- 1Fujian Provincial Key Laboratory of Soil Environmental Health and Regulation, College of Resources and Environment, Fujian Agriculture and Forestry University, Fuzhou, Fujian, China
- 2Hebei Provincial Key Laboratory of Soil Ecology, Center for Agricultural Resources Research, Institute of Genetic and Developmental Biology, Chinese Academy of Sciences, Shijiazhuang, Hebei, China
- 3Zhejiang Provincial Key Laboratory of Agricultural Resources and Environment, Institute of Soil and Water Resources and Environmental Science, Zhejiang University, Hangzhou, China
- 4Faculty of Agriculture and Life Sciences, Lincoln University, Lincoln, New Zealand
- 5Faculty of Agricultural and Environmental Sciences, Grassland and Fodder Sciences, University of Rostock, Rostock, Germany
- 6State Key Laboratory for Managing Biotic and Chemical Threats to the Quality and Safety of Agro-Products, Key Laboratory of Biotechnology in Plant Protection of Ministry of Agriculture and Zhejiang Province, Institute of Plant Virology, Ningbo University, Ningbo, China
- 7AgResearch Ltd., Hamilton, New Zealand
Numerous studies have investigated the effects of nitrogen (N) addition on soil organic carbon (SOC) decomposition. However, most studies have focused on the shallow top soils <0.2 m (surface soil), with a few studies also examining the deeper soil depths of 0.5–1.0 m (subsoil). Studies investigating the effects of N addition on SOC decomposition in soil >1.0 m deep (deep soil) are rare. Here, we investigated the effects and the underlying mechanisms of nitrate addition on SOC stability in soil depths deeper than 1.0 m. The results showed that nitrate addition promoted deep soil respiration if the stoichiometric mole ratio of nitrate to O2 exceeded the threshold of 6:1, at which nitrate can be used as an alternative acceptor to O2 for microbial respiration. In addition, the mole ratio of the produced CO2 to N2O was 2.57:1, which is close to the theoretical ratio of 2:1 expected when nitrate is used as an electron acceptor for microbial respiration. These results demonstrated that nitrate, as an alternative acceptor to O2, promoted microbial carbon decomposition in deep soil. Furthermore, our results showed that nitrate addition increased the abundance of SOC decomposers and the expressions of their functional genes, and concurrently decreased MAOC, and the ratio of MAOC/SOC decreased from 20% before incubation to 4% at the end of incubation. Thus, nitrate can destabilize the MAOC in deep soils by stimulating microbial utilization of MAOC. Our results imply a new mechanism on how above-ground anthropogenic N inputs affect MAOC stability in deep soil. Mitigation of nitrate leaching is expected to benefit the conservation of MAOC in deep soil depths.
1. Introduction
Globally, the stock of soil organic carbon (SOC) is estimated to be as high as 2300 Pg in the 3 m depth, which is about 3-fold the size of the atmospheric carbon dioxide (CO2) pool (770 Pg) (Lal, 2004). The annual CO2 emissions due to soil respiration are reported to range from 60 to 100 Pg C yr–1, which is an order of magnitude greater than current fossil fuel CO2 emissions (Bond-Lamberty and Thomson, 2010; Oertel et al., 2016; Xu and Shang, 2016) and account for 5∼25% of total annual CO2 emissions globally (Raich and Potter, 1995; Wang et al., 2018). CO2 is the dominant greenhouse gas and the atmospheric concentration of CO2 has increased from 277 μl l–1 in 1750 to 411 μl l–1 in 2019 (Friedlingstein et al., 2020; Walker et al., 2021). Thus, any enhanced loss of CO2 via SOC decomposition has significant implications for global warming (Zhang et al., 2020).
Anthropogenic nitrogen (N) inputs are reported to significantly affect SOC content (Mazzoncini et al., 2011; Riggs and Hobbie, 2016; Chen et al., 2021). Globally, anthropogenic N inputs have increased from 156 Tg N yr–1 in 1995 to 193 Tg N yr–1 in 2010 (Galloway et al., 2008; Fowler et al., 2015), and it is estimated that by 2050 the global rate of N inputs will double the rate in 1995 (Penuelas et al., 2020). A considerable portion of the anthropogenically derived N is transformed into nitrate, which can leach to depth (>1 m) within the soil profile (Van Meter et al., 2016; Xin et al., 2019; Yang et al., 2020; Gao et al., 2021). As the soil profile deepens, persistent hypoxia or even anoxia can establish, potentially resulting in nitrate being reduced via the denitrification or dissimilatory nitrate reduction to ammonium (DNRA) pathways, which require SOC as an electron donor (Laursen and Seitzinger, 2002; Giblin et al., 2013). Consequently, anthropogenic N inputs potentially affect SOC decomposition not only at the soil surface but also in the deeper soil profile.
The SOC in deep soil is expected to respond to N addition differently from that of surface soil due to carbon sources being distinctively different between the surface soil and deep soil (Salomé et al., 2010). In surface soil, plant residues and root exudates are important sources of SOC. In line with this, increased CO2 emission following N addition were found to be derived from plant residues and root exudates (Schulte-Uebbing and de Vries, 2018; Xu et al., 2021). This mechanism would be expected to be less significant in deep soil since the contribution of plant residue and roots to SOC sharply decreased with the increasing soil depth (Poirier et al., 2018). Furthermore, oxygen (O2), an electron acceptor for SOC oxidation, is more available to SOC decomposers in surface soil than in deep soil. The soil O2 concentration generally declines sharply from the surface to a depth of approximately 1.0 m, then continues to decline slowly with the increase of soil depth (Sierra and Renault, 1998; Orem et al., 2011; Kautz, 2015). Thus, nitrate in deep soil has a larger opportunity to replace O2 as an alternative electron acceptor for SOC oxidation. Compared with the SOC in surface soil, the SOC in deep soil is generally absorbed or co-precipitated with minerals as mineral associated organic carbon (MAOC), which potentially decreases its accessibility for soil microbial decomposition (Stuckey et al., 2018; Jilling et al., 2021). The observed increase in decomposers, induced by N addition in deep soil, is expected to enhance the potential for decomposers to destabilize MAOC (Feng et al., 2022).
Thus, the response of the carbon following N addition differs since the distinct SOC in surface and deep soils. Most previous studies have only investigated the response of SOC following N addition in the surface soil (<1.0 m depth). Many of these studies reported that N addition increased SOC content (Riggs et al., 2015; Philben et al., 2019), while other studies reported that N addition decreased (Mo et al., 2008; Treseder, 2008; Bulseco et al., 2019) or did not affect SOC content (Högberg, 2007; Lu et al., 2011), this may be attributed to the form of N, the level of application and soil type. While, few studies have investigated the effects of N addition on carbon decomposition in deep soil (Li et al., 2014; Xu et al., 2021). Such information is relevant for understanding MAOC stability in the deep soil and carbon sequestration.
In this study, we investigated the responses and relevant mechanisms of MAOC stability following nitrate addition to deep soil. Nitrate was selected as the N source because it is the main form of anthropogenic N that leaches into deep soil.
2. Materials and methods
2.1. Experimental site and sample collection
Soil samples were collected from the campus of the Fujian Agriculture and Forestry University, Fuzhou, China (26°06′ N, 119°13′ E). Three depths of soil (1.5–1.7, 2.0–2.2, and 2.5–2.7 m) were collected. Deep soil in this study is defined as soil depths >1.0 m. The soil samples were passed through a 2 mm sieve to remove as much live and dead root material, then basic soil physicochemical properties were determined, which are shown in Table 1. Soils were placed in sealed ziplock bags, with excess air removed using vacuum package machine to minimize exposure to O2, and stored at −20°C about 3 days, then we started the experiments. Soils were thawed at 4°C and preincubated at 20°C (Fontaine et al., 2007; Condron et al., 2014) for 5 days under anaerobic condition to recover microbial activity prior to commencing experiments.
The SOC content was determined using an elemental analyzer (Vario Macro Cube, Elementar, Germany). Soil moisture content was determined by drying fresh soil samples to constant weight at 105°C oven. Soil samples were extracted with 1 M KCl solution (soil: liquid ratio was 1:5) and then filtered (0.45 μm, Jinteng, China). The soil extracts were then analyzed for dissolved organic carbon (DOC) concentration using a total organic carbon analyzer (TOC-LCPH, Shimadzu, Japan), for nitrate, nitrite and ammonium concentrations using a UV-1800 spectrophotometer (Shimadzu, Japan) and the colorimetric method (Norman and Stucki, 1981; Dorich and Nelson, 1983; Norman et al., 1985), and for pH using a pH meter (LeiCi PHSJ-3F, China). After extracting soil samples with distilled water (soil: water ratio was 1:5) and filtering, the electrical conductivity (EC) was determined with a conductivity meter (LeiCi DDSJ-308F, China). Soil texture was determined using a laser particle analyzer (Malvern Mastersizer 3000, UK) according to the protocol (Pieri et al., 2006).
