- 1MATIS, Department of Research and Innovation, Reykjavík, Iceland
- 2Department of Earth Sciences, University of Hawai’i at Mānoa, Honolulu, HI, United States
- 3Icelandic Meteorological Office, Reykjavík, Iceland
- 4Faculty of Food Science and Nutrition, University of Iceland, Reykjavík, Iceland
Skaftárkatlar are two subglacial lakes located beneath the Vatnajökull ice cap in Iceland associated with geothermal and volcanic activity. Previous studies of these lakes with ribosomal gene (16S rDNA) tag sequencing revealed a limited diversity of bacteria adapted to cold, dark, and nutrient-poor waters. In this study, we present analyses of metagenomes from the lake which give new insights into its microbial ecology. Analyses of the 16S rDNA genes in the metagenomes confirmed the existence of a low-diversity core microbial assemblage in the lake and insights into the potential metabolisms of the dominant members. Seven taxonomic genera, Sulfuricurvum, Sulfurospirillum, Acetobacterium, Pelobacter/Geobacter, Saccharibacteria, Caldisericum, and an unclassified member of Prolixibacteraceae, comprised more than 98% of the rDNA reads in the library. Functional characterisation of the lake metagenomes revealed complete metabolic pathways for sulphur cycling, nitrogen metabolism, carbon fixation via the reverse Krebs cycle, and acetogenesis. These results show that chemolithoautotrophy constitutes the main metabolism in this subglacial ecosystem. This assemblage and its metabolisms are not reflected in enrichment cultures, demonstrating the importance of in situ investigations of this environment.
1. Introduction
Subglacial lakes can form where water collects at hydrostatic stable points beneath an ice sheet, when geothermal heat, pressure-induced freezing point depression, and/or high salinity prevent complete freezing (Livingstone et al., 2022). These lakes are relatively isolated ecosystems that can host life despite low temperatures, low nutrient abundance, and the absence of sunlight as an energy source. Terrestrial subglacial lakes are considered accessible if imperfect, analogues to ice-covered environments in the past and present Solar system: the ice-covered oceans of “Snowball” Earth (Kirschvink et al., 2000), lakes beneath the south polar cap of Mars (Lauro et al., 2020) and oceans in some of the icy satellites of Jupiter and Saturn (Kivelson et al., 2000, 2002; Thomas et al., 2016). Studies of these systems complement research on other lakes with comparatively thin (metres) ice covers, e.g., the Dry Valleys lakes (Chinn, 1993) and epishelf lakes of Antarctica (Davies et al., 2017), where sufficient photosynthetic active radiation can reach the water column and support phototrophic communities (Howard-Williams et al., 1998).
There are more than 700 reported subglacial lakes in Antarctica (Livingstone et al., 2022), three in Iceland under the Vatnajökull glacier (Bjornsson, 2003), two in Greenland (Palmer et al., 2013), and one recently discovered in the Canadian Arctic (Rutishauser et al., 2018). The biological exploration of some of these lakes has begun, namely Lake Vostok (Karl et al., 1999; Priscu et al., 1999; Christner et al., 2001; Bulat, 2016; Gura and Rogers, 2020), Subglacial Lake Whillans (Tulaczyk et al., 2014), Subglacial Lake Mercer in Antarctica (Priscu et al., 2021), and all three Icelandic lakes (Gaidos et al., 2004, 2009; Marteinsson et al., 2013). In the cases of Antarctica and Iceland, the data unambiguously point to the presence of active assemblages of bacterial taxa which are distinct from the distribution in the overlying ice or surrounding glaciated terrain (Gaidos et al., 2004, 2009; Marteinsson et al., 2013; Achberger et al., 2016).
The three main Icelandic lakes, called Grímsvötn, Western, and Eastern Skaftárkatlar lakes, are distinguished by their location on active volcanoes and are maintained by geothermal melting at the base of the 250–300-m thick Vatnajökull ice cap. The chemistry of the approximately 100-m-deep water columns of these lakes is substantially influenced by volcanic gases and hydrothermal fluids, maintaining an anoxic and highly sulphidic environment (Agustsdottir and Brantley, 1994; Johannesson et al., 2007). Mixing of anoxic lake water with oxygenated glacial meltwater creates chemical disequilibrium that can serve as an energy source for chemolithotrophic microorganisms (Gaidos et al., 2004, 2009; Marteinsson et al., 2013). Previous investigations have used molecular tag-based methods to identify the dominant microbial taxa in the water column of the Skaftárkatlar lakes and link chemical reactions of the inorganic substrates thought present in the lakes with potential metabolisms of these taxa, e.g., acetogenesis, sulphide oxidation, sulphate reduction, iron reduction, and hydrogen oxidation by members of Acetobacterium, Geobacter, Sulfuricurvum, Sulfurospirillum, and Desulfosporosinus (Gaidos et al., 2009; Marteinsson et al., 2013). In Subglacial Lake Whillans, the chemoautotrophic microbial taxa, distinct from that taxa of the Icelandic subglacial lakes, use reduced nitrogen, iron or sulphur compounds as energy sources (Christner et al., 2014).
Here, we performed a metagenomic analysis on four water column samples collected from the Eastern Skaftárkatlar lake in 2007, as well as on enrichment cultures from the same samples (Marteinsson et al., 2013). Our study aimed to: (1) confirm the previously reported microbial community structure, (2) investigate the metabolic strategies of the microorganisms in the lake, and (3) analyse potential pathways within microbes grown in enrichment cultures under different conditions. Compared to the previous study done on the same water samples but only with a 16S rRNA sequencing approach, we adopted a metagenomic approach as a more informative, less biassed method to gain further understanding of the microbial diversity and community structure but also and most importantly of the functional capabilities both at the taxon and community level occurring in such a specific environment. Our analyses show unambiguously that the microbial communities of this Icelandic subglacial lake are dominated by seven taxonomic genera: Sulfuricurvum, Sulfurospirillum, Acetobacterium, Pelobacter/Geobacter, Saccharibacteria, Caldisericum, and an unclassified Prolixibacteraceae. Chemolithoautotrophy is the main metabolism of these communities and is adapted to their environment with oxidation/reduction of sulphur, nitrogen metabolism, carbon fixation via the reverse Krebs cycle, and acetogenesis.
2. Materials and methods
2.1. Environmental samples
Water column samples were collected from the East Skaftárkatlar lake, in June 2007 as described by Marteinsson et al. (2013). Two boreholes A and B were drilled through the overlying 280-m-thick ice sheet with a sterilising hot water drill (Thorsteinsson et al., 2008). Each collected sample was about 1 L and the results of chemical analyses of the samples (Marteinsson et al., 2013) are reported in Supplementary Table 1. As B1 and B4 samples appeared chemically homogeneous (Supplementary Table 1), a pool of the B1 to B4 samples was created, Bmix, to increase total DNA yield.
2.2. Enrichment samples
The Emix sample consists of biomass pooled from enrichment cultures that showed growth as previously described (Marteinsson et al., 2013). Media were designed to cultivate chemolithotrophs and chemo-organotrophs at 4, 60, and 80°C. Briefly, different enrichment media were used and inoculated with 1% of water lake samples under anaerobic conditions. Sample of 0.22 μm micron-filtered lake water was used as a medium for enrichment cultures labelled “WN2,” “WO2,” “C-J,” and “A-J” for respectively “Water N2,” “Water O2,” “Enrichment C-jökull” (meaning glacier in Icelandic), and “Enrichment A-jökull.” A supplement yeast extract solution (0.01%), vitamin solution, Balch element solution (Balch et al., 1979), S0, and resazurin were added to WN2 and WO2 enrichment cultures. WO2 was used aerobically whereas WN2 was incubated with pure N2 and supplemented with 0.025% final wt v–1 Na2S.9H2O. A volume of sterile water was supplemented with 1X of yeast-acetate medium (Gaidos et al., 2009) and used as media for C-J and A-J. C-J was incubated with 80/20% H2/CO2 and 0.025% final wt. v–1 Na2S.9H2O whereas A-J was incubated aerobically. Finally, enrichment cultures were done aerobically with 162 Thermus medium (Degryse et al., 1978) and Reasoner’s 2A (R2A) medium (Reasoner and Geldreich, 1985) whereas Thermotoga and Yeast Peptone Sulphur (YPS) media were used anaerobically with pure N2 (Marteinsson et al., 1997, 2001). Pellets of cells from these enrichments were obtained by centrifugation at 8,000 rpm for 25 min and used for DNA extraction.
2.3. DNA extraction and sequencing
DNA was extracted from filtered water samples and enrichment cultures as previously described (Gaidos et al., 2009). Three different DNA samples were sequenced: A3, Bmix, and Emix (Table 1). The DNA was sent to the Marine Biological Laboratory at the Woods Hole Institute for sequencing on an Illumina HiSeq (Illumina, Inc., CA, USA) as part of the Census of Deep Life of the Deep Carbon Observatory. DNA was sheared using a Covaris S2 sonicator (Covaris, Woburn, MA, USA) and libraries were constructed with the Nugen Ovation Ultralow Library protocol (NuGEN Technologies, San Carlos, CA, USA). Expected insert size of 175 bp enabled overlapping reads. Amplified libraries were visualised on an Agilent DNA1000 chip (Agilent, Santa Clara, CA, USA) or Caliper HiSens Bioanalyzer assay (Perkin Elmer, Waltham MA, USA), pooled at equimolar concentrations and size selected using a Sage PippinPrep 2% cassette (Sage Science, Beverly, MA, USA). The library pool was quantified using a Kapa Biosystems qPCR library quantification kit (Kapa Biosystems, Wilmington, MA, USA), then sequenced on the HiSeq1000 (Illumina) in a 2 × 108 bp paired-end sequencing run using dedicated read indexing. The samples were then demultiplexed (barcode-based sorting of sequences from different samples) with CASAVA (v.1.8.2; Illumina) while removing the Illumina adaptors. Reads were then merged using FLASH v1.2.11 (Magoč and Salzberg, 2011) with default parameters. Short reads and low-quality bases were then removed using the clc_quality_trim command in CLC (v4.4.0.122465; options used: minlength 90, badfraction 0, i.e., no low-quality nucleotides allowed; CLC Bio, Aarhus, Denmark).
