- 1Department of Medical and Biological Physics, Gomel State Medical University, Gomel, Belarus
- 2Laboratory of Bionanoscopy, Institute of Radiobiology of NAS of Belarus, Gomel, Belarus
- 3Laboratory of Biological Electron Microscopy, École Polytechnique Fédérale de Lausanne (EPFL), University of Lausanne (UNIL), Lausanne, Switzerland
- 4Department of Microbiology, Gomel State Medical University, Gomel, Belarus
- 5Centre Universitaire Romand de Médecine Légale, Unité Facultaire d’Anatomie et de Morphologie (UFAM), University of Lausanne (UNIL), Lausanne, Switzerland
- 6International Joint Research Group VUB-EPFL NanoBiotechnology & NanoMedicine (NANO), Vrije Universiteit Brussel and École Polytechnique Fédérale de Lausanne (EPFL), Lausanne, Switzerland
- 7Alliance Research Group VUB-UGent NanoMicrobiology (NAMI), Research Group Structural Biology Brussels, Vrije Universiteit Brussel, Brussels, Belgium
Introduction: Patients undergoing cancer treatment by radiation therapy commonly develop Candida albicans infections (candidiasis). Such infections are generally treated by antifungals that unfortunately also induce numerous secondary effects in the patient. Additional to the effect on the immune system, ionizing radiation influences the vital activity of C. albicans cells themselves; however, the reaction of C. albicans to ionizing radiation acting simultaneously with antifungals is much less well documented. In this study, we explored the effects of ionizing radiation and an antifungal drug and their combined effect on C. albicans.
Methods: The study essentially relied on a novel technique, referred to as optical nanomotion detection (ONMD) that monitors the viability and metabolic activity of the yeast cells in a label and attachment-free manner.
Results and discussion: Our findings demonstrate that after exposure to X-ray radiation alone or in combination with fluconazole, low-frequency nanoscale oscillations of whole cells are suppressed and the nanomotion rate depends on the phase of the cell cycle, absorbed dose, fluconazole concentration, and post-irradiation period. In a further development, the ONMD method can help in rapidly determining the sensitivity of C. albicans to antifungals and the individual concentration of antifungals in cancer patients undergoing radiation therapy.
Introduction
The optical nanomotion detection (ONMD) technique was recently developed to study the nanomotion pattern of single cells as well as cellular populations (Willaert et al., 2020). The technique is simpler than its atomic force microscopy (AFM)–based counterpart (Longo et al., 2013) since it is easily accessible and most importantly label- and attachment-free. It was essentially developed to perform rapid antifungal susceptibility tests on yeast (Willaert et al., 2020; Radonicic et al., 2022). Recent experiments using the AFM- and optical-based nanomotion detection method demonstrated that cellular nanomotion is directly linked to the metabolic activity of the cell. Cell metabolic activity changes of several strains of Candida (C. albicans DSY294, C. glabrata DSY562, and C. lusitaniae DSY4606) in ethanol-induced life-death transition have been studied by observing cellular nanomotions (Willaert et al., 2020). Concentration- and time-dependent effects of several antifungals (amphotericin B, caspofungin, and fluconazole) on the C. albicans nanomotion was investigated using both the AFM- and optical-based nanomotion detection techniques (Kasas et al., 2015; Willaert et al., 2020).
It is known that ionizing radiation influences cellular metabolic activity. The changes in metabolic activity after yeast X-ray irradiation were observed for C. guilliermondii (35 kV, 300 Gy) (Navasardyan et al., 2017). The exposure of cells and tissues to ionizing radiation leads to oxidizing events that change the structure of biologically important molecules. The changes are induced by the direct interaction of ionizing radiation with the target macromolecules or via the products released by the radiolysis of water. These early events induce, among others, oxidative stress that leads to cellular metabolic and proliferative changes that ultimately can result in cellular death (Azzam et al., 2012). Cancer is nowadays a major public health problem at the planetary scale. Among the therapeutic options to fight this disease, radiotherapy is widely used alone or in combination with other treatments to cure or reduce the risk of cancer recurrence. One of the acute side effects of radiation therapy is candidiasis. As an illustration, head and neck cancer patients undergoing radiation therapy often develop oral candidiasis over the course of their treatment (Mañas et al., 2012; da Silva et al., 2017). After the radiation therapy starts Candida colonization can be observed in about 75% of patients. Local manifestations of fungal infection occurred in 26% of colonized patients (Pinel et al., 2012). Nowadays the mechanisms underlying radiotherapy-induced candidiasis are not clear. Radiation-induced free radicals and DNA damage modify various cellular signaling pathways regulating cell proliferation, differentiation, and death that cause inflammation and activate apoptosis (Ingrosso et al., 2021).
Candida albicans is an opportunistic pathogenic microorganism that co-exists in humans as a commensal without harming the host, but if the immune status of the host organism or its microbiota is violated, which is the case with radiotherapy, it can cause extensive colonization of the mucous membrane and local or systemic disease (Casadevall and Pirofski, 2007; Costa-de-Oliveira and Rodrigues, 2020). Gordee and Simpson (1967) showed that the pre-irradiation by X-rays significantly reduced the number of C. albicans cells required for the LD50 of experimentally infected mice (Gordee and Simpson, 1967). On the other hand, ionizing radiation influences Candida cells themselves, which can potentially modify their virulence and sensitivity to antifungal drugs. In vitro studies demonstrate that ionizing radiation can either inhibit Candida proliferation or it can potentiate its virulence, the outcome depending on the source of irradiation, Candida strain, and culture conditions (Ueta et al., 2001; Ben-Yosefa et al., 2005; Cagnacci et al., 2008). It should be emphasized that ionizing radiation effects strongly depend on the phase of the cell cycle. Budding yeast cells in S and M phases are known to be more resistant to radiation than non-budding cells in the G1 phase (Beam et al., 1954; Langguth and Beam, 1973). But proliferating yeast cells predominantly in S/G2 phase exhibit greater X-ray-induced chromosomal loss and DNA deletion than stationary (G1/G0)-phase yeast cells (Parry et al., 1979; Hafer et al., 2010).
The effect of antifungal drugs on the cellular nanomotion of yeast have been explored in several recent studies; however, how ionizing radiation influences yeast nanomotion as well as the combined effect of radiation and antifungal drugs on yeast nanomotion has not yet been studied. Therefore, we investigated the effect of X-ray radiation and an antifungal separately and in combination on the nanomotion of C. albicans cells using the ONMD method. The results obtained can help in further understanding the mechanisms of candidiasis pathogenesis and the development of strategies to determine the sensitivity of C. albicans to antifungal drugs during radiotherapy.
Materials and methods
Strains and cell growth
Candida albicans (ATCC 10231) were grown on Sabouraud glucose-peptone agar, HiMedia Laboratories, India (40 g/L glucose, 10 g/L peptone, 15 g/L agar) for 2 days at a temperature of 28°C. Several colonies of the same type from Sabouraud agar were transferred with a cotton swab into a test tube with 10 mL of Sabouraud broth. Under the control of a densitometer, the turbidity of the suspension was adjusted to 10 McFarland, and the estimated concentration of the cell suspension was 108 cells/ml. This concentration of cells was experimentally selected to be optimal for microscopy when 100–150 Candida cells were observed in one field of view.
Candida albicans ATCC 10231 is a fluconazole (FLC) susceptible strain (Silva et al., 2022). A stock solution of FLC (10 mg/mL) (Pharmland) was prepared in sterile distilled water. The FLC solution was introduced into vials containing C. albicans suspensions in Sabouraud broth to achieve a concentration of 10 or 1000 mg/L. The FLC concentration of 10 mg/L corresponds to 20 × MIC (minimum inhibitory concentration) and 0.625 MFC (minimum fungicidal concentration), and the concentration of 1000 mg/L corresponds to 2,000 × MIC and 62.5 MFC (Silva et al., 2022).
After irradiation, the vials were incubated on an orbital shaker incubator (ES-20, Biosan, Latvia) at 28°C and 180 rpm. This was supposed to slow down the adhesion of cells to the plastic of the vial. Before performing the ONMD measurements (points 3–24 h), the suspension was transferred to a sterile tube and diluted with Sabouraud broth until a concentration of 108 cells/ml (10 McFarland).