2.2. Experimental design
2.2.1. Experiment 1: Effects of nitrate addition on deep soil respiration, microbial community structure and key functional genes responsible for C degradation
In order to determine the effect of nitrate addition on CO2 emission from deep soil, two treatments were conducted: (1) nitrate addition treatment: 15 g of fresh soil was placed in to 120 ml flasks and a KNO3 solution was added to the flasks to reach 100 mg NO3–-N kg–1 dry soil; (2) control treatment: 15 g fresh soil received an equal amount of distilled water. Moisture is reported to be the most important factor affecting SOC mineralization (Huang and Hall, 2017). Thus, three gravimetric water contents were applied: 35% (2 ml 50 mM KNO3), 70% (5 ml 20 mM KNO3), and 200% (20 ml 5 mM KNO3). There was a total of 54 flasks (2 treatments × 3 soil depths × 3 soil moisture contents × 3 replicates). All flasks were sealed with air-tight butyl rubber septa and aluminum caps. The headspace gas was alternatively evacuated (0.1 kPa) and flushed with pure helium (99.999%, 120 kPa) five times to remove O2 (Yuan et al., 2019), the initial O2 concentrations was 35.5 μmol L–1 at this time. All flasks were incubated at 20°C (Fontaine et al., 2007; Condron et al., 2014) in the dark for 55 days.
During the incubation, the headspace CO2 concentrations were periodically determined using a robotized sampling and analysis system (Molstad et al., 2007). Briefly, the robotized system consisted of an incubation system linked with a gas collection and analysis system. It enabled sampling of the headspace gas by puncturing the butyl rubber septa of the anaerobic flasks and then pumping of 2 ml sample gas through the loop of the GC with a peristaltic pump. An electron capture detector (ECD) was used for determination of N2O and a thermal conductivity detector (TCD) was used to measure CO2, O2 and N2.
At the end of the 70% water content incubation, soil samples from the nitrate addition and control treatments, for each soil depth, were collected to determine the soil microbial community structure, and the key functional genes responsible for C degradation. Soil microbial DNA was extracted from these samples using the PowerSoil DNA isolation kit (MoBio, Carlsbad, CA) according to the manufacturer’s instructions. V3-V4 variable region of the 16S rRNA gene were amplified with primers 338F (ACTCCTACGGGAGGCAGCAG)/806R (GGACTACHVGGGTWTCTAAT). The sequencing operation was completed by Beijing Allwegene technology Co., Ltd. (Beijing, China). Sample sequences were clustered with a threshold of 97% similarity to obtain representative operational taxonomic units (OTUs). Paired-end sequencing was performed using an Illumina Miseq PE300 platform (Wu et al., 2019).
The total RNA was extracted from 1 g soil samples using the RNA Extraction Kit (Tiangen Biochemical Science Technologies Co., Ltd., Beijing, China) according to the manufacturer’s protocols. The RNA concentration and purity were determined using an ND-2000 spectrophotometer (Thermo Scientific), then RNA integrity was assessed using a Tanon 1600 imaging system (Tanon Science and Technology Co., Ltd., Shanghai, China). The primers were synthesized by Invitrogen (Shanghai, China), subsequently, RNA was converted to cDNA using the Prime Script™ RT reagent Kit with gDNA Eraser (TaKaRa). Then quantitative Real-Time PCR (qRT-PCR) was performed using an ABI7500 quantitative PCR system (Applied Biosystems, USA) with each sample conducted in triplicate. The relative abundances of genes responsible for the degradation of starch, hemicellulose, cellulose, chitin, aromatics, lignin and lignin from labile to recalcitrant (amyA, arA, cbhI, chi, AceB, lip, and laccase-like multi-copper oxidase (Lmco), respectively) were determined using the 2–ΔΔCt method (Wang et al., 2019), with the 16S rRNA gene used as an internal reference gene, the denitrification genes for qRT-PCR were narG, nirK, and nosZ genes. The primer sequences of qRT-PCR are presented in Supplementary Tables 1, 2.
2.2.2. Experiment 2: Effects of supplemental amount of nitrate on deep soil CO2 emissions
We further tested whether the increase in soil CO2 emissions was linearly correlated with the supplemental rate of nitrate addition, using the soil sample from 2.0 to 2.2 m depth with a 70% water content, including the subsequent experiments. The reason for continuing using 2.0–2.2 m depth was the higher level of nitrate concentration in this layer than other layers and the reason for continuing using 70% moisture content was more reasonable and a real condition to explore the mechanism. Five nitrate levels were applied: 0, 10, 20, 50, and 100 mg NO3–-N kg–1 dry soil. Each level was replicated three times and flasks were incubated in the dark at 20°C for 98 days. The CO2 concentration was determined every 7 days and analysis methods were identical to that used in Experiment 1.
2.2.3. Experiment 3: Effects of ammonium addition on deep soil CO2 emissions
We further tested if other inorganic N types beside nitrate, e.g., ammonium, could promote deep soil CO2 emissions. Three treatments were applied, (1) nitrate addition treatment: 15 g fresh soil of the 2.0–2.2 m depth was cultured in 120 ml flasks with 5 ml of 20 mM KNO3 (the final nitrate content was 100 mg NO3–-N kg–1 dry soil); (2) ammonium addition treatment: 15 g fresh soil of the 2.0–2.2 m depth was cultured in 120 ml flasks with 5 ml of 20 mM NH4Cl (the final ammonium content was 100 mg NH4+-N kg–1 dry soil); (3) control treatment: 15 g fresh soil of the 2.0–2.2 m depth received 5 ml of distilled water. The flasks were incubated in the dark at 20°C for 98 days and headspace gas sampling occurred every 7 days.
2.2.4. Experiment 4: Effects of O2 level on the stimulation of nitrate on deep soil CO2 emissions
We further determined if nitrate acted as an alternative electron acceptor to O2 in stimulating deep soil CO2 emission. The initial O2 concentration in the flasks was set at 1% by volume. During the incubation, the O2 concentration was expected to gradually decrease. Two treatments were set up: (1) 1% O2 treatment: 15 g fresh soil from the 2.0 to 2.2 m depth was incubated with 5 ml of distilled water in 120 ml flasks; (2) 1% O2 + NO3– treatment: 15 g fresh soil from the 2.0 to 2.2 m depth was incubated with 5 ml of 20 mM KNO3 solution in 120 ml flasks. The headspace of the flasks was alternatively evacuated (0.1 kPa) and re-flushed with high-purity helium/O2 mixture gas (1% O2 and 99% helium, 120 kPa) five times, the initial O2 concentrations was 565 μmol L–1 at this time, and supplemented with 1% O2 again when O2 concentrations declined below 100 μmol L–1. A total of 96 flasks were prepared (48 flasks for each treatment) and incubated under dark conditions at 20°C for 98 days. At the beginning of the incubation, three flasks from each treatment were randomly selected for periodically determining the headspace O2 and CO2 concentrations at a frequency of four measurements per month, using the robotized sampling and analyzing system as noted above in Experiment 1. To calculate the stoichiometric mole ratio of nitrate and oxygen when nitrate was used as an electron acceptor, during the incubation, three flasks of each treatment were randomly selected each week to destructively sample the soil for determining the nitrate concentrations.
2.2.5. Experiment 5: Effects of nitrate addition on microbial biomass N and C contents, MAOC, and redox potential in deep soil
We further tested whether the promotion of microbial respiration by nitrate would stimulate microbial proliferation and consequently increase the microbial utilization of on MAOC in deep soil. Two treatments were conducted: (1) 15 g soil samples from the 2.0 to 2.2 m depth were incubated with 5 ml of 20 mM KNO3; (2) 15 g soil samples from the 2.0 to 2.2 m depth were incubated with 5 ml of distilled water. A total of 54 flasks (27 flasks for each treatment) were prepared and their headspaces were exchanged with high-purity helium as described in Experiment 1. The flasks were incubated in the dark at 20°C for 98 days. Three flasks for each treatment were randomly selected every 14-day for destructive sampling to determine the MAOC content using the citrate-bicarbonate-dithionite (CBD) method (Lalonde et al., 2012). At the end of the incubation, three flasks from each treatment were used to measure the microbial biomass carbon (MBC) and nitrogen (MBN) using the fumigation-extraction method (Vance et al., 1987) and perform 16S DNA gene copy numbers together to estimate microbial proliferation. The last three flasks for each treatment were used to measure soil redox potential (Eh) using an Eh meter (Model HLY-216, China) and pH by using the probe inserted into the soil.
2.3. Statistical analysis
The statistical package SPSS 24.0 (SPSS Inc., Chicago, IL, USA) was used to perform all statistical analysis. Analysis of variance (ANOVA) was used to determine the difference (P < 0.05) among treatments after the Shapiro–Wilk and Levene tests were used to confirm the normality and homogeneity of the data.
3. Results and discussion
3.1. Nitrate addition promote microbial respiration in deep soil by acting as an alternative electron acceptor to O2
The results of Experiment 1 showed that there was little difference in the cumulative CO2 emissions from deep soil between the nitrate addition treatment and the control treatment during the initial 10 days of incubation (Figure 1). With increasing incubation time, the cumulative CO2 emissions differed significantly between the two treatments (Figure 1). At the end of Experiment 1 (55 days of incubation), the cumulative CO2 emissions under the nitrate addition treatment had increased 40–140% relative to the control treatment, with the increase dependent on soil water moisture content and soil depth (Supplementary Figure 1). The 200% water content significantly contributed to ΔCO2 at three depths compared to the 35 and 70% water contents, soil moisture affects the various life activities of soil microorganisms, under low soil moisture conditions microbial activity may be limited, while increased moisture could significantly enhance microbial activity, leading to an improvement in soil respiration. Compared to depths 1.5–1.7, 2.0–2.2, and 2.5–2.7 m depth had higher ΔCO2 at 35, 70, and 200% water content, reaching 60, 120, and 150%, respectively, indicating a higher sensitivity for the deeper soils. These results demonstrate that nitrate addition stimulated the microbial respiration in the deep soil depths under anaerobic conditions. Results of Experiment 2, where the increase in CO2 emission was significantly correlated (P < 0.01) with the nitrate addition rate (Figure 2), also support this.