2.4. Biodiversity analysis based on rDNA miTAGs
Reads corresponding to rDNA genes, a.k.a. miTAGs for metagenome Illumina tags (Logares et al., 2014), were extracted from the metagenomes using Hidden Markov Models (HMM) in Meta-RNA (Huang et al., 2009). The reads were assembled on Geneious (R10, Biomatters, Auckland, New Zealand) with conservative settings (“Fastest,” <1% mismatch, otherwise default parameters) to avoid chimeric assembly. Using iterative mappings of the rest of the reads with Geneious (Biomatters), the contigs were manually extended and assembled-when the coverage was sufficient- into complete ribosomal operons containing the 16S, 23S, and 5S rDNA genes and homogeneous coverage, allowing more reliable taxonomic assignments than with partial or single genes. These assembled rDNA genes were then annotated using either BLASTN+ against public databases NT and SILVA (Camacho et al., 2009; Quast et al., 2012) or the online RDP classifier (Wang et al., 2007). Most contigs get a significant assignment (>98% 16S identity) with the notable exception among the dominant taxa of Caldisericum sp. (96% identity against the closest 16S barcode and only 83% identity against the closest genome of Caldisericum exile). The rDNA genes were then aligned and trimmed to about 1,819; 3,731, and 115 bp (for 16S, 23S, and 5S, respectively) and used as references to recruit the ribosomal fraction of the raw metagenomic reads with high stringency (≥50% alignment coverage and ≥98% identity). From this point, we assumed that each assemblage represents a distinct taxon. To better reflect the actual community structure, read counts per taxon were then normalised based on actual gene length and the number of copies of the operon in each taxon. The estimation of the copy number of each ribosomal operon was obtained from ribosomal RNA operon copy number database (rrnDB) (Stoddard et al., 2014) by rounding the average number of rDNA operon copies at the taxonomic level retained for each operon (see Table 2).
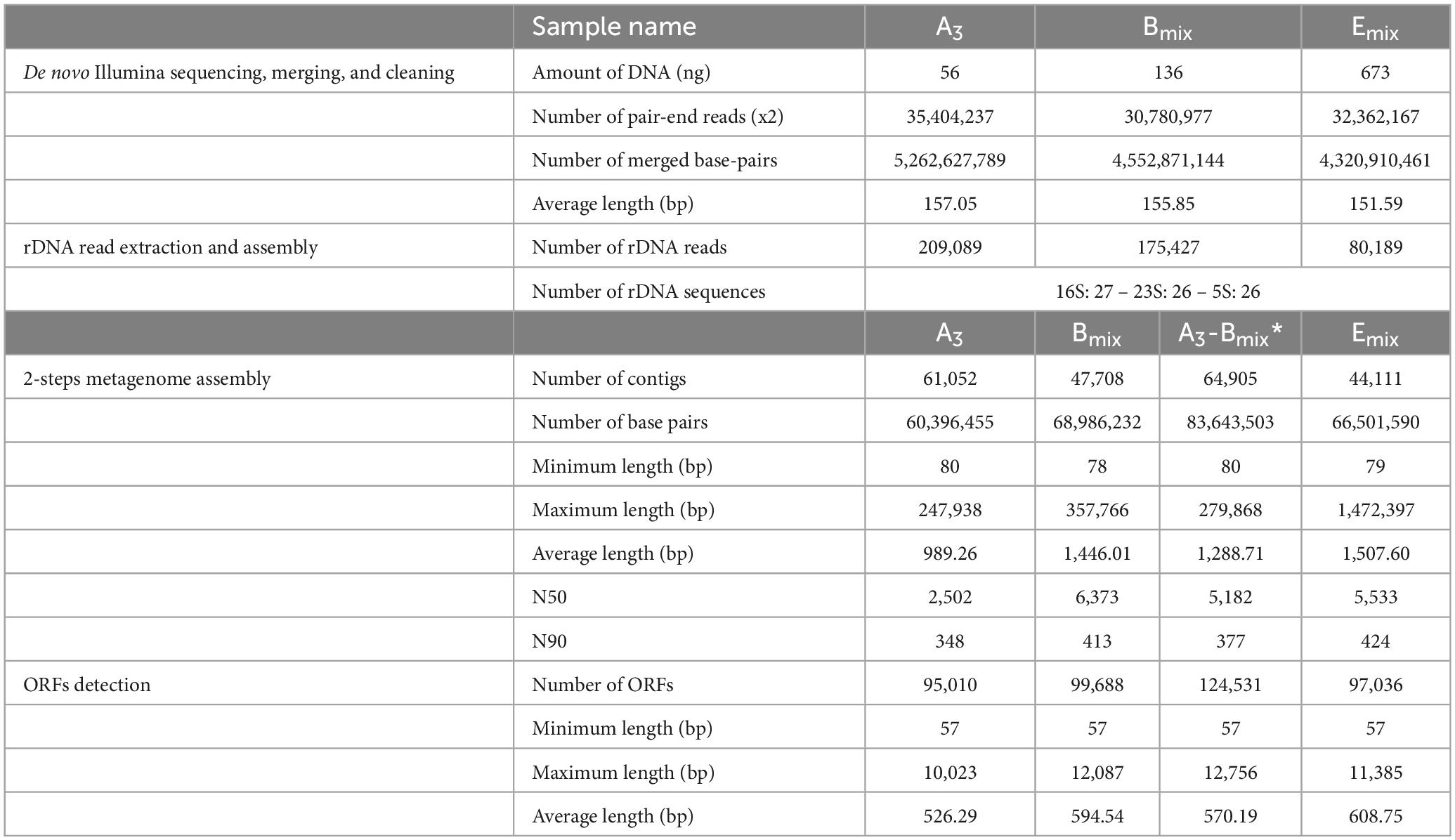
Table 2. Genetic materials of East Skaftárkatlar lake and associated analyses used in this study (*A3-Bmix co-assembly).
Additionally, binning experiments based on nucleotide composition, GC%, and differential coverage in A3, Bmix, and Emix [CONCOCT (Alneberg et al., 2014)] were tried and failed at properly separating some closely related taxa, partly due to an overall low sequencing depth and lack of variation in the taxonomic profile of available samples.
2.5. Microbial metagenome, functional potential
With regards to their comparable community composition shown through the rDNA miTAG analyses (see section “Results” and Figure 1), the raw metagenomes A3 and Bmix were co-assembled de novo to improve the functional analyses. At first, default configurations of IDBA-UD (Peng et al., 2012) and SPAdes (Bankevich et al., 2012) were run to generate reliable contigs. The resulting contigs were then assembled into “supercontigs” using Geneious (Biomatters; default “Fastest” with 2% mismatch allowed). These “supercontigs” and the other unassembled original contigs were used for the functional exploration of the water column metagenome. MetaGeneMark (Zhu et al., 2010) was used online with default parameters to detect open reading frames (ORFs) even truncated at the edge of contigs. Function and taxonomy were then assigned to these genes by amino-acid alignment against the Kyoto Encyclopedia of Genes and Genomes database (KEGG, FTP Release 12-02-2018) (Kanehisa and Goto, 2000; Kanehisa et al., 2015) using DIAMOND (with 60% identity cut-off) (Buchfink et al., 2015) and their abundance in the metagenome was estimated by recruiting the raw metagenomics reads using BLASTN (Camacho et al., 2009). The results were manually checked and when 80% of the genes involved in a pathway were detected, the pathways were considered as present. Errors in the taxonomic assignments of the ORFs are generally caused by low taxonomic resolution or the absence of close relatives in the reference dataset and may result on genes being assigned to close relatives of the represented diversity. These were reduced by collapsing branches of the trees using TaxonomyCollapsor.1 This algorithm sorts the leaves on the phylogenetic trees according to decreasing abundance (as read counts) and flags as significant those for which the cumulated abundance contains 95% of the total abundance. Leaves that do not reach the significance criteria are then reassigned by order of priority to their closest significant relative of the same rank or at a higher taxonomical level.
