- 1State Key Laboratory of Soil and Sustainable Agriculture, Institute of Soil Science, Chinese Academy of Sciences, Nanjing, China
- 2University of Chinese Academy of Sciences, Beijing, China
- 3College of Tropical Crops, Hainan University, Haikou, China
Introduction: Brachiaria humidicola, a tropical grass, could release root exudates with biological nitrification inhibition (BNI) capacity and reduce soil nitrous oxide (N2O) emissions from grasslands. However, evidence of the reduction effect in situ in tropical grasslands in China is lacking.
Methods: To evaluate the potential effects of B. humidicola on soil N2O emissions, a 2-year (2015–2017) field experiment was established in a Latosol and included eight treatments, consisting of two pastures, non-native B. humidicola and a native grass, Eremochloa ophiuroide, with four nitrogen (N) application rates. The annual urea application rates were 0, 150, 300, and 450 kg N ha−1.
Results: The average 2-year E. ophiuroides biomass with and without N fertilization were 9.07–11.45 and 7.34 t ha−1, respectively, and corresponding values for B. humidicola increased to 31.97–39.07 and 29.54 t ha−1, respectively. The N-use efficiencies under E. ophiuroide and B. humidicola cultivation were 9.3–12.0 and 35.5–39.4%, respectively. Annual N2O emissions in the E. ophiuroides and B. humidicola fields were 1.37 and 2.83 kg N2O-N ha−1, respectively, under no N fertilization, and 1.54–3.46 and 4.30–7.19 kg N2O-N ha−1, respectively, under N fertilization.
Discussions: According to the results, B. humidicola cultivation increased soil N2O emissions, especially under N fertilization. This is because B. humidicola exhibited the more effective stimulation effect on N2O production via denitrification primarily due to increased soil organic carbon and exudates than the inhibition effect on N2O production via autotrophic nitrification. Annual yield-scaled N2O emissions in the B. humidicola treatment were 93.02–183.12 mg N2O-N kg−1 biomass, which were significantly lower than those in the E. ophiuroides treatment. Overall, our results suggest that cultivation of the non-native grass, B. humidicola with BNI capacity, increased soil N2O emissions, while decreasing yield-scaled N2O emissions, when compared with native grass cultivation.
1. Introduction
Nitrous oxide (N2O) is a potent greenhouse gas with a significant 100-year global warming potential that is 265 times higher than that of carbon dioxide on a per-molecule basis (IPCC, 2013). In addition, N2O depletes stratospheric ozone, which protects the earth from biologically damaging ultraviolet radiation (Ravishankara et al., 2009). Notably, the concentration of atmospheric N2O has increased from 270 ppb during the pre-industrial era to 335.55 ppb in 2022, with an average annual increase rate of 0.90 ppb over the last 2 decades (Lan et al., 2022). Agriculture reportedly emitted approximately 4.1 Tg N2O-N year−1, accounting for approximately 66% of total global anthropogenic N2O emissions (UNEP, 2013). Using the dynamic land ecosystem model, Dangal et al. (2019) estimated that the net N2O emission from the global grasslands was 2.2 Tg N2O-N year−1, which was responsible for 54% of the total agricultural N2O emissions.
To meet the increasing food demands, nitrogen (N) fertilizer and agricultural land are growing substantially (Foley et al., 2011). The global synthetic N fertilizer consumption has increased from 12 to 112 Tg N while that has risen from 0.8 to 24 Tg N in China during the 1961–2020.1 However, the N-use efficiency (NUE) in China was only 28–35%, which is much lower than the global average (Liu et al., 2013; Han et al., 2015; Zhang et al., 2015).The heavy reliance of N fertilizers in agriculture has contributed to the stimulation of nitrifier activity and the trend toward high-nitrifying soil environments (Poudel et al., 2002; Bellamy et al., 2005).
Nitrification is closely related to N utilization and loss, and has become a key process to improve NUE and reduce N pollution (Subbarao et al., 2006; Beeckman et al., 2018). Nitrification is a microbes-driven process of oxidizing ammonia (NH3) to nitrite and further to nitrate (NO3−) and producing N2O as a byproduct (Stein, 2020). The NO3− produced during nitrification serves as a substrate and denitrification further reduces NO3− to dinitrogen and produces N2O as an intermediate product (Coskun et al., 2017a). Nitrification inhibitors can depress the activities of nitrifiers in soil, thereby delaying NH3 oxidation and reducing N2O emissions and NO3− production (Rodgers, 1986; Coskun et al., 2017b). To date, a few synthetic nitrification-inhibiting compounds have been efficiently adopted in the field, such as nitrapyrin, dicyandiamide, and 3,4-dimethyl pyrazole phosphate (Weiske et al., 2001; Zerulla et al., 2001; Niu et al., 2018). Meta-analysis revealed that the combination application of nitrification inhibitors and urea reduced NO3− leaching by 48% and N2O emissions by 44% (Burzaco et al., 2014), and increased crop yields by 7.5% and NUE by 12.9% (Abalos et al., 2014a). However, synthetic nitrification inhibitors have certain limitations such as low cost-effectiveness, application challenges, poor biological stability, and environmental pollution risks (Subbarao et al., 2012; Coskun et al., 2017b; Wang et al., 2021).
Natural compounds with biological nitrification inhibition (BNI) have been found from litters, root exudates, tissue extracts, and rhizosphere of plants such as grasses, trees, and crops (Wang et al., 2021), including methyl 3-(4 hydroxyphenyl) propionate (MHPP), sorgoleone and sakuranetin from sorghum (Subbarao et al., 2013), 1,9-decanediol from rice (Sun et al., 2016) and brachialactone from Brachiaria humidicola grass (Subbarao et al., 2009). Some root-secreted biological nitrification inhibitors (BNIs) like sorgoleone, sakuranetin, and brachialactone as well as linolenic acid and linoleic acid found in B. humidicola can inhibit both ammonia mono-oxygenase and hydroxylamine oxidoreductase activities (Coskun et al., 2017b), while 1,9-decanediol and MHPP only inhibits activity of ammonia mono-oxygenase (Zakir et al., 2008; Nardi et al., 2013, 2020; Sun et al., 2016; Lu et al., 2019). Up to date, the functional validation of the BNIs is mainly performed in the pure culture system of a single strain Nitrosomonas europaea, and the effect in the complicated soil system remains to be tested (Subbarao et al., 2015). For example, sakuranetin isolated from sorghum shows a strong inhibitory activity in vitro-cultural bioassay but losses the inhibitory effect in soil-assay (Subbarao et al., 2013). Gopalakrishnan et al. (2009) found that the inhibition effect of BNIs is affected by soil type, and the BNIs derived from B. humidicola in Cambisol can inhibit 90% nitrification with comparable effects to dicyandiamide (50 mg kg−1 soil), but are less effective in Andosol during the 60-day incubation.
Forage grasses with biological nitrification inhibition (BNI) capacity exhibit approximately 2-fold greater productivity than those lacking such capacity in nutrient-limited ecosystems, based on an estimate of a newly developed model (Lata et al., 1999; Boudsocq et al., 2009). The B. humidicola, reportedly exhibits the strongest BNI function among tropical grasses reduces the NH3 oxidation rate and N2O emissions significantly during a 3-year field experiment, when compared with soybean-planted or plant-free soils (Subbarao et al., 2009). During a short-term (29 days) monitoring period in Colombia, cumulative N2O emissions from a B. humidicola cv. Tully field was decreased by approximately 60% when compared with that in a Brachiaria hybrid cv. Mulato field under bovine urine amendment (Byrnes et al., 2017). In contrast, no significant effect on N2O emissions of the two forage genotypes was observed under cattle dung amendment in the same experimental site (Lombardi et al., 2022).
Latosol is a most widely distributed soil and covers 51.26% of the total area in Hainan Province, China. In the present study, a 2-year field experiment was conducted in a Latosol cultivated with B. humidicola and a native grass species, Eremochloa ophiuroides. We hypothesized that in situ N2O emissions from grasslands under cultivation with Brachiaria with higher BNI capacity are lower than in those cultivated with Eremochloa. The objectives of the present study were: (1) to determine whether the N2O emissions from a B. humidicola field are lower than those from an E. ophiuroides field in tropical Hainan Province, China; and (2) to evaluate the mitigation effects of B. humidicola on yield-scaled N2O emissions under the different N application rates. We also established an incubation with soils from the field experiment using a 15N tracing technique to evaluate the influence of B. humidicola on the N transformation process rates and N2O production rates via nitrification and denitrification (Xie et al., 2022).
2. Materials and methods
2.1. Study site
The field site was located in Danzhou, Hainan Province, China (109°29′ E, 19°30′ N). The region is characterized by a tropical monsoon climate, with a rainy season from May to October, and a dry season from November to April. The mean annual temperature and precipitation are 23.1°C and 1,823 mm, respectively. The soil is derived from granite and classified as a Latosol, with a sandy loam texture. Latosol is a most widely distributed soil in Hainan Province. The properties of surface soil (0–20 cm) prior to the field experiment are shown in Table 1.
2.2. Experimental design
A field experiment was established in August 2015 and included eight treatments, consisting of two pastures, Brachiaria humidicola CIAT679 and Eremochloa ophiuroides, with four N application rates. The annual urea application rates were 0, 150, 300, and 450 kg N ha−1, which were designated as BCK, BN1, BN2, and BN3, respectively, for B. humidicola, and ECK, EN1, EN2, and EN3, respectively, for E. ophiuroides. The plots measured 3 m × 4 m. The treatments, which had three replicates, were set up based on a randomized complete block design. During the first season from August 2015 to August 2016, 60 and 40% of urea was applied as basal fertilizer and top-dressing fertilizer, respectively, in the fertilized treatments. In the second season from August 2016 to August 2017, urea was added with three splits: 40% as basal fertilizer, and 30% as top-dressing fertilizer on 13 March and 9 June 2017, respectively. Calcium superphosphate (150 kg P2O5 ha−1) and potassium chloride (105 kg K2O ha−1) were applied as basal fertilizer. All the fertilizers were dissolved in water and uniformly spread into the soil. Harvested plant samples were oven-dried at 60°C to a constant weight, and then ground to less than 0.2 mm for analysis. Field management practices were similar to local practices and standardized at all plots. Specific dates are listed in Table 2.