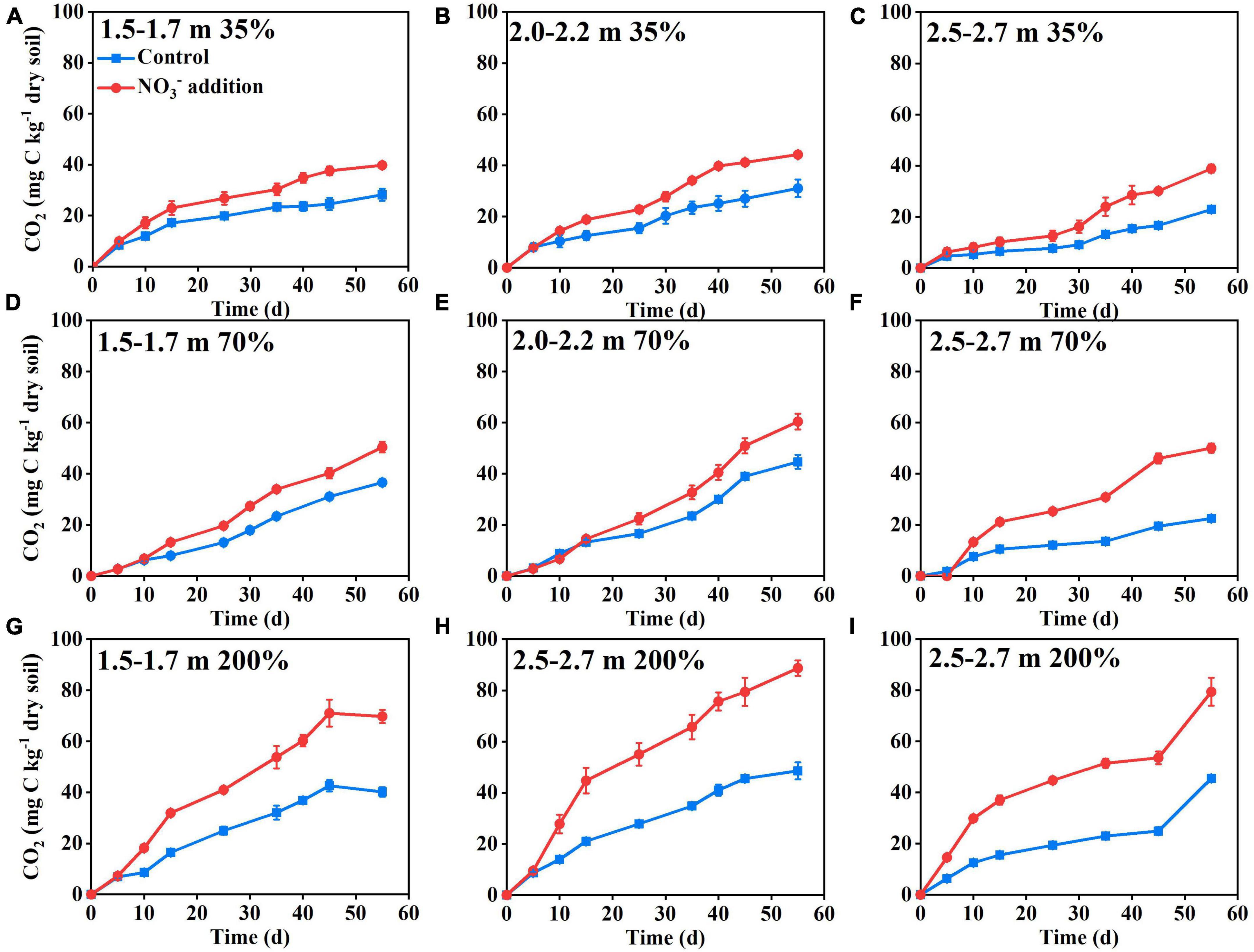
Figure 1. Nitrate addition effects on the cumulative CO2 emissions from deep soil depths: 1.5–1.7 m (A,D,G), 2.0–2.2 m (B,E,H), and 2.5–2.7 m (C,F,I) with soil gravimetric water contents of 35% (A–C), 70% (D–F), and 200% (G–I) in Experiment 1. The blue lines and red lines represent control and nitrate addition treatments, respectively. Data are shown as the mean ± standard deviation (n = 3).
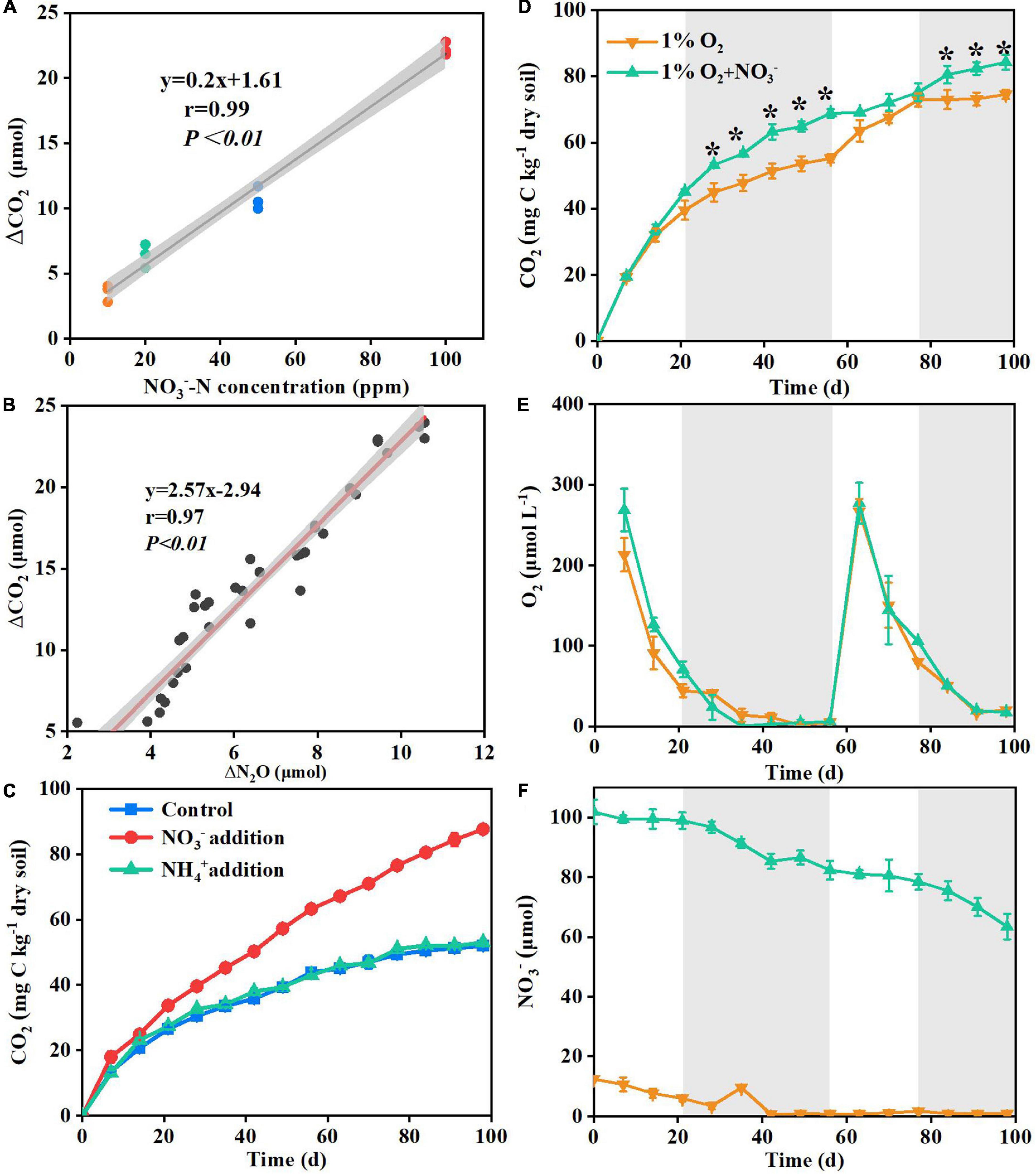
Figure 2. Correlation between the rate of the supplemented nitrate and the increasing concentration of ΔCO2 under the nitrate addition treatment in Experiment 2 (A); correlation between the increasing amounts of the produced ΔCO2 and ΔN2O at 100 ppm NO3–-N treatment in Experiment 2 (B); ammonium versus nitrate addition effects on the cumulative CO2 emissions from deep soil in Experiment 3 (C); dynamics of CO2 (D), O2 (E) and NO3– (F) concentrations in flasks under 1% O2 and 1% O2 + NO3– treatments in Experiment 4. Delta indicates the value of nitrate addition treatment minus non-nitrate control within each sampling day. The gray areas in panels (A,B) indicate 95% confidence intervals. The gray areas in panels (D–F) indicate anaerobic conditions with O2 concentrations below 100 μmol L– 1. Asterisk denotes significant difference (P < 0.05) between the two treatments. Data are shown as the mean ± standard deviation (n = 3).
