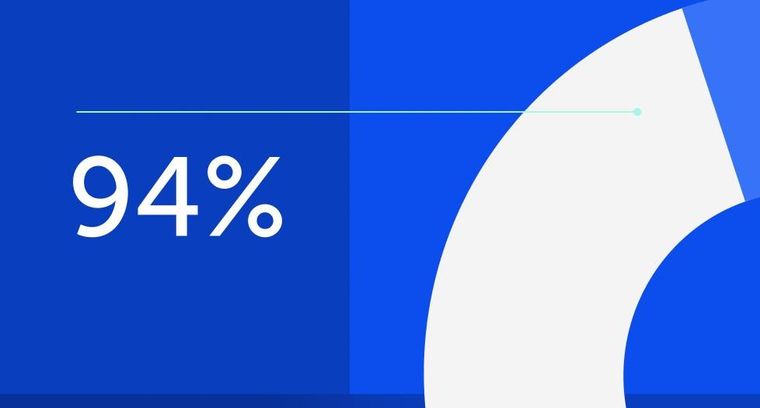
94% of researchers rate our articles as excellent or good
Learn more about the work of our research integrity team to safeguard the quality of each article we publish.
Find out more
ORIGINAL RESEARCH article
Front. Microbiol., 05 May 2023
Sec. Infectious Agents and Disease
Volume 14 - 2023 | https://doi.org/10.3389/fmicb.2023.1113642
Bacillus cereus G9241 was isolated from a welder who survived a pulmonary anthrax-like disease. Strain G9241 carries two virulence plasmids, pBCX01 and pBC210, as well as an extrachromosomal prophage, pBFH_1. pBCX01 has 99.6% sequence identity to pXO1 carried by Bacillus anthracis and encodes the tripartite anthrax toxin genes and atxA, a mammalian virulence transcriptional regulator. This work looks at how the presence of pBCX01 and temperature may affect the lifestyle of B. cereus G9241 using a transcriptomic analysis and by studying spore formation, an important part of the B. anthracis lifecycle. Here we report that pBCX01 has a stronger effect on gene transcription at the mammalian infection relevant temperature of 37°C in comparison to 25°C. At 37°C, the presence of pBCX01 appears to have a negative effect on genes involved in cell metabolism, including biosynthesis of amino acids, whilst positively affecting the transcription of many transmembrane proteins. The study of spore formation showed B. cereus G9241 sporulated rapidly in comparison to the B. cereus sensu stricto type strain ATCC 14579, particularly at 37°C. The carriage of pBCX01 did not affect this phenotype suggesting that other genetic elements were driving rapid sporulation. An unexpected finding of this study was that pBFH_1 is highly expressed at 37°C in comparison to 25°C and pBFH_1 expression leads to the production of Siphoviridae-like phage particles in the supernatant of B. cereus G9241. This study provides an insight on how the extrachromosomal genetic elements in B. cereus G9241 has an influence in bacterial phenotypes.
The Bacillus cereus sensu lato group contains bacterial species from diverse ecological niches and life styles (Jensen et al., 2003). All members of the group, which includes Bacillus anthracis, Bacillus cereus, and Bacillus thuringiensis amongst other ecologically diverse species, have a similar chromosomal genetic composition. It is clear that the distinct phenotypes and life cycles of various species are primarily defined by the different extrachromosomal elements they carry (Rasko et al., 2005). The insect pathogenic B. thuringiensis strains encode insecticidal toxins, such as the delta endotoxins, on plasmids such as the large pBtoxis plasmid (Stein et al., 2006). Members of the B. cereus sensu stricto species (from here on referred to as B. cereus) can carry a range of different plasmids that define the severity of opportunistic human infections they can cause. A good example being the pCER270 plasmid which encodes the cereulide toxin biosynthesis gene cluster (Rasko et al., 2007), responsible for severe food poisoning. In the case of B. anthracis, the causative agent of anthrax, carriage of the anthrax toxin plasmid pXO1 and the capsule plasmid pXO2 facilitate a lethal mammalian infection life cycle. Interestingly, all B. anthracis strains have a frame shift mutation in the gene for the quorum sensing global regulator, PlcR, which controls the secretion of toxins and enzymes in other members of the B. cereus sensu lato group (Agaisse et al., 1999).
B. cereus strain G9241 (from here on referred to as BcG9241) was previously identified as the causative agent of an anthrax-like pneumonia infection of a metal worker in Louisiana in 1994. Phenotypic and whole genome sequencing analyses confirmed that the isolate was a B. cereus sensu stricto species which carries a close homologue of the B. anthracis plasmid pXO1, named pBCX01, encoding the tripartite anthrax toxins and their mammalian host responsive regulator AtxA1 (Hoffmaster et al., 2004). BcG9241 also carries a plasmid named pBC210 which, whilst unrelated to the B. anthracis plasmid pXO2, also encodes capsule genes, albeit distinct from those on pXO2 and a second atxA1 like regulator gene designated atxA2. The third extrachromosomal element found in BcG9241 is pBFH_1, a linear phagemid (Oh et al., 2011). Although the sequence is available for pBFH_1, it is not known if/how it contributes to the lifestyle of BcG9241. Whilst BcG9241 carries these three plasmids and produces anthrax toxins, BcG9241 is resistant to γ-phage and penicillin and carries an intact copy of the plcR gene, hence why it is referred to as a B. cereus strain (Hoffmaster et al., 2004). A small number of other B. cereus strains that are capable of causing an anthrax-like disease have also been isolated and are collectively referred to as ‘cross-over’ strains, an in-depth description of these strains is given in a recent review (Baldwin, 2020). Since this review was published, two further ‘cross-over’ strains have been isolated from patients with an anthrax like disease (Dawson et al., 2021).
B. cereus and B. anthracis differ from each other in the disease states they cause and also have very different lifestyles. B. cereus is an opportunistic pathogen causing primarily gastrointestinal infections in humans. B. cereus is also often found in the guts of many invertebrates, which both facilitates its dissemination in the environment and provides a niche for a potential necromonic lifestyle (Margulis et al., 1998; Swiecicka and Mahillon, 2006). In contrast, B. anthracis is primarily adapted to cause acute mammalian infections abandoning the more generalist lifestyle of its recent B. cereus ancestor. B. anthracis infects its mammalian host as a spore, often picked up from the soil by grazing livestock or wildlife through inhalation or ingestion (Bellan et al., 2013). The animal then dies from the acute anthrax disease. Vegetative B. anthracis cells present in the carcass sporulate as nutrients become limited and re-enter the soil as a spore, completing the life cycle (Lindeque and Turnbull, 1994). This life cycle relies on B. anthracis being able to successfully kill its host and sporulate once its host is deceased. Current studies of BcG9241 have focused on the contribution of the anthrax toxins and the tetrasaccharide and hyaluronic acid capsules to the ability of BcG9241 to cause a fatal anthrax-like disease (Scarff et al., 2018). The contribution of chromosomal regulation by pBCX01 or AtxA1 to virulence is less well understood and the sporulation of BcG9241 has so far not been investigated.
