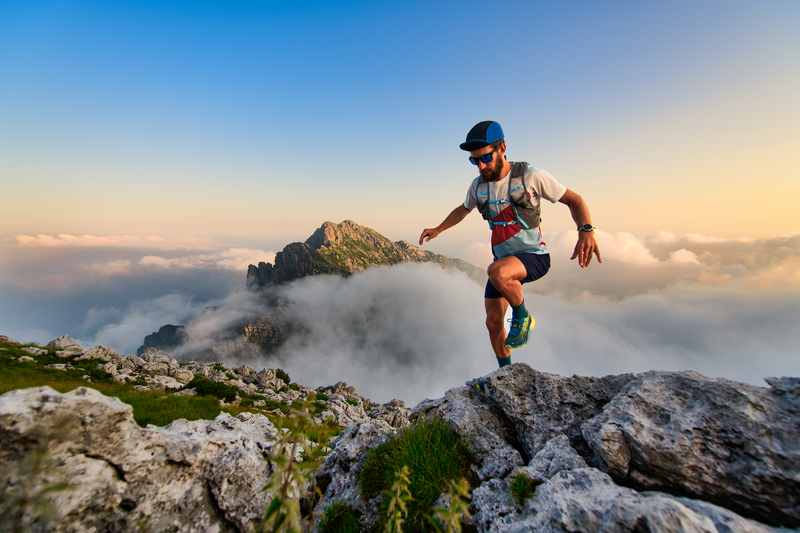
94% of researchers rate our articles as excellent or good
Learn more about the work of our research integrity team to safeguard the quality of each article we publish.
Find out more
ORIGINAL RESEARCH article
Front. Microbiol. , 05 May 2023
Sec. Microbe and Virus Interactions with Plants
Volume 14 - 2023 | https://doi.org/10.3389/fmicb.2023.1136168
Phosphatidylcholine (PC) plays crucial biological roles in eukaryotic cells. In Saccharomyces cerevisiae, apart from phosphatidylethanolamine (PE) methylation pathway, PC is also synthesized via CDP–choline pathway. Phosphocholine cytidylyltransferase Pct1 is the rate-limiting enzyme to catalyze the conversion from phosphocholine to CDP–choline in this pathway. Here, we report the identification and functional characterization of an ortholog of the budding yeast PCT1 in Magnaporthe oryzae, named MoPCT1. Targeted gene deletion mutants of MoPCT1 were impaired in vegetative growth, conidiation, appressorium turgor accumulation and cell wall integrity. Also, the mutants were severely compromised in appressorium-mediated penetration, infectious growth and pathogenicity. Western blot analysis revealed that cell autophagy was activated by the deletion of MoPCT1 under nutrient-rich conditions. Moreover, we found several key genes in PE methylation pathway, such as MoCHO2, MoOPI3, and MoPSD2, were significantly up-regulated in the ΔMopct1 mutants, indicating that a pronounced compensation effect exists between the two PC biosynthesis pathways in M. oryzae. Interestingly, in the ΔMopct1 mutants, histone H3 was hypermethylated and expression levels of several methionine cycling-related genes were significantly up-regulated, suggesting that MoPCT1 is involved in histone H3 methylation and methionine metabolism. Taken together, we conclude that the phosphocholine cytidylyltransferase coding gene MoPCT1 plays important roles in vegetative growth, conidiation and appressorium-mediated plant infection by M. oryzae.
Phosphatidylcholine (PC) is the most abundant cell membrane phospholipid that involved in various crucial biological processes (Dowd et al., 2001; Deng et al., 2008; Flis et al., 2015; Haider et al., 2018). The biosynthesis pathways of PC have been extensively studied in Saccharomyces cerevisiae. It is now clear that two main pathways are involved in PC biosynthesis: (i) CDP-choline (CDP-C) pathway: exogenous choline (C) is firstly phosphorylated by choline kinase Cki1 to form choline phosphate, which then is activated with CTP by phosphocholine cytidylyltransferase Pct1 to produce CDP-C. In the last step, CDP-C is catalyzed by choline phosphotransferase Cpt1 with diacylglycerol (DAG) to form PC (Dowd et al., 2001); (ii) Phosphatidylethanolamine (PE) methylation pathway: PE is methylated through three steps by the methyltransferases Cho2 and Opi3 using S-adenosyl-L-methionine (SAM) as methyl donor to synthesize PC (Dowd et al., 2001).
In the budding yeast, Pct1 (also known as Cct1) is the rate-limiting enzyme of CDP-C pathway and its function is highly conserved in eukaryotes (McMaster and Bell, 1994; Dowd et al., 2001). In Drosophila, mutations in Cct1 result in a number of defects on oogenesis and ovarian morphogenesis, including a loss of germline stem cell maintenance, mispositioning of the oocyte, a shortened operculum and branched ovariole phenotype (Gupta and Schüpbach, 2003). Recently, in the insect pathogenic fungus Metarhizium robertsii, it has been showed that deletion of MrPCT (homologous to S. cerevisiae PCT1) does not affect cellular content of total PC but impair fungal virulence and increase accumulation of triacylglycerol. Moreover, under nutrient-rich conditions, MrPCT negatively regulates cell autophagy (Chen et al., 2018). However, the functions of PCT1 in plant pathogenic fungi remain largely unknown.
The filamentous ascomycete fungus Magnaporthe oryzae (synonym of Pyricularia oryzae) is the causative agent of rice blast, causing huge annual loss of rice production around the world (Talbot, 2003). In the last decades, the interaction between M. oryzae and host plants has been developed as an excellent model system to study fungal-plant interactions due to its scientific and economic importance (Talbot, 2003; Ebbole, 2007; Wilson and Talbot, 2009). However, roles of phospholipid metabolism in morphological development and virulence have not well investigated in the rice blast fungus. Recently, it has been reported by Liu et al. that ceramide, a kind of sphingolipid, is essential for appressorial development and acts upstream from the protein kinase C-mediated cell wall integrity pathway (Liu et al., 2019). Here, we reported the identification and functional characterization of a putative phosphocholine cytidylyltransferase encoding gene MoPCT1 in M. oryzae. Targeted gene deletion mutants of MoPCT1 were severely impaired in vegetative growth, conidiation, cell wall integrity, appressorium turgor accumulation, and plant infection. Western blot analysis revealed that cell autophagy was activated by the deletion of MoPCT1 under nutrient-rich conditions. Also, we found that histone H3 of the ΔMopct1 mutants was hypermethylated and methionine cycle was accelerated by enhancing the expression of methionine cycling-related genes. Our findings provide novel insights into understanding the role of PC biosynthesis during fungal development and plant infection in M. oryzae.
