- 1College of Animal Science and Technology, College of Veterinary Medicine, Zhejiang A&F University, Hangzhou, Zhejiang, China
- 2Zhejiang Provincial Animal Husbandry Technology Promotion and Monitoring Station of Breeding Livestock and Poultry, Hangzhou, China
- 3College of Animal Science, Zhejiang University, Hangzhou, China
- 4Kemin (China) Technologies Co., Ltd., Zhuhai, China
The growth and health statuses of calves during the early stages of development have a significant effect on milk production during their first lactation period. Using appropriate milk replacers helps meet the long-term targets of dairy farmers. This study aimed to examine the effects of milk, milk replacer, and milk replacer plus ethoxyquin on growth performance, antioxidant status, immune function, and the gut microbiota of Holstein dairy calves. A total of 36 neonatal dairy calves were randomly divided into three groups and fed different diets: one group was fed milk, another group was fed milk replacer, and the third group was given milk replacer plus ethoxyquin. The supplementation with ethoxyquin was started on day 35 of the feeding period. The calves were weaned on day 45, and the experiment was conducted until day 49. The blood and fecal samples were collected at the end of the animal experiment. The results showed that milk replacers induced poor growth performance (body weight and average daily gain). However, milk replacer plus ethoxyquin aided in growth performance, enhanced the starter intake and blood antioxidative ability, and elevated the concentration of fecal valeric acid. Moreover, fecal fermentation and 16S rRNA analyses showed that milk replacer plus ethoxyquin altered the microbial composition (reducing Alistipes and Ruminococcaceae and increasing Bacteroides and Alloprevotella). Pearson's correlation assays showed that alterations in fecal microbiota strongly correlated with average daily gain and antioxidative ability. The results indicated the potential of milk replacer plus ethoxyquin in modulating the growth of dairy calves and in enhancing their ability to combat stress.
Introduction
The growth and health statuses of calves during their first lactation period have a significant effect on milk production (Chester-Jones et al., 2017). Feeding ad-lib quantities of milk to dairy calves has been shown to cause higher growth rates at an early age (Khan et al., 2011; Iqbal et al., 2014). With the increasing demand for high production performance, milk replacer is an alternative that can boost growth performance but reduce the pre-weaning feeding cost.
Milk replacer is a type of artificial milk made of non-milk protein sources and is designed to meet the nutritional requirements of breast milk as per established standards. Compared to whole milk, the quality of milk replacer is not easily affected by external factors such as diet and season (Bernabucci et al., 2015; Toral et al., 2015). Calves fed milk replacers had a higher starter intake and longer-lasting effects on the rumen environment compared to those fed whole milk or pasteurized waste milk (Zhang et al., 2019). Exiting evidence also showed that milk replacers increase the survival rate of the Awassi lamb (Emsen et al., 2004). During the early stages of development, the gut microbiota is important to the host's health, as a stable gut bacterial community is a prerequisite for the host to perform normal physiological functions, metabolism, and immune functions (Gensollen et al., 2016; Li et al., 2021), while an imbalance may result in gastrointestinal diseases (Wang et al., 2019). Whether the gut bacterial community changes with the milk replacer is unknown.
Abrupt weaning, usually done at 6 weeks of age, is a source of stress for young animals and may lead to a reduction in body weight (de Passillé et al., 2011; Ungerfeld et al., 2011), diarrhea (Khan et al., 2007), and compromised intestinal barrier function (Li et al., 2018). Abrupt weaning makes young animals particularly vulnerable to infectious diseases as the immune system is not yet fully developed (Godbout and Glaser, 2006). Proper additives are a promising approach to protecting an animal from weaning stress (Kim et al., 2020; Mattioli et al., 2020). Ethoxyquin is widely used in animal feeds to protect against lipid peroxidation (Błaszczyk et al., 2013). Previous studies have demonstrated that feeding ethoxyquin can improve cows' lactation performance and antioxidant status, as well as partially mitigate the negative effects of feeding oxidized fat (Váquez-Añón et al., 2008; Boerman et al., 2014). Whether feeding ethoxyquin during the weaning period could help combat weaning stress is worth exploring.
We hypothesized that (1) a milk replacer could replace whole milk in dairy calves and (2) feeding ethoxyquin could mitigate negative effects during the weaning period. The objectives of this study were not only to determine the effects of milk, milk replacer, and milk replacer plus ethoxyquin on the growth performance and weaning stress of dairy calves but also to profile the changes in the gut microbiota.