2.3. Nitrous oxide flux measurement
Nitrous oxide fluxes were measured using the static chamber method. Before grass planting, a stainless steel chamber with a rectangular base (50 cm × 50 cm × 15 cm) and a 5-cm groove around the upper edge was permanently fit 10 cm into the soil. During gas sampling, a stainless chamber (50 cm × 50 cm × 50 cm) was inserted into the groove, which was filled with water to ensure airtightness. The chamber was covered with reflective film and foam to minimize air temperature change inside the chamber. A rubber plug with a mercury thermometer was fit tightly into the hole on the top of the chamber for use in measuring the chamber temperature while gas sampling. Two vents welded with stainless tubes were punched on top of the chamber, one connected to a rubber tube with a three-way stopcock for gas collection, and another one for ensuring air pressure equilibrium inside and outside the chamber. Gas samples were obtained once every other day during 1 week after each fertilization and once a week during the other period. Sampling was conducted between 7:00 am and 12:00 pm to minimize diurnal variation. Four gas samples were extracted from the chamber at 0, 10, 20, and 30 min after chamber closure using airtight plastic syringes and instantly injected into 20-ml pre-evacuated vials fitted with butyl rubber stoppers. The N2O concentrations were analyzed using a gas chromatograph (GC; Agilent 7890, Agilent Technologies, Santa Clara, CA, United States) equipped with a 63Ni electron capture detector and a thermal conductivity detector. The N2O fluxes were calculated using the following equation (Niu et al., 2018):
where F is the flux in N2O (μg N2O-N m−2 h−1); ρ is the density of N2O at 0°C and 760 mm Hg (kg m−3); V is the effective volume of the chamber (m3); S is the soil area covered by the chamber (m2); ΔC/Δt is the rate of N2O concentration increase in the chamber (ppbv N2O-N h−1); and, T is mean air temperature inside the chamber during sampling (°C).
2.4. Auxiliary variables measurement
Soil temperature was measured at 5 cm depth using a geothermometer. Soil water content was measured at three different positions in each plot with time domain reflectometry (TDR) probes and expressed as water-filled pore space (WFPS, %) as follows (Niu et al., 2018):
where total soil porosity = 1 - (soil bulk density/2.65), 2.65 being the soil particle density (g cm−3).
Surface (0–20 cm) soil samples were collected at five different positions in each plot fortnightly using a stainless steel soil sampler and thoroughly mixed to form a composite sample. The samples were taken to the laboratory immediately and stored at −20°C before analysis. Soil exchangeable ammonium-N (NH4+-N) and nitrate-N (NO3−-N) were extracted with 2 M KCl (soil/KCl solution ratio of 1:5) by agitating for 1 h on a rotary shaker, and the concentrations were measured using a colorimetric method on a segmented flow analyzer (Skalar, The Netherlands; Chen et al., 2014). Dissolved organic C (DOC) was extracted with deionized water at a soil water ratio of 1:5, which was shaken for 0.5 h, followed by centrifugation for 15 min at 2,300 × g and filtration (<0.45 μm). Subsequently, the DOC was analyzed using the combustion oxidation nondispersive infrared absorption method on a TOC analyzer (vario TOC Cube, Elementar, Hanau, Germany).
Soil samples were collected after the end of the field experiments. Soil pH was determined from soil-water suspensions (1:2.5 v/v) using a pH meter (SevenCompact, Mettler Toledo, Swiss). Soil organic C (SOC) was measured using the wet oxidation-redox titration method (Walkley and Black, 1934). Total N content in soil and plant was determined using an elemental analyzer (Vario MAX, Elementar, Germany). Soil available P was extracted with 0.05 M HCl and 0.025 M H2SO4, and determined using the molybdenum blue colorimetric method (Ye et al., 2019). Available K was extracted with ammonium acetate and analyzed using a flame photometer (Lu, 2000).
2.5. Data calculation and statistical analysis
Annual cumulative N2O emissions (EN2O, kg N2O-N ha−1) were calculated using the following equation (Chen et al., 2014):
where f is the N2O flux (μg N2O-N m−2 h−1); i is the ith measurement; (ti + 1 –ti) is the interval between the th and the (i + 1)th measurement time (d); n is the total number of measurements; and 24 × 10−5 was used for unit conversion.
The N2O emission factor of applied fertilizer N (EF, %) was calculated using the following equation:
where Efertilizer and Econtrol are the cumulative N2O emissions from the fertilized and control treatments, respectively; and Napplied is the amount of fertilizer N applied to the corresponding treatment.
The yield-scaled N2O emission (mg N2O-N kg−1 biomass) was computed using the following equation (Venterea et al., 2011):
where EN2O is the annual cumulative N2O emissions (kg N2O-N ha−1); and yield is the amount of grass biomass harvested annually (kg ha−1).
Fertilizer N-use efficiency (NUE, %) was calculated as follows:
where Nfertilizer and Ncontrol are the amount of N uptake in aboveground biomass (kg N ha−1) in the fertilized and control treatments, respectively; and Napplied is the amount of the N applied to the corresponding treatment (kg N ha−1).
Significant differences among treatments were evaluated using one-way ANOVA followed by the Duncan test at p < 0.05. Spearman’s correlation analysis was used to determine the relationships between N2O flux and soil WFPS, soil inorganic N, soil dissolved organic C, and air temperature. All statistical analyses were performed using IBM SPSS Statistics 26 for Windows (IBM corp., Armonk, NY, United States).
3. Results
3.1. Soil characteristics
After 2 years of grass cultivation, soil pH in all the treatments increased when compared with that in the pre-treatment soil (Table 3). The maximum soil pH was observed in the ECK treatment without N fertilization, and soil pH decreased with an increase in N application rate in both grasslands. SOC increased by 17.5–22.8% under B. humidicola cultivation and only by 5.8–15.1% under E. ophiuroides, when compared with the pre-treatment soil. Cultivation of both pastures promoted soil N accumulation significantly (p < 0.05); however, there were no significant differences in soil N accumulation among treatments under different N application rates (Table 3).
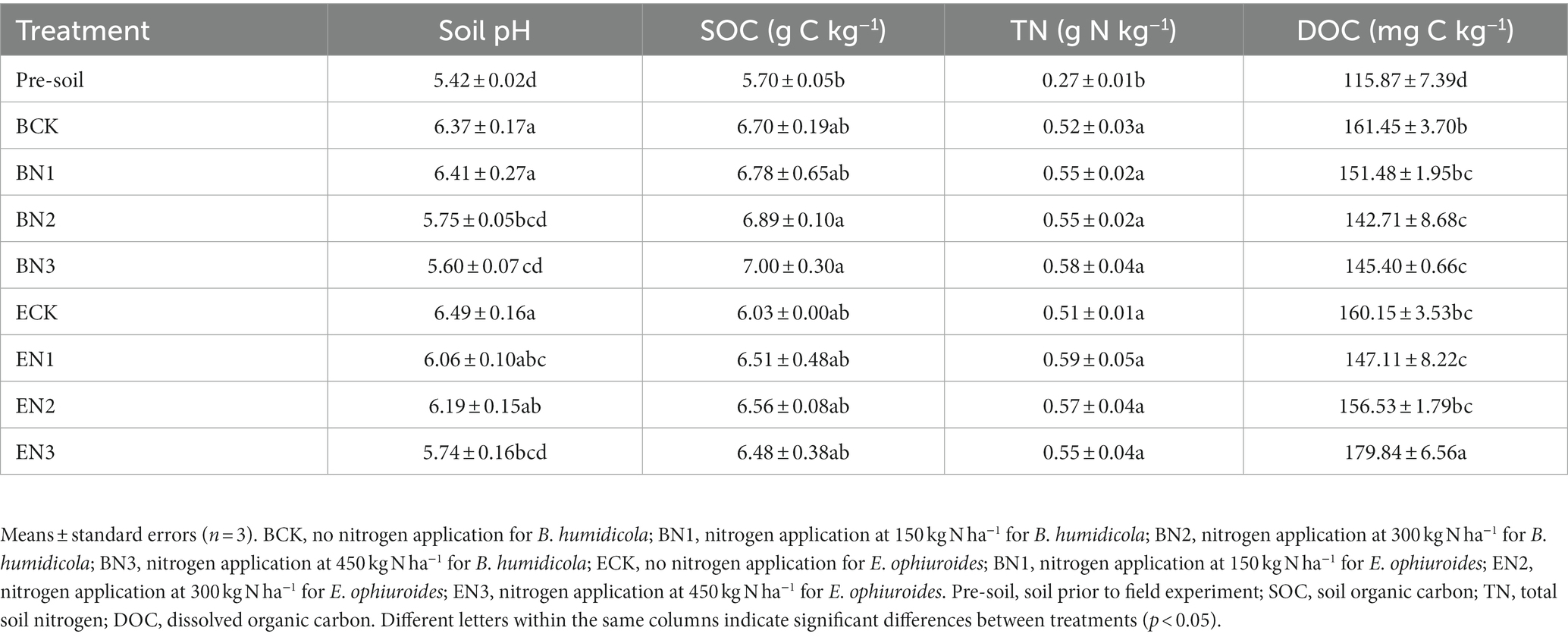
Table 3. Soil properties before and after 2 years of Brachiaria humidicola and Eremochloa ophiuroides cultivation.
3.2. Grass yield
The biomass of B. humidicola ranged from 29.54 to 31.37 t ha−1 in the BCK treatment, which was 3.1–6.0-fold that of E. ophiuroide during the two seasons (Table 4). The N application increased biomass yield of B. humidicola by 11.3–25.8% (p < 0.05) but did not increase the biomass yield of E. ophiuroides, during the first season. During the 2016–2017 season, however, the biomass of both grasses was enhanced with N fertilization, and increased with an increase in the N application rate (p < 0.05).