To investigate the roles of CDP-C pathway of PC biosynthesis in pathogenesis of M. oryzae, we firstly identified a putative phosphocholine cytidylyltransferase encoding gene in M. oryzae genome by BLAST search using S. cerevisiae PCT1 as a guide, termed MoPCT1(MGG_01003). And then, we carried out a targeted gene deletion of MoPCT1, using the background strain Guy11. A null mutant ΔMopct1 and a complemented transformant ΔMopct1-C were generated (Supplementary Figure S1).
To determine the role of MoPCT1 in growth and sporulation, the wild-type strain Guy11, the null mutant ΔMopct1, and the complemented transformant ΔMopct1-C were cultured on CM and MM medium. The ΔMopct1 mutant showed a significant reduction in vegetative growth, compared with the wild-type Guy11 and the complemented ΔMopct1-C on CM or MM (Figures 1A,B). Consistently, when the hypha blocks with same size were cultured in liquid CM for 2 days, the ΔMopct1 mutant formed much smaller mycelium pellets than those of the wild-type and the complemented strains (Figure 1A). Next, the ability to form conidia was evaluated by carefully washing the surface of 10-day-old cultures on CM plates. The ΔMopct1 mutant produced significantly less conidia with (14.78 ± 0.96) × 106 spores per plate, compared to the wild-type strain Guy11 and the complemented ΔMopct1-C with (59.28 ± 2.28) × 106 spores per plate and (48.95 ± 2.57) × 106 spores per plate, respectively (Figure 1C). These results indicate that MoPCT1 is important for vegetative growth and conidiation of M. oryzae.
Figure 1. MoPCT1 is important for vegetative growth and sporulation of Magnaporthe oryzae. (A) Colonies of the wild-type Guy11, the null mutant ΔMopct1 and the complemented strain ΔMopct1-C cultured on CM (top penal) and MM (bottom penal) plates at 28°C for 10 days; mycelial plugs (5 × 5 mm) of each strain were cultured in liquid CM medium at 28°C for 48 h (middle panel). The scale bar = 5 mm. (B) Radial growth of each strain on CM and MM plates. (C) Statistical analysis of conidiation. Error bars represent standard deviation. Single asterisk indicates significant difference (P < 0.05). Double asterisks indicate significant difference (P < 0.01).
In order to determine whether deletion of MoPCT1 influences appressorium formation, we carried out appressorium induction experiments. Conidia from different strains were allowed to germinate on a hydrophobic surface. By 24 h, we observed that the appressorium formation rate of the ΔMopct1 mutant was not significantly different from that of the wild-type Guy11 or the complemented ΔMopct1-C (Figure 2A). To further determine whether deletion of MoPCT1 leads to alteration of appressorium turgor, we carried out cytorrhysis assays. Appressoria were allowed to form on plastic cover slips for 24 h, and the proportion of collapsed appressoria after exposure to different glycerol solutions was calculated. We found that, under 1 M glycerol solution, the percentages of collapsed appressoria of the wild-type and complemented strains were 14.82 ± 2.11% and 18.97 ± 6.12%, respectively, significantly lower than (78.49 ± 4.57) % of the ΔMopct1 mutant (Figure 2B). Even when the induction time for appressorium formation was extended to 48 h, the appressorium turgor defect of the mutant was not restored (data not shown). These results indicate that MoPCT1 is dispensable for appressorium formation but crucial for appressorium turgor generation in M. oryzae. Next, cuticle penetration and invasive growth assays were performed on onion and barley epidermis. By 48 hpi, most conidia of Guy11 and ΔMopct1-C could penetrate cuticle and form dense infectious hyphae on either onion or barley epidermis. However, most appressoria formed by ΔMopct1 could not infect the epidermis (Figure 2C). To quantitatively evaluate fungal infectious development on onion epidermis, the infection structures were divided into four types (Figure 2D). On onion epidermis, up to 67% appressoria of Guy11 and 58% appressoria of ΔMopct1-C could form type 4, in contrast to 94.67% appressoria formed by the ΔMopct1 mutant could not penetrate the epidermis cell surfaces (Figure 2D). Similar results were observed on barley epidermis (data not shown).
Figure 2. MoPCT1 plays an important role in appressorium turgor generation and host penetration. (A) Appressorium formation rates. Conidia suspensions of the tested strains were incubated on hydrophobic plastic coverslips and allowed to form appressoria for 24 h. AP, appressorium. (B) Cytorrhysis assays. Appressoria of Guy11 and ΔMopct1 induced for 24 h were put into gradient concentrations of glycerol (1, 2, 3, and 4 M) and the proportion of collapsed appressoria was calculated. (C) Penetration assays on epidermis of barley and onion. Conidial suspensions (5 × 104 conidia ml−1) of each strain were inoculated onto the epidermis of barley or onion. After 48 h at 28°C in dark, the epidermis was observed under a light microscopy. OE, onion epidermis; BE, barley epidermis. The scale bar = 20 μm. (D) Statistical analysis of penetration and invasion growth on onion epidermis cells after 48 h for induction. Error bars represent standard deviations. Type 1, no penetration; type 2, invasion hyphae without branch; type 3, invasion hyphae with a single branch; type 4, invasion hyphae with branches.
To further determine whether MoPCT1 is required for pathogenicity, conidial suspensions of the wild-type Guy11, the ΔMopct1 mutant, and the ΔMopct1-C were sprayed onto 7-day-old barley or 14-day-old rice leaves, respectively. After 5 days, the wild-type strain and the complemented ΔMopct1-C caused numerous typical spreading lesions on the host leaves, in contrast to much less and smaller lesions caused by the ΔMopct1 mutant (Figure 3A). In addition, the cut-leaf assays displayed that pathogenicity of the ΔMopct1 mutant was severely compromised on intact barley leaves, even when they were abraded to remove the surface cuticle (Figure 3B). These results indicate that MoPCT1 is required for fungal penetration and host intracellular colonization by M. oryzae.
Figure 3. ΔMopct1 is significantly impaired in pathogenicity. (A) Spray-inoculation assays. Barley and rice seedlings were spray-inoculated with 10 ml conidial suspension (5 × 104 conidia ml−1) of each strain. Photographs were taken at 5 days post inoculation (dpi). (B) Barley cut leaf inoculation assays. Conidia suspensions (5 × 104 conidia ml−1) of various strains were dropped onto barley leaf segments wounded or unwounded. Photographs were taken at 5 dpi.