There was a lag in the CO2 emission response to nitrate addition during the incubation (Figure 1). This lag was probably caused by the residual O2 in the soil pores which removed the need for nitrate to be used as an alternative electron acceptor (Parkin and Tiedje, 1984; Song et al., 2019). This was tested by monitoring the responses of soil respiration to varying O2 concentration. The results showed that the promoting effects of nitrate addition on soil respiration appeared when the headspace O2 concentration was below 100 μmol L–1, then disappeared after the injection of additional O2, and finally re-appeared after the O2 concentration was again below 100 μmol L–1 (Figure 2). By calculation, we found that the role of nitrate was activated when the stoichiometric mole ratio of nitrate to O2 exceeded 6:1 (77.4 μmol/12.7 μmol). Further evidence supporting the effect of nitrate in promoting soil respiration was the mole ratio of the CO2 to N2O produced under the nitrate addition treatment, which was 2.57:1 and close to the theoretical mole ratio 2:1 (Mørkved et al., 2006) when nitrate is used as an electron acceptor for microbial respiration (Figure 2).
Apart from acting as an alternative electron acceptor for microbial respiration, nitrate is a key N source for soil microbes (Geisseler et al., 2010; Wang et al., 2015). Previous studies have shown that N addition can promote surface soil respiration by serving as a nutrient (Soong et al., 2018). In order to test whether the positive effects of nitrate on soil respiration were caused as the result of enhanced N supply, equal amounts of nitrate-N or ammonium-N were added into the 2.0–2.2 m depth soil in Experiment 3. The results showed that, contrary to the nitrate-N treatment, the ammonium-N treatment did not significantly increase soil respiration (Figure 2). These results indicated that deep soil respiration could not be facilitated by merely changing the soil N status without acting as an electron acceptor. Briefly, above results suggested that the positive effects of nitrate on deep soil respiration were the result of it acting as an electron acceptor.
3.2. The enhancement of microbial respiration by nitrate promotes microbial growth and consequently destabilize MAOC in deep soil
The increase in microbial access to an electron acceptor for respiration following nitrate addition tends to promote microbial assimilation and reproduction (Dyckmans et al., 2006). As Figure 3 shows, the soil ammonium concentration did not change significantly (P > 0.05) between the beginning and end of the incubations, which indicated that dissimilatory nitrate reduction to ammonium (DNRA) was negligible. In addition, study showed that DNRA may be a minimal pathway at high nitrate concentrations (Handler et al., 2022), it is generally believed that low nitrogen and high carbon will tilt the balance to DNRA (Van Den Berg et al., 2016; Pandey et al., 2020; Wei et al., 2022), the opposite is the high nitrogen and lower carbon contents in this study. Denitrification was the main nitrate reduction pathway, the amount of nitrate consumed (65 μmol N) was significantly larger than the cumulative amount of the N2O plus N2 (31 μmol N) produced by day 98 (Experiment 2). This indicates that about ∼50% of the added nitrate could have been assimilated by microbes for cell proliferation. In deep soils, microorganisms are expected to be in short supply of both C and N, because the microbial available C and N species, such as glucose, nitrate and ammonia, generally decrease sharply from the surface soil to deep soil. Consequently, the nitrate addition is expected to relief the microbial N starvation in deep soil and in turn promote the microbial growth there. In support of this were the measured increases (P < 0.05) in microbial DNA concentration (Supplementary Figure 2), MBC and MBN, and 16S DNA gene copy numbers (Figure 4) under nitrate addition relative to the control treatment at the end of Experiment 1 and 5. Thus, nitrate addition promoted microbial growth.
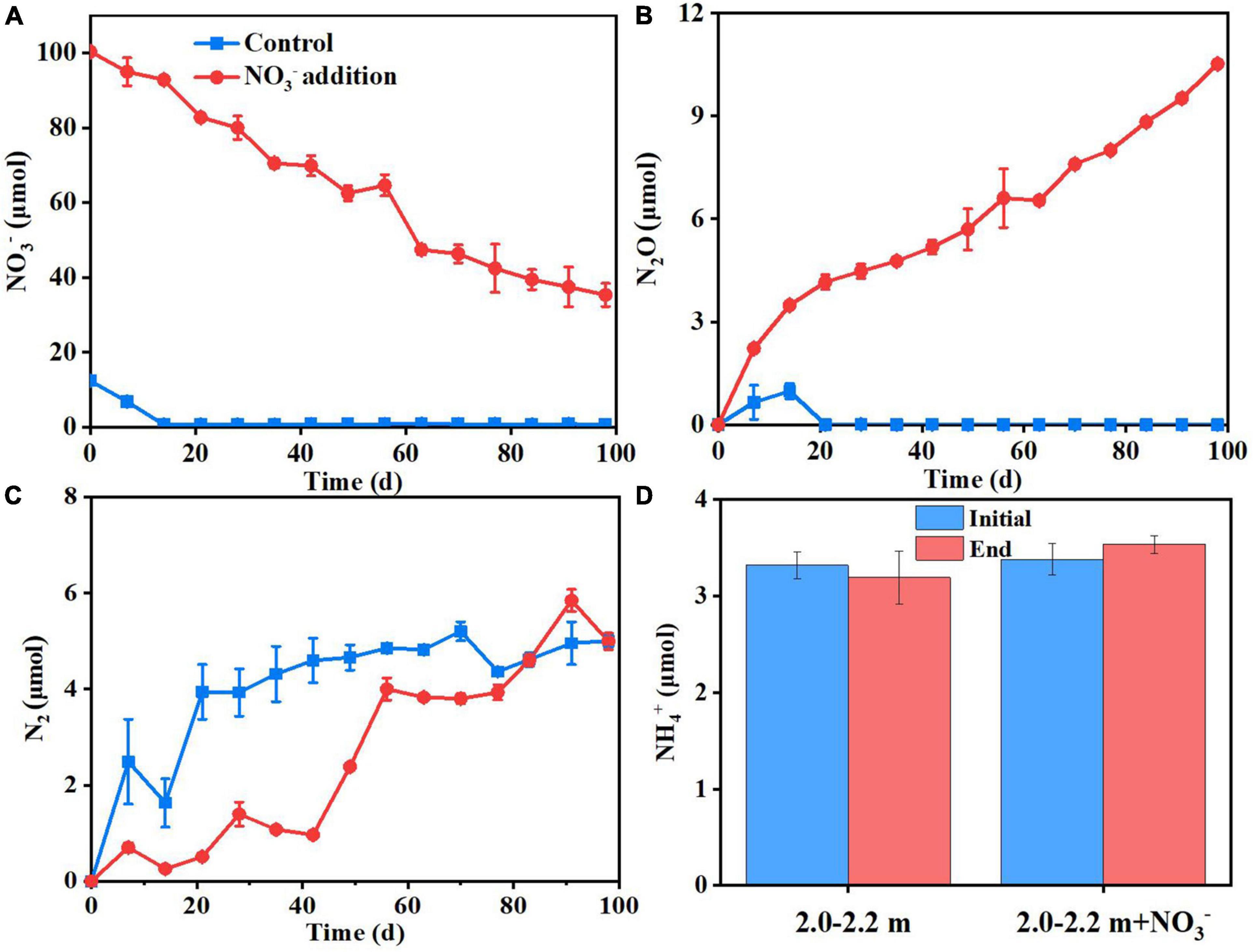
Figure 3. Dynamics of NO3– (A), N2O (B), and N2 (C) concentrations in flasks under control and nitrate addition treatments. NH4+ concentrations at initial and end of the incubation under two treatments (D).