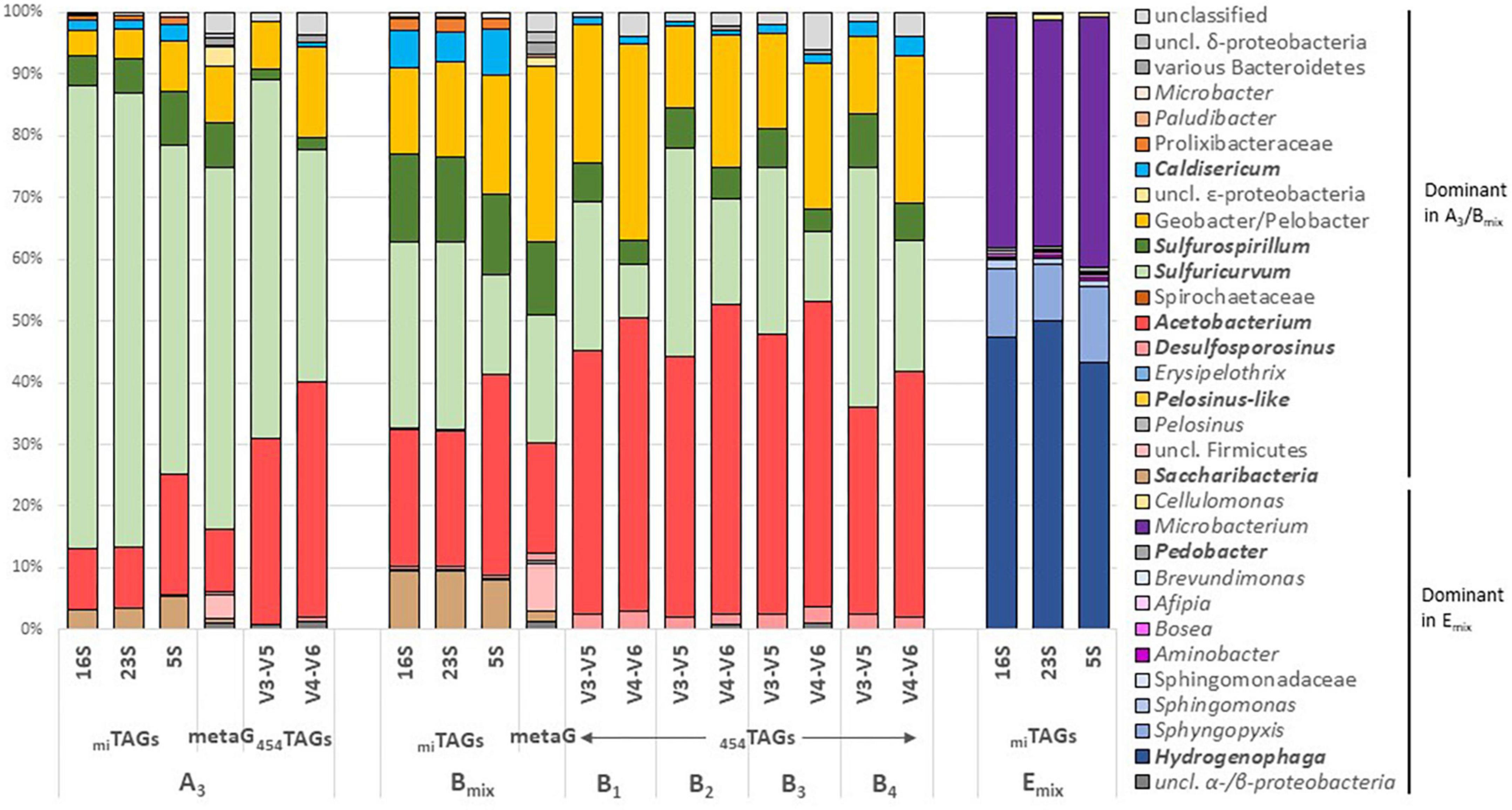
Figure 1. Community structure of metagenomes A3, Bmix, and Emix from East Skaftárkatlar lake based on metagenome extracted rDNA miTAGs. Assignments were performed using BLASTN against SILVA 128 (Quast et al., 2012; Yilmaz et al., 2013).
3. Results
3.1. Metagenome sequence yield
DNA was extracted and sequenced from samples collected from the bottom of the lake (sample A3), from a mixture of four water column samples collected at different depths (sample Bmix), and from enrichments grown under various culture conditions (sample Emix) (Tables 1, 2). The metagenomes A3 and Bmix allow us to investigate the microbial community of the lake water column whereas Emix permits to identify the growing organisms that might be rare in the environment but can be enriched under laboratory conditions. The sequencing of all the DNA available for A3, Bmix, and Emix resulted in 30–35 × 106 overlapping paired reads per sample with respectively an average merged length of 157.05 bp (σ = 15.65 bp), 155.85 bp (σ = 19.84 bp), and 151.59 bp (σ = 18.96 bp) (Table 2).
3.2. Diversity and community structure based on ribosomal DNA sequences
Hidden Markov Models were used to identify 209,089, 175,427, and 80,189 rDNA reads in the A3, Bmix, and Emix libraries, respectively (Table 2). Conservative de novo assembly and manual improvements of these assemblies yielded 25 distinct high-quality full-length rDNA operons (each consisting of 5S, 16S, and 23S genes and intergenic regions) and 4 partial operons (missing all or part of a rDNA gene) (Table 3 and Supplementary Table 2). The libraries sequenced from the natural samples A3 and Bmix both contain seven taxa with relative read abundance >1%, namely Acetobacterium sp., Sulfuricurvum sp., Sulfurospirillum sp., Geobacter sp./Pelobacter sp., Caldisericum sp., Saccharibacteria sp., and an unclassified Prolixibacteraceae. Together, those taxa recruit 98.0 to 99.2% of the total rDNA reads in A3 and Bmix, respectively. Based on 16S-rDNA miTAGs, Shannon’s α-diversity is 1.18 and 1.83 for A3 and Bmix, respectively, while evenness is 0.14 and 0.33, respectively. While the A3 metagenome is dominated by Sulfuricurvum sp. (74% of the 16S reads), followed by Acetobacterium (16%), Bmix seems to be more evenly distributed, consisting of Sulfuricurvum (30%), Acetobacterium sp. (22%), Sulfurospirillum (14%) and Geobacter sp./Pelobacter sp. (13%). The community structure observed for the three samples based on 16S-, 23S-, and 5S-rDNA miTAGs [metagenome Illumina tags (Logares et al., 2014)] was compared to that observed in samples A3 and B1–4 obtained by Marteinsson et al. (2013) using 16S rDNA pyrosequencing (Figure 1).
Unlike these first four taxa, which are relatively close to their cultivated relatives (over 97% identity), the lower abundance taxa Caldisericum sp. candidate (1.7% of 16S-rDNA miTAGs in A3, 6.0% in Bmix) and Saccharibacteria sp. (3.3% in A3, 9.5% in Bmix) are more distant from their closest relatives in public databases. The Caldisericum sp. candidate 16S and 23S assembled contigs show an alignment identity of 83% with publicly available C. exile AZM16c01 (Mori et al., 2009). Saccharibacteria sp. rDNA contigs show 94% identity (16S and 23S) with the genome of Candidatus Saccharibacteria bacterium GW2011_GWC2_44_17 (Brown et al., 2015), which belongs to the recently described phylum Saccharibacteria (Ferrari et al., 2014). Other taxa were also found belonging to the Prolixibacteraceae family or the Microbacter genus and to Paludibacter, Desulfosporosinus, Brevundimonas, and Pelosinus in A3. Taxa related to members of Desulfosporosinus, Paludibacter, and Pelosinus were also detected in Bmix as well as Erysipelothrix and Hydrogenophaga. In contrast, about 97% of the miTAGs in Emix were recruited by four full-length ribosomal operons assigned to Hydrogenophaga sp. (49%), Microbacterium sp. (36%), Sphingopyxis sp. (10%), and Sphingomonas sp. (1.2%) (Figure 1 and Table 3).
A dozen different short (about 100 bp) fragments of 16S rDNA genes that showed identity >95% to various archaeal lineages (Crenarchaeota, Euryarchaeota, and Thaumarchaeota) were assembled from A3 and Emix. The corresponding reads originally accounted for about 7% of the raw 16S reads extracted from metagenomes A3 and Emix. The full-length 16S rDNA gene of Euryarchaeota Candidatus Methanoplasma termitum MpT1 (CP010070) was used as a reference to recruit the corresponding reads in A3 (Supplementary Figure 1). Recruited reads do not homogeneously cover the reference gene, instead, all the reads match two overlapping segments of the reference 16S located between positions 850–957 and 891–1,000. Hence, no full-length 16S rDNA belonging to Archaea was detected in the metagenome and those fragments were considered artefacts and discarded from the rDNA pool used for community structure evaluation.
3.3. Metabolic profiling of the subglacial East Skaftárkatlar lake microbiome
The conservative co-assembly of A3 and Bmix resulted in 64,905 contigs (assembly contiguity statistics: N50 = 5,182 bp, N90 = 377 bp) (Table 2). The online MetaGeneMark algorithm detected 124,531 ORFs in these contigs to which we assigned functions and analysed further. Using a 60% amino acid identity cut-off, we identified 58,203 ORFs (47%) having a significant hit against genes from the KEGG (Kanehisa et al., 2015). Those taxa that dominated the miTAGs analyses (Acetobacterium, Sulfuricurvum, Sulfurospirillum, and Geobacter/Pelobacter) also dominated these identified ORFs (Figure 2).
