genetic code evolution started with the incorporation of glycine, followed by other small hydrophilic amino acids. Certainly, once the genetic code is sophisticated to the extent of being able to take on surprises and make choices and generate novel information (Marshall, 2021), there can be a rush to infest the entire hydrothermal mound. And life is ready for its diaspora. While relatively slow to colonize the ocean crust at first, as an entropy generator able to pick up any stray leftovers from other disequilibria generating systems, life takes over the surfaces on, and within, our planet eventuating in photosynthesis (Russell and Arndt, 2005; Figure 4).
Figure 4. Emergence of the deep biosphere. Autotrophic life emerges and rapidly infects a hydrothermal alkaline mound (Figure 1) and differentiates interdependently into the precursors of the bacteria and archaea, grows by expansion downward and laterally into the surrounding sediments and serpentinizing ocean crust, thus initiating the deep biosphere, a hypothesis now broadly supported by recent research (Fones et al., 2019, 2021; Boyd et al., 2020; Berkemer and McGlynn, 2020; Leong et al., 2021; Colman et al., 2022). Numbers 1–3 relate to life’s emergence, while 4 marks the supposed point of differentiation of the archaea and the bacteria. Roman numerals V–VI mark evolutionary stages of the archaea, and number 5 shows the stages of evolution of the bacteria in the deep biosphere (redrawn from Russell and Arndt, 2005).
In the present AVT, life is rooted in methanotrophic acetogenic microbes respiring nitrate (Nitschke and Russell, 2013). The taproot itself is grounded in fougerite, which sees to the harnessing of ambient H2, CH4, and CO2 driven by the natural proton motive force and respiration of oxidized nitrogen entities (Barnes and O’Neil, 1969; Neal and Stanger, 1983; Ducluzeau et al., 2009, 2014). Once this system is up and running at the outer margins of the submarine alkaline hydrothermal mound, its requirements are, nevertheless, highly constricting. The evolutionary breakout (‘break in’) comes with metabolism’s discovery of how to survive on the much reduced free energy from readily available H2 and CO2 within the mound itself. We speculate that Christian Schöne et al.’s (2022) exciting finding of the facile conversion of an archaeal methanogen to a carbon-monoxide-dependent acetogen through the removal of cellular function could be read as an indication of how “reverse methanotrophy” segued to an acetogenesis, perhaps related to the differentiation of the progenote into the archaea and bacteria (Russell and Nitschke, 2017; cf., Lyu et al., 2022). This admittedly contentious suggestion sees a parallel in human society having to burn hydrocarbons while ‘waiting for nuclear fusion(s)’.
Entrainment of the earliest microbes in a hydrothermal effluent to the ocean would lead to their immediate starvation. Thus, it is surmised that the only survivors are those who are caught up in an involuntary and random growth and expansion in a downward front to inaugurate the deep biosphere (Pedersen, 1993; Parkes et al., 1994; Parkes and Wellsbury, 2004; Russell and Arndt, 2005; Glasby, 2006; Schrenk et al., 2013). In such conditions, the ‘law of natural rejection’ would see all but the most efficient cells or cellular cooperatives die-off.
In the oceanic crust itself, these survivors would have missed the profusion of the mound and been drastically thinned out and stripped of non-essential genes (Fones et al., 2019, 2021). Fones et al. (2021) make the cogent argument that the absence of CO2 in this new environment drives the adaptation of methanogens to generate their own. Discrete ocean downdrafts are another source of CO2. Yet, the remaining feedstocks, while restricted, are otherwise not so different in the serpentinizing throat and ultramafic surrounds, perhaps also supplying the electron-donating H2, CH4, HCOO−, CO, and CH3COO− (Windman et al., 2007; White et al., 2020). The latter four entities also supply the substrate carbon (White et al. 2020). Such alkaline fluids are known to support microbial nitrate and nitrite bacterial reduction (Albina et al., 2021).
Colman et al. (2022) offer a window into the effect of such conditions in their exhaustive study of the Semail ophiolite. Not only are reproduction rates much diminished but genetic diversity too is “streamlined” for survival (Colman et al., 2022). Furthermore, syntrophy, gene swapping both within and across domains, and the sharing of nutrients keep the microbiome operating (Wolin, 1982; Russell and Arndt, 2005; Tiago and Veríssimo, 2013; Kohl et al., 2016; Brazelton et al. 2017; Suzuki et al., 2017, 2018; Colman et al. 2022).
Extrapolating across the 4 billion years since life’s onset, we gauge from Colman et al. (2022) that the autotrophic acetogenic analogs comparable to what they term type II Acetothermia would survive displacement from the mound into the hyperalkaline waters in equilibrium with incipient serpentinization. These types of Acetothermia employ an archaeal-like carbon-monoxide dehydrogenase and ferredoxin-based complexes to achieve acetogenesis. Moreover, they have the capacity for respiratory growth using nitrate (Youssef et al., 2019). Other bacteria revealed by metagenomics are the sulfate-reducing bacteria (Brazelton et al., 2017; Rempfert et al., 2017; Templeton et al., 2021).
Although apparently missing from the Semail ophiolite, sequences of an anaerobic Methanotroph group 1 (ANME-1) have been identified in the serpentinization-driven alkaline Cabeço de Vide aquifer in Portugal, and unclassified anaerobic methanotrophic euryarchaeota (ANME) MAG are recorded from Lost City (Tiago and Veríssimo, 2013; Nothaft et al., 2021). However, suggestions that these findings provide an inkling of support for the methanotrophy-first speculation of Nitschke and Russell (2013) are put on hold by the likelihood of the present-day “contamination from surface waters” (Merkel et al., 2013; Postec et al., 2015; Trutschel et al., 2022).
Experiments on, and analyses of, AVT look to an evolutionary tree with its deepest roots in cosmogenesis—yet reaches upward and along the lowest branches of the acetyl coenzyme-A and an incomplete reverse TCA cycle (Nitschke and Russell, 2010; Russell et al., 2013; Carroll, 2016).
Findings and some predictions of AVT are that:
1. A natural proton disequilibria measuring between 2 to 5 pH units imposed across an FeS membrane separating a hydrogen-bearing alkaline solution from a carbonic ocean drives the hydrogenation of the CO2 to formate/CO (Russell and Hall, 1997; Branscomb and Russell, 2013; Vasiliadou et al., 2019) is verified (Hudson et al., 2020), although most of the iron in the membrane is now argued to be in fougerite/green rust rather than in FeS (Russell et al., 2013).
2. An expectation of AVT is that methane derived from the lower mantle and entrained in the alkaline hydrothermal fluid will oxidize to a methyl group within green rust (Nitschke and Russell, 2013), itself (re)oxidized by NO and nitrate provided through …
3. appreciable volcanic and hurricane cloud-to-cloud lightning, bolide impacts, and photochemistry from the CO2 + N2 atmosphere dissolved in the ocean as CO2, NO3−, and NO2− (Mancinelli and McKay, 1988; Gallardo and Rodhe, 1997; Kasting and Siefert, 2001; Ducluzeau et al., 2009; Wong et al., 2017; Navarro-González et al., 2019).
4. Although conclusion 3 is challenged by Ranjan et al. (2019), the view that the nitrogen oxides were present in the Earth’s early atmosphere and that their derivatives also invaded the ocean is now strongly reinforced by Buessecker et al. (2022) who suggest green rust to be responsible for carrying NO to depth bound as nitrosyl (and see Heays et al., 2022; Nishizawa, 2022) …
5. in turn supporting the denitrifying methanotrophic acetogenesis hypothesis as the first pathway to life, predating the more demanding acetyl coenzyme-A pathway (Nitschke and Russell, 2013; Russell and Nitschke, 2017) …
6. and that the amination of pyruvate and oxalate to alanine and glycine which can be accomplished via fougerite (Nitschke and Russell 2013) is verified by Barge et al. (2019, 2020) …
7. while the condensation of amino acids to peptides within the 2D interlayers of peptides has support from Muñoz-Santiburcio and Marx (2017), Erastova et al. (2017), Grégoire et al. (2016, 2018), and Holden et al. (2022).
8. Fougerite interlayers are capable of dampening externally imposed disequilibria to produce an enzyme-like flux-force linearity to interactants (Branscomb and Russell, 2019), …
9. although indications of binding, binding-change, and disequilibria-conversion mechanisms (Russell, 2018) await experimentation using the operando X-ray absorption spectroscopy and allied techniques (Fracchia et al., 2018).
10. That the deep biosphere—initially the serpentinizing Hadean ocean crust—is first populated with respiring denitrifying methanotrophic archaea and acetogenic bacteria from their point of ‘origin’ (the progenote) in a submarine alkaline hydrothermal mound (Russell and Arndt, 2005; Nitschke and Russell, 2013; Ménez, 2020) is supported by circumstantial evidence; e.g., the bacterium Acetothermia is capable of respiration with nitrate (Fones et al., 2019, 2021; Youssef et al., 2019; Boyd et al., 2020; Colman et al., 2022) and an archaeon methanotroph, cf. ANME-1 (Tiago and Veríssimo, 2013; Brazelton et al., 2017). We might imagine these progenotes happening upon new locations with high concentrations of dissolved ions and gases, carried there passively by percolating solutions.
The production of alkaline hydrothermal waters through the serpentinization of mafic to ultramafic rocks, as introduced by Ivan Barnes et al., underpins AVT (Barnes and O’Neil, 1969; Barnes et al., 1972; Russell et al., 1989; Macleod et al., 1994). These authors also figure in the description of the Lost City vents discovered in 2000 (Kelley et al., 2001). The serpentinizing system that produces such alkaline submarine emissions has, through a reading of Cottrell (1979), since been described in terms of a disequilibria- (‘free-energy’-) converting cracking engine (Russell et al., 2013). While AVT originally assumed a sulfide mound to be generated at the spring-to-ocean interface, it became apparent that the double-layer oxyhydroxide green rust (fougerite) would be the major ferroferric precipitate along with some sulfide and Mg-rich clays and silica (Russell and Hall, 1997; Russell and Arndt, 2005). Because of its physicochemical flexibility, this macromolecular 2D mineral could also be considered the necessary nanoengine/protomotor enzyme—in this case to convert the disequilibria between H2 + OH− + CH4 and CO2 + NO3− + H+ to the rudiments of the denitrifying methanotrophic acetyl coenzyme-A pathway—the interlayers acting as precursor metabolic channels toward further downstream organic synthesis (Nitschke and Russell, 2013; cf. Srere, 1987).
The current AVT for life’s emergence has it that fougerite-rich hydrothermal electrochemical gardens (fine chimney stalks and spires) mark the precellular stage [Russell et al., 2005, 2013 (Figure 3B); Barge et al., 2015b; Chin et al., 2020; Nitschke et al., 2022 (Figure 3)]. We imagine a well-ordered convective alkaline updraft feeding reductants to the also well-ordered macromolecular fougerite comprising the growing spires and chimneys, which in turn allows a well-ordered infiltration and vectorial flow of protons and anions from the ocean directed through the interlayers (Borrego-Sánchez et al., 2022). The result is an effusing tangle of organic molecules probably dominated by peptides to coat the inner wall of the spires (Akbari and Palsson, 2022b; Römling, 2022). Portions of this organic film spall off and are entrained in the flow, along with other organic molecules released by delamination and/or by diagenetic alteration to magnetite at depth in the mound. Some of this organic material may be ‘attracted’ to form cellular structures that are capable of sensing and responding to oscillations and fluctuations in supply and perhaps prove eventually to be self-sustaining, and, on the development of emerging genetic algorithms, would be able to make choices and generate novel information and rapidly infect the mound (Cain, 1949; Marshall, 2021).
Thus, beginning with the insights of Ivan Barnes et al., we argue that:
1. Serpentinization is the inescapable response of the Hadean Earth’s ultramafic crust to the circulation of ocean water. Moreover, it is equally inescapable that the preorganized macromolecule fougerite is a prerequisite for the dissipation of the disequilibria resulting from the return of serpentinite’s effluent to its original source, the Hadean carbonic ocean (Tatzel et al., 2022).
2. Just as cracks in the Hadean ocean floor are a prerequisite for priming serpentinization, so do aqueous interlayers within redox/pH-sensitive fougerite nanocrysts acquiesce to the forceful vectorial invasions from either flank of the precipitate membrane of pent-up ions, charged and uncharged fuels and oxidants, eventuating in protometabolisms (Nitschke and Russell, 2013).