X-ray irradiation
The C. albicans suspension was placed in ventilated vials for cell cultures (cell culture flask T-25, standard surface, with filter cap, Sarstedt, Germany). The suspension of cells in vials had the form of a flat layer 2–3 mm thick at room temperature. The vials were exposed to X-ray irradiation using the X-RAD 320 biological irradiation unit with a tube voltage of 320 kV, a current of 12.5 mA with a power of 5 Gy/min, SSD of 40 sm, filter 1 (2 mm Al). The controlled experimental conditions were as follows: the temperature was 20.9 ± 0.7°C and the humidity was 62.5 ± 2.5%. As a reference, non-irradiated samples (untreated control) were used, which were kept under the same experimental conditions as the irradiated samples.
The irradiation of the cell suspensions was carried out in accordance with two experimental protocols. In protocol 1, the C. albicans suspensions (5 ml) were irradiated in fractions of 40 min with a dose of 200 Gy accumulated during each fraction, with a break between fractions of 5 min. A total of 4 fractions of irradiation were carried out, the absorbed dose after each fraction of irradiation was 200, 400, 600, and 800 Gy (the cell morphology of the control and the irradiated sample is shown in Supplementary Figure 1). The first protocol was used in the study of the effect of X-rays on yeast colony formation and yeast growth kinetics. In protocol 2, the C. albicans suspensions (7 ml) with or without the addition of FLC at concentrations of 10 and 1000 mg/L were irradiated by X-rays in the absorbed doses of 300 and 600 Gy. At the dose rate of 5 Gy/min, the process of the irradiation of the cell sample to reach the absorbed dose of 600 Gy took 2 h. To exclude the differences in incubation time before movie recording, the cell samples with the absorbed dose of 300 Gy, control cell samples and cell samples with only FLC treatment were kept under controlled temperature and humidity conditions for 2 h. The period after the start of the experiment and before the first record of ONMD movies was 2 h 20 min for all samples. The second protocol was used in the experiments using the ONMD method.
Yeast colony formation and yeast growth
The plated cells were incubated for 48 h at 28°C, after which the number of colonies with a diameter of 1.0–1.5 2-3 mm was counted. Further, pure cultures from small colonies were accumulated, and their identification was carried out to exclude contamination. The resulting strains were placed in Sabouraud broth with the addition of 30% (v/v) glycerin and frozen at −62°C for long-term storage.
To assess the growth kinetics of pure cultures grown on Sabouraud agar for 2 days at 28°C, suspensions were prepared in a sterile isotonic solution of sodium chloride with an optical density (the absorbance) of 10 McFarland. The resulting suspensions were introduced into glass tubes with Sabouraud broth until an optical density of 0.5 McFarland (densitometer control) was reached, and the estimated concentration was 5 × 106 cells/ml. The cells were incubated in an ES-20 incubator shaker (Biosan, Latvia) at 35°C with orbital stirring of 200 rpm. The optical density was measured at 600 nm every 30 min to determine the biomass of the yeast. The non-irradiated culture served as a control. Afterward, the early stage of growth kinetics (the first 4 h) was fitted by the exponential function:
where OD600 is the optical density at 600 nm depending on the total amount of biomass at time t, a is the initial OD600, parameter R0 is related to the yeast growth rate, and b is an arbitrary constant. The parameters a, b, and R0 were determined by fitting the experimentally obtained data (OriginPro software).
The dependence of parameter R0 on the absorbed dose (D) was fitted by the exponential function:
where c, k, and d are arbitrary constants determined by fitting the experimentally data (OriginPro software).
Optical nanomotion experiment
Twenty μl of the yeast cell suspension was dispensed onto a glass slide (MiniMed, Russia) between two Scotch tapes fixed parallel to each other and perpendicular to the longer side of the slide, then covered by a coverslip (Figure 1). Scotch tapes in such construction create an imaging chamber with a thickness of 150 μm. To stabilize the cell movement state in the chamber, the yeast cells were allowed to sediment for a period of 10 min before starting the measurement. The motion of cells was observed by taking movies (Supplementary Video 1) of 300 frames with a framerate of about 6 frames per second (fps) using a MiniMed 502 microscope with a 40× objective, a BestScope camera, and the TC-Capture software. Recordings were done at room temperature and humidity of 45 ± 5 %.
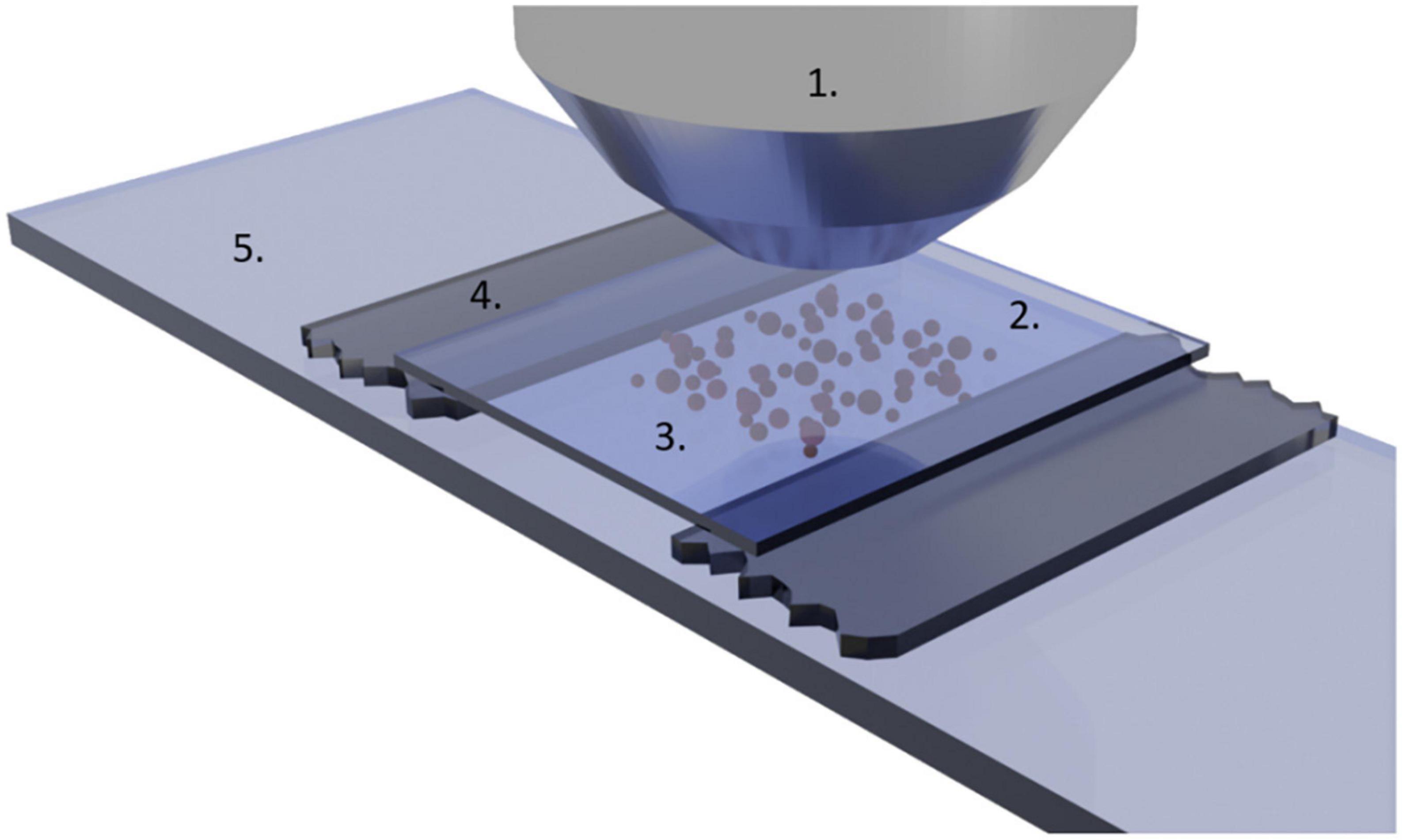
Figure 1. Experimental setup for optical nanomotion detection. (1) Microscope objective, (2) coverslip, (3) analysis chamber with yeast cells (not at scale), (4) 150-micrometer high double face rubber tape, and (5) sample holder glass.