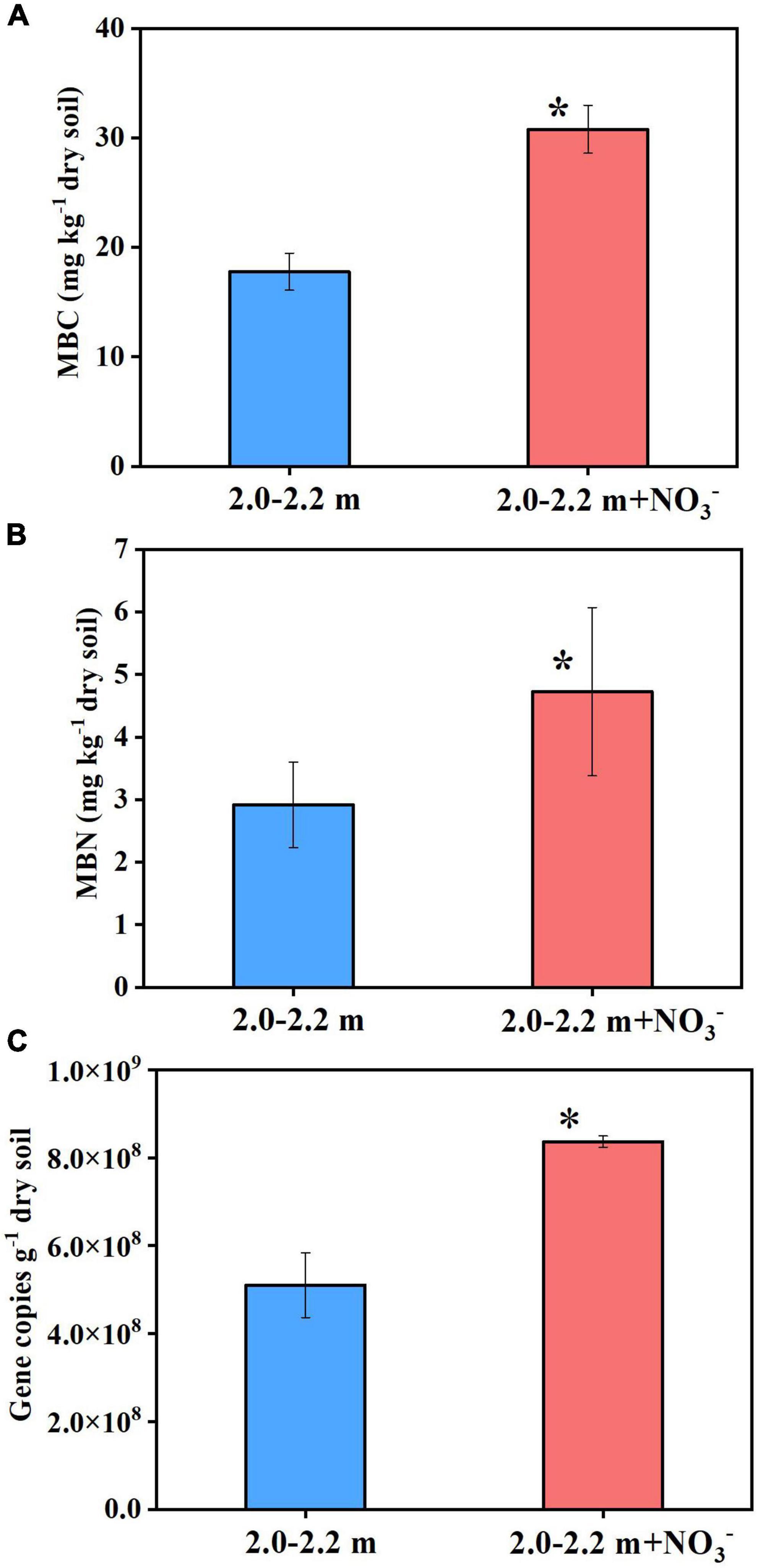
Figure 4. Nitrate addition effects on the MBC (A), MBN (B), and 16S DNA gene copies (C) in deep soil after 98 days at the end of Experiment 5. Data are shown as the mean ± standard deviation (n = 3). Asterisk denotes significant difference (P < 0.05) between the two treatments.
Apart from increasing microbial biomass, the nitrate addition treatment in Experiment 1 also significantly changed the soil microbial community composition (Supplementary Figure 2). Compared with the control treatment, nitrate addition significantly increased the relative abundances of Bacillus, Aquabacterium, Sediminibacterium, and Acidibacter at the genus level across all depths, and Caproiciproducens at 1.5–1.7 and 2.0–2.2 m (Supplementary Figure 3). It has been suggested that Bacillus and Aquabacterium contribute to denitrification in terrestrial and possibly other ecosystems (Verbaendert et al., 2011; Zhang et al., 2016). In addition, Bacillus and Aquabacterium were previously reported to play a key role in accelerating SOC decomposition (Lin et al., 2019; Yin et al., 2019). Caproiciproducens genus could accelerate the use of carbon sources for conversion to CO2 (Kim et al., 2015). In this study, the amounts of CO2 and N2O emitted were correlated with the relative abundances of Bacillus, Aquabacterium and Sediminibacterium (Supplementary Figure 4), indicating that these microbes could have contributed to the positive effects of nitrate addition on deep soil respiration. In addition, the expressions of functional genes of narG and nirK under the nitrate addition treatment was significantly (P < 0.05) higher than control treatment, while the nosZ gene was not significantly different between the two treatments (P > 0.05) at 2.0–2.2 and 2.5–2.7 m depths except for a decrease for the nitrate addition treatment at 1.5 m depth (Figure 5).
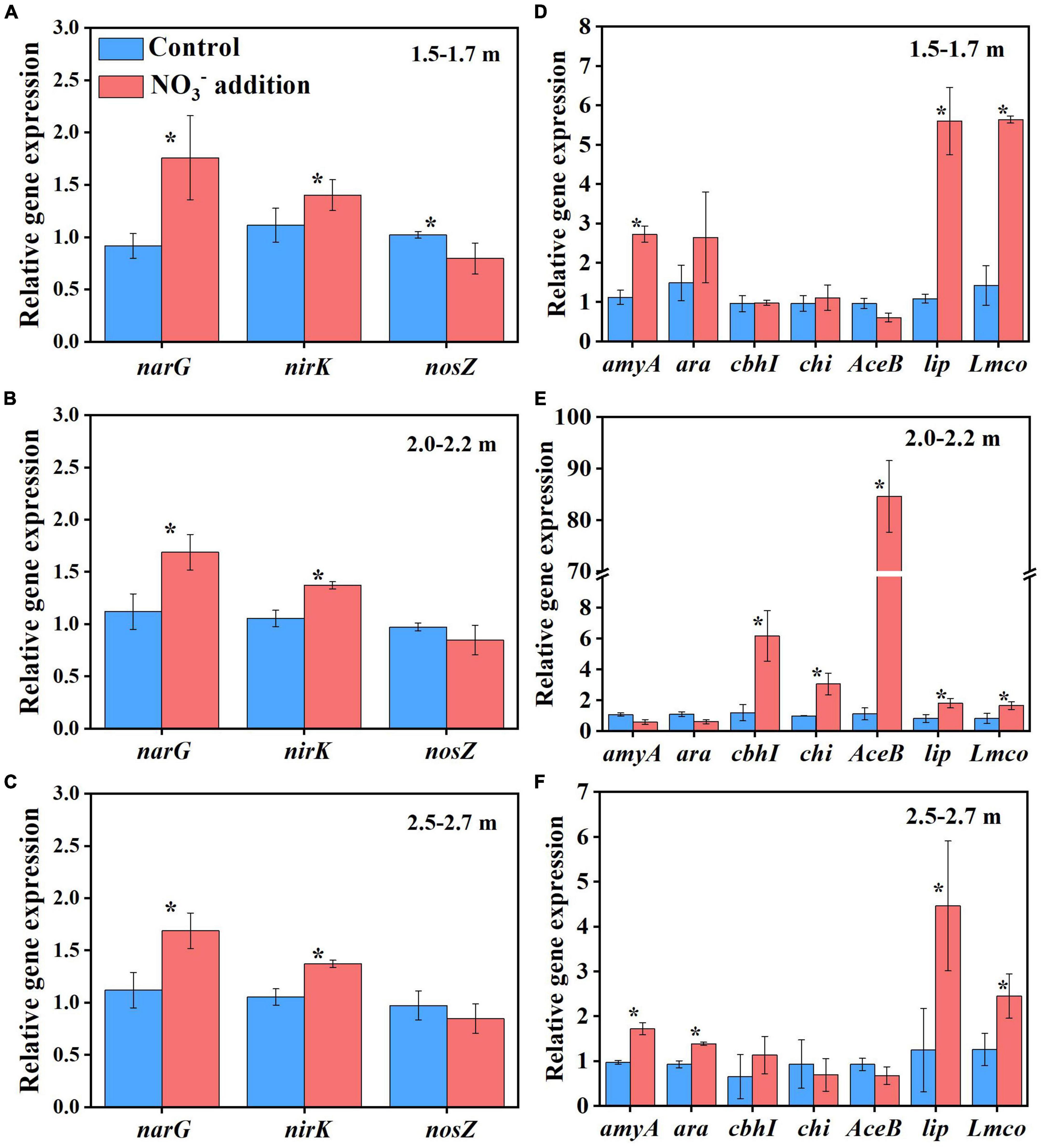
Figure 5. Nitrate addition effects on the relative gene abundances of denitrification genes (narG, nirK, and nosZ) and key and recognized C degradation genes (amyA, arA, cbhI, chi, AceB, lip, and Lmco) in deep soil depths of 1.5–1.7 m (A,D), 2.0–2.2 m (B,E), and 2.5–2.7 m (C,F) after 55 days at the end of Experiment 1. Data are shown as the mean ± standard deviations (n = 3). Asterisk denotes significant difference (P < 0.05) between the two treatments.
The SOC in deep soils is generally bound to soil minerals, which protect SOC from microbial attack (Han et al., 2016; Gartzia-Bengoetxea et al., 2020). Previously, it was reported that the fluctuation of pH and Eh may also cause solubilization of MAOC, with the solubilization rapidly activated when the Eh decreased below 150 mV (Grybos et al., 2009). In this study, the pH and Eh were not significantly different (P > 0.05) between the control treatment (5.09 ± 0.07 and 156.67 ± 4.04 mV) and the nitrate addition treatment (5.15 ± 0.06 and 153.00 ± 4.36 mV) at the end of incubation (Supplementary Figure 5), indicating that the pH and Eh were not responsible for the difference in MAOC between the two treatments. On the contrary, the expressions of functional genes typically responsible for carbon decomposition, such as amyA at 1.5–1.7 and 2.5–2.7 m, AceB at 2.0–2.2 m and lip and Lmco at all three depths were significantly greater (P < 0.05) under the nitrate addition treatment than under the control treatment at the end of Experiment 1 (Figure 5). The increases in carbon decomposer abundance, as noted above, and their functional gene expression were previously reported to increase the microbial utilization of MAOC (Li H. et al., 2021). Our results show that the content of the MAOC under the nitrate addition treatment was significantly lower than that under the control treatment from as early as day 28 of the incubation, and the ratio of MAOC/SOC decreased from 20% before incubation to 4% at the end of incubation (Figure 6), which is in accordance with previous studies reporting that N addition not only modified the composition and abundance of bacteria, but also decreased the MAOC complexes (Qin et al., 2020; Li J. et al., 2021). These results indicate that the increase in microbial utilization of MAOC, under the nitrate addition treatment, destabilizes the MAOC.