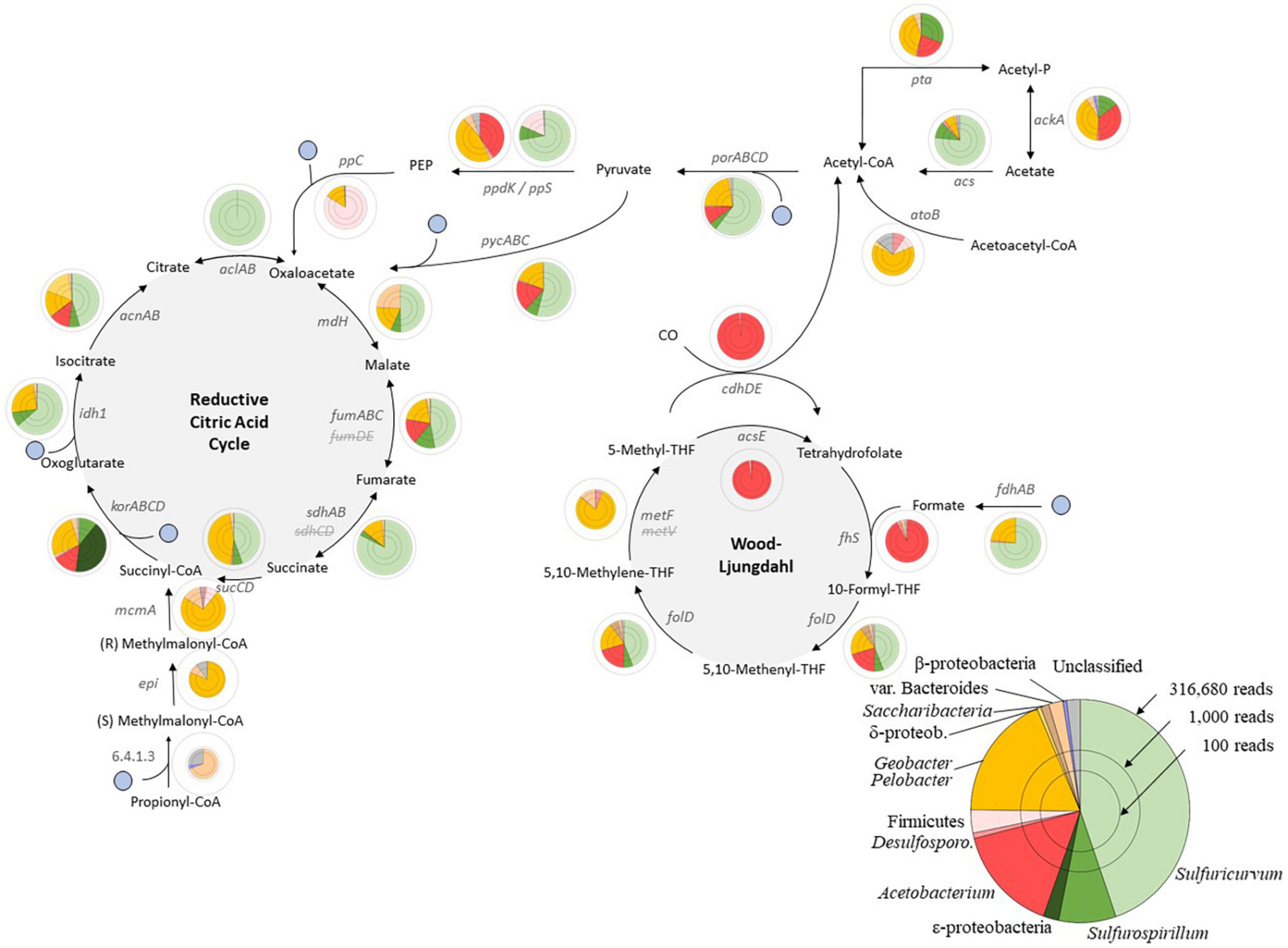
Figure 2. Carbon fixation in East Skaftárkatlar lake and global functional gene recruitment profile. Circular diagrams represent the abundance and taxonomic assignment of the associated reads, with the radius representing the log(nb of reads). CO2/HCO3–.
3.3.1. Carbon fixation
Nearly complete pathways for the reductive citric acid cycle and acetogenesis (Wood-Ljungdahl cycle), both allowing fixation of CO2 or HCO3–, were identified (Figure 2). Sulfuricurvum, Sulfurospirillum, and Geobacter/Pelobacter taxa were found to have most of the genes involved in the reductive citric acid cycle. The genes coding for subunits of fumarate hydratase (fumD and fumE) and succinate dehydrogenase (sdhC and sdhD) were not detected.
3.3.2. Sulphur metabolism
Complete pathways for assimilatory sulphate reduction and dissimilatory reduction of sulphur species were detected with notable taxonomic specificity (Figure 3B). The genes coding for sulphate adenylyltransferase (sat), adenylylsulphate reductase (aprAB), and dissimilatory sulphite reductase (dsrABC) were identified and belong mainly to Desulfosporosinus and Acetobacterium. These enzymes take sulphate to adenosine-5-phosphosulfate (APS), APS to sulphite, and sulphite to sulphide for dissimilatory reduction of sulphate. Thiosulfate might be oxidised to sulphate by Sulfuricurvum via the thiosulfate sulfurtransferase (TST).
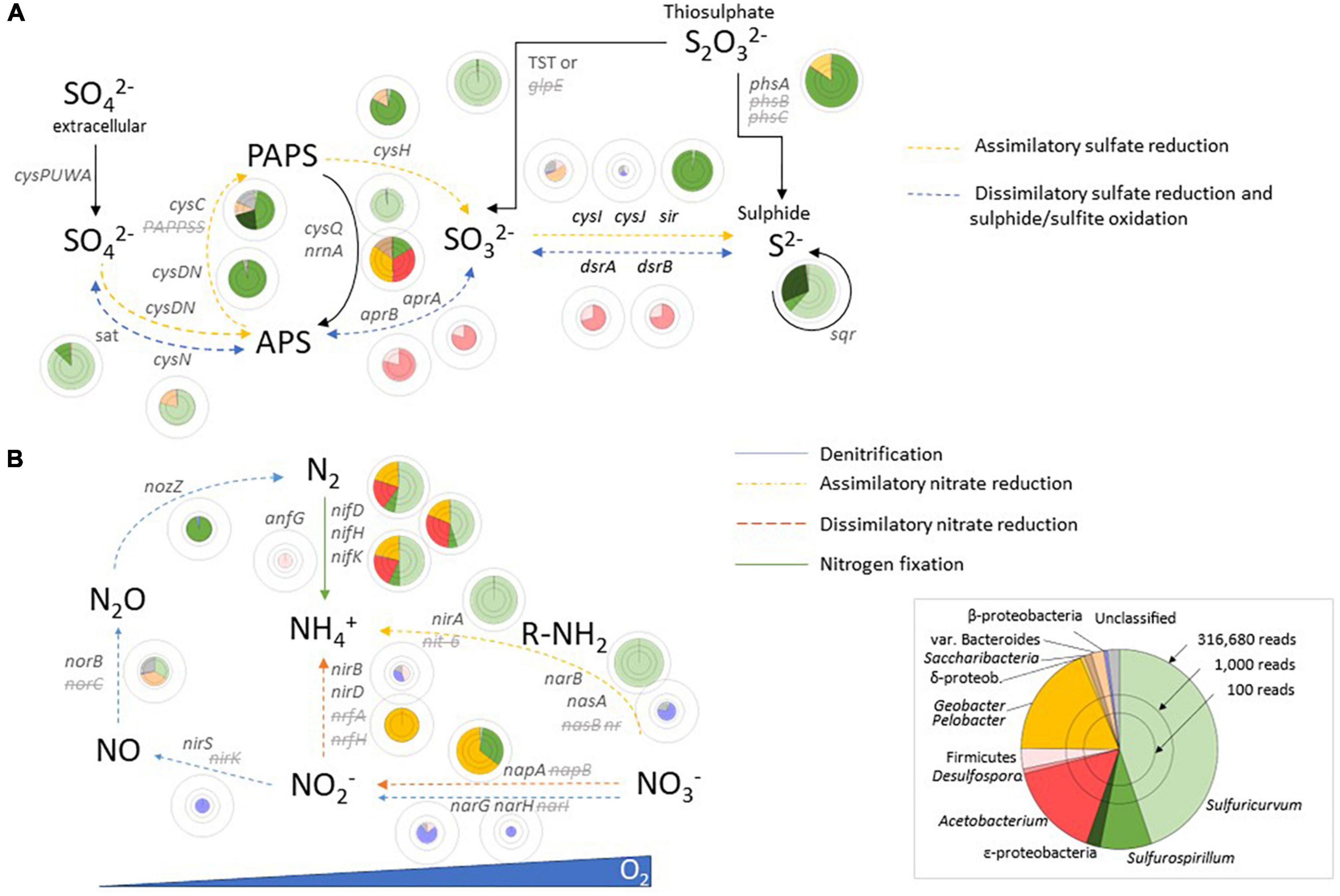
Figure 3. Sulphur metabolism (A) and nitrogen metabolism (B) in East Skaftárkatlar lake. APS, adenosine-5-phosphosulfate; PAPS, 3-phospho adenosine-5-phosphosulfate; PEP, phosphoenolpyruvate.
This sequence of genes does not appear in Sulfurospirillum and instead the presence of a polysulphide reductase chain A (phsA), or a homologue of phsA, suggests that reduction of sulphur species for energy conservation only includes reduction of elemental sulphur or thiosulphate to sulphate in this taxon. Genes coding for assimilatory sulphate reduction such as sulphate adenylyltransferases subunits 1 and 2 (cysDN), adenylylsulphate kinase (cysC), phosphoadenosine phosphosulphate reductase (cysH), and sulphite reductase (ferredoxin) (sir) were indeed assigned preferentially to Sulfurospirillum.
Moreover, the main enzymes involved in sulphur-disproportionation were not detected in the co-assembly metagenome. In that pathway, thiosulphate, sulphite, or elemental sulphur can serve as both electron or acceptor donors and are converted into sulphate and hydrogen sulphide.
3.3.3. Nitrogen metabolism
Some of the genes involved in nitrogen metabolism were detected in the metagenome (Figure 3B). No taxon has a complete assimilatory nitrate reduction pathway. The genes coding for the ferredoxin-nitrate reductase (narB) and the catalytic subunit of the assimilatory nitrate reductase (nasA) were detected but not the genes coding for the NADPH nitrate reductase (NR) or the assimilatory nitrate reductase electron transfer (nasB). Likewise, the gene coding for the ferredoxin-nitrite reductase (nirA) was detected but not the gene coding for the assimilatory nitrite reductase (nit-6). The detected genes are mainly taxonomically related to Sulfuricurvum. Some of the genes involved in dissimilatory nitrate reduction were found: napA but not napB from the cluster of genes coding the NapAB protein and narG, narH but not narI from the NarGHI cluster. Nitrite can then be reduced to ammonia with the nitrite reductase coded by the nirB and nirD genes found in our metagenomes. Genes involved in dissimilatory nitrate reduction seem to be related mainly to Geobacter/Pedobacter. Nitrogen fixation seems to be widespread in the Skaftárkatlar biome as all the genes coding for the dinitrogenase (nifD, nifK, nifH, and anfG), a molybdenum-iron protein reducing dinitrogen to ammonia, were detected, and were assigned to Sulfurospirillum, Sulfuricurvum, Acetobacterium, and Geobacter/Pelobacter.