3. However while fougerite may be considered to provide simple messages to govern a product, it is presumed that prion-like offspring may offer seemingly autonomous and ‘intentional exploration’ of space and time for similar disequilibria (Maury, 2009).
4. However, a second chapter on life’s emergence awaits an understanding of the paths from the earliest coherent pregenetic governing algorithms of life to the emergence of the “modern synthesis”—both barely written introductions to the subsequent well-versed chapters of life’s unitingly diversifying Gaian commonwealth where “everything is everywhere (though the environment selects)” (Baas Becking, 1931, 1934; Quispel, 1998; Abel and Trevors, 2006; Igamberdiev, 2021); pace (Martiny et al. 2006).
An understanding of how life both emerges and thrives requires the use of stochastic or trajectory thermodynamics—equilibrium thermodynamics is absolutely inappropriate for the task (Astumian, 2018, 2020; Bartlett and Beckett, 2019; Branscomb Russell, 2019; Horowitz and Gingrich, 2020; Feng et al., 2021; Ueltzhöffer et al., 2021). Furthermore, of course, experiments are required to test the hypothesis that self-sustaining hydrothermal ‘electro-chemical gardens’ comprising the minerals fougerite and mackinawite as nanoengines and nanoengine mountings are up to the task of engendering the earliest steps of life (Figures 2, 3). Thereafter, computer modeling and artificial intelligence beckon as ways of further resolving sequences of life’s emergence (Ugliengo, 2019; Hassabis and Revell, 2021).
The original contributions presented in the study are included in the article/supplementary material, further inquiries can be directed to the corresponding author.
The author confirms being the sole contributor of this work and has approved it for publication.
Eric Boyd, Elbert Branscomb, Steffen Buessecker, Simon Duval, Orion Farr, Emma Forrest, Wolfgang Nitschke, Piero Ugliengo, and Michael Wong are thanked for their help and discussions. Any mistakes or misconceptions are my own.
The author declares that the research was conducted in the absence of any commercial or financial relationships that could be construed as a potential conflict of interest.
All claims expressed in this article are solely those of the authors and do not necessarily represent those of their affiliated organizations, or those of the publisher, the editors and the reviewers. Any product that may be evaluated in this article, or claim that may be made by its manufacturer, is not guaranteed or endorsed by the publisher.
Abel, D. L., and Trevors, J. T. (2006). Self-organization vs. self-ordering events in life-origin models. Phys Life Rev 3, 211–228. doi: 10.1016/j.plrev.2006.07.003
Adam, G., and Delbrück, M. (1968). Reduction of dimensionality in biological diffusion processes. Struct. Chem. Mol. Biol. 198, 198–215.
Aithal, A., Dagar, S., and Rajamani, S. (2023). Metals in prebiotic catalysis: a possible evolutionary pathway for the emergence of metalloproteins. ACS Omega 8, 5197–5208. doi: 10.1021/acsomega.2c07635
Akbari, A., and Palsson, B. O. (2022a). Positively charged mineral surfaces promoted the accumulation of organic intermediates at the origin of metabolism. PLoS Comp. Biol. 18:e1010377. doi: 10.1371/journal.pcbi.1010377
Akbari, A., and Palsson, B. (2022b). Metabolic homeostasis and growth in abiotic cells. bioRxiv. [Epub ahead of preprint]. doi: 10.1101/2022.10.16.512448
Albers, E., Bach, W., Pérez-Gussinyé, M., McCammon, C., and Frederichs, T. (2021). Serpentinization-driven H2 production from continental break-up to mid-ocean ridge spreading: unexpected high rates at the West Iberia margin. Front. Earth Sci. 9:487. doi: 10.3389/feart.2021.673063
Albina, P., Durban, N., Bertron, A., Albrecht, A., Robinet, J. C., and Erable, B. (2021). Nitrate and nitrite bacterial reduction at alkaline pH and high nitrate concentrations, comparison of acetate versus dihydrogen as electron donors. J. Envir. Manag. 280:111859. doi: 10.1016/j.jenvman.2020.111859
Anashkin, V. A., Malinen, A. M., Bogachev, A. V., and Baykov, A. A. (2021). Catalytic asymmetry in homodimeric H+-pumping membrane pyrophosphatase demonstrated by non-hydrolyzable pyrophosphate analogs. Internl J. molec. Sci. 22:9820. doi: 10.3390/ijms22189820
Appel, P. W. U. (1980). On the early Archaean Isua iron-formation, West Greenland. Precambrian Res. 11, 73–87. doi: 10.1016/0301-9268(80)90081-9
Armen, R. S., Alonso, D. O., and Daggett, V. (2004). Anatomy of an amyloidogenic intermediate: conversion of β-sheet to α-sheet structure in transthyretin at acidic pH. Structure 12, 1847–1863. doi: 10.1016/j.str.2004.08.005
Arrhenius, G. (1984). “Minerals with channel structure as substrates for nucleotide and peptide synthesis” in Fundamental Studies and the Future of Science. ed. C. Wickramasinghe (Cardiff UK: University College Cardiff Press), 301–319.
Arrhenius, G. O. (2003). Crystals and life. Helv. Chim. Acta 86, 1569–1586. doi: 10.1002/hlca.200390135
Arrhenius, G., Gedulin, B., and Mojzsis, S. (1993). “Phosphate in models for chemical evolution” in Chemical Evolution and Origin of Life. eds. C. Ponnamperuma and J. Chela-Flores (Hampton, VA: A. Deepak Publishing), 25–50.
Arrhenius, G., Sales, B., Mojzsis, S., and Lee, T. (1997). Entropy and charge in molecular evolution—the case of phosphate. J. Theor. Biol. 187, 503–522. doi: 10.1006/jtbi.1996.0385
Asimakidou, T., Makridis, A., Veintemillas-Verdaguer, S., Morales, M. P., Kellartzis, I., Mitrakas, M., et al. (2020). Continuous production of magnetic iron oxide nanocrystals by oxidative precipitation. Chem. Eng. J. 393:124593. doi: 10.1016/j.cej.2020.124593
Astumian, R. D. (2007). Design principles for Brownian molecular machines: how to swim in molasses and walk in a hurricane. Phys. Chem. Chem. Phys. 9, 5067–5083. doi: 10.1039/b708995c
Astumian, R. D. (2012). Microscopic reversibility as the organizing principle of molecular machines. Nat. Nanotech. 7, 684–688. doi: 10.1038/nnano.2012.188
Astumian, R. D. (2018). Trajectory and cycle-based thermodynamics and kinetics of molecular machines: the importance of microscopic reversibility. Acc. Chem. Res. 51, 2653–2661. doi: 10.1021/acs.accounts.8b00253
Astumian, R. D., Mukherjee, S., and Warshel, A. (2016). The physics and physical chemistry of molecular machines. ChemPhysChem 17, 1719–1741. doi: 10.1002/cphc.201600184
Astumian, R. D., Pezzato, C., Feng, Y., Qiu, Y., McGonigal, P. R., Cheng, C., et al. (2020). Non-equilibrium kinetics and trajectory thermodynamics of synthetic molecular pumps. Mat. Chem. Front. 4, 1304–1314. doi: 10.1039/D0QM00022A
Baas Becking, L. G. M. (1931). Gaia of leven en aarde. Oratie gewoon hoogleraar, Rijksuniv. Leiden, Mart. Nijhoff’s Gravenhage, 1–20.
Baas-Becking, L. G. M. (1934). Geobiologie of inleiding tot de milieukunde. Van Stockum & Zoon NV, The Hague.
Ballard, R. D., and Grassle, J. F. (1979). Incredible world of deep sea rifts. Natl. Geogr. 156, 680–705.
Baltscheffsky, M., Baltscheffsky, H., and Von Stedingk, L. V. (1966). “Light-induced energy conversion and the inorganic pyrophosphatase reaction in chromatophores from Rhodospirillum rubrum” in Brookhaven Symposia in Biology, 246–257.
Baltscheffsky, M., Schultz, A., and Baltscheffsky, H. (1999). H+-PPases: a tightly membrane-bound family. FEBS Lett. 457, 527–533. doi: 10.1016/S0014-5793(99)90617-8
Banks, D. A. (1985). A fossil hydrothermal worm assemblage from the Tynagh lead–zinc deposit in Ireland. Nature 313, 128–131. doi: 10.1038/313128a0
Baranov, V. I., Gribov, L. A., and Mikhailov, I. V. (2016). Processes of the development of the molecular world at early stages of its formation: evidence from the investigation of the behavior of the vibrational entropy of molecules. Geochem. Int. 54, 929–935. doi: 10.1134/S0016702916110021
Baranov, P. V., Venin, M., and Provan, G. (2009). Codon size reduction as the origin of the triplet genetic code. PLoS One 4:e5708. doi: 10.1371/journal.pone.0005708
Barge, L. M., Abedian, Y., Russell, M. J., Doloboff, I. J., Cartwright, J. H., Kidd, R. D., et al. (2015a). From chemical gardens to fuel cells: generation of electrical potential and current across self-assembling iron mineral membranes. Angew. Chem. Int. Ed. 54, 8184–8187. doi: 10.1002/anie.201501663
Barge, L. M., Cardoso, S. S. S., Cartwright, J. H. E., Cooper, G. J. T., Cronin, L., De Wit, A., et al. (2015b). From chemical gardens to Chemobrionics. Chem. Rev. 115, 8652–8703. doi: 10.1021/acs.chemrev.5b00014
Barge, L. M., Doloboff, I. J., White, L. M., Russell, M. J., and Kanik, I. (2012). Characterization of iron-phosphate-silicate chemical garden structures. Langmuir 28, 3714–3721. doi: 10.1021/la203727g
Barge, L. M., Flores, E., Baum, M. M., VanderVelde, D. G., and Russell, M. J. (2019). Redox and pH gradients drive amino acid synthesis in iron oxyhydroxide mineral systems. Proc. Natl. Acad. Sci. U. S. A. 116, 4828–4833. doi: 10.1073/pnas.1812098116
Barge, L. M., Jones, J. P., Pagano, J. J., Martinez, E., and Bescup, J. (2020). Three-dimensional analysis of a simulated prebiotic hydrothermal chimney. ACS Earth Space Chem. 4, 1663–1669. doi: 10.1021/acsearthspacechem.0c00186
Barnes, I., and O’Neil, J. R. (1969). The relationship between fluids in some fresh alpine-type ultramafics and possible modern serpentinisation, Western United States. Geol. Soc. Am. Bull. 80, 1947–1960. doi: 10.1130/0016-7606(1969)80[1947:TRBFIS]2.0.CO;2
Barnes, I., O’Neil, J. R., and Trescases, J. J. (1978). Present day serpentinization in New Caledonia, Oman and Yugoslavia. Geochim. Cosmochim. Acta 42, 144–145. doi: 10.1016/0016-7037(78)90225-9
Barnes, I., Rapp, J. B., O’Neil, J. R., Sheppard, R. A., and Gude, A. J. (1972). Metamorphic assemblages and the direction of flow of metamorphic fluids in four instances of serpentinization. Contrib. Min. Pet. 35, 263–276. doi: 10.1007/BF00371220
Baross, J. A., and Hoffman, S. E. (1985). Submarine hydrothermal vents and associated gradient environments as sites for the origin and evolution of life. Orig. Life Evol. Biosph. 15, 327–345. doi: 10.1007/BF01808177
Bartlett, S. J., and Beckett, P. (2019). Probing complexity: thermodynamics and computational mechanics approaches to origins studies. Interface Focus 9:20190058. doi: 10.1098/rsfs.2019.0058
Berkemer, S. J., and McGlynn, S. E. (2020). A new analysis of archaea-bacteria domain separation: variable phylogenetic distance and the tempo of early evolution. Mol. Biol. Evol. 37, 2332–2340. doi: 10.1093/molbev/msaa089
Bernhardt, H. S. (2019). Making molecules with clay: layered double hydroxides, pentopyranose nucleic acids and the origin of life. Life 9:19. doi: 10.3390/life9010019
Bernhardt, H. S., and Patrick, W. M. (2014). Genetic code evolution started with the incorporation of glycine, followed by other small hydrophilic amino acids. J. Mol. Evol. 78, 307–309. doi: 10.1007/s00239-014-9627-y
Bianchi, A., Giorgi, C., Ruzza, P., Toniolo, C., and Milner-White, E. J. (2012). A synthetic hexapeptide designed to resemble a proteinaceous p-loop nest is shown to bind inorganic phosphate. ProteinsStruct, Funct, Genet 80, 1418–1424. doi: 10.1002/prot.24038
Bindeman, I. N., and O’Neil, J. (2022). Earth’s earliest hydrosphere recorded by the oldest hydrothermally-altered oceanic crust: triple oxygen and hydrogen isotopes in the 4.3-3.8 Ga Nuvvuagittuq belt, Canada. Earth Planet. Sci. Lett. 586:117539. doi: 10.1016/j.epsl.2022.117539
Boigenzahn, H., and Yin, J. (2022). Glycine to oligoglycine via sequential trimetaphosphate activation steps in drying environments. Orig. Life Evol. Biosph. 52, 249–261. doi: 10.1007/s11084-022-09634-7
Borrego-Sánchez, A., Gutiérrez-Ariza, C., Sainz-Díaz, C. I., and Cartwright, J. H. (2022). The effect of the presence of amino acids on the precipitation of inorganic chemical-garden membranes: biomineralization at the origin of life. Langmuir 38, 10538–10547. doi: 10.1021/acs.langmuir.2c01345
Boyce, A. J., Coleman, M. L., and Russell, M. J. (1983). Formation of fossil hydrothermal chimneys and mounds from Silvermines, Ireland. Nature 306, 545–550. doi: 10.1038/306545a0
Boyd, E. S., Amenabar, M. J., Poudel, S., and Templeton, A. S. (2020). Bioenergetic constraints on the origin of autotrophic metabolism. Phil. Trans. Roy. Soc. A 378:20190151. doi: 10.1098/rsta.2019.0151
Boyd, E. S., Spietz, R. L., Kour, M., and Colman, D. R. (2023). A naturalist perspective of microbiology: examples from methanogenic archaea. Environ. Microbiol. 25, 184–198. doi: 10.1111/1462-2920.16285
Boyer, P. D. (1979). “The binding-change mechanism of ATP synthesis” in Membrane Bioenergetics. eds. C. P. Lee, G. Schatz, and L. Ernster (Reading, MA: Addison-Wesley), 461–479.