Materials and methods
Experimental design and animal management
A total of 36 male Holstein calves were enrolled in this experiment. They were paired into 12 blocks based on their body weight and the date of their birth before being randomly assigned to one of three treatment groups within each block. The three treatments were control group (C, fed fresh milk), milk replacer group (MR, fed the milk replacer), and milk replacer plus ethoxyquin group (MRE, fed the milk replacer plus ethoxyquin). The ethoxyquin (Endox®5) was purchased from Kemin (China) Technologies Co. Ltd., Zhuhai. The dose of ethoxyquin used was 350 mg/kg of starter intake, and the feeding commenced on day 35.
The calves were given a total of 6 L of colostrum, with 4 L administered within the first hour after birth, and the remaining 2 L administered 5 h later. The calves were removed from their dam within 3 h of birth. The nutrient content of the milk replacer (Swot Technology Co., Ltd., Hangzhou) is presented in Table 1. Before feeding, the milk replacer was reconstituted with warm water (39°C) to 12.5% solids. The amount of milk or milk replacer fed to the calves was 12% v/w of their body weight, and the liquid feed was offered to them three times a day. They were fed from bottles at first but were then trained to drink from buckets. The day of birth was considered 1 day of age (DOA). After 38 days, the allowance of liquid feed was reduced by 50% each day, and the frequency of feeding was reduced to two times per day. At 42 days of age, the liquid feed was given one time per day. Weaning ended on day 45.
The pellets of a starter and alfalfa hay were offered ad libitum to the calves in individual buckets beginning at 7 DOA and 10 DOA, respectively. The amount of starter pellets intake was measured weekly to calculate the dose of ethoxyquin used. The chemical composition of the starter and alfalfa hay is shown in Table 2. All the calves were housed in individual hutches and managed similarly, with used sand as the bedding material. The sand was replaced one time a week to keep the bedding material clean. During the experiment, the windows of the hutches were opened for ventilation. The overall timeline of the experimental protocol is summarized and presented in Figure 1.
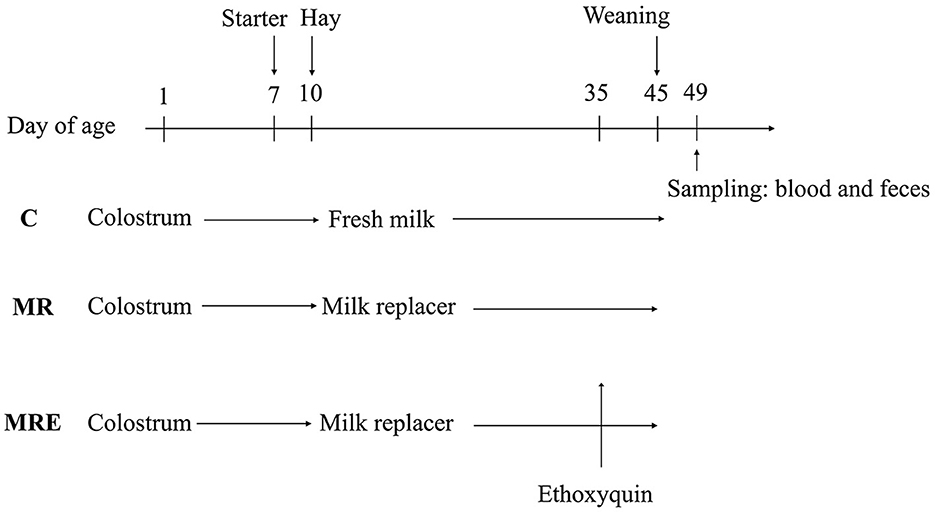
Figure 1. Overall timeline of the experimental protocol showing the change of treatments, starter, weaning and sample collection. C, calves fed fresh milk; MR, calves fed milk replacer; MRE, calves fed milk replacer plus ethoxyquin at 350 mg/kg group.
Intake and growth measures
The calves were weighed immediately after birth and weekly thereafter. The intake of starter pellets was recorded for each calf weekly.
Blood sampling and analysis
Blood samples were collected at approximately 10 a.m. via the jugular venipuncture and divided into two tubes. One of the tubes contained K2-EDTA, and the samples were centrifuged at 3,500 g and 4°C for 15 min to obtain plasma. The sample in the other tube was allowed to clot at room temperature for 30 min to obtain serum. After centrifugation, all of the supernatants were then stored at −80°C for further analyses.