3.3.4. Hydrogen
Potential utilisation of dihydrogen was investigated, revealing the presence of genes coding for the quinone-reactive Ni/Fe-hydrogenase small (hydA) and large subunit (hydB) and assigned to Sulfuricurvum and Sulfurospirillum. The genes coding for the different NADP-reducing hydrogenase subunits (hndB, hndC, and hndD) were identified to belong to Acetobacterium. The presence of these genes supports the use of H2 as an electron donor in these taxa.
3.3.5. Other metabolisms
Gene coding for arsenate reductase with disulphide as an acceptor was found (arsC) and assigned to Sulfuricurvum and Sulfurospirillum. Sulphide oxidation can be tied to the reduction of arsenate to arsenide by arsenate respiration. This ability was described for Sulfurospirillum (Stolz et al., 1999). Genes involved in anaerobic fumaric respiration such as fumarate reductase, flavoprotein subunit (frdA), and iron-sulphur subunit (fdrB) were identified and assigned to Sulfuricurvum, Sulfurospirillum, and Geobacter/Pelobacter. Only the cooS gene from the gene cluster coding for enzymes allowing anaerobic carboxydotrophy was detected and affiliated to Acetobacterium. Genes coding for enzymes involved in the dissimilatory reduction of Fe3+ were not detected.
4. Discussion
This study presents the first analysis of metagenomes originating from volcanic subglacial lake: one sample collected from the bottom of the lake (A3), a second from four pooled water column samples collected from different depths in the lake (Bmix), and a third from combined enrichments, i.e., growth under conditions that attempted to mimic those in the subglacial lake (Emix). The metagenomic analysis of the collected samples enabled a reconstruction of the potential metabolic pathways existing in the lake and a more robust description of the microbial community structure than before. Environmental metagenome analysis such as these have been shown to be more quantitative for microbial community studies, compared to amplicon-tag-based studies, due to the absence of Polymerase Chain Reaction (PCR) amplification bias (Parada et al., 2016; Brumfield et al., 2020).
This study not only confirms a previous study of the lake using 16S rRNA tag sequencing and Fluorescence In Situ Hybridisation (FISH) (Marteinsson et al., 2013) but also provides more robust evidence that only a few bacterial taxa dominate the microbial community in the lake water column (Sulfuricurvum, Acetobacterium, Geobacter/Pelobacter, and Sulfurospirillum). Metagenomic analyses in this study also confirm the presence of lower abundance taxa (e.g., Caldisericum and Desulfosporosinus) and reveal new additional lineages (Saccharibacteria and Pelosinus) which had not been observed by the previous amplicon-based studies. The relatively low microbial diversity (∼20 taxa) represented in the metagenome of this ecosystem resulted in high sampling depth and allowed assemblage of full-length 16S, 23S, and often also 5S sequences for most of the taxa. Full-length sequences permitted a more precise taxonomic assignment of the most abundant community members as well as 14 minor taxa with relative abundance ranging from 0.05 to 1%. Many of these taxa are closely related to psychrotolerant strains, including a member of Brevundimonas, a taxon detected in the Arctic (Trivedi et al., 2018) and Antarctic (González-Toril et al., 2009) and which is known to be resistant to cold temperatures (Dartnell et al., 2010) and the genus Pedobacter, also found in Arctic environments (Zhou et al., 2012; Peter and Sommaruga, 2016; Trivedi et al., 2018).
In a broader context, this study has confirmed previous results (Marteinsson et al., 2013) that the community in the East Skaftárkatlar lake is substantially different from that of other subglacial lakes such as Subglacial Lake Whillans in Antarctica. The former is dominated by Betaproteobacteria such as Polaromonas, Sideroxydans or Thiobacillus, Bacteroidetes, and Actinobacteria (Achberger et al., 2016) while the East Skaftárkatlar lake is dominated by Epsilonbacteria: Sulfuricurvum and Sulfurospirillum, and Firmicutes: Acetobacterium. East Skaftárkatlar could be significantly influenced by hydrothermal activity emanating from the underlying lake bed and thus can host, among others, sulphur oxidisers or reducers (Johannesson et al., 2007). Furthermore, the lake is a mix of glacial melt (containing oxygen) as well as sulphide from disproportionation of dissolved volcanic SO2 that supports a sulphur cycle. Then, Sulfurospirillum deleyianum is known to be able to oxidise sulphide with nitrate, producing ammonium and intracellular elemental sulphur (Eisenmann et al., 1995). This ability is also known in Sulfuricurvum species, the most abundant member of the microbial community in the sample collected at the bottom of the lake 75% (A3) compared to 30% in the water column samples (Bmix). The relative abundance of Sulfuricurvum correlates with the sulphate concentration, which was about five times higher in A3 than in the other pooled sample Bmix (4.71 ppm in A3 vs. from 0.29 to 1.43 ppm in B1 to B4, Supplementary Table 1). Compared to A3, a higher diversity was observed in Bmix (Shannon’s α-diversity of 1.18 and 1.83, respectively, data not shown) including three additional taxa: Peptococcaceae, Bacteroidetes, and Caldisericum spp. which might indicate that growth and metabolism are faster than the mixing time of the water column across any vertical or lateral chemical gradients in the lake. The higher diversity could also be a result of the fact that Bmix is derived from a mixture of samples.
Despite many different enrichment conditions, none of the dominant taxa found in the water column samples were enriched under the conditions of our incubations. The absence of culturable members of the main diversity is not unexpected as uncultivated phyla frequently dominate diverse environments (Lloyd et al., 2018). Interestingly, the dominant taxa found in Emix, Microbacterium, Hydrogenophaga, and Sphingopyxis were only marginally detectable in the metagenome (<10 raw rDNA reads) of the two environmental samples A3 and Bmix (Figure 1) and not detected in the rRNA tag sequences of Marteinsson et al. (2013).
Remarkably, no evidence of members of Archaea in the lake was detected in the previous 16S rRNA amplicon-tag sequencing study (Marteinsson et al., 2013). This includes all known chemolithotrophic methanogens, which could potentially compete with homoacetogens for H2. In this metagenome sequencing-based study, which is not affected by PCR bias, we identified a significant number of archaea-like sequences in A3 (data not shown). Nevertheless, a detailed analysis of those reads shows that they are not randomly distributed over a complete reference archaeal 16S rDNA gene (Supplementary Figure 1) and are instead localised to two overlapping regions. The absence of flanking sequence to these clusters and their exclusive location indicates that those reads do not originate from a complete gene. Therefore, we are not able to detect the presence of archaea in the lake with our metagenomic approach. The DNA extraction method used might also be a bias with an incomplete lysis of recalcitrant archaeal cells as it was noted in previous subsurface communities (Webster et al., 2003).
Since light is absent in the lake, chemoautotrophy must be responsible for the microbial growth as primary producers such as in subglacial lakes Vostok or Whillans (Rogers et al., 2013; Vick-Majors et al., 2016). The metabolic pathways detected in a combination of A3 and Bmix for carbon fixation are shown in Figure 2. Acetobacterium sp. contains most of the genes involved in the Wood–Ljungdahl homoacetogenesis pathway. This pathway uses H2 as an electron donor and CO2 both as an electron acceptor and carbon source to generate acetyl-CoA. Acetogenesis seems to be an important energy source in this ecosystem and supports the previous findings that the lakes contain taxon with homoacetogens as the closest relatives (Gaidos et al., 2004, 2009; Marteinsson et al., 2013). The closest cultured relative to Acetobacterium is Acetobacterium woodii, a strain using the Wood–Ljungdahl (reverse acetyl-CoA) pathway and H2 to fix CO2 into acetate and maintain a sodium ion gradient for ATP synthesis (Poehlein et al., 2012). Prokaryotes living close to the thermodynamic limit, like methanogens and acetogens, use the reductive acetyl-coA pathway for both CO2 fixation and energy conservation (Ragsdale and Pierce, 2008; Thauer et al., 2008). We propose that environmental factors such as low temperature and high H2 concentration in this ecosystem favour acetogens over methanogens (Nozhevnikova et al., 1994). The absence of methanogens in this unique ecosystem could also potentially be explained by the presence of bacteria such as Sulphate Reducing Bacteria (SRB) that out-compete methanogens for hydrogen or produced acetate in the lake, with lower Ks values for H2 and acetate (Kristjansson et al., 1982; Schönheit et al., 1982).