This study looks at how closely life cycle related phenotypic traits of BcG9241 resemble those of B. anthracis and how pBCX01 may influence this. To do this we have carried out RNAseq experiments to elucidate how carriage of pBCX01 influences the transcriptome of BcG9241 at the mammalian relevant temperature of 37°C compared to the more environmentally appropriate temperature of 25°C. We have also assessed the sporulation phenotype of BcG9241. Sporulation is an essential part of the B. anthracis life cycle and is intrinsically linked to other cellular processes, including virulence, through a complex regulatory network (Tan and Ramamurthi, 2014). The results presented here, as well as other work done by our group (Manoharan et al., 2023), show that BcG9241 phenotypes have a strong temperature dependence allowing it to phenocopy B. anthracis-like behaviour at 37°C, whilst conversely behaving like B. cereus sensu stricto at 25°C. pBCX01 has a strong influence on the transcriptome at 37°C, including the suppression of the transcription of genes involved in metabolic and biosynthetic pathways whilst simultaneously upregulating the expression of genes involved in the import of nutrients, an effect which is not seen at 25°C. We propose that this may facilitate mammalian virulence, allowing BcG9241 to have a growth advantage whilst simultaneously restricting nutrients available to the host. In addition, an unexpected finding of our study showed a significant increase in the pBFH_1 lysogenic phagemid expression at 37°C compared to 25°C. Interestingly, close homologues of this phagemid are also carried by other ‘cross-over’ strains. We further demonstrated that pBFH_1 produces Siphoviridae-like phage particles in the supernatant of BcG9241. Our observations regarding sporulation show that BcG9241 has a temperature dependent sporulation phenotype and sporulates rapidly compared to B. cereus ATCC 14579 (from here on referred to as BcATCC 14579), particularly at 37°C, which is independent of the presence of pBCX01.
In summary, our results show that pBCX01 has allowed BcG9241 to adopt a life cycle that resembles that of B. anthracis and that the response of BcG9241 to temperature is crucial to this. We have also demonstrated that other genetic elements contribute to this temperature dependent phenotype independently of pBCX01. To allow us to examine the influence of pBCX01 on bacterial phenotypes, we constructed a plasmid cured strain designated BcG9241 ∆pBCX01.
To determine if pBCX01 influences BcG9241 at a transcriptional level, mRNA was extracted from cultures of BcG9241 WT (WT) and BcG9241 ∆pBCX01 (∆pBCX01) growing exponentially (OD600 = 0.5) at both 25°C and 37°C. An RNAseq analysis was performed to compare the transcriptomes of WT and ∆pBCX01. A comparison of the transcriptomes of each strain at 25°C and 37°C was also performed. The full datasets generated can be seen in the Supplementary datasets 1–4. In this analysis, a fold change of >2 and p-value <0.05 was used as a cut-off for differential expression.
The number of genes found to be differentially expressed between 37°C and 25°C during exponential growth of the WT strain was 250 (Figure 1A, shown in green), whilst for the ∆pBCX01 strain the number was considerably higher, exhibiting 1,184 differentially expressed genes (DEGs; Figure 1A, shown in orange). Furthermore, there were only 177 genes that are differentially expressed between 25°C and 37°C shared between WT and ∆pBCX01 (Figure 1A). This demonstrates that the pBCX01 plasmid has a potent influence on temperature dependent expression of the chromosomal genes. To compare the functions of temperature dependent DEGs of WT and ∆pBCX01, we performed gene enrichment analyses using the STRING online tool (Szklarczyk et al., 2019) and focused on the KEGG pathways (Kanehisa, 2019) that were found to be enriched. Unfortunately, this database does not recognise the BcG9241 locus tags, therefore all genes of the BcG9241 genome were assigned a B. anthracis Sterne locus tag, in cases where there was amino acid homology of 90% or more. As such, BcG9241 genes that had no homologue in the B. anthracis genome have been excluded in this analysis.
Figure 1. Venn diagrams of genes that are differentially expressed between (A) 37° C and 25° C in BcG9241 WT (green) and BcG9241 ∆pBCX01 (orange) and (B) BcG9241 WT and ∆pBCX01 when cultured at either 25°C (green) or 37°C (red).
In ∆pBCX01, significantly DEGs between 37°C and 25°C showed a significant enrichment for genes involved in KEGG pathways associated with an increased growth rate. These include genes involved in pathways relating to metabolism, the ribosome and biosynthesis of secondary metabolites and amino acids (Table 1). The majority of these DEGs had a higher relative transcript abundance at 37°C compared to 25°C which is consistent with BcG9241 having an increased protein production and higher growth rate at 37°C compared to 25°C (Supplementary Figure S1). In contrast, the genes with temperature dependent differential expression in the WT strain did not show the same profile of functions associated with an increased growth rate. In the WT there were 16 genes involved in metabolic pathways that had a higher relative transcript abundance at 37°C compared to 25°C but surprisingly, no genes involved in amino acid biosynthesis or ribosomal genes. This is unexpected as both strains exhibit an increased growth rate at 37°C in comparison to that at 25°C (Supplementary Figure S1). An analysis of the genes that had a higher relative transcript abundance at 25°C compared to 37°C in the WT showed there was a significant enrichment for genes involved in bacterial chemotaxis. Five of the six of these differentially expressed bacterial chemotaxis genes were not found to be differentially expressed in ∆pBCX01.
Table 1. KEGG pathway enrichment analysis results of genes with a significantly higher transcript abundance at 37°C relative to 25°C at exponential phase in BcG9241 WT and BcG9241 ∆pBCX01.
To look at the direct effect of pBCX01 on the transcriptome at both 37°C and 25°C a list of genes that were differentially expressed between WT and ∆pBCX01 was generated for cultures grown at the two temperatures. The number of genes found to be differentially expressed between WT and ∆pBCX01 when cultures were grown at 37°C was 694 (Figure 1B, shown in red), whereas when cultures were grown at 25°C the number of DEG was lower at 84 (Figure 1B, shown in green). Of the 84 genes differentially expressed between WT and ∆pBCX01 at 25°C, 62 of them were also differentially expressed at 37°C between WT and ∆pBCX01. In addition to this, many of the genes with the highest differential expression between WT and ∆pBCX01 at 25°C are also seen to be differentially expressed at 37°C (Supplementary Tables S1, S2). This indicates that at 37°C pBCX01 has a greater influence on transcription than it does at 25°C.
To take a closer look at the DEGs between WT and ∆pBCX01 at 37°C the list of DEGs was split into those that had a higher relative transcript abundance in either the WT or ∆pBCX01. As before, STRING was used to perform an enrichment analysis for each list of DEGs. Genes with higher relative transcript abundance in WT compared to ∆pBCX01 at 37°C had a significant enrichment of genes encoding transmembrane proteins (Table 2). Many of these transmembrane proteins have predicted transport functions, including transporters of cobalt, formate/nitrite, phosphonates and amino acids. Four of the top 15 most differentially expressed genes showing higher transcription in WT have a predicted transporter function (Supplementary Table S1). One of these encoded an EcsB family protein involved in regulation and secretion of extracellular proteins. A gene encoding InhA1 also had a higher transcription level in WT compared to ∆pBCX01 at 37°C. InhA1 is a metalloprotease involved in breaking down host proteins for nutrients (Terwilliger et al., 2015) as well as mediating escape of B. cereus from macrophage (Haydar et al., 2018). Interestingly, pBCX01 also has a positive effect on genes predicted to encode transcriptional regulators as well as the gene encoding the transition state regulator AbrB.
Table 2. KEGG pathway enrichment analysis results of genes significantly affected by the presence of pBCX01 at 37°C exponential phase.
The analysis of genes with higher relative transcript abundance in ∆pBCX01 compared to WT showed that there was a significant enrichment for genes involved in metabolism, biosynthesis of secondary metabolites and amino acids. There is a strong similarity between the DEGs seen between 37°C and 25°C in ∆pBCX01 and the DEGs with higher transcript abundance in ∆pBCX01 when grown at 37°C. Both have significant enrichments in genes involved in metabolism, biosynthesis of secondary metabolites and amino acids. This pattern of DEGs suggests that at 37°C pBCX01 is suppressing the expression of groups of genes involved in metabolism, biosynthesis of secondary metabolites and biosynthesis of amino acids, processes that would normally be associated with an increase in growth rate.
Interestingly, the five transcripts with the highest relative abundance in ∆pBCX01 compared to WT when grown at 37°C were all around 110 nucleotides in length and encoded adjacent to each other in the genome (Supplementary Table S1). Homologues of these five genes can be found in the same arrangement in other B. cereus and B. anthracis genomes. The five genes are all homologues of each other exhibiting a DUF3948 domain, but with no known function.