Nanomotion data processing
The ONMD algorithm described previously was used for data processing (Willaert et al., 2020). The main part of the program is based on the algorithm of Guizar-Sicairos et al. (2008) work. The software calculates the cell displacement for each frame. The data of the cell displacements were represented as the following data set:
where i was an adjacent number of cell in a sample (i = 1, k; k varied from 5 to 30), j was an adjacent number of a frame for each cell in a sample (j = 1, n). For each cell the average rate of cellular nanomotion for a certain Δt was calculated using the Euclidean distance Si,j between two cell positions adjacent in time and time interval between two adjacent frames Δt:
The average time interval was Δ t = 0.21 ± 0.02 s. Based on the obtained results data sets (t,V)i,j were formed for each cell sample. The nanomotion cell rate was measured in nm/10 ms. A discrete Fourier transform was performed to the data set (t,V)i,j using Fast Fourier Transform (FFT) option of OriginLab software. Discrete Fourier transform is the basis for many signal processing procedures. It converts a signal from the time domain into the frequency domain. For further analysis, we used RMS amplitude frequency spectrum. Image J software was used to determine the cell diameter using light microscopy images. The diameter of G0-phase cells normally having a spheroidal shape was determined as the average of their maximal and minimal sizes. The diameter of budding cells (in S/G2 phase of the cell cycle) having the shape of two linked spheroids were roughly approximated as a diameter of one spheroidal cell times 1.3.
Statistical analysis
Statistical analysis of experimental data was performed using OriginPro, version 2019b and Statistical calculator ‘‘Statistical Kingdom.’’1 The data were checked for compliance with the normal distribution law using the Kolmogorov-Smirnov test. The data are represented as either the median and limits of the interquartile range [Me(LQ; UQ)] or the mean and the limits of 95% confidence interval (95% CI). Multiple comparison analysis was performed using Kruskal-Wallis ANOVA by ranks test with Bonferroni’s correction and Dunn’s test.
Results
The effect of X-rays on the C. albicans colony formation and growth
In the first step, we studied the effect of X-rays on C. albicans colony morphology and growth rate. It permitted us to determine the X-ray doses to use in further ONMD experiments. The colonies that were not exposed to X-rays displayed a typical morphology, i.e., a diameter of 2–3 mm with a hemispherical shape and smooth edges. The samples irradiated by X-rays displayed two different types of colonies: colonies with typical morphology and small colonies with a diameter of about 1 mm (Figures 2A, B). By increasing the absorbed dose in the range of 0–800 Gy, a decrease in the number of colonies with typical morphology and an increase in the number of small colonies was observed (Figure 2C).
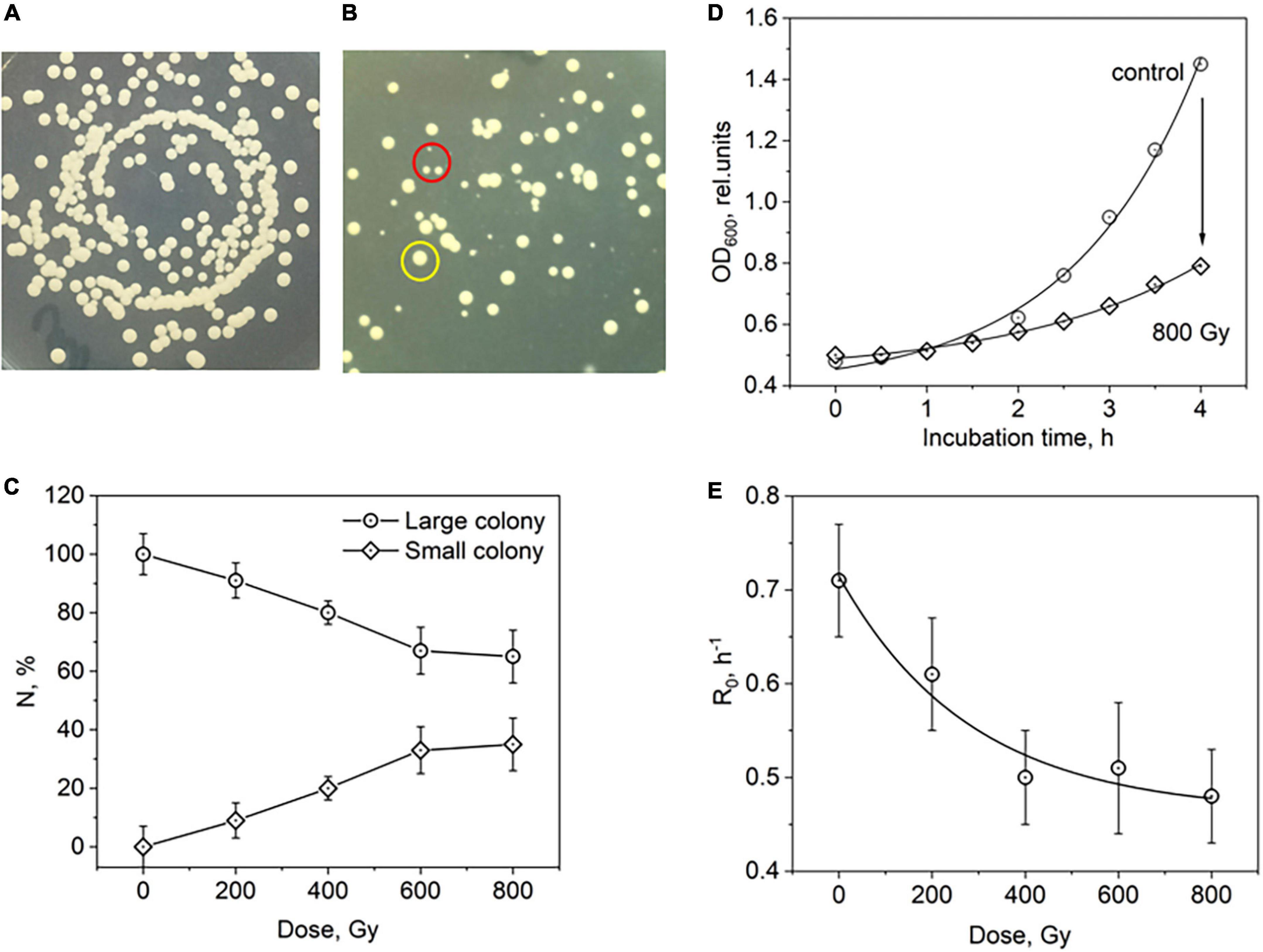
Figure 2. X-ray absorbed dose effect on Candida albicans colony formation and growth parameters. (A,B) X-ray induced changes in the C. albicans colony formation. (A) Control sample (non-irradiated control sample), (B) yeast cell sample after irradiation by X-rays a dose of 800 Gy. Two types of yeast colonies, large (yellow circle) and small (red circle), are presented in the sample. (C) The percentage of two types of yeast colonies depending on the absorbed dose. (D) The dependence of C. albicans biomass on the adsorbed dose of X-ray radiation for the non-irradiated control sample and cells exposed to X-rays (800 Gy). The yeast biomass was assessed using the absorbance (OD) at 600 nm. The kinetic curves of yeast growth were fitted with exponential function: OD600 = a + beR0t, R2>0.994. Parameter R0 is related to yeast growth rate and measured in h– 1. (E) The X-ray induced decrease in the yeast growth rate. The dose-dependence of yeast growth rate was fitted with exponential function: R0 = 0.46273 + 0.252e−(x/282.57), R2 = 0.961, d = 282.57 Gy.
Ionizing radiation is known to inhibit cell growth and cell division. We studied the yeast growth kinetics at the early stage of growth during the first 4 h (Figure 2D). The growth kinetics at this stage is well fitted by an exponential curve. The coefficient Ro in the exponential function reflects the growth rate of the yeast biomass. With an increase in the absorbed dose in the dose range up to 800 Gy, this coefficient decreases exponentially (Figure 2E). Both obtained dose-dependent kinetics for the number of small colony appearance and the growth rate decrease revealed the quick change of the parameters in the range of 0–400 Gy and their relative stabilization in range of 600-800 Gy (Figures 2C, E). For the next experiments, we used an absorbed dose of 600 Gy as relative a high dose (HD) and a dose of 300 Gy as a relative low dose (LD).