Complete pathways for assimilatory sulphur reduction into sulphide were detected and mainly assigned to Sulfurospirillum sp. whereas the dissimilatory reduction of sulphur in sulphide was assigned mainly to Acetobacterium sp. (Figure 3A). This difference might be the result of Sulfurospirillum only performing assimilatory sulphate reduction (i.e., to produce organosulphur compounds) and Acetobacterium the dissimilatory one to conserve energy. Sulfuricurvum was described as a sulphur oxidiser (Han et al., 2012) and not as a sulphate reducer which can explain why assimilatory genes were mainly assigned to Sulfurospirillum. Moreover, the reason why Sulfurospirillum has phsA gene and not standard dissimilatory genes might be explained by the low sulphate concentration in the lake making sulphate reduction difficult. Sulphide production might be energetically unfavourable against the background of high sulphide in the lake (around 1 mM). Thus sulphur-cycling species might take sulphur only to sulphite and then cycle it back to sulphate or sulphur under microaerobic conditions. Sulfuricurvum sp. and Sulfurospirillum sp. also play a role in activating sulphur with a polysulphide reductase. These results emphasise the central role of sulphur species as sources of electrons (S2–, SO42–, and S2O32–) for chemolithoautotrophy in the lake and, as expected, in the metabolism of amino acids. Respiratory sulphate reduction is a common process in environments with a high sulphate concentration whereas in sulphate-depleted anoxic environments, acetogenesis is favoured over sulphate reduction (Laanbroek et al., 1982; Muyzer and Stams, 2008; Stams and Plugge, 2009).
No complete nitrogen metabolic pathways were found in the co-assembly metagenome except for nitrogen fixation. The genes involved in the nitrogen fixation pathway were detected and belong mainly to Sulfuricurvum sp. The genus Sulfuricurvum sp. has not been reported as being diazotrophic but its sequenced representative Sulfuricurvum kujiense YK-1T also possesses the necessary genes (Kodama and Watanabe, 2004; Han et al., 2012). The dissimilatory nitrate reduction to ammonium, which is an important reaction of the reductive branch of the nitrogen cycle (Simon and Klotz, 2013), is mainly taxonomically affiliated with Geobacter/Pedobacter genus. Whereas nitrification is an important chemoautotrophic pathway of new organic carbon production in Subglacial Lake Whillans (Christner et al., 2014), none of the genes involved in this pathway were found in our metagenomes. The presence of nir (NADH-dependent) but absence of nrf (periplasmic) genes might imply that assimilatory reduction but not respiratory reduction (for energy conservation) might be occurring in this oligotrophic environment.
The study of microbial communities inhabiting subglacial lakes is of importance as such ecosystems remain underexplored. Only four subglacial lakes were sampled for microbial analyses at the time this paper was written (Gaidos et al., 2004, 2009; Christner et al., 2014; Priscu et al., 2021). The results in our research support the former hypothesis that was based on a taxonomy study, but it also gives insight into the metabolic pathways. The ecosystem in this subglacial lake appears to have a chemolithoautotrophic foundation with sulphur and carbon cycling that is fuelled by H2, CO2, and sulphur species originating from the geothermal activity and by O2 from melted ice. By identifying the potential metabolic pathways to specific taxa in the microbial assemblage, we have gained insight into the ecology of microbiomes in the water column of such an extreme environment. However, we should assume that other ecological niches may exist, e.g., on the bottom of the lake, in the lake sediments, or at the ice/lake interface, and these remain undiscovered. Moreover, nearly half of the sequence reads in our metagenomic library were not assigned and are a source for future advances. Also, new targeted sampling with larger volume is needed e.g., samples collected close to potential geothermal vents at the bottom of the lake might reveal thermophiles belonging to both the Bacteria and Archaea by sequencing and cultivation strategies. Even if analysed samples were collected in 2007, the results of this study might still correlate with the current situation of the lake as the latter has experienced similar conditions and has undergone continuous filling and draining (called jökulhlaup) since records have been kept.
Finally, due to recent Arctic warming, ice caps are thinning, notably in Iceland (Gudmundsson et al., 2008). Despite their isolation from the surface, these lakes are influenced by the dynamics and melting of glaciers, which are changing with climate change (Livingstone et al., 2022). The existence of subglacial lakes is thus threatened in the long run. The complete exploration of the microbial diversity of this ecosystem needs to happen quickly to monitor such future changes.
Data availability statement
The data presented in this study are deposited in NCBI Sequences Read Archive repository under the accession number: SRP011365 (https://www.ncbi.nlm.nih.gov/sra/?term=SRP011365).
Author contributions
PV, GF, and VM conceived and designed the study and analysed the data. AK performed the DNA extractions. GF performed the bioinformatic analyses. PV, GF, EG, and VM wrote the main manuscript text. All authors reviewed the manuscript.
Funding
This project had received funding from the European Union’s Horizon 2020 Research and Innovation Programme under the Marie Skłodowska-Curie actions grant agreement no. 704956. Sampling expeditions and sample analysis were provided by the Icelandic Centre for Research (Contract No. 080222023). Partial support for fieldwork was provided by the National Aeronautics and Space Administration through the NASA Astrobiology Institute under Cooperative Agreement No. NNA04CC08A issued through the Office of Space Science.
Acknowledgments
We would like to thank the Meteorological Office of Iceland (Veðurstofa Íslands) for realising the original and later collections of samples. The Illumina sequencings were made possible by the Deep Carbon Observatory’s Census of Deep Life supported by the Alfred P. Sloan Foundation. Sequencing was performed at the Marine Biological Laboratory (Woods Hole, MA, United States) and we are grateful for the assistance of Mitch Sogin, Susan Huse, Joseph Vineis, Andrew Voorhis, Sharon Grim, and Hilary Morrison at MBL.
Conflict of interest
The authors declare that the research was conducted in the absence of any commercial or financial relationships that could be construed as a potential conflict of interest.
Publisher’s note
All claims expressed in this article are solely those of the authors and do not necessarily represent those of their affiliated organizations, or those of the publisher, the editors and the reviewers. Any product that may be evaluated in this article, or claim that may be made by its manufacturer, is not guaranteed or endorsed by the publisher.
Supplementary material
The Supplementary Material for this article can be found online at: https://www.frontiersin.org/articles/10.3389/fmicb.2023.1122184/full#supplementary-material
Footnotes
References
Achberger, A. M., Christner, B. C., Michaud, A. B., Priscu, J. C., Skidmore, M. L., Vick-Majors, T. J., et al. (2016). Microbial community structure of subglacial lake whillans. West Antarctica. Front. Microbiol. 7:1457. doi: 10.3389/fmicb.2016.01457
Agustsdottir, A. M., and Brantley, S. L. (1994). Volatile fluxes integrated over 4 decades at Grímsvötn volcano, Iceland. J. Geophys. Research-Solid Earth 99, 9505–9522. doi: 10.1029/93JB03597
Alneberg, J., Bjarnason, B. S., De Bruijn, I., Schirmer, M., Quick, J., Ijaz, U. Z., et al. (2014). Binning metagenomic contigs by coverage and composition. Nat. Methods 11, 1144–1146. doi: 10.1038/nmeth.3103
Balch, W. E., Fox, G. E., Magrum, L. J., Woese, C. R., and Wolfe, R. S. (1979). Methanogens: re-evaluation of a unique biological group. Microbiol. Rev. 43, 260–296. doi: 10.1128/mr.43.2.260-296.1979
Bankevich, A., Nurk, S., Antipov, D., Gurevich, A. A., Dvorkin, M., Kulikov, A. S., et al. (2012). SPAdes: a new genome assembly algorithm and its applications to single-cell sequencing. J. Comp. Biol. 19, 455–477. doi: 10.1089/cmb.2012.0021
Bjornsson, H. (2003). Subglacial lakes and jokulhlaups in Iceland. Global Plan. Change 35, 255–271. doi: 10.1016/S0921-8181(02)00130-3
Brown, C. T., Hug, L. A., Thomas, B. C., Sharon, I., Castelle, C. J., Singh, A., et al. (2015). Unusual biology across a group comprising more than 15% of domain Bacteria. Nature 523:208. doi: 10.1038/nature14486