The plasma was analyzed for glucose, non-esterified fatty acids (NEFA), urea nitrogen (BUN), total protein (TP), and albumin (ALB) using commercial kits (Jiancheng Bioengineering Institute, Nanjing, China). The serum was used for analyzing the antioxidative status and immunity parameters. The total antioxidant capacity (T-AOC) was analyzed using the ferric antioxidant reducing power (FRAP) (Wang and Zuo, 2015). The activity of glutathione peroxidase (GSH-PX), as well as the malondialdehyde (MDA) concentration, were measured using commercial kits (Jiancheng Bioengineering Institute, Nanjing, China) following the manufacturer's instructions. The catalase (CAT) activity was determined using a commercial kit (Jiancheng Bioengineering Institute) based on the decomposition of hydrogen peroxide (H2O2). ELISA was used to determine the IgA, IgG, and IgM concentrations in serum (Cow IgA ELISA kit, catalog no. H108; Cow IgG ELISA kit, catalog no. H106; Cow IgM ELISA kit, catalog no. H109; and Jiancheng Bioengineering Institute, Nanjing, China).
Feces collection and volatile fatty acid analysis
The feces were sampled in the last three consecutive days of the experiment so that the samples were represented every 3 h in a 24-h feeding cycle. After sampling, the fecal samples (about 200 g for each calf) were stored in liquid nitrogen immediately.
Before the analysis, all the fecal samples were pooled, mixed, and homogenized using a sterile slap homogenizer. Approximately 4 g of the samples was mixed in 4 mL of distilled water for volatile fatty acid (VFA) extraction and analysis. The concentrations and proportions of VFA (including acetic acid, propionic acid, butyric acid, valeric acid, isobutyric acid, isovaleric acid, and isoacids) were analyzed using gas chromatography (Agilent Technologies 7820A GC system, Santa Clara, USA) according to previously described methods (Li et al., 2019).
Fecal microbiological analysis
DNA extraction and PCR amplification
Five samples from the treatment group were used for microbiological analysis. Approximately 1 g of the subsample was used for metagenomic DNA extraction. Microbial DNA was extracted from the fecal samples using the E.Z.N.A.® soil DNA kit (Omega Bio-tek, Norcross, GA, U.S.) according to the manufacturer's protocols. The final DNA concentration and purification were determined using a NanoDrop 2,000 UV-vis spectrophotometer (Thermo Scientific, Wilmington, USA), and DNA quality was checked using 1% agarose gel electrophoresis. The V3-V4 hypervariable regions of the bacterial 16S rRNA were amplified with primers 338F (ACTCCTACGGGAGGCAGCAG) and 806R (GGACTACHVGGGTWTCTAAT) by a thermocycler PCR system (GeneAmp 9700, ABI, USA). The PCR reactions were conducted using the following program: 3 min of denaturation at 95°C, 28 cycles of 30 s at 95°C, 30 s for annealing at 55°C, and 45 s for elongation at 72°C, followed by a final extension at 72°C for 10 min. PCR reactions were performed in triplicates in a 20-μL mixture containing 4 μL of 5 × FastPfu Buffer, 2 μL of 2.5 mM dNTPs, 0.8 μL of each primer (5 μM), 0.4 μL of FastPfu Polymerase, and 10 ng of template DNA. The resulting PCR products were extracted from a 2% agarose gel and further purified using the AxyPrep DNA Gel Extraction Kit (Axygen Biosciences, Union City, CA, USA) and quantified using QuantiFluor™-ST (Promega, USA) according to the manufacturer's instructions.
Illumina MiSeq sequencing and processing
Purified amplicons were pooled in equimolar amounts and paired-end sequenced (2 × 300) on an Illumina MiSeq platform (Illumina, San Diego, USA) according to the standard protocols by Majorbio Bio-Pharm Technology Co. Ltd. (Shanghai, China). Raw FASTQ files were quality-filtered using Trimmomatic and merged using FLASH in accordance with the following criteria: (1) The reads were truncated at any site receiving an average quality score of < 20 over a 50-bp sliding window; (2) sequences whose overlap was longer than 10 bp were merged according to their overlap with a mismatch of no more than 2 bp; (3) sequences of each sample were separated according to barcodes (exactly matching), primers (allowing two nucleotide mismatches); and reads containing ambiguous bases were removed. Operational taxonomic units (OTUs) were clustered with a 97% similarity cutoff using UPARSE (version 7.1 http://drive5.com/uparse/) with a novel “greedy” algorithm that performs chimera filtering and OTU clustering simultaneously. The taxonomy of each 16S rRNA gene sequence was analyzed using the RDP Classifier algorithm (http://rdp.cme.msu.edu/) against the database using a confidence threshold of 70%.