To compare the overall transcriptional profiles for all four conditions (WT grown at 37°C and 25°C and ∆pBCX01 grown at 37°C and 25°C) a principal component analysis (PCA) plot was generated using data from each biological replicate (Figure 2). Principal component one describes 55% of the variance and principal component two describes 20% of the variance between all samples. All biological replicates generally cluster together. There is some overlap between the WT and ∆pBCX01 samples cultured at 25°C, supporting the hypothesis that pBCX01 has a stronger influence on the transcriptome at 37°C than at 25°C. Interestingly, the PCA plot shows that the WT 37°C samples cluster closer to all the 25°C samples than they do to the ∆pBCX01 37°C samples. This may be due to activity of pBCX01 at 37°C and its suppressive effect on genes that would otherwise be upregulated in response to a more optimal growth temperature.
Figure 2. A principal component analysis generated from a DEseq2 analysis of the RNAseq data from BcG9241 WT grown at either 37°C or 25°C and BcG9241 ∆pBCX01 grown at either 37°C or 25°C. The x-axis, showing principal component 1, describes 55% of the variance in the data and the y-axis, showing principal component 2, describes 20% of the variance in the data.
The results of the transcriptome analysis showing that pBCX01 had a negative effect on genes that are associated with an increase growth rate could suggest that at 37°C, WT has a slower growth rate than ∆pBCX01. Nevertheless, this does not appear to be the case when grown in nutrient rich LB, although at 37°C the growth rate of ∆pBCX01 is slightly higher than WT, the difference is negligible (Supplementary Figure S1). We therefore aimed to investigate whether pBCX01 had any effect on growth rate when cultured in a media designed to resemble the nutrient availability of the mammalian host. Additionally, we wanted to specifically assess the effect of pBCX01 on the ability of BcG9241 to synthesise amino acids as many amino acid biosynthesis genes were seen to be negatively affected by pBCX01. A negative effect of AtxA on amino acid genes has been previously observed in B. anthracis where either the atxA gene had been removed, or when the bacteria were grown under conditions where AtxA is known to be less active (Bourgogne et al., 2003; Panda et al., 2014; Raynor et al., 2018). This strongly suggests that it may be the pBCX01 encoded AtxA1 activity which causes the observed down regulation of amino acid biosynthesis genes.
The media used for these experiments, referred to as blood serum mimic (BSM), was designed by Terwilliger et al., to mimic the nutrient availability of the blood, and was modified here by the addition of biotin and thiamin. Individual amino acids were omitted from the BSM media to assess the ability of WT and ∆pBCX01 to synthesise specific amino acids. BcATCC 14579 was also included in these experiments to see how the growth of WT and ∆pBCX01 compare to a typical B. cereus sensu stricto strain. It should be noted that for BcG9241 to grow in BSM we had to supplement this medium with 0.04 μM biotin and 0.056 mM thiamine, or no growth was observed. It was anticipated that if WT exhibited a lower expression of the relevant amino acid biosynthesis genes than ∆pBCX01, then it may have a slower growth rate in media in which any of the relevant amino acids were limiting. To predict which amino acids might be most affected by the influence of pBCX01 a KEGG metabolic pathway analysis was used to construct a map of amino acid biosynthesis genes that were differentially expressed between WT and ∆pBCX01 (Supplementary Figure S2). Based on this analysis; arginine, histidine, isoleucine, leucine, lysine, methionine, tryptophan and valine were selected as candidates to be individually removed from the defined growth media for testing.
Our findings indicated that both WT and ∆pBCX01 were unable to grow without leucine or valine being present in the media and that BcATCC 14579 could not grow without methionine or valine. In media with either, arginine, isoleucine, lysine or tryptophan removed or with all amino acids present, WT grew slightly better than ∆pBCX01 and although BcATCC 14579 initially grew faster, WT reached a higher OD600 than either BcATCC 14579 or ∆pBCX01. In media with multiple amino acids removed, BcATCC 14579 was unable to grow, presumably because of the lack of methionine. Whilst WT grew slightly better than ∆pBCX01, there was very little growth of either strains over 24 h. Growth curves can be seen in Figure 3.
Figure 3. Growth in AA limiting media. Growth curves showing optical density measured at 600mn wavelength (OD600) plotted against time of BcG9241 WT (blue line), BcG9241 ∆pBCX01 (red line) and BcATCC 14579 (green line). The bars show standard error of three biological replicates. Cultures were grown at 37°C with shaking in blood serum mimic (BSM) media with a certain amino acid omitted. The amino acid written above each graph indicates which amino acid was omitted from the growth media.
The transcriptomic comparison of both WT and ∆pBCX01 grown at 25°C and 37°C during exponential growth phase also revealed that the pBFH_1 phagemid genes become highly transcribed during growth at 37°C compared to 25°C. The most highly induced genes at 37°C in WT included two encoding subunits of the predicted phage terminase protein, presumably involved in packing the phage genome into the maturing capsid structure. The large (AQ16_5899) and small ATPase subunit (AQ16_5898) genes were log2-fold 3.86 and 4.14 times higher at 37°C compared to 25°C, respectively, (Supplementary Table S3). Transcription of 12 genes encoding capsid or tail proteins were also highly induced at 37°C (Figure 4-red squares). Perhaps not unexpectedly a phage anti-repressor encoding gene (AQ16_5855) was seen to be induced at 37°C. Furthermore, many transcriptional activators carried by pBFH_1 showed higher transcription at 37°C, including two Xre superfamily like transcriptional regulators (AQ16_5850 and 5853). Interestingly a phagemid autolysin regulatory protein was also induced at 37°C. Taken together this suggested that the lysogenic pBFH_1 phagemid enters a lytic cycle upon exposure to the higher temperature, as evidenced by the increased transcription of virion structural protein genes. The transcriptomic comparison of ∆pBCX01 grown at 25°C and 37°C also showed that the majority of pBFH_1 genes were more highly expressed at 37°C in comparison to 25°C. Of the 15 genes most highly expressed at 37°C in comparison to 25°C, 10 were pBFH_1 encoded genes (Supplementary Table S5).
Figure 4. Genes on the pBFH_1 phagemid are more highly transcribed when BcG9241 is grown at 37°C, compared to at 25°C. BcG9241 was grown at 25°C and 37°C to exponential phase. RNA was extracted and RNA-seq performed. RNA-seq reads were processed, normalised, compared using DESEQ2 and plotted as the log2-fold change in the transcriptional level of each gene at 25°C compared to at 37°C. Almost all genes were more highly transcribed during growth at 37°C (red circles/squares), with the exception of a gene encoding a Fur-regulated protein (blue circle). All known genes encoding capsid and tail proteins (red squares) were higher at 37°C. All points plotted are significant (p-value < 0.05; n = 3).
A mass spectrometry proteomic analysis of supernatants of mid-exponentially phase WT culture grown at 37°C and 25°C was carried out by our group (Manoharan et al., 2023). In agreement with the transcriptomic analysis, the proteomic analysis showed that the 8 highest proteins and 40% of the total proteins higher at 37°C compared to 25°C were encoded on the pBFH_1 phagemid (Table 3).
Table 3. Supernatant proteins showing the largest increase in abundance at 37°C compared to 25°C during exponential growth of BcG9241 WT.
