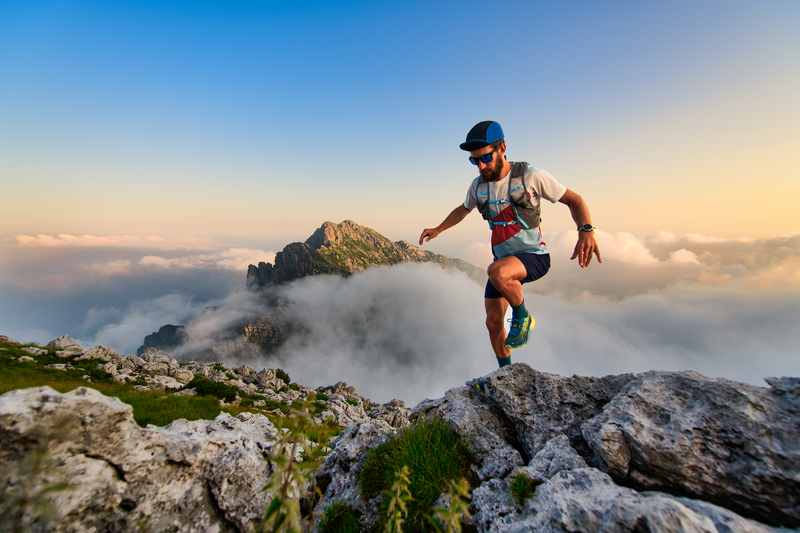
95% of researchers rate our articles as excellent or good
Learn more about the work of our research integrity team to safeguard the quality of each article we publish.
Find out more
ORIGINAL RESEARCH article
Front. Microbiol. , 05 April 2023
Sec. Food Microbiology
Volume 14 - 2023 | https://doi.org/10.3389/fmicb.2023.1135806
This article is part of the Research Topic Bacteriophages in the Fight Against Foodborne Pathogens View all 7 articles
Non-typhoidal Salmonella is the tremendously predominant source of acquired foodborne infection in humans, causing salmonellosis which is a global threat to the healthcare system. This threat is even worse when it is combined with the incidence of multidrug-resistant Salmonella strains. Bacteriophage therapy has been proposed as a promising potential candidate to control a diversity of foodborne infective bacteria. The objective of this study designed to isolate and characterize lytic phages infecting zoonotic multi-drug resistant and strong biofilm producer Salmonella enterica serovar Enteritidis EG.SmE1 and then apply the isolated phage/s as a biocontrol agent against infections in ready-to-eat food articles including milk, water, apple juice, and chicken breasts. One lytic phage (LPSent1) was selected based on its robust and stable lytic activity. Phage LPSent1 belonged to the genus Jerseyvirus within the Jerseyvirinae subfamily. The lysis time of phage LPSent1 was 60 min with a latent period of 30 min and each infected cell burst about 112 plaque-forming units. Phage LPSent1 showed a narrow host range. Furthermore, the LPSent1 genome did not encode any virulence or lysogenic genes. In addition, phage LPSent1 had wide pH tolerance, prolonged thermal stability, and was stable in food articles lacking its susceptible host for 48 h. In vitro applications of phage LPSent1 inhibited free planktonic cells and biofilms of Salmonella Enteritidis EG.SmE1 with a lower occurrence to form phage-resistant bacterial mutants which suggests promising applications on food articles. Application of phage LPSent1 at multiplicities of infections of 100 or 1000 showed significant inhibition in the bacterial count of Salmonella Enteritidis EG.SmE1 by 5 log10/sample in milk, water, apple juice, and chicken breasts at either 4°C or 25°C. Accordingly, taken together these findings establish phage LPSent1 as an effective, promising candidate for the biocontrol of MDR Salmonella Enteritidis in ready-to-eat food.
Salmonella enterica, a gram-negative facultative intracellular rod-shaped bacterium that is a member of the family Enterobacteriaceae, is one of the major widespread foodborne pathogens causing diseases and death worldwide (WHO, 2017). Salmonella enterica has approximately more than 2,600 serovars and roughly all are pathogenic (Porwollik et al., 2004; Gal-Mor, 2019) of which S. Typhi are host-specific, while other serovars like S. Typhimurium and S. Enteritidis are generalists. S. Typhi is associated with typhoid fever, Non-typhoidal Salmonella species (NTS), including S. Enteritidis and S. Typhimurium, mainly cause gastrointestinal infection which may lead to hospitalization and death (Musyoka et al., 2018).
Globally, NTS are the extremely prevalent cause of acquired foodborne infection in humans, causing salmonellosis (Esmael et al., 2021b). Salmonellosis is transmitted in humans through the oral-fecal route by the consumption of contaminated food either of animal origin (milk, meat, poultry, and eggs) or green vegetables contaminated by manure (Youssef et al., 2021). The infection symptoms include diarrhea, fever, vomiting, abdominal crumbs as well many other symptoms which arise one or two days after infection and can be persisted for a week (Wilson and Wilson, 2021). Although symptoms of salmonellosis are mild, and patients normally recover with no therapy, in some cases, it resulted in dehydration that may become severe and lead to hospitalization and death in children and immunocompromised patients (Musyoka et al., 2018). NTS have resulted in persistent infection in sub-Saharan Africa that showed bacteremia and septicemia with a mortality rate of ∼21% (Haselbeck et al., 2017). The World Health Organization (WHO) has declared that NTS results annually in about 94 million hospitalizations and 155,000 deaths worldwide (Majowicz et al., 2010). According to the Centers for Disease Control and Prevention (CDC), there are 23,000 illnesses and about 450 deaths caused by NTS species every year in the United States.
In poultry, Salmonella is identified to be present asymptomatically in the gastrointestinal tracts or generate enteric infection symptoms (Pandey et al., 2021). As a result, the disease remains undetected, and its appearance is related to the human consumption of contaminated food products (Foley et al., 2008). NTS-infected animals can spread the infection through contaminated feeds, the environment, or by direct contact with another infected animal (Atterbury et al., 2007). NTS infections are impacted by two factors: its broad host range and the presence of multi-drug resistance (MDR).
MDR is the antimicrobial resistance displayed by the bacteria to at least one agent in three or more antimicrobial categories. Some Salmonella strains encode several antimicrobial resistance genes that confer an MDR trait against more than one antibiotic. Lately, the occurrence of MDR Salmonella serovars has boosted the malfunction of antibiotic therapies (Medeiros et al., 2011; Agyare et al., 2019). Over several decades, the consumption of low doses of antibiotics in the poultry industry was a general practice (Moore and Evenson, 1946) not only for prophylactic or therapeutic concerns but, also, to promote growth (Waibel et al., 1954; Libby and Schaible, 1955), as and this improper use causes the emergence of MDR. The used antibiotics could not kill the whole gut bacteria, and certain resilient strains might survive and turn out to be resistant. Eventually, the resistant bacteria pass their antibiotic-resistant genes on to other susceptible bacteria.
Depending on the country’s economy, degree of development, livestock farming, and types of animals, antibiotics usage as a growth promoter varies in form and scope (Archawakulathep et al., 2014). The use of antibiotics to promote growth in poultry industry has been banned in the European Union (EU) since 2006, and in the US in 2017 (European Union, 2005; AccessScience Editors, 2017). On the 28th of January 2022, the EU has taken the unprecedented step for sustainable antibiotic-free broilers production as the use of routinely fed a diet of antibiotics is now strictly banned and come into enforce. In the U.S. antibiotics. Although, poultry breeders in low- and middle-income countries, where antibiotic laws are not strictly enforced, still employ antibiotics intentionally to promote growth without any veterinary prescription (Maron et al., 2013). In Egypt, previous studies reported the incidence of MDR NTS contaminating different food articles (Diab et al., 2019; Youssef et al., 2021). Therefore, NTS human infections have become a major threat to healthcare systems around the world due to the annual increase in morbidity rates.
In food articles and industrial facilities, Salmonella frequently lives not only as free planktonic cells but also as sessile multicellular surface-associated forms known as biofilms. Salmonella virulence is attributed to the formation of biofilms, because bacteria in the complex biofilm communities are more resistant to antibiotics, resulting in a chronic Salmonella carries infection (Gonzalez-Escobedo et al., 2011; Gonzalez-Escobedo and Gunn, 2013; Zeineldin et al., 2023). Standard control procedures such as using special preservatives and heat treatment in liquid food are predominantly applied to control Salmonella load in food products and to reduce biofilms (Pérez-Díaz et al., 2008; Neetoo and Mahomoodally, 2014; Chylkova et al., 2017; Musyoka et al., 2018). Although these strategies are effective, the concern about the undesirable side effects given by the chemical stabilizers is discouraging (Pawlowska et al., 2012). In addition, heat treatment results in the degradation of important nutrients. Moreover, the application of antibiotics in food products is generally deterred because of non-specific antibacterial activity, long-term environmental stability, and the prevalence of MDR bacteria (Medeiros et al., 2011; Agyare et al., 2019).
On the contrary, due to bacteriophages’ nature of being obligate parasites, self-replicating ability, and host specificity, they are deemed as attractive antibacterial agents. Bacteriophages have received much more interest over antibiotics as a novel natural approach to control bacteria in food products and to ease their biofilms as well (Goodridge and Bisha, 2011; Matsuzaki et al., 2014; Lin et al., 2017; Esmael et al., 2021b). Moreover, phages do not cause hurt to eukaryotic cells (Kutter et al., 2010; McCallin et al., 2013) and no reports have described any bacteriophage infection in humans so far (Kutter et al., 2010; Keen et al., 2015). Therefore, phage treatment appears to be a good candidate as an antibacterial control in food. Different studies showed that phages were effective to control foodborne bacteria in different food materials (O’Flynn et al., 2006; Spricigo et al., 2013; Bao et al., 2015; Huang et al., 2018a; Esmael et al., 2021b; Alharbi et al., 2022).
Likewise, certain phages have been confirmed, by the FDA, as Generally Recognized as Safe (GRAS), being commercially available and used to combat Salmonella and their biofilms in food products, e.g., Armament, Salmonelex, SalmoFresh, and many others (Goodridge and Bisha, 2011; Sukumaran et al., 2015; Moye et al., 2018).