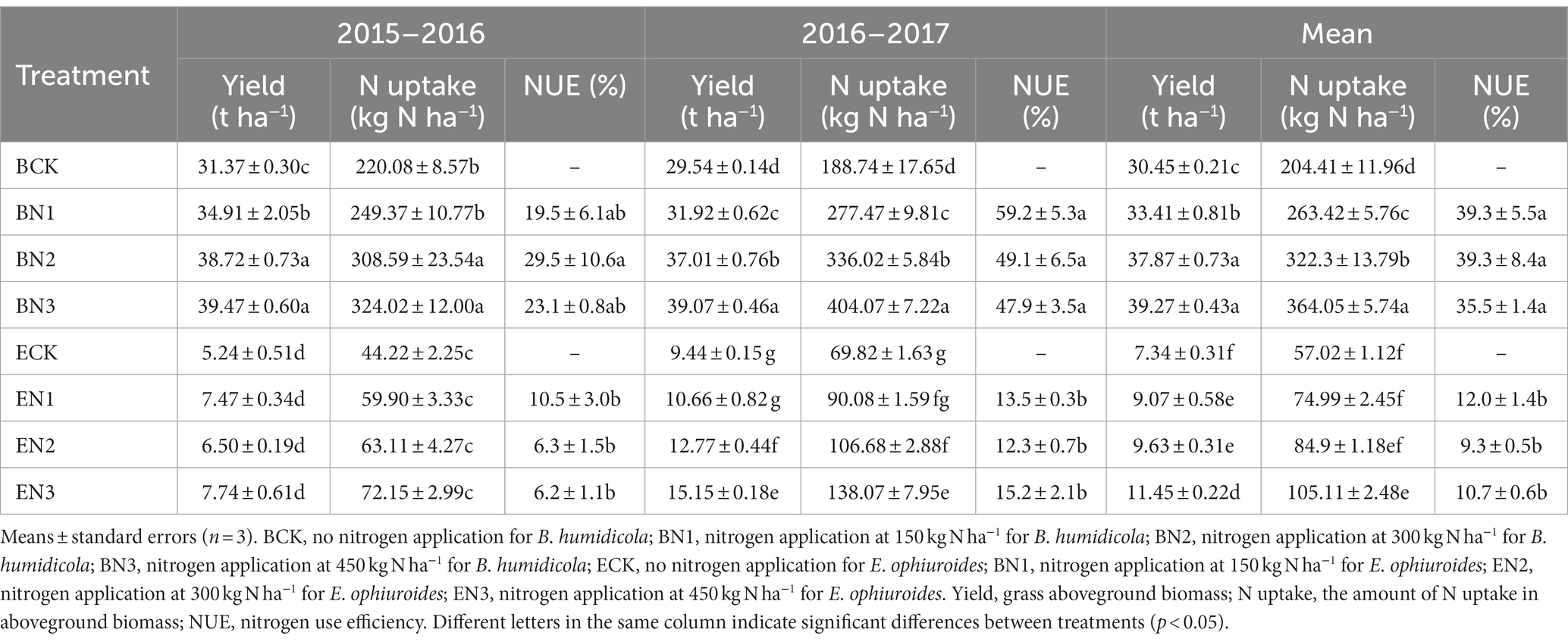
Table 4. Yield, nitrogen uptake, and nitrogen use efficiency of Brachiaria humidicola and Eremochloa ophiuroides during two growth seasons.
The amounts of N uptake by B. humidicola under no N fertilization were 220.08 and 188.74 kg N ha−1 during the 2015–2016 and 2016–2017 seasons, respectively, and decreased to 44.22 and 69.82 kg N ha−1 for E. ophiuroide, respectively (Table 4). The mean NUE of the N applied under B. humidicola was 19.5–29.5% during the 2015–2016 season and increased to 47.9–59.2% during the 2016–2017 season, which was significantly higher than that under E. ophiuroides during both seasons.
3.3. Soil and environmental variables
Air temperature (AT) ranged from 5.4 to 32.6°C, with an average of 24.8°C over the 2-year measurement period, and there was no apparent difference between two growth seasons (Figure 1). Soil temperature (ST) at 5 cm depth ranged from 13.7 to 34.8°C, a trend similar to that of AT (ST = 0.758AT + 7.062, R2 = 0.42, p < 0.01). Mean rainfall was 2,341 and 2,373 mm during the 2015–2016 and 2016–2017 seasons, respectively. Precipitation mainly occurred in the rainy season, from May to October, accounting for 87% of the total annual precipitation. Soil moisture fluctuated from 5.1 to 58.3% WFPS, and the mean WFPS in all the treatments was 33.5–38.3%, with no significant differences among treatments.
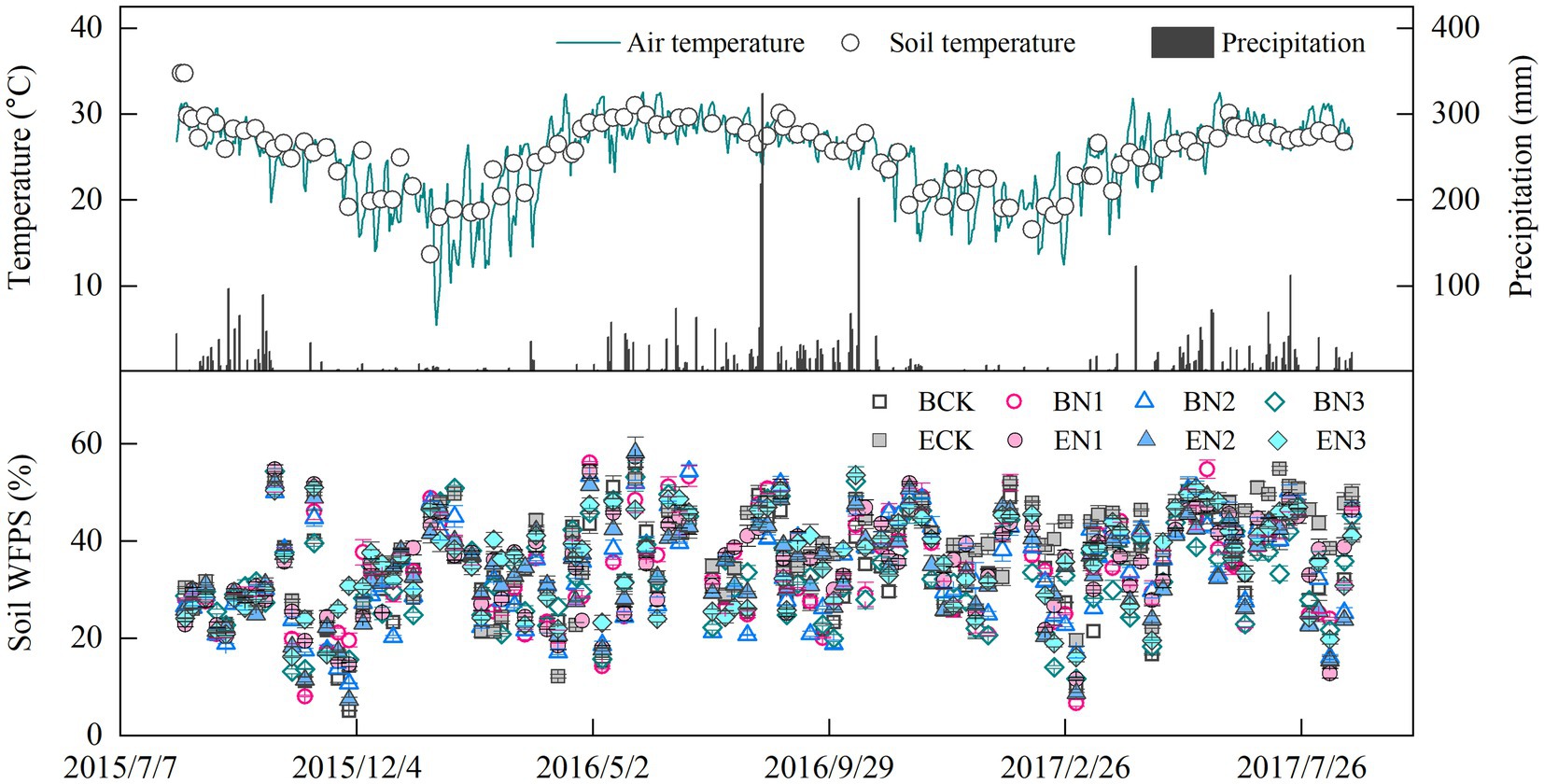
Figure 1. Temporal variation in precipitation, air temperature, and soil temperature at 5 cm depth, and water-filled pore space (WFPS). Vertical bars denote the standard errors of the mean (n = 3).
Soil NH4+-N concentration peaks occurred approximately 1 week after each fertilization, and decreased to a constant level 40 days later (Figure 2A). The mean soil NH4+-N concentrations under the BCK and ECK treatments were 4.60 and 4.05 mg N kg−1, respectively and increased to 10.46–14.93 and 10.09–15.91 mg N kg−1 in the BN and EN treatments, respectively, increasing with increases in the N application rate. Mean soil NH4+-N concentrations were not significantly different between the B. humidicola and E. ophiuroides fields under similar N application rates. Soil NO3−-N concentrations in the BCK and ECK treatments were on average 3.21 and 2.59 mg N kg−1, respectively (Figure 2B). Under N fertilization, mean soil NO3−-N concentrations increased to 5.61, 6.02, and 8.42 mg N kg−1 in the BN1, BN2, and BN3 treatments, respectively, which were higher than the corresponding values under E. ophiuroides cultivation, excluding BN2 (p < 0.05).