Brumfield, K. D., Huq, A., Colwell, R. R., Olds, J. L., and Leddy, M. B. (2020). Microbial resolution of whole genome shotgun and 16S amplicon metagenomic sequencing using publicly available NEON data. PLoS One 15:e0228899. doi: 10.1371/journal.pone.0228899
Buchfink, B., Xie, C., and Huson, D. H. (2015). Fast and sensitive protein alignment using DIAMOND. Nat. Methods 12:59. doi: 10.1038/nmeth.3176
Bulat, S. A. (2016). Microbiology of the subglacial Lake Vostok: first results of borehole-frozen lake water analysis and prospects for searching for lake inhabitants. Philos. Trans. R. Soc. Mathematical Phys. Eng. Sci. 374:20140292. doi: 10.1098/rsta.2014.0292
Camacho, C., Coulouris, G., Avagyan, V., Ma, N., Papadopoulos, J., Bealer, K., et al. (2009). BLAST+: architecture and applications. BMC Bioinformatics 10:421. doi: 10.1186/1471-2105-10-421
Chinn, T. (1993). Physical hydrology of the dry valley lakes. Phys. Biogeochem. Proc. Antarctic Lakes 59, 1–51. doi: 10.1029/AR059p0001
Christner, B. C., Mosley-Thompson, E., Thompson, L. G., and Reeve, J. N. (2001). Isolation of bacteria and 16S rDNAs from Lake Vostok accretion ice. Environ. Microbiol. 3, 570–577. doi: 10.1046/j.1462-2920.2001.00226.x
Christner, B. C., Priscu, J. C., Achberger, A. M., Barbante, C., Carter, S. P., Christianson, K., et al. (2014). A microbial ecosystem beneath the West Antarctic ice sheet. Nature 512, 310–313. doi: 10.1038/nature13667
Dartnell, L. R., Hunter, S. J., Lovell, K. V., Coates, A. J., and Ward, J. M. (2010). Low-temperature ionizing radiation resistance of Deinococcus radiodurans and Antarctic Dry Valley bacteria. Astrobiology 10, 717–732. doi: 10.1089/ast.2009.0439
Davies, B. J., Hambrey, M. J., Glasser, N. F., Holt, T., Rodés, A., Smellie, J. L., et al. (2017). Ice-dammed lateral lake and epishelf lake insights into Holocene dynamics of marguerite trough ice stream and george VI Ice Shelf, Alexander Island, Antarctic Peninsula. Quaternary Sci. Rev. 177, 189–219. doi: 10.1016/j.quascirev.2017.10.016
Degryse, E., Glansdorff, N., and Pierard, A. (1978). Comparative analysis of extreme thermophilic bacteria belonging to genus Thermus. Arch. Microbiol. 117, 189–196. doi: 10.1007/BF00402307
Eisenmann, E., Beuerle, J., Sulger, K., Kroneck, P. M., and Schumacher, W. (1995). Lithotrophic growth of Sulfurospirillum deleyianum with sulfide as electron donor coupled to respiratory reduction of nitrate to ammonia. Arch. Microbiol. 164, 180–185. doi: 10.1007/BF02529969
Ferrari, B., Winsley, T., Ji, M., and Neilan, B. (2014). Insights into the distribution and abundance of the ubiquitous candidatus Saccharibacteria phylum following tag pyrosequencing. Sci. Rep. 4:3957. doi: 10.1038/srep03957
Gaidos, E., Lanoil, B., Thorsteinsson, T., Graham, A., Skidmore, M., Han, S. K., et al. (2004). A viable microbial community in a subglacial volcanic crater lake. Iceland. Astrobiology 4, 327–344. doi: 10.1089/ast.2004.4.327
Gaidos, E., Marteinsson, V., Thorsteinsson, T., Johannesson, T., Runarsson, A. R., Stefansson, A., et al. (2009). An oligarchic microbial assemblage in the anoxic bottom waters of a volcanic subglacial lake. ISME J. 3, 486–497. doi: 10.1038/ismej.2008.124
González-Toril, E., Amils, R., Delmas, R. J., Petit, J.-R., Komárek, J., and Elster, J. (2009). Bacterial diversity of autotrophic enriched cultures from remote, glacial Antarctic, Alpine and Andean aerosol, snow and soil samples. Biogeosciences 6, 33–44. doi: 10.5194/bg-6-33-2009
Gudmundsson, M. T., Larsen, G., Höskuldsson, Á, and Gylfason, ÁG. (2008). Volcanic hazards in Iceland. Jokull 58, 251–268. doi: 10.33799/jokull2008.58.251
Gura, C., and Rogers, S. O. (2020). Metatranscriptomic and metagenomic analysis of biological diversity in subglacial lake vostok (Antarctica). Biology 9:55. doi: 10.3390/biology9030055
Han, C., Kotsyurbenko, O., Chertkov, O., Held, B., Lapidus, A., Nolan, M., et al. (2012). Complete genome sequence of the sulfur compounds oxidizing chemolithoautotroph Sulfuricurvum kujiense type strain (YK-1 T). Standards Genomic Sci. 6:94. doi: 10.4056/sigs.2456004
Howard-Williams, C., Schwarz, A.-M., Hawes, I., and Priscu, J. (1998). Optical properties of the McMurdo Dry Valley lakes. Antarctic Res. Ser. 72, 189–203. doi: 10.1029/AR072p0189
Huang, Y., Gilna, P., and Li, W. (2009). Identification of ribosomal RNA genes in metagenomic fragments. Bioinformatics 25, 1338–1340. doi: 10.1093/bioinformatics/btp161
Johannesson, T., Thorsteinsson, T., Stefansson, A., Gaidos, E. J., and Einarsson, B. (2007). Circulation and thermodynamics in a subglacial geothermal lake under the Western Skafta cauldron of the Vatnajokull ice cap, Iceland. Geophys. Res. Lett. 34, 502–508. doi: 10.1029/2007GL030686
Kanehisa, M., and Goto, S. (2000). KEGG: kyoto encyclopedia of genes and genomes. Nucleic Acids Res. 28, 27–30. doi: 10.1093/nar/28.1.27
Kanehisa, M., Sato, Y., Kawashima, M., Furumichi, M., and Tanabe, M. (2015). KEGG as a reference resource for gene and protein annotation. Nucleic Acids Res. 44, D457–D462. doi: 10.1093/nar/gkv1070
Karl, D., Bird, D., Björkman, K., Houlihan, T., Shackelford, R., and Tupas, L. (1999). Microorganisms in the accreted ice of Lake Vostok, Antarctica. Science 286, 2144–2147. doi: 10.1126/science.286.5447.2144
Kirschvink, J. L., Gaidos, E. J., Bertani, L. E., Beukes, N. J., Gutzmer, J., Maepa, L. N., et al. (2000). Paleoproterozoic snowball Earth: extreme climatic and geochemical global change and its biological consequences. Proc. Natl. Acad. Sci. U S A. 97, 1400–1405. doi: 10.1073/pnas.97.4.1400
Kivelson, M. G., Khurana, K. K., Russell, C. T., Volwerk, M., Walker, R. J., and Zimmer, C. (2000). Galileo magnetometer measurements: a stronger case for a subsurface ocean at Europa. Science 289, 1340–1343. doi: 10.1126/science.289.5483.1340
Kivelson, M. G., Khurana, K. K., and Volwerk, M. (2002). The permanent and inductive magnetic moments of ganymede. Icarus 157, 507–522. doi: 10.1006/icar.2002.6834
Kodama, Y., and Watanabe, K. (2004). Sulfuricurvum kujiense gen. nov., sp nov., a facultatively anaerobic, chemolithoautotrophic, sulfur-oxidizing bacterium isolated from an underground crude-oil storage cavity. Int. J. Systematic Evol. Microbiol. 54, 2297–2300. doi: 10.1099/ijs.0.63243-0
Kristjansson, J. K., Schönheit, P., and Thauer, R. K. (1982). Different K s values for hydrogen of methanogenic bacteria and sulfate reducing bacteria: an explanation for the apparent inhibition of methanogenesis by sulfate. Arch. Microbiol. 131, 278–282. doi: 10.1007/BF00405893
Laanbroek, H. J., Abee, T., and Voogd, I. L. (1982). Alcohol conversion by Desulfobulbus propionicus Lindhorst in the presence and absence of sulfate and hydrogen. Arch. Microbiol. 133, 178–184. doi: 10.1007/BF00414998
Lauro, S. E., Pettinelli, E., Caprarelli, G., Guallini, L., Rossi, A. P., Mattei, E., et al. (2020). Multiple subglacial water bodies below the south pole of Mars unveiled by new MARSIS data. Nat. Astronomy 5, 63–70. doi: 10.1038/s41550-020-1200-6
Livingstone, S. J., Li, Y., Rutishauser, A., Sanderson, R. J., Winter, K., Mikucki, J. A., et al. (2022). Subglacial lakes and their changing role in a warming climate. Nat. Rev. Earth Environ. 3, 106–124. doi: 10.1038/s43017-021-00246-9
Lloyd, K. G., Steen, A. D., Ladau, J., Yin, J., and Crosby, L. (2018). Phylogenetically novel uncultured microbial cells dominate earth microbiomes. mSystems 3:e00055-18. doi: 10.1128/mSystems.00055-18
Logares, R., Sunagawa, S., Salazar, G., Cornejo-Castillo, F. M., Ferrera, I., Sarmento, H., et al. (2014). Metagenomic 16S rDNA I llumina tags are a powerful alternative to amplicon sequencing to explore diversity and structure of microbial communities. Environ. Microbiol. 16, 2659–2671. doi: 10.1111/1462-2920.12250
Magoč, T., and Salzberg, S. L. (2011). FLASH: fast length adjustment of short reads to improve genome assemblies. Bioinformatics 27, 2957–2963. doi: 10.1093/bioinformatics/btr507
Marteinsson, V. T., Birrien, J. L., and Prieur, D. (1997). In situ enrichment and isolation of thermophilic microorganisms from deep-sea vent environments. Canadian J. Microbiol. 43, 694–697. doi: 10.1139/m97-100
Marteinsson, V. T., Kristjansson, J. K., Kristmannsdottir, H., Dahlkvist, M., Saemundsson, K., Hannington, M., et al. (2001). Discovery and description of giant submarine smectite cones on the seafloor in Eyjafjordur, northern Iceland, and a novel thermal microbial habitat. Appl. Environ. Microbiol. 67, 827–833. doi: 10.1128/AEM.67.2.827-833.2001
Marteinsson, V. T., Runarsson, A., Stefansson, A., Thorsteinsson, T., Johannesson, T., Magnusson, S. H., et al. (2013). Microbial communities in the subglacial waters of the Vatnajokull ice cap. Iceland. ISME J. 7, 427–437. doi: 10.1038/ismej.2012.97