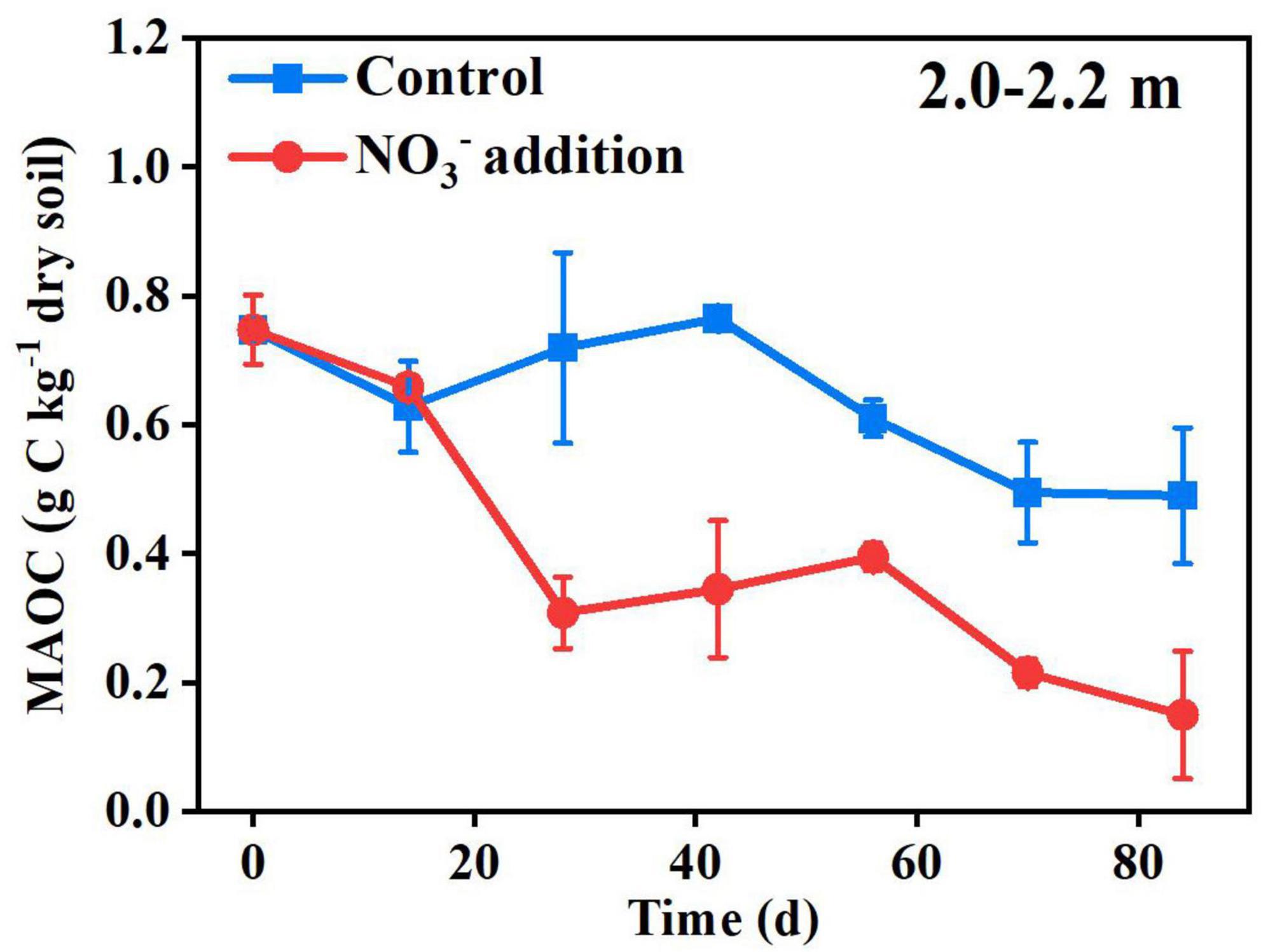
Figure 6. Nitrate addition effects on the content of MAOC in the 2.0–2.2 m depth over time in Experiment 5. Data are shown as the mean ± standard deviations (n = 3).
4. Conclusion
This study demonstrated that nitrate acted as an alternative electron acceptor to O2 for microbial respiration and consequently promoted the growth of SOC decomposers in deep soil (depths > 1 m). The increases in SOC decomposer abundances and functional genes known to align with SOC decomposition in turn increased the microbial utilization of the MAOC, resulting in the acceleration of SOC decomposition in deep soil. Our results have implications for understanding the contribution of deep SOC to atmospheric CO2 in response to anthropogenic reactive N enrichment of the environment. According to the results of this study, increased nitrate leaching under anaerobic conditions will enhance the decomposition of MAOC in deep soil. Since the promoting effects of nitrate on soil respiration is derived from its role as alternative respiration acceptor to O2, the potential of nitrate to destabilize MAOC is expected to be favored in deep soils with clay texture and higher water content. Consequently, reducing nitrate leaching will assist in preserving MAOC in deep soil.
Data availability statement
The data presented in this study are deposited in the NCBI repository, accession number: PRJNA911917.
Author contributions
WS: methodology, visualization, formal analysis, and writing – original draft. CH, YL, TC, NW-M, TG, and JL: review and editing. SZ: supervision and review and editing. SQ: funding acquisition, conceptualization, and writing – review and editing. All authors read and approved the final manuscript.
Funding
This work was supported by the National Key R&D Program of China (2021YFD1500400), the National Natural Science Foundation of Hebei Province (D2022503014), and the National Natural Science Foundation of China (No. 41771331).
Conflict of interest
JL was employed by AgResearch Ltd.
The remaining authors declare that the research was conducted in the absence of any commercial or financial relationships that could be construed as a potential conflict of interest.
Publisher’s note
All claims expressed in this article are solely those of the authors and do not necessarily represent those of their affiliated organizations, or those of the publisher, the editors and the reviewers. Any product that may be evaluated in this article, or claim that may be made by its manufacturer, is not guaranteed or endorsed by the publisher.
Supplementary material
The Supplementary Material for this article can be found online at: https://www.frontiersin.org/articles/10.3389/fmicb.2023.1120466/full#supplementary-material
References
Bond-Lamberty, B., and Thomson, A. (2010). A global database of soil respiration data. Biogeosciences 7, 1915–1926. doi: 10.5194/bg-7-1915-2010
Bulseco, A. N., Giblin, A. E., Tucker, J., Murphy, A. E., Sanderman, J., Hiller-Bittrolff, K., et al. (2019). Nitrate addition stimulates microbial decomposition of organic matter in salt marsh sediments. Glob. Chang. Biol. 25, 3224–3241. doi: 10.1111/gcb.14726
Chen, Y., Liu, X., Hou, Y., Zhou, S., and Zhu, B. (2021). Particulate organic carbon is more vulnerable to nitrogen addition than mineral-associated organic carbon in soil of an alpine meadow. Plant Soil 458, 93–103. doi: 10.1007/s11104-019-04279-4
Condron, L. M., Hopkins, D. W., Gregorich, E. G., Black, A., and Wakelin, S. A. (2014). Long-term irrigation effects on soil organic matter under temperate grazed pasture. Eur. J. Soil Sci. 65, 741–750. doi: 10.1111/ejss.12164
Dorich, R., and Nelson, D. (1983). Direct colorimetric measurement of ammonium in potassium chloride extracts of soils. Soil Sci. Soc. Am. J. 47, 833–836. doi: 10.2136/sssaj1983.03615995004700040042x
Dyckmans, J., Flessa, H., Lipski, A., Potthoff, M., and Beese, F. (2006). Microbial biomass and activity under oxic and anoxic conditions as affected by nitrate additions. J. Plant Nutr. Soil Sci. 169, 108–115. doi: 10.1002/jpln.200521735
Feng, X., Qin, S., Zhang, D., Chen, P., Hu, J., Wang, G., et al. (2022). Nitrogen input enhances microbial carbon use efficiency by altering plant-microbe-mineral interactions. Glob. Chang. Biol. 28, 4845–4860. doi: 10.1111/gcb.16229
Fontaine, S., Barot, S., Barre, P., Bdioui, N., Mary, B., and Rumpel, C. (2007). Stability of organic carbon in deep soil layers controlled by fresh carbon supply. Nature 450, 277–280. doi: 10.1038/nature06275
Fowler, D., Steadman, C. E., Stevenson, D., Coyle, M., Rees, R. M., Skiba, U. M., et al. (2015). Effects of global change during the 21st century on the nitrogen cycle. Atmos. Chem. Phys. 15, 13849–13893. doi: 10.5194/acp-15-13849-2015
Friedlingstein, P., O’sullivan, M., Jones, M. W., Andrew, R. M., Hauck, J., Olsen, A., et al. (2020). Global carbon budget 2020. Earth Syst. Sci. Data 12, 3269–3340. doi: 10.5194/essd-12-3269-2020
Galloway, J. N., Townsend, A. R., Erisman, J. W., Bekunda, M., Cai, Z., Freney, J. R., et al. (2008). Transformation of the nitrogen cycle: Recent trends, questions, and potential solutions. Science 320, 889–892. doi: 10.1126/science.1136674
Gao, J., Wang, S., Li, Z., Wang, L., Chen, Z., and Zhou, J. (2021). High nitrate accumulation in the vadose zone after land-use change from croplands to orchards. Environ. Sci. Technol. 55, 5782–5790. doi: 10.1021/acs.est.0c06730
Gartzia-Bengoetxea, N., Virto, I., Arias-Gonzalez, A., Enrique, A., Fernandez-Ugalde, O., and Barre, P. (2020). Mineral control of organic carbon storage in acid temperate forest soils in the Basque Country. Geoderma 358:113998. doi: 10.1016/j.geoderma.2019.113998
Geisseler, D., Horwath, W. R., Joergensen, R. G., and Ludwig, B. (2010). Pathways of nitrogen utilization by soil microorganisms–a review. Soil Biol. Biochem. 42, 2058–2067. doi: 10.1016/j.soilbio.2010.08.021
Giblin, A. E., Tobias, C. R., Song, B., Weston, N., Banta, G. T., and Rivera-Monroy, V. H. (2013). The importance of dissimilatory nitrate reduction to ammonium (DNRA) in the nitrogen cycle of coastal ecosystems. Oceanography 26, 124–131. doi: 10.5670/oceanog.2013.54
Grybos, M., Davranche, M., Gruau, G., Petitjean, P., and Pedrot, M. (2009). Increasing pH drives organic matter solubilization from wetland soils under reducing conditions. Geoderma 154, 13–19. doi: 10.1016/j.geoderma.2009.09.001
Han, L., Sun, K., Jin, J., and Xing, B. (2016). Some concepts of soil organic carbon characteristics and mineral interaction from a review of literature. Soil Biol. Biochem. 94, 107–121. doi: 10.1016/j.soilbio.2015.11.023
Handler, A. M., Suchy, A. K., and Grimm, N. B. (2022). Denitrification and DNRA in urban accidental wetlands in phoenix, Arizona. J. Geophys. Res. Biogeosci. 127:e2021JG006552. doi: 10.1029/2021JG006552