Earlier, five Salmonella enterica serovars were isolated from a poultry farm in Benha city, Qalubiya governorate, Egypt. Antibiotic susceptibility testing of these bacteria identified two MDR isolates (S. Enteriditis EG.SmE1 and S. Typhimurium EG.SmT3). In a previous study, we described the isolation, characterization, and application of three lytic Salmonella phages against S. Typhimurium EG.SmT3 to combat food-borne salmonellosis in various food articles. In this study, keeping in view the remarkable efficiency of lytic bacteriophages in limiting bacteria, we describe the efficacy of the newly isolated phage LPSent1 as a good candidate against S. Enteriditis EG.SmE1. Moreover, we investigate its efficacy to control zoonotic MDR Salmonella and their biofilms in ready-to-consume food articles including milk, water, Apple Juice, and chicken breasts.
Bacteria were preserved at −80°C in Brain-Heart-Infusion broth supplemented with 20% (v/v) glycerol. Fresh cultures were prepared prior to each experiment by inoculating a single colony into 5 mL tryptic soy broth (TSB, DifcoTM, USA) and incubating at 37°C for 16 h while shaking at 200 rpm.
The Kirby-Bauer disk diffusion method (Esmael et al., 2020, 2021a,2021b) was employed to check the antibiotic sensitivity pattern on Mueller-Hinton agar medium against a selection of thirteen antibiotics (Oxoid, Hampshire, UK): ampicillin (10 μg), amoxicillin (25 μg), ciprofloxacin (5 μg), amikacin (30 μg), gentamycin (10 μg), streptomycin (10 μg), tetracycline (30 μg), chloramphenicol (30 μg), aztreonam (30 μg), trimethoprim-sulfamethoxazole (25 μg), cephalexin (30 μg), cefoxitin (30 μg), and ceftriaxone (30 μg). Inhibition zones were measured and results were expounded according to the Clinical and Laboratory Standards Institute (CLSI) (Clinical and Laboratory Standards Institute, 2019). Tested bacteria was defined as MDR when acquired non-susceptibility to at least one agent in three or more antimicrobial categories.
The potential of biofilm production by the five Salmonella serovars in the current study was assessed as described previously (Stepanovic et al., 2007; Esmael et al., 2021a). A final concentration of 4 log10 CFU/mL of fresh Salmonella cultures was inoculated individually into Luria broth (LB) medium without NaCl in each well of a 96-well microtiter plate and incubated at 37°C for 24 h. Negative control wells containing Salmonella-free LB were involved. Consequently, the plates were emptied to remove the free planktonic cells, rinsed three times gently with phosphate-buffered saline (PBS), and air-dried. The residual attached bacteria were fixed with 98% methanol for 10 min., the methanol was decanted, and the plates were again allowed to air-dry. The fixed bacteria were then stained with 1% crystal violet for 45 min., rinsed three times gently with water to remove the excess crystal violet, then the stained cells were solubilized in 33% acetic acid.
A microplate reader (BMG LABTECH GmbH, Allmendgrun, Germany) was used to measure optical densities at 600 nm. To evaluate and categorize biofilm-generating serovars, an optical density cutoff (ODc) representing the background noise, or the threshold was used as described by Stepanovic et al. (2007), where ODc = average OD of negative control + (3 × SD of negative control). Bacteria were categorized into non-biofilm producers (OD ≤ ODc), weak biofilm producers (2 × ODc ≥ OD > ODc), moderate biofilm producers (4 × ODc ≥ OD > 2 × ODc), and strong biofilm producers (OD > 4 × ODc).
The MDR and strong biofilm producer Salmonella enterica serovar Enteritidis EG.SmE1 was selected for bacteriophage isolation, purification, and propagation. Before environmental screening for lytic phages, S. Enteriditis EG.SmE1 was examined for lysogens (prophages) using chemical mitomycin C-mediated induction as described before (Klieve, 2005; Esmael et al., 2021a,b). Briefly, S. Enteriditis EG.SmE1 was grown in 5 mL of TSB medium until a mid-log phase (0.3 at 600 nm), then treated with mitomycin C (Sigma-Aldrich, St. Louis, MO, USA) at a final concentration of 0.2 μg/mL. The treated bacteria were then incubated at 37°C and bacterial growth was followed for 16 h by measuring the absorbance at OD600nm. At various time points (0.5 h, 1 h, 1.5 h, 2 h, 6 h, 10 h, 12 h, and 16 h), 500 μL aliquots of the treated bacteria were collected, cell debris was removed by centrifugation at 8,000 × g for 20 min, and then the lysates were passage through a 0.45 μm membrane filter. Collected lysates were then tested by spotting 10 μL from each lysate onto a lawn of S. Enteriditis EG.SmE1 and the plates were then incubated at 37°C for 24 h.
Several environmental samples, including raw sewage water, agricultural farm ditches, and chicken feces, were collected and screened for bacteriophages against S. Enteriditis EG.SmE1 as illustrated before (Akhtar et al., 2014; Esmael et al., 2021b; Teklemariam et al., 2022). The collected water samples were processed to remove solids and cellular entities by centrifugation at 10,000 × g for 10 min and then were passage through 0.22 μm membrane filters (Mixed Cellulose Ester, MF-Millipore, Burlington, MA, USA). Chicken feces (10 g) were suspended in 50 mL TSB and were then administered in the same way as the water samples. Lytic phages were isolated from the processed samples via the enrichment method as described previously (Van Twest and Kropinski, 2009). In brief, 5 mL of sterile 2 × TSB medium pre-inoculated with 150 μL of fresh S. Enteriditis EG.SmE1 was mixed individually with 5 mL of the 0.22 μm-filtered samples, the tubes were then incubated at 37°C with continuous shaking at 200 rpm for 24 h. Enriched samples were later centrifuged at 10,000 × g for 10 min, the supernatants were collected and filtered using 0.22-μm membrane filters.
The presence of lytic phages was detected by spotting 10 μL of the enriched supernatants on lawns of S. Enteriditis EG.SmE1, then the plates were incubated at 37°C and were then observed for the formation of any lysis (Kropinski et al., 2009; Esmael et al., 2021b). To resuspend phages in the lysis zones, about 5 mL of filter-sterilized phage diluent (Salt-magnesium buffer) was added on top of the plate and gently shaken overnight at room temperature. Plate lysates were cleaned or purified through three rounds of single-plaque purification using the double-layer agar (DLA) method (Clokie and Kropinski, 2009). In brief, a single phage plaque was picked using sterile toothpicks, resuspended in 100 μL of SM buffer, and then kept overnight at room temperature. The process of isolating a single plaque and plating using the DLA method was repeated three times successively. The plate lysate was then collected, centrifuged at 4,000 × g for 5 min to remove any remaining debris, and stored at 4°C.
The isolated phage was propagated and concentrated as mentioned earlier (Yamamoto et al., 1970; Esmael et al., 2021b). About 100 mL of a mid-exponential culture (0.3 at 600nm) of the indicator S. Enteriditis EG.SmE1 was inoculated with a purified phage suspension at a multiplicity of infection (MOI) of 1, then the mix was incubated for 24 h at 37°C while shaking at 200 rpm. Then the lysate was centrifuged at 4,000 × g for 5 min to remove the bacterial debris., free phages in the supernatant were then treated with 10% (w/v) Polyethylene glycol (PEG) 6,000 overnight at 4°C to permit phage precipitation on the PEG. Then the phage-PEG complex was pelleted by centrifugation at 10,000 × g for 30 min at 4°C, then the supernatant was gently poured off and the phage-PEG pellets were resuspended in SM buffer. A chloroform-based extraction method was deployed to release phages from the PEG particles as described previously (Yamamoto et al., 1970). Finally, the concentrated phages were filter-sterilized using 0.22-μm membrane filters, aliquoted into cryotubes, and stored at 4°C. The phage stock (PFU/mL) was titrated in triplicate using S. Enteriditis EG.SmE1 by the DLA method.
Morphological features of the isolated phage were determined by transmission electron microscope (A JEOL JEM-2100), located at the Electron Microscope Facility, Al-Mansoura University, Egypt. Ten microliters of a highly purified phage preparation (∼1012 PFU/mL) were transferred and fixed for 5 min. onto the surface of carbon-coated copper TEM film (Electron Microscopy Sciences) and were negatively stained with 2% (w/v) phosphotungstic acid, pH 7.2, for 1 min (Ackermann, 2012). After air-drying at room temperature for 1 h, the stained phage particles were observed under TEM.
The growth curve of the isolated phage was evaluated as explained earlier (Esmael et al., 2021a,b). Salmonella Enteriditis EG.SmE1 (1 × 107CFU/mL) was challenged (at 37°C while shaking at 200 rpm) with the isolated phage at an MOI of 1 for 5 min., then centrifuged at 5000 × g for 5 min to remove free unabsorbed phages in the supernatant. The pellet was then washed twice and then resuspended in 10 mL of sterile TSB, again reincubated at 37°C while shaking at 200 rpm. Aliquots of the resuspended bacteria were collected every 5 min for 1 hour post-infection (p.i.) and phage titers were counted using the double-layer agar (DLA) method.
The temperature stability of phage LPSent1 (10 log10 PFU/mL) was assessed at 30, 40, 50, 60, 70, and 80°C at pH 7.0 in an adjusted water bath incubator for either 30 min or 60 min as described before (Esmael et al., 2021a,b). For pH stability, phage LPSent1 (10 log10 PFU/mL) was diluted in SM buffer at different pH ranges (From 2-13) and incubated at 37°C for 24 h. Instantly after the thermal or pH treatment, serial dilutions of the treated phage were prepared, and the number of plaques was measured using the DLA assay. Phage residual percentage after each treatment was measured as follows:
Residual activity (%) = (phage count (PFU/mL) after treatment÷ Original phage count (PFU/mL)) × 100.
LPSent1 genomic DNA was isolated using the direct plaque sequencing method as described before by Kot et al. (2014). The concentration of the isolated DNA was estimated using a NanoDrop ND-1000 UV-Vis spectrophotometer. Phage genomic DNA was stored at −20°C for further analysis. Phage DNA libraries were prepared using the Illumina DNA Prep kit and IDT 10 bp UDI indices, and sequenced on an Illumina NextSeq 2000, producing 2 × 151 bp reads. Demultiplexing, quality control, and adapter trimming were performed with bcl-convert (v3.9.3), and short reads were assembled with Unicycler (Wick et al., 2017). The assembled genome was annotated with prokka (v1.14.5) (Seemann, 2014) and the NCBI open-reading frame (ORF) finder search server. Putative functions of the identified coding sequences (CDSs) in each ORF were evaluated using the BLASTp search algorithm on the NCBI website. Detection of genes encoding tRNAs was evaluated using tRNAscan-SE v.1.3.1 (Lowe and Eddy, 1997). Assembled LPSent1 genomic DNA was searched for antimicrobial resistance genes using the ResFinder web service (Florensa et al., 2022). A genomic circular map of phage LPSent1 was built up and designed using CG view (Stothard and Wishart, 2005). The complete genome sequence of phage LPSent1 has been deposited in the GenBank database under the accession number OQ091358.