Boyer, P. D. (1997). The ATP synthase—a splendid molecular machine. Annu. Rev. Biochem. 66, 717–749. doi: 10.1146/annurev.biochem.66.1.717
Brady, M. P., Tostevin, R., and Tosca, N. J. (2022). Marine phosphate availability and the chemical origins of life on earth. Nat. Commun. 13, 1–9. doi: 10.1038/s41467-022-32815-x
Branscomb, E., Biancalani, T., Goldenfeld, N., and Russell, M. (2017). Escapement mechanisms and the conversion of disequilibria; the engines of creation. Phys. Rep. 677, 1–60. doi: 10.1016/j.physrep.2017.02.001
Branscomb, E., and Russell, M. J. (2013). Turnstiles and bifurcators: the disequilibrium converting engines that put metabolism on the road. Biochim. Biophys. Acta Bioenerg. 1827, 62–78. doi: 10.1016/j.bbabio.2012.10.003
Branscomb, E., and Russell, M. J. (2018). Frankenstein or a submarine alkaline vent: who is responsible for abiogenesis? Part 2: as life is now, so it must have been in the beginning. BioEssays 40:1700182. doi: 10.1002/bies.201700182
Branscomb, E., and Russell, M. J. (2019). On the beneficent thickness of water. Interface focus 9:20190061. doi: 10.1098/rsfs.2019.0061
Brazelton, W. J., Thornton, C. N., Hyer, A., Twing, K. I., Longino, A. A., Lang, S. Q., et al. (2017). Metagenomic identification of active methanogens and methanotrophs in serpentinite springs of the Voltri massif, Italy. Peer J 5:e2945. doi: 10.7717/peerj.2945
Bromberg, Y., Aptekmann, A. A., Mahlich, Y., Cook, L., Senn, S., Miller, M., et al. (2022). Quantifying structural relationships of metal-binding sites suggests origins of biological electron transfer. Sci. Adv. 8:eabj3984. doi: 10.1126/sciadv.abj3984
Buessecker, S., Imanaka, H., Ely, T., Hu, R., Romaniello, S. J., and Cadillo-Quiroz, H. (2022). Mineral-catalysed formation of marine NO and N2O on the anoxic early earth. Nat. Geosci. 15, 1056–1063. doi: 10.1038/s41561-022-01089-9
Carey, L. D., Koshak, W., Peterson, H., and Mecikalski, R. M. (2016). The kinematic and microphysical control of lightning rate, extent, and NOX production. J. Geophys. Res. Atmosph. 121, 7975–7989. doi: 10.1002/2015JD024703
Carroll, S. (2016). The Big Picture on the Origins of Life, Meaning, and the Universe Itself. Dutton, New York, NY.
Carter, C. W. (2020). Escapement mechanisms: efficient free energy transduction by reciprocally-coupled gating. Proteins: Struct. Funct. Bioinf. 88, 710–717. doi: 10.1002/prot.25856
Cartwright, J. H., García-Ruiz, J. M., Novella, M. L., and Otálora, F. (2002). Formation of chemical gardens. J. Colloid Interf. Sci. 256, 351–359. doi: 10.1006/jcis.2002.8620
Cartwright, J. H., and Russell, M. J. (2019). The origin of life: the submarine alkaline vent theory at 30. Interface Focus 9:20190104. doi: 10.1098/rsfs.2019.0104
Cathles, L. M. (1990). Scales and effects of fluid flow in the upper crust. Science 248, 323–329. doi: 10.1126/science.248.4953.323
Chernoff, Y. O. (2004). Amyloidogenic domains, prions and structural inheritance: rudiments of early life or recent acquisition? Curr. Opin. Chem. Biol. 8, 665–671. doi: 10.1016/j.cbpa.2004.09.002
Childers, W. S., Mehta, A. K., Ni, R., Taylor, J. V., and Lynn, D. G. (2010). Peptides organized as bilayer membranes. Angew. Chemie. 122, 4198–4201. doi: 10.1002/ange.201000212
Childers, W. S., Ni, R., Mehta, A. K., and Lynn, D. G. (2009). Peptide membranes in chemical evolution. Curr. Opin. Chem. Biol. 13, 652–659. doi: 10.1016/j.cbpa.2009.09.027
Chin, K., Pasalic, J., Hermis, N., and Barge, L. M. (2020). Chemical gardens as electrochemical systems: in situ characterization of simulated prebiotic hydrothermal vents by impedance spectroscopy. ChemPlusChem 85, 2619–2628. doi: 10.1002/cplu.202000600
Coca, J. R., Erana, H., and Castilla, J. (2021). Biosemiotics comprehension of PrP code and prion disease. Biosystems 210:104542. doi: 10.1016/j.biosystems.2021.104542
Colman, D. R., Kraus, E. A., Thieringer, P. H., Rempfert, K., Templeton, A. S., Spear, J. R., et al. (2022). Deep-branching acetogens in serpentinized subsurface fluids of Oman. Proc. Natl. Acad. Sci. U. S. A. 119:e2206845119. doi: 10.1073/pnas.2206845119
Corliss, J. B. (1971). The origin of metal-bearing submarine hydrothermal solutions. J. Geophys. Res. 76, 8128–8138. doi: 10.1029/JC076i033p08128
Corliss, J. B., Baross, J. A., and Hoffman, S. E. (1980). Submarine Hydrothermal Systems—A Probable Site for the Origin of Life. Corvallis, Oregon State University, School of Oceanography, Spec. Pub. 80–87.
Corliss, J. B., Baross, J. A., and Hoffman, S. E. (1981). An hypothesis concerning the relationship between submarine hot springs and the origin of life on earth. Oceanol. Acta 4, 59–69.
Corliss, J. B., Dymond, J., Gordon, L. I., Edmond, J. M., von Herzen, R. P., Ballard, R. D., et al. (1979). Submarine thermal springs on the Galápagos rift. Science 203, 1073–1083. doi: 10.1126/science.203.4385.1073
Cottrell, A. (1979). The natural philosophy of engines. Contemp. Physics 20, 1–10. doi: 10.1080/00107517908227799
Davis, E., and Becker, K. (1999). Tidal pumping of fluids within and from the oceanic crust: new observations and opportunities for sampling the crustal hydrosphere. Earth Planet. Sci. Lett. 172, 141–149. doi: 10.1016/S0012-821X(99)00197-1
Delbrück, M. (1970). A physicist’s renewed look at biology: twenty years later. Science 168, 1312–1315. doi: 10.1126/science.168.3937.1312
Desvaux, M., and Hébraud, M. (2006). The protein secretion systems in listeria: inside out bacterial virulence. FEMS Microbiol. Rev. 30, 774–805. doi: 10.1111/j.1574-6976.2006.00035.x
Di Giulio, M. (2008). An extension of the coevolution theory of the origin of the genetic code. Biol. Dir. 3:37. doi: 10.1186/1745-6150-3-37
Ding, Y., Batista, B., Steinbock, O., Cartwright, J. H., and Cardoso, S. S. (2016). Wavy membranes and the growth rate of a planar chemical garden: enhanced diffusion and bioenergetics. Proc. Natl. Acad. Sci. U. S. A. 113, 9182–9186. doi: 10.1073/pnas.1607828113
Ducluzeau, A. L., Schoepp-Cothenet, B., Baymann, F., Russell, M. J., and Nitschke, W. (2014). Free energy conversion in the LUCA: quo vadis? Biochim. Biophys. Acta Bioenerg. 1837, 982–988. doi: 10.1016/j.bbabio.2013.12.005
Ducluzeau, A. L., van Lis, R., Duval, S., Schoepp-Cothenet, B., Russell, M. J., and Nitschke, W. (2009). Was nitric oxide the first deep electron sink? Trends Biochem. Sci. 34, 9–15. doi: 10.1016/j.tibs.2008.10.005
Duval, S., Baymann, F., Schoepp-Cothenet, B., Trolard, F., Bourrié, G., Grauby, O., et al. (2019). Fougerite: the not so simple progenitor of the first cells. Interface Focus 9:20190063. doi: 10.1098/rsfs.2019.0063
Duval, S., Branscomb, E., Trolard, F., Bourrié, G., Grauby, O., Heresanu, V., et al. (2020). On the why’s and how’s of clay minerals’ importance in life’s emergence. Appl. Clay Sci. 195:105737. doi: 10.1016/j.clay.2020.105737
Endres, R. G. (2017). Entropy production selects nonequilibrium states in multistable systems. Sci. Rep. 7, 1–13. doi: 10.1038/s41598-017-14485-8
Erastova, V., Degiacomi, M. T., Fraser, D. G., and Greenwell, H. C. (2017). Mineral surface chemistry control for origin of prebiotic peptides. Nat. Commun. 8, 1–9. doi: 10.1038/s41467-017-02248-y
Eschenmoser, A. (1994). Chemistry of potentially prebiological natural products. Orig. Life Evol. Biosph. 24, 389–423. doi: 10.1007/BF01582017
Etaix, L. E., and Orgel, L. E. (1978). Phosphorylation of nucleosides in aqueous solution using trimetaphosphate: formation of nucleoside triphosphates. J. Carbohydr. Nucleosides Nucleotides 5, 91–110.