The effect of X-rays and antifungal drug on the nanomotion of C. albicans
In a next step, we studied C. albicans nanomotion after irradiation with X-rays, FLC treatment and the combination of the two factors. To assess the effect of the incubation time after irradiation or FLC treatment we monitored the cellular oscillations at 2.5, 3, 3.5, and 24 h after the exposure. The nanomotion of control yeast cells was revealed not to be time dependent, at least within a 24 h period (Figure 3A). In the case of irradiation by HD (600 Gy) X-rays, the nanomotion depended on the incubation time, with a maximal value at 3–4 h (Figure 3B). By the end of the 24-h incubation period, the nanomotion activity of yeast cells decreased to 5.3 (3.6, 8.0) times compared to the maximal observed value at 3.5 h and to 3.7 (2.5, 6.4) times as compared to control values (Kruskal-Wallis ANOVA by ranks test, Dunn’s test post-hoc analysis: p < 10–6).
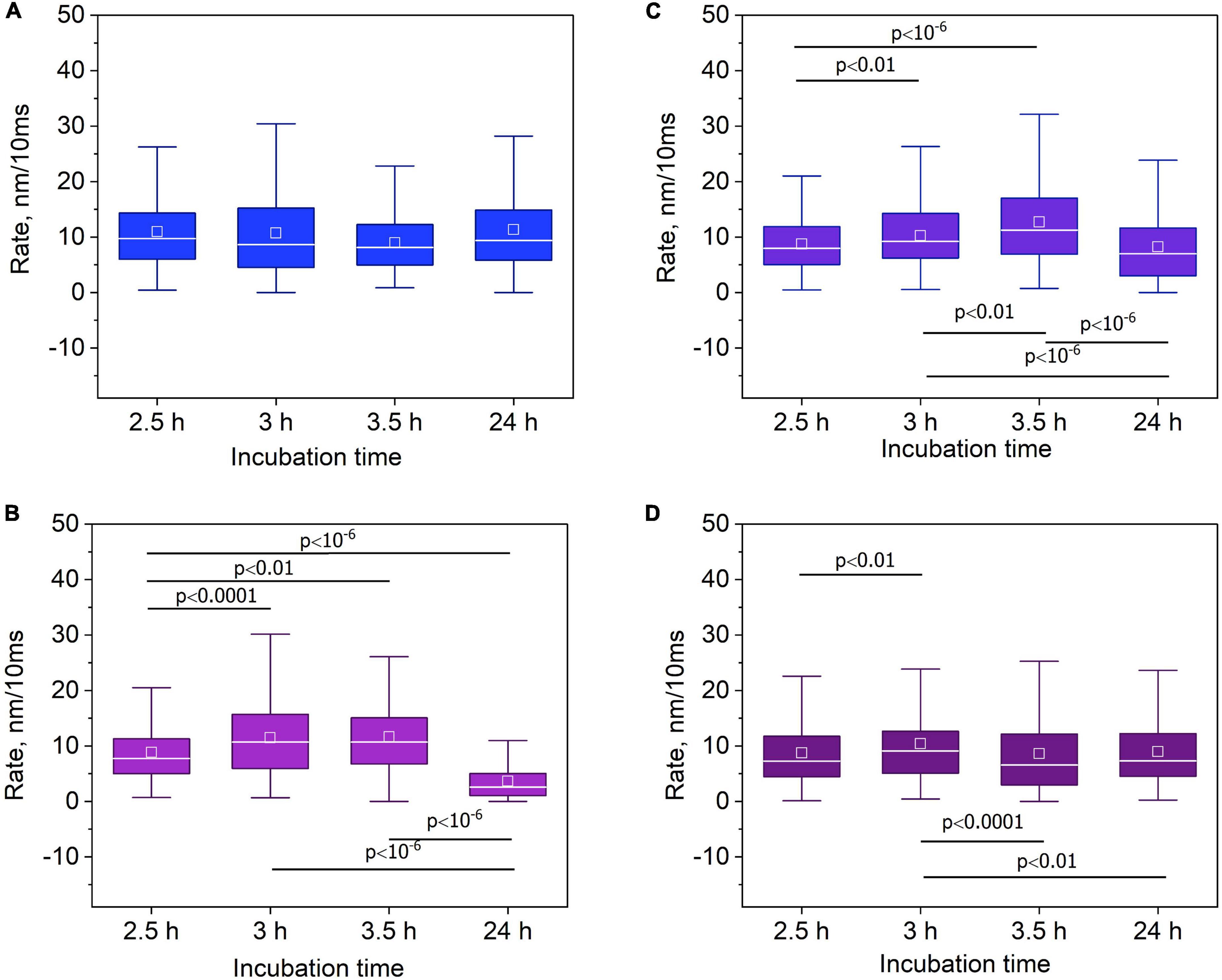
Figure 3. The effect of X-ray irradiation and antifungal drug treatment on the nanomotion rate of Candida albicans. The absorbed dose was 600 Gy, the concentration of FLC was 1000 mg/L. (A) The absence of significant changes in the nanomotion rate for the control cell sample (non-irradiated and untreated) (Multiple comparison (Dunn’s test) post-hoc analysis with Bonferroni’s correction: p > 0.008333). (B) Time-dependent behavior of the nanomotion rate for the irradiated cell samples. ANOVA by ranks test (p < 10– 6); multiple comparison (Dunn’s test) post-hoc analysis. (C) Time-dependent behavior of the nanomotion rate for the FLC-treated cell samples. ANOVA by ranks test (p < 10– 6); multiple comparison (Dunn’s test) post-hoc analysis. (D) Time-dependent behavior of the nanomotion rate for the FLC-treated and irradiated cell samples. ANOVA by ranks test (p = 0.006913); multiple comparison (Dunn’s test) post-hoc analysis. Data are represented as median (line), mean (square), IQR (box), and 1.5 IQR (whiskers).
A similar nanomotion pattern was observed after treatment with FLC at high concentration (1000 mg/L) (Figure 3C). The maximal nanomotion activity was reached after 3.5 h following the antifungal treatment. By the end of a 24-h period the nanomotion significantly decreased to 1.6 (1.5;2.3) times compared to the observed value at 3.5 h (Kruskal-Wallis ANOVA by ranks test, Dunn’s test post-hoc analysis: p < 10–6). After the combined action of the two factors, i.e., radiation and antifungal treatment, the kinetic curve did not present a clear maximum and a decrease in the nanomotion rate started after 3 h (Figure 3D); importantly, in a lower extent as compared to either irradiation or FLC treatment alone.
Our results and literature data show that the nanomotion rate is time-dependent and dose-dependent when the cells are exposed to X-rays, and time-dependent and concentration-dependent when the cells treated with antifungal drugs (Willaert et al., 2020). Using a relatively low (10 mg/L) and relatively high (1000 mg/L) concentration of FLC, we studied the C. albicans nanomotion after exposure to X-rays at the absorbed dose of 300 and 600 Gy at a fixed incubation time (3.5 h) (Supplementary Figure 2 and Supplementary Table 1). A low radiation dose influenced insignificantly the nanomotion in case of the absence of FLC. At low FLC (10 mg/L) concentration, the X rays slightly inhibited the nanomotion of yeast cells. At high FLC concentrations and a dose of 300 Gy the distribution of the nanomotion rates showed two peaks: one peak was close to the control values (about 8–9 nm/10 ms) and another one was shifted to the range of lower values (about 6–7 nm/10 ms). A high radiation dose intensified the nanomotion as compared to a lower dose in control cell samples. FLC supports to inhibition of the HD radiation-induced elevated level of nanomotion.