Huang, W., and Hall, S. J. (2017). Elevated moisture stimulates carbon loss from mineral soils by releasing protected organic matter. Nat. Commun. 8:1774. doi: 10.1038/s41467-017-01998-z
Jilling, A., Keiluweit, M., Gutknecht, J. L. M., and Grandy, A. S. (2021). Priming mechanisms providing plants and microbes access to mineral-associated organic matter. Soil Biol. Biochem. 158:108265. doi: 10.1016/j.soilbio.2021.108265
Kautz, T. (2015). Research on subsoil biopores and their functions in organically managed soils: A review. Renew. Agr. Food Syst. 30, 318–327.
Kim, B. C., Jeon, B. S., Kim, S., Kim, H., Um, Y., and Sang, B. I. (2015). Caproiciproducens galactitolivorans gen. nov., sp. nov., a bacterium capable of producing caproic acid from galactitol, isolated from a wastewater treatment plant. Int. J. Syst. Evol. Microbiol. 65, 4902–4908. doi: 10.1099/ijsem.0.000665
Lal, R. (2004). Soil carbon sequestration impacts on global climate change and food security. Science 304, 1623–1627. doi: 10.1126/science.1097396
Lalonde, K., Mucci, A., Ouellet, A., and Gelinas, Y. (2012). Preservation of organic matter in sediments promoted by iron. Nature 483, 198–200. doi: 10.1038/nature10855
Laursen, A. E., and Seitzinger, S. P. (2002). The role of denitrification in nitrogen removal and carbon mineralization in Mid-Atlantic Bight sediments. Cont. Shelf Res. 22, 1397–1416.
Li, H., BöLscher, T., Winnick, M., Tfaily, M. M., Cardon, Z. G., and Keiluweit, M. (2021). Simple plant and microbial exudates destabilize mineral-associated organic matter via multiple pathways. Environ. Sci. Technol. 55, 3389–3398. doi: 10.1021/acs.est.0c04592
Li, J., Cheng, B., Zhang, R., Li, W., Shi, X., Han, Y., et al. (2021). Nitrogen and phosphorus additions accelerate decomposition of slow carbon pool and lower total soil organic carbon pool in alpine meadows. Land Degrad. Dev. 32, 1761–1772. doi: 10.1002/ldr.3824
Li, Y., Song, C., Hou, C., and Wang, X. (2014). Effects of nitrogen addition on carbon mineralization in boreal peatlands soil in northeast China: A laboratory study. Fresenius Environ. Bull. 23, 970–975.
Lin, Y., Ye, G., Kuzyakov, Y., Liu, D., Fan, J., and Ding, W. (2019). Long-term manure application increases soil organic matter and aggregation, and alters microbial community structure and keystone taxa. Soil Biol. Biochem. 134, 187–196. doi: 10.1016/j.soilbio.2019.03.030
Lu, M., Zhou, X., Luo, Y., Yang, Y., Fang, C., Chen, J., et al. (2011). Minor stimulation of soil carbon storage by nitrogen addition: A meta-analysis. Agric. Ecosyst. Environ. 140, 234–244. doi: 10.1016/j.agee.2010.12.010
Mazzoncini, M., Sapkota, T. B., Barberi, P., Antichi, D., and Risaliti, R. (2011). Long-term effect of tillage, nitrogen fertilization and cover crops on soil organic carbon and total nitrogen content. Soil Till. Res. 114, 165–174. doi: 10.1016/j.still.2011.05.001
Mo, J., Zhang, W., Zhu, W., Gundersen, P., Fang, Y., Li, D., et al. (2008). Nitrogen addition reduces soil respiration in a mature tropical forest in southern China. Glob. Chang. Biol. 14, 403–412. doi: 10.1111/j.1365-2486.2007.01503.x
Molstad, L., Dörsch, P., and Bakken, L. R. (2007). Robotized incubation system for monitoring gases (O2, NO, N2O N2) in denitrifying cultures. J. Microbiol. Meth. 71, 202–211. doi: 10.1016/j.mimet.2007.08.011
Mørkved, P. T., Dörsch, P., Henriksen, T. M., and Bakken, L. R. (2006). N2O emissions and product ratios of nitrification and denitrification as affected by freezing and thawing. Soil Biol. Biochem. 38, 3411–3420. doi: 10.1016/j.soilbio.2006.05.015
Norman, R. J., and Stucki, J. W. (1981). The determination of nitrate and nitrite in soil extracts by ultraviolet spectrophotometry. Soil Sci. Soc. Am. J. 45, 347–353. doi: 10.2136/sssaj1981.03615995004500020024x
Norman, R. J., Edberg, J. C., and Stucki, J. W. (1985). Determination of nitrate in soil extracts by dual-wavelength ultraviolet spectrophotometry. Soil Sci. Soc. Am. J. 49, 1182–1185. doi: 10.2136/sssaj1985.03615995004900050022x
Oertel, C., Matschullat, J., Zurba, K., Zimmermann, F., and Erasmi, S. (2016). Greenhouse gas emissions from soils—A review. Geochemistry 76, 327–352. doi: 10.1016/j.chemer.2016.04.002
Orem, W., Gilmour, C., Axelrad, D., Krabbenhoft, D., Scheidt, D., Kalla, P., et al. (2011). Sulfur in the South Florida ecosystem: Distribution, sources, biogeochemistry, impacts, and management for restoration. Crit. Rev. Environ. Sci. Technol. 41, 249–288. doi: 10.1080/10643389.2010.531201
Pandey, C., Kumar, U., Kaviraj, M., Minick, K., Mishra, A., and Singh, J. (2020). DNRA: A short-circuit in biological N-cycling to conserve nitrogen in terrestrial ecosystems. Sci. Total Environ. 738:139710. doi: 10.1016/j.scitotenv.2020.139710
Parkin, T. B., and Tiedje, J. M. (1984). Application of a soil core method to investigate the effect of oxygen concentration on denitrification. Soil Biol. Biochem. 16, 331–334. doi: 10.1016/0038-0717(84)90027-0
Penuelas, J., Janssens, I. A., Ciais, P., Obersteiner, M., and Sardans, J. (2020). Anthropogenic global shifts in biospheric N and P concentrations and ratios and their impacts on biodiversity, ecosystem productivity, food security, and human health. Glob. Chang. Biol. 26, 1962–1985. doi: 10.1111/gcb.14981
Philben, M., Zheng, J., Bill, M., Heikoop, J., Perkins, G., Yang, Z., et al. (2019). Stimulation of anaerobic organic matter decomposition by subsurface organic N addition in tundra soils. Soil Biol. Biochem. 130, 195–204. doi: 10.1016/j.soilbio.2018.12.009
Pieri, L., Bittelli, M., and Pisa, P. R. (2006). Laser diffraction, transmission electron microscopy and image analysis to evaluate a bimodal Gaussian model for particle size distribution in soils. Geoderma 135, 118–132. doi: 10.1016/j.geoderma.2005.11.009
Poirier, V., Roumet, C., and Munson, A. D. (2018). The root of the matter: Linking root traits and soil organic matter stabilization processes. Soil Biol. Biochem. 120, 246–259. doi: 10.1016/j.soilbio.2018.02.016
Qin, L., Tian, W., Yang, L., Freeman, C., and Jiang, M. (2020). Nitrogen availability influences microbial reduction of ferrihydrite-organic carbon with substantial implications for exports of iron and carbon from peatlands. Appl. Soil Ecol. 153:103637. doi: 10.1016/j.apsoil.2020.103637
Raich, J. W., and Potter, C. S. (1995). Global patterns of carbon-dioxide emissions from soils. Glob. Biogeochem. Cyc. 9, 23–36. doi: 10.1029/94gb02723
Riggs, C. E., and Hobbie, S. E. (2016). Mechanisms driving the soil organic matter decomposition response to nitrogen enrichment in grassland soils. Soil Biol. Biochem. 99, 54–65. doi: 10.1016/j.soilbio.2016.04.023
Riggs, C. E., Hobbie, S. E., Bach, E. M., Hofmockel, K. S., and Kazanski, C. E. (2015). Nitrogen addition changes grassland soil organic matter decomposition. Biogeochemistry 125, 203–219. doi: 10.1007/s10533-015-0123-2