In S. cerevisiae, Pct1 is mainly located in the nucleoplasm and nuclear membranes (MacKinnon et al., 2009). There is a conserved sequence at the N terminal of Pct1, which is presumed to be nuclear localization signal (MacKinnon et al., 2009). By protein sequence alignment analysis, we identified a hypothetical nuclear localization signal in MoPct1, named NLS (Figure 4A). To determine whether the predicted NLS of MoPct1 is required for its nuclear localization, a complete MoPCT1 gene fragment and the fragment without the NLS were ligated with GFP and then the resulting constructs were transformed into the ΔMopct1 mutant, respectively. Consequently, strains expressing MoPct1-GFP or MoPct1ΔNLS-GFP were obtained. By a laser confocal microscope, we observed that MoPct1-GFP mainly localized to nucleus of conidium cells, while MoPct1ΔNLS-GFP was diffusely distributed in cytoplasm (Figure 4B), indicating the NLS is required for nuclear localization of MoPct1. To investigate the effect of MoPct1 NLS on pathogenicity of the blast fungus, we carried out spray-inoculation assays on barley seedlings. Surprisingly, the strains expressing the MoPct1ΔNLS-GFP were fully pathogenic to barley leaves (Figure 4C). Further phenotypic analysis of the strains showed that absence of NLS signal did not affect mycelium growth and sporulation of M. oryzae (Supplementary Figure S2). These results suggested that the NLS of MoPct1 is essential for transportation of the protein to nucleus but dispensable for mycelium growth, conidiation and pathogenicity by M. oryzae.
Figure 4. MoPCT1 mainly localizes to the nucleus and NLS of MoPct1 is dispensable for pathogenicity by Magnaporthe oryzae. (A) The nuclear localization signal (NLS) of MoPct1 was predicted by the alignment with Saccharomyces cerevisiae Pct1. The red line indicates the predicted NLS of MoPct1. (B) GFP fluorescence was examined under a fluorescent confocal microscopy. Bars = 5 μm. (C) Spray-inoculation assays. Barley seedlings were spray-inoculated with 10 ml conidial suspension (5 × 104 conidia ml−1) of each strain. Photographs were taken at 5 dpi.
Cell wall integrity is essential for the maintenance of appressorium turgor (Xu et al., 1998). To determine whether knockout of MoPCT1 affects cell wall integrity of M. oryzae, we measured the sensitivity of different strains to cell wall stress. The wild-type Guy11, the ΔMopct1 mutant, and the complemented ΔMopct1-C were inoculated on CM medium added cell wall stressors, including Congo red (CR) and calcofluor-white (CFW). After 10 days post-inoculation, colony diameters of different cultures were measured. The results showed that the growth reduction rate of the ΔMopct1 mutant was significantly higher than the wild-type Guy11 and the complemented ΔMopct1-C under the condition supplemented with 400 μg/ml CR, 200 μg/ml CFW or 400 μg/ml CFW (Figure 5), indicating that the null mutant is hypersensitive to cell wall stress and MoPCT1 is required for cell wall integrity of M. oryzae.
Figure 5. Deletion of MoPCT1 leads to hypersensitivity to cell wall stressors. (A) The strains incubated on CM supplemented with Congo red (CR) or calcofluor white (CFW) at 28°C for 10 days. (B) Colony growth reduction rates of the tested strains on different medium. Growth reduction rate = (diameter of Guy11-diameter of a tested strain)/diameter of Guy11. Standard deviations were calculated based on three independent experiments. Error bars represent standard deviation. Double asterisks indicate significant difference (P < 0.01).
To determine whether autophagy is affected by deletion of MoPCT1 in M. oryzae, we tested the degradation process of GFP-MoAtg8 by Western blot assays. The GFP-MoAtg8 fusion protein was constructed and transformed into the wild-type Guy11 and the ΔMopct1 mutant, respectively. Mycelium of the wild-type or the mutant expressed GFP-MoAtg8 was cultured in CM liquid medium for 36 h (28°C, 180 rpm), and then transferred part of the mycelium to MM-N medium to induce cell autophagy for 8 h. All the mycelium was collected and total protein of the tested strains was extracted. Western blot analysis displayed that a clear full-length GFP-MoAtg8 band (40 kDa) and a free GFP band (26 kDa) were detected in both the wild-type Guy11 and the ΔMopct1 mutant (Figure 6A). By calculating the ratio of free GFP to total GFP (sum of free GFP and GFP-MoATG8), we found that under CM condition, the GFP ratio of Guy11 and ΔMopct1 was 0.28 and 0.38, respectively. The proportion of free GFP in ΔMopct1 was significantly higher than that of Guy11, suggesting the autophagy level of the ΔMopct1 was higher under nutrient-rich conditions than the wild-type strain Guy11. When hyphae were shifted to nitrogen starvation conditions (MM-N medium) for 8 h, an increasingly stronger free GFP band were detected both in the wild-type Guy11 and the ΔMopct1, indicating that deletion of MoPCT1 does not affect the response to nitrogen starvation in M. oryzae.
Figure 6. Deletion of MoPCT1 activates cell autophagy under non-induced conditions. (A) GFP-MoAtg8 proteolysis assays. Immunoblot assays with total lysates from CM or MM-N cultures of various strains using anti-GFP antibody or anti-GAPDH antibody. (B) Expression levels of autophagy-related genes under CM or MM-N conditions in the ΔMopct1 mutant compared to that in the wild-type Guy11. Error bars represent standard deviation. Double asterisks indicate significant difference (P < 0.01).
Then we examined the expression levels of several key autophagy-related genes including MoATG1, MoATG8 and MoATG13. The expressions of the three genes in mycelium of the ΔMopct1 mutant were significantly up-regulated in CM condition compared with the wild-type, but no significant difference was observed in MM-N condition (Figure 6B), indicating that MoPCT1 negatively regulates autophagy process of M. oryzae under nutrition-rich conditions. In addition, the expressions of the ATG-related genes during appressoria infection on barley leaves were determined by qRT-PCR. We found that only MoATG1 expression in the mutant was significantly up-regulated (Supplementary Figure S3A).