To better characterize the obtained nanomotion signals we carried out spectral analysis, which reflects the structure and the nature of the oscillating cells (Jelínek et al., 2009; Wohl and Sherman, 2021). The analysis permitted identifying frequencies that significantly decreased after 24 h upon X-rays irradiation. Spectral analysis was performed for Candida cells in two different phases of the cell cycle. Non-budding yeasts were classified as G1 or G0 phase and budding yeasts as S or G2 phase. A high percentage of cells were in the S/G2 phase of the cell cycle (70%) 24 h after the start of the experiment. Initial cultures at time zero were comprised predominantly (>90%) of G1/G0-phase cells, which agrees with literature data on S. cerevisiae (Hafer et al., 2010). From the nanomechanical point of view, the oscillatory behavior of non-budding and budding yeast cells is expected to be different because of the difference in the mass of single cells in the G1/G0 phase (one cell) and in S/G2 phase (two new cells). It should be pointed out that all results presented in the previous paragraph were obtained using yeast cells in G1/G0 phase.
Figure 4 displays the nanomotion spectral parameters after 24 h exposure as a function of the cell cycle. The scheme of the analysis of cell nanomotion data for the control and X-ray irradiated cultures is represented in Figure 4C. For cells in the G0 phase (Figure 4A), a deep dip at frequencies of 0.55–1.60 Hz, which appeared in the spectrum of cell oscillation RMS amplitudes after irradiation with X-rays, attracts attention. As one would expect for budding cells, the oscillation amplitudes of the control and the irradiated culture were lower for almost all analyzed frequencies (Figures 4A, B). This frequency range coincides with the one that was mentioned previously as a “cell activity region” for cell nanomotion (Willaert et al., 2020). Because the different factors differently influenced the cell morphology, we analyzed the relationships between RMS amplitude averaged over the frequency range of 0.55–1.60 Hz and the averaged cell diameter (Figure 4D). In general, budding cells (points 1′-4′) oscillate with lower amplitude at the nanoscale compared to no-budding cells (points 1–4). But for FLC-treated cells, the difference between amplitudes for two cell cycle phases is absent (points 3-3′). A similar situation is observed for X-ray irradiated cells (points 2-2′). For the combined action of X-rays and the antifungal drug, the difference between the amplitudes of budding and non-budding cells was significant (points 4′-4) and like the ones observed for the control culture (points 1′-1).
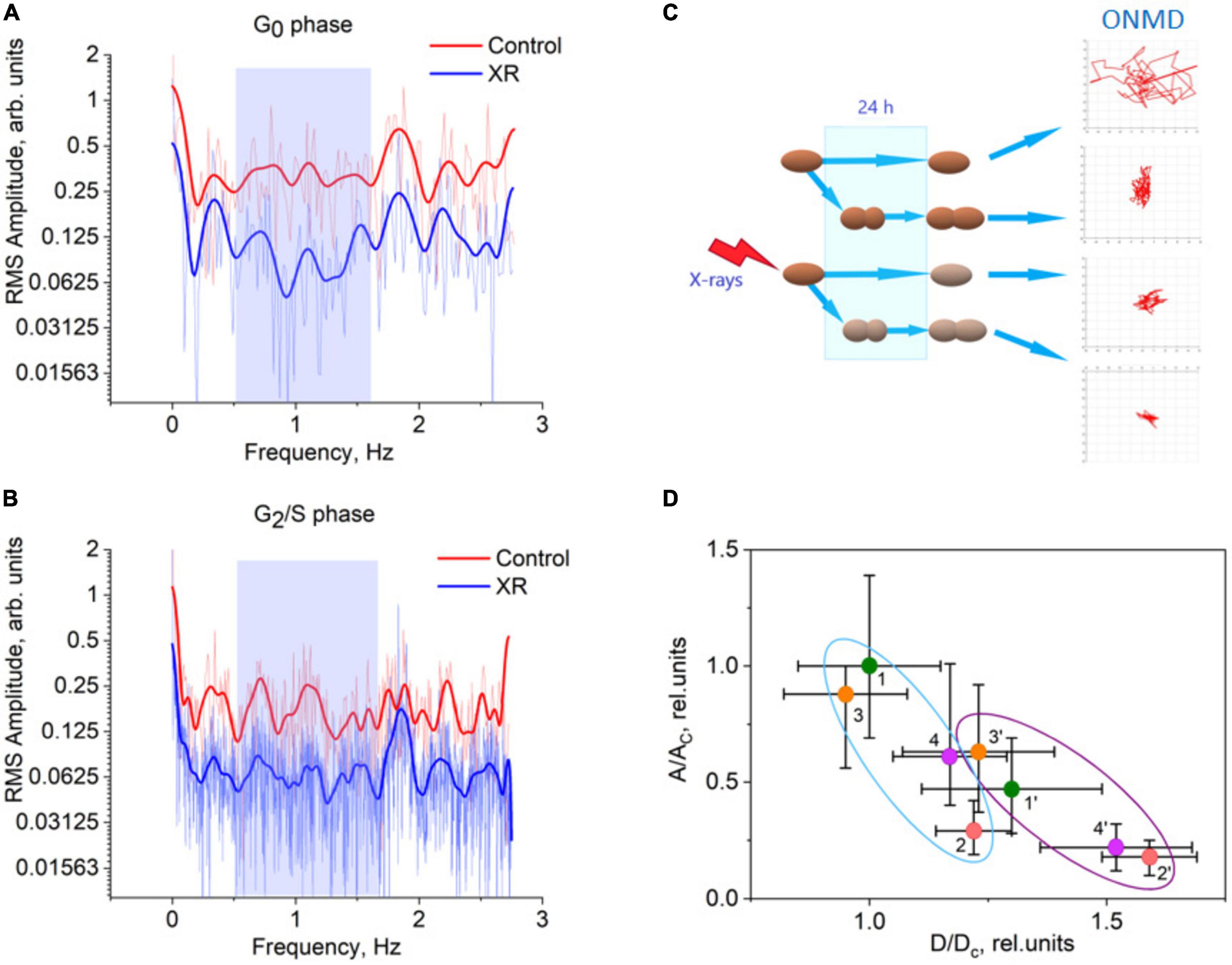
Figure 4. Spectral analysis of Candida albicans nanomotion rate at 24 h incubation time after experiment start. (A,B) Fourier transform spectrum for the RMS amplitude of the nanomotion rate. G0-phase yeast cells (A) S/G2-phase yeast cells (B). The blue rectangle in the figures shows the frequency range of 0.55–1.60 Hz that lies between two Fourier spectrum peaks that is characteristic for all the C. albicans samples studied (about 0.3 and 1.9 Hz). Pale curves represent experimental data, bright colored curves represent the results of the curve smoothing by the FFT filter method. (C) The scheme of the analysis of cell nanomotion data for control (non-irradiated and untreated) and X-ray irradiated cultures. (D) The relative RMS amplitude of cell nanomotion rate averaged over the frequency range of 0.55–1.60 Hz against the relative averaged cell diameter for C. albicans after their exposure to various factors. Relative RMS amplitude was calculated with respect to the median RMS amplitude of the control yeast culture. A is the RMS amplitude of the studied sample, Ac is the RMS amplitude of the control (non-irradiated and untreated) sample. Relative cell diameter was assessed using light microscopy images and ImageJ software and calculated with respect to the median cell diameter of the control yeast culture. D is the cell diameter in the studied C. albicans sample, Dc is the cell diameter of the control C. albicans culture. The diameter of cells normally having spheroidal shape was assessed as the average of their maximal and minimal sizes. The diameter of budding cells having the shape of two linked together spheroids is roughly approximated as the diameter of spheroidal cell times 1.3. The cell samples were marked using the following numbers and colors: control (1, 1′, green circles), X-ray irradiated (2, 2′, scarlet circles), FLC-treated (3, 3′, orange circles) and FLC-treated and X-ray irradiated (4, 4′, lilac circles), where 1, 2, 3, and 4 (included into light blue oval) represent the data for G0-phase cells, 1′, 2′, 3′, and 4′ (included into violet oval) represent the data for G2/S-phase cells.