MAFFT online server (Katoh et al., 2019) was used to align the nucleotide sequences of phage LPSent1 complete genome, and terminase large subunit gene against 13 phages in the Jerseyvirus genus, the formed alignment was then imported into the MEGA X program version 10.2.4 to construct the phylogenetic tree using the neighbor-joining method and 1000 bootstraps (Kumar et al., 2018).
The host range of the phage LPSent1 was revealed against a group of 18 Salmonella strains and 6 non-Salmonella strains (Supplementary Table 1) as described earlier (Clokie and Kropinski, 2009; De Melo et al., 2019) with some modifications. The first set of analyses was performed using undiluted phage preparation, aliquots of 10 μL of phage stock at a titer of 1 × 107 PFU/mL were spotted, in triplicates onto the surface of the agar overlay of the tested bacteria. The plates were incubated for a period of up to 24 h at 37°C, then the plates were observed for the formation of inhibition lytic zones. The second set of analyses using bacterial isolates that showed clear or turbid zones was done using the same method described above, except using serially diluted (up to 107 dilutions) phage preparations other than the undiluted stock. The plates were incubated as in the first set while this time, the plates were observed for the formation of typical plaques.
The efficiency of plating (EOP) of the isolated phage on the susceptible isolates was calculated as described before (Mirzaei and Nilsson, 2015; Huang et al., 2018a; Esmael et al., 2021b). A volume of 50 μL of the diluted phage stock (containing about 50 PFU) was mixed with 200 μL of the susceptible bacterial culture at the mid-exponential phase of growth, then the samples were assayed using the DLA method. EOP value was estimated, as follows:
EOP = number of PFUs on the tested bacteria/number of PFUs on S. Enteriditis EG.SmE1.
The incidence of the development of bacteriophage-insensitive mutants (BIMs) was assessed as previously described (O’Flaherty et al., 2005). Phage LPSent1 was mixed with S. Enteriditis EG.SmE1 at an MOI of 10 and incubated for 20 min at 37°C, then, the suspension was diluted and platted using the DLA assay. Plates were incubated overnight at 37°C, any developed colonies confronted were counted and BIM frequency (Bacterial viable counts after phage infection divided by the original bacteria count) was calculated. Experiments were conducted in triplicate.
The bactericidal activity of phage LPSent1 against free cells of S. Enteriditis EG.SmE1 was determined at different MOIs as described before (Huang et al., 2018a; Esmael et al., 2021a,b). Briefly, 100 μL of S. Enteriditis EG.SmE1 culture (7 log10 CFU/mL) at the exponential phase was transferred into each well of a 96-well microtiter plate, then challenged with phage LPSent1 at an MOI of 0.1, 1, 5, or 10. Negative control wells contained phage-free S. Enteriditis EG.SmE1. Plates were incubated at 37°C in a static condition and bacterial growth was monitored for 6 h post-infection by measuring optical densities at 600 nm using a microplate reader (680 XR reader, Bio-Rad, Hercules, CA, USA).
The efficacy of phage LPSent1 to inhibit biofilms of S. Enteriditis EG.SmE1 was measured by the quantitative colorimetric method as described before (Cerca et al., 2005) with some adaptations. To each well of a 96-well flat-bottomed microtiter plate, 100 μL of S. Enteriditis EG.SmE1 (final count of 6 log10 CFU/mL) was inoculated into sterile LB medium without NaCl for 24 h at 37°C, then the free unattached planktonic cells were gently aspirated. Then bacteria in the wells were challenged with phage LPSent1 at different MOIs (5, 10, 50). Phage LPSent1 was diluted in TSB and 100 μL aliquots of the diluted phage were added to each well at the corresponding MOI. Positive control wells received equivalent volumes of TSB. In a similar experiment, phage LPSent1 was added to wells every 6 h gently substituting the well-free materials (containing media, free phages, and planktonic Salmonella cells). The plates were further incubated under the same conditions, then the free materials of each plate were aspirated, and the biomass of the established biofilms was quantified. The plates were rinsed three times with phosphate buffer saline, then the plates were air-dried, fixed, stained with crystal violet, and analyzed as mentioned earlier. The absorbance (at 600 nm) was measured at 6, 12, 18, and 24 h using a microplate reader (BMG LABTECH GmbH, Allmendgrun, Germany).
Stability of phage LPSent1 in milk, apple juice, water, and on chicken breast, experiments were conducted for two days as described previously (Huang et al., 2018b; Islam et al., 2019; Esmael et al., 2021b) at two different temperatures 4°C and 25°C representing food storage and food processing temperatures. Cow pasteurized milk, apple juice, and boneless chicken breasts were bought from local retailers while the water utilized was sterile municipal faucet water. Briefly, 8 log10 PFU/mL of the isolated pure phage was mixed with sterile milk, water, and apple juice, the same titer was spotted on the surface of sterile chicken breast pieces (1 cm2). The phage-treated food samples were then incubated for 48 h at either 4°C or 25°C, and aliquots were taken at 0, 2, 6, 12, 16, 24, 36, and 48 h, and phage titers were enumerated using DLA method.
The efficacy of applying phage LPSent1 as a biocontrol against S. Enteriditis EG.SmE1 in the above-mentioned food matrices was performed by measuring the recovered Salmonella load post-phage treatment as described previously (Huang et al., 2018b; Islam et al., 2019; Esmael et al., 2021b). To assay on milk, water, and apple juice, 10 μL of fresh S. Enteriditis EG.SmE1 (final count of 5 log10 CFU/mL) was added individually to each food article, then the phage was added at a MOIs of 100 or 1000. Chicken breast slices (1 cm2 each) were infected with S. Enteriditis EG.SmE1 (5 log10 CFU/mL), slices were air-dried for 30 min., and subsequently, were dipped in the phage solutions at MOIs of 100 or 1000. Equivalent volumes of SM buffer were added instead of the phage for the negative control treatments. for 48 h at either 4°C or 25°C, aliquots were taken at 0, 2 h, 6 h, 12 h, 16 h, 24 h, 36 h, and 48 h to count the recovered bacteria as explained previously (Islam et al., 2019).
Student’s t-test was used to determine the significance of differences; P-values of ≤ 0.05 were considered significant.
The universal infection problem caused by Salmonella spp. denotes a considerable fraction of bacterial infections developed by the consumption of polluted food and water. The World Health Organization declared Salmonella is 1 of 4 key global reasons of diarrheal illnesses, and almost 106 human cases caused by Salmonella spp. in the U.S. are foodborne (Scallan et al., 2011). The antibiotic sensitivity examination of the five isolates of Salmonella enterica serovars, previously isolated from a poultry farm in Egypt, was estimated against a range of thirteen antibiotics belonging to 10 different classes (Table 1).
Table 1. Antibiotic susceptibility pattern of five Salmonella enterica serovars against a selection of thirteen antibiotics.
The antibiogram data identified that the five Salmonella isolates resisted at least 23% of the examined antibiotics, however, a high resistance percentage of 61.5% and 53% for S. Enteriditis EG.SmE1 and S. Typhimurium EG.SmT3 respectively. Remarkably, all the tested bacteria were identified as MDR because they resisted at least one antibiotic within more than three different classes. MDR Salmonella have been isolated previously from Egypt (Abdelhakim et al., 2011; Abdel-Maksoud et al., 2015; Mahmoud et al., 2018; Merwad and Abdel-Haliem, 2018; Esmael et al., 2021b; Abd-Elghany et al., 2022). In Egypt there are no boundaries on antibiotic remedies and antibiotics can be acquired easily without any medical prescription from drugstores and pharmacies (Sobhy et al., 2012; Sabry et al., 2014; Awad et al., 2016; Esmael et al., 2020). Moreover, the poultry industry is one of the main foundations of contamination with veterinary antibiotics into the surrounding environment (Dahshan et al., 2015), as a result, the emergence of MDR bacteria is unceasing very fast.
Biofilms occur as cumulative bunches of densely populated bacteria that could be from single or multiple species. Biofilms of Salmonella help for the spread and persistence since they resist antibiotics, disinfectants, physical and chemical stresses (Humphrey, 2004; Spector and Kenyon, 2012) and lead to numerous foodborne infection outbreaks (Corcoran et al., 2014). The capability of the five Salmonella enterica serovars to form multicellular communities or biofilms was evaluated (Figure 1). The optical density of an uninoculated tryptone soy broth (negative control; bacteria-free medium) was used as a cutoff value (ODc) and was measured to be 0.06. Quantitative biofilm data generated by the five Salmonella serovars identified three categories based on their biofilm potency. One isolate, S. Enteritidis EG.SmE2, was identified as a weak biofilm producer (0.12 ≥ OD > 0.06). Two isolates, S. Typhimurium EG.SmT1 and S. Typhimurium EG.SmT2, were found to be moderate biofilm producers (0.24 ≥ OD > 0.12). Two isolates, S. Enteritidis EG.SmE1 and S. Typhimurium EG.SmT3, were identified as strong biofilm producers (OD > 0.24). Bacterial biofilms can be generated on food bodies, contaminated food utensils, and water. It is worth noting that the generation of bacterial biofilms, as a bacterial virulence factor, is of great concern as it has been linked to the increasing rates of antimicrobial resistance (Corcoran et al., 2014) which make the traditional treatment strategies not effective anymore.
Figure 1. Quantitative evaluation of biofilm formation by five Salmonella serovars. Bacteria in the current study were classified into three categories; weak biofilm producers (S. Enteritidis EG.SmE2), moderate biofilm producers (S. Typhimurium EG.SmT1 and S. Typhimurium EG.SmT2), strong biofilm producer (S. Enteritidis EG.SmE1 and S. Typhimurium EG.SmT3). The negative control represents the optical density cutoff value. Data shown represent the average of three reads ± standard deviations.