Fallick, A. E., Ilich, M., and Russell, M. J. (1991). A stable isotope study of the magnesite deposits associated with the alpine-type ultramafic rocks of Yugoslavia. Econ. Geol. 86, 847–861. doi: 10.2113/gsecongeo.86.4.847
Farr, O., Elzinga, E. J., and Yee, N. (2022). Effect of Ni2+, Zn2+, and Co2+ on green rust transformation to magnetite. Geochem. Trans. 23:3. doi: 10.1186/s12932-022-00080-y
Feng, Y., Ovalle, M., Seale, J. S., Lee, C. K., Kim, D. J., Astumian, R. D., et al. (2021). Molecular pumps and motors. J. Am. Chem. Soc. 143, 5569–5591. doi: 10.1021/jacs.0c13388
Fischbach, M. A., and Walsh, C. T. (2006). Assembly-line enzymology for polyketide and nonribosomal peptide antibiotics: logic, machinery, and mechanisms. Chem. Rev. 106, 3468–3496. doi: 10.1021/cr0503097
Fischer, E. (1894). Einfluss der Configuration auf die Wirkung der Enzyme. Ber. Dtsch. Chem. Ges. 27, 2985–2993. doi: 10.1002/cber.18940270364
Flores, E., Martinez, E., Rodriguez, L. E., Weber, J. M., Khodayari, A., VanderVelde, D. G., et al. (2021). Effects of amino acids on phosphate adsorption onto iron (oxy) hydroxide minerals under early earth conditions. ACS Earth Space Chem. 5, 1048–1057. doi: 10.1021/acsearthspacechem.1c00006
Fones, E. M., Colman, D. R., Kraus, E. A., Nothaft, D. B., Poudel, S., Rempfert, K. R., et al. (2019). Physiological adaptations to serpentinization in the Samail ophiolite, Oman. ISME J. 13, 1750–1762. doi: 10.1038/s41396-019-0391-2
Fones, E. M., Colman, D. R., Kraus, E. A., Stepanauskas, R., Templeton, A. S., Spear, J. R., et al. (2021). Diversification of methanogens into hyperalkaline serpentinizing environments through adaptations to minimize oxidant limitation. ISME J. 15, 1121–1135. doi: 10.1038/s41396-020-00838-1
Fracchia, M., Visibile, A., Ahlberg, E., Vertova, A., Minguzzi, A., Ghigna, P., et al. (2018). α-And γ-FeOOH: stability, reversibility, and nature of the active phase under hydrogen evolution. ACS Appl. Energ. Mater. 1, 1716–1725. doi: 10.1021/acsaem.8b00209
Früh-Green, G. L., Kelley, D. S., Bernasconi, S. M., Karson, J. A., Ludwig, K. A., Butterfield, D. A., et al. (2003). 30,000 years of hydrothermal activity at the lost City vent field. Science 301, 495–498. doi: 10.1126/science.1085582
Fujioka, K., Yamanaka, T., Gamo, T., Inagaki, F., Miwa, T., Sato, H., et al. (2002). Serpentines as a capsule of the deep subsurface biosphere: evidence from the Chamorro seamount, Mariana forearc. JAMSTECH J. Deep Sea Res. 20, 1–16.
Galimov, E. M. (2014). Life is a product of molecular ordering “machine”. Geochem. Int. 52, 1190–1196. doi: 10.1134/S0016702914130059
Gallardo, L., and Rodhe, H. (1997). Oxidized nitrogen in the remote Pacific: the role of electrical discharges over the oceans. J. Atmos. Chem. 26, 147–168. doi: 10.1023/A:1005738402496
Gamow, G. (1954). Lock and key. Possible relation between deoxyribonucleic acid and protein structures. Nature 173:318. doi: 10.1038/173318a0
Gamow, G., and Yčas, M. (1955). Statistical correlation of protein and ribonucleic acid composition. Proc. Natl. Acad. Sci. U. S. A. 41, 1011–1019. doi: 10.1073/pnas.41.12.1011
Gatenby, R. A., and Frieden, B. R. (2017). Cellular information dynamics through transmembrane flow of ions. Sci. Rep. 7:15075. doi: 10.1038/s41598-017-15182-2
Génin, J.-M. R., Aïssa, R., Géhin, A., Abdelmoula, M., Benali, O., Ernstsen, V., et al. (2005). Fougerite and FeII–III hydroxycarbonate green rust; ordering, deprotonation and/or cation substitution; structure of hydrotalcite-like compounds and mythic ferrosic hydroxide Fe(OH)(2+x). Solid State Sci. 7, 545–572. doi: 10.1016/j.solidstatesciences.2005.02.001
Génin, J.-M. R., Renard, A., and Ruby, C. (2008). Fougérite FeII-III oxyhydroxycarbonate in environmental chemistry and nitrate reduction. Hyperfine Interac. 186, 31–37. doi: 10.1007/s10751-008-9837-z
Génin, J.-M. R., Ruby, C., and Upadhyay, C. (2006). Structure and thermodynamics of ferrous, stoichiometric and ferric oxyhydroxycarbonate green rusts; redox flexibility and fougerite mineral. Solid State Sci. 8, 1330–1343. doi: 10.1016/j.solidstatesciences.2006.05.010
Gerbois, D., Ona-Nguema, G., Morin, G., Abdelmoula, M., Laverman, A. M., Mouchel, J.-M., et al. (2014). Nitrite reduction by biogenic hydrocarbonate Green rusts: evidence for hydroxy-nitrite Green rust formation as an intermediate reaction product. Environ. Sci. Technol. 48, 4505–4514. doi: 10.1021/es404009k
Glasby, G. P. (2006). Abiogenic origin of hydrocarbons: an historical overview. Resour. Geol. 56, 83–96. doi: 10.1111/j.1751-3928.2006.tb00271.x
Glasby, G. P., and Kasahara, J. (2001). Influence of tidal effects on the periodicity of earthquake activity in diverse geological settings with particular emphasis on submarine hydrothermal systems. Earth-Sci. Rev. 52, 261–297. doi: 10.1016/S0012-8252(00)00031-3
Gonzalez, D. L., Giannerini, S., and Rosa, R. (2012). On the origin of the mitochondrial genetic code: towards a unified mathematical framework for the management of genetic information. Nat. Prec. 7:1. doi: 10.1038/npre.2012.7136.1
Gonzalez, D. L., Giannerini, S., and Rosa, R. (2019). On the origin of degeneracy in the genetic code. Interface Focus 9:20190038. doi: 10.1098/rsfs.2019.0038
Goodwin, J. T., Mehta, A. K., and Lynn, D. G. (2012). Digital and analog chemical evolution. Acc. Chem. Res. 45, 2189–2199. doi: 10.1021/ar300214w
Greenwald, J., Kwiatkowski, W., and Riek, R. (2018). Peptide amyloids in the origin of life. J. Mol. Biol. 430, 3735–3750. doi: 10.1016/j.jmb.2018.05.046
Greenwald, J., and Riek, R. (2010). Biology of amyloid: structure, function, and regulation. Struct. 18, 1244–1260. doi: 10.1016/j.str.2010.08.009
Greenwell, H. C., and Coveney, P. V. (2006). Layered double hydroxide minerals as possible prebiotic information storage and transfer compounds. Orig. Life Evol. Biosph. 36, 13–37. doi: 10.1007/s11084-005-2068-2
Grégoire, B., Erastova, V., Geatches, D. L., Clark, S. J., Greenwell, H. C., and Fraser, D. G. (2016). Insights into the behaviour of biomolecules on the early earth: the concentration of aspartate by layered double hydroxide minerals. Geochim. Cosmochim. Acta 176, 239–258. doi: 10.1016/j.gca.2015.12.026
Grégoire, B., Greenwell, H. C., and Fraser, D. G. (2018). Peptide formation on layered mineral surfaces: the key role of brucite-like minerals on the enhanced formation of alanine dipeptides. ACS Earth Space Chem. 2, 852–862. doi: 10.1021/acsearthspacechem.8b00052
Gribov, L. A., Baranov, V. I., and Mikhailov, I. V. (2021). Some basic statements of the general theory of the universe evolution at the first stages of life. Geochem. Int. 59, 1106–1112. doi: 10.1134/S0016702921110021
Hafenbradl, D., Keller, M., Wächtershäuser, G., and Stetter, K. O. (1995). Primordial amino acids by reductive amination of α-oxo acids in conjunction with the oxidative formation of pyrite. Tetrahed. Lett. 36, 5179–5182. doi: 10.1016/00404-0399(50)10086-
Hagmann, M. (2002). Between a rock and a hard place. Science 295, 2006–2007. doi: 10.1126/science.295.5562.2006
Halevy, I., Alesker, M., Schuster, E. M., Popovitz-Biro, R., and Feldman, Y. (2017). A key role for green rust in the Precambrian oceans and the genesis of iron formations. Nat. Geosci. 10, 135–139. doi: 10.1038/ngeo2878
Hansen, H. C., Guldberg, S., Erbs, M., and Koch, C. B. (2001). Kinetics of nitrate reduction by green rusts—effects of interlayer anion and Fe (II): Fe (III) ratio. Appl. Clay Sci. 18, 81–91. doi: 10.1016/S0169-1317(00)00029-6
Hansen, H. C., Koch, C. B., Nancke-Krogh, H., Borggaard, O. K., and Sørensen, J. (1996). Abiotic nitrate reduction to ammonium: key role of green rust. Environ. Sci. Technol. 30, 2053–2056. doi: 10.1021/es950844w
Harrison, S. A., and Lane, N. (2018). Life as a guide to prebiotic nucleotide synthesis. Nat. Commun. 9:5176. doi: 10.1038/s41467-018-07220-y
Harrison, S. A., Palmeira, R. N., Halpern, A., and Lane, N. (2022). A biophysical basis for the emergence of the genetic code in protocells. Biochim. Biophys. Acta Bioenerg. 1863:148597. doi: 10.1016/j.bbabio.2022.148597
Hartman, H. (1975). Speculations on the origin and evolution of metabolism. J. Mol. Evol. 4, 359–370. doi: 10.1007/BF01732537
Hassabis, D., and Revell, T. (2021). With AI, you might unlock some of the secrets about how life works. New Scientist 249, 44–49. doi: 10.1016/S0262-4079(20)32269-7
Hayward, S., and Milner-White, E. J. (2008). The geometry of α-sheet: implications for its possible function as amyloid precursor in proteins. Proteins: Struct. Funct. Bioinform. 71, 415–425. doi: 10.1002/prot.21717
Hayward, S., and Milner-White, E. J. (2021). Determination of amino acids that favour the αL region using Ramachandran propensity plots. Implications for α-sheet as the possible amyloid intermediate. J. Struct. Biol. 213:107738. doi: 10.1016/j.jsb.2021.107738
Heays, A. N., Kaiserová, T., Rimmer, P. B., Knížek, A., Petera, L., Civiš, S., et al. (2022). Nitrogen oxide production in laser-induced breakdown simulating impacts on the hadean atmosphere. J. Geophys. Res. Planets 127:e2021JE006842. doi: 10.1029/2021JE006842
Hedderich, R. (2004). Energy-converting [NiFe] hydrogenases from archaea and extremophiles: ancestors of complex I. J. Bioenerg. Biomembr. 36, 65–75. doi: 10.1023/B:JOBB.0000019599.43969.33
Heinen, W., and Lauwers, A. M. (1996). Organic sulfur compounds resulting from the interaction of iron sulfide, hydrogen sulfide and carbon dioxide in an anaerobic aqueous environment. Orig. Life Evol. Biosph. 26, 131–150. doi: 10.1007/BF01809852
Heller, R., Duda, J. P., Winkler, M., Reitner, J., and Gizon, L. (2021). Habitability of the early earth: liquid water under a faint young Sun facilitated by strong tidal heating due to a closer moon. Paläontol. Z. 95, 563–575. doi: 10.1007/s12542-021-00582-7
Helmbrecht, V., Weingart, M., Klein, F., Braun, D., and Orsi, W. D. (2022). White and green rust chimneys accumulate RNA in a ferruginous chemical garden. arXiv preprint arXiv:2212.02793 [Epub ahead of preprint].
Hitchcock, D. R., and Lovelock, J. E. (1967). Life detection by atmospheric analysis. Icarus 7, 149–159. doi: 10.1016/0019-1035(67)90059-0
Holden, D. T., Morato, N. M., and Cooks, R. G. (2022). Aqueous microdroplets enable abiotic synthesis and chain extension of unique peptide isomers from free amino acids. Proc. Natl. Acad. Sci. U. S. A. 119:e2212642119. doi: 10.1073/pnas.2212642119
Hooks, M. R., Webster, P., Weber, J. M., Perl, S., and Barge, L. M. (2020). Effects of amino acids on iron-silicate chemical garden precipitation. Langmuir 36, 5793–5801. doi: 10.1021/acs.langmuir.0c00502
Horowitz, J. M., and Gingrich, T. R. (2020). Thermodynamic uncertainty relations constrain non-equilibrium fluctuations. Nat. Phys. 16, 15–20. doi: 10.1038/s41567-019-0702-6
Huang, X. L. (2019). “Iron oxide nanoparticles: an inorganic phosphatase” in Nanocatalysts. eds. I. Sinha and M. Shukla (London: IntechOpen), 97–124.