To investigate the role of MoPCT1 in PC synthesis, the Guy11 strain and the ΔMopct1 were inoculated on CM medium supplemented with choline chloride (CC), CDP-C, or PC, respectively. Colony diameter was measured after 10 days post incubation at 28°C. The results showed that the defects in growth, asexual sporulation and pathogenicity of the ΔMopct1 mutant were not restored by adding exogenous CC to CM medium (Figures 7A–D), indicating that the utilization of exogenous choline via CDP–C pathway for PC synthesis is blocked by the deletion of MoPCT1. Surprisingly, adding exogenous CDP-C or PC, which are downstream products of the reaction catalyzed by MoPct1, also failed to recover the phenotypic defects of the ΔMopct1(Figures 7A–D). To further test whether deletion of MoPCT1 causes the defect in PC production, the PC content of the Guy11 strain and the ΔMopct1 was detected by ELISA method. The results exhibited that the content of PC in ΔMopct1 was (318.67 ± 5.00) pg./ml, while the content of PC in Guy11 and ΔMopct1-C was (266.3 ± 21.39) pg./ml and (289.14 ± 9.34) pg./ml, respectively, (Figure 7E). It indicated that deletion of MoPCT1 did not lead to reduction of PC, but caused the significant increase of PC content in M. oryzae. We hypothesize that the PE methylation pathway of PC synthesis may be activated in the ΔMopct1 mutant to maintain intracellular PC synthesis. To test the hypothesis, we determined transcriptional expression of MoCHO2 and MoOPI3, which encode the two key enzymes in the PE methylation pathway, as well as MoPSD1 and MoPSD2, which encodes enzymes to catalyze PE synthesis by RT-qPCR. The results showed that expression levels of MoPSD2, MoCHO2 and MoOPI3 in the ΔMopct1 mutant were significantly up-regulated (Figure 7F), indicating that the PE methylation pathway of PC synthesis is activated by the loss of MoPCT1.
Figure 7. Deletion of MoPCT1 activates the PE methylation pathway of PC biosynthesis. (A) Growth of the ΔMopct1 mutant on CM added 1 mM PC, 0.1 mM CDP-C, or 1 mM CC. The plates were cultured at 28°C for 10 days. (B) Radial growth of each strain under different condition. (C) Statistical analysis of conidiation. Error bars in (B) and (C) represent standard deviation. (D) Spray-inoculation assays. Barley seedlings were spray-inoculated with 10 ml conidial suspension (5 × 104 conidia ml−1) of each strain with different additions (1 mM PC, 0.1 mM CDP-C, or 1 mM CC). Photographs were taken at 5 dpi. (E) Bar chart of PC content for Guy11 and ΔMopct1. (F) Expression levels of the genes involved in PE methylation pathway. Means and standard deviations were calculated based on three independent experiments. Error bars represent standard deviation. Double asterisks indicate significant difference (P < 0.01).
In S. cerevisiae, PE methylation is a major consumer of SAM. Deletion of CHO2 could result in SAM accumulation, thus causes the hypermethylation of Histone H3 (Ye et al., 2017). To determine whether activation of PE methylation in the ΔMopct1 affects H3 methylation, we measured the level of H3 methylation by Western blot assays. Unexpectedly, the methylation levels on various sites of histone H3 under CM condition notably increased in the ΔMopct1 compared with that in the wild-type strain, such as H3K4, H3K27, and H3K36 (Figure 8). On the contrary, there were no differences of the methylation levels of histone H3 between the wild-type Guy11 and the ΔMopct1 on MM medium (Figure 8). These results suggest that deletion of MoPCT1 results in excess methyl flow into the histone methylation under CM condition.
Figure 8. Disruption of MoPCT1 leads to the hypermethylation of histone H3 under nutrition-rich condition. Levels of methylated H3 at specific lysine sites in Guy11 and ΔMopct1 under CM or MM conditions. WT: Guy11; Δ: ΔMopct1. The data are representative of at least three independent experiments.
Methyl is produced from SAM to form SAH, which is a key part of methionine metabolism cycle, and therefore SAM is the methyl donor for biological methylation modifications (Stipanuk, 2004; Brosnan and Brosnan, 2006). Since activation of the PE methylation pathway and hypermethylation of histone H3 in the ΔMopct1 consume large amounts of methyl groups, to determine whether the methyl depletion affects SAM content and methionine cycle, we detected the contents of SAM, homocysteine (HCY), methionine (MET), and S-adenosylhomocysteine (SAH) in the ΔMopct1 by LC/MS. The results showed that the content of HCY, SAM or SAH in the ΔMopct1 was not statistically different from that of the Guy11 strain. However, the content of MET in the ΔMopct1 was significantly increased (Figure 9A).
Figure 9. Disruption of MoPCT1 causes acceleration of methionine metabolism cycle. (A) LC–MS/MS analysis. Intracellular levels of methionine-related metabolites determined by multiple reaction monitoring (MRM) using LC–MS/MS. There were 6 replicates per sample. Single asterisk indicates significant difference (P < 0.05). (B) and (C) RT-qPCR analysis. Expression of the genes associated with methionine metabolic cycle in different strains growth on CM (B) or MM (C) was determined. Means and standard deviations were calculated based on three independent experiments. Error bars represent standard deviation. Double asterisks indicate significant difference (P < 0.01).
We hypothesized that the large amount of methyl provided by the ΔMopct1 mutants may be caused by the acceleration of the entire methionine cycle. To test this hypothesis, expression levels of methionine cycling-related genes were detected by RT-qPCR. The results showed that the expression levels of MoMET6, MoMET17, MoSAM1, and MoSAH1, key genes of methionine cycling, were significantly up-regulated in mycelium of the ΔMopct1 compared with Guy11 under CM condition (Figure 9B). Moreover, the expressions of MoSAH1, MoSAH1, and MoMET6 during appressoria infection were up-regulated too (Supplementary Figure S3B). These results suggested that the flux of methionine metabolism is enhanced under nutrition condition. However, under the MM condition, the expressions of MoSAM1 and MoSAH1 in the ΔMopct1 were not significantly different compared to Guy11, while the expressions of MoMET6 and MoMET17 in the ΔMopct1 were significantly decreased (Figure 9C). These results indicated that the methionine cycle was activated in the ΔMopct1 under nutrition-rich conditions, which may provide a large number of methyl groups for PE methylation pathway and histone H3 methylation.