The foregoing experiments revealed that S. Enteritidis EG.SmE1 and S. Typhimurium EG.SmT3 are extreme MDRs and strong biofilm producers. Traditional interference approaches for opposing MDR foodborne Salmonella are not effective enough to resolve the impasse of food quality and food safety. Hence, non-antibiotic biocontrol alternative therapies are of great interest to eradicate pathogenic bacteria from raw and retail food products. Bacteriophages are diverse and ubiquitous in every ecosystem with nearly 1031 virions on the earth (Rohwer and Segall, 2015; Othman et al., 2021; Abo-ELmaaty et al., 2022). Bacteriophages display a robust lytic efficacy against their bacterial host regardless of their antibiotic resistance pattern and can be a promising potential candidate to solve the dilemma (Esmael et al., 2021a,b; Teklemariam et al., 2023).
Previously we isolated three lytic bacteriophages targeting S. Typhimurium EG.SmT3 and the efficacy of those phages to combat Salmonella in food were established (Esmael et al., 2021b), therefore, S. Enteritidis EG.SmE1 was targeted in the current study as a reference host for the isolation of lytic bacteriophages. S. Enteritidis EG.SmE1 was prophage-free as validated using a mitomycin-C induction experiment, which advocated that it is an appropriate isolation host for lytic phages.
A total of 3 lytic bacteriophages were effectively isolated from three different sites; phages LPSent1 and LPSent2 were isolated from raw sewage water, while phage LPSent3 was isolated from a wastewater treatment plant. The three isolated phages showed remarkable discrepancies in plaque sizes and morphology. Phage LPSent1 formed a larger and clear plaque with distinct edges with a diameter of 1 mm. Likewise, phages LPSent2 and LPSent3 formed clear plaques of a diameter of 0.6 and 0.5 mm, respectively. To pick out the highly efficient phage, lytic activity was evaluated against S. Enteritidis EG.SmE1, as shown in Figure 2A. The data indicated that the three phages had inhibited S. Enteritidis EG.SmE1 growth 2 h p.i.; but, phages LPSent2 and LPSent3 lost their activity by 2.5 h p.i. Phage LPSent1 was robust and maintained a stable lytic activity, therefore, it was selected for further analysis.
Figure 2. (A) Lysis ability comparison of the three isolated phages on S. Enteritidis EG.SmE1 as a host at an MOI of 1 in TSB. (B) Growth-kinetics of phage LPSent1 on S. Enteritidis EG.SmE1, phage burst size was calculated as 112 PFU/cell, L refers to the latent period. (C) Transmission electron micrograph of phage LPSent10, scale bar = 100 nm. Data shown in (A,B) are the mean of three replicates and error bars show the standard deviation in the values.
In order to make the most of the benefits of bacteriophage-based applications, the cautious choice of the applicant phages is the primary point in the course and is vital for their effective application to improve food protection. We characterized phage LPSent1 to tailor the treatment or the application protocol. In addition, it emphasizes that phage LPSent1 does not have any resistance or pathogenic traits to the inhabitant human microbiota.
To examine phage LPSent1 infection cycle, a one-step growth curve was performed as shown in Figure 2B. Growth kinetics at MOI of 1 indicated a short latent period of 30 min and the phage required about 60 min to complete its infection cycle with an average burst size of 112 phages per single infected bacterium. Parameters like latency period and burst size have been formerly defined as appropriate to depict the lytic capacity of a phage (Rivera et al., 2022).
TEM examination showed that phage LPSent1 had an isometric capsid along with a contractile tail, with fixation structures (Figure 2C). Phage LPSent1 had an icosahedral head with a diameter of 89 ± 2.10 nm, the tail length was around 203.01 ± 2.77 nm. These results proposed that it has a siphovirus-like morphotype with a characteristic icosahedral head, collar, long non-contractile tail, tail fibers, base plate, and a spike.
Environmental stability is a limiting factor that confers an advantageous criterion of a phage and defines the aptitude to endure efficacy if extra heat or pH is to be added at any step during the decontamination process. Thermal and pH tolerance of phage LPSent1 was calculated by measuring the residual phage titer after incubation under a range of temperature and pH values (Figure 3). Phage LPSent1 is a thermostable bacteriophage with a tolerance range from 30°C to 70°C (Figure 3A). Phage LPSent1 significantly lost about 85% of its initial titer when heated at 80°C for 30 min. No viable LPSent1 plaques were recovered upon heating at 80°C for 60 min.
Figure 3. Thermal and pH stability test of phage LPSent1. (A) Thermal stability of LPSent1. (B) pH stability of LPSent1. Temperature stability tests were performed at 40, 50, 60, 70, and 80°C for 30 min or 60 min at pH 7, the control temperature was set at 30°C for 30 min or 60 min. pH experiments were performed at pH range 2-13 for 24 h at 37°C, pH 7 represented the control value around which the deviations were measured. The data (n = 3) displayed the percentages of the residual phages after each treatment, as normalized from the control ± standard error and were analyzed by Student’s t-test; *indicates significantly different from the controls, p ≤ 0.05.
Phage LPSent1 also exhibited a high degree of pH stability throughout a pH range of 4-12 for 24 h (Figure 3B). LPSent1 titers were significantly dropped totally at extremely acidic or alkaline pH values, as no visible plaques were recovered at pH < 4 or > 12. Earlier studies displayed that most lytic bacteriophages are highly stable at pH < 4 or > 9 (Chow and Rouf, 1983; Woo and Ahn, 2014; Jung et al., 2017). As a result, phage LPSent1 can be applied in a wide range of foods due to its broad thermal stability and pH stability.
The host range of phage LPSent1 was evaluated using undiluted and diluted phage stocks against a group of 18 Salmonella strains and 6 non-Salmonella strains as shown in Table 2. Spotting undiluted phage on the tested bacteria resulted in clear or turbid zones in 13 strains of the tested Salmonella strains (n = 18). Out of those 13, only 9 strains showed remarkable plaques when challenged with the diluted phage stock. The formation of individual plaques, using diluted phage stock, confirmed the susceptibility of 9 strains to phage LPSent1. However, the strains that initially showed turbidity or clear zones, when challenged with the undiluted phage stock, but could not form individual plaques, with diluted phage stock, were likely due to toxic bacteriocins produced during phage preparation or because of the disruption of bacterial cell membranes (Rendueles et al., 2022). Neither the undiluted nor the diluted phage stocks preparations broke the boundary of the genus as they could not lyse any of the 6 non-Salmonella strains tested.
The efficiency of plating (EOP) of phage LPSent1 was calculated for the 9 susceptible strains that formed visible plaques as shown in Table 2. Other than the reference host, phage LPSent1 showed a high efficiency (0.5-1) against all the tested S. Enteritidis strains but moderate to low EOP was detected against S. Typhimurium or S. Kentucky strains. The results showed that phage LPSent1 had a robust lytic activity with a wide host range against several strains of S. Enteritidis, S. Typhimurium and S. Kentucky. Bacteriophage Felix 01 is a good example of a wide host range; it lyses nearly 98.5% of the tested Salmonella (Welkos et al., 1974). However, using a phage cocktail might solve the limitation of a narrow host range bacteriophages.
The Illumina NextSeq 2000 was used to sequence a dsDNA genome of 51,432 bp for phage LPSent1 and it is displayed in a linear topology in Figure 4A. Most of the sequenced phages in the genebank databases have linear dsDNA genomes and are members of the order Caudovirales (Hatfull and Hendrix, 2011).
Figure 4. Phage LPSent1 whole genome analysis. (A) The LPSent1 genome organization represented as a circle. The 59 CDSs are represented as blue or red arrows showing the predicted genes transcribed clockwise (outer side) and counterclockwise (inner side) along the genome. The genome map is a circle, but there is a break at the 12 o’clock position (at the dotted straight red line) because the phage genome is a linear molecule. CDSs with known functions (blue arrows) are labeled with their putative functions along with their position; however, other non-labeled CDSs represent hypothetical proteins (red arrows). (B) Multiple alignment of phage LPSent1 with the most similar phage (phage S55) using progressive mauve alignment on default settings for Salmonella phage LPSent1 (top) and Salmonella phage S55 (bottom). The degree of DNA sequence similarity is indicated by the height of each colored block. Homologous regions are connected by lines between genomes, and blocks below the center line in Salmonella phage S55 indicate sequences with inverse orientation in comparison to phage LPSent1 arrangement. White spaces within blocks represent small-localized areas of the genome sequences that were not aligned.
Analysis of the nucleotide composition of phage LPSent1 indicated a G + C content of 49.1% and a total of 57 putative open reading frames (ORFs) were identified (using the standard genetic code and ATG as initiation codon). Thirty ORFs are located on the leading strand and 27 ORFs are on the complementary strand, the minimum and maximum lengths of ORFs are 177 and 2,463 bp, respectively. BLASTp search annotated 26 ORFs encoded putative hypothetical proteins with unknown functions, while 31 ORFs wew homologus to functional proteins in the GenBank database in which have a high identity to annotated proteins of phages in Jerseylikevirus the genus.
The LPSent1 genome functionally encodes genes that include DNA replication/regulation, packaging, host cell lysis, and structural proteins (Supplementary Table 3 and Figure 4A). Phage LPSent1 did not encode any virulence genes, integrases, antimicrobial-resistant genes, or lysogenic genes which is a chief principle for phages proposed to be used in biocontrol applications (Omwandho and Kubota, 2010; Mahony et al., 2011; Esmael et al., 2021b). Previous studies reported the efficacy of lytic phages in the Siphoviridae family to combat different Salmonella serovars (Huang et al., 2018b). These indications specify that phage LPSent1 is considered a potentially promising candidate to biocontrol the MDR S. Enteritidis EG.SmE1 in different food matrices.
To identify the relationship between phage LPSent1 with other phages, BlastN comparison search of the non-redundant database at the NCBI was accomplished. LPSent1 genome sequence identified a 73% nucleotide homology (Figure 4B and Supplementary Table 2) with the previously sequenced Salmonella phage S55 belonging to the genus Jerseyvirus within the Jerseyvirinae subfamily.
Neighbor-joining (NJ) phylogenetic trees between phage LPSent1 and 12 additional phages in the Jerseyvirus genus were constructed based on (a) terminase large subunit (b) whole genome sequence comparisons in order to further examine and confirm the taxonomic rank of phage LPSent1. The phylogenetic tree constructed using the terminase large subunit gene (Figure 5A) showed that phage LPSent1 was closely linked to that of Salmonella phage S55 (MT653137.1). However, the phylogenetic tree inferred relying on the whole genome sequences (Figure 5B) showed the formation of an isolated phylogenetic position of phage LPsent1 within the unclassified Jerseyvirus clade. The phylogenetic analyses supported that phage LPsent1 is a novel species within the Jerseyvirus genus.