The identification of pBFH_1 structural proteins in the supernatants of WT grown at 37°C, led us to investigate if intact phage particles were present. Particulate material was filtered from supernatants and visualised using negative stain transmission electron microscopy. Figure 5 shows the presence of a phage with Siphoviridae like morphology. The average tail dimensions are 193 × 10 nm and the average head diameter is 60 nm which is consistent with the morphology of the Siphoviridae family (Hendrix et al., 2012). Like other Siphoviridae the phage particles appear to have icosahedral shaped heads and a tail made up of stacked discs. This classification is consistent with the analysis of the pBFH_1 sequence by Phaster, which assigns the structural capsid proteins as Siphoviridae (Zhou et al., 2011). Furthermore, PCR analysis of the phage preparations confirmed that intact phage particles carrying pBFH_1 DNA were present. Interestingly we were not able to detect phage proteins in the cellular proteome at 37°C (Manoharan et al., 2023) potentially indicating that the phage had been rapidly induced and released from only a sub-population of the culture. Indeed, this is consistent with the observation that there was no reduction in growth rate or apparent lysis of the whole culture at 37°C, and a testament to the sensitivity of the proteomic analysis.
Figure 5. Transmission electron micrograph of the pBFH_1 phagemid virion isolated from supernatant of a BcG9241 WT culture grown at 37°C. Average tail length: 192.57 nm and average head diameter: 60.21 nm.
Nucleotide BLAST analysis was carried out to identify any other pBFH_1-like phagemids carried by other B. cereus or B. anthracis strains. All strains identified that exhibited homology to the pBFH_1 genome with a percentage coverage of 61% and above were B. cereus strains that were also carrying pXO1/pBCX01, pXO2 or pBC210 plasmids (Table 4). Other strains with sequences homologous to the pBFH_1 phagemid only had a sequence coverage of 43% or below in B. cereus strains and 17% or below in B. anthracis strains. There were two ‘cross-over’ strains that did not show any sequence homology to pBFH_1, 03BB102 and 03BB108. Interestingly neither 03BB102 nor 03BB108 carry pXO2 or pBC210-like plasmids, and furthermore their pBCX01 plasmids have only partial homology to pXO1.
Table 4. Presence of BcG9241 pBFH_1 phagemid homologues in other B. cereus strains carrying pBCX01 or pBC210.
Bacillus anthracis sporulates rapidly under laboratory conditions (Koch, 1876), a phenotype necessary for its obligate infective lifecycle (Ross and Billing, 1957). To determine if the WT strain shares this sporulation phenotype, it was visualised using microscopy after 24 and 48 h growth on LB agar at both 25°C and 37°C. For comparison, BcATCC 14579 was also imaged under the same conditions (Figure 6A). After 24 h growth at 25°C WT cells remained mainly vegetative with little or no endospore formation. However, after 48 h growth, a limited number of endospores could be observed. In contrast, after 24 h growth at 37°C, the WT culture was almost 100% mature spores and we could observe no remaining vegetative cells. Conversely even after 48 h growth on LB agar at 37°C, BcATCC1479 displayed no endospore formation at either temperature. These findings show a striking difference in the sporulation tendency of WT compared to BcATCC1479, and also that WT sporulated more rapidly at 37°C compared to 25°C.
Figure 6. Temperature dependent sporulation of BcG9241 and BcATCC 14579 in LB. (A) BcG9241 WT and BcATCC 14579 were grown for 24 or 48 h on LB agar at either 25°C or 37°C. A loop of bacterial lawn was taken and used to inoculate 25 μL of PBS. 5 μL of this suspension was mounted and images were taken at 1,000× magnification. No spore formation is seen under any condition for BcATCC 14579. Whilst BcG9241 WT had not formed any spores within 24 h at 25°C, by 48 h growth endospores have begun to form. In contrast, after only 24 h growth at 37°C, fully mature spores had formed and few if any vegetative cells remained. (B) BcG9241 WT was cultured in LB broth at 25°C and 37°C with aeration. At 24 and 48 h samples were collected and a sporulation assay was performed to quantify percentage of spores. Data is plotted as the % of the culture that had formed spores. After 24 h at 37°C, 100% of the cell population had formed spores. However, at 25°C, only 0.06% of cells have formed spores. *indicates significance to a p-value of <0.05 using Welch’s t-test. **indicates significance to a p-value of 0.0014 using an ordinary one-way ANOVA. Error bars are one standard deviation and all tests are in triplicate (n = 3). See text for an explanation of readings of >100%.
To quantify the temperature dependant sporulation of WT, a sporulation assay was carried out in LB broth at 37°C and 25°C. Representative microscopic images were taken to confirm results of the sporulation assay (Supplementary Figure S3). After 24 h growth there was a significant difference in percentage of spores between the WT cultures grown at 37°C and those at 25°C. After 24 h at 37°C, measurements suggested 114% of cells had formed spores, compared to a negligible percentage at 25°C (Figure 6B). At 48 h measurements suggest that at 37°C 153% of spores have formed, at 25°C this is significantly lower at 88%. The results of the sporulation assay are consistent with the microscopy analysis of samples (Supplementary Figure S3). The phenomenon of a > 100% spore count (using this assay) has been observed in previous publications (Dale et al., 2018), and may arise from spore activation triggered by heat treatment. When comparing the proportion of spores formed in liquid and solid media we can see that WT sporulated around 24 h earlier on the LB agar (Figure 6A; Supplementary Figure S3).
A sporulation assay comparing the percentage spore formation of WT, ∆pBCX01 and BcATCC 14579 at 37°C and 25°C was carried out to determine whether the presence of pBCX01 contributes to the rapid sporulation phenotype of BcG9241 when grown under sporulation inducing conditions. Plasmid pBCX01 carries a Rap-Phr system with 100% identity to that encoded on pXO1 from B. anthracis (Bongiorni et al., 2007). This system is thought to prevent sporulation of B. anthracis under infection relevant conditions when optimal toxin production and rapid vegetative growth is advantageous. Therefore, these experiments were carried in the sporulation inducing media, modified G medium (MGM), to allow the effect of pBCX01 to be studied under conditions that do not inhibit spore formation.
WT, ∆pBCX01 and BcATCC 14579 were grown in MGM at 37°C for 8 h and 25°C for 20 h before the percentage spore formation was assessed. These assays showed that the sporulation phenotype of BcG9241 is not significantly affected by the presence of pBCX01 at either 37°C or 25°C (Figure 7). Both WT and ∆pBCX01 form a significantly higher percentage of spores than BcATCC 14579 at 37°C or 25°C at the time points sampled (Figure 7).
Figure 7. Percentage spore formation in MGM. BcG9241 WT, BcG9241 ∆pBCXO1 and BcATCC 14579 were grown in modified G medium (MGM) for 8 h at 37°C (A) or 20 h at 25°C (B) with shaking at 200 rpm. Percentage of spores in each sample was calculated using a sporulation assay. The black and grey bars show the average percentage of spores from three biological replicates with the standard error of the mean as a line above the bar. The horizontal lines indicate the statistical comparisons made between two samples using an unpaired, Welch’s t-test where ‘not significant’ (ns) = p > 0.05, * = p ≤ 0.05, ** = p ≤ 0.01, *** = p ≤ 0.001.
As BcG9241 sporulates rapidly at 37°C compared to BcATCC 14579 we decided to compare the sporulation processes of each strain. We constructed fluorescent reporter strains, designed to express GFP at two critical transcriptional check-points in the sporulation cascade. The aim was to investigate which point in the sporulation cascade is influenced by a temperature difference. Two promoters were selected to create the GFP reporters that would be specifically activated at (i) the initiation of the sporulation cascade and (ii) completion of DNA engulfment into the forespore. These two promoters are activated by the regulators; Spo0A, in its phosphorylated form, (Strauch et al., 1992) and Sigma G (Regan et al., 2012) respectively. These reporter strains showed that both WT and ∆pBCX01 activated the sporulation cascade at a slightly earlier time point than BcATCC 14579 and reached the late forespore stage at around 3 h before BcATCC 14579 when grown at 37°C in MGM (Supplementary Figures S4, S5). For brevity the findings of this more detailed analysis have been included in the Supplementary data.