In S. cerevisiae, phosphocholine cytidylyltransferase Pct1 is the rate-limiting enzyme in CDP-C pathway for PC synthesis (Dowd et al., 2001). Recently, it has been reported that deletion of MrPCT (homologous to PCT1) significantly reduces the growth rate and pathogenicity in the insect pathogenic fungus M. robertsii (Chen et al., 2018). Consistently, in this study, we found that MoPCT1 was crucial for vegetative growth, conidiation, appressorium turgor generation and plant infection in the rice blast fungus M. oryzae. Interestingly, we also found that MoPCT1 was involved in cell wall integrity and cell autophagy of the fungus. Therefore, we conclude that CDP-C pathway for PC synthesis plays important roles in fungal development and pathogenicity in M. oryzae. To our knowledge, the orthologs of the MoPCT1 gene has not been functionally characterized previously in phytopathogenic fungi.
Appressorium differentiated from a conidium is a key specialized structure to infect host cells by M. oryzae (Howard et al., 1991; Talbot, 2003). In this study, we found that deletion of MoPCT1 did not affect appressorium formation but significantly reduced appressorium turgor generation (Figures 2A,B). Many researchers have reported that the accumulation of appressorium turgor is related to cell wall integrity, transport and metabolism of nutrients, cell cycle regulation, autophagy, and other processes (Xu et al., 1998; Thines et al., 2000; Veneault-Fourrey et al., 2006; Jeon et al., 2008; Saunders et al., 2010). By staining experiments, we observed that the mobilization and degradation patterns of glycogen stores and lipid droplets during appressorium formation in the ΔMopct1 were similar to that in the Guy11 strain (data not shown). However, we found that ΔMopct1 exhibited high sensitivity to cell wall stressors such as Congo red or calcofluor-white (Figure 5), indicating that the ΔMopct1 displays a significant defect in cell wall integrity. Previously, in M. oryzae, it has been shown that the Mps1-MAPK signaling pathway is mainly involved in the regulation of cell wall integrity and appressorium-mediated penetration and infection (Xu et al., 1998; Fujikawa et al., 2009). However, we found that deletion of MoPCT1 did not affect the phosphorylation level of Mps1 (Supplementary Figure S4). Therefore, how MoPCT1 affects cell wall integrity in M. oryzae remains elusive.
In addition, it has been reported that MrPCT negatively regulated autophagy process in M. robertsii (Chen et al., 2018). Similarly, we found that the autophagy level of the ΔMopct1 was significantly higher than that of Guy11 under nutrient-rich conditions (Figure 6A), and the expression of autophagy-related genes, such as MoATG1, MoATG8, and MoATG13, was significantly increased in mycelium of ΔMopct1 (Figure 6B), indicating that MoPCT1 negatively regulates autophagy in M. oryzae. Previous studies have shown that autophagy is a prerequisite for host infection of pathogen, and the deletion of some non-selective autophagy-related genes can lead to different degrees of reduced pathogenicity or even complete loss of M. oryzae (Kershaw and Talbot, 2009).
In the study of two PC metabolic pathways in rats, treatment of rat liver cells with the SAH hydrolase inhibitor 3-deazaadenosine (DZA) inhibited the activity of PE methylated pathway methyltransferases (Cho2 and Opi3), whereas the activity of Pct1 homologous protease increased, suggesting that there may be a compensation mechanism between the two pathways (Pritchard et al., 1982). In this study, exogenous addition of PC did not restore phenotypic defects of the ΔMopct1 mutant (Figures 7A–D). Meanwhile, ELISA results showed that PC content in the ΔMopct1 was not decreased compared with wild-type Guy11 (Figure 7E) Therefore, we speculate that blocking of CDP-C pathway may activate PE methylation pathway to maintain proper intracellular PC content level in the ΔMopct1 mutants. This view was supported by the results of RT-qPCR assay. We found that the expression of the key genes MoCHO2 and MoOPI3 in PE methylation pathway was significantly up-regulated in the ΔMopct1 compared to the wild-type Guy11 (Figure 7F).
In S. cerevisiae, the PE methylation pathway is the main methyl consumption pathway, and blocking of PE methylation pathway will lead to accumulation of SAM, resulting in the hypermethylation of histone H3 and phosphatase PP2A (Ye et al., 2017). In this study, we found that the PE methylation pathway was dramatically activated in the ΔMopct1 (Figure 7F), whereas under CM conditions, the methylation levels of H3 in the ΔMopct1 was significantly increased at multiple sites, including H3K4, H3K27, and H3K36, compared with Guy11 (Figure 8). Therefore, in M. oryzae, even in the case of excessive methyl consumption by the activation of PE methylation pathway, methyl groups were still sufficient for methylation reaction of histone H3. SAM is a major intracellular methyl donor and an intermediate in the methionine metabolic cycle (Stipanuk, 2004; Brosnan and Brosnan, 2006). In this study, LC/MS analysis exhibited that there was no significant difference in the content of methionine cycling-related metabolites, including SAM, SAH and HCY between Guy11 and the ΔMopct1, However MET content was significantly increased in the ΔMopct1 (Figure 9A). Also, we found that the expression levels of methionine cycling-related genes in the ΔMopct1 were significantly up-regulated under CM conditions, such as MoMET6, MoMET17, MoSAM1, MoSAH1 (Figure 9B). Among them, MoMET6, MoSAM1, and MoSAH1 were also up-regulated during the appressorium infection stage (Supplementary Figure S3B). In contrast, under the MM condition, the expressions of MoSAM1 and MoSAH1 in the ΔMopct1 were not significantly different compared to Guy11, while the expressions of MoMET6 and MoMET17 in the ΔMopct1 were significantly decreased (Figure 9C). These results were consistent with the methylation levels of histone H3 under CM and MM (Figure 8).
The hypermethylation of histone H3 will cause changes in the overall transcription level of genes and affect the metabolism of sulfur-containing amino acids in budding yeast (Ye et al., 2017). Recently, roles of histone H3 methylation in fungal differentiation and pathogenicity have been explored in M. oryzae (Pham et al., 2015; Huh et al., 2017; Zhou et al., 2021). Approximately 5% of M. oryzae genes showed significant changes depended on H3K4me2 or H3K4me3 during infection-related morphogenesis (Pham et al., 2015). In addition, deletion of MoJMJ1, a JmjC-domain-containing histone demethylases encoding gene, led to increased methylation levels of H3K4me3, ΔMojmj1 showed significant defects in vegetative growth, sporulation, appressorium formation, and infection hypha growth (Huh et al., 2017). In this study, consistently, deletion of MoPCT1 also resulted in increased methylation levels of multiple sites of histone H3 in M. oryzae (Figure 8), which may be one of the causes of phenotypic defects of M. oryzae. We speculate that the blocking of CDP-C pathway activates MET metabolism-related cycle and may provide sufficient methyl for H3 hypermethylation. Meanwhile, accelerating MET cycle in the mutant may be an effective way to compensate methyl consumption for the activated PE methylation pathway. However, the exact regulatory mechanisms are still unknown. Taken together, MoPCT1 plays pleiotropic roles in regulating methionine metabolism and histone H3 hypermethylation to govern M. oryzae morphogenesis and pathogenicity.