Huang, X. L. (2022). What are inorganic nanozymes? Artificial or inorganic enzymes. New J. Chem. 46, 15273–15291. doi: 10.1039/D2NJ02088B
Huber, C., Eisenreich, W., Hecht, S., and Wächtershäuser, G. (2003). A possible primordial peptide cycle. Science 301, 938–940. doi: 10.1126/science.1086501
Huber, C., and Wächtershäuser, G. (1997). Activated acetic acid by carbon fixation on (Fe, Ni)S under primordial conditions. Science 276, 245–247. doi: 10.1126/science.276.5310.245
Huber, C., and Wächtershäuser, G. (1998). Peptides by activation of amino acids with CO on (Ni,Fe)S. Science 281, 670–672. doi: 10.1126/science.281.5377.670
Hudson, R., de Graaf, R., Rodin, M. S., Ohno, A., Lane, N., McGlynn, S. E., et al. (2020). CO2 reduction driven by a pH gradient. Proc. Natl. Acad. Sci. U. S. A. 117, 22873–22879. doi: 10.1073/pnas.2002659117
Igamberdiev, A. U. (2021). The drawbridge of nature: evolutionary complexification as a generation and novel interpretation of coding systems. Biosystems 207:104454. doi: 10.1016/j.biosystems.2021.104454
Javoy, M., and Pineau, F. (1991). The volatiles record of a “popping” rock from the mid-Atlantic ridge at 14 N: chemical and isotopic composition of gas trapped in the vesicles. Earth Planet. Sci. Lett. 107, 598–611. doi: 10.1016/0012-821X(91)90104-P
Jheeta, S., Chatzitheodoridis, E., Devine, K., and Block, J. (2021). The way forward for the origin of life: prions and prion-like molecules first hypothesis. Life 11:872. doi: 10.3390/life11090872
Kaila, V. R. K. (2021). Resolving chemical dynamics in biological energy conversion: long-range proton-coupled electron transfer in respiratory complex I. Acc. Chem. Res. 54, 4462–4473. doi: 10.1021/acs.accounts.1c00524
Kampschreur, M. J., Kleerebezem, R., de Vet, W. W., and van Loosdrecht, M. C. (2011). Reduced iron induced nitric oxide and nitrous oxide emission. Water Res. 45, 5945–5952. doi: 10.1016/j.watres.2011.08.056
Kandemir, B., Kubie, L., Guo, Y., Sheldon, B., and Bren, K. L. (2016). Hydrogen evolution from water under aerobic conditions catalyzed by a cobalt ATCUN metallopeptide. Inorg. Chem. 55, 1355–1357. doi: 10.1021/acs.inorgchem.5b02157
Kandler, O., and König, H. (1998). Cell wall polymers in archaea (Archaebacteria). Cellul. Mol. Life Sci. 54, 305–308. doi: 10.1007/s000180050156
Kasting, J. F., and Siefert, J. L. (2001). The nitrogen fix. Nature 412, 26–27. doi: 10.1038/35083660
Kelley, D. S. (1996). Methane-rich fluids in the oceanic crust. J. Geophys. Res.-solid Earth 101, 2943–2962.
Kelley, D. S., Karson, J. A., Blackman, D. K., Früh-Green, G. L., Butterfield, D. A., Lilley, M. D., et al. (2001). An off-axis hydrothermal vent field near the mid-Atlantic ridge at 30 N. Nature 412, 145–149. doi: 10.1038/35084000
Kitadai, N., Nakamura, R., Yamamoto, M., Takai, K., Li, Y., Yamaguchi, A., et al. (2018). Geoelectrochemical CO production: implications for the autotrophic origin of life. Sci. Adv. 4:eaao7265. doi: 10.1126/sciadv.aao7265
Kleinkauf, H., and von Döhren, H. (1996). A nonribosomal system of peptide biosynthesis. Eur. J. Biochem. 236, 335–351. doi: 10.1111/j.1432-1033.1996.00335.x
Kohl, L., Cumming, E., Cox, A., Rietze, A., Morrissey, L., Lang, S. Q., et al. (2016). Exploring the metabolic potential of microbial communities in ultra-basic, reducing springs at the cedars, CA, USA: experimental evidence of microbial methanogenesis and heterotrophic acetogenesis. J. Geophys. Res. Biogeosci. 121, 1203–1220. doi: 10.1002/2015JG003233
Kolb, V., Zhang, S., Xu, Y., and Arrhenius, G. (1997). Mineral induced phosphorylation of glycolate ion–a metaphor in chemical evolution. Orig. Life Evol. Biosph. 27, 485–503. doi: 10.1023/A:1006582526535
Konhauser, K. O., Lalonde, S. V., Amskold, L., and Holland, H. D. (2007). Was there really an Archean phosphate crisis? Science 315, 1234–2007. doi: 10.1126/science.1136328
Kordium, V. A. (2021). Defining life and evolution: essay on the origin, expansion, and evolution of living matter. Biosystems 209:104500. doi: 10.1016/j.biosystems.2021.104500
Kosolapova, A. O., Antonets, K. S., Belousov, M. V., and Nizhnikov, A. A. (2020). Biological functions of prokaryotic amyloids in interspecies interactions: facts and assumptions. Intern. J. Mol. Sci. 21:7240. doi: 10.3390/ijms21197240
Krishnamurthy, R., Arrhenius, G., and Eschenmoser, A. (1999). Formation of glycolaldehyde phosphate from glycolaldehyde in aqueous solution. Orig. Life Evol. Biosph. 29, 333–354. doi: 10.1023/A:1006698208873
Krishnamurthy, R., Pitsch, S., and Arrhenius, G. (1996). Mineral induced synthesis of ribose phosphates. Orig. Life Evol. Biosph. 26, 240–241. doi: 10.1007/BF02459734
Kuma, K., Paplawsky, W., Gedulin, B., and Arrhenius, G. (1989). Mixed-valence hydroxides as bioorganic host minerals. Orig. Life Evol. Biosph. 19, 573–601. doi: 10.1007/BF01808119
Kurland, C. G. (2010). The RNA dreamtime: modern cells feature proteins that might have supported a prebiotic polypeptide world but nothing indicates that RNA world ever was. BioEssays 32, 866–871. doi: 10.1002/bies.201000058
Lahav, N. (1985). The synthesis of primitive ‘living’ forms: definitions, goals, strategies and evolution synthesizers. Orig. Life Evol. Biosph. 16, 129–149. doi: 10.1007/BF01809467
Lane, N. (2022). Transformer: The Deep Chemistry of Life and Death. W. W. Norton & Company, New York, NY.
Lane, N., Allen, J. F., and Martin, W. (2010). How did LUCA make a living? Chemiosmosis in the origin of life. BioEssays 32, 271–280. doi: 10.1002/bies.200900131
Larsen, P., Nielsen, J. L., Dueholm, M. S., Wetzel, R., Otzen, D., and Nielsen, P. H. (2007). Amyloid adhesins are abundant in natural biofilms. Environ. Microbiol. 9, 3077–3090. doi: 10.1111/j.1462-2920.2007.01418.x
Larter, R. C., Boyce, A. J., and Russell, M. J. (1981). Hydrothermal pyrite chimneys from the Ballynoe baryte deposit, Silvermines, county Tipperary, Ireland. Miner Depos 16, 309–318. doi: 10.1007/BF00202742
Leong, J. A., Howells, A. E., Robinson, K. J., Cox, A., Debes, R. V., Fecteau, K., et al. (2021). Theoretical predictions versus environmental observations on serpentinization fluids: lessons from the Samail ophiolite in Oman. J. Geophys. Res. Solid Earth 126:e2020JB020756. doi: 10.1029/2020JB020756
Levitt, D. (2023). What’s Gotten Into You: The Story of Your Body’s Atoms, From the Big Bang Through Last Night’s Dinner. New York: HarperCollins.
Levy, M., and Ellington, A. D. (2003). Peptide-templated nucleic acid ligation. J. Mol. Evol. 56, 607–615. doi: 10.1007/s00239-002-2429-7
Li, J., Browning, S., Mahal, S. P., Oelschlegel, A. M., and Weissmann, C. (2010). Darwinian evolution of prions in cell culture. Science 327, 869–872. doi: 10.1126/science.1183218
Li, K. M., Wilkinson, C., Kellosalo, J., Tsai, J. Y., Kajander, T., Jeuken, L. J., et al. (2016). Membrane pyrophosphatases from Thermotoga maritima and Vigna radiata suggest a conserved coupling mechanism. Nat. Commun. 7, 1–11. doi: 10.1038/ncomms13596
Lin, S. M., Tsai, J. Y., Hsiao, C. D., Huang, Y. T., Chiu, C. L., Liu, M. H., et al. (2012). Crystal structure of a membrane-embedded H+-translocating pyrophosphatase. Nature 484, 399–403. doi: 10.1038/nature10963
Lowell, R. P., and Rona, P. A. (2002). Seafloor hydrothermal systems driven by serpentinization of peridotite. Geophys. Res. Lett. 29:1531. doi: 10.1029/2001GL014411
Ludwig, K. A., Shen, C. C., Kelley, D. S., Cheng, H., and Edwards, R. L. (2011). U–Th systematics and 230Th ages of carbonate chimneys at the lost City hydrothermal field. Geochim. Cosmochim. Acta 75, 1869–1888. doi: 10.1016/j.gca.2011.01.008
Lupi, O., Dadalti, P., Cruz, E., and Sanberg, P. R. (2006). Are prions related to the emergence of early life? Med. Hyp. 67, 1027–1033. doi: 10.1016/j.mehy.2006.04.056
Lyu, Z., Rotaru, A. E., Pimentel, M., Zhang, C. J., and Simon, K. M. R. (2022). The methane moment-cross-boundary significance of methanogens: preface. Front. Microbiol. 13:1055494. doi: 10.3389/fmicb.2022.1055494
Macleod, G., Mckeown, C., Hall, A. J., and Russell, M. J. (1994). Hydrothermal and oceanic pH conditions at 4Ga relevant to the origin of life. Orig. Life Evol. Biosph. 24, 19–41. doi: 10.1007/BF01582037
Maden, B. (2000). Tetrahydrofolate and tetrahydromethanopterin compared: functionally distinct carriers in C1 metabolism. Biochem. J. 350, 609–629. doi: 10.1042/bj3500609
Mancinelli, R. L., and McKay, C. P. (1988). The evolution of nitrogen cycling. Orig. Life Evol. Biosph. 18, 311–325. doi: 10.1007/BF01808213
Mandon, C. L., Christenson, B. W., Schipper, C. I., Seward, T. M., and Garaebiti, E. (2019). Metal transport in volcanic plumes: a case study at White Island and Yasur volcanoes. J. Volc. Geotherm. Res. 369, 155–171. doi: 10.1016/j.jvolgeores.2018.11.024
Marshall, P. (2021). Biology transcends the limits of computation. Progr. Biophys. Mol. Biol. 165, 88–101. doi: 10.1016/j.pbiomolbio.2021.04.006
Martin, W. F. (2020). Older than genes: the acetyl CoA pathway and origins. Front. Microbiol. 11:817. doi: 10.3389/fmicb.2020.00817
Martin, W., and Russell, M. J. (2003). On the origin of cells: an hypothesis for the evolutionary transitions from abiotic geochemistry to chemoautotrophic prokaryotes, and from prokaryotes to nucleated cells. Philos. Trans. R. Soc. Lond. 358, 59–85. doi: 10.1098/rstb.2002.1183
Martin, W., and Russell, M. J. (2007). On the origin of biochemistry at an alkaline hydrothermal vent. Philos. Trans. R. Soc. Lond. 362, 1887–1926. doi: 10.1098/rstb.2006.1881
Martiny, J. B. H., Bohannan, B. J., Brown, J. H., Colwell, R. K., Fuhrman, J. A., Green, J. L., et al. (2006). Microbial biogeography: putting microorganisms on the map. Nat. Rev. Microbiol. 4, 102–112. doi: 10.1038/nrmicro1341
Maury, C. P. (2009). Self-propagating beta-sheet polypeptide structures as prebiotic informational molecular entities: the amyloid world. Orig. Life Evol. Biosph. 39, 141–150. doi: 10.1007/s11084-009-9165-6
Mayer, R. J., Kaur, H., Rauscher, S. A., and Moran, J. (2021). Mechanistic insight into metal ion-catalyzed transamination. J. Am. Chem. Soc. 143, 19099–19111. doi: 10.1021/jacs.1c08535
McGlynn, S. E., Kanik, I., and Russell, M. J. (2012). Peptide and RNA contributions to iron–Sulphur chemical gardens as life’s first inorganic compartments, catalysts, capacitors and condensers. Philos. Trans. R. Soc. Lond. A Phys. Sci. 370, 3007–3022. doi: 10.1098/rsta.2011.0211