Figure 5. Neighbor-joining phylogenetic tree based on (A) terminase large subunit gene, (B) complete genome sequences of phage LPSent1 and related phages in Jerseyviruses. Numbers are shown next to the branches indicated by the percentage of replicate trees of the bootstrap test (1000 replicates).
Susceptibility of planktonic cells of S. Enteritidis EG.SmE1 against phage LPSent1 was investigated at different MOIs for 6 h P.I. (Figure 6). The results showed that the efficacy of phage LPSent1 to inhibit the growth of planktonic cultures of S. Enteritidis EG.SmE1 is more robust in high MOIs as compared with the uninfected control. Additionally, the disruption lytic activity was retained for 6 h p.i. It is worth mentioning that the infection at all MOIs resulted in an extended lag phase up to 2 h post-infection, demonstrating that phage LPSent1 had an inhibition effect on S. Enteritidis EG.SmE1. Challenging with high phages MOIs increase the likelihood of phages to attach to the receptor sites on their hosts and accordingly enhance their lytic activity to eliminate large numbers of the host within a short time (Tanji et al., 2004; Bai et al., 2019; Esmael et al., 2021b).
Figure 6. In vitro virulence of phage LPSent1 on free planktonic cells of S. Enteritidis EG.SmE1 at different multiplicity of infections (MOIs). The lysis activity of phage LPSent1 was evaluated at MOIs of 0.1, 1, 5, or 10. Bacterial cells were incubated for 24 h at 37°C, bacterial growth was monitored for 6 h by measuring optical density at 600 nm using a microplate reader (680 XR reader, Bio-Rad, Hercules, CA, USA). Data shown are the means of three replicates and error bars show the standard deviation in the values.
The ability of S. Enteritidis EG.SmE1 to recover after initial infection at lower MOIs might be likely because of the emergence of bacteriophage-insensitive mutants (Guenther et al., 2012). One of the foremost worries of phage applications is the formation of Bacteriophage Insensitive (BIM) (Coffey et al., 2010). The frequency of S. Enteritidis EG.SmE1 to form BIM was assessed after incubation with phage LPSent1. The experiment showed that S. Enteritidis formed lower resistant mutants (1.34 × 10–11) against phage LPSent1, proposing that it might overcome the host-resistant mechanism (Labrie et al., 2010).
The effectiveness of phage LPSent1 to disrupt surface-attached biofilm of S. Enteritidis EG.SmE1 was evaluated for 24 h (Figure 7) at different MOIs (5, 10, and 50). The quantity of viable S. Enteritidis EG.SmE1 cells (embedded in the biofilm-recovered solution) were reduced following either single or multiple phage applications. A single application of phage LPSent1 (Figure 7A) resulted in a significant inhibition in the established biofilm of S. Enteritidis EG.SmE1 following phage infection at 6 h. Interestingly, biofilm eradication rates were significantly higher when repeated applications of phage LPSent1 (Figure 7B) were used as compared to a single application. In addition, in either treatment, the higher the phage MOIs, the higher the biofilm eradication rate was detected, with an MOI of 50 displaying the highest reduction rates. Many previous studies advocate the efficiency of bacteriophages to eradicate Salmonella biofilms (Islam et al., 2019; Esmael et al., 2021b; Hosny et al., 2022; Mondal et al., 2022).
Figure 7. In vitro virulence of phage LPSent1 on biofilms of S. Enteritidis EG.SmE1 at different MOIs. (A) A single treatment with phage LPSent1. (B) Multiple treatments with phage LPSent1. Phage LPSent1 was applied at three different MOIs (5, 10, 50) while untreated cells were used as a negative control. Values represent the mean (n = 3) with a standard deviation of three determinations of each point and were analyzed by Student’s t-test; *indicates significantly different from the corresponding control, p ≤ 0.05.
Bacteriophages’ firmness in medium-lacking their susceptible hosts is a critical barrier in the fate of phage biocontrol applications. The stability of phage LPSent1 within food matrices including milk, potable water, chicken breasts, and apple juice was checked at both 4°C (the refrigerator storage temperature) and 25°C (room temperature at which the food is being administered or consumed) as shown in Figure 8. The data indicated that phage LPSent1 was retained in food with a small titer reduction within 48 h. However, phage LPSent1 titers dropped by roughly 0.9 and 1.5 log PFU/mL after 48 h incubation at 25°C on chicken breasts and in apple juice, respectively. We have previously shown the stability of a phage cocktail for 48 h at 4°C and 25°C in water, milk, and on chicken breast (Esmael et al., 2021b). Another study showed stability in phage titers for 24 h before it showed significant deterioration in cabbage and chicken breasts, however, better stability was shown in milk (Bao et al., 2015). The results indicated that phage LPSent1 was stable in the tested food samples and could be a potential promising candidate for controlling Salmonella in food.
Figure 8. Analysis of LPSent1 stability in four food matrices (milk, water, apple juice and chicken breast). Phage-treated food samples were incubated for 48 h at either 4°C (A) or at 25°C (B), and aliquots were taken at 0, 2, 6, 12, 16, 24, 36, and 48 h, and residual phage titers were measured as PFU/mL. Values represent the mean with a standard deviation of three determinations of each point.
The influence of phage LPSent1 against the MDR S. Enteritidis EG.SmE1 contaminated food was assessed (Figure 9) at two different temperatures (4°C and 25°C). Food samples were contaminated with S. Enteritidis EG.SmE1 at a final count of 5 log10 CFU/mL and then challenged with the phage either at 100 or 1000 MOIs. In milk assay, the viable count of S. Enteritidis EG.SmE1 dropped below the detection threshold (< 1 CFU/100 μL) after 12 h and 16 h at 4°C challenging with phage LPSent1 at MOIs of 1000 and 100, respectively (Figure 9A). While at 25°C, no bacterial count was detected after 2h and 12 h at MOIs of 1000 and 100, respectively (Figure 9E). Previously, we showed the same inhibition values using a phage cocktail against the MDR S. Typhimurium EG.SmT3 (Esmael et al., 2021b).
Figure 9. Effectiveness of phage LPSent1 in reducing the S. Enteritidis EG.SmE1 in milk, water, apple juice, and on chicken breasts at either 4°C or 25°C. (A,E) Application in milk at 4°C or 25°C respectively, (B,F) application in water at 4°C or 25°C respectively, (C,G) application in apple juice at 4°C or 25°C respectively, and (D,H) application on chicken breasts at 4°C or 25°C respectively. Phage LPSent1 was applied at two different MOIs (100 and 1,000) to each food article and incubated for 48 h at either 4°C or 25°C. Bacterial load was estimated at different time points by measuring the mean CFU/mL ± standard deviation of three replicates of each time point.
Biocontrol in water was similarly investigated (Figures 9B, F). Relative to the non-treated controls S. Enteritidis EG.SmE1 counts declined below the detection limit and no viable counts were detected after 12 h and 16 h at 4°C post-phage infections using MOIs of 1000 and 100, respectively. Salmonella counts were dropped faster at 25°C, it only took 2 h and 6 h post-phage LPSent1 treatment at MOIs of 1000 and 100, respectively for the complete elimination of Salmonella in water. In the apple juice assay (Figure 9C), there were almost no viable counts of S. Enteritidis EG.SmE1 after 16 h at 4°C following treatment with LPSent1 at MOIs of 1000 and 100. While at 25°C, no viable bacterial count was detected after 6h and 16 h at MOIs of 1000 and 100, respectively (Figure 9G). Phage LPSent1 bestowed a noticeable reduction of viable S. Enteritidis EG.SmE1 counts on chicken breast slices at either 4°C or 25°C. When applied at 4°C using MOIs of 1000 and 100, phage LPSent1 reduced the viable bacterial counts below the detection limit after 12 h and 16 h, respectively (Figure 9D). In the case of application at 25°C (Figure 9H), no viable bacterial counts were detected after 2 h and 16 h after adding LPSent1 at MOIs of 1000 and 100, respectively.
Interestingly our results showed a promising reduction rate in the growth of the MDR S. Enteritidis EG.SmE1 in the tested food articles using phage LPSent1 for 2 days, especially when applied at 25°C, as compared with the phage-free controls. It is worth mentioning that, phages’ virulence is decreased at low temperatures as at low temperatures the bacterial host growth is hindered and hence the phage virulence is decreased (Bigwood et al., 2008; Guenther and Loessner, 2011; Huang et al., 2018b). Earlier studies reported a significant reduction rate of nearly 3 Log10 units in the recovered Salmonella Enteritidis using phage cocktails at MOIs of 1000, and 10,000 in different food matrices (Goodridge and Bisha, 2011; Kang et al., 2013; Spricigo et al., 2013; Bao et al., 2015; Grant et al., 2016; Osvaldo et al., 2016; Sharma et al., 2017; Islam et al., 2019; Esmael et al., 2021b).
The current study explains the environmental isolation of a robust and lytic bacteriophage against an antibiotic-resistant pathogenic S. Enteritidis EG.SmE1. Phage LPSent1 is a lytic bacteriophage with robust pH and thermal stability. Phage LPSent1 efficiently reduced free planktonic cells and biofilms of the MDR S. Enteritidis EG.SmE1. The in vitro application of phage LPSent1 on artificially contaminated foods was assessed on milk, water, apple juice, and on chicken breast and showed a significant reduction of the recovered bacteria as compared with the untreated controls. Additionally, the genome of phage LPSent1 was sequenced and analyzed in the current study. Subsequently, the current work identifies phage LPSent1 as a promising potential bio-control agent in the food industry.
The datasets presented in this study can be found in online repositories. The names of the repository/repositories and accession number(s) can be found in the article/Supplementary material.
RA-H contributed to the conception, design, project implementation, and manuscript revision. MA, IA, SA, and KA contributed to the conception and manuscript preparation and revision. AE contributed to the conception, design, project implementation, run the experiments, data interpretation, and wrote and revised the manuscript. All authors contributed to the article and approved the submitted version.
This research was funded by Institutional Fund Projects under grant no. IFPRC-109-130-2020. Therefore, authors gratefully acknowledge technical and financial support from the Ministry of Education and King Abdulaziz University, Jeddah, Saudi Arabia.
The authors declare that the research was conducted in the absence of any commercial or financial relationships that could be construed as a potential conflict of interest.
All claims expressed in this article are solely those of the authors and do not necessarily represent those of their affiliated organizations, or those of the publisher, the editors and the reviewers. Any product that may be evaluated in this article, or claim that may be made by its manufacturer, is not guaranteed or endorsed by the publisher.