Discussion
In the first part of this work, we studied the time-dependent changes of nanomotion of C. albicans cells after exposure to X-rays. In classical radiobiology, the effect of ionizing radiation on cells is characterized by their survival and proliferation potential. The dependence of C. guilliermondii yeast cell survival fraction after X-ray exposure on the absorbed dose has been shown to be represented as a sigmoid curve (Navasardyan et al., 2017). The sigmoid survival curve indicates the presence of a well-developed mechanism of radiation-induced damage repair in cells. With an increase in the radiation dose, the effectiveness of the repair system decreases due to damage to the repair system itself. The Candida yeast species are more radio-resistant compared to other yeast cells such as for example Saccharomyces cerevisiae. For C. guilliermondii, the LD50 (semi-lethal dose) value for X-rays is about 720 Gy (Navasardyan et al., 2017), and for S. cerevisiae 300 Gy (Navasardyan et al., 2017) or even 60 Gy (Guo et al., 2018a). The radiation-induced decrease in the percentage of surviving cells leads to the lengthening of the lag phase of the growth of X-ray-irradiated yeast. Further incubation of damaged cells proceeds with DNA repair, and the rate of biomass accumulation gradually increases; by about the end of the first day, the biomass of irradiated yeast can reach the value characteristic of non-irradiated yeast (Navasardyan et al., 2017). The absorbed doses of X-rays used in the present work is sub-semi-lethal dose for C. albicans. X-rays gradually inhibit the yeast growth rate within the early stage of the sigmoid curve of their biomass increase but can potentially stimulate repair and proliferation in cells with an intensification of cellular metabolic processes. Recently in a study on the damage-repair response of S. cerevisiae following heavy-ion beam irradiation (semi-lethal dose is 80 Gy), it has been established that the peak of the damage-repair response occurred 75 min post-irradiation (Guo et al., 2020). Based on the analyses of multiple biological effects, the phenotypic damages in S. cerevisiae cells induced by a semi-lethal dose of X-ray irradiation could be repaired to a great extent within 4 h, and the 0–4 h period is an important time frame to investigate the biological effects induced by semi-lethal doses of X-rays (Guo et al., 2018b). Our results are in agreement with this hypothesis. We observed a significant increase in the nanomotion rate of C. albicans 3 to 4 h post irradiation in a sub-semi-lethal dose that can be explained by the transitory increase of the metabolic activity.
The post-irradiation change in metabolic activity can be assessed by monitoring CO2 production (Masini et al., 1997). It was revealed that the CO2 production by S. cerevisiae yeast cells irradiated by soft X-rays decreased in the dose range of 0 to 40 Gy, and for a dose of 2000 Gy it slightly increased. Also, metabolic oscillations (measured by the CO2 production of cells) were evidenced in cell suspensions and the oscillation frequencies were changed after the X-ray irradiation (Masini et al., 1997).
In our experiments a significant decrease of the nanomotion of X-ray-irradiated C. albicans by 24 h was observed for cell oscillations with frequencies lying in the range of 0.55–1.60 Hz. The radiation-induced decrease of the oscillation amplitude seems to be slightly dependent on the cell cycle phase. The amplitude of the nanomotion rate within the frequency range of 0.55–1.60 Hz for G0-phase cells decreased by 3.37 ± 0.68 times and for G2/S-phase cells by 2.61 ± 0.32 times. The ionizing radiation effects are well-known to be determined by the cell cycle phase. The budding yeast cells are more resistant to radiation inactivation than non-budding yeast (Raju et al., 1972; Langguth and Beam, 1973). As a rule, exponentially growing yeast cells are more resistant to ionizing radiation damage than cells in the stationary phase or G1 (Tippins and Parry, 1982). Both yeast and mammalian cultures in vitro are most susceptible to radiation mutations in the early stages of the cell cycle. In the later stages of the cell cycle, yeast cells are most sensitive to the formation of homologous DNA deletion. It was shown that for S. cerevisiae within the first 30 h after X-ray irradiation, the magnitude of radiation-induced DNA deletions correlated positively with the fraction of cells in the S/G2 phase (Hafer et al., 2010).
In a second part of this work, we compared the nanoscale oscillatory behavior of C. albicans after X-ray irradiation and after antifungal drug treatment. Though FLC exhibits concentration-dependent fungicidal activity, it was shown that no membrane destruction could be observed in a wide range of concentrations (Lee and Lee, 2018). At high concentrations of FLC, both potassium efflux and cell shrinkage have been observed. FLC also causes concentration-dependent apoptotic responses, including phosphatidylserine externalization and DNA fragmentation. It was also involved in glutathione depletion followed by oxidative damage. A high concentration of FLC causes the disruption of mitochondrial homeostasis, including mitochondrial membrane depolarization and accumulation of calcium and reactive oxygen species (ROS) in C. albicans (Kobayashi et al., 2002; Wang et al., 2009; Mahl et al., 2015) and in C. neoformans ( Peng et al., 2018; Dbouk et al., 2019). It was verified that the ROS were involved in FLC-mediated growth inhibition by determining ROS-scavenging proteins and metallothioneins (Dbouk et al., 2019). ROS are generated during basic cellular processes, or due to external stress-inducing conditions, including exposure to X-rays. So, the mechanisms of action of both factors, FLC and X-rays, on C. albicans include ROS, and, therefore, the change in the cell nanomotion in both cases may have common features. A temporary increase in the nanomotion rate during the first 4 h for irradiated cells and cells treated with FLC was observed for both treatments. It illustrates a similar cellular response to two very different disturbances. However, there are also obvious differences in the effects of X-ray and FLC on C. albicans nanomotion. After exposure to X-ray radiation alone or in combination with FLC, low-frequency nanoscale oscillations of whole cells are significantly suppressed depending on the phase of the cell cycle, absorbed dose, FLC concentration, and post-irradiation period.
There are some limitations of the present work. In this study, we used as a model a C. albicans strain that is sensitive to FLC and studied only its planktonic phase. One important characteristic of C. albicans is that it can exist in three phases, i.e., planktonic yeast, pseudohyphae, and hyphae phase differing in their cell morphology, function, and growth conditions (Saghrouni et al., 2013; Chen et al., 2020). Yeast cells which are the default cell morphology under most in vitro conditions, are round or oval with a unicellular morphology. The choice of the radiation dose used in the work, which is 5–10 times higher than that usually used in radiotherapy in the clinic, was based on the high radioresistance of fungal cells to the action of photons of ionizing electromagnetic radiation such as X-rays. When using ionizing particle beams with high LET, the local doses achieved during a course of radiation therapy (radioimmunotherapy and radionuclide therapy) can be very high and comparable to those critical for the viability of fungal cells (Roosen et al., 2021).
Further development of the study is seen in the expansion of types or strains of fungi, as well as the expansion of the range of doses of X-rays, including those widely used for medical purposes. The potential use of the method in the clinic can be justified by the relatively high speed of evaluation of the sensitivity of C. albicans to antifungals and the individual concentration of antifungal drugs in cancer patients undergoing radiation therapy. Since ionizing radiation affects the metabolic activity and functioning of Candida cells, a unique combination of ionizing radiation and antifungals can be experimentally determined to achieve the best treatment result with minimal side effects. To implement a clinically applicable method for assessing cellular activity, it is also necessary to conduct a comparative assessment of the parameters of cell nanomotion in parallel with classical methods for assessing cell viability, such as metabolic activity assay (XTT assay) and colony-forming unit counting. The revealed features of the C. albicans nanomotion and an understanding of the mechanisms of their change under the action of ionizing radiation and antifungals can help to rapidly determine the sensitivity of C. albicans to antifungals and the concentrations of antifungals during repeated courses of radiation therapy for patients with candidiasis.
Moreover, in addition to the medical technology based on the ONMD method discussed in this paper, another technology can potentially be developed, for example, a technology for quality control of irradiation of food products to increase their shelf life and reduce the probability of contamination by microorganisms and toxins that are products of the vital activity of microorganisms, including fungi and mold (Calado et al., 2014).
Long-lasting space flights are well documented to reduce the efficiency of the immune system (Cogoli, 1996; Kelsen et al., 2012) and make astronauts more sensitive to fungal infections (Horneck et al., 2010; Hammond et al., 2013; Senatore et al., 2018; Singh et al., 2018). This technology could rapidly assess sensitivity or resistance to antifungals in microgravity and elevated cosmic radiation environments (e.g., The International Space Station, the Moon, Mars, etc.).