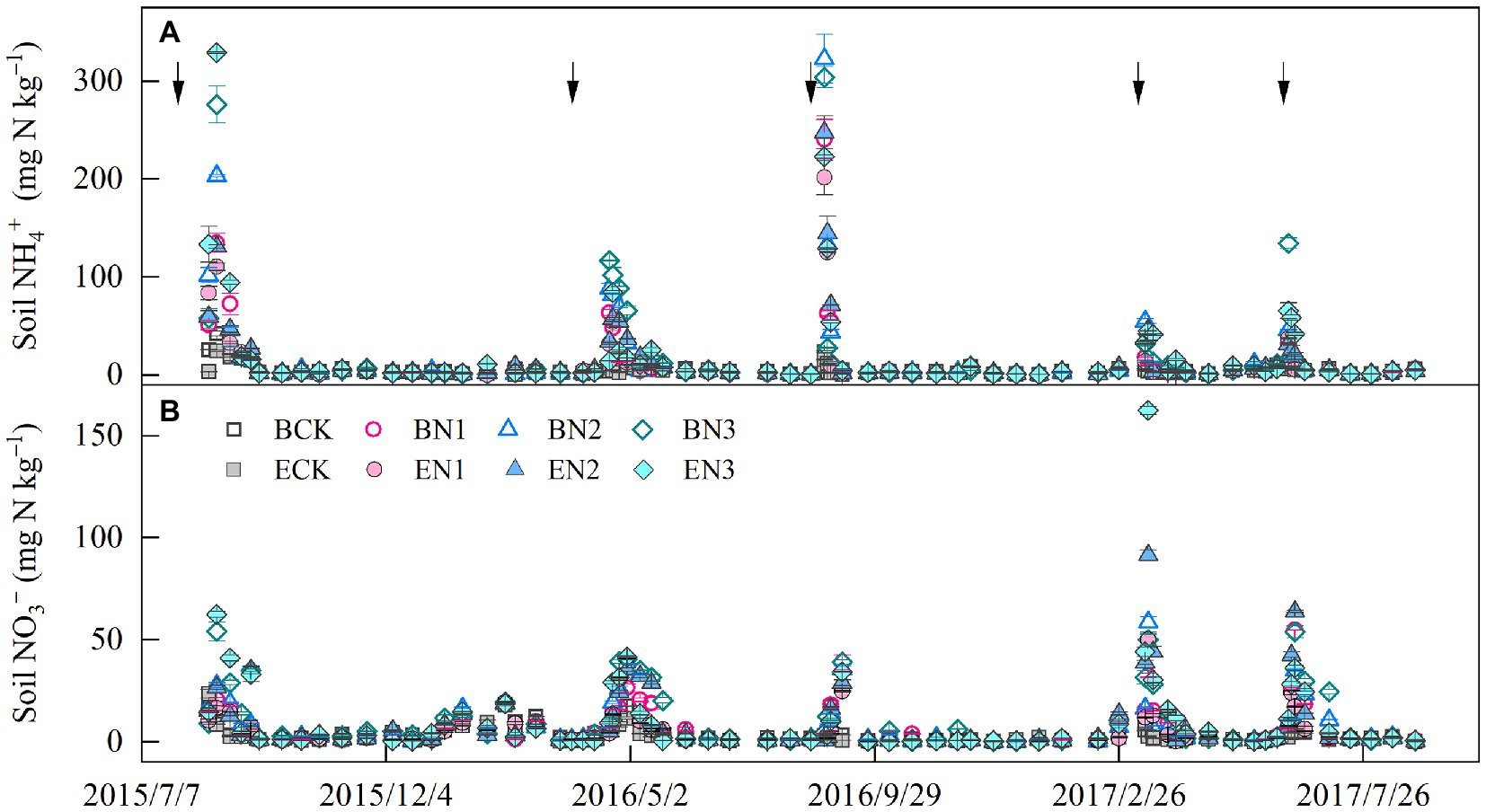
Figure 2. Soil ammonium (A) and nitrate (B) concentration dynamics in the 0–20-cm layer. Vertical bars denote the standard errors of the mean (n = 3). The solid arrows indicate the N fertilization time.
3.4. Nitrous oxide emissions
Nitrous oxide flux peaks emerged after each fertilization, and increased with an increase in the N application rates. The highest flux was 544.60 μg N2O-N m−2 h−1 in the BN3 treatment on 31 August 2016, which was 3-fold that in the EN3 treatment (Figure 3). During the 2016–2017 season, the peak flux in the BN3 treatments (344.60 μg N2O-N m−2 h−1) was also observed on 20 June 2017, which, however, was only 1.2-fold greater than that in the EN3 treatment. The N2O fluxes were significantly (p < 0.01) correlated with soil moisture, NH4+-N, NO3−-N, and air temperature (Table 5).
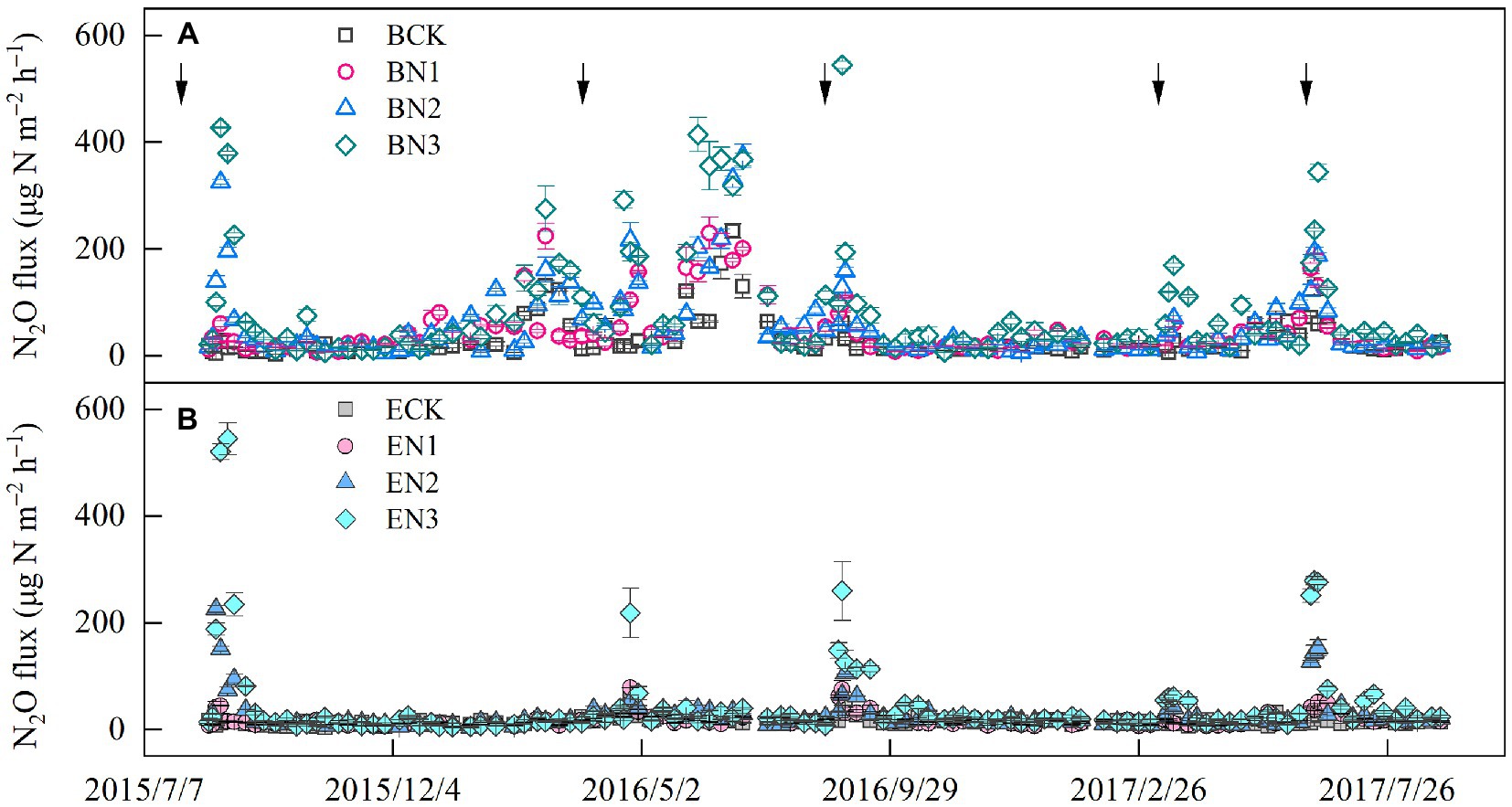
Figure 3. Temporal variation in nitrous oxide (N2O) flux in Brachiaria humidicola (A) and Eremochloa ophiuroides (B) soil. Solid line arrows indicate the timing of fertilizer application. Vertical bars denote the standard errors of the mean (n = 3). The solid arrows indicate the nitrogen (N) fertilization time.
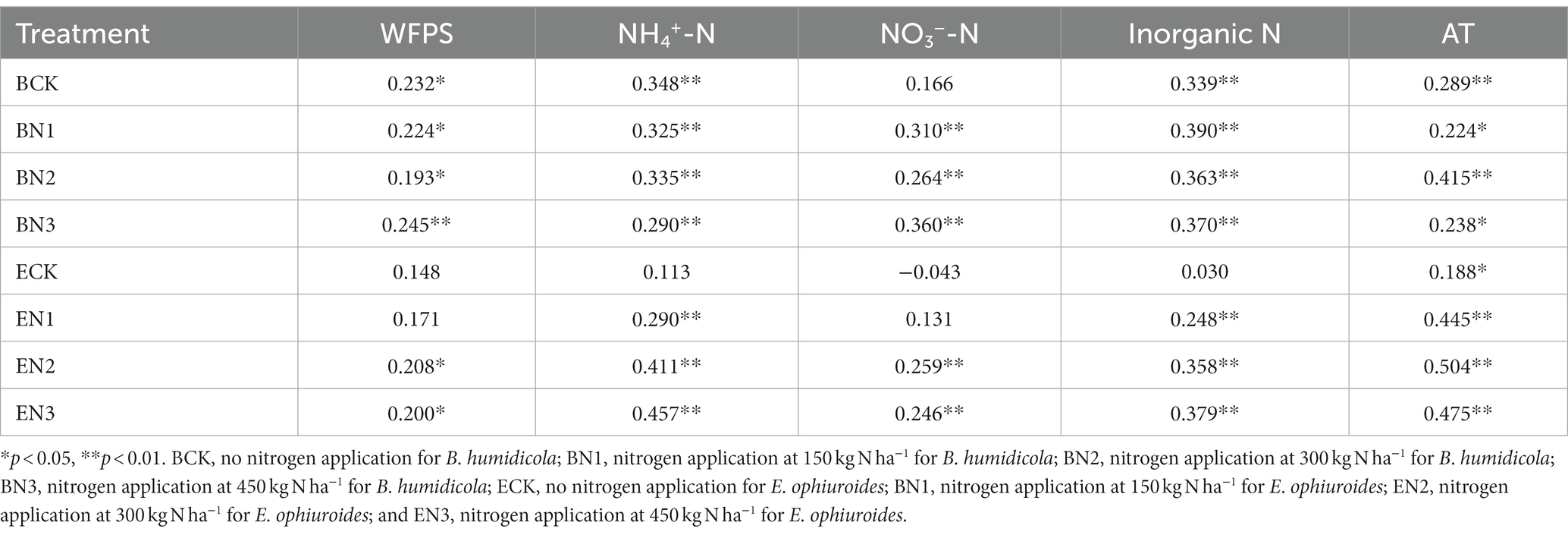
Table 5. Correlation between nitrous oxide (N2O) flux and soil moisture (WFPS), ammonium-nitrogen (NH4+-N), nitrate-nitrogen (NO3−-N), inorganic nitrogen (NH4+-N plus NO3−-N), and air temperature (AT).