McMahon, S. (2019). Earth’s earliest and deepest purported fossils may be iron-mineralized chemical gardens. Proc. R. Soc. Lond. B 286:20192410. doi: 10.1098/rspb.2019.2410
Mellersh, A. R., and Wilkinson, A.-S. (2000). RNA bound to a solid phase can select an amino acid and facilitate subsequent amide bond formation. Orig. Life Evol. Biosph. 30, 3–7. doi: 10.1023/A:1006620421068
Ménez, B. (2020). Abiotic hydrogen and methane: fuels for life. Elements 16, 39–46. doi: 10.2138/gselements.16.1.39
Merkel, A. Y., Huber, J. A., Chernyh, N. A., Bonch-Osmolovskaya, E. A., and Lebedinsky, A. V. (2013). Detection of putatively thermophilic anaerobic methanotrophs in diffuse hydrothermal vent fluids. Appl. Environ. Microb. 79, 915–923. doi: 10.1128/AEM.03034-12
Mielke, R. E., Robinson, K. J., White, L. M., McGlynn, S. E., McEachern, K., Bhartia, R., et al. (2011). Iron-sulfide-bearing chimneys as potential catalytic energy traps at life’s emergence. Astrobiology 11, 933–950. doi: 10.1089/ast.2011.0667
Mielke, R. E., Russell, M. J., Wilson, P. R., McGlynn, S., Coleman, M., Kidd, R., et al. (2010). Design, fabrication and test of a hydrothermal reactor for origin-of-life experiments. Astrobiology 10, 799–810. doi: 10.1089/ast.2009.0456
Miller, S. L., and Bada, J. L. (1988). Submarine hot springs and the origin of life. Nature 334, 609–611. doi: 10.1038/334609a0
Miller, H. M., Matter, J. M., Kelemen, P., Ellison, E. T., Conrad, M. E., Fierer, N., et al. (2016). Modern water/rock reactions in Oman hyperalkaline peridotite aquifers and implications for microbial habitability. Geochim. Cosmochim. Acta 179, 217–241. doi: 10.1016/j.gca.2016.01.033
Milner-White, E. J. (2019). Protein three-dimensional structures at the origin of life. Interface Focus 9:20190057. doi: 10.1098/rsfs.2019.0057
Milner-White, E. J., and Russell, M. J. (2005). Sites for phosphates and iron-sulfur thiolates in the first membranes: 3 to 6 residue anion-binding motifs (nests): Orig. Life Evol. Biosph. 35, 19–27. doi: 10.1007/s11084-005-4582-7
Milner-White, E. J., and Russell, M. J. (2008). Predicting the conformations of proteins and peptides in early evolution. Biol Dir. 3:3. doi: 10.1186/1745-6150-3-3
Milner-White, E. J., and Russell, M. J. (2011). A peptide era heralding the emergence of life. Genes 2, 671–688. doi: 10.3390/genes2040671
Milner-White, E. J., Watson, J. D., Qi, G., and Hayward, S. (2006). Amyloid formation may involve α- to β-sheet interconversion via peptide plane flipping. Structure 14, 1369–1376. doi: 10.1016/j.str.2006.06.016
Mitchell, P. (1959). The origin of life and the formation and organizing functions of natural membranes. In: Proceedings of the First International Symposium on the Origin of Life on the Earth, A. I. Oparin, A.G. Pasynskiĭ, A.E. Brauntsteĭn and T.E. Pavlovskaya (Eds.). Moscow: House Academy of Science USSR, 1957; English Edition edited by F. Clark, and R.L.M. Synge, Pergamon Press, New York, 437–443.
Mitchell, P. (1961). Coupling of phosphorylation to electron and hydrogen transfer by a chemi-osmotic type of mechanism. Nature 191, 144–148. doi: 10.1038/191144a0
Mloszewska, A. M., Pecoits, E., Cates, N. L., Mojzsis, S. J., O’Neil, J., Robbins, L. J., et al. (2012). The composition of Earth’s oldest iron formations: the Nuvvuagittuq Supracrustal Belt (Québec, Canada). Earth Planet. Sci. Lett. 317-318, 331–342. doi: 10.1016/j.epsl.2011.11.020
Muchowska, K. B., Varma, S. J., and Moran, J. (2019). Synthesis and breakdown of universal metabolic precursors promoted by iron. Nature 569, 104–107. doi: 10.1038/s41586-019-1151-1
Muchowska, K. B., Varma, S. J., and Moran, J. (2020). Nonenzymatic metabolic reactions and life’s origins. Chem. Rev. 120, 7708–7744. doi: 10.1021/acs.chemrev.0c00191
Müller, F., Escobar, L., Xu, F., Węgrzyn, E., Nainytė, M., Amatov, T., et al. (2022). A prebiotically plausible scenario of an RNA–peptide world. Nature 605, 279–284. doi: 10.1038/s41586-022-04676-3
Muñoz-Santiburcio, D., and Marx, D. (2017). Chemistry in nanoconfined water. Chem. Sci. 8, 3444–3452. doi: 10.1039/C6SC04989C
Navarro-González, R., Navarro, K. F., Coll, P., McKay, C. P., Stern, J. C., Sutter, B., et al. (2019). Abiotic input of fixed nitrogen by bolide impacts to Gale crater during the Hesperian: insights from the Mars science laboratory. J. Geophys. Res. Planets 124, 94–113. doi: 10.1029/2018JE005852
Neal, C., and Stanger, G. (1983). Hydrogen generation from mantle source rocks in Oman. Earth Planet. Sci. Lett. 66, 315–320. doi: 10.1016/0012-821X(83)90144-9
Neal, C., and Stanger, G. (1984). Calcium and magnesium hydroxide precipitation from alkaline groundwaters in Oman, and their significance to the process of serpentinization. Mineral. Mag. 48, 237–241. doi: 10.1180/minmag.1984.048.347.07
Nirenberg, M., and Leder, P. (1964). RNA codewords and protein synthesis. I. the effect of trinucleotides upon the binding of sRNA to ribosomes. Science 145, 1399–1407. doi: 10.1126/science.145.3639.1399
Nishizawa, M. (2022). Abiotic path of Archean nitrogen. Nat. Geosci. 15, 962–963. doi: 10.1038/s41561-022-01072-4
Nitschke, W., and Russell, M. J. (2009). Hydrothermal focusing of chemical and chemiosmotic energy, supported by delivery of catalytic Fe, Ni, Mo/W, co, S and se, forced life to emerge. J. Mol. Evol. 69, 481–496. doi: 10.1007/s00239-009-9289-3
Nitschke, W., and Russell, M. J. (2010). Just like the universe the emergence of life had high enthalpy and low entropy beginnings. J. Cosmol. 10, 3200–3216.
Nitschke, W., and Russell, M. J. (2012). Redox bifurcations: mechanisms and importance to life now, and at its origin: a widespread means of energy conversion in biology unfolds…. BioEssays 34, 106–109. doi: 10.1002/bies.201100134
Nitschke, W., and Russell, M. J. (2013). Beating the acetyl coenzyme-a pathway to the origin of life. Philos. Trans. R. Soc. Lond. Ser. B Biol. Sci. 368:20120258. doi: 10.1098/rstb.2012.0258
Nitschke, W., Schoepp-Cothenet, B., Duval, S., Zuchan, K., Farr, O., Baymann, F., et al. (2022). Aqueous electrochemistry; the toolbox for life’s emergence from redox disequilibria. Electrochem. Sci. Adv. :e2100192. doi: 10.1002/elsa.202100192
Nothaft, D. B., Templeton, A. S., Rhim, J. H., Wang, D. T., Labidi, J., Miller, H. M., et al. (2021). Geochemical, biological, and clumped isotopologue evidence for substantial microbial methane production under carbon limitation in serpentinites of the Samail ophiolite. Oman. J. Geophys. Res: Biogeosci. 126:e2020JG006025. doi: 10.1029/2020JG006025
Oda, A., and Fukuyoshi, S. (2015). Predicting three-dimensional conformations of peptides constructed of only glycine, alanine, aspartic acid, and valine. Orig. Life Evol. Biosph. 45, 183–193. doi: 10.1007/s11084-015-9418-5
Ooka, H., McGlynn, S. E., and Nakamura, R. (2019). Electrochemistry at deep‐sea hydrothermal vents: utilization of the thermodynamic driving force towards the autotrophic origin of life. Chem. Electro. Chem. 6, 1316–1323. doi: 10.1002/celc.201801432
Palmeira, R. N., Colnaghi, M., Harrison, S. A., Pomiankowski, A., and Lane, N. (2022). The limits of metabolic heredity in protocells. Proc. Roy. Soc. B. 289:20221469. doi: 10.1098/rspb.2022.1469
Papineau, D., De Gregorio, B. T., Cody, G. D., O’Neil, J., Steele, A., Stroud, R. M., et al. (2011). Young poorly crystalline graphite in the >3.8-Gyr-old Nuvvuagittuq banded iron formation. Nat. Geosci. 4, 376–379. doi: 10.1038/ngeo1155
Parkes, R. J., Cragg, B. A., Bale, S. J., Getlifff, J. M., Goodman, K., Rochelle, P. A., et al. (1994). Deep bacterial biosphere in Pacific Ocean sediments. Nature 371, 410–413. doi: 10.1038/371410a0
Parkes, R. J., and Wellsbury, P. (2004). “Deep biospheres” in Microbial Diversity and Bioprospecting. ed. A. T. Bull (Washington, DC: ASM Press), 120–129.
Paukert, A. N., Matter, J. M., Kelemen, P. B., Shock, E. L., and Havig, J. R. (2012). Reaction path modeling of enhanced in situ CO2 mineralization for carbon sequestration in the peridotite of the Samail ophiolite, Sultanate of Oman. Chem. Geol. 330-331, 86–100. doi: 10.1016/j.chemgeo.2012.08.013
Pauling, L. (1946). Molecular architecture and biological reactions. Chem. Eng. News 24, 1375–1377. doi: 10.1021/cen-v024n010.p1375
Pedersen, K. (1993). The deep subterranean biosphere. Earth-Sci. Rev. 34, 243–260. doi: 10.1016/0012-8252(93)90058-F
Peretó, J., Velasco, A. M., Becerra, A., and Lezcano, A. (1999). Comparative biochemistry of CO2 fixation and the evolution of autotrophy. Internatl. Microbiol. 2, 3–10.
Perret, C. J. (1960). A new kinetic model of a growing bacterial population. Microbiol. 22, 589–617. doi: 10.1099/00221287-22-3-589
Pinna, S., Kunz, C., Halpern, A., Harrison, S. A., Jordan, S. F., Ward, J., et al. (2022). A prebiotic basis for ATP as the universal energy currency. PLoS Biol. 20:e3001437. doi: 10.1371/journal.pbio.3001437
Pitsch, S., Eschenmoser, A., Gedulin, B., Hui, S., and Arrhenius, G. (1995). Mineral induced formation of sugar phosphates. Orig. Life Evol. Biosph. 25, 297–334. doi: 10.1007/BF01581773
Pons, M. L., Quitté, G., Fujii, T., Rosing, M. T., Reynard, B., Moynier, F., et al. (2011). Early Archean serpentine mud volcanoes at Isua, Greenland, as a niche for early life. Proc. Natl. Acad. Sci. U. S. A. 108, 17639–17643. doi: 10.1073/pnas.1108061108
Popov, E. M. (1999). Protein folding as a nonlinear nonequilibrium thermodynamic process. IUBMB Life 47, 443–453. doi: 10.1080/15216549900201473
Postec, A., Quéméneur, M., Bes, M., Mei, N., Benaïssa, F., Payri, C., et al. (2015). Microbial diversity in a submarine carbonate edifice from the serpentinizing hydrothermal system of the Prony Bay (New Caledonia) over a 6-year period. Front. Microbiol. 6:857. doi: 10.3389/fmicb.2015.00857
Proskurowski, G., Lilley, M. D., Kelley, D. S., and Olson, E. J. (2006). Low temperature volatile production at the lost city hydrothermal field, evidence from a hydrogen stable isotope geothermometer. Chem. Geol. 229, 331–343. doi: 10.1016/j.chemgeo.2005.11.005
Prusiner, S. B. (1998). Nobel lecture: prions. Proc. Natl. Acad. Sci. U. S. A. 95, 13363–13383. doi: 10.1073/pnas.95.23.13363
Quispel, A. (1998). Lourens GM Baas Becking (1895–1963), inspirator for many (micro)biologists. Int. Microbiol. 1, 69–72.