All the mutants used in this study were generated from M. oryzae wild-type strain Guy11. Standard growth and storage procedures for fungal strains were performed as described previously (Talbot et al., 1993). Escherichia coli strain T1 (TransGen, Beijing, China) was used for routine bacterial transformations and maintenance of various plasmids in this study.
For generating the MoPCT1 gene replacement vector pKO-PCT1, the 1.5 kb upstream and 1.5 kb downstream sequences of target genes were amplified with primer pairs PCT1-up-F/R and PCT1-down-F/R, respectively. Hygromycin-resistant fragments were amplified by HPT-F/R. The PCR products were cloned into pKOV21 vector to generate pKO-PCT1 (Supplementary Figure S1A). The resulting vectors were transformed into protoplasts of M. oryzae Guy11 to generate null mutants, as previously described (Talbot et al., 1993). Hygromycin B (Roche, Mannheim, Germany) was added to a final concentration of 200 μg/ml for transformant selection.
For construction of the complementation vector pHB-PCT1, a 1.5 kb native promoter region and 1.9 kb full length region of the MoPCT1 gene were amplified with primer pairs HB-PCT1-F/R. The PCR products were cloned into pCB1532 according to the manufacturer’s instructions of One Step Cloning Kit (Vazyme, Nanjing, China). Then, the plasmid pHB-PCT1 was transformed into protoplasts of the ΔMopct1 mutants. Transformants were screened for sulfonylurea resistance on BDCM and verified by PCR.
All primers were synthesized by Sangon Biotech in Shanghai, China, and the sequence of primers is shown in Supplementary Table S1.
Vegetative growth was assessed by measurement of colony diameter in plate cultures of different strains grown on different medium at 28°C for 10 days. The level of sporulation was assessed by harvesting conidia from the surface of 10-day-old strains grown on CM solid medium and determining the concentration of the resulting conidial suspension using a hemocytometer (Corning, China).
Appressorium formation of M. oryzae requires induction at 25°C in darkness for 24 or 48 h. For the rates of appressorium formation, conidial suspensions (5 × 104 conidia ml−1) were placed on hydrophobic coverslips for 24 h and measured by microscopic examination of at least 100 conidia. For inoculation to onion and barley epidermis surfaces in vitro, conidial suspensions (5 × 104 conidia ml−1) were carefully inoculated on onion or barley epidermis surfaces and incubated for 48 h. Then the appressorium were observed and photographed by optical microscope. Each test was repeated at least three times.
Two-week-old seedlings of the rice cultivar ‘CO39’ and 7-day-old seedlings of the barley cultivar ‘Golden Promise’ were used for infection assays. For cut-leaf assays, leaf fragments were cut from barley seedlings and placed in plastic plates containing wetted filter papers. Wounded barley leaves were prepared by breaking the cuticle by abrasion with an emery board, and moisturized as same as the cut-leaf assays. Then the conidial suspensions (5 × 104 conidia ml−1) were placed onto the leaf surface and incubated at 28°C for 5 days. For spray-inoculation assays, conidial suspensions (5 × 104 conidia ml−1 diluted in 0.05% Tween-20) were sprayed on rice or barley plants. Inoculated seedlings were kept in black plastic bags for 24 h and then grown at 25°C and 90% relative humidity for 4 days. Disease lesions were photographed after 5 days of incubation. Each test was repeated at least three times.
Guy11 and ΔMopct1 were inoculated on cellophane coated CM solid medium and cultured at 28°C for 10 days. Then same weight of Guy11 and ΔMopct1 mycelia were collected, and the supernatant was taken as samples after grinding with lysate and centrifuged at high speed. Content of total phosphatidylcholine in samples were analyzed using a PC ELISA kit according to the manufacturer’s protocol (Meilian, Shanghai, China) and measured using a microplate reader (SynergyMx, BioTek, USA).
Total RNA was extracted using PureLink RNA Mini Kit (Invitrogen, USA) from mycelia and appressoria. Synthesis of the cDNA and RT-qPCR were performed as described previously (Yue et al., 2017). HiScript® II qRT SuperMix for qPCR (Vazyme, Nanjing, China) were used for reverse transcription. ChamQTM Universal SYBR®qPCR Master Mix (Vazyme, Nanjing, China) were used for RT-qPCR. The relative expression level of each gene was calculated using the 2-ΔΔCT method (LivaK and Schmittgen, 2001). β-tubulin (MGG_00604) was used as an endogenous reference. All the primers used for RT-qPCR assays are listed in Supplementary Table S1. Means and standard deviations were calculated based on three independent experiments.
Total protein was extracted from mycelia cultured in liquid CM or MM at 28°C, 180 rpm for 36 h. For measuring the autophagy levels, part of mycelia cultured on CM for 36 h were transferred into liquid MM-N medium cultured for 8 h. Hyphae of each sample were filtered with gauze and washed with sterile distilled water, then squeezed dry for total protein extraction. The extraction of total protein was performed as described previously (Lv et al., 2017). Denatured protein samples were separated on an 10% (15%) SDS-PAGE gel and then transferred to PVDF membrane (Solarbio, Beijing, China). The expression of Mps1 were detected with the anti-p44/42 MAPK antibody (anti-MAPK) and the phosphorylation level of Mps1 were detected with the phospho-p44/42 MAPK antibody (anti-TpEY) (Cell Signaling Technology). The other antibodies used in this work were list in Supplementary Table S2. FDTM FDbio-Femto Ecl chemiluminescent substrate (Fdbio science, Hangzhou, China) was used for antigen antibody detections.
The mycelium plugs were inoculated on CM medium covered with cellophane for 10 days, then the surface mycelium was scraped and collected into 2 ml EP tubes and stored at −80°C. The samples were delivered to the company named Clim5 Technology (Hangzhou, China) for the UPLC–MS/MS analysis. There were six replicates per sample.
The raw data supporting the conclusions of this article will be made available by the authors, without undue reservation.
ZX and ZW conceived and designed the experiments, analyzed the data, and wrote the paper. ZX, WL, QT, YX, and ZW performed the experiments. All authors contributed to the article and approved the submitted version.