Annual N2O emissions in the B. humidicola fields were higher than those in the E. ophiuroides fields, regardless of N fertilization rate (p < 0.05; Figure 4A). They were also greater during the first (2015–2016) season than during the second (2016–2017) season in the case of B. humidicola but not in the case of E. ophiuroides. Annual N2O emissions in the B. humidicola fields under BCK were 3.64 and 2.02 kg N2O-N ha−1 during the 2015–2016 and 2016–2017 season, respectively, with an average of 2.83 kg N2O-N ha−1. Under N fertilization, annual N2O emissions from the B. humidicola field increased to 5.72–9.54 and 2.88–4.84 kg N2O-N ha−1 during the 2015–2016 and 2016–2017 season, respectively. In the E. ophiuroides field, N2O emissions under no N fertilization (ECK) were 1.38 and 1.35 kg N2O-N ha−1 during the 2015–2016 and 2016–2017 seasons, respectively, and increased to 1.43–3.28 and 1.65–3.64 kg N2O-N ha−1 under N fertilization, respectively. The annual N2O emissions increased linearly with an increase in the N application rate in the B. humidicola fields (EN2O = 0.0092 Napplied + 2.76, R2 = 0.97); conversely, they exhibited exponential correlations with the N application rate in the E. ophiuroides fields (EN2O = 1.24e0.021Napplied, R2 = 0.95).
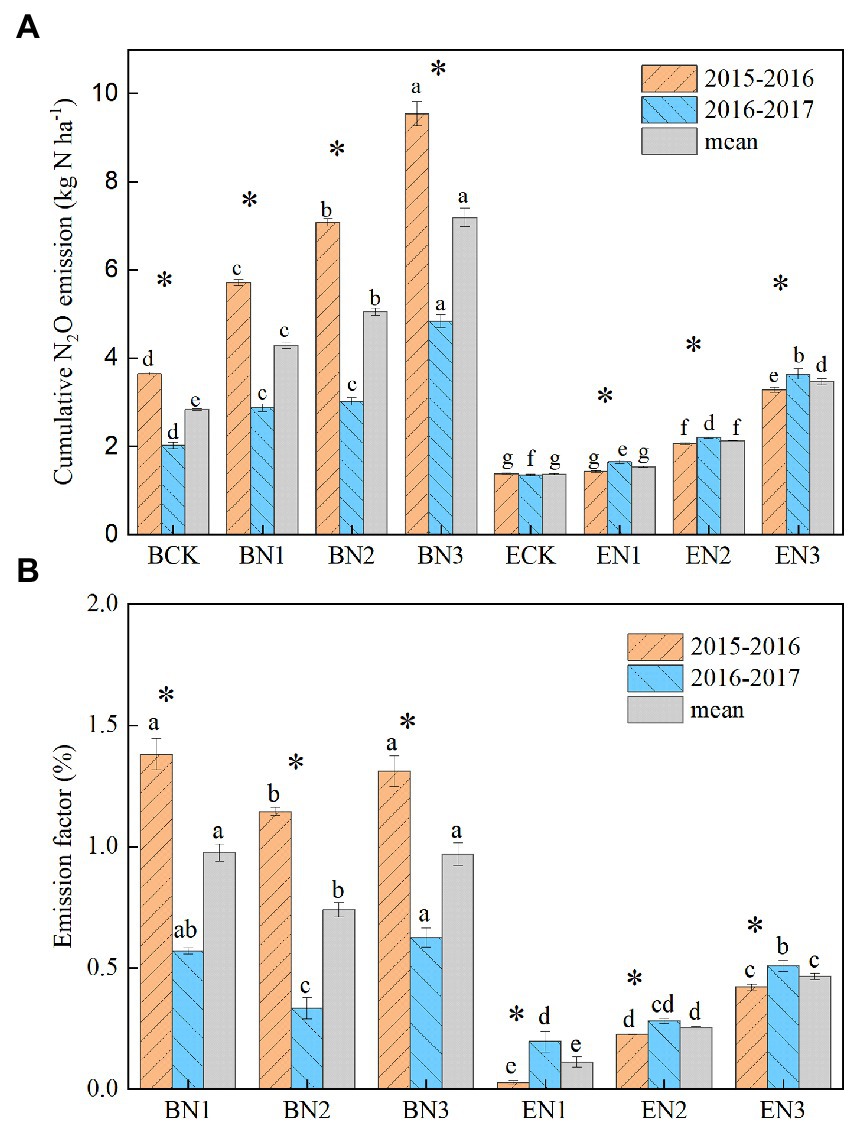
Figure 4. Annual soil nitrous oxide (N2O) emission (A) and emission factor of the fertilizer nitrogen applied (B) in the Brachiaria humidicola and Eremochloa ophiuroides fields. Vertical bars denote the standard errors of the mean (n = 3). Different letters indicate significant differences between treatments for the same measurement year and mean at p < 0.05. *indicates the significant difference between 2 years for the same treatment at p < 0.05.
The N2O emission factor (EF) of the N applied ranged from 0.74 to 0.98% for B. humidicola, and decreased to 0.11–0.47% for E. ophiuroides over the 2 years (Figure 4B). The EF increased with an increase in the N application rate only under E. ophiuroides cultivation.
3.5. Yield-scaled nitrous oxide emissions
The mean yield-scaled N2O emissions in the BCK and ECK treatments were 95 and 206 mg N2O-N kg−1 biomass, respectively, over the 2 years (Figure 5). Under N fertilization, they increased to 128, 132, and 183 mg N2O-N kg−1 biomass in the BN1, BN2, and BN3 treatments, respectively, which were significantly lower than the corresponding values in the EN treatments by 26.76–46.04%. The reduction increased with an increase in the N application rate.
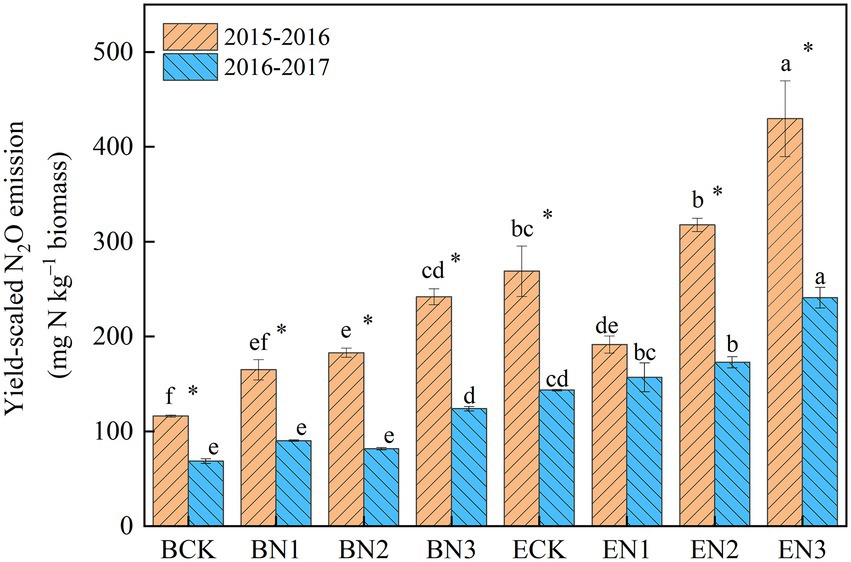
Figure 5. Yield-scaled N2O emissions from the Brachiaria humidicola and Eremochloa ophiuroides field. Vertical bars denote the standard errors of the mean (n = 3). Different letters indicate the significant differences between treatments for the same measurement year and for mean at p < 0.05. *indicates the significant difference between two measurement years for the same treatment at p < 0.05.
4. Discussion
Annual N2O emissions from this tropical grassland varied from 1.35 to 9.54 kg N2O-N ha−1, which was within the 0–29.1 kg N2O-N ha−1 range in grasslands as reported previously (Mosier et al., 1996; Wolf et al., 2010; Merbold et al., 2014; Luo et al., 2017). Out of expectation, N2O emissions from the B. humidicola field were 1.3–2.6-fold higher than those from the E. ophiuroides field under N fertilization. Additionally, the N2O emission factor of the N applied was increased to 0.74–0.98% under B. humidicola from 0.11–0.47% under E. ophiuroides. Our results suggest that cultivation of exotic, tropical forage grass B. humidicola with BNI capacity by replacing native E. ophiuroides stimulated N2O emission. To our knowledge, this is the first study to find the stimulation effect of B. humidicola on N2O emissions in the field when compared with the native grass. Apparently, more field studies are required to evaluate the impact of plants with BNI capacity on N2O emissions at the ecosystem and global scale, as suggested by Lata et al. (2022).