An analysis of the influence of both pBCX01 and temperature on the transcriptomic landscape of BcG9241 clearly demonstrates that pBCX01 has a strong influence on the transcriptome of BcG9241 at the mammalian infection relevant temperature of 37°C. Notably there is significantly less influence of this plasmid at the more environmentally appropriate temperature of 25°C. Our findings are consistent with experiments in B. anthracis where pXO1 has been shown to contribute to both plasmid and chromosomal gene regulation under mammalian infection relevant conditions (McKenzie et al., 2014).
Comparing the differential expression at 37°C and 25°C between WT and ∆pBCX01, we see that under 37°C growth conditions removal of pBCX01 had a positive effect on genes one would associate with an increase growth rate in comparison to 25°C. Unexpectedly, when the plasmid is present (in the WT strain) the same pattern was not seen even though both strains exhibit a similar increase in growth rate at 37°C compared to 25°C. This suggests that the rich growth medium used is able to provide sufficient resources to support this increased biomass production irrespective of changes in the transcriptome. The influence of pBCX01 on the transcriptome results in the suppression of transcription of genes involved in various aspects of central metabolism and the biosynthesis of secondary metabolites and amino acids. This effect is likely more important during an actual infection process. Consistent with our findings, previous studies have shown that in B. anthracis, AtxA has a negative effect on the expression of amino acid biosynthesis genes (Bourgogne et al., 2003; Panda et al., 2014). Taken together these results suggest that at 37°C pBCX01 is having a large effect on gene expression and is acting to suppress the metabolic and biosynthetic processes associated with a higher growth rate. Whereas at 25°C, the plasmid is having a minimal effect on the transcriptome and there is no evidence of a similar suppression of metabolic and biosynthetic genes we see at 37°C. Another temperature dependent effect of pBCX01 on the transcriptome appears to be a suppression of the transcription of bacterial chemotaxis genes at 37°C but not at 25°C.
Although pBCX01 has a negative effect on the expression of genes encoding amino acid biosynthesis proteins, the WT was still able to synthesise most amino acids not made available in the defined growth media, and indeed even had a growth advantage over ∆pBCX01. It therefore appears that the level of amino acid biosynthesis enzymes in WT, is still sufficient to service the need for biomass production in vitro. The suppressive effect of pBCX01 may represent an adaptation to favour amino acid import during a mammalian infection, rather than expend unnecessary energy in de novo synthesis. In support of this, we note the expression of amino acid transporters was enhanced by the presence of pBCX01, again suggesting that pBCX01 promotes the uptake of available nutrients in favour of de novo biosynthesis.
Another striking effect of the carriage of pBCX01 at 37°C is an increase in the transcription of many diverse transmembrane proteins. Furthermore, both pBCX01 and temperature had an effect on the expression of multiple genes associated with maintenance of the cell membrane of BcG9241. This implies that both the virulence plasmid and temperature both effect how BcG9241 interacts with its environment. We note that pBCX01 encodes the hasACB operon for the synthesis of a hyaluronic acid capsule as well as other surface associated proteins such as BslA and an S-layer associated protein, which has been shown to mediate adhesion to host cells (Wang et al., 2013; Scarff et al., 2018). It is possible that one or more of the uncharacterized transmembrane proteins showing a higher transcript abundance in the WT strain might be involved in the secretion or assembly of the hyaluronic acid capsule or other plasmid encoded surface proteins. Another notable consequence of the carriage of pBCX01 is an increase in transcription of several genes encoding proteins containing helix-turn-helix domains. Indeed, two of these genes were in the top three most differentially expressed genes showing higher levels when pBCX01 is present. This suggests that the presence of pBCX01, and possibly AtxA, can activate the expression of other DNA binding proteins that likely regulate chromosomal genes.
Modulation of metabolism has been shown to be a strategy used by many bacterial pathogens to aid successful infection (Olive and Sassetti, 2016). We propose that the influence of AtxA, or other pBCX01 encoded factors, on the transcription of chromosomal genes may facilitate virulence in BcG9241. This may act by promoting the use of imported host nutrients, whilst at the same time suppressing unnecessary biosynthesis of required nutrients and metabolites for growth and biomass production. This strategy may give BcG9241 a growth advantage during infection and therefore increasing pathogenic potential. Indeed, higher numbers of tissue resident bacilli has previously been associated with an increased likelihood of fatal outcomes in anthrax infections (Guarner et al., 2003). Furthermore, depriving and weakening the host cells of nutrients through increased import has also been proposed to constitute a specific pathogenic strategy in a phenomenon named “nutritional virulence” (Abu Kwaik and Bumann, 2013). Finally, certain metabolic products produced by a pathogen can also have the potential to activate an immune response (Wynosky-Dolfi et al., 2014; Gaudet et al., 2015). Therefore, the modulation of the metabolism of BcG9241 by pBCX01 may serve to limit the production of potential immune reactive metabolic products.
Sporulation is an essential part of the B. anthracis lifestyle and it has been shown to sporulate rapidly under favourable conditions (Davies, 1960). Analysis of BcG9241 sporulation showed that it is also able to sporulate rapidly compared to BcATCC 14579 in both nutrient rich and nutrient poor sporulation media. This difference is also more pronounced at 37°C than at 25°C. Whilst there is a clear difference between the sporulation phenotypes of BcG9241 and BcATCC 14579, our findings demonstrate that it is not facilitated by AtxA or the presence of pBCX01 as ∆pBCX01 exhibits the same rapid sporulation phenotype. This suggests that there are other genetic elements driving the rapid sporulation phenotype in BcG9241. Interestingly, this work has identified that the pBFH_1 plasmid is highly expressed at 37°C in comparison to 25°C and leads to the production of Siphoviridae-like phage particles. There have been multiple examples of bacteriophages enhancing the sporulation efficiency of their host and it is possible that higher expression and production of pBFH_1 proteins at 37°C is influencing the regulation of sporulation (Silver-Mysliwiec and Bramucci, 1990; Peng and Yuan, 2018). As shown in Table 4, pBFH_1-like plasmids are maintained by multiple ‘cross-over’ strains suggesting that it could play an essential role in the biology of a B. cereus strain causing an anthrax-like disease.
The bacterial strains used in this study were BcG9241 (Hoffmaster et al., 2004), BcG9241 ∆pBCX01 and B. cereus reference strain ATCC 14579 (American Type Culture Collection, Manassas, VA). Overnight cultures were grown in 5 mL of Luria Bertani (LB) broth with appropriate antibiotics at either 25°C or 37°C. Before Bacillus cultures were seeded, pre-cultures were used to synchronise bacterial cell growth by diluting overnight cultures 1 in 100 in 5 mL LB and grown to exponential phase. Pre-cultures were then diluted to an OD600 = 0.005 in a specified media and volume.
Overnight cultures of BcG9241 and BcG9241 ∆pBCX01 were grown at 25°C or 37°C. Cultures were diluted to OD600 = 0.05 and incubated at the corresponding temperature until they had grown to OD600 = 0.5. This pre-culture was diluted to OD600 = 0.005 and grown in 50 mL of LB broth. Cells were cultured to mid exponential phase and collected by centrifugation at 10,000g for 1 min and pellets were resuspended in 5x volume of Qiagen RNA-protect. Resuspended pellets were stored at-20°C or used immediately. 1 mL of QIAzol (Qiagen) was added to each pellet suspension before being transferred to Lysing Matrix B tubes (MP Biomedicals). Cells were lysed using the FastPrep®-24 Classic instrument with a COOLPREP™ adapter (MP Biomedicals). Bead beating was conducted at 6 ms−1 for 40 s for 2 cycles, with a 300 s pause between cycles. Lysates were centrifuged for 1 min at 10,000g and supernatant extracted. Cell lysates were processed for total RNA extraction using the Rneasy Micro Kit (Qiagen) and treatment with Rnase-free Dnase using the Ambion™ Dnase I protocol (ThermoFisher Scientific), following the manufacturer’s protocols. Details of RNAseq library preparation, sequencing and analysis methods can be found in Supplementary method.