The α diversity was analyzed using Mothur1.30.2 (https://www.mothur.org/wiki/Download_mothur). The β diversity analysis was based on the unweighted UniFrac distance and was performed using QIIME1.9.1. The microbiota composition at different levels was determined based on tax_summary and R package version 3.3.1, and the difference between the groups was analyzed using a one-way ANOVA and Tukey's test. The LDA effect size analysis (LEfSe) was conducted to screen differentially abundant bacterial taxa with an LDA score of >3.0.
Statistical analysis
All the gathered data were analyzed using the MIXED procedure of SAS version 9.1 (SAS Institute Inc., Cary, NC). The repeated measures were used for the body weight (BW), average daily gain (ADG), and starter intake, and the model contained the effects of treatment, time, and the interaction of treatment and time. The initial BW was used for a covariate analysis. The linear model was used for fecal VFA concentrations and blood parameters. The results were expressed as least-squares means and standard errors of the mean. The correlations between fecal microbiota and performance, rumen fermentation, and blood parameters were calculated using Spearman's correlation coefficient. A heatmap diagram was drawn to visualize the data and identify the relationships between the variables. Statistical significance was determined for the treatment difference with a P ≤ 0.05, while a P-value of 0.05 < P ≤ 0.10 was considered indicative of a trend.
Results
In total, five calves (1 in the C group, 3 in the MR group, and 1 in the MRE group) developed abomasal bloating. Consequently, they were removed from the experiment.
BW, ADG, and starter intake of the calves
The overall effects on BW, ADG, and starter intake are shown in Table 3. The results indicated a decrease in BW with MR treatment (P = 0.02), while MRE treatment led to an increase in starter intake (P < 0.01). Significant time effects were observed for both BW and starter intake (P < 0.01). The interaction between week × treatment was observed for both BW and starter intake (P < 0.01), and we detected a trend for the week × treatment interaction for ADG (P = 0.09). Compared to the C group, the calves in the MR group showed a decrease in BW at weeks 4 and 7, while both the MR and MRE groups showed a decrease in BW at weeks 5 and 6 (Figure 2, P < 0.05). In particular, the BW of the MR group at week 7 was significantly lower than that of both the C and MRE groups (P < 0.05). Additionally, at weeks 5 and 6, the starter intake was higher in the MRE group compared to the C and MR groups (P < 0.05).
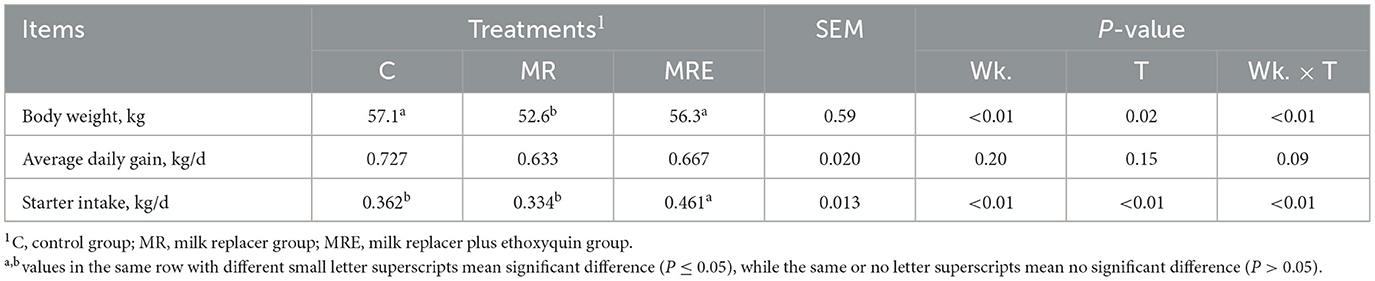
Table 3. Effects of milk, milk replacer and milk replacer plus ethoxyquin on growth of Holstein calves (N = 11,9, and 11 in C, MR, and MRE, respectively).