Previous study suggested that Brachiaria genotype with high BNI capacity reduced almost 50% of N2O emission when compared with soybean or plant-free soils (Subbarao et al., 2009). Byrnes et al. (2017) reported that B. humidicola cv. Tully with high BNI capacity reduced approximately 60% of N2O emissions in the field when compared with the Brachiaria hybrid cv. Mulato having low BNI capacity during the 29-day monitoring period. Planting B. humidicola with high BNI capacity reduced soil N2O emissions by 18.3% when compared with B. humidicola with low BNI capacity in a 21-day pot experiment (Teutscherová et al., 2022). The active substances with BNI capacity, such as methyl-p-coumarate, methyl ferulate, and brachialactone, have been identified from exudates of B. humidicola (Gopalakrishnan et al., 2009; Subbarao et al., 2009). Brachialactone can simultaneously block the enzymatic pathways of ammonia monooxygenase and hydroxylamino oxidoreductase (Subbarao et al., 2009). The inhibitory potential reportedly increases with an increase in grass root density (Subbarao et al., 2007; Boudsocq et al., 2009). Subbarao et al. (2009) estimated that B. humidicola roots can potentially release 2.6 × 106–7.5 × 106 ATU (allylthiourea units) ha−1 day−1 BNI activity in the South American savannas, which is equivalent to the application of 6.2–18 kg ha−1 nitrapyrin based on 1 ATU being equal to 0.6 μg of nitrapyrin. Karwat et al. (2017) demonstrated that B. humidicola, like dicyandiamide, significantly suppresses soil nitrification potential. In a previous study, using the 15N tracing incubation with soils collected from the B. humidicola and E. ophiuroides plots at the field experiment end, we found that B. humidicola decreased the autotrophic nitrification rate and N2O production rate via nitrification by 27.3 and 14.7%, respectively when compared with E. ophiuroides (Xie et al., 2022). This indicated that in the test soil, B. humidicola efficiently inhibited nitrification and N2O production via nitrification.
Subbarao et al. (2009) observed that cultivation of B. humidicola reduced the abundance of both ammonia-oxidizing archaea (AOA) and bacteria (AOB) in a Vertisol with pH 7.40 when compared with soil cultivated with soybean. Hink et al. (2018) further reported that although both AOA and AOB were capable of N2O production under high NH4+-N concentrations, the contribution of AOB was greater in a soil with pH 6.50. In the test acid soil with pH 5.42, it is likely that both AOA and AOB participated in NH3 oxidation and N2O production. Further investigations are required to determine the relative importance of AOA and AOB in N2O production, and the suppression effects of B. humidicola on AOA and AOB activity (Nuñez et al., 2018).
Byrnes et al. (2017) suggested that by increasing N uptake, B. humidicola with high BNI capacity more efficiently decreased soil NO3−-N availability and potential denitrification than B. humidicola with low BNI capacity, thereby reducing N2O emissions. Abalos et al. (2014b) reported that mixed cultivation of Folium perennial L. and Poi trivialize L. decreased soil NO3−-N concentrations and consequent N2O emissions when compared with either monoculture at an N application rate of 60 kg N ha−1. They suggested that the trends were attributed to L. perennial taking up N using the “scale strategy” by increasing root biomass, and P. trivialize absorbing N via the “precision strategy” by providing access to N hotspots that were not emptied by L. perennial. In the present study, although B. humid cola cultivation increased N uptake, N2O emissions were positively correlated with pasture yield and N uptake, indicating that increased N uptake by B. humid cola did not reduce N2O emissions. In the present study, the mean soil NO3−-N concentration under N fertilization ranged from 5.60 mg N kg−1 in the BN1 treatment to 8.45 mg N kg−1 in the BN3 treatment, which was higher than the 5 mg N kg−1 threshold for occurrence of denitrification (Dobbie and Smith, 2003), and indicated that although B. humid cola efficiently increased N uptake and partially inhibited nitrification, soil NO3−-N under N fertilization was still higher than the threshold value for denitrification in the test field.
Using 15N paired incubation (15NH4NO3 and NH415NO3), we found that the N2O production rate during denitrification in the B. humid cola soil increased by 7.7-fold when compared with the E. ophiuroides soil and the contribution of denitrification to N2O emissions sharply enhanced from 9.7% in the E. ophiuroides soil to 47.1% (Xie et al., 2022). In the present study, B. humidicola biomass was 3–6-fold greater than that of E. ophiuroides and SOC was more efficiently increased under B. humidicola. Horrocks et al. (2019) also observed that 1-year cultivation of B. humidicola increases SOC content and improves aggregate stability in Colombia. Fisher et al. (1994) and Amézquita et al. (2004) attributed the SOC enhancement to rapid accumulation of B. humidicola roots and exudates. Plant reportedly release as much as 40% of net photosynthetic C into the rhizosphere (Marschner, 2011), which in turn provides more labile C substrates for denitrifiers (Wu et al., 2017). Enhanced SOC at least exhibits two stimulation effects on denitrification. Firstly, enhanced SOC promotes the formation of anaerobic microsites for denitrification by stimulating aggregation (Bollmann and Conrad, 2004). Secondly, increased organic C availability reduces the soil moisture threshold for the occurrence of denitrification (Rochette et al., 2000; Van Groenigen et al., 2004; Chantigny et al., 2013) resulting in increased denitrification potentials. Our results indicate that cultivation of exotic B. humidicola with a much higher biomass compared with E. ophiuroides stimulated N2O production during denitrification by providing more organic C, which in turn masked N2O reduction by inhibiting nitrification, thereby enhancing N2O emissions.
Comparing yield-scaled N2O emissions has been suggested to be an effective way of evaluating the tradeoff between production and environmental impacts and determining the economic feasibility of N2O emission mitigation methods (van Groenigen et al., 2010; Grassini and Cassman, 2012). In the present study, yield-scaled N2O emissions from B. humidicola field with and without N fertilization during two seasons were 128.80–183.02 and 93.02 g N kg−1 biomass, respectively, which were significantly lower than the corresponding values under E. ophiuroides cultivation (171.07–221.62 and 186.93 g N kg−1 biomass, respectively). In addition, we observed interannual shifts in yield-scaled N2O emissions in both grasslands, which was primarily driven by changes in annual N2O emission in B. humidicola fields, whereas they were driven by changes in biomass yield in E. ophiuroides fields. The lower yield-scaled N2O emissions under B. humidicola cultivation compared with under E. ophiuroides indicated that although B. humidicola increased annual N2O emissions, it was more environmentally friendly based on its higher forage productivity and NUE.
5. Conclusion
In the present study, B. humidicola exhibited higher yields and NUE, and in contrast and unexpectedly, induced higher soil N2O emissions when compared with E. ophiuroides. Although cultivation of B. humidicola with high BNI capacity reduced N2O production rate via nitrification, however, it more efficiently enhanced N2O production rate than E. ophiuroides via denitrification due to increased SOC and exudate concentrations, thereby increasing N2O emissions (Figure 6). When compared with under E. ophiuroides, however, the lower yield-scaled N2O emissions under B. humidicola cultivation indicated that although B. humidicola increased annual N2O emissions, it was more environmentally friendly based on its higher forage productivity and NUE.
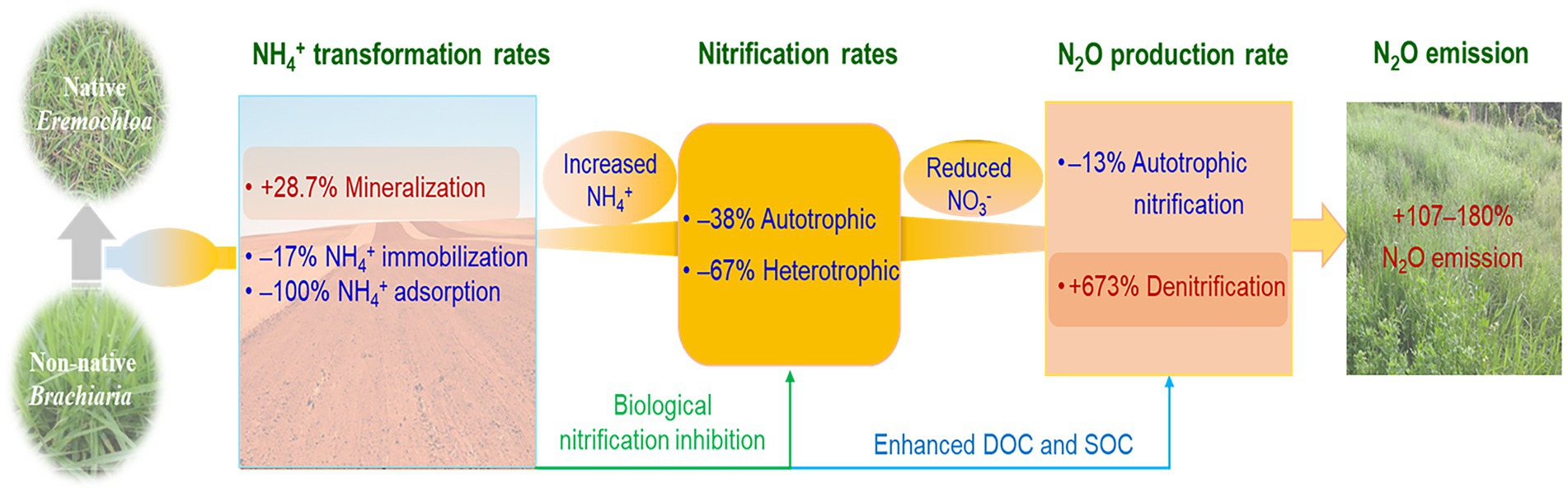
Figure 6. Schematic diagram showing how Brachiaria humidicola cultivation stimulated the nitrous oxide (N2O) emission in the study grassland. Nitrogen transformation rates and N2O production rates via autotrophic nitrification and denitrification are cited from Xie et al. (2022).
Data availability statement
The original contributions presented in the study are included in the article/supplementary material, further inquiries can be directed to the corresponding author.
Author contributions
WD and DL: conceptualization. LX, YN, and DL: field experiment. LX and ZC: data analysis. LX, WD, and LM: writing. WD: funding acquisition. All authors contributed to the article and approved the submitted version.
Funding
This work was financially supported from the National Natural Science Foundation of China (41730753, 41977049 and 42077029), the International Partnership Program of Chinese Academy of Sciences (151432KYSB20200001), and International Atomic Energy Agency coordinated research project (D15020).
Acknowledgments
We would like to gratefully acknowledge the Hainan University Danzhou Campus for providing the study site and assistance in field experiment. And special thanks to Fang Yage for the collaboration in field work.
Conflict of interest
The authors declare that the research was conducted in the absence of any commercial or financial relationships that could be construed as a potential conflict of interest.