The Supplementary Material for this article can be found online at: https://www.frontiersin.org/articles/10.3389/fmicb.2023.1135806/full#supplementary-material
Abd-Elghany, S. M., Fathy, T. M., Zakaria, A. I., Imre, K., Morar, A., Herman, V., et al. (2022). Prevalence of multidrug-resistant Salmonella enterica serovars in buffalo meat in Egypt. Foods Basel Switz. 11:2924. doi: 10.3390/foods11182924
Abdelhakim, A., Yamina, M., Mohammed, S. S., Hala, M. A., Mervat, G. E. A., Souhila, A., et al. (2011). Resistance to -lactams of human and veterinary Salmonella isolates in Egypt and Algeria. Afr. J. Microbiol. Res. 5, 802–808. doi: 10.5897/ajmr10.717
Abdel-Maksoud, M., Abdel-Khalek, R., El-Gendy, A., Gamal, R. F., Abdelhady, H. M., and House, B. L. (2015). Genetic characterisation of multidrug-resistant Salmonella enterica serotypes isolated from poultry in Cairo, Egypt. Afr. J. Lab. Med. 4, 1–7. doi: 10.4102/ajlm.v4i1.158
Abo-ELmaaty, S. A., Esmael, A., Bdelrahman, S., Tarek, E., and Hassan, M. G. (2022). Studies on the application of bacteriophages and silver_nanparticles in the treatment of Pseudomonas spp. Egypt. Acad. J. Biol. Sci. G Microbiol. 14, 23–39. doi: 10.21608/eajbsg.2022.217580
AccessScience Editors (2017). U.S. bans antibiotics use for enhancing growth in livestock. New York, NY: McGraw Hill.
Ackermann, H. W. (2012). Bacteriophage electron microscopy. Adv. Virus Res. 82, 1–32. doi: 10.1016/B978-0-12-394621-8.00017-0
Agyare, C., Etsiapa Boamah, V., Ngofi Zumbi, C., and Boateng Osei, F. (2019). “Antibiotic use in poultry production and its effects on bacterial resistance,” in Antimicrobial resistance – a global threat, ed. Y. Kumar (London: IntechOpen). doi: 10.5772/intechopen.79371
Akhtar, M., Viazis, S., and Diez-Gonzalez, F. (2014). Isolation, identification and characterization of lytic, wide host range bacteriophages from waste effluents against Salmonella enterica serovars. Food Control 38, 67–74. doi: 10.1016/j.foodcont.2013.09.064
Alharbi, M. G., Al-Hindi, R. R., Esmael, A., Alotibi, I. A., Azhari, S. A., Alseghayer, M. S., et al. (2022). The “big six”: Hidden emerging foodborne bacterial pathogens. Trop. Med. Infect. Dis. 7:356. doi: 10.3390/tropicalmed7110356
Archawakulathep, A., Ta Thi Kim, C., Meunsene, D., Handijatno, D., Hassim, H. A., Rovira, H. R., et al. (2014). Perspectives on antimicrobial resistance in livestock and livestock products in ASEAN countries. Thai J. Vet. Med. 44, 5–13.
Atterbury, R. J., Van Bergen, M. A. P., Ortiz, F., Lovell, M. A., Harris, J. A., De Boer, A., et al. (2007). Bacteriophage therapy to reduce salmonella colonization of broiler chickens. Appl. Environ. Microbiol. 73, 4543–4549. doi: 10.1128/AEM.00049-07
Awad, H. A., Mohamed, M. H., Badran, N. F., Mohsen, M., and Abd-Elrhman, A. S. A. (2016). Multidrug-resistant organisms in neonatal sepsis in two tertiary neonatal ICUs, Egypt. J. Egypt. Public Health Assoc. 91, 31–38. doi: 10.1097/01.EPX.0000482038.76692.3
Bai, J., Jeon, B., and Ryu, S. (2019). Effective inhibition of Salmonella Typhimurium in fresh produce by a phage cocktail targeting multiple host receptors. Food Microbiol. 77, 52–60. doi: 10.1016/j.fm.2018.08.011
Bao, H., Zhang, P., Zhang, H., Zhou, Y., Zhang, L., and Wang, R. (2015). Bio-control of salmonella enteritidis in foods using bacteriophages. Viruses 7, 4836–4853. doi: 10.3390/v7082847
Bigwood, T., Hudson, J. A., Billington, C., Carey-Smith, G. V., and Heinemann, J. A. (2008). Phage inactivation of foodborne pathogens on cooked and raw meat. Food Microbiol. 25, 400–406. doi: 10.1016/j.fm.2007.11.003
Cerca, N., Martins, S., Pier, G. B., Oliveira, R., and Azeredo, J. (2005). The relationship between inhibition of bacterial adhesion to a solid surface by sub-MICs of antibiotics and subsequent development of a biofilm. Res. Microbiol. 156, 650–655. doi: 10.1016/j.resmic.2005.02.004
Chow, M. S., and Rouf, M. A. (1983). Isolation and partial characterization of two aeromonas hydrophila bacteriophages. Appl. Environ. Microbiol. 45, 1670–1676. doi: 10.1128/aem.45.5.1670-1676.1983
Chylkova, T., Cadena, M., Ferreiro, A., and Pitesky, M. (2017). Susceptibility of salmonella biofilm and planktonic bacteria to common disinfectant agents used in poultry processing. J. Food Prot. 80, 1072–1079. doi: 10.4315/0362-028X.JFP-16-393
Clinical and Laboratory Standards Institute (2019). Performance standards for antimicrobial susceptibility testing CLSI supplement M100, 29th Edn. Wayne, PA: Clinical and Laboratory Standards Institute.
Clokie, M. R. J., and Kropinski, A. M. (2009). Bacteriophages: Methods and protocols: Isolation, characterization, and interactions, Vol. 1. Totowa, NJ: Humana Press, 971–978.
Coffey, B., Mills, S., Coffey, A., McAuliffe, O., and Ross, R. P. (2010). Phage and their lysins as biocontrol agents for food safety applications. Annu. Rev. Food Sci. Technol. 1, 449–468. doi: 10.1146/annurev.food.102308.124046
Corcoran, M., Morris, D., De Lappe, N., O’Connor, J., Lalor, P., Dockery, P., et al. (2014). Commonly used disinfectants fail to eradicate Salmonella enterica biofilms from food contact surface materials. Appl. Environ. Microbiol. 80, 1507–1514. doi: 10.1128/AEM.03109-13
Dahshan, H., Abd-Elall, A. M. M., Megahed, A. M., Abd-El-Kader, M. A., and Nabawy, E. E. (2015). Veterinary antibiotic resistance, residues, and ecological risks in environmental samples obtained from poultry farms, Egypt. Environ. Monit. Assess. 187:2. doi: 10.1007/s10661-014-4218-3
De Melo, A. C. C., Da Mata Gomes, A., Melo, F. L., Ardisson-Araújo, D. M. P., De Vargas, A. P. C., Ely, V. L., et al. (2019). Characterization of a bacteriophage with broad host range against strains of Pseudomonas aeruginosa isolated from domestic animals. BMC Microbiol. 19:134. doi: 10.1186/s12866-019-1481-z
Diab, M. S., Zaki, R. S., Ibrahim, N. A., and Abd El Hafez, M. S. (2019). Prevalence of multidrug resistance non-typhoidal Salmonellae isolated from layer farms and humans in Egypt. J. Worlds Poult. Res. 9, 280–288. doi: 10.36380/SCIL.2019.WVJ35
Esmael, A., Azab, E., Gobouri, A. A., Nasr-eldin, M. A., Moustafa, M. M. A., Mohamed, S. A., et al. (2021b). Isolation and characterization of two lytic bacteriophages infecting a multi-drug resistant salmonella typhimurium and their efficacy to combat salmonellosis in ready-to-use foods. Microorganisms 9, 1–19. doi: 10.3390/microorganisms9020423
Esmael, A., Abo-Elmaaty, S. A., Khafaga, E. M., Abdelrahman, S., and Hassan, M. G. (2021a). Efficacy of three lytic bacteriophages for eradicating biofilms of multidrug-resistant Proteus mirabilis. Arch. Virol. 166, 3311–3322. doi: 10.1007/s00705-021-05241-5
Esmael, A., Hassan, M. G., Amer, M. M., Abdelrahman, S., Hamed, A. M., Abd-raboh, H. A., et al. (2020). Antimicrobial activity of certain natural-based plant oils against the antibiotic-resistant acne bacteria. SAUDI J. Biol. Sci. 27, 448–455. doi: 10.1016/j.sjbs.2019.11.006
European Union (2005). Ban on antibiotics as growth promoters in animal feed enters into effect. Brussels: European Union.