This work was supported by the Natural Science Foundation of China to ZW (Grant No. 32070141).
The authors declare that the research was conducted in the absence of any commercial or financial relationships that could be construed as a potential conflict of interest.
All claims expressed in this article are solely those of the authors and do not necessarily represent those of their affiliated organizations, or those of the publisher, the editors and the reviewers. Any product that may be evaluated in this article, or claim that may be made by its manufacturer, is not guaranteed or endorsed by the publisher.
The Supplementary material for this article can be found online at: https://www.frontiersin.org/articles/10.3389/fmicb.2023.1136168/full#supplementary-material
Brosnan, J. T., and Brosnan, M. E. (2006). The sulfur-containing amino acids: an overview. J. Nutr. 136, 1636S–1640S. doi: 10.1093/jn/136.6.1636S
Caracuel-Rios, Z., and Talbot, N. J. (2007). Cellular differentiation and host invasion by the rice blast fungus Magnaporthe grisea. Curr. Opin. Microbiol. 10, 339–345. doi: 10.1016/j.mib.2007.05.019
Chen, Y., Li, B., Cen, K., Lu, Y., Zhang, S., and Wang, C. (2018). Diverse effect of phosphatidylcholine biosynthetic genes on phospholipid homeostasis, cell autophagy and fungal developments in Metarhizium robertsii. Environ. Microbiol. 20, 293–304. doi: 10.1111/1462-2920.13998
Deng, L., Nagasawa, J., Ono, Y., Ishikawa, Y., Kakihara, T., Fukuda, R., et al. (2008). Manipulation of major membrane lipid synthesis and its effects on sporulation in Saccharomyces cerevisiae. Biosci. Biotechnol. Biochem. 72, 2362–2368. doi: 10.1271/bbb.80265
Dowd, S. R., Bier, M. E., and Patton-Vogt, J. L. (2001). Turnover of phosphatidylcholine in Saccharomyces cerevisiae. The role of the CDP-choline pathway. J. Biol. Chem. 276, 3756–3763. doi: 10.1074/jbc.M003694200
Ebbole, D. J. (2007). Magnaporthe as a model for understanding host-pathogen interactions. Annu. Rev. Phytopathol. 45, 437–456. doi: 10.1146/annurev.phyto.45.062806.094346
Flis, V. V., Fankl, A., Ramprecht, C., Zellnig, G., Leitner, E., Hermetter, A., et al. (2015). Phosphatidylcholine supply to peroxisomes of the yeast Saccharomyces cerevisiae. PLoS One 10:e0135084. doi: 10.1371/journal.pone.0135084
Fujikawa, T., Kuga, Y., Yano, S., Yoshimi, A., Tachiki, T., Abe, K., et al. (2009). Dynamics of cell wall components of Magnaporthe grisea during infectious structure development. Mol. Microbiol. 73, 553–570. doi: 10.1111/j.1365-2958.2009.06786.x
Gibellini, F., and Smith, T. K. (2010). The Kennedy pathway--De novo synthesis of phosphatidylethanolamine and phosphatidylcholine. IUBMB Life 62, 414–428. doi: 10.1002/iub.337
Gupta, T., and Schüpbach, T. (2003). Cct1, a phosphatidylcholine biosynthesis enzyme, is required for Drosophila oogenesis and ovarian morphogenesis. Development 130, 6075–6087. doi: 10.1242/dev.00817
Haider, A., Wei, Y. C., Lim, K., Barbosa, A. D., Liu, C. H., Weber, U., et al. (2018). PCYT1A regulates phosphatidylcholine homeostasis from the inner nuclear membrane in response to membrane stored curvature elastic stress. Dev. Cell 45, 481–495.e8. doi: 10.1016/j.devcel.2018.04.012
Howard, R. J., Ferrari, M. A., Roach, D. H., and Money, N. P. (1991). Penetration of hard substrates by a fungus employing enormous turgor pressures. Proc. Natl. Acad. Sci. U. S. A. 88, 11281–11284. doi: 10.1073/pnas.88.24.11281
Huh, A., Dubey, A., Kim, S., Jeon, J., and Lee, Y. H. (2017). MoJMJ1, encoding a histone demethylase containing JmjC domain, is required for pathogenic development of the rice blast fungus Magnaporthe oryzae. Plant Pathol. J. 33, 193–205. doi: 10.5423/PPJ.OA.11.2016.0244
Jeon, J., Goh, J., Yoo, S., Chi, M. H., Choi, J., Rho, H. S., et al. (2008). A putative MAP kinase kinase kinase, MCK1, is required for cell wall integrity and pathogenicity of the rice blast fungus Magnaporthe oryzae. Mol. Plant Microbe. Interact. 21, 525–534. doi: 10.1094/MPMI-21-5-0525
Kershaw, M. J., and Talbot, N. J. (2009). Genome-wide functional analysis reveals that infection-associated fungal autophagy is necessary for rice blast disease. Proc. Natl. Acad. Sci. U. S. A. 106, 15967–15972. doi: 10.1073/pnas.0901477106
Klionsky, D. J., Abdalla, F. C., Abeliovich, H., Abraham, R. T., Acevedo-Arozena, A., Adeli, K., et al. (2012). Guidelines for the use and interpretation of assays for monitoring autophagy. Autophagy 8, 445–544. doi: 10.4161/auto.19496
Li, Y., Wu, M., Yu, Q., Su, Z. Z., Dong, B., Lu, J. P., et al. (2020). PoMet3 and PoMet14 associated with sulfate assimilation are essential for conidiogenesis and pathogenicity in Pyricularia oryzae. Curr. Genet. 66, 765–774. doi: 10.1007/s00294-020-01055-1
Liu, X. H., Liang, S., Wei, Y. Y., Zhu, X. M., Li, L., Liu, P. P., et al. (2019). Metabolomics analysis identifies sphingolipids as key signaling moieties in appressorium morphogenesis and function in Magnaporthe oryzae. MBio 10, e01467–e01419. doi: 10.1128/mBio.01467-19
LivaK, K. J., and Schmittgen, T. D. (2001). Analysis of relative gene expression data using real-time quantitive PCR and the 2–ΔΔCT method. Methods 25, 402–408. doi: 10.1006/meth.2001.1262
Lv, W., Wang, C., Yang, N., Que, Y., Talbot, N. J., and Wang, Z. (2017). Genome-wide functional analysis reveals that autophagy is necessary for growth, sporulation, deoxynivalenol production and virulence in fusarium graminearum. Sci. Rep. 7:11062. doi: 10.1038/s41598-017-11640-z
MacKinnon, M. A., Curwin, A. J., Gaspard, G. J., Suraci, A. B., Fernández-Murray, J. P., and McMaster, C. R. (2009). The Kap60-Kap95 karyopherin complex directly regulates phosphatidylcholine synthesis. J. Biol. Chem. 284, 7376–7384. doi: 10.1074/jbc.M809117200
McMaster, C. R., and Bell, R. M. (1994). Phosphatidylcholine biosynthesis in Saccharomyces cerevisiae. Regulatory insights from studies employing null and chimeric sn-1,2-diacylglycerol choline- and ethanolamine phosphotransferases. J. Biol. Chem. 269, 28010–28016.