Ramstead, M. J., Constant, A., Badcock, P. B., and Friston, K. J. (2019). Variational ecology and the physics of sentient systems. Phys Life Rev 31, 188–205. doi: 10.1016/j.plrev.2018.12.002
Ranjan, S., Todd, Z. R., Rimmer, P. B., Sasselov, D. D., and Babbin, A. R. (2019). Nitrogen oxide concentrations in natural waters on early earth. Geochem. Geophys. Geosyst. 20, 2021–2039. doi: 10.1029/2018GC008082
Rasmussen, B., Muhling, J. R., and Fischer, W. W. (2021). Greenalite nanoparticles in alkaline vent plumes as templates for the origin of life. Astrobiology 21, 246–259. doi: 10.1089/ast.2020.2270
Raza, A. (2019). The First Cell: And the Human Costs of Pursuing Cancer to the Last. New York: Basic Books.
Rempfert, K. R., Miller, H. M., Bompard, N., Nothaft, D., Matter, J. M., Kelemen, P., et al. (2017). Geological and geochemical controls on subsurface microbial life in the Samail ophiolite. Oman. Front. Microbiol. 8:56. doi: 10.3389/fmicb.2017.00056
Rimola, A., Balucani, N., Ceccarelli, C., and Ugliengo, P. (2022). Tracing the primordial chemical life of glycine: a review from quantum chemical simulations. Internl J. Mol. Sci. 23:4252. doi: 10.3390/ijms23084252
Rode, B. M. (1999). Peptides and the origin of life 1. Peptides 20, 773–786. doi: 10.1016/S0196-9781(99)00062-5
Römling, U. (2022). Is biofilm formation intrinsic to the origin of life? Environl Microbiol. :16179, 1462–2920. doi: 10.1111/1462-2920.16179
Ruby, C., Abdelmoula, M., Naille, S., Renard, A., Khare, V., Ona-Nguema, G., et al. (2010). Oxidation modes and thermodynamics of FeII–III oxyhydroxycarbonate green rust: dissolution–precipitation versus in situ deprotonation. Geochim. Cosmochim. Acta 74, 953–966. doi: 10.1016/j.gca.2009.10.030
Russell, M. J. (1996). The generation at hot springs of ores, microbialites and life. Ore Geol. Rev. 10, 199–214. doi: 10.1016/0169-1368(95)00023-2
Russell, M. J. (1975). Lithogeochemical environment of the Tynagh base-metal deposit, Ireland, and its bearing on ore deposition. Trans. Instn. Min. Metall. (Appl. Earth Sci.: sect B) 84, B128–133.
Russell, M. J. (2018). Green rust: the simple organizing ‘seed’ of all life? Life 8:35. doi: 10.3390/life8030035
Russell, M. J., and Arndt, N. T. (2005). Geodynamic and metabolic cycles in the hadean. Biogeosciences 2, 97–111. doi: 10.5194/bg-2-97-2005
Russell, M. J., Daniel, R. M., and Hall, A. J. (1993). On the emergence of life via catalytic iron sulphide membranes. Terra Nova 5, 343–347. doi: 10.1111/j.1365-3121.1993.tb00267.x
Russell, M. J., Daniel, R. M., Hall, A. J., and Sherringham, J. (1994). A hydrothermally precipitated catalytic iron sulphide membrane as a first step toward life. J. Mol. Evol. 39, 231–243. doi: 10.1007/BF00160147
Russell, M. J., and Hall, A. J. (1997). The emergence of life from iron monosulphide bubbles at a submarine hydrothermal redox and pH front. J. Geol. Soc. Lond. 154, 377–402. doi: 10.1144/gsjgs.154.3.0377
Russell, M. J., Hall, A. J., Boyce, A. J., and Fallick, A. E. (2005). 100th anniversary special paper: on hydrothermal convection systems and the emergence of life. Econ. Geol. 100, 419–438. doi: 0361-0128/01/3503/419-20
Russell, M. J., Hall, A. J., Cairns-Smith, A. G., and Braterman, P. S. (1988). Submarine hot springs and the origin of life. Nature 336:117. doi: 10.1038/336117a0
Russell, M. J., Hall, A. J., and Mellersh, A. R. (2003). “On the dissipation of thermal and chemical energies on the early earth: the onsets of hydrothermal convection, chemiosmosis, genetically regulated metabolism and oxygenic photosynthesis” in Natural and Laboratory-simulated Thermal Geochemical Processes. ed. R. Ikan (Dordrecht: Kluwer Academic Publishers), 325–388.
Russell, M. J., Hall, A. J., and Turner, D. (1989). In vitro growth of iron sulphide chimneys: possible culture chambers for origin-of-life experiments. Terra Nova 1, 238–241. doi: 10.1111/j.1365-3121.1989.tb00364.x
Russell, M. J., and Martin, W. (2004). The rocky roots of the acetyl coenzyme-a pathway. Trends Biochem. Sci. 29, 358–363. doi: 10.1016/j.tibs.2004.05.007
Russell, M. J., and Nitschke, W. (2017). Methane: fuel or exhaust at the emergence of life? Astrobiology 17, 1053–1066. doi: 10.1089/ast.2016.1599
Russell, M. J., Nitschke, W., and Branscomb, E. (2013). The inevitable journey to being. Philos. Trans. R. Soc. B Biol. Sci. 368:20120254. doi: 10.1098/rstb.2012.0254
Russell, M. J., and Skauli, H. (1991). A history of theoretical developments in carbonate-hosted base metal deposits and a new tri-level enthalpy classification. Econ. Geol. Monogr. 8, 96–116.
Say, R. F., and Fuchs, G. (2010). Fructose 1,6-bisphosphate aldolase/phosphatase may be an ancestral gluconeogenic enzyme. Nature 464, 1077–1081. doi: 10.1038/nature08884
Schoepp-Cothenet, B., van Lis, R., Atteia, A., Baymann, F., Capowiez, L., Ducluzeau, A.-L., et al. (2013). On the universal core of bioenergetics. Biochim. Biophys. Acta Bioenerg. 1827, 79–93. doi: 10.1016/j.bbabio.2012.09.005
Scholz-Starke, J., Primo, C., Yang, J., Kandel, R., Gaxiola, R. A., and Hirschi, K. D. (2019). The flip side of the Arabidopsis type I proton-pumping pyrophosphatase (AVP1): using a transmembrane H+ gradient to synthesize pyrophosphate. J. Biol. Chem. 294, 1290–1299. doi: 10.1074/jbc.RA118.006315
Schöne, C., Poehlein, A., Jehmlich, N., Adlung, N., Daniel, R., von Bergen, M., et al. (2022). Deconstructing Methanosarcina acetivorans into an acetogenic archaeon. Proc. Natl. Acad. Sci. U. S. A. 119:e2113853119. doi: 10.1073/pnas.2113853119
Schrenk, M. O., Brazelton, W. J., and Lang, S. Q. (2013). Serpentinization, carbon, and deep life. Rev. Mineral. Geochem. 75, 575–606. doi: 10.2138/rmg.2013.75.18
Schrödinger, E. (1944). What is Life? The Physical Aspect of the Living Cell. Cambridge, England: Cambridge University Press.
Schumann, U., and Huntrieser, H. (2007). The global lightning-induced nitrogen oxides source. Atmos. Chem. Phys. 7, 3823–3907. doi: 10.5194/acp-7-3823-2007
Shibuya, T., and Takai, K. (2022). Liquid and supercritical CO2 as an organic solvent in hadean seafloor hydrothermal systems: implications for prebiotic chemical evolution. Progr. Earth Planet. Sci. 9, 1–15. doi: 10.1186/s40645-022-00510-6
Shock, E. L. (1992). Chemical environments of submarine hydrothermal systems. Orig. Life Evol. Biosph. 22, 67–107. doi: 10.1007/BF01808019
Sibson, R. H., Moore, J. M. M., and Rankin, A. H. (1975). Seismic pumping—a hydrothermal fluid transport mechanism. J. Geol. Soc. Lond. 131, 653–659. doi: 10.1144/gsjgs.131.6.0653
Sojo, V., Herschy, B., Whicher, A., Camprubí, E., and Lane, N. (2016). The origin of life in alkaline hydrothermal vents. Astrobiology 16, 181–197. doi: 10.1089/ast.2015.1406
Spiess, F. N., Macdonald, K. C., Atwater, T., Ballard, R., Carranza, A., Cordoba, D., et al. (1980). East Pacific rise: hot springs and geophysical experiments. Science 207, 1421–1433. doi: 10.1126/science.207.4438.1421
Srere, P. A. (1987). Complexes of sequential metabolic enzymes. Annu. Rev. Biochem. 56, 89–124. doi: 10.1146/annurev.bi.56.070187.000513
Stojanowic, A., and Hedderich, R. (2004). CO2 reduction to the level of formylmethanofuran in Methanosarcina barkeri is non-energy driven when CO is the electron donor. FEMS Microbiol. Lett. 235, 163–167. doi: 10.1111/j.1574-6968.2004.tb09582.x
Suzuki, S., Ishii, S. I., Hoshino, T., Rietze, A., Tenney, A., Morrill, P. L., et al. (2017). Unusual metabolic diversity of hyperalkaliphilic microbial communities associated with subterranean serpentinization at the cedars. ISME J. 11, 2584–2598. doi: 10.1038/ismej.2017.111
Suzuki, S., Nealson, K. H., and Ishii, S. I. (2018). Genomic and in-situ transcriptomic characterization of the candidate phylum NPL-UPL2 from highly alkaline highly reducing serpentinized groundwater. Front. Microbiol. 9:3141. doi: 10.3389/fmicb.2018.03141
Szent-Györgyi, A. (1968). Bioelectronics: intermolecular electron transfer may play a major role in biological regulation, defense, and cancer. Science 161, 988–990. doi: 10.1126/science.161.3845.988
Takahashi, Y., and Mihara, H. (2004). Construction of a chemically and conformationally self-replicating system of amyloid-like fibrils. Bioorg. Med. Chem. 12, 693–699. doi: 10.1016/j.bmc.2003.11.022
Tatzel, M., Frings, P. J., Oelze, M., Herwartz, D., Lünsdorf, N. K., and Wiedenbeck, M. (2022). Chert oxygen isotope ratios are driven by Earth’s thermal evolution. Proc. Natl. Acad. Sci. U. S. A. 119:e2213076119. doi: 10.1073/pnas.2213076119
Templeton, A. S., Ellison, E. T., Glombitza, C., Morono, Y., Rempfert, K. R., Hoehler, T. M., et al. (2021). Accessing the subsurface biosphere within rocks undergoing active low-temperature serpentinization in the Samail ophiolite (Oman drilling project). J. Geophys. Res. Biogeosci. 126:e2021JG006315. doi: 10.1029/2021JG006315
Tiago, I., and Veríssimo, A. (2013). Microbial and functional diversity of a subterrestrial high pH groundwater associated to serpentinization. Environ. Microbiol. 15, 1687–1706. doi: 10.1111/1462-2920.12034
Timm, J., Pike, D. H., Mancini, J. A., Tyryshkin, A. M., Poudel, S., Siess, J. A., et al. (2023). Design of a minimal di-nickel hydrogenase peptide. Science. Advances 9:eabq1990. doi: 10.1126/sciadv.abq1990
Tosca, N. J., Guggenheim, S., and Pufahl, P. K. (2016). An authigenic origin for Precambrian greenalite: implications for iron formation and the chemistry of ancient seawater. Geol. Soc. Am. Bull. 128, 511–530. doi: 10.1130/B31339.1
Tosca, N. J., Jiang, C. Z., Rasmussen, B., and Muhling, J. (2019). Products of the iron cycle on the early earth. Free Radic. Biol. Medic. 140, 138–153. doi: 10.1016/j.freeradbiomed.2019.05.005
Trixler, F. (2021). “Origin of nucleic acids” in Prebiotic Chemistry and the Origin of Life. eds. A. Neubeck and S. McMahon (Cham: Springer), 117–137.