For the generation of strain BcG9241 ∆pBCX01 (pBCX01−/pBC210+/pBFH1+), a liquid culture of BcG9241 was grown overnight in LB broth at 42°C with shaking at 200 rpm. On each of six consecutive days, the culture was diluted 1/1,000 into fresh LB broth and grown as above. Dilutions of the final culture were plated onto NBY-Bicarbonate agar (Wilson et al., 2011) and growth at 37°C in 5% CO2 to promote capsule production. Individual colonies were passaged several times onto fresh NBY-bicarbonate plates and incubated as described above. Since AtxA, positive regulator of the capsule operon (Uchida et al., 1997), is encoded in pBCX01 plasmid, pBCX01-mutant colonies show a rough appearance, distinguishable from the smooth colonies producing the capsule. For PCR screening of candidate strains, individual rough colonies were used to conduct colony-PCR using GoTaq Green master mix (Promega) and a primer set unique to different regions on each of the BcG9241 plasmids pBCX01 and pBC210. Control reactions designed to amplify a region of BcG9241 chromosome (plcR and ribosomal 16S genes) and pBFH1 plasmid were included (Supplementary Table S5). PCR assays were done under the following conditions: an initial step of 10 min at 95°C followed by 30 cycles of 95°C for 5 min, 45°C for 1 min, and 72°C for 1.5 min; final extension for 7 min. As a result of this screening BcG9241 ∆pBCX01 was selected for further experiments. This strain was sequenced on an illumina MiSeq platform to confirm the loss of pBCX01 and a lack of any other genomic alterations (not shown).
Exponential pre-cultures of BcG9241 were diluted to OD 0.005 in 50 mL of LB broth and grown to exponential phase at 37°C. The culture was then spun at 6,000g for 10 min. The supernatant was removed and filtered through an 100 K Amicon Ultra-15 centrifugal filter column (Millipore) by centrifugation at 4,500g until all supernatant had been filtered. 500 μL of SM buffer (100 mM NaCl, 8 mM MgSO4 and 50 mM Tris HCl) was added to the column filter and vortexed vigorously to resuspend any bacteriophage caught by the filter. The sample was removed from the column and filtered through a 0.2 μm filter to remove any bacteria. Samples were stored at 4°C. To confirm the presence of pBFH_1 phagemid particles, samples were first treated with Dnase I (NEB) then heated to 75°C for 10 min to inactivate the Dnase. To break open any phage particles, proteinase K was added and incubated at 37°C for 1 h before boiling at 100°C for 1 h. The samples were then screened for the presence of phagemid DNA using two sets of primers (pBFH1_5877_Foward, pBFH1_5877_Reverse, pBFH1_5899_Foward and pBFH1_5899_Reverse) each targeting a pBFH_1 phagemid gene. A primer pair targeting the fusA gene was also used to control for the presence of non-phage particle DNA. Phage samples were negatively stained using 2% uranyl acetate for 4 min. Micrographs were collected on the JEOL2011 electron microscope using a US1000 CCD camera (Gatan Inc) at various levels of magnification.
Growth curves were carried out using modified BSM media (Terwilliger et al., 2015). BSM media was prepared according to the methods given in Terwilliger et al., and was modified by the addition of 0.04 μM of biotin and 0.056 mM of thiamine, a full list of components can be found in (Supplementary Table S6). Pre-cultures of BcG9241 WT, BcG9241 ∆pBCX01 and BcATCC 14579 grown in LB broth were first washed with modified BSM media, where all amino acids of interest had been omitted, three times before diluting to an OD600 = 0.1 in modified BSM media with the appropriate amino acid omitted. Samples were then aliquoted into a 96 well plate with 100 mL per well with three technical replicates and grown at 37°C with 700 rpm shaking. OD600 readings were taken every 15 min for 24 h. Three biological replicates were carried out for each sample with one biological replicate per plate. All OD600 readings were normalised to a blank media reading and plotted.
All cultures were grown at either 37°C or 25°C. Pre-cultures were diluted to an OD = 0.005 into 50 mL LB broth or 20 mL of MGM broth (Supplementary Table S6) and grown with 200 rpm shaking before samples were taken at specified time points. Samples were diluted and plated on to LB agar to calculate total number of CFU before heat shocking at 65°C for 30 min to kill any BcG9241 vegetative cells and 70°C for 15 min to kill any BcATCC 14579 vegetative cells. Samples were then platted onto LB agar within minutes of heat shock treatment to avoid growth of germinated cells. Temperature to kill vegetative cells of BcG9241 was reduced to 65°C as 70°C affected viability of BcG9241 spores. Heating at 65°C for 30 min was sufficient to kill vegetative cells of BcG9241. Percentage of spores was then calculated by dividing the number of a CFU of a sample post heat shock by the number of CFU pre heat shock and multiplying this number by 100.
2 μL of sample was applied to a prepared agarose pad and a cover slip placed over them. Images were captured on a Leica DMi8 premium-class modular research microscope with a Leica EL6000 external light source, using an ORCA-Flash4.0 V2 Digital CMOS Hamamatsu Camera at 100× magnification.
The original contributions presented in the study are publicly available. The data presented in the study are deposited in the ArrayExpress repository, accession number E-MTAB-12883.
GT-J planned and performed experiments and wrote much of the manuscript. SM assisted in experiments and wrote parts of the manuscript. TB planned and performed some of the experiments. CH-R planned and performed some of the experiments. LB provided certain bacterial strains and provided advice on handling them. PO provided advice on handling the pathogenic strains and assisted in interpreting the results. AH assisted in some experimental work and in interpreting certain results. NW experimental planning, secured funding, assisted in interpreting results and provided guidance and edits for writing the manuscript. All authors contributed to the article and approved the submitted version.
GT-J was funded by the BBSRC MIBTP doctoral training programme at Warwick University, United Kingdom. SM and TB were funded by the WCPRS scholarship programme provided by Warwick University, with funding contributions from DSTL (MoD) at Porton Down, United Kingdom (DSTL project references; DSTLX1000093952 and DSTLX-1000128995). CH-R was funded by an EU Marie Curie fellowship awarded whilst at the University of Bath, United Kingdom (FP7-PEOPLE-2010-IEF project 273155). AH was funded by a start-up financial package awarded to NRW upon starting at Warwick University Medical School, United Kingdom. LB is funded by Cardiff school of biological sciences, United Kingdom. PO is funded by the DSTL at Porton Down, United Kingdom. NW is funded by Warwick University, United Kingdom.
We would like to thank the Petra Oyston at the DSTL for advice throughout this project.
The authors declare that the research was conducted in the absence of any commercial or financial relationships that could be construed as a potential conflict of interest.
All claims expressed in this article are solely those of the authors and do not necessarily represent those of their affiliated organizations, or those of the publisher, the editors and the reviewers. Any product that may be evaluated in this article, or claim that may be made by its manufacturer, is not guaranteed or endorsed by the publisher.