Florensa, A. F., Kaas, R. S., Clausen, P. T. L. C., Aytan-Aktug, D., and Aarestrup, F. M. (2022). ResFinder – an open online resource for identification of antimicrobial resistance genes in next-generation sequencing data and prediction of phenotypes from genotypes. Microb. Genomics 8:000748. doi: 10.1099/mgen.0.000748
Foley, S. L., Lynne, A. M., and Nayak, R. (2008). Salmonella challenges: Prevalence in swine and poultry and potential pathogenicity of such isolates. J. Anim. Sci. 86, E149–E162. doi: 10.2527/jas.2007-0464
Gal-Mor, O. (2019). Persistent infection and long-term carriage of typhoidal and nontyphoidal salmonellae. Clin. Microbiol. Rev. 32, e88–e18. doi: 10.1128/CMR.00088-18
Gonzalez-Escobedo, G., and Gunn, J. S. (2013). Identification of salmonella enterica serovar typhimurium genes regulated during biofilm formation on cholesterol gallstone surfaces. Infect. Immun. 81, 3770–3780. doi: 10.1128/IAI.00647-13
Gonzalez-Escobedo, G., Marshall, J. M., and Gunn, J. S. (2011). Chronic and acute infection of the gall bladder by Salmonella Typhi: Understanding the carrier state. Nat. Rev. Microbiol. 9, 9–14. doi: 10.1038/nrmicro2490
Goodridge, L. D., and Bisha, B. (2011). Phage-based biocontrol strategies to reduce foodborne pathogens in foods. Bacteriophage 1, 130–137. doi: 10.4161/bact.1.3.17629
Grant, A., Hashem, F., and Parveen, S. (2016). Salmonella and Campylobacter: Antimicrobial resistance and bacteriophage control in poultry. Food Microbiol. 53, 104–109. doi: 10.1016/j.fm.2015.09.008
Guenther, S., and Loessner, M. J. (2011). Bacteriophage biocontrol of Listeria monocytogenes on soft ripened white mold and red-smear cheeses. Bacteriophage 1, 94–100. doi: 10.4161/bact.1.2.15662
Guenther, S., Herzig, O., Fieseler, L., Klumpp, J., and Loessner, M. J. (2012). Biocontrol of Salmonella Typhimurium in RTE foods with the virulent bacteriophage FO1-E2. Int. J. Food Microbiol. 154, 66–72. doi: 10.1016/j.ijfoodmicro.2011.12.023
Haselbeck, A. H., Panzner, U., Im, J., Baker, S., Meyer, C. G., and Marks, F. (2017). Current perspectives on invasive nontyphoidal Salmonella disease. Curr. Opin. Infect. Dis. 30, 498–503. doi: 10.1097/QCO.0000000000000398
Hatfull, G. F., and Hendrix, R. W. (2011). Bacteriophages and their genomes. Curr. Opin. Virol. 1, 298–303. doi: 10.1016/j.coviro.2011.06.009
Hosny, R. A., Shalaby, A. G., Nasef, S. A., and Sorour, H. K. (2022). Antibiofilm activity of a lytic Salmonella phage on different Salmonella enterica serovars isolated from broiler farms. Int. Microbiol. 1–13. doi: 10.1007/s10123-022-00294-1
Huang, C., Shi, J., Ma, W., Li, Z., Wang, J., Li, J., et al. (2018a). Isolation, characterization, and application of a novel specific Salmonella bacteriophage in different food matrices. Food Res. Int. 111, 631–641. doi: 10.1016/j.foodres.2018.05.071
Huang, C., Virk, S. M., Shi, J., Zhou, Y., Willias, S. P., Morsy, M. K., et al. (2018b). Isolation, characterization, and application of bacteriophage LPSE1 against Salmonella enterica in ready to eat (RTE) foods. Front. Microbiol. 9:1046. doi: 10.3389/fmicb.2018.01046
Humphrey, T. (2004). Salmonella, stress responses and food safety. Nat. Rev. Microbiol. 2, 504–509. doi: 10.1038/nrmicro907
Islam, M. S., Zhou, Y., Liang, L., Nime, I., Liu, K., Yan, T., et al. (2019). Application of a phage cocktail for control of Salmonella in foods and reducing biofilms. Viruses 11:841. doi: 10.3390/v11090841
Jung, L., Ding, T., and Ahn, J. (2017). Evaluation of lytic bacteriophages for control of multidrug-resistant Salmonella Typhimurium. Ann. Clin. Microbiol. Antimicrob. 16:66. doi: 10.1186/s12941-017-0237-6
Kang, H. W., Kim, J. W., Jung, T. S., and Woo, G. J. (2013). wksl3, a new biocontrol agent for Salmonella enterica serovars enteritidis and typhimurium in foods: Characterization, application, sequence analysis, and oral acute toxicity study. Appl. Environ. Microbiol. 79, 1956–1968. doi: 10.1128/AEM.02793-12
Katoh, K., Rozewicki, J., and Yamada, K. D. (2019). MAFFT online service: Multiple sequence alignment, interactive sequence choice and visualization. Brief. Bioinform. 20, 1160–1166. doi: 10.1093/bib/bbx108
Keen, E. C., Adhya, S. L., and Wormser, G. P. (2015). Phage therapy: Current research and applications. Clin. Infect. Dis. 61, 141–142. doi: 10.1093/cid/civ257
Klieve, A. V. (2005). “Bacteriophages,” in Methods in gut microbial ecology for ruminants, eds H. P. Makkar and C. S. McSweeney (Dordrecht: Springer), 39–46. doi: 10.1007/1-4020-3791-0_3
Kot, W., Vogensen, F. K., Sørensen, S. J., and Hansen, L. H. (2014). DPS – A rapid method for genome sequencing of DNA-containing bacteriophages directly from a single plaque. J. Virol. Methods 196, 152–156. doi: 10.1016/j.jviromet.2013.10.040
Kropinski, A. M., Mazzocco, A., Waddell, T. E., Lingohr, E., and Johnson, R. P. (2009). Enumeration of bacteriophages by double agar overlay plaque assay. Methods Mol. Biol. 501, 69–76. doi: 10.1007/978-1-60327-164-6_7
Kumar, S., Stecher, G., Li, M., Knyaz, C., and Tamura, K. (2018). MEGA X: Molecular evolutionary genetics analysis across computing platforms. Mol. Biol. Evol. 35, 1547–1549. doi: 10.1093/molbev/msy096
Kutter, E., De Vos, D., Gvasalia, G., Alavidze, Z., Gogokhia, L., Kuhl, S., et al. (2010). Phage therapy in clinical practice: Treatment of human infections. Curr. Pharm. Biotechnol. 11, 69–86. doi: 10.2174/138920110790725401
Labrie, S. J., Samson, J. E., and Moineau, S. (2010). Bacteriophage resistance mechanisms. Nat. Rev. Microbiol. 8, 317–327. doi: 10.1038/nrmicro2315
Libby, D. A., and Schaible, P. J. (1955). Observations on growth responses to antibiotics and arsonic acids in poultry feeds. Science 121, 733–734. doi: 10.1126/science.121.3151.733
Lin, D. M., Koskella, B., and Lin, H. C. (2017). Phage therapy: An alternative to antibiotics in the age of multi-drug resistance. World J. Gastrointest. Pharmacol. Ther. 8:162. doi: 10.4292/wjgpt.v8.i3.162
Lowe, T. M., and Eddy, S. R. (1997). tRNAscan-SE: A program for improved detection of transfer RNA genes in genomic sequence. Nucleic Acids Res. 25, 955–964.
Mahmoud, M., Askora, A., Barakat, A. B., Rabie, O. E. F., and Hassan, S. E. (2018). Isolation and characterization of polyvalent bacteriophages infecting multi drug resistant Salmonella serovars isolated from broilers in Egypt. Int. J. Food Microbiol. 266, 8–13. doi: 10.1016/j.ijfoodmicro.2017.11.009
Mahony, J., McAuliffe, O., Ross, R. P., and van Sinderen, D. (2011). Bacteriophages as biocontrol agents of food pathogens. Curr. Opin. Biotechnol. 22, 157–163. doi: 10.1016/j.copbio.2010.10.008
Majowicz, S. E., Musto, J., Scallan, E., Angulo, F. J., Kirk, M., O’Brien, S. J., et al. (2010). The global burden of nontyphoidal Salmonella gastroenteritis. Clin. Infect. Dis. 50, 882–889. doi: 10.1086/650733
Maron, D. F., Smith, T. J. S., and Nachman, K. E. (2013). Restrictions on antimicrobial use in food animal production: An international regulatory and economic survey. Glob. Health 9:48. doi: 10.1186/1744-8603-9-48
Matsuzaki, S., Uchiyama, J., Takemura-Uchiyama, I., and Daibata, M. (2014). Perspective: The age of the phage. Nature 509, S9–S9. doi: 10.1038/509S9a
McCallin, S., Alam Sarker, S., Barretto, C., Sultana, S., Berger, B., Huq, S., et al. (2013). Safety analysis of a Russian phage cocktail: From MetaGenomic analysis to oral application in healthy human subjects. Virology 443, 187–196. doi: 10.1016/j.virol.2013.05.022
Medeiros, M. A. N., De Oliveira, D. C. N., Dos Prazeres Rodrigues, D., and De Freitas, D. R. C. (2011). Prevalence and antimicrobial resistance of Salmonella in chicken carcasses at retail in 15 Brazilian cities. Rev. Panam. Salud Publica 30, 555–560. doi: 10.1590/S1020-49892011001200010
Merwad, A. M. A., and Abdel-Haliem, M. E. F. (2018). Isolation and initial characterization of a myoviridae phage for controlling ZoonotiC Salmonella typhimurium AND Salmonella enteritidis from broilers in Egypt. Slov. Vet. Res. 55, 5–15. doi: 10.26873/SVR-621-2018
Mirzaei, M. K., and Nilsson, A. S. (2015). Isolation of phages for phage therapy: A comparison of spot tests and efficiency of plating analyses for determination of host range and efficacy. PLoS One 10:e0118557. doi: 10.1371/journal.pone.0118557
Mondal, P., Mallick, B., Dutta, M., and Dutta, S. (2022). Isolation, characterization, and application of a novel polyvalent lytic phage STWB21 against typhoidal and nontyphoidal Salmonella spp. Front. Microbiol. 13:980025. doi: 10.3389/fmicb.2022.980025
Moore, P. R., and Evenson, A. (1946). Use of sulfasuxidine, streptothricin, and streptomycin in nutritional studies with the chick. J. Biol. Chem. 165, 437–441.