Our work is the first attempt to demonstrate the applicability of the ONMD method for assessing the viability and metabolic activity of fungi cells (C. albicans) after the action of ionizing radiation (X-rays) alone and in combination with antifungals. Although the study has a number of limitations, its results allow us to advance in understanding the mechanisms of cell response to ionizing radiation and antifungal agents and their combinations.
Data availability statement
The original contributions presented in this study are included in the article/Supplementary material, further inquiries can be directed to the corresponding author.
Author contributions
MS: conceptualization, data analysis, supervision, visualization, and original draft preparation. IC: investigation and data curation. NS: investigation, visualization, data analysis, and software. MV: formal analysis, visualization, and software. DT: conceptualization, data curation, formal analysis, and original draft preparation. RW: conceptualization, supervision, and writing—review and editing. SK: conceptualization, project administration, supervision, draft preparation, and writing—review and editing. All authors contributed to the article and approved the submitted version.
Funding
We acknowledge funding by the Belgian Federal Science Policy Office (BELSPO) and the European Space Agency (ESA), grant number PRODEX project Flumias Nanomotion; FWO, grant number I002620; FWO-SNSF, grant number 310030L_197946; MV and SK were supported by SNSF grants CRSII5_173863.
Acknowledgments
The Research Council of the Vrije Universiteit Brussel (Belgium) and the University of Ghent (Belgium) are acknowledged to support the Alliance Research Group VUB-UGhent NanoMicrobiology (NAMI), and the International Joint Research Group (IJRG) VUB-EPFL NanoBiotechnology and NanoMedicine (NANO).
Conflict of interest
The authors declare that the research was conducted in the absence of any commercial or financial relationships that could be construed as a potential conflict of interest.
Publisher’s note
All claims expressed in this article are solely those of the authors and do not necessarily represent those of their affiliated organizations, or those of the publisher, the editors and the reviewers. Any product that may be evaluated in this article, or claim that may be made by its manufacturer, is not guaranteed or endorsed by the publisher.
Supplementary material
The Supplementary Material for this article can be found online at: https://www.frontiersin.org/articles/10.3389/fmicb.2023.1133027/full#supplementary-material
Footnotes
References
Azzam, E. I., Jay-Gerin, J.-P., and Pain, D. (2012). Ionizing radiation-induced metabolic oxidative stress and prolonged cell injury. Cancer Lett. 327, 48–60.
Beam, C. A., Mortimer, R. K., Wolfe, R. G., and Tobias, C. A. (1954). The relation of radioresistance to budding in Saccharomyces cerevisiae. Arch. Biochem. Biophys. 49, 110–122. doi: 10.1016/0003-9861(54)90172-1
Ben-Yosefa, R., Zeiraa, M., and Polacheckb, I. (2005). The effect of radiation therapy on fungal growth: Results of in vitro and in vivo studies. J. Infect. 50, 450–452.
Cagnacci, S., Grasso, R., Marchese, A., Corvò, R., Debbia, E., and Rossi, L. (2008). The susceptibility of Candida albicans to gamma-radiations and ketoconazole depends on transitional filamentation. Open Microbiol. J. 2, 66–73. doi: 10.2174/1874285800802010066
Calado, T., Venancio, A., and Abrunhosa, L. (2014). Irradiation for mold and mycotoxin control: A review. Compr. Rev. Food Sci. Food Saf. 13, 1049–1061. doi: 10.1111/1541-4337.12095
Casadevall, A., and Pirofski, L. A. (2007). Accidental virulence, cryptic pathogenesis, Martians, lost hosts, and the pathogenicity of environmental microbes. Eukaryot. Cell 6, 2169–2174. doi: 10.1128/EC.00308-07
Chen, H., Zhou, X., Ren, B., and Cheng, L. (2020). The regulation of hyphae growth in Candida albicans. Virulence 11, 337–348. doi: 10.1080/21505594.2020.1748930
Cogoli, A. (1996). Gravitational physiology of human immune cells: A review of in vivo, ex vivo and in vitro studies. J. Gravit. Physiol. 3, 1–9.
Costa-de-Oliveira, S., and Rodrigues, A. G. (2020). Candida albicans antifungal resistance and tolerance in bloodstream infections: The triad yeast-host-antifungal. Microorganisms 8:154. doi: 10.3390/microorganisms8020154
da Silva, E. M., Mansano, E., Miazima, E., Rodrigues, F., Hernandes, L., and Svidzinski, T. (2017). Radiation used for head and neck cancer increases virulence in Candida tropicalis isolated from a cancer patient. BMC Infect. Dis. 17:783. doi: 10.1186/s12879-017-2879-6
Dbouk, N. H., Covington, M. B., Nguyen, K., and Chandrasekaran, S. (2019). Increase of reactive oxygen species contributes to growth inhibition by fluconazole in Cryptococcus neoformans. BMC Microbiol. 19:243. doi: 10.1186/s12866-019-1606-4
Gordee, R. S., and Simpson, P. J. (1967). Relationships of x-irradiation to the enhancement of Candida albicans infections. J. Bacteriol. 94, 6–12. doi: 10.1128/jb.94.1.6-12.1967
Guizar-Sicairos, M., Thurman, S. T., and Fienup, J. R. (2008). Efficient subpixel image registration algorithms. Opt. Lett. 33, 156–158. doi: 10.1364/ol.33.000156
Guo, X., Zhang, M., Liu, R., Gao, Y., Yang, Y., Li, W., et al. (2018a). Repair characteristics and time-dependent effects in Saccharomyces cerevisiae cells after X-ray irradiation. World J. Microbiol. Biotech. 35:1. doi: 10.1007/s11274-018-2566-9
Guo, X., Zhang, M., Gao, Y., Li, W., and Lu, D. (2018b). Determining survival fractions of Saccharomyces cerevisiae in response to ionizing radiation in liquid culture. J. Radiat. Res. 59, 760–764. doi: 10.1093/jrr/rry070
Guo, X., Zhang, M., Gao, Y., Lu, D., Li, W., and Zhou, L. (2020). Repair characteristics and time-dependent effects in response to heavy-ion beam irradiation in Saccharomyces cerevisiae: A comparison with X-ray irradiation. Appl. Microbiol. Biotechnol. 104, 4043–4057. doi: 10.1007/s00253-020-10464-8
Hafer, K., Rivina, L., and Schiestl, R. H. (2010). Cell cycle dependence of ionizing radiation-induced DNA deletions and antioxidant radioprotection in Saccharomyces cerevisiae. Radiat. Res. 173, 802–808. doi: 10.1667/RR1661.1
Hammond, T. G., Stodieck, L., Birdsall, H. H., Becker, J. L., Koenig, P., Hammond, J. S., et al. (2013). Effects of microgravity on the virulence of Listeria monocytogenes, Enterococcus faecalis, Candida albicans, and methicillin-resistant Staphylococcus aureus. Astrobiology 13, 1081–1090. doi: 10.1089/ast.2013.0986
Horneck, G., Klaus, D. M., and Mancinelli, R. L. (2010). Space microbiology. Microbiol. Mol. Biol. Rev. 74, 121–156. doi: 10.1128/MMBR.00016-09
Ingrosso, G., Saldi, S., Marani, S., Wong, A. Y. W., Bertelli, M., Aristei, C., et al. (2021). Breakdown of symbiosis in radiation-induced oral mucositis. J. Fungi 7:290. doi: 10.3390/jof7040290
Jelínek, F., Cifra, M., Pokorný, J., Vaniš, J., Šimša, J., Hašek, J., et al. (2009). Measurement of electrical oscillations and mechanical vibrations of yeast cells membrane around 1 kHz. Electromagn. Biol. Med. 28, 223–232. doi: 10.1080/15368370802710807
Kasas, S., Ruggeri, F. S., Benadiba, C., Maillard, C., Stupar, P., Tournu, H., et al. (2015). Detecting nanoscale vibrations as signature of life. Proc. Natl. Acad. Sci. U.S.A. 112, 378–381. doi: 10.1073/pnas.1415348112
Kelsen, J., Bartels, L. E., Dige, A., Hvas, C. L., Frings-Meuthen, P., Boehme, G., et al. (2012). 21 Days head-down bed rest induces weakening of cell-mediated immunity—some spaceflight findings confirmed in a ground-based analog. Cytokine 59, 403–409. doi: 10.1016/j.cyto.2012.04.032
Kobayashi, D., Kondo, K., Uehara, N., Otokozawa, S., Tsuji, N., Yagihashi, A., et al. (2002). Endogenous reactive oxygen species is an important mediator of miconazole antifungal effect. Antimicrob. Agents Chemother. 46, 3113–3117. doi: 10.1128/AAC.46.10.3113-3117.2002
Langguth, E. N., and Beam, C. A. (1973). Repair mechanisms and cell cycle dependent variations in X-ray sensitivity of diploid yeast. Radiat. Res. 53, 226–234.