Publisher’s note
All claims expressed in this article are solely those of the authors and do not necessarily represent those of their affiliated organizations, or those of the publisher, the editors and the reviewers. Any product that may be evaluated in this article, or claim that may be made by its manufacturer, is not guaranteed or endorsed by the publisher.
Footnotes
References
Abalos, D., De Deyn, G. B., Kuyper, T. W., and van Groenigen, J. W. (2014b). Plant species identity surpasses species richness as a key driver of N2O emissions from grassland. Glob. Chang. Biol. 20, 265–275. doi: 10.1111/gcb.12350
Abalos, D., Jeffery, S., Sanz-Cobena, A., Guardia, G., and Vallejo, A. (2014a). Meta-analysis of the effect of urease and nitrification inhibitors on crop productivity and nitrogen use efficiency. Agric. Ecosyst. Environ. 189, 136–144. doi: 10.1016/j.agee.2014.03.036
Amézquita, E., Thomas, R. J., Rao, I. M., Molina, D. L., and Hoyos, P. (2004). Use of deep-rooted tropical pastures to build-up an arable layer through improved soil properties of an Oxisol in the Eastern Plains (llanos Orientales) of Colombia. Agric. Ecosyst. Environ. 103, 269–277. doi: 10.1016/j.agee.2003.12.017
Beeckman, F., Motte, H., and Beeckman, T. (2018). Nitrification in agricultural soils: impact, actors and mitigation. Curr. Opin. Biotechnol. 50, 166–173. doi: 10.1016/j.copbio.2018.01.014
Bellamy, P. H., Loveland, P. J., Bradley, R. I., Lark, R. M., and Kirk, G. J. D. (2005). Carbon losses from all soils across England and Wales 1978–2003. Nature 437, 245–248. doi: 10.1038/nature04038
Bollmann, A., and Conrad, R. (2004). Influence of O2 availability on NO and N2O release by nitrification and denitrification in soils. Glob. Chang. Biol. 4, 387–396. doi: 10.1046/j.1365-2486.1998.00161.x
Boudsocq, S., Lata, J. C., Mathieu, J., Abbadie, L., and Barot, S. (2009). Modelling approach to analyse the effects of nitrification inhibition on primary production. Funct. Ecol. 23, 220–230. doi: 10.1111/j.1365-2435.2008.01476.x
Burzaco, J. P., Ciampitti, I. A., and Vyn, T. J. (2014). Nitrapyrin impacts on maize yield and nitrogen use efficiency with spring-applied nitrogen: field studies vs. meta-analysis comparison. Agron. J. 106, 753–760. doi: 10.2134/agronj2013.0043
Byrnes, R. C., Nùñez, J., Arenas, L., Rao, I., Trujillo, C., Alvarez, C., et al. (2017). Biological nitrification inhibition by Brachiaria grasses mitigates soil nitrous oxide emissions from bovine urine patches. Soil Biol. Biochem. 107, 156–163. doi: 10.1016/j.soilbio.2016.12.029
Chantigny, M. H., Pelster, D. E., Perron, M. H., Rochette, P., Angers, D. A., Parent, L. É., et al. (2013). Nitrous oxide emissions from clayey soils amended with paper sludges and biosolids of separated pig slurry. J. Environ. Qual. 42, 30–39. doi: 10.2134/jeq2012.0196
Chen, Z., Ding, W., Luo, Y., Yu, H., Xu, Y., Müller, C., et al. (2014). Nitrous oxide emissions from cultivated black soil: a case study in Northeast China and global estimates using empirical model. Glob. Biogeochem. Cy. 28, 1311–1326. doi: 10.1002/2014GB004871
Coskun, D., Britto, D. T., Shi, W., and Kronzucker, H. J. (2017a). Nitrogen transformations in modern agriculture and the role of biological nitrification inhibition. Nat. Plants 3:17074. doi: 10.1038/nplants.2017.74
Coskun, D., Britto, D. T., Shi, W., and Kronzucker, H. J. (2017b). How plant root exudates shape the nitrogen cycle. Trends Plant Sci. 22, 661–673. doi: 10.1016/j.tplants.2017.05.004
Dangal, S. R. S., Tian, H., Xu, R., Chang, J., Canadell, J. G., Ciais, P., et al. (2019). Global nitrous oxide emissions from pasturelands and rangelands: magnitude, spatiotemporal patterns, and attribution. Glob. Biogeochem. Cy. 33, 200–222. doi: 10.1029/2018GB006091
Dobbie, K. E., and Smith, K. A. (2003). Impact of different forms of N fertilizer on N2O emissions from intensive grassland. Nutr. Cycl. Agroecosyst. 67, 37–46. doi: 10.1023/A:1025119512447
Fisher, M. J., Rao, I. M., Ayarza, M. A., Lascano, C. E., Sanz, J. I., Thomas, R. J., et al. (1994). Carbon storage by introduced deep-rooted grasses in the south American savannas. Nature 371, 236–238. doi: 10.1038/371236a0
Foley, J. A., Ramankutty, N., Brauman, K. A., Cassidy, E. S., Gerber, J. S., Johnston, M., et al. (2011). Solutions for a cultivated planet. Nature 478, 337–342. doi: 10.1038/nature10452
Gopalakrishnan, S., Watanabe, T., Pearse, S. J., Ito, O., Hossain, A. K. M., and Subbarao, G. V. (2009). Biological nitrification inhibition by Brachiaria humidicola roots varies with soil type and inhibits nitrifying bacteria, but not other major soil microorganisms. Soil Sci. Plant Nutr. 55, 725–733. doi: 10.1111/j.1747-0765.2009.00398.x
Grassini, P., and Cassman, K. G. (2012). High-yield maize with large net energy yield and small global warming intensity. Proc. Natl. Acad. Sci. U. S. A. 109, 1074–1079. doi: 10.1073/pnas.1201296109
Han, M., Okamoto, M., Beatty, P. H., Rothstein, S. J., and Good, A. G. (2015). The genetics of nitrogen use efficiency in crop plants. Annu. Rev. Genet. 49, 269–289. doi: 10.1146/annurev-genet-112414-055037
Hink, L., Gubry-Rangin, C., Nicol, G. W., and Prosser, J. I. (2018). The consequences of niche and physiological differentiation of archaeal and bacterial ammonia oxidisers for nitrous oxide emissions. ISME J. 12, 1084–1093. doi: 10.1038/s41396-017-0025-5
Horrocks, C. A., Arango, J., Arevalo, A., Nuñez, J., Cardoso, J. A., and Dungait, J. A. J. (2019). Smart forage selection could significantly improve soil health in the tropics. Sci. Total Environ. 688, 609–621. doi: 10.1016/j.scitotenv.2019.06.152
IPCC (2013). Climate change 2013: The physical science basis. Contribution of working group I to the fifth assessment report of the intergovernmental panel on climate change. Cambridge University.
Karwat, H., Moreta, D., Arango, J., Núñez, J., Rao, I., Rincón, Á., et al. (2017). Residual effect of BNI by Brachiaria humidicola pasture on nitrogen recovery and grain yield of subsequent maize. Plant Soil 420, 389–406. doi: 10.1007/s11104-017-3381-z
Lan, X., Thoning, K.W., and Dlugokencky, E.J. (2022). Trends in globally-averaged CH4, N2O, and SF6 determined from NOAA global monitoring laboratory measurements. Version 2023–2001.