Moye, Z. D., Woolston, J., and Sulakvelidze, A. (2018). Bacteriophage applications for food production and processing. Viruses 10:205. doi: 10.3390/v10040205
Musyoka, J. N., Abong, G. O., Mbogo, D. M., Fuchs, R., Low, J., Heck, S., et al. (2018). Effects of acidification and preservatives on microbial growth during storage of orange fleshed sweet potato puree. Int. J. Food Sci. 2018:8410747. doi: 10.1155/2018/8410747
Neetoo, H., and Mahomoodally, F. (2014). Use of antimicrobial films and edible coatings incorporating chemical and biological preservatives to control growth of Listeria monocytogenes on cold smoked salmon. Biomed Res. Int. 2014:534915. doi: 10.1155/2014/534915
O’Flaherty, S., Ross, R. P., Meaney, W., Fitzgerald, G. F., Elbreki, M. F., and Coffey, A. (2005). Potential of the Polyvalent anti-Staphylococcus bacteriophage K for control of antibiotic-resistant staphylococci from hospitals. Appl. Environ. Microbiol. 71, 1836–1842. doi: 10.1128/AEM.71.4.1836-1842.2005
O’Flynn, G., Coffey, A., Fitzgerald, G. F., and Ross, R. P. (2006). The newly isolated lytic bacteriophages st104a and st104b are highly virulent against Salmonella enterica. J. Appl. Microbiol. 101, 251–259. doi: 10.1111/j.1365-2672.2005.02792.x
Omwandho, C. O. A., and Kubota, T. (2010). Salmonella enterica serovar Enteritidis: A mini-review of contamination routes and limitations to effective control. Jpn. Agric. Res. Q. 44, 7–16. doi: 10.6090/jarq.44.7
Osvaldo, L., del Campo Nohelia, C., Karina, R., and Bal, C. (2016). Biocontrol of Salmonella Typhimurium growth in tomato surface by bacteriophage P22. Afr. J. Microbiol. Res. 10, 528–534. doi: 10.5897/ajmr2015.7784
Othman, A. M., Esmael, A., Abdelrahman, S., Shraf, A., and Hassan, M. G. (2021). Biocontrol of Acinetobacter baumannii-S-MH Using Bacteriophages Isolated from Sewage Water. Egypt. Acad. J. Biol. Sci. G Microbiol. 13, 17–32. doi: 10.21608/eajbsg.2021.203035
Pandey, M., Goud, E. S. K., Pandey, M., and Goud, E. S. K. (2021). Non-typhoidal salmonellosis: A major concern for poultry industry. London: IntechOpen. doi: 10.5772/intechopen.96400
Pawlowska, A. M., Zannini, E., Coffey, A., and Arendt, E. K. (2012). Green preservatives’: Combating fungi in the food and feed industry by applying antifungal lactic acid bacteria. Adv. Food Nutr. Res. 66, 217–238. doi: 10.1016/B978-0-12-394597-6.00005-7
Pérez-Díaz, I. M., Truong, V. D., Webber, A., and McFeeters, R. F. (2008). Microbial growth and the effects of mild acidification and preservatives in refrigerated sweet potato puree. J. Food Prot. 71, 639–642. doi: 10.4315/0362-028X-71.3.639
Porwollik, S., Boyd, E. F., Choy, C., Cheng, P., Florea, L., Proctor, E., et al. (2004). Characterization of Salmonella enterica subspecies I genovars by use of microarrays. J. Bacteriol. 186, 5883–5898. doi: 10.1128/JB.186.17.5883-5898.2004
Rendueles, C., Duarte, A. C., Escobedo, S., Fernández, L., Rodríguez, A., García, P., et al. (2022). Combined use of bacteriocins and bacteriophages as food biopreservatives. A review. Int. J. Food Microbiol. 368:109611. doi: 10.1016/j.ijfoodmicro.2022.109611
Rivera, D., Moreno-Switt, A. I., Denes, T. G., Hudson, L. K., Peters, T. L., Samir, R., et al. (2022). Novel Salmonella Phage, vB_Sen_STGO-35-1, characterization and evaluation in chicken meat. Microorganisms 10:606. doi: 10.3390/microorganisms10030606
Rohwer, F., and Segall, A. M. (2015). In retrospect: A century of phage lessons. Nature 528, 46–48. doi: 10.1038/528046a
Sabry, N. A., Farid, S. F., and Dawoud, D. M. (2014). Antibiotic dispensing in Egyptian community pharmacies: An observational study. Res. Soc. Adm. Pharm. 10, 168–184. doi: 10.1016/j.sapharm.2013.03.004
Scallan, E., Griffin, P. M., Angulo, F. J., Tauxe, R. V., and Hoekstra, R. M. (2011). Foodborne illness acquired in the United States–unspecified agents. Emerg. Infect. Dis. 17, 16–22. doi: 10.3201/eid1701.091101p2
Seemann, T. (2014). Prokka: Rapid prokaryotic genome annotation. Bioinformatics 30, 2068–2069. doi: 10.1093/bioinformatics/btu153
Sharma, M., Dashiell, G., Handy, E. T., East, C., Reynnells, R., White, C., et al. (2017). Survival of salmonella newport on whole and fresh-cut cucumbers treated with lytic bacteriophages. J. Food Prot. 80, 668–673. doi: 10.4315/0362-028X.JFP-16-449
Sobhy, N., Aly, F., El Kader, O. A., Ghazal, A., and Elbaradei, A. (2012). Community-acquired methicillin-resistant Staphylococcus aureus from skin and soft tissue infections (in a sample of Egyptian population): Analysis of mec gene and staphylococcal cassette chromosome. Braz. J. Infect. Dis. 16, 426–431. doi: 10.1016/j.bjid.2012.08.004
Spector, M. P., and Kenyon, W. J. (2012). Resistance and survival strategies of Salmonella enterica to environmental stresses. Food Res. Int. 45, 455–481. doi: 10.1016/j.foodres.2011.06.056
Spricigo, D. A., Bardina, C., Cortés, P., and Llagostera, M. (2013). Use of a bacteriophage cocktail to control Salmonella in food and the food industry. Int. J. Food Microbiol. 165, 169–174. doi: 10.1016/j.ijfoodmicro.2013.05.009
Stepanovic, S., Vukoviæ, D., Hola, V., Di Bonaventura, G., Djukiæ, S., Æirkoviæ, I., et al. (2007). Quantification of biofilm in microtiter plates: Overview of testing conditions and practical recommendations for assessment of biofilm production by staphylococci. APMIS 115, 891–899. doi: 10.1111/j.1600-0463.2007.apm_630.x
Stothard, P., and Wishart, D. S. (2005). Circular genome visualization and exploration using CGView. Bioinformatics 21, 537–539. doi: 10.1093/bioinformatics/bti054
Sukumaran, A. T., Nannapaneni, R., Kiess, A., and Sharma, C. S. (2015). Reduction of Salmonella on chicken meat and chicken skin by combined or sequential application of lytic bacteriophage with chemical antimicrobials. Int. J. Food Microbiol. 207, 8–15. doi: 10.1016/j.ijfoodmicro.2015.04.025
Tanji, Y., Shimada, T., Yoichi, M., Miyanaga, K., Hori, K., and Unno, H. (2004). Toward rational control of Escherichia coli O157:H7 by a phage cocktail. Appl. Microbiol. Biotechnol. 64, 270–274. doi: 10.1007/s00253-003-1438-9
Teklemariam, A. D., Alharbi, M. G., Al-Hindi, R. R., Alotibi, I., Aljaddawi, A. A., Azhari, S. A., et al. (2022). Isolation and characterization of Chi-like Salmonella Bacteriophages Infecting Two Salmonella enterica Serovars, Typhimurium and Enteritidis. Pathogens 11:1480. doi: 10.3390/pathogens11121480
Teklemariam, A. D., Al-Hindi, R. R., Alharbi, M. G., Alotibi, I., Azhari, S. A., Qadri, I., et al. (2023). Isolation and characterization of a novel lytic phage, vB_PseuP-SA22, and its efficacy against carbapenem-resistant Pseudomonas aeruginosa. Antibiotics 12:497. doi: 10.3390/antibiotics12030497
Van Twest, R., and Kropinski, A. M. (2009). Bacteriophage enrichment from water and soil. Methods Mol. Biol. 501, 15–21. doi: 10.1007/978-1-60327-164-6_2
Waibel, P. E., Abbott, O. J., Baumann, C. A., and Bird, H. R. (1954). Disappearance of the growth response of chicks to dietary antibiotics in an “old”. Environ. Poult. Sci. 33, 1141–1146. doi: 10.3382/ps.0331141
Welkos, S., Schreiber, M., and Baer, H. (1974). Identification of Salmonella with the O-1 bacteriophage. Appl. Microbiol. 28, 618–622. doi: 10.1128/am.28.4.618-622.1974
Wick, R. R., Judd, L. M., Gorrie, C. L., and Holt, K. E. (2017). Unicycler: Resolving bacterial genome assemblies from short and long sequencing reads. PLoS Comput. Biol. 13:e1005595. doi: 10.1371/journal.pcbi.1005595
Wilson, M., and Wilson, P. J. K. (2021). “Gastroenteritis due to Salmonella,” in Close encounters of the microbial kind (Cham: Springer), 451–461. doi: 10.1007/978-3-030-56978-5_33
Woo, J., and Ahn, J. (2014). Assessment of synergistic combination potential of probiotic and bacteriophage against antibiotic-resistant Staphylococcus aureus exposed to simulated intestinal conditions. Arch. Microbiol. 196, 719–727. doi: 10.1007/s00203-014-1013-z
Yamamoto, K. R., Alberts, B. M., Benzinger, R., Lawhorne, L., and Treiber, G. (1970). Rapid bacteriophage sedimentation in the presence of polyethylene glycol and its application to large-scale virus purification. Virology 40, 734–744. doi: 10.1016/0042-6822(70)90218-7
Youssef, R. A., Abbas, A. M., El-Shehawi, A. M., Mabrouk, M. I., and Aboshanab, K. M. (2021). Serotyping and antimicrobial resistance profile of enteric nontyphoidal Salmonella recovered from febrile neutropenic patients and poultry in Egypt. Antibiotics 10:493. doi: 10.3390/antibiotics10050493
Keywords: bacteriophages, Salmonella Enteritidis (S. Enteritidis), multi-drug resistant (MDR), biofilms, biocontrol
Citation: Al-Hindi RR, Alharbi MG, Alotibi I, Azhari SA, Algothmi KM and Esmael A (2023) Application of a novel lytic Jerseyvirus phage LPSent1 for the biological control of the multidrug-resistant Salmonella Enteritidis in foods. Front. Microbiol. 14:1135806. doi: 10.3389/fmicb.2023.1135806
Received: 01 January 2023; Accepted: 13 March 2023;
Published: 05 April 2023.
Edited by:
Ana Oliveira, University of Minho, PortugalReviewed by:
Hongduo Bao, Jiangsu Academy of Agricultural Sciences (JAAS), ChinaCopyright © 2023 Al-Hindi, Alharbi, Alotibi, Azhari, Algothmi and Esmael. This is an open-access article distributed under the terms of the Creative Commons Attribution License (CC BY). The use, distribution or reproduction in other forums is permitted, provided the original author(s) and the copyright owner(s) are credited and that the original publication in this journal is cited, in accordance with accepted academic practice. No use, distribution or reproduction is permitted which does not comply with these terms.
*Correspondence: Rashad R. Al-Hindi, cmhpbmRpQGthdS5lZHUuc2E=; Ahmed Esmael, YWhtZWQuZXNtYWVsQGZzYy5idS5lZHUuZWc=, YTdtZWRlc21hZWxAZ21haWwuY29t
Disclaimer: All claims expressed in this article are solely those of the authors and do not necessarily represent those of their affiliated organizations, or those of the publisher, the editors and the reviewers. Any product that may be evaluated in this article or claim that may be made by its manufacturer is not guaranteed or endorsed by the publisher.
Research integrity at Frontiers
Learn more about the work of our research integrity team to safeguard the quality of each article we publish.