Salomé, C., Nunan, N., Pouteau, V., Lerch, T. Z., and Chenu, C. (2010). Carbon dynamics in topsoil and in subsoil may be controlled by different regulatory mechanisms. Glob. Chang. Biol. 16, 416–426. doi: 10.1111/j.1365-2486.2009.01884.x
Schulte-Uebbing, L., and de Vries, W. (2018). Global-scale impacts of nitrogen deposition on tree carbon sequestration in tropical, temperate, and boreal forests: A meta-analysis. Glob. Chang. Biol. 24, 416–431. doi: 10.1111/gcb.13862
Sierra, J., and Renault, P. (1998). Temporal pattern of oxygen concentration in a hydromorphic soil. Soil Sci. Soc. Am. J. 62, 1398–1405. doi: 10.2136/sssaj1998.03615995006200050036x
Song, X., Ju, X., Topp, C. F. E., and Rees, R. M. (2019). Oxygen regulates nitrous oxide production directly in agricultural soils. Environ. Sci. Technol. 53, 12539–12547. doi: 10.1021/acs.est.9b03089
Soong, J. L., Maranon-Jimenez, S., Cotrufo, M. F., Boeckx, P., Bode, S., Guenet, B., et al. (2018). Soil microbial CNP and respiration responses to organic matter and nutrient additions: Evidence from a tropical soil incubation. Soil Biol. Biochem. 122, 141–149. doi: 10.1016/j.soilbio.2018.04.011
Stuckey, J. W., Goodwin, C., Wang, J., Kaplan, L. A., Vidal-Esquivel, P., and Beebe, T. P. Jr., et al. (2018). Impacts of hydrous manganese oxide on the retention and lability of dissolved organic matter. Geochem. Trans. 19:6. doi: 10.1186/s12932-018-0051-x
Treseder, K. K. (2008). Nitrogen additions and microbial biomass: A meta-analysis of ecosystem studies. Ecol. Lett. 11, 1111–1120. doi: 10.1111/j.1461-0248.2008.01230.x
Van Den Berg, E. M., Boleij, M., Kuenen, J. G., Kleerebezem, R., and Van Loosdrecht, M. C. (2016). DNRA and denitrification coexist over a broad range of acetate/N-NO3– ratios, in a chemostat enrichment culture. Front. Microbiol. 7:1842. doi: 10.3389/fmicb.2016.01842
Van Meter, K. J., Basu, N. B., Veenstra, J. J., and Burras, C. L. (2016). The nitrogen legacy: Emerging evidence of nitrogen accumulation in anthropogenic landscapes. Environ. Res. Lett. 11:035014. doi: 10.1088/1748-9326/11/3/035014
Vance, E. D., Brookes, P. C., and Jenkinson, D. S. (1987). An extraction method for measuring soil microbial biomass-C. Soil Biol. Biochem. 19, 703–707. doi: 10.1016/0038-0717(87)90052-6
Verbaendert, I., Boon, N., De Vos, P., and Heylen, K. (2011). Denitrification is a common feature among members of the genus Bacillus. Syst. Appl. Microbiol. 34, 385–391. doi: 10.1016/j.syapm.2011.02.003
Walker, A. P., De Kauwe, M. G., Bastos, A., Belmecheri, S., Georgiou, K., Keeling, R. F., et al. (2021). Integrating the evidence for a terrestrial carbon sink caused by increasing atmospheric CO2. New Phytol. 229, 2413–2445. doi: 10.1111/nph.16866
Wang, B., Li, H., Li, Z., Jian, L., Gao, Y., Qu, Y., et al. (2019). Maternal folic acid supplementation modulates the growth performance, muscle development and immunity of Hu sheep offspring of different litter size. J. Nutr. Biochem. 70, 194–201. doi: 10.1016/j.jnutbio.2019.05.011
Wang, M., Li, X., Wang, S., Wang, G., and Zhang, J. (2018). Patterns and controls of temperature sensitivity of soil respiration in a meadow steppe of the Songnen Plain, Northeast China. PLoS One 13:0204053. doi: 10.1371/journal.pone.0204053
Wang, Y., Cheng, S., Fang, H., Yu, G., Xu, X., Xu, M., et al. (2015). Contrasting effects of ammonium and nitrate inputs on soil CO2 emission in a subtropical coniferous plantation of southern China. Biol. Fert. Soils 51, 815–825. doi: 10.1007/s00374-015-1028-x
Wei, Z., Senbayram, M., Zhao, X., Li, C., Jin, K., Wu, M., et al. (2022). Biochar amendment alters the partitioning of nitrate reduction by significantly enhancing DNRA in a paddy field. Biochar 4, 1–18. doi: 10.1007/s42773-022-00166-x
Wu, D., Su, Y., Xi, H., Chen, X., and Xie, B. (2019). Urban and agriculturally influenced water contribute differently to the spread of antibiotic resistance genes in a mega-city river network. Water Res. 158, 11–21. doi: 10.1016/j.watres.2019.03.010
Xin, J., Liu, Y., Chen, F., Duan, Y., Wei, G., Zheng, X., et al. (2019). The missing nitrogen pieces: A critical review on the distribution, transformation, and budget of nitrogen in the vadose zone-groundwater system. Water Res. 165:114977. doi: 10.1016/j.watres.2019.114977
Xu, C., Xu, X., Ju, C., Chen, H., Wilsey, B. J., Luo, Y., et al. (2021). Long-term, amplified responses of soil organic carbon to nitrogen addition worldwide. Glob. Chang. Biol. 27, 1170–1180. doi: 10.1111/gcb.15489
Xu, M., and Shang, H. (2016). Contribution of soil respiration to the global carbon equation. J. Plant Physiol. 203, 16–28. doi: 10.1016/j.jplph.2016.08.007
Yang, S., Wu, H., Dong, Y., Zhao, X., Song, X., Yang, J., et al. (2020). Deep nitrate accumulation in a highly weathered subtropical critical zone depends on the regolith structure and planting year. Environ. Sci. Technol. 54, 13739–13747. doi: 10.1021/acs.est.0c04204
Yin, Y., Gu, J., Wang, X., Zhang, Y., Zheng, W., Chen, R., et al. (2019). Effects of rhamnolipid and Tween-80 on cellulase activities and metabolic functions of the bacterial community during chicken manure composting. Bioresour. Technol. 288:121507. doi: 10.1016/j.biortech.2019.121507
Yuan, H., Zhang, Z., Li, M., Clough, T., Wrage-Monnig, N., Qin, S., et al. (2019). Biochar’s role as an electron shuttle for mediating soil N2O emissions. Soil Biol. Biochem. 133, 94–96. doi: 10.1016/j.soilbio.2019.03.002
Zhang, X., Li, A., Szewzyk, U., and Ma, F. (2016). Improvement of biological nitrogen removal with nitrate-dependent Fe(II) oxidation bacterium Aquabacterium parvum B6 in an up-flow bioreactor for wastewater treatment. Bioresour. Technol. 219, 624–631. doi: 10.1016/j.biortech.2016.08.041
Keywords: nitrate leaching, global warming, greenhouse gas emission, MAOC, deep soil
Citation: Song W, Hu C, Luo Y, Clough TJ, Wrage-Mönnig N, Ge T, Luo J, Zhou S and Qin S (2023) Nitrate as an alternative electron acceptor destabilizes the mineral associated organic carbon in moisturized deep soil depths. Front. Microbiol. 14:1120466. doi: 10.3389/fmicb.2023.1120466
Received: 10 December 2022; Accepted: 23 January 2023;
Published: 08 February 2023.
Edited by:
Baoli Zhu, Institute of Subtropical Agriculture (CAS), ChinaReviewed by:
Chao Wang, Institute of Applied Ecology (CAS), ChinaSimon Guerrero-Cruz, Asian Institute of Technology, Thailand
Zhe Wang, Technical University of Munich, Germany
Copyright © 2023 Song, Hu, Luo, Clough, Wrage-Mönnig, Ge, Luo, Zhou and Qin. This is an open-access article distributed under the terms of the Creative Commons Attribution License (CC BY). The use, distribution or reproduction in other forums is permitted, provided the original author(s) and the copyright owner(s) are credited and that the original publication in this journal is cited, in accordance with accepted academic practice. No use, distribution or reproduction is permitted which does not comply with these terms.
*Correspondence: Shuping Qin, cWluc2h1cGluZ0BzanppYW0uYWMuY24=