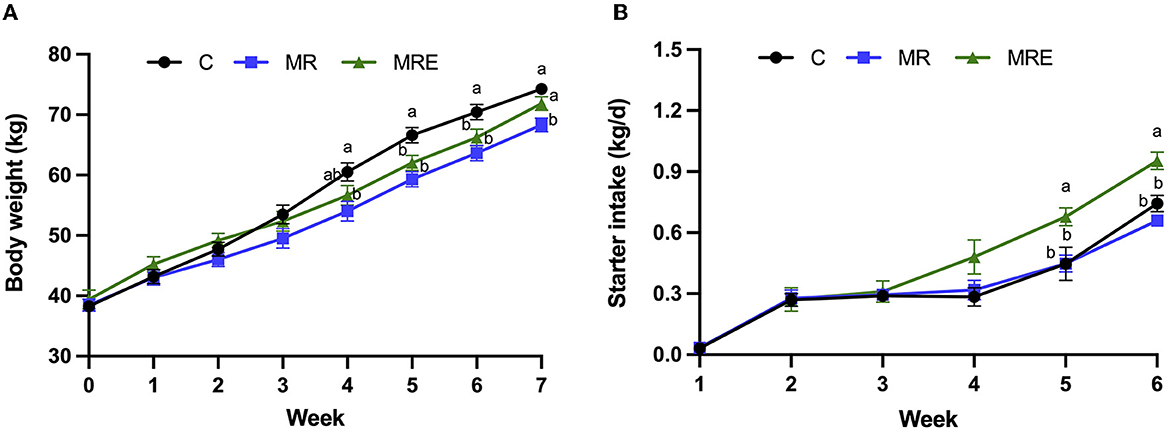
Figure 2. Growth performance of Holstein calves. C, calves fed fresh milk; MR, calves fed milk replacer; MRE, calves fed milk replacer plus ethoxyquin. (A) Body weight; (B) Starter pellets intake. Values were means ± SEM. abThe mean values with different superscripts are different at P < 0.05. N = 11.9, and 11 in C, MR, and MRE, respectively.
Blood antioxidant ability and immunity parameters
The blood antioxidant ability and immunity parameters are shown in Table 4. The NEFA concentration was higher in the MRE group compared to the C and MR groups (P < 0.05), and the TP concentration tended to elevate (P = 0.06). There were no changes in the concentrations of glucose, BUN, or ALB. For antioxidant ability, the T-AOC was higher in the MRE group than in both the C and MR groups (P < 0.05). GSH-PX, CAT, and MDA also tended to increase (P = 0.07, 0.07, and 0.09, respectively). No difference was found in the immunity parameters (IgA, IgG, and IgM).
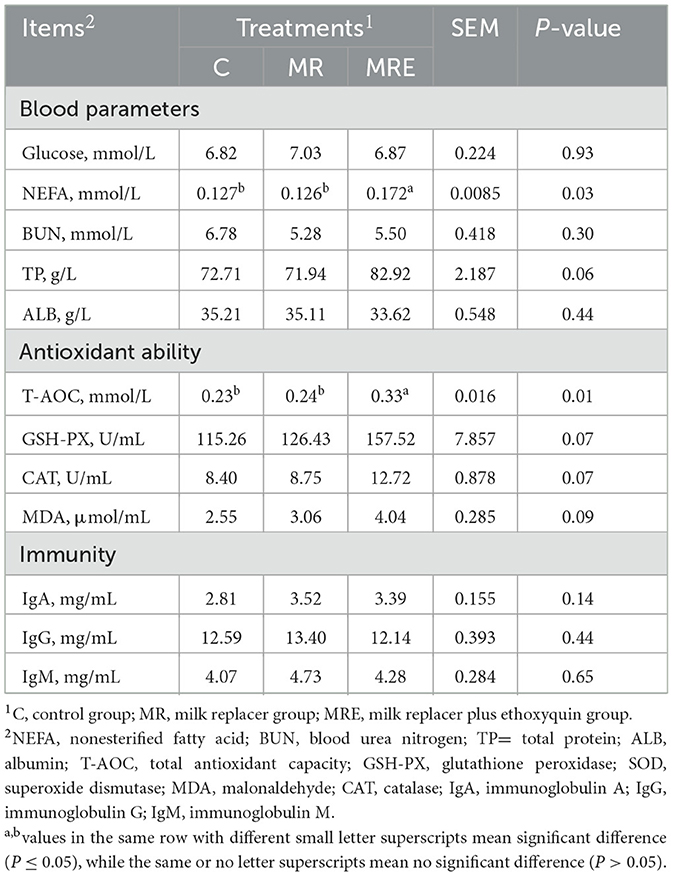
Table 4. Effects of milk, milk replacer and milk replacer plus ethoxyquin on blood metabolites of Holstein calves (N = 11.9, and 11 in C, MR, and MRE, respectively).