The Supplementary material for this article can be found online at: https://www.frontiersin.org/articles/10.3389/fmicb.2023.1113642/full#supplementary-material
Abu Kwaik, Y., and Bumann, D. (2013). Microbial quest for food in vivo: “nutritional virulence” as an emerging paradigm. Cell. Microbiol. 15, 882–890. doi: 10.1111/cmi.12138
Agaisse, H., Gominet, M., Økstad, O. A., Kolsto, A.-B., and Lereclus, D. (1999). PlcR is a pleiotropic regulator of extracellular virulence factor gene expression in Bacillus thuringiensis. Mol. Microbiol. 32, 1043–1053. doi: 10.1046/j.1365-2958.1999.01419.x
Baldwin, V. M. (2020). You Can’t B. cereus – a review of Bacillus cereus strains that cause anthrax-like disease. Front. Microbiol. 11:1731. doi: 10.3389/fmicb.2020.01731
Bellan, S. E., Turnbull, P. C. B., Beyer, W., and Getzd, W. M. (2013). Effects of experimental exclusion of scavengers from carcasses of anthrax-infected herbivores on Bacillus anthracis sporulation, survival, and distribution. Appl. Environ. Microbiol. 79, 3756–3761. doi: 10.1128/AEM.00181-13
Bongiorni, C., Stoessel, R., and Perego, M. (2007). Negative regulation of Bacillus anthracis sporulation by the Spo0E family of phosphatases. J. Bacteriol. 189, 2637–2645. doi: 10.1128/JB.01798-06
Bourgogne, A., Drysdale, M., Hilsenbeck, S. G., Peterson, S. N., and Koehler, T. M. (2003). Global effects of virulence gene regulators in a Bacillus anthracis strain with both virulence plasmids. Infect. Immun. 71, 2736–2743. doi: 10.1128/IAI.71.5.2736-2743.2003
Dale, J. L., Raynor, M. J., Ty, M. C., Hadjifrangiskou, M., and Koehler, T. M. (2018). A dual role for the Bacillus anthracis master virulence regulator AtxA: control of sporulation and anthrax toxin production. Front. Microbiol. 9:482. doi: 10.3389/fmicb.2018.00482
Davies, D. G. (1960). The influence of temperature and humidity on spore formation and germination in Bacillus anthracis. J. Hyg. 58, 177–186. doi: 10.1017/S0022172400038250
Dawson, P., Schrodt, C. A., Feldmann, K., Traxler, R. M., Gee, J. E., Kolton, C. B., et al. (2021). Fatal anthrax pneumonia in welders and other metalworkers caused by Bacillus cereus group bacteria containing anthrax toxin genes — U.S. Gulf Coast states, 1994–2020. Morb. Mortal. Wkly Rep. 70, 1453–1454. doi: 10.15585/mmwr.mm7041a4
Dupke, S., Barduhn, A., Franz, T., Leendertz, F. H., Couacy-Hymann, E., Grunow, R., et al. (2019). Analysis of a newly discovered antigen of Bacillus cereus biovar anthracis for its suitability in specific serological antibody testing. J. Appl. Microbiol. 126, 311–323. doi: 10.1111/jam.14114
Gaudet, R. G., Sintsova, A., Buckwalter, C. M., Leung, N., Cochrane, A., Li, J., et al. (2015). Cytosolic detection of the bacterial metabolite HBP activates TIFA-dependent innate immunity. Science 348, 1251–1255. doi: 10.1126/science.aaa4921
Guarner, J., Jernigan, J. A., Shieh, W. J., Tatti, K., Flannagan, L. M., Stephens, D. S., et al. (2003). Pathology and pathogenesis of bioterrorism-related inhalational anthrax. Am. J. Pathol. 163, 701–709. doi: 10.1016/S0002-9440(10)63697-8
Haydar, A., Tran, S. L., Guillemet, E., Darrigo, C., Perchat, S., Lereclus, D., et al. (2018). InhA1-mediated cleavage of the metalloprotease NprA allows Bacillus cereus to escape from macrophages. Front. Microbiol. 9:1063. doi: 10.3389/fmicb.2018.01063
Hendrix, R. W., Casjens, S. R., and Lavigne, R. (2012). “Family – Siphoviridae,” in Virus taxonomy, ninth report of the international committee on taxonomy of Viruses.
Hoffmaster, A. R., Hill, K. K., Gee, J. E., Marston, C. K., De, B. K., Popovic, T., et al. (2006). Characterization of Bacillus cereus isolates associated with fatal pneumonias: strains are closely related to Bacillus anthracis and harbor B. anthracis virulence genes. J. Clin. Microbiol. 44, 3352–3360. doi: 10.1128/JCM.00561-06
Hoffmaster, A. R., Ravel, J., Rasko, D. A., Chapman, G. D., Chute, M. D., Marston, C. K., et al. (2004). Identification of anthrax toxin genes in a Bacillus cereus associated with an illness resembling inhalation anthrax. Proc Natl Acad Sci U. S. A. 101, 8449–8454. doi: 10.1073/pnas.0402414101
Jensen, G. B., Hansen, B. M., Eilenberg, J., and Mahillon, J. (2003). The hidden lifestyles of Bacillus cereus and relatives. Environ. Microbiol. 5, 631–640. doi: 10.1046/j.1462-2920.2003.00461.x
Johnson, S. L., Daligault, H. E., Davenport, K. W., Jaissle, J., Frey, K. G., Ladner, J. T., et al. (2015). Complete genome sequences for 35 biothreat assay-relevant Bacillus species. Genome Announc. 3, 10–11. doi: 10.1128/genomeA.00151-15
Kanehisa, M. (2019). Toward understanding the origin and evolution of cellular organisms. Protein Sci. 28, 1947–1951. doi: 10.1002/pro.3715
Koch, R. (1876). The etiology of anthrax, based on the life history of Bacillus anthracis. Beitrage zur Biologie der Pflanzen 2, 277–310.
Lindeque, P. M., and Turnbull, P. C. (1994). Ecology and epidemiology of anthrax in the Etosha National Park, Namibia. Onderstepoort J. Vet. Res. 61, 71–83.
Manoharan, S., Taylor-Joyce, G., Brooker, T. A., Hernandez-Rodriguez, C. S., Hapeshi, A., Baldwin, V., et al. (2023). From cereus to anthrax and back again: Assessment of the temperature-dependent phenotypic switching in the “cross-over” strain Bacillus cereus G9241. Front. Microbiol. 14:1113562. doi: 10.3389/fmicb.2023.1113562
Margulis, L., Jorgensen, J. Z., Dolan, S., Kolchinsky, R., Rainey, F. A., and Lo, S. C. (1998). The Arthromitus stage of Bacillus cereus: intestinal symbionts of animals. Proc Natl Acad Sci U. S. A. 95, 1236–1241. doi: 10.1073/pnas.95.3.1236
Marston, C. K., Ibrahim, H., Lee, P., Churchwell, G., Gumke, M., Stanek, D., et al. (2016). Anthrax toxin-expressing Bacillus cereus isolated from an anthrax-like eschar. PLoS One 11, 1–7. doi: 10.1371/journal.pone.0156987
McKenzie, A. T., Pomerantsev, A. P., Sastalla, I., Martens, C., Ricklefs, S. M., Virtaneva, K., et al. (2014). Transcriptome analysis identifies Bacillus anthracis genes that respond to CO2 through an AtxA-dependent mechanism. BMC Genomics 15:229. doi: 10.1186/1471-2164-15-229
Oh, S.-Y., Budzik, J. M., Garufi, G., and Schneewind, O. (2011). Two capsular polysaccharides enable Bacillus cereus G9241 to cause anthrax-like disease. Mol. Microbiol. 80, 455–470. doi: 10.1111/j.1365-2958.2011.07582.x
Olive, A. J., and Sassetti, C. M. (2016). Metabolic crosstalk between host and pathogen: sensing, adapting and competing. Nat. Rev. Microbiol. 14, 221–234. doi: 10.1038/nrmicro.2016.12
Panda, G., Basak, T., Tanwer, P., Sengupta, S., dos Santos, V. A. P. M., and Bhatnagar, R. (2014). Delineating the effect of host environmental signals on a fully virulent strain of Bacillus anthracis using an integrated transcriptomics and proteomics approach. J. Proteome 105, 242–265. doi: 10.1016/j.jprot.2013.12.018