Pham, K. T., Inoue, Y., Vu, B. V., Nguyen, H. H., Nakayashiki, T., Ikeda, K., et al. (2015). MoSET1 (histone H3K4 methyltransferase in Magnaporthe oryzae) regulates global gene expression during infection-related morphogenesis. PLoS Genet. 11:e1005385. doi: 10.1371/journal.pgen.1005385
Pritchard, P. H., Chiang, P. K., Cantoni, G. L., and Vance, D. E. (1982). Inhibition of phosphatidylethanolamine N-methylation by 3-deazaadenosine stimulates the synthesis of phosphatidylcholine via the CDP-choline pathway. J. Biol. Chem. 257, 6362–6367.
Saint-Macary, M. E., Barbisan, C., Gagey, M. J., Frelin, O., Beffa, R., Lebrun, M. H., et al. (2015). Methionine biosynthesis is essential for infection in the rice blast fungus Magnaporthe oryzae. PLoS One 10:e0111108. doi: 10.1371/journal.pone.0111108
Saunders, D. G., Dagdas, Y. F., and Talbot, N. J. (2010). Spatial uncoupling of mitosis and cytokinesis during appressorium-mediated plant infection by the rice blast fungus Magnaporthe oryzae. Plant Cell 22, 2417–2428. doi: 10.1105/tpc.110.074492
Stipanuk, M. H. (2004). Sulfur amino acid metabolism: pathways for production and removal of homocysteine and cysteine. Annu. Rev. Nutr. 24, 539–577. doi: 10.1146/annurev.nutr.24.012003.132418
Talbot, N. J. (2003). On the trail of a cereal killer: exploring the biology of Magnaporthe grisea. Annu. Rev. Microbiol. 57, 177–202. doi: 10.1146/annurev.micro.57.030502.090957
Talbot, N. J., Ebbole, D. J., and Hamer, J. E. (1993). Identification and characterization of MPG1, a gene involved in pathogenicity from the rice blast fungus Magnaporthe grisea. Plant Cell 5, 1575–1590. doi: 10.1105/tpc.5.11.1575
Thines, E., Weber, R. W., and Talbot, N. J. (2000). MAP kinase and protein kinase A-dependent mobilization of triacylglycerol and glycogen during appressorium turgor generation by Magnaporthe grisea. Plant Cell 12, 1703–1718. doi: 10.1105/tpc.12.9.1703
Veneault-Fourrey, C., Barooah, M., Egan, M., Wakley, G., and Talbot, N. J. (2006). Autophagic fungal cell death is necessary for infection by the rice blast fungus. Science 312, 580–583. doi: 10.1126/science.1124550
Wilson, R. A., and Talbot, N. J. (2009). Under pressure: investigating the biology of plant infection by Magnaporthe oryzae. Nat. Rev. Microbiol. 7, 185–195. doi: 10.1038/nrmicro2032
Xu, J. R., Staiger, C. J., and Hamer, J. E. (1998). Inactivation of the mitogen-activated protein kinase Mps1 from the rice blast fungus prevents penetration of host cells but allows activation of plant defense responses. Proc. Natl. Acad. Sci. U. S. A. 95, 12713–12718. doi: 10.1073/pnas.95.21.12713
Yan, X., Que, Y., Wang, H., Wang, C., Li, Y., Yue, X., et al. (2013). The MET13 methylenetetrahydrofolate reductase gene is essential for infection-related morphogenesis in the rice blast fungus Magnaporthe oryzae. PLoS One 8:e76914. doi: 10.1371/journal.pone.0076914
Ye, C., Sutter, B. M., Wang, Y., Kuang, Z., and Tu, B. P. (2017). A metabolic function for phospholipid and histone methylation. Mol. Cell 66, 180–193.e8. doi: 10.1016/j.molcel.2017.02.026
Yue, X., Que, Y., Deng, S., Xu, L., Oses-Ruiz, M., Talbot, N. J., et al. (2017). The cyclin dependent kinase subunit Cks1 is required for infection-associated development of the rice blast fungus Magnaporthe oryzae. Environ. Microbiol. 19, 3959–3981. doi: 10.1111/1462-2920.13796
Keywords: Magnaporthe oryzae , phosphatidylcholine, CDP-cho pathway, gene function, pathogenicity, histone methylation, methionine metabolism
Citation: Xu Z, Tong Q, Lv W, Xiao Y and Wang Z (2023) Phosphocholine cytidylyltransferase MoPct1 is crucial for vegetative growth, conidiation, and appressorium-mediated plant infection by Magnaporthe oryzae. Front. Microbiol. 14:1136168. doi: 10.3389/fmicb.2023.1136168
Received: 02 January 2023; Accepted: 12 April 2023;
Published: 05 May 2023.
Edited by:
Christian Koch, Friedrich-Alexander-Universität Erlangen-Nürnberg, GermanyReviewed by:
Xueming Zhu, Zhejiang Academy of Agricultural Sciences, ChinaCopyright © 2023 Xu, Tong, Lv, Xiao and Wang. This is an open-access article distributed under the terms of the Creative Commons Attribution License (CC BY). The use, distribution or reproduction in other forums is permitted, provided the original author(s) and the copyright owner(s) are credited and that the original publication in this journal is cited, in accordance with accepted academic practice. No use, distribution or reproduction is permitted which does not comply with these terms.
*Correspondence: Zhengyi Wang, emh5d2FuZ0B6anUuZWR1LmNu
Disclaimer: All claims expressed in this article are solely those of the authors and do not necessarily represent those of their affiliated organizations, or those of the publisher, the editors and the reviewers. Any product that may be evaluated in this article or claim that may be made by its manufacturer is not guaranteed or endorsed by the publisher.
Research integrity at Frontiers
Learn more about the work of our research integrity team to safeguard the quality of each article we publish.