Fermentation parameters
As shown in Table 5, the concentration of acetic acid tended to decrease, and the concentration of valeric acid was increased in the MRE group compared to the C and MR groups (P = 0.08 and 0.05, respectively). Moreover, the ruminal isoacids (expressed as the percentage of total VFA) tended to increase (P = 0.07).
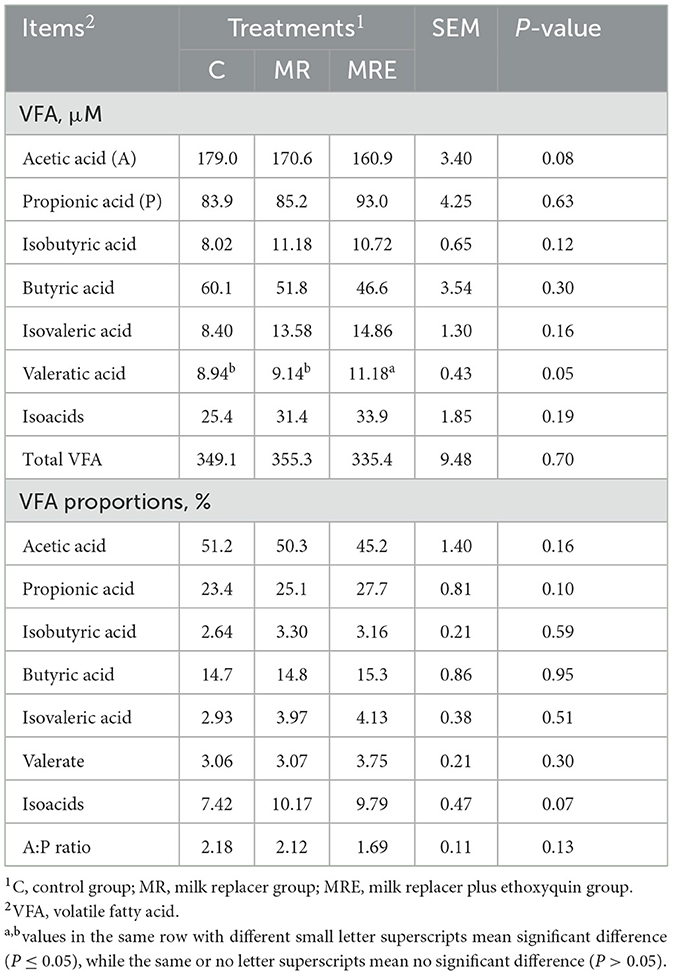
Table 5. Effects of milk, milk replacer and milk replacer plus ethoxyquin on fecal fermentation profiles of Holstein calves (N = 11,9, and 11 in C, MR, and MRE, respectively).
Microbial community composition
The alterations in the fecal microbiota were investigated. The coverage for each sample was >99%, indicating sufficient sequencing depth to detect most of the fecal bacteria of the calves in this study. The total OTUs in the C, MR, and MRE groups were 958, 955, and 1,061, respectively (Figure 3A). Four indicators were used to reflect the microflora's richness (Chao, ACE) and diversity (Shannon, Simpson). As shown in Table 6, the richness was decreased in the MRE group compared to the C group (P < 0.05), with no difference in diversity. The β diversity was displayed in a PCA scatterplot and shown in Figure 3B, indicating a clear shift between the C and MRE groups.