Pena-Gonzalez, A., Marston, C. K., Rodriguez-R, L. M., Kolton, C. B., Garcia-Diaz, J., Theppote, A., et al. (2017). Draft genome sequence of Bacillus cereus LA2007, a human-pathogenic isolate harboring anthrax-like plasmids. Genome Announc. 5:e00181-17. doi: 10.1128/genomeA.00181-17
Peng, Q., and Yuan, Y. (2018). Characterization of a novel phage infecting the pathogenic multidrug-resistant Bacillus cereus and functional analysis of its endolysin. Appl. Microbiol. Biotechnol. 102, 7901–7912. doi: 10.1007/s00253-018-9219-7
Rasko, D. A., Altherr, M. R., Han, C. S., and Ravel, J. (2005). Genomics of the Bacillus cereus group of organisms. FEMS Microbiol. Rev. 29, 303–329. doi: 10.1016/j.femsre.2004.12.005
Rasko, D. A., Rosovitz, M. J., Økstad, O. A., Fouts, D. E., Jiang, L., Cer, R. Z., et al. (2007). Complete sequence analysis of novel plasmids from emetic and periodontal Bacillus cereus isolates reveals a common evolutionary history among the B. cereus-group plasmids, including Bacillus anthracis pXO1. J. Bacteriol. 189, 52–64. doi: 10.1128/JB.01313-06
Raynor, M. J., Roh, J. H., Widen, S. G., Wood, T. G., and Koehler, T. M. (2018). Regulons and protein–protein interactions of PRD-containing Bacillus anthracis virulence regulators reveal overlapping but distinct functions. Mol. Microbiol. 109, 1–22. doi: 10.1111/mmi.13961
Regan, G., Itaya, M., and Piggot, P. J. (2012). Coupling of σG activation to completion of engulfment during sporulation of Bacillus subtilis survives large perturbations to DNA translocation and replication. J. Bacteriol. 194, 6264–6271. doi: 10.1128/JB.01470-12
Ross, K. F., and Billing, E. (1957). The water and solid content of living bacterial spores and vegetative cells as indicated by refractive index measurements. J. Gen. Microbiol. 16, 418–425. doi: 10.1099/00221287-16-2-418
Scarff, J. M., Seldina, Y. I., Vergis, J. M., Ventura, C. L., and O’Brien, A. D. (2018). Expression and contribution to virulence of each polysaccharide capsule of Bacillus cereus strain G9241. PLoS One 13:e0202701. doi: 10.1371/journal.pone.0202701
Silver-Mysliwiec, T. H., and Bramucci, M. G. (1990). Bacteriophage-enhanced sporulation: comparison of spore-converting bacteriophages PMB12 and SP10. J. Bacteriol. 172, 1948–1953. doi: 10.1128/jb.172.4.1948-1953.1990
Stein, C., Jones, G. W., Chalmers, T., and Berry, C. (2006). Transcriptional analysis of the toxin-coding plasmid pBtoxis from Bacillus thuringiensis subsp. israelensis. Appl. Environ. Microbiol. 72, 1771–1776. doi: 10.1128/AEM.72.3.1771-1776.2006
Strauch, M. A., Trach, K. A., Day, J., and Hoch, J. A. (1992). Spo0A activates and represses its own synthesis by binding at its dual promoters. Biochimie 74, 619–626. doi: 10.1016/0300-9084(92)90133-Y
Swiecicka, I., and Mahillon, J. (2006). Diversity of commensal Bacillus cereus sensu lato isolated from the common sow bug (Porcellio scaber, isopoda). FEMS Microbiol. Ecol. 56, 132–140. doi: 10.1111/j.1574-6941.2006.00063.x
Szklarczyk, D., Gable, A. L., Lyon, D., Junge, A., Wyder, S., Huerta-Cepas, J., et al. (2019). STRING v11: protein-protein association networks with increased coverage, supporting functional discovery in genome-wide experimental datasets. Nucleic Acids Res. 47, D607–D613. doi: 10.1093/nar/gky1131
Tan, I. S., and Ramamurthi, K. S. (2014). Spore formation in Bacillus subtilis. Environ. Microbiol. Rep. 6, 212–225. doi: 10.1111/1758-2229.12130
Terwilliger, A., Swick, M. C., Pflughoeft, K. J., Pomerantsev, A., Lyons, C. R., Koehler, T. M., et al. (2015). Bacillus anthracis overcomes an amino acid auxotrophy by cleaving host serum proteins. J. Bacteriol. 197, 2400–2411. doi: 10.1128/JB.00073-15
Uchida, I., Makino, S. I., Sekizaki, T., and Terakado, N. (1997). Cross-talk to the genes for Bacillus anthracis capsule synthesis by atxA, the gene encoding the trans-activator of anthrax toxin synthesis. Mol. Microbiol. 23, 1229–1240. doi: 10.1046/j.1365-2958.1997.3041667.x
Wang, Y., Oh, S., Hendrickx, A. P. A., Lunderberg, J. M., and Schneewind, O. (2013). Bacillus cereus G9241 S-layer assembly contributes to the pathogenesis of anthrax-like disease in mice. J. Bacteriol. 195, 596–605. doi: 10.1128/JB.02005-12
Wilson, M. K., Vergis, J. M., Alem, F., Palmer, J. R., Keane-Myers, A. M., Brahmbhatt, T. N., et al. (2011). Bacillus cereus G9241 makes anthrax toxin and capsule like highly virulent B. anthracis Ames but behaves like attenuated toxigenic nonencapsulated B. anthracis Sterne in rabbits and mice. Infect. Immun. 79, 3012–3019. doi: 10.1128/IAI.00205-11
Wright, A. M., Beres, S. B., Consamus, E. N., Long, S. W., Flores, A. R., Barrios, R., et al. (2011). Rapidly progressive, fatal, inhalation anthrax-like infection in a human: case report, pathogen genome sequencing, pathology, and coordinated response. Arch. Pathol. Lab. Med. 135, 1447–1459. doi: 10.5858/2011-0362-SAIR.1
Wynosky-Dolfi, M. A., Snyder, A. G., Philip, N. H., Doonan, P. J., Poffenberger, M. C., Avizonis, D., et al. (2014). Oxidative metabolism enables salmonella evasion of the NLRP3 inflammasome. J. Exp. Med. 211, 653–668. doi: 10.1084/jem.20130627
Keywords: anthrax, plasmids, emerging pathogen, regulation, Bacillus cereus
Citation: Taylor-Joyce G, Manoharan S, Brooker TA, Hernández-Rodríguez CS, Baillie L, Oyston PCF, Hapeshi A and Waterfield NR (2023) The influence of extrachromosomal elements in the anthrax “cross-over” strain Bacillus cereus G9241. Front. Microbiol. 14:1113642. doi: 10.3389/fmicb.2023.1113642
Received: 01 December 2022; Accepted: 31 January 2023;
Published: 05 May 2023.
Edited by:
Axel Cloeckaert, Institut National de Recherche pour l’Agriculture, l’Alimentation et l’Environnement, FranceReviewed by:
Frédéric Carlin, Institut National de Recherche pour l’Agriculture, l’Alimentation et l’Environnement, FranceCopyright © 2023 Taylor-Joyce, Manoharan, Brooker, Hernández-Rodríguez, Baillie, Oyston, Hapeshi and Waterfield. This is an open-access article distributed under the terms of the Creative Commons Attribution License (CC BY). The use, distribution or reproduction in other forums is permitted, provided the original author(s) and the copyright owner(s) are credited and that the original publication in this journal is cited, in accordance with accepted academic practice. No use, distribution or reproduction is permitted which does not comply with these terms.
*Correspondence: Nicholas R. Waterfield, bi5yLndhdGVyZmllbGRAd2Fyd2ljay5hYy51aw==
Disclaimer: All claims expressed in this article are solely those of the authors and do not necessarily represent those of their affiliated organizations, or those of the publisher, the editors and the reviewers. Any product that may be evaluated in this article or claim that may be made by its manufacturer is not guaranteed or endorsed by the publisher.
Research integrity at Frontiers
Learn more about the work of our research integrity team to safeguard the quality of each article we publish.