Lata, J. C., Durand, J., Lensi, R., and Abbadie, L. (1999). Stable coexistence of contrasted nitrification statuses in a wet tropical savanna ecosystem. Funct. Ecol. 13, 762–768. doi: 10.1046/j.1365-2435.1999.00380.x
Lata, J. C. C., Le Roux, X., Koffi, K. F., Ye, L., Srikanthasamy, T., Konare, S., et al. (2022). The causes of the selection of biological nitrification inhibition (BNI) in relation to ecosystem functioning and a research agenda to explore them. Biol. Fertil. Soils 58, 207–224. doi: 10.1007/s00374-022-01630-3
Liu, X., Zhang, Y., Han, W., Tang, A., Shen, J., Cui, Z., et al. (2013). Enhanced nitrogen deposition over China. Nature 494, 459–462. doi: 10.1038/nature11917
Lombardi, B., Loaiza, S., Trujillo, C., Arevalo, A., Vázquez, E., Arango, J., et al. (2022). Greenhouse gas emissions from cattle dung depositions in two Urochloa forage fields with contrasting biological nitrification inhibition (BNI) capacity. Geoderma 406:115516. doi: 10.1016/j.geoderma.2021.115516
Lu, R. (2000). Analytical Methods for Soil and Agro-Chemistry. Beijing: China Agricultural Science and Technology Press
Lu, Y. F., Zhang, X. N., Jiang, J. F., Kronzucker, H. J., Shen, W. S., and Shi, W. M. (2019). Effects of the biological nitrification inhibitor 1,9-decanediol on nitrification and ammonia oxidizers in three agricultural soils. Soil Biol. Biochem. 129, 48–59. doi: 10.1016/j.soilbio.2018.11.008
Luo, J., Wyatt, J., van der Weerden, T. J., Thomas, S. M., de Klein, C. A. M., Li, Y., et al. (2017). Potential hotspot areas of nitrous oxide emissions from grazed pastoral dairy farm systems. Adv. Agron. 145, 205–268. doi: 10.1016/bs.agron.2017.05.006
Marschner, P. (2011). Marschner’s Mineral Nutrition of Higher Plants (3rd Edn). London, UK: Elsevier, 651
Merbold, L., Eugster, W., Stieger, J., Zahniser, M., Nelson, D., and Buchmann, N. (2014). Greenhouse gas budget (CO2, CH4 and N2O) of intensively managed grassland following restoration. Glob. Chang. Biol. 20, 1913–1928. doi: 10.1111/gcb.12518
Mosier, A. R., Duxbury, J. M., Freney, J. R., Heinemeyer, O., and Minami, K. (1996). Nitrous oxide emissions from agricultural fields: assessment, measurement and mitigation. Plant Soil 181, 95–108. doi: 10.1007/BF00011296
Nardi, P., Akutsu, M., Pariasca-Tanaka, J., and Wissuwa, M. (2013). Effect of methyl 3-4-hydroxyphenyl propionate, a sorghum root exudate, on N dynamic, potential nitrification activity and abundance of ammonia-oxidizing bacteria and archaea. Plant Soil 367, 627–637. doi: 10.1007/s11104-012-1494-y
Nardi, P., Laanbroek, H. J., Nicol, G. W., Renella, G., Cardinale, M., Pietramellara, G., et al. (2020). Biological nitrification inhibition in the rhizosphere: determining interactions and impact on microbially mediated processes and potential applications. FEMS Microbiol. Rev. 44, 874–908. doi: 10.1093/femsre/fuaa037
Niu, Y., Luo, J., Liu, D., Müller, C., Zaman, M., Lindsey, S., et al. (2018). Effect of biochar and nitrapyrin on nitrous oxide and nitric oxide emissions from a sandy loam soil cropped to maize. Biol. Fertil. Soils 54, 645–658. doi: 10.1007/s00374-018-1289-2
Nuñez, J., Arevalo, A., Karwat, H., Egenolf, K., Miles, J., Chirinda, N., et al. (2018). Biological nitrification inhibition activity in a soil-grown biparental population of the forage grass, Brachiaria humidicola. Plant Soil 426, 401–411. doi: 10.1007/s11104-018-3626-5
Poudel, D. D., Horwath, W. R., Lanini, W. T., Temple, S. R., and Van Bruggen, A. H. C. (2002). Comparison of soil N availability and leaching potential, crop yields and weeds in organic, low-input and conventional farming systems in northern California. Agric. Ecosyst. Environ. 90, 125–137. doi: 10.1016/S0167-8809(01)00196-7
Ravishankara, A. R., Daniel, J. S., and Portmann, R. W. (2009). Nitrous oxide (N2O): the dominant ozone-depleting substance emitted in the 21st century. Science 326, 123–125. doi: 10.1126/science.1176985
Rochette, P., van Bochove, E., Prévost, D., Angers, D. A., Coté, D., and Bertrand, N. (2000). Soil carbon and nitrogen dynamics following application of pig slurry for the 19th consecutive year. II. Nitrous oxide fluxes and mineral nitrogen. Soil Sci. Soc. Am. J. 64, 1396–1403. doi: 10.2136/sssaj2000.6441396x
Rodgers, G. A. (1986). Nitrification inhibitors in agriculture. J. Environ. Sci. Health Part A 21, 701–722. doi: 10.1080/10934528609375320
Stein, L. Y. (2020). The long-term relationship between microbial metabolism and greenhouse gases. Trends Microbiol. 28, 500–511. doi: 10.1016/j.tim.2020.01.006
Subbarao, G. V., Ito, O., Sahrawat, K. L., Berry, W. L., Nakahara, K., Ishikawa, T., et al. (2006). Scope and strategies for regulation of nitrification in agricultural systems - challenges and opportunities. Crit. Rev. Plant Sci. 25, 303–335. doi: 10.1080/07352680600794232
Subbarao, G. V., Nakahara, K., Hurtado, M. P., Ono, H., Moreta, D. E., Salcedo, A. F., et al. (2009). Evidence for biological nitrification inhibition in Brachiaria pastures. Proc. Natl. Acad. Sci. U. S. A. 106, 17302–17307. doi: 10.1073/pnas.0903694106
Subbarao, G. V., Nakahara, K., Ishikawa, T., Ono, H., Yoshida, M., Yoshihashi, T., et al. (2013). Biological nitrification inhibition (BNI) activity in sorghum and its characterization. Plant Soil 366, 243–259. doi: 10.1007/s11104-012-1419-9
Subbarao, G. V., Rondon, M., Ito, O., Ishikawa, T., Rao, I. M., Nakahara, K., et al. (2007). Biological nitrification inhibition (BNI) - is it a widespread phenomenon? Plant Soil 294, 5–18. doi: 10.1007/s11104-006-9159-3
Subbarao, G. V., Sahrawat, K. L., Nakahara, K., Ishikawa, T., Kishii, M., Rao, I. M., et al. (2012). Biological nitrification inhibition-a novel strategy to regulate nitrification in agricultural systems. Adv. Agron. 114, 249–301. doi: 10.1016/B978-0-12-394275-3.00001-8
Subbarao, G. V., Yoshihashi, T., Worthington, M., Nakahara, K., Ando, Y., Sahrawat, K. L., et al. (2015). Suppression of soil nitrification by plants. Plant Sci. 233, 155–164. doi: 10.1016/j.plantsci.2015.01.012
Sun, L., Lu, Y. F., Yu, F. W., Kronzucker, H. J., and Shi, W. M. (2016). Biological nitrification inhibition by rice root exudates and its relationship with nitrogen-use efficiency. New Phytol. 212, 646–656. doi: 10.1111/nph.14057
Teutscherová, N., Vázquez, E., Trubač, J., Villegas, D. M., Subbarao, G. V., Pulleman, M., et al. (2022). Gross N transformation rates in soil system with contrasting Urochloa genotypes do not confirm the relevance of BNI as previously assessed in vitro. Biol. Fertil. Soils 58, 321–331. doi: 10.1007/s00374-021-01610-z
Van Groenigen, J. W., Kasper, G. J., Velthof, G. L., Van Den Pol-Van Dasselaar, A., and Kuikman, P. J. (2004). Nitrous oxide emissions from silage maize fields under different mineral nitrogen fertilizer and slurry applications. Plant Soil 263, 101–111. doi: 10.1023/B:PLSO.0000047729.43185.46
van Groenigen, J. W., Velthof, G. L., Oenema, O., Van Groenigen, K. J., and Van Kessel, C. (2010). Towards an agronomic assessment of N2O emissions: a case study for arable crops. Eur. J. Soil Sci. 61, 903–913. doi: 10.1111/j.1365-2389.2009.01217.x
Venterea, R. T., Bijesh, M., and Dolan, M. S. (2011). Fertilizer source and tillage effects on yield-scaled nitrous oxide emissions in a corn cropping system. J. Environ. Qual. 40, 1521–1531. doi: 10.2134/jeq2011.0039
Walkley, A., and Black, I. A. (1934). An examination of Degtjareff method for determining soil organic matter and a proposed modification of the chromic acid titration method. Soil Sci. 37, 29–38. doi: 10.1097/00010694-193401000-00003
Wang, X., Bai, J., Xie, T., Wang, W., Zhang, G., Yin, S., et al. (2021). Effects of biological nitrification inhibitors on nitrogen use efficiency and greenhouse gas emissions in agricultural soils: a review. Ecotoxicol. Environ. Saf. 220:112338. doi: 10.1016/j.ecoenv.2021.112338
Weiske, A., Benckiser, G., and Ottow, J. C. G. (2001). Effect of the new nitrification inhibitor DMPP in comparison to DCD on nitrous oxide (N2O) emissions and methane (CH4) oxidation during 3 years of repeated applications in field experiments. Nutr. Cycl. Agroecosyst. 60, 57–64. doi: 10.1023/a:1012669500547
Wolf, B., Zheng, X., Brüggemann, N., Chen, W., Dannenmann, M., Han, X., et al. (2010). Grazing-induced reduction of natural nitrous oxide release from continental steppe. Nature 464, 881–884. doi: 10.1038/nature08931
Wu, D., Senbayram, M., Well, R., Brüggemann, N., Pfeiffer, B., Loick, N., et al. (2017). Nitrification inhibitors mitigate N2O emissions more effectively under straw-induced conditions favoring denitrification. Soil Biol. Biochem. 104, 197–207. doi: 10.1016/j.soilbio.2016.10.022
Xie, L., Liu, D., Müller, C., Jansen-Willems, A., Chen, Z., Niu, Y., et al. (2022). Brachiaria humidicola cultivation enhances soil nitrous oxide emissions from tropical grassland by promoting the denitrification potential: a 15N tracing study. Agriculture 12:1940. doi: 10.3390/agriculture12111940
Ye, G., Lin, Y., Liu, D., Chen, Z., Luo, J., Bolan, N., et al. (2019). Long-term application of manure over plant residues mitigates acidification, builds soil organic carbon and shifts prokaryotic diversity in acidic Ultisols. Appl. Soil Ecol. 133, 24–33. doi: 10.1016/j.apsoil.2018.09.008
Zakir, H. A. K. M. K. M. A. K. M., Subbarao, G. V. V., Pearse, S. J. J., Gopalakrishnan, S., Ito, O., Ishikawa, T., et al. (2008). Detection, isolation and characterization of a root-exuded compound, methyl 3-(4-hydroxyphenyl) propionate, responsible for biological nitrification inhibition by sorghum (Sorghum bicolor). New Phytol. 180, 442–451. doi: 10.1111/j.1469-8137.2008.02576.x
Zerulla, W., Barth, T., Dressel, J., Erhardt, K., von Locquenghien, K. H., Pasda, G., et al. (2001). 3, 4-Dimethylpyrazole phosphate (DMPP)–a new nitrification inhibitor for agriculture and horticulture. Biol. Fertil. Soils 34, 79–84. doi: 10.1007/s003740100380
Keywords: biological nitrification inhibition, Brachiaria humidicola, denitrification, N2O emissions, yield-scaled N2O emission
Citation: Xie L, Liu D, Chen Z, Niu Y, Meng L and Ding W (2023) Non-native Brachiaria humidicola with biological nitrification inhibition capacity stimulates in situ grassland N2O emissions. Front. Microbiol. 14:1127179. doi: 10.3389/fmicb.2023.1127179
Edited by:
Upendra Kumar, National Rice Research Institute (ICAR), IndiaReviewed by:
Rui Liu, China Agricultural University, ChinaM. Manjunath, Central Research Institute for Dryland Agriculture (ICAR), India
Copyright © 2023 Xie, Liu, Chen, Niu, Meng and Ding. This is an open-access article distributed under the terms of the Creative Commons Attribution License (CC BY). The use, distribution or reproduction in other forums is permitted, provided the original author(s) and the copyright owner(s) are credited and that the original publication in this journal is cited, in accordance with accepted academic practice. No use, distribution or reproduction is permitted which does not comply with these terms.
*Correspondence: Weixin Ding, wxding@issas.ac.cn