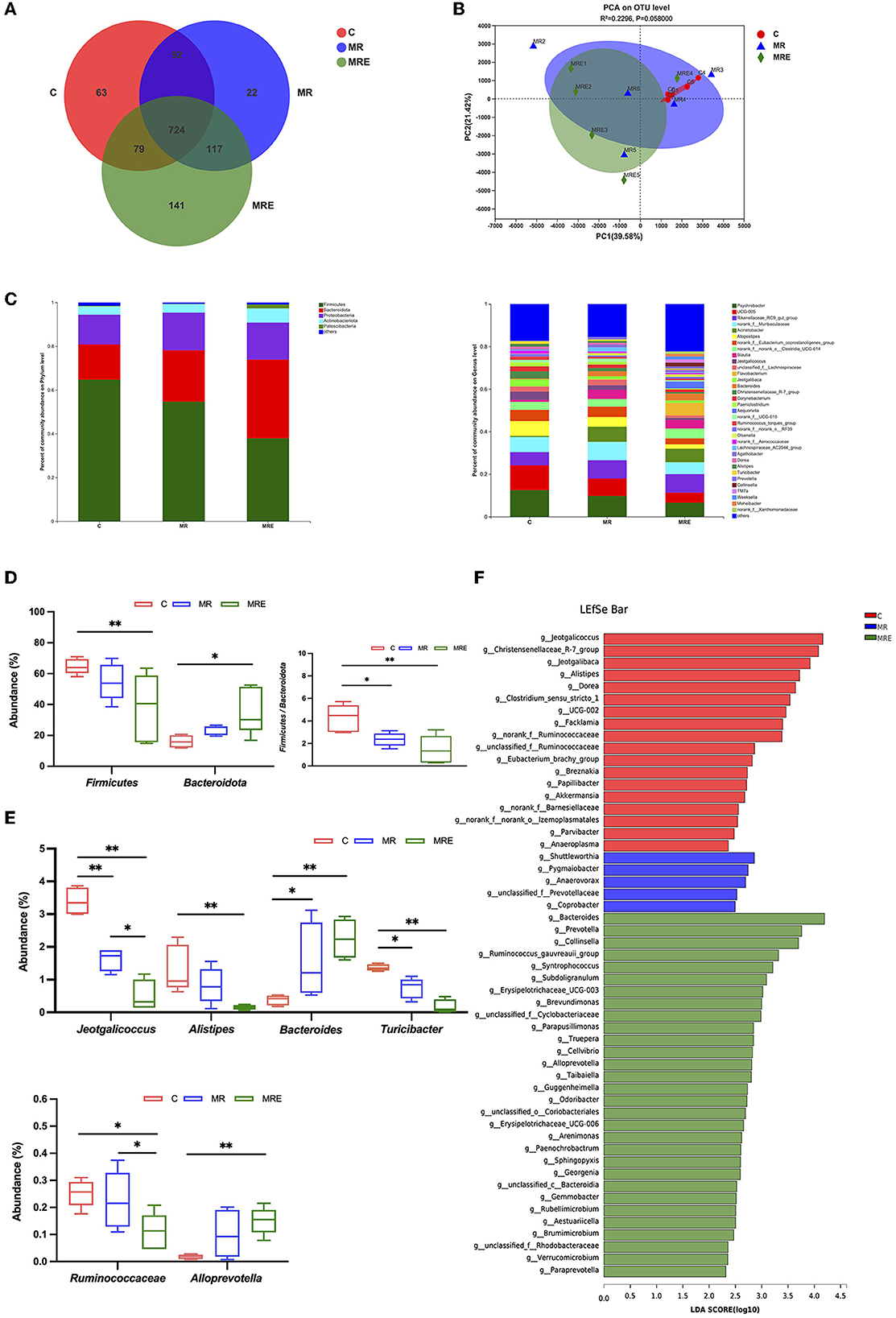
Figure 3. Analysis of the diversity, composition, and taxonomic biomarkers of fecal microbiota. C, calves fed fresh milk; MR, calves fed milk replacer; MRE, calves fed milk replacer plus ethoxyquin. N = 5 in each group. (A) Venn diagram presenting the operational taxonomic units (OTUs) from each group. (B) β diversity shown in a Principal component analysis (PCA) scatterplot. (C) A bar graph of microbial composition at both the phylum and genus levels. (D) A box plot of the significant phylum among groups. (E) A box plot of the significant genera among groups. (F) Histogram of Linear discriminant analysis (LDA) scores representing the taxonomic biomarkers by LDA effect size (LEfSe) analysis. LDA score (log10) >2 suggests the enriched taxa in cases. The data were analyzed by one-way ANOVA and Tukey's test. *P < 0.05, **P < 0.01.