Mori, K., Yamaguchi, K., Sakiyama, Y., Urabe, T., and Suzuki, K.-I. (2009). Caldisericum exile gen. nov., sp. nov., an anaerobic, thermophilic, filamentous bacterium of a novel bacterial phylum, Caldiserica phyl. nov., originally called the candidate phylum OP5, and description of Caldisericaceae fam. nov., Caldisericales ord. nov. and Caldisericia classis nov. Int. J. Systematic Evol. Microbiol. 59, 2894–2898. doi: 10.1099/ijs.0.010033-0
Muyzer, G., and Stams, A. J. M. (2008). The ecology and biotechnology of sulphate-reducing bacteria. Nat. Rev. Microbiol. 6, 441–454. doi: 10.1038/nrmicro1892
Nozhevnikova, A. N., Kotsyurbenko, O. R., and Simankova, M. V. (1994). “Acetogenesis at low temperature,” in Acetogenesis. Chapman & Hall Microbiology Series, ed. H. L. Drake (Boston, MA: Springer), 416–431. doi: 10.1007/978-1-4615-1777-1_15
Palmer, S. J., Dowdeswell, J. A., Christoffersen, P., Young, D. A., Blankenship, D. D., Greenbaum, J. S., et al. (2013). Greenland subglacial lakes detected by radar. Geophys. Res. Lett. 40, 6154–6159. doi: 10.1002/2013GL058383
Parada, A. E., Needham, D. M., and Fuhrman, J. A. (2016). Every base matters: assessing small subunit rRNA primers for marine microbiomes with mock communities, time series and global field samples. Environ. Microbiol. 18, 1403–1414. doi: 10.1111/1462-2920.13023
Peng, Y., Leung, H. C., Yiu, S.-M., and Chin, F. Y. (2012). IDBA-UD: a de novo assembler for single-cell and metagenomic sequencing data with highly uneven depth. Bioinformatics 28, 1420–1428. doi: 10.1093/bioinformatics/bts174
Peter, H., and Sommaruga, R. (2016). Shifts in diversity and function of lake bacterial communities upon glacier retreat. ISME J. 10:1545. doi: 10.1038/ismej.2015.245
Poehlein, A., Schmidt, S., Kaster, A.-K., Goenrich, M., Vollmers, J., Thürmer, A., et al. (2012). An ancient pathway combining carbon dioxide fixation with the generation and utilization of a sodium ion gradient for ATP synthesis. PLoS One 7:e33439. doi: 10.1371/journal.pone.0033439
Priscu, J. C., Adams, E. E., Lyons, W. B., Voytek, M. A., Mogk, D. W., Brown, R. L., et al. (1999). Geomicrobiology of subglacial ice above Lake Vostok. Antarctica. Science 286, 2141–2144. doi: 10.1126/science.286.5447.2141
Priscu, J. C., Kalin, J., Winans, J., Campbell, T., Siegfried, M. R., Skidmore, M., et al. (2021). Scientific access into Mercer Subglacial Lake: scientific objectives, drilling operations and initial observations. Ann. Glaciol. 62, 340–352. doi: 10.1017/aog.2021.10
Quast, C., Pruesse, E., Yilmaz, P., Gerken, J., Schweer, T., Yarza, P., et al. (2012). The SILVA ribosomal RNA gene database project: improved data processing and web-based tools. Nucleic Acids Res. 41, D590–D596. doi: 10.1093/nar/gks1219
Ragsdale, S. W., and Pierce, E. (2008). Acetogenesis and the Wood–Ljungdahl pathway of CO2 fixation. Biochim. Biophys. Acta (BBA)-Proteins Proteom. 1784, 1873–1898. doi: 10.1016/j.bbapap.2008.08.012
Reasoner, D. J., and Geldreich, E. E. (1985). A new medium for the enumeration and subculture of bacteria from potable water. Appl. Environ. Microbiol. 49, 1–7. doi: 10.1128/aem.49.1.1-7.1985
Rogers, S., Shtarkman, Y., Koçer, Z., Edgar, R., Veerapaneni, R., and Elia, T. (2013). Ecology of subglacial lake vostok (Antarctica), based on metagenomic/metatranscriptomic analyses of accretion ice. Biology 2, 629. doi: 10.3390/biology2020629
Rutishauser, A., Blankenship, D. D., Sharp, M., Skidmore, M. L., Greenbaum, J. S., Grima, C., et al. (2018). Discovery of a hypersaline subglacial lake complex beneath Devon Ice Cap, Canadian Arctic. Sci. Adv. 4:eaar4353. doi: 10.1126/sciadv.aar4353
Schönheit, P., Kristjansson, J. K., and Thauer, R. K. (1982). Kinetic mechanism for the ability of sulfate reducers to out-compete methanogens for acetate. Arch. Microbiol. 132, 285–288. doi: 10.1007/BF00407967
Simon, J., and Klotz, M. G. (2013). Diversity and evolution of bioenergetic systems involved in microbial nitrogen compound transformations. Biochim. Biophys. Acta (BBA)-Bioenergetics 1827, 114–135. doi: 10.1016/j.bbabio.2012.07.005
Stams, A. J., and Plugge, C. M. (2009). Electron transfer in syntrophic communities of anaerobic bacteria and archaea. Nat. Rev. Microbiol. 7, 568–577. doi: 10.1038/nrmicro2166
Stoddard, S. F., Smith, B. J., Hein, R., Roller, B. R., and Schmidt, T. M. (2014). rrnDB: improved tools for interpreting rRNA gene abundance in bacteria and archaea and a new foundation for future development. Nucleic Acids Res. 43, D593–D598. doi: 10.1093/nar/gku1201
Stolz, J. F., Ellis, D. J., Blum, J. S., Ahmann, D., Lovley, D. R., and Oremland, R. S. (1999). Sulfurospirillum barnesii sp nov and Sulfurospirillum arsenophilum sp nov., new members of the Sulfurospirillum clade of the epsilon Proteobacteria. Int. J. Systematic Bacteriol. 49, 1177–1180. doi: 10.1099/00207713-49-3-1177
Thauer, R. K., Kaster, A.-K., Seedorf, H., Buckel, W., and Hedderich, R. (2008). Methanogenic archaea: ecologically relevant differences in energy conservation. Nat. Rev. Microbiol. 6, 579–591. doi: 10.1038/nrmicro1931
Thomas, P. C., Tajeddine, R., Tiscareno, M. S., Burns, J. A., Joseph, J., Loredo, T. J., et al. (2016). Enceladus’s measured physical libration requires a global subsurface ocean. Icarus 264, 37–47. doi: 10.1016/j.icarus.2015.08.037
Thorsteinsson, T., Elefsen, S. O., Gaidos, E., Lanoil, B., Johannesson, T., Kjartansson, V., et al. (2008). A hot water drill with built-in sterilization: design, testing and performance. Jokull 51, 71–82. doi: 10.33799/jokull2007.57.071o
Trivedi, C. B., Lau, G. E., Grasby, S. E., Templeton, A. S., and Spear, J. R. (2018). Low-temperature sulfidic-ice microbial communities, borup fiord pass, Canadian high Arctic. Front. Microbiol. 9:1622. doi: 10.3389/fmicb.2018.01622
Tulaczyk, S., Mikucki, J. A., Siegfried, M. R., Priscu, J. C., Barcheck, C. G., Beem, L. H., et al. (2014). WISSARD at Subglacial Lake Whillans, West Antarctica: scientific operations and initial observations. Ann. Glaciol. 55, 51–58. doi: 10.3189/2014AoG65A009
Vick-Majors, T. J., Mitchell, A. C., Achberger, A. M., Christner, B. C., Dore, J. E., Michaud, A. B., et al. (2016). Physiological ecology of microorganisms in subglacial lake whillans. Front. Microbiol. 7:1705. doi: 10.3389/fmicb.2016.01705
Wang, Q., Garrity, G. M., Tiedje, J. M., and Cole, J. R. (2007). Naive Bayesian classifier for rapid assignment of rRNA sequences into the new bacterial taxonomy. Appl. Environ. Microbiol. 73, 5261–5267. doi: 10.1128/AEM.00062-07
Webster, G., Newberry, C. J., Fry, J. C., and Weightman, A. J. (2003). Assessment of bacterial community structure in the deep sub-seafloor biosphere by 16S rDNA-based techniques: a cautionary tale. J. Microbiol. Methods 55, 155–164. doi: 10.1016/S0167-7012(03)00140-4
Yilmaz, P., Parfrey, L. W., Yarza, P., Gerken, J., Pruesse, E., Quast, C., et al. (2013). The SILVA and “All-species Living Tree Project (LTP)” taxonomic frameworks. Nucleic Acids Res. 42, D643–D648. doi: 10.1093/nar/gkt1209
Zhou, Z., Jiang, F., Wang, S., Peng, F., Dai, J., Li, W., et al. (2012). Pedobacter arcticus sp. nov., a facultative psychrophile isolated from Arctic soil, and emended descriptions of the genus Pedobacter, Pedobacter heparinus, Pedobacter daechungensis, Pedobacter terricola, Pedobacter glucosidilyticus and Pedobacter lentus. Int. J. Systematic Evol. Microbiol. 62, 1963–1969. doi: 10.1099/ijs.0.031104-0
Keywords: subglacial lakes, Iceland, microbial assemblage, metagenome, metabolism
Citation: Vannier P, Farrant GK, Klonowski A, Gaidos E, Thorsteinsson T and Marteinsson Vþ (2023) Metagenomic analyses of a microbial assemblage in a subglacial lake beneath the Vatnajökull ice cap, Iceland. Front. Microbiol. 14:1122184. doi: 10.3389/fmicb.2023.1122184
Received: 12 December 2022; Accepted: 13 March 2023;
Published: 30 March 2023.
Edited by:
Prashant Kumar Singh, Mizoram University, IndiaReviewed by:
Marek Stibal, Charles University, CzechiaNaveen Chandra Joshi, Amity University, Noida, India
Copyright © 2023 Vannier, Farrant, Klonowski, Gaidos, Thorsteinsson and Marteinsson. This is an open-access article distributed under the terms of the Creative Commons Attribution License (CC BY). The use, distribution or reproduction in other forums is permitted, provided the original author(s) and the copyright owner(s) are credited and that the original publication in this journal is cited, in accordance with accepted academic practice. No use, distribution or reproduction is permitted which does not comply with these terms.
*Correspondence: Pauline Vannier, cGF1bGluZS52YW5uaWVyQG1hdGlzLmlz; Viggó þór Marteinsson, dmlnZ28ubWFydGVpbnNzb25AbWF0aXMuaXM=
†These authors have contributed equally to this work and share first authorship