Trolard, F., and Bourrié, G. (2012). “Fougerite a natural layered double hydroxide in gley soil: habitus, structure, and some properties” in Clay Minerals in Nature: Their Characterization, Modification and Application. eds. M. Valaskova and G. S. Martynkova (Rijeka, Croatia: InTech), 171–188.
Trolard, F., Bourrié, G., Abdelmoula, M., Refait, P., and Feder, F. (2007). Fougerite, a new mineral of the pyroaurite-iowaite group: description and crystal structure. Clays Clay Miner. 55, 323–334. doi: 10.1346/CCMN.2007.0550308
Trolard, F., Duval, S., Nitschke, W., Ménez, B., Pisapia, C., Nacib, J. B., et al. (2022). Mineralogy, geochemistry and occurrences of fougerite in a modern hydrothermal system and its implications for the origin of life. Earth Sci. Rev. 225:103910. doi: 10.1016/j.earscirev.2021.103910
Trutschel, L. R., Chadwick, G. L., Kruger, B., Blank, J. G., Brazelton, W. J., Dart, E. R., et al. (2022). Investigation of microbial metabolisms in an extremely high pH marine-like terrestrial serpentinizing system: Ney Springs. Sci. Total Environ. 836:155492. doi: 10.1016/j.scitotenv.2022.155492
Tutolo, B. M., Seyfried, W. E. Jr., and Tosca, N. J. (2020). A seawater throttle on H2 production in Precambrian serpentinizing systems. Proc. Natl. Acad. Sci. U. S. A. 117, 14756–14763. doi: 10.1073/pnas.1921042117
Ueda, H., and Shibuya, T. (2021). Composition of the primordial ocean just after its formation: constraints from the reactions between the primitive crust and a strongly acidic, CO2-rich fluid at elevated temperatures and pressures. Fortschr. Mineral. 11:389. doi: 10.3390/min11040389
Ueltzhöffer, K., Da Costa, L., Cialfi, D., and Friston, K. (2021). A drive towards thermodynamic efficiency for dissipative structures in chemical reaction networks. Entropy 23:1115. doi: 10.3390/e23091115
Ugliengo, P. (2019). The rise of computer modeling in prebiotic chemistry: comment on “prebiotic chemistry and origins of life research with atomistic computer simulations” by a. Pérez-villa et al. Phys Life Rev 34, 139–142. doi: 10.1016/j.plrev.2019.03.013
Van Santen, R. A. (1984). The Ostwald step rule. J. Phys. Chem. 88, 5768–5769. doi: 10.1021/j150668a002
Vasiliadou, R., Dimov, N., Szita, N., Jordan, S. F., and Lane, N. (2019). Possible mechanisms of CO2 reduction by H2 via prebiotic vectorial electrochemistry. Interface Focus 9:20190073. doi: 10.1098/rsfs.2019.0073
von Döhren, H., Dieckmann, R., and Pavela-Vrancic, M. (1999). The nonribosomal code. Chem. Biol. 6, R273–R279. doi: 10.1016/S1074-5521(00)80014-9
Wächtershäuser, G. (1988). Before enzymes and templates: theory of surface metabolism. Microbio. Rev. 52, 452–484. doi: 10.1128/mr.52.4.452-484.1988
Wächtershäuser, G. (1990). Evolution of the first metabolic cycles. Proc. Natl. Acad. Sci. U. S. A. 87, 200–204. doi: 10.1073/pnas.87.1.200
Walker, S. I., and Davies, P. C. (2017). “The “hard problem” of life” in From matter to life: information and causality. eds. S. I. Walker, P. C. Davies, and G. F. Ellis (Cambridge, England: Cambridge University Press), 19–37.
Wander, M. C., Rosso, K. M., and Schoonen, M. A. (2007). Structure and charge hopping dynamics in green rust. J. Phys. Chem. C 111, 11414–11423. doi: 10.1021/jp072762n
Wang, Q., Barge, L. M., and Steinbock, O. (2019). Microfluidic production of pyrophosphate catalyzed by mineral membranes with steep pH gradients. Chem. Eur. J. 25, 4732–4739. doi: 10.1002/chem.201805950
Weiss, M. C., Sousa, F. L., Mrnjavac, N., Neukirchen, S., Roettger, M., Nelson-Sathi, S., et al. (2016). The physiology and habitat of the last universal common ancestor. Nat. Microbiol. 1:16116. doi: 10.1038/nmicrobiol.2016.116
Whicher, A., Camprubi, E., Pinna, S., Herschy, B., and Lane, N. (2018). Acetyl phosphate as a primordial energy currency at the origin of life. Orig. Life Evol. Biosph. 48, 159–179. doi: 10.1007/s11084-018-9555-8
White, L. M., Shibuya, T., Vance, S. D., Christensen, L. E., Bhartia, R., Kidd, R., et al. (2020). Simulating serpentinization as it could apply to the emergence of life using the JPL hydrothermal reactor. Astrobiology 20, 307–326. doi: 10.1089/ast.2018.1949
Wicken, J. S. (1987). Evolution, Thermodynamics and Information: Extending the Darwinian Program. Oxford University Press, New York, NY.
Wills, P. R., and Carter, C. W. (2018). Insuperable problems of the genetic code initially emerging in an RNA world. BioSyst 164, 155–166. doi: 10.1016/j.biosystems.2017.09.006
Windman, T., Zolotova, N., Schwandner, F., and Shock, E. L. (2007). Formate as an energy source for microbial metabolism in chemosynthetic zones of hydrothermal ecosystems. Astrobiology 7, 873–890. doi: 10.1089/ast.2007.0127
Woese, C. R., Dugre, D. H., Saxinger, W. C., and Dugre, S. A. (1966). The molecular basis for the genetic code. Proc. Natl. Acad. Sci. U. S. A. 55, 966–974. doi: 10.1073/pnas.55.4.966
Wolin, M. J. (1982). “Hydrogen transfer in microbial communities” in Microbial Interactions and Communities. eds. A. T. Bull and J. H. Slater (London: Academic Press), 323–356.
Wong, M. L., Charnay, B. D., Gao, P., Yung, Y. L., and Russell, M. J. (2017). Nitrogen oxides in early Earth’s atmosphere as electron acceptors for life’s emergence. Astrobiology 17, 975–983. doi: 10.1089/ast.2016.1473
Wong, M. L., and Prabhu, A. (2023). Cells as the first data scientists. J. R. Soc. Interface 20:202022081020220810. doi: 10.1098/rsif.2022.0810
Yamagata, Y., and Inomata, K. (1997). Condensation of glycylglycine to oligoglycines with trimetaphosphate in aqueous solution. II: catalytic effect of magnesium ion. Orig. Life Evol. Biosph. 27, 339–344. doi: 10.1023/A:1006529421813
Yamagata, Y., Watanabe, H., Saitoh, M., and Namba, T. (1991). Volcanic production of polyphosphates and its relevance to prebiotic evolution. Nature 352, 516–519. doi: 10.1038/352516a0
Yamamoto, M., Nakamura, R., Kasaya, T., Kumagai, H., Suzuki, K., and Takai, K. (2017). Spontaneous and widespread electricity generation in natural deep-sea hydrothermal fields. Angew. Chemie 129, 5819–5822. doi: 10.1002/ange.201701768
Yamamoto, M., Takaki, Y., Kashima, H., Tsuda, M., Tanizaki, A., Nakamura, R., et al. (2022). In situ electrosynthetic bacterial growth using electricity generated by a deep-sea hydrothermal vent. ISME J. 17, 12–20. doi: 10.1038/s41396-022-01316-6
Yamanaka, J., Inomata, K., and Yamagata, Y. (1988). Condensation of oligoglycines with trimeta- and tetrametaphosphate in aqueous solutions. Orig. Life Evol. Biosph. 18, 165–178. doi: 10.1007/BF01804669
Yarus, M. (2011). Getting past the RNA world: the initial Darwinian ancestor. Cold Spring Harb. Perspect. Biol. 3:a003590. doi: 10.1101/cshperspect.a003590
Yarus, M. (2017). The genetic code and RNA-amino acid affinities. Life 7:13. doi: 10.3390/life7020013
Yarus, M. (2021). Fitting the standard genetic code into its triplet table. Proc. Natl. Acad. Sci. U. S. A. 118:e2021103118. doi: 10.1073/pnas.2021103118
Yarus, M., Caporaso, J. G., and Knight, R. (2005). Origins of the genetic code: the escaped triplet theory. Annu. Rev. Biochem. 74, 179–198. doi: 10.1146/annurev.biochem.74.082803.133119
Yarus, M., Widmann, J. J., and Knight, R. (2009). RNA–amino acid binding: a stereochemical era for the genetic code. J. Mol. Evol. 69, 406–429. doi: 10.1007/s00239-009-9270-1
Yi, J., Kaur, H., Kazöne, W., Rauscher, S. A., Gravillier, L. A., Muchowska, K. B., et al. (2022). A nonenzymatic analog of pyrimidine nucleobase biosynthesis. Angew. Chemie 134:e202117211. doi: 10.1002/anie.202117211
Yockey, H. P. (1995). Comments on let there be life; thermodynamic reflections on biogenesis and evolution by Avshalom C. Elitzur. J. Theor. Biol. 176, 349–355. doi: 10.1006/jtbi.1995.0204
Yoshida, M., Muneyuki, E., and Hisabori, T. (2001). ATP synthase—a marvellous rotary engine of the cell. Nat. Rev. Mol. Cell Biol. 2, 669–677. doi: 10.1038/35089509
Youssef, N. H., Farag, I. F., Rudy, S., Mulliner, A., Walker, K., Caldwell, F., et al. (2019). The wood–Ljungdahl pathway as a key component of metabolic versatility in candidate phylum Bipolaricaulota (Acetothermia, OP1). Envir. Microbiol. Rep. 11, 538–547. doi: 10.1111/1758-2229.12753
Zajkowski, T., Lee, M. D., Mondal, S. S., Carbajal, A., Dec, R., Brennock, P. D., et al. (2021). The hunt for ancient prions: archaeal prion-like domains form amyloid-based epigenetic elements. Mol. Biol. Evol. 38, 2088–2103. doi: 10.1093/molbev/msab010
Zhang, S. (2012). Lipid-like self-assembling peptides. Accounts Chem. Res. 45, 2142–2150. doi: 10.1021/ar300034v
Keywords: Acetothermia, double layer oxyhydroxides, entropy disproportionation, fougerite/green rust, serpentinization, submarine alkaline vent theory (AVT)
Citation: Russell MJ (2023) A self-sustaining serpentinization mega-engine feeds the fougerite nanoengines implicated in the emergence of guided metabolism. Front. Microbiol. 14:1145915. doi: 10.3389/fmicb.2023.1145915
Received: 16 January 2023; Accepted: 22 March 2023;
Published: 15 May 2023.
Edited by:
Axel Schippers, Federal Institute For Geosciences and Natural Resources, GermanyReviewed by:
Katja Laufer-Meiser, GEOMAR Helmholtz Center for Ocean Research Kiel (HZ), GermanyCopyright © 2023 Russell. This is an open-access article distributed under the terms of the Creative Commons Attribution License (CC BY). The use, distribution or reproduction in other forums is permitted, provided the original author(s) and the copyright owner(s) are credited and that the original publication in this journal is cited, in accordance with accepted academic practice. No use, distribution or reproduction is permitted which does not comply with these terms.
*Correspondence: Michael J. Russell, TWljaGFlbC5KLlJ1c3NlbGw4MEBnbWFpbC5jb20=
Disclaimer: All claims expressed in this article are solely those of the authors and do not necessarily represent those of their affiliated organizations, or those of the publisher, the editors and the reviewers. Any product that may be evaluated in this article or claim that may be made by its manufacturer is not guaranteed or endorsed by the publisher.
Research integrity at Frontiers
Learn more about the work of our research integrity team to safeguard the quality of each article we publish.