Lee, W., and Lee, D. G. (2018). A novel mechanism of fluconazole: Fungicidal activity through dose-dependent apoptotic responses in Candida albicans. Microbiology (Reading) 164, 194–204. doi: 10.1099/mic.0.000589
Longo, G., Alonso-Sarduy, L., Rio, L. M., Bizzini, A., Trampuz, A., Notz, J., et al. (2013). Rapid detection of bacterial resistance to antibiotics using AFM cantilevers as nanomechanical sensors. Nat. Nanotechnol. 8, 522–526. doi: 10.1038/nnano.2013.120
Mahl, C., Behling, C., Hackenhaar, F., de Carvalho e Silva, M., Putti, J., Salomon, T., et al. (2015). Induction of ROS generation by fluconazole in Candida glabrata: Activation of antioxidant enzymes and oxidative DNA damage. Diagn. Microbiol. Infect. Dis. 82, 203–208. doi: 10.1016/j.diagmicrobio.2015.03.019
Mañas, A., Cerezo, L., de la Torre, A., García, M., Alburquerque, H., Ludeña, B., et al. (2012). Epidemiology and prevalence of oropharyngeal candidiasis in Spanish patients with head and neck tumors undergoing radiotherapy treatment alone or in combination with chemotherapy. Clin. Transl. Oncol. 14, 740–746. doi: 10.1007/s12094-012-0861-8
Masini, A., Batani, D., Previdi, F., Conti, A., Pisani, F., Botto, C., et al. (1997). X-ray irradiation of yeast cells. Proc. SPIE 3157, 203–217.
Navasardyan, L., Marutyan, S., Hovnanyan, K., and Trchounian, A. (2017). Survival and changes in morphology, mitotic and metabolic activity of yeast Candida guilliermondii exposed to X-irradiation. Ind. J. Biochem. Biophys. 54, 273–280.
Parry, J. M., Sharp, D., Tippins, R. S., and Parry, E. M. (1979). Radiation-induced mitotic and meiotic aneuploidy in the yeast. Mutat. Res. 61, 37–55. doi: 10.1016/0027-5107(79)90005-8
Peng, C. A., Gaertner, A. A. E., Henriquez, S. A., Fang, D., Colon-Reyes, R. J., Brumaghim, J. L., et al. (2018). Fluconazole induces ROS in Cryptococcus neoformans and contributes to DNA damage in vitro. PLoS One 13:e0208471. doi: 10.1371/journal.pone.0208471
Pinel, B., Cassou-Mounat, T., and Bensadoun, R. J. (2012). Oropharyngeal candidiasis and radiotherapy. Cancer Radiother. 16, 222–229. doi: 10.1016/j.canrad.2011.11.004
Radonicic, V., Yvanoff, C., Villalba, M. I., Kasas, S., and Willaert, R. G. (2022). The dynamics of single-cell nanomotion behaviour of Saccharomyces cerevisiae in a microfluidic chip for rapid antifungal susceptibility testing. Fermentation 8:195. doi: 10.3390/fermentation8050195
Raju, M. R., Gnanapurani, M., Stackler, B., Madhvanath, U., Howard, J., Lyman, J. T., et al. (1972). Influence of linear energy transfer on the radioresistance of budding haploid yeast cells. Radiat. Res. 51, 310–317.
Roosen, J., Klaassen, N., Westlund Gotby, L., Overduin, C., Verheij, M., Konijnenberg, M., et al. (2021). To 1000 Gy and back again: A systematic review on dose-response evaluation in selective internal radiation therapy for primary and secondary liver cancer. Eur. J. Nucl. Med. Mol. Imaging 48, 3776–3790. doi: 10.1007/s00259-021-05340-0
Saghrouni, F., Ben Abdeljelil, J., Boukadida, J., and Ben Said, M. (2013). Molecular methods for strain typing of Candida albicans: A review. J. Appl. Microbiol. 114, 1559–1574. doi: 10.1111/jam.12132
Senatore, G., Mastroleo, F., Leys, N., and Mauriello, G. (2018). Effect of microgravity and space radiation on microbes. Future Microbiol. 13, 831–847. doi: 10.2217/fmb-2017-0251
Silva, A. F. D., Farias, J. R., Franco, D. C. G., Galiza, A. A., Motta, E. P., Oliveira, A. D. S., et al. (2022). Anti-Candida albicans activity of ononin and other secondary metabolites from Platonia insignis MART. Metabolites 12:1014. doi: 10.3390/metabo12111014
Singh, N. K., Wood, J. M., Karouia, F., and Venkateswaran, K. (2018). Succession and persistence of microbial communities and antimicrobial resistance genes associated with international space station environmental surfaces. Microbiome 6:204. doi: 10.1186/s40168-018-0585-2
Tippins, R. S., and Parry, J. M. (1982). A comparison of the radiosensitivity of stationary, exponential, and G1 phase wild type and repair deficient yeast cultures: Supporting evidence for stationary phase yeast cells being in G0. Int. J. Radiat. Biol. Relat. Stud. Phys. Chem. Med. 41, 215–220. doi: 10.1080/09553008214550231
Ueta, E., Tanida, T., Yoneda, K., Yamamoto, T., and Osaki, T. (2001). Increase of Candida cell virulence by anticancer drugs and irradiation. Oral Microbiol. Immunol. 16, 243–249. doi: 10.1034/j.1399-302x.2001.160408.x
Wang, Y., Jia, X.-M., Jia, J.-H., Li, M.-B., Cao, Y.-Y., Gao, P.-H., et al. (2009). Ascorbic acid decreases the antifungal effect of fluconazole in the treatment of candidiasis. Clin. Exp. Pharmacol. Physiol. 36, e40–e46. doi: 10.1111/j.1440-1681.2009.05187.x
Willaert, R. G., Vanden Boer, P., Malovichko, A., Alioscha-Perez, M., Radotić, K., Bartolić, D., et al. (2020). Single yeast cell nanomotions correlate with cellular activity. Sci. Adv. 6:eaba3139. doi: 10.1126/sciadv.aba3139
Keywords: optical nanomotion detection, Candida albicans, X-rays, antifungal drugs, fluconazole, spectral analysis
Citation: Starodubtseva MN, Chelnokova IA, Shkliarava NM, Villalba MI, Tapalski DV, Kasas S and Willaert RG (2023) Modulation of the nanoscale motion rate of Candida albicans by X-rays. Front. Microbiol. 14:1133027. doi: 10.3389/fmicb.2023.1133027
Received: 28 December 2022; Accepted: 06 March 2023;
Published: 21 March 2023.
Edited by:
Jonathan Schmitz, Vanderbilt University Medical Center, United StatesReviewed by:
Melphine Harriott, Belmont University, United StatesShelley Campeau, Scientific and Medical Affairs Consulting LLC, United States
Ewerton Garcia de Oliveira Mima, São Paulo State University, Brazil
Copyright © 2023 Starodubtseva, Chelnokova, Shkliarava, Villalba, Tapalski, Kasas and Willaert. This is an open-access article distributed under the terms of the Creative Commons Attribution License (CC BY). The use, distribution or reproduction in other forums is permitted, provided the original author(s) and the copyright owner(s) are credited and that the original publication in this journal is cited, in accordance with accepted academic practice. No use, distribution or reproduction is permitted which does not comply with these terms.
*Correspondence: Maria N. Starodubtseva, maria.n.starodubtseva@gmail.com
†These authors have contributed equally to this work