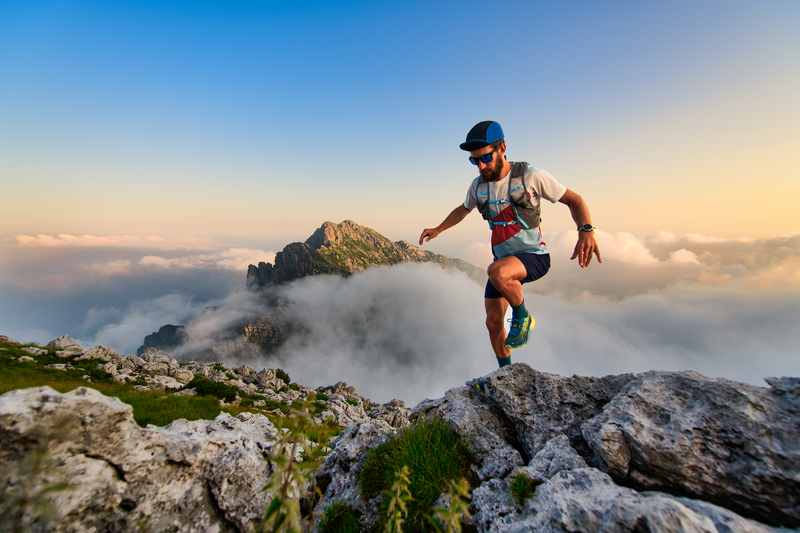
94% of researchers rate our articles as excellent or good
Learn more about the work of our research integrity team to safeguard the quality of each article we publish.
Find out more
ORIGINAL RESEARCH article
Front. Microbiol. , 11 April 2023
Sec. Antimicrobials, Resistance and Chemotherapy
Volume 14 - 2023 | https://doi.org/10.3389/fmicb.2023.1132042
Hinokitiol (β-thujaplicin) is an important component of the essential oil extracted from Chamaecyparis obtuse, which prevents the decay and decomposition of temple and shrine buildings in Japan. Hinokiol has been shown to have a detrimental effect on various fungi such as Candida albicans and saprophytic fungi. However how hinokitiol works against Aspergillus fumigatus (A. fumigatus) has not been claimed. This study aims to investigate the adverse effects of hinokitiol on the disruption of the cell wall and cell membrane of A. fumigatus and to explore possible potential mechanisms or pathways. According to our results, hinokitiol negatively altered mycelium morphology, growth density, and cell plasma composition content. When incubated with human corneal epithelial cells (HCECs), hinokitiol saw a safe effect with concentrations below 12 μg/ml. Hinokitiol was shown to increase the cell membrane’s permeability by decreasing the cell membrane’s ergosterol content. The integrity of the cell wall was disrupted, as well as a significant increase in chitin degradation and chitinase activity. As determined by RNA-seq results, subsequent analysis, and qRT-PCR, altered transcript levels of cell walls and cell membranes-related genes (such as eglC) illustrated how hinokitiol affected the genetic profile of A. fumigatus. With this study, we recommend hinokitiol as an effective anti-A. fumigatus agent by reducing the amounts of key components in the cell wall and membrane by preventing production and accelerating breakdown.
Aspergillus fumigatus is considered a potent opportunistic pathogen that can cause a wide range of diseases in humans worldwide (Latgé and Chamilos, 2019). Its ability to adapt and proliferate in harsh environments contributes to resistance and survival against human host defense systems (Latgé and Chamilos, 2019; Fisher et al., 2022). Currently, Aspergillosis can be treated with a few therapeutic agents from three main classes: echinocandins, antimycotics, and amphotericin B (Pasqualotto and Denning, 2008; Wiederhold, 2018; Latgé and Chamilos, 2019). Their antifungal properties are limited by their toxicity and have other drawbacks in clinical applications (Fan et al., 2020). A new need for innovative agents to combat A. fumigatus emerged as a result.
Compounds with powerful antifungal properties derived from natural plants have recently received much attention. In Japan, Chamaecyparis obtuse is an excellent building material in the construction of Buddhist temples and Shinto shrines (Anderson and Gripenberg, 1948; Inamori et al., 2006). Hinokitiol, a naturally occurring substance associated with Chamaecyparis obtuse, was found to have excellent antifungal properties (Komaki et al., 2008; Rebia et al., 2019). Hinokitiol was found to prevent the growth of Candida species by disrupting the respiratory chain and blocking the signaling of the Renin-angiotensin system (Komaki et al., 2008; Jin et al., 2021). And it also has a desirable anti-biofilm effect on fluconazole-resistant Candida albicans (Kim et al., 2017). Moreover, in Japan, hinokitiol is approved for the treatment of oral candidiasis (Sakaguchi, 2017). It has also been shown to prevent postharvest diseases during the storage of fruits or vegetables, such as those caused by Fusarium sp (Wang et al., 2020), Sclerotinia sclerotiorum (Qiao et al., 2022), and Alternaria alternata (Vanitha et al., 2019).
To date, no studies have reported the antifungal effect of hinokitiol on A. fumigatus, and the exact mechanism by which hinokitiol is used to injure A. fumigatus is not clear. We hypothesize that hinokitiol acts as an antifungal agent by affecting the cell wall and cell membrane of A. fumigatus. In this study, we evaluated specific cell wall and membrane damage conditions and growth inhibition induced by hinokitiol and identified and validated Hub genes by bioinformatics analysis. In conclusion, we provide hinokitiol as a potential new anti-A. fumigatus agent.
Sigma-Aldrich Inc. (Shanghai, China) provided the hinokitiol powder (CAS 499–44-5), which was then dissolved into dimethyl sulfoxide (DMSO) to a storage concentration of 32 mg/ml and diluted to acceptable working solutions to reach various final concentrations.
The standard A. fumigatus strain (CGMCC 3.14869) was purchased from China General Microbiological Culture Collection Center. Mycelium was obtained following the required number of days (mentioned in the required methods of different experiments) of conidia cultivation on the Sabouraud’s agar medium at 28°C. Conidia on the surface of the medium were collected, counted by a hemocytometer, and adjusted to a final concentration of 1 × 107 /ml.
MIC was determined using the standard microdilution method developed by the American Clinical and Laboratory Standards Institute (CLSI) (Wootton et al., 2020). Conidia suspension (2 × 105 CFU/ml) (CFU means colony-forming unit) was cultured with hinokitiol in various concentrations (2, 4, 8, 16, and 32 μg/ml) and 0.1% DMSO at 28°C in 96-well plates (100 μl per well). A microplate reader was used to read the optical density (OD) at 620 nm at 12 h, 24 h, 48 h, 72 h, and 96 h.
Conidia suspension (2 × 105 CFU/ml) (CFU means colony-forming unit) was cultured with hinokitiol in various concentrations (2, 4, 8, 16, 32,64, and 128 μg/ml) and 0.1% DMSO at 28°C in 96-well plates (100 μl per well). 5 μl of medium from each well was removed and incubated on Sabouraud‘s agar medium for 48 h. When the quantity of CFU (colony forming units) is less than 10, 99% of A. fumigatus conidia are killed, and the medication concentration is the minimal fungicidal concentration.
Conidia suspension (2 × 105 CFU/ml) was cultured for 24 h at 28°C in 96-well plates (100 μl per well) to generate mycelium. 2, 4, 8, 16, and 32 μg/ml of hinokitiol were added with 0.1% DMSO to each well before being incubated for 24 h. A microplate reader was used to read the OD at 620 nm.
HCECs (provided by University of Xiamen, Fujian, China) were incubated according to the previous method by Yin et al. (2022). Cell suspension (3 × 105/mL) to be tested was incubated in a 96-well plate with different concentrations of hinokitiol for 24 h under the original condition in dark. The absorbance values were measured at 450 nm 3 h after the addition of 10 μl of cell counting kit-8 (CCK-8) each well. 6 replicates were set up for each sample.
We seeded the A. fumigatus conidia (2 × 105 CFU/ml) into 6-well plates and cultured there for 24 h at 28°C to generate mycelium. After being washed the mycelium was centrifuged at 12,000 rpm for 10 min before being transferred to a fresh 6-well plate. The mycelium was rinsed with phosphate buffer (PBS), then collected and fixed with 2.5% glutaraldehyde at 4°C overnight after being incubated with 0.1% DMSO or hinokitiol (8 μg/ml) at 28°C for 24 h. The following procedures were followed as per standard procedures to prepare the mycelium sample for TEM observation (Shen et al., 2020). We examined the samples using a JEOL-1200EX transmission electron microscope.
We seeded the A. fumigatus conidia (2 × 105 CFU/ml) into 6-well plates and cultured there for 24 h at 28°C to generate mycelium. The mycelium was washed, centrifuged at 12,000 rpm for 10 min, and then transferred to a new 6-well plate. The mycelium was rinsed with PBS after being incubated with 0.1% DMSO or hinokitiol (8 μg/ml) at 28°C for 24 h. They were then collected and fixed with 2.5% glutaraldehyde at 4°C for 2 h. PBS was used to clean the samples, and they were then mixed for an hour at 4°C with 1% (v/v) osmium tetroxide in PBS. Samples were then gently dehydrated in graded ethanol, critical point dried in CO2, coated with gold, and seen under an SEM (VEGA3; TESCAN Company, Shanghai, China) at magnifications of 2000 and 5,000 (bar =20 or 10 μm).
Aspergillus fumigatus mycelium was prepared and collected as previously indicated and was treated with hinokitiol (4 μg/ml, 8 μg/ml) or without hinokitiol (0.1% DMSO) for 24 h, 36 h, or 48 h. Each group received a 15-min addition of 50 μg/ml propidium iodide (PI) solution before being incubated in the dark at room temperature. Bright-field and fluorescence images were both taken using a fluorescence microscope (Nikon, Tokyo, Japan, 100×) with green excitation light or not. Image J software was used to calculate the fluorescence intensity.
Aspergillus fumigatus mycelium was prepared in sabouraud medium for 5 days and was treated with hinokitiol (4 μg/ml, 8 μg/ml) or without hinokitiol (0.1% DMSO) for 48 h. Mycelium was washed in sterile distilled water, filtered, and fully dried. Total ergosterol contents of A. fumigatus mycelium with different hinokitiol treatments were determined by the method described previously (OuYang et al., 2019) with a slight modification. 48 h were spent vacuum-freezing the samples before 0.1 g of each group was saponified in 4 ml of freshly made 30% (w/v) methanolic KOH and 8 ml of 100% ethanol at 90°C. 3 ml of petroleum ether was used three times to extract the mixtures containing 10 ml of saturated sodium chloride solution. After placing the samples in the extraction flask, the supernatants were collected. At 60 to 90°C, the petroleum ether solution was then distilled. With the help of 10 ml anhydrous ethanol, the solute crystallized and was retrieved. High-Performance Liquid Chromatography (HPLC) analysis was carried out using a HITACHI high-performance liquid chromatograph (Chromaster, HITA-CHI, Japan). At a constant temperature, the sample extracts were separated and examined on a C18 column (250 mm × 4.6 mm, 5 μm). The mobile phase was made up of Solvent A (methanol). A flow of 1 ml per minute was present. Chromatographic peaks were discovered by measuring retention times and spectra against the specified standard. The detection wavelength was set at 282 nm. For the analysis, 20 μl aliquots were directly injected into the HPLC. Ergosterol standard curve is provided in Supplementary Table S1.
Aspergillus fumigatus mycelium was prepared and collected as previously indicated and the experimental groups and the control groups were classified using the same approach as in the cell membrane permeability assay. We utilized calcofluor white (Sigma, St. Louis, MO, United States) and fluorescence microscopy to observe. The collected mycelia were stained with 10 μl KOH (10%) followed by 10 μl of calcofluor white stain as the manufacturer’s instructions.
The experimental groups and the control groups were classified using the same approach as in the cell membrane permeability assay. We assayed chitin content according to the previous method described with slight modifications (Ke et al., 2022). The mycelium samples were cultured in a 96-well plate. They were twice rinsed with distilled water before being incubated for 5 min in the dark with Congo red (0.025%). The materials were examined using a microplate reader (Tecan, Switzerland) with excitation and emission wavelengths of 540 and 600–850 nm, respectively.
Aspergillus fumigatus mycelium was prepared in sabouraud medium for 5 days and was treated with hinokitiol (4 μg/ml, 8 μg/ml) or without hinokitiol (0.1% DMSO) for 24 and 48 h. Mycelium was washed in sterile distilled water, filtered, and fully dried. We ground the mycelium into a paste with the chitinase extraction reagent in an ice bath and then centrifuged them at 8000 rpm at 4°C for 25 min. The supernatant was collected to get the extracted enzyme solution, and a commercial BC0820 kit was used to measure chitinase activity (Solarbio Technology, Beijing, China).
Conidia suspension (2 × 105/mL) containing 0.1% DMSO or hinokitiol (4 μg/ml) was incubated on a 6-well plate at 28°C. The Control (DMSO treated) and Test (HK treated) samples were harvested after incubation for 36 h and then stored at −80°C for RNA-Seq analysis. All the experiments were carried out in 3 independent biological replicates. Total RNA was extracted using the TRIzol reagent according to the manufacturer’s protocol. RNA purity and quantification were evaluated using the NanoDrop 2000 spectrophotometer (Thermo Scientific, United States). RNA integrity was assessed using the Agilent 2,100 Bioanalyzer (Agilent Technologies, Santa Clara, CA, United States). Then the libraries were constructed using TruSeq Stranded mRNA LT Sample Prep Kit (Illumina, San Diego, CA, USA) according to the as manufacturer’s instructions. The transcriptome sequencing and analysis were conducted by OE Biotech Co., Ltd. (Shanghai, China). The Sequence Read Archive has been released in NCBI1 and the 6 runs can be searched by accession numbers SRR22582946, SRR22582945, SRR22582944, SRR22582943, SRR22582942, and SRR22582941.
Hub genes were screened from the differently expressed genes (DEGs) in the RNA-seq result. We utilized the STRING database (version 11.52) to analyze the protein–protein interactions (PPI) of Hub DEGs. Cytoscape software (version 3.8.0) was used to visualize the PPI network. The Molecular Complex Detection (MCODE) plugin of Cytoscape was then used to determine the important gene modules in the PPI networks. Finally, the cytoHubba plugin was used to rank the genes and highlight Hub genes. Overall, the features we got from different plugins were represented in the PPI network.
Conidia suspension (2 × 105/mL) containing 0.1% DMSO or hinokitiol (4 μg/ml) was incubated on a 6-well plate at 28°C. The Control (DMSO treated) and Test (HK treated) samples were harvested after incubation for 36 h. The total RNA was prepared using the Fungal Total RNA198 Isolation Kit (B518629, Sangon Biotech, Shanghai, China), and reverse transcribed with HiScript III RT SuperMix (Vazyme, Nanjing, China) according to the manufacturer’s instructions. The PCR method was based on previous studies (Sun et al., 2019). Primers used for the qRT-PCR are listed in Supplementary Table S2.
For comparisons with data from the control group or HK treatment group, we performed an unpaired, two-tailed Student’s t-test or one-way ANOVA with post hoc analysis. GraphPadPrism 8.0 (San Diego, California, United States) and ImageJ1.44p (National Institutes of Mental Health, USA) were used for statistical analyzes, and values were presented as the mean ± standard deviation (SD). p < 0.05 (*p < 0.05, **p < 0.01, ***p < 0.001) was considered statistically significant (ns = no significance). All the experiments were carried out in 3 independent biological replicates.
According to MIC data, the germination of A. fumigatus conidia and growth of A. fumigatus mycelium were inhibited by hinokitiol at 2 μg/ml, with the extent varying proportionally to concentrations from 2 to 32 μg/ml (Figures 1A,B). The 0.1% DMSO treatment group showed no meaningful effect on the growth of A. fumigatus compared to the control group. The time-kill curve in Figure 1C showed that as the hinokitiol dose increased, the absorbance values at the same time point gradually decreased, with a higher difference at 48 h between 4 μg/ml and 8 μg/ml treated groups. MFC assay results indicated that A. fumigatus conidia were 100% killed by hinokitiol at 16 μg/ml (Figure 1D).
Figure 1. Antifungal activity of hinokitiol against A. fumigatus. (A) A. fumigatus conidia MIC. (B) A. fumigatus mycelium MIC. N means the normal group and D means 0.1% DMSO group. (C) Time-kill curve. (D) MFC assay. (E) HCECs viability assay. (F) F1-3 are SEM and F4–6 are TEM. F1,4 are untreated control groups, F2-3 and F5-6 are treated with 8 μg/ml hinokitiol (**** p < 0.0001).
Fungal keratitis is a serious blinding eye disease caused by a fungal infection, which has an acute onset, rapid progression, and poor prognosis. The common causative fungi are Aspergillus spp., Candida spp. and Fusarium spp. (Brown et al., 2021; Sharma et al., 2022).The effect of hinokitiol on the proliferative capacity of HCECs was investigated, and the results are shown in Figure 1E. The proliferative capacity of HCECs experienced a significant rise at 2 and 4 μg/ml of hinokitiol, and then saw a gradual drop thereafter. With the exposure of 8 and 10 μg/ml of hinokitiol, no differences in cell proliferation degree were seen compared to the control group. It was not until 12 μg/ml of hinokitiol that HCECs were inhibited to grow. Therefore, hibokitiol with concentrations being below 10 μg/ml is considered to be high-safe and low-toxic.
We used TEM and SEM to examine the micromorphological alterations in hinokitiol-treated A. fumigatus, focusing on the cell wall and cell membrane. SEM revealed that hinokitiol-treated mycelium grew in a chaotic and uneven manner (Figures 1F2,F3) compared to the control (Figure 1F1). They atrophied and deformed, collapsed or flattened and hollowed. The mycelium in Figure 1F2 had twisted and virtually shattered shapes. Figure 1F3 also showed branching and odd shape. TEM results of mycelium ultrastructure displayed that the cell membrane was coiled, discontinuous, and inconspicuous under the action of hinokitiol. The cell membrane separated from the cell wall became ruptured (Figures 1F5,F6). The hinokitiol-treated group likewise exhibited a fuzzy structure of the cell wall that dissolved into fragments and was noticeably shallower than in the control group (Figures 1F5,F6). The findings suggested that hinokitiol interfered with cell wall integrity and cell membrane continuity in some way.
We used a propidium iodide dye that is only taken up by cells with impaired cell integrity to evaluate cell membrane permeability. Hinokitiol (4 g/ml, 8 g/ml) was applied to the mycelium for 24 h, 36 h, and 48 h. In bright fields, A. fumigatus mycelium width and density decreased with medication concentrations and length of time, while the fluorescence intensity in fluorescence images rose gradually (Figures 2A–C). Besides that, the percentage of mycelium stained by PI solution also increased in the same manner. The fluorescence was extensively distributed in the field of vision in the 8 g/ml, 48-h treatment group, and the brilliant spots representing exudation were extremely dense. We utilized the software ImageJ to quantitatively clarified the fluorescence intensity in each PI staining field which showed a clear rising trend over time and across concentration (Figure 2D). Overall, hinokitiol was shown to have a detrimental impact on the pattern of mycelium growth, cell integrity; and increased cell membrane permeability depending on the duration of action and drug concentration proporationally.
Figure 2. The cell membrane was influenced by hinokitiol. (A–C) PI staining. Bright-field and fluorescence images were taken with a fluorescence microscope. The control group was treated with 0.1% DMSO. (D) ImageJ analysis. (E) HPLC. The extracted ingredient retrieved by ethanol was examined by a high-performance liquid chromatograph. The numbers at around 10 min mean the levels of ergosterol content. The control group was treated with 0.1% DMSO (*** p < 0.001, **** p < 0.0001).
Quantitative measurement of the main component of A. fumigatus cell membrane, ergosterol, was assayed and the ratio of dry weight (DW) of mycelium was calculated. We demonstrated that there was a substantial difference in ergosterol levels between each group with or without hinokitiol treatment. After being exposed to hinokitiol with 4 or 8 μg/ml for 48 h, the HPLC result showed that ergosterol content decreased compared with the control group (Figure 2E). The ratios of DW in the control, 4 μg/ml, 8 μg/ml group were 5.160734 mg/g, 0.664238 mg/g, and 0.317686 mg/g, respectively. Therefore, we found that hinokitiol may compromise cell membrane integrity by losing ergosterol content in cell membranes.
We tested calcofluor white staining to explore how hinokitol damage A. fumigatus cell wall. Control mycelium witnessed a strong fluorescence intensity in its chitin-rich cell wall and septa (Figures 3A1–A3). After being treated with hinokitiol, the overall fluorescence intensity of the cell wall and septum fell in a hinokitiol concentration and time-dependent manner (Figures 3A4–A9). In addition, we found that in the 8 μg/ml treatment group especially, the fluorescence profile became blurred and the background fluorescence was greatly increased as indicated by arrows (Figures 3A7–A9). It suggested that the cell wall was disrupted by hinokitiol, where the chitin content leaked out and dispersed. Besides that, the fungus cultured with hinokitiol became narrower in diameter, more branched, and shorter in mycelium length.
Figure 3. Hinokitiol targeted the cell wall. (A) Calcofluor white staining. Fluorescence images were taken with a fluorescence microscope. (B,C) Congo red staining. Microplate reader with excitation and emission wavelengths of 540 and 600–850 nm, respectively. (D) Chitinase activity. (n = 3/group; ns P > 0.05, **** p < 0.0001).
Then the chitin content of A. fumigatus in the control and treatment groups was further measured (Figures 3B,C). Both graphs displayed that hinokitiol reduced the chitin content compared to the group without hinokitiol. In the comparison between the different exposure period groups, after being treated with hinokitiol, the increase degrees in chitin content levels were smaller in the 4 and 8 μg/ml hinokitiol-treated groups than that in the control group, for the difference in chitin content levels between the control and treated groups increased in the same time point. The results indicated that hinokitiol disrupted the cell wall components, and chitin, and inhibited the synthesis of cell wall components.
Subsequently, we examined the activity of chitinase in A. fumigatus after treatment with different concentrations of hinokitiol for 24 and 48 h (Figure 3D). Overall, chitinase activity proved to increase in hinokitiol-treated groups in both 24 h and 48 h group (p < 0.0001), despite seeing a drop in chitinase activity at 8 μg/ml (p<0.0001). With the same concentration of hinokitol, there was no statistically significant difference between the chitinase activities of the two groups at two treatment periods (p>0.05). The figure suggested that hinokitiol influenced the biological activity of chitinase, which might explain why the chitin content of the A. fumigatus cell wall decreased after hinokitiol exposure. And the higher concentration of hinokitiol could be able to damage the whole bioactivity, responsible for the fall of chitinase activity at 8 μg/ml.
To explore the effects at the gene level, we used RNA-seq analysis to investigate the transcriptional characteristics of A. fumigatus treated with (4 μg/ml, 36 h) or without hinokitiol treatment. RNA-seq results and derived DEGs related to the cell wall or cell membrane remodeling were subsequently analyzed and divided into two heat maps (Figures 4A,B). There are 67 genes in Figure 4A and 197 genes in Figure 4B. The heat maps provide a global view of specific DEGs, with red representing up-regulation trends and blue representing down-regulation trends. Figure 4A displays genes associated with the fungal cell wall, most of which are involved in the synthesis and degradation of mannans, glucan, and chitin, in addition to some genes related to the activities of the fungal cell wall such as germination, septogenesis, and extracellular sensing. Figure 4B shows genes related to fungal cell membranes, which are involved in the transmembrane transport of various substances in fungi, the formation of cell membranes, regulation of ion channel activity, and mediating the membrane structure with the endoplasmic reticulum, Golgi apparatus, and other organelles to form an integral membrane structure.
Figure 4. RNA-seq analysis and qRT-RNA validation. (A,B) Heat maps. Related to the cell wall and cell membrane, respectively. (C) PPI network. Gene screening and STRING database analysis were performed on Hub genes in advance. (D) qRT-PCR validation. The up direction of bars represents increased expressions of genes and the down direction of bars means decreased expressions of genes.
Nineteen hub genes associated with the cell wall (Figure 4C) and 10 hub genes related to the cell membrane (Supplementary Figure S3) were obtained and were considered to play an important function in A. fumigatus cultured with hinokitiol (Figure 4C). EglC, pgxB, plyA, gel3, gel1, chiA1, crf1, and ftrA from the PPI network performed qRT-PCR experiments. Among them, eglC, which encodes an endoglucanase, is thought to play the most indispensable role. PCR results validated the reliability of RNA-seq, as the expression trends of these genes were the same with those of heat maps, albeit to a different extent (Figure 4D).
Hinokitiol, also named β-thujaplicin, was shown to be biologically active against harmful insects and wood decay fungi because of its protection of wooden structures in Japan for almost 800 years (INAMORI et al., 2000). It is reported to be extracted from the acid oil produced by distillation of the building material Chamaecyparis obtuse and has the same basic structural elements as tropolone, which is known for its ability to repel termites. Research interests have been greatly stimulated by the antifungal properties of hinokitiol. As a powerful antifungal agent and potential defense against Candida albicans, hinokitiol has been demonstrated by previous studies. In our study, we verified the presence of an impaired state by measuring the absorbance of conidia and mycelium growth of A. fumigatus as well as micromorphological findings by electron microscopy. In addition, HCECs proliferative capacity was not significantly inhibited by hinokitiol (under 12 μg/ml), indicating hinokitiol as a potential agent with potential for application for humans. Overall, hinokitiol is likely to be a promising drug for the treatment of fungal infectious diseases.
Subsequently, we found that the cell membrane was significantly disrupted by the disturbance of hinokitiol by various experimental methods; with the increase of treatment dose and time, cell membrane permeability increased and the amount of ergosterol in the cell membrane decreased. The integrity of the cell membrane was critical in preserving fungal vitality (OuYang et al., 2019). As a promising drug target, cell membranes have been studied to achieve effective medicinal development (Sant et al., 2016). Their disruption could produce alterations in hyphae due to increased cell permeabilization, which usually means leakage of small molecular compounds and ions, lesions, and inconsistencies in cell metabolism (Shao et al., 2013; Guo et al., 2022). For example, AgNPs can disrupt cell membrane permeability and disrupt lipid bilayers causing ion leakage (Abdallah et al., 2023). The main components of the cell membrane of fungi are various lipids divided into three classes which are glycerophospholipids, sphingolipids, and sterols. The reduction of lipid composition results in a loss of cell membrane structural stability increased permeability to water-soluble materials, and morphological change (Tao et al., 2014; OuYang et al., 2016). Among them, ergosterol is the most abundant sterol component of them and is specific to fungi (Sant et al., 2016). Previous studies suggested that the impairment of ergosterol biosynthesis was the mechanism of damaging cell membranes of fungi of a few valid antifungal drugs (Tian et al., 2012; Guo et al., 2022). Previous study showed that nystatin can bind ergosterol to form broken channels in the cell membrane and alter permeability by causing the outflow of sodium, potassium and hydrogen ions (Thompson et al., 2009). Imidazole and triazoles were considered to be the most successful antifungal drugs targeting ergosterol synthesis (Sant et al., 2016). Aside from providing a protective barrier, cell membrane also plays critical roles in promoting virulence because of the mediation of the secretion of virulence factors, endocytosis, cell wall synthesis, and invasive mycelium morphogenesis (Douglas and Konopka, 2016). Therefore, based on the above results, hinokitiol proved to be detrimental to the cell membrane, and the decrease in ergosterol content may lead to diminished virulence of A. fumigatus.
Because of its roles in shielding itself from external distraction, engaging with the host immune system through particular virulence components, and being a potential target for antifungal drugs, the cell wall is thought to be a crucial organelle of A. fumigatus, which was also considered to be one of the virulence factors (Bowman and Free, 2006; Latgé, 2007; Dichtl et al., 2012; Latgé and Chamilos, 2019). It is composed of a branched β-1,3-glucan as a core skeleton, to which chitin, β-1,4-glucan, and galactomannan are linked, surrounded by other polysaccharides as a cement, such as α-1,3-glucan and mannans (van de Veerdonk et al., 2017; Latgé and Chamilos, 2019). Chitin is an integral part of the fungal cell wall and, together with β-1,3-glucan, plays a key role in maintaining cellular integrity and can confer structural rigidity during growth and morphogenesis (Corrêa et al., 2020). Chitin accounts for 10–20% of the dry weight of the cell wall of A. fumigatus and is of considerable inportance. When A. fumigatus is placed in a difficult environment, a cell wall’s dynamic structure can be altered in a variety of ways to withstand its harmful effects (van de Veerdonk et al., 2017). Under normal conditions, the composition and degradation of chitin occur in a dynamic equilibrium, which, once disrupted, is likely to lead to cell death (Ke et al., 2022). For example, in previous studies, drugs such as cinnamaldehyde, propolis extract and chitosan oligosaccharides were able to target chitin as the main target for fungal destructive effects (OuYang et al., 2019; Corrêa et al., 2020; Ke et al., 2022). In our study, we found that hinokitiol was able to cause roughness and unevenness of the cell wall, shortening, fracture and blurring of the mycelium, and reduction, leakage, and dispersion of chitin in the cell wall and septum. The loss of chitin content, interfering biosynthesis, and triggering degradation leading to deformity and broken of A. fumigatus were observed in our reports. In addition, chitinases are present in all fungal species and play an important role in building and maintaining the cell wall structure and function of fungi. The breakdown of chitin by chitinase leads to the disruption of cell wall integrity and thus participates in the morphogenesis of the fungus (Roncero and Vázquez de Aldana, 2020). The anti-cell wall activities of hinokitiol may spring from the ability to disrupt the balance of chitin synthesis and decomposition, which results in morphological abnormalities and even fragmentation and fracture of fungal cells, leading to loss of cell wall integrity and osmotic instability.
To further study the antifungal mechanism of hinokitiol on a molecular level, we analyzed RNA-seq data of A. fumigatus treated by hinokitiol or not by gene screening, STRING database analysis, PPI protein network analysis, Hub gene selection, and gene function determination. Besides that, we verified the reliability of RNA-seq results by PCR. Hub genes we got explained the possible influence manner by which hinokitiol did to A. fumigatus. EglC, bgt1, pgxB, plyA, gel3, gel1, chiA1, crf1, and ftrA were performed by qRT-PCR and the reliability of RNA-seq was validated. EglC, encoding an endoglucanase, has been recently suggested its greatest action on polysaccharide (Hasper et al., 2002). The endoglucanase EglC is able to inhibit the growth of A. fumigatus by degrading cell walls (Champer et al., 2016) and also engages in the delivery of proteins anchored to the cell membrane in Aspergillus nidulans. EglC is also involved in the delivery of proteins anchored to the Aspergillus nidulans cell membrane. Previous studies have shown that eglC in Aspergillus nidulans is expressed as a GPI-anchored protein, which can be delivered to the cell membrane surface in a polarized manner and is involved in the composition of lipid and ergosterol of cell membrane (Peñalva et al., 2020). In our research, eglc was considered as the most indispensable gene related to both cell walls and cell membranes and its upregulated expression in the exposure of hinokitiol may be important for its ability to cause increased xyloglucan hydrolysis (Hasper et al., 2002), disruption of cell wall structure and homeostasis imbalance; it is possible that the upregulated transcript level of eglC is due to the negative feedback of reduced GPI after cell membrane disruption. Gel1 and gel3, belonging to the β-1,3-glucanosyltransferase gels family(gel1-7), were essential for both morphogenesis and virulence in A. fumigatus for the elongating and branching activities of β-1,3-glucan chains (Mouyna et al., 2005; Zhao et al., 2013, 2014; Aimanianda et al., 2017). Crf1 from the transglycosylases crfs (crf1-2) family has been reported in articles, which is responsible for cross-linking of chitin and glucan (Arroyo et al., 2007). Crf1 and gel1 were considered to be the candidates for a promising vaccine as their highly expressed conserved character in A. fumigatus (Arroyo et al., 2007). Gas and crh family from Saccharomyces cerevisiae were mentioned in previous research. Poacic acid is a cell wall-targeted antifungal agent exhibiting its function of inhibiting the activity of gas and crh (Piotrowski et al., 2015; García et al., 2021), which are orthologous protein families of gel and crf from A. fumigatus, respectively (Arroyo et al., 2007). The activity of these enzymes plays an indispensable role in constructing the outer skeleton of fungi under pressures outside and are expected to be antifungal drug targets. Our research elaborated that the significantly different expression pattern of eglC, gel1, gel3, and crf1 compared with the control group and then their critical positions of them in the PPI network has also been highlighted.
Studies have shown that the disruption of the yeast cell wall can initiate the CWI (cell wall integrity) pathway and induce changes in gene transcription profiles (García et al., 2021). Hinokitiol, by interfering with the expression of these enzyme genes, may prevent the elongation and branching of β-1,3-glucan, inhibit the maturation process of glycosylation, and disturb the cross-linkng of glucan and chitin. The expression level of chiA1(one of the chitinases family) increased in RNA-seq which probably means chitin hydrolysis was enhanced by hinokitiol in the cell wall of A. fumigatus, and probably explained the reason for the elevated chitinase activity in our previous experiment (Yang and Zhang, 2019). FtrA encodes high-affinity iron permease, with the ability to modulate iron ion transmembrane transport (Li et al., 2023). Previous studies showed that hinokitiol could disrupt iron homeostasis, leading to respiratory chain dysfunction of Candida strains (Jin et al., 2021). Here we found that the interfering process might involve the engagement of cell membranes and Fe transporter permease ftrA. Therefore, we demonstrate that the influence of these genes in the involvement of cell wall and cell membrane structure and function is not negligible, which further illustrates the ability of hinokitiol to damage fungi.
Overall, RNA-seq results revealed their expression pattern differences and demonstrated that they may play an important role in participating in cell wall remodeling by affecting the expression of several hydrolytic enzyme. The synthesis, prolongation, maturation, and cross-linking of glycan components were disturbed, thus affecting the formation of the cell wall; in addition, the catabolism of cell wall components may be promoted by up-regulation of hydrolase gene transcription, and the content of polysaccharides and chitin decreased, ultimately leading to the rupture of the cell wall structure. While altered expression of cell membrane-associated genes eglC and ftrA was also identified to be responsible for the function of hinokitiol; altered expression of fungal extracellular structure-anchoring proteins and transport permeases may have affected cell membrane permeability and stability.
In conclusion, our present studies revealed that hinokitiol altered the morphology of A. fumigatus. Hinokitiol interfered with the ergosterol biosynthesis process to disrupt the normal structures of membranes, resulting in component leakage of cells and reducing ergosterol content in cell membranes. Hinokitiol also promoted the hydrolysis process of polysaccharide components, inhibited chitin biosynthesis in cell walls, and caused the malformation of fungi by influencing chitinase activity and the expressions of Hub genes. All the negative alteration of cell integrity eventually led to a bioactivity and virulence reduction of A. fumigatus much likely. These results suggested that hinokitiol acted its antifungal function against A. fumigatus by targeting the cell membrane and cell wall.
The datasets presented in this study can be found in online repositories. The names of the repository/repositories and accession number(s) can be found in the article/Supplementary material.
FM designed, and implemented most of the research and wrote the first version of the manuscript. XL conducted vital parts of the experiments and reviewed the manuscript. CL reviewed and edited the manuscript. XP, QW, and QX collected and analyzed the data. GZ and JL supervised the study. All authors read and approved the manuscript.
This work was supported by Taishan Scholar Project of Shandong Province [grant numbers tsqn201812151].
The authors declare that the research was conducted in the absence of any commercial or financial relationships that could be construed as a potential conflict of interest.
All claims expressed in this article are solely those of the authors and do not necessarily represent those of their affiliated organizations, or those of the publisher, the editors and the reviewers. Any product that may be evaluated in this article, or claim that may be made by its manufacturer, is not guaranteed or endorsed by the publisher.
The Supplementary material for this article can be found online at: https://www.frontiersin.org/articles/10.3389/fmicb.2023.1132042/full#supplementary-material
Abdallah, B. M., Rajendran, P., and Ali, E. M. (2023). Potential treatment of dermatophyte trichophyton rubrum in rat model using topical green biosynthesized silver nanoparticles with Achillea santolina Extract. Molecules 28:1536. doi: 10.3390/molecules28041536
Aimanianda, V., Simenel, C., Garnaud, C., Clavaud, C., Tada, R., Barbin, L., et al. (2017). The dual activity responsible for the elongation and branching of β-(1,3)-glucan in the fungal cell wall. MBio 8:e00619. doi: 10.1128/mBio.00619-17
Anderson, A. B., and Gripenberg, J. (1948). Antibiotic substances from the heart wood of Thuja plicata D. Don; the constitution of beta-thujaplicin. Acta Chem. Scand. 2, 644–650. doi: 10.3891/acta.chem.scand.02-0644
Arroyo, J., Sarfati, J., Baixench, M. T., Ragni, E., Guillén, M., Rodriguez-Peña, J. M., et al. (2007). The GPI-anchored Gas and Crh families are fungal antigens. Yeast 24, 289–296. doi: 10.1002/yea.1480
Bowman, S. M., and Free, S. J. (2006). The structure and synthesis of the fungal cell wall. BioEssays 28, 799–808. doi: 10.1002/bies.20441
Brown, L., Leck, A. K., Gichangi, M., Burton, M. J., and Denning, D. W. (2021). The global incidence and diagnosis of fungal keratitis. Lancet Infect. Dis. 21, e49–e57. doi: 10.1016/S1473-3099(20)30448-5
Champer, J., Ito, J. I., Clemons, K. V., Stevens, D. A., and Kalkum, M. (2016). Proteomic analysis of pathogenic fungi reveals highly expressed conserved cell wall proteins. J. Fungi (Basel) 2:6. doi: 10.3390/jof2010006
Corrêa, J. L., Veiga, F. F., Jarros, I. C., Costa, M. I., Castilho, P. F., de Oliveira, K. M. P., et al. (2020). Propolis extract has bioactivity on the wall and cell membrane of Candida albicans. J. Ethnopharmacol. 256:112791. doi: 10.1016/j.jep.2020.112791
Dichtl, K., Helmschrott, C., Dirr, F., and Wagener, J. (2012). Deciphering cell wall integrity signalling in Aspergillus fumigatus: identification and functional characterization of cell wall stress sensors and relevant Rho GTPases. Mol. Microbiol. 83, 506–519. doi: 10.1111/j.1365-2958.2011.07946.x
Douglas, L. M., and Konopka, J. B. (2016). Plasma membrane organization promotes virulence of the human fungal pathogen Candida albicans. J. Microbiol. 54, 178–191. doi: 10.1007/s12275-016-5621-y
Fan, Y., Li, C., Peng, X., Jiang, N., Hu, L., Gu, L., et al. (2020). Perillaldehyde ameliorates Aspergillus fumigatus keratitis by activating the Nrf2/HO-1 signaling pathway and inhibiting dectin-1-mediated inflammation. Invest. Ophthalmol. Vis. Sci. 61:51. doi: 10.1167/iovs.61.6.51
Fisher, M. C., Alastruey-Izquierdo, A., Berman, J., Bicanic, T., Bignell, E. M., Bowyer, P., et al. (2022). Tackling the emerging threat of antifungal resistance to human health. Nat. Rev. Microbiol. 20, 557–571. doi: 10.1038/s41579-022-00720-1
García, R., Itto-Nakama, K., Rodríguez-Peña, J. M., Chen, X., Sanz, A. B., de Lorenzo, A., et al. (2021). Poacic acid, a β-1,3-glucan-binding antifungal agent, inhibits cell-wall remodeling and activates transcriptional responses regulated by the cell-wall integrity and high-osmolarity glycerol pathways in yeast. FASEB J. 35:e21778. doi: 10.1096/fj.202100278R
Guo, L., Li, Y., Mao, X., Tao, R., Tao, B., and Zhou, Z. (2022). Antifungal activity of polymethoxylated flavonoids (PMFs)-loaded citral nanoemulsion against Penicillium italicum by causing cell membrane damage. J. Fungi (Basel) 8:388. doi: 10.3390/jof8040388
Hasper, A. A., Dekkers, E., van Mil, M., van de Vondervoort, P. J., and de Graaff, L. H. (2002). EglC, a new endoglucanase from Aspergillus niger with major activity towards xyloglucan. Appl. Environ. Microbiol. 68, 1556–1560. doi: 10.1128/AEM.68.4.1556-1560.2002
Inamori, Y., Morita, Y., Sakagami, Y., Okabe, T., and Ishida, N. (2006). The excellence of Aomori Hiba (Hinokiasunaro) in its use as building materials of Buddhist temples and Shinto shrines. Biocontrol Sci. 11, 49–54. doi: 10.4265/bio.11.49
Inamori, Y., Sakagami, Y., Morita, Y., Shibata, M., Sugiura, M., Kumeda, Y., et al. (2000). Antifungal activity of Hinokitiol-related compounds on wood-rotting fungi and their insecticidal activities. Biol. Pharm. Bull. 23, 995–997. doi: 10.1248/bpb.23.995
Jin, X., Zhang, M., Lu, J., Duan, X., Chen, J., Liu, Y., et al. (2021). Hinokitiol chelates intracellular iron to retard fungal growth by disturbing mitochondrial respiration. J. Adv. Res. 34, 65–77. doi: 10.1016/j.jare.2021.06.016
Ke, Y., Ding, B., Zhang, M., Dong, T., Fu, Y., Lv, Q., et al. (2022). Study on inhibitory activity and mechanism of chitosan oligosaccharides on Aspergillus flavus and Aspergillus fumigatus. Carbohydr. Polym. 275:118673. doi: 10.1016/j.carbpol.2021.118673
Kim, D. J., Lee, M. W., Choi, J. S., Lee, S. G., Park, J. Y., and Kim, S. W. (2017). Inhibitory activity of hinokitiol against biofilm formation in fluconazole-resistant Candida species. PLoS One 12:e0171244. doi: 10.1371/journal.pone.0171244
Komaki, N., Watanabe, T., Ogasawara, A., Sato, N., Mikami, T., and Matsumoto, T. (2008). Antifungal mechanism of hinokitiol against Candida albicans. Biol. Pharm. Bull. 31, 735–737. doi: 10.1248/bpb.31.735
Latgé, J. P. (2007). The cell wall: a carbohydrate armour for the fungal cell. Mol. Microbiol. 66, 279–290. doi: 10.1111/j.1365-2958.2007.05872.x
Latgé, J. P., and Chamilos, G. (2019). Aspergillus fumigatus and aspergillosis in 2019. Clin. Microbiol. Rev. 33:e00140. doi: 10.1128/CMR.00140-18
Li, H., Dai, J., Shi, Y., Zhu, X., Jia, L., and Yang, Z. (2023). Molecular regulatory mechanism of the iron-ion-promoted asexual sporulation of Antrodia cinnamomea in submerged fermentation revealed by comparative transcriptomics. J. Fungi (Basel) 9:235. doi: 10.3390/jof9020235
Mouyna, I., Morelle, W., Vai, M., Monod, M., Léchenne, B., Fontaine, T., et al. (2005). Deletion of GEL2 encoding for a beta(1-3)glucanosyltransferase affects morphogenesis and virulence in Aspergillus fumigatus. Mol. Microbiol. 56, 1675–1688. doi: 10.1111/j.1365-2958.2005.04654.x
OuYang, Q., Duan, X., Li, L., and Tao, N. (2019). Cinnamaldehyde exerts its antifungal activity by disrupting the cell wall integrity of Geotrichum citri-aurantii. Front. Microbiol. 10:55. doi: 10.3389/fmicb.2019.00055
OuYang, Q., Tao, N., and Jing, G. (2016). Transcriptional profiling analysis of Penicillium digitatum, the causal agent of citrus green mold, unravels an inhibited ergosterol biosynthesis pathway in response to citral. BMC Genomics 17:599. doi: 10.1186/s12864-016-2943-4
Pasqualotto, A. C., and Denning, D. W. (2008). New and emerging treatments for fungal infections. J. Antimicrob. Chemother. 61, i19–i30. doi: 10.1093/jac/dkm428
Peñalva, M. A., Moscoso-Romero, E., and Hernández-González, M. (2020). Tracking exocytosis of a GPI-anchored protein in Aspergillus nidulans. Traffic 21, 675–688. doi: 10.1111/tra.12761
Piotrowski, J. S., Okada, H., Lu, F., Li, S. C., Hinchman, L., Ranjan, A., et al. (2015). Plant-derived antifungal agent poacic acid targets β-1,3-glucan. Proc. Natl. Acad. Sci. U. S. A. 112, E1490–E1497. doi: 10.1073/pnas.1410400112
Qiao, Y., Zhang, M., Cao, Y., Mi, Q., Liang, S., Feng, J., et al. (2022). Postharvest sclerotinia rot control in carrot by the natural product hinokitiol and the potential mechanisms involved. Int. J. Food Microbiol. 383:109939. doi: 10.1016/j.ijfoodmicro.2022.109939
Rebia, R. A., Binti Sadon, N. S., and Tanaka, T. (2019). Natural antibacterial reagents (Centella, Propolis, and hinokitiol) Loaded into Poly[(R)-3-hydroxybutyrate-co-(R)-3-hydroxyhexanoate] composite nanofibers for biomedical applications. Nanomaterials (Basel) 9:1665. doi: 10.3390/nano9121665
Roncero, C., and Vázquez de Aldana, C. R. (2020). Glucanases and chitinases. Curr. Top. Microbiol. Immunol. 425, 131–166. doi: 10.1007/82_2019_185
Sakaguchi, H. (2017). Treatment and prevention of oral candidiasis in elderly patients. Med. Mycol. J. 58, J43–J49. doi: 10.3314/mmj.17.004
Sant, D. G., Tupe, S. G., Ramana, C. V., and Deshpande, M. V. (2016). Fungal cell membrane-promising drug target for antifungal therapy. J. Appl. Microbiol. 121, 1498–1510. doi: 10.1111/jam.13301
Shao, X., Cheng, S., Wang, H., Yu, D., and Mungai, C. (2013). The possible mechanism of antifungal action of tea tree oil on Botrytis cinerea. J. Appl. Microbiol. 114, 1642–1649. doi: 10.1111/jam.12193
Sharma, N., Bagga, B., Singhal, D., Nagpal, R., Kate, A., Saluja, G., et al. (2022). Fungal keratitis: A review of clinical presentations, treatment strategies and outcomes. Ocul. Surf. 24, 22–30. doi: 10.1016/j.jtos.2021.12.001
Shen, T., Wang, Q., Li, C., Zhou, B., Li, Y., and Liu, Y. (2020). Transcriptome sequencing analysis reveals silver nanoparticles antifungal molecular mechanism of the soil fungi Fusarium solani species complex. J. Hazard. Mater. 388:122063. doi: 10.1016/j.jhazmat.2020.122063
Sun, L., Li, X., Ma, H., He, R., and Donkor, P. O. (2019). Global gene expression changes reflecting pleiotropic effects of Irpex lacteus induced by low--intensity electromagnetic field. Bioelectromagnetics 40, 104–117. doi: 10.1002/bem.22171
Tao, N., Jia, L., and Zhou, H. (2014). Anti-fungal activity of Citrus reticulata Blanco essential oil against Penicillium italicum and Penicillium digitatum. Food Chem. 153, 265–271. doi: 10.1016/j.foodchem.2013.12.070
Thompson, G. R., Cadena, J., and Patterson, T. F. (2009). Overview of antifungal agents. Clin. Chest Med. 30, 203–215. doi: 10.1016/j.ccm.2009.02.001
Tian, J., Ban, X., Zeng, H., He, J., Chen, Y., and Wang, Y. (2012). The mechanism of antifungal action of essential oil from dill (Anethum graveolens L.) on Aspergillus flavus. PLoS One 7:e30147. doi: 10.1371/journal.pone.0030147
van de Veerdonk, F. L., Gresnigt, M. S., Romani, L., Netea, M. G., and Latgé, J. P. (2017). Aspergillus fumigatus morphology and dynamic host interactions. Nat. Rev. Microbiol. 15, 661–674. doi: 10.1038/nrmicro.2017.90
Vanitha, T., Thammawong, M., Umehara, H., Nakamura, N., and Shiina, T. (2019). Effect of hinokitiol impregnated sheets on shelf life and quality of “KEK-1” tomatoes during storage. Packag. Technol. Sci. 32, 641–648. doi: 10.1002/pts.2479
Wang, Y., Liu, X., Chen, T., Xu, Y., and Tian, S. (2020). Antifungal effects of hinokitiol on development of Botrytis cinerea in vitro and in vivo. Postharvest Biol. Technol. 159:111038. doi: 10.1016/j.postharvbio.2019.111038
Wiederhold, N. P. (2018). The antifungal arsenal: alternative drugs and future targets. Int. J. Antimicrob. Agents 51, 333–339. doi: 10.1016/j.ijantimicag.2017.09.002
Wootton, M., Davies, L., Pitman, K., and Howe, R. A. (2020). Evaluation of susceptibility testing methods for Burkholderia cepacia complex: a comparison of broth microdilution, agar dilution, gradient strip and EUCAST disc diffusion. Clin. Microbiol. Infect. 2020:30708. doi: 10.1016/j.cmi.2020.11.012
Yang, J., and Zhang, K. Q. (2019). Chitin synthesis and degradation in fungi: Biology and enzymes. Adv. Exp. Med. Biol. 1142, 153–167. doi: 10.1007/978-981-13-7318-3_8
Yin, M., Li, C., Zhang, L., Zhang, L., Lin, J., Jiang, N., et al. (2022). Mechanism of antifungal activity and therapeutic action of β-ionone on Aspergillus fumigatus keratitis via suppressing LOX1 and JNK/p38 MAPK activation. Int. Immunopharmacol. 110:108992. doi: 10.1016/j.intimp.2022.108992
Zhao, W., Li, C., Liang, J., and Sun, S. (2014). The Aspergillus fumigatus β-1,3-glucanosyltransferase Gel7 plays a compensatory role in maintaining cell wall integrity under stress conditions. Glycobiology 24, 418–427. doi: 10.1093/glycob/cwu003
Keywords: hinokitiol, Aspergillus fumigatus, antifungal, cell wall, cell membrane
Citation: Meng F, Liu X, Li C, Peng X, Wang Q, Xu Q, Sui J, Zhao G and Lin J (2023) Hinokitiol inhibits Aspergillus fumigatus by interfering with the cell membrane and cell wall. Front. Microbiol. 14:1132042. doi: 10.3389/fmicb.2023.1132042
Received: 26 December 2022; Accepted: 20 March 2023;
Published: 11 April 2023.
Edited by:
Geelsu Hwang, University of Pennsylvania, United StatesReviewed by:
Zhangyong Song, Southwest Medical University, ChinaCopyright © 2023 Meng, Liu, Li, Peng, Wang, Xu, Sui, Zhao and Lin. This is an open-access article distributed under the terms of the Creative Commons Attribution License (CC BY). The use, distribution or reproduction in other forums is permitted, provided the original author(s) and the copyright owner(s) are credited and that the original publication in this journal is cited, in accordance with accepted academic practice. No use, distribution or reproduction is permitted which does not comply with these terms.
*Correspondence: Jing Lin, bGluamluZ195a0AxMjYuY29t; bGluamluZ0BxZHVob3NwaXRhbC5jbg==
†ORCID: Jing Lin https://orcid.org/0000-0002-6775-3125
Disclaimer: All claims expressed in this article are solely those of the authors and do not necessarily represent those of their affiliated organizations, or those of the publisher, the editors and the reviewers. Any product that may be evaluated in this article or claim that may be made by its manufacturer is not guaranteed or endorsed by the publisher.
Research integrity at Frontiers
Learn more about the work of our research integrity team to safeguard the quality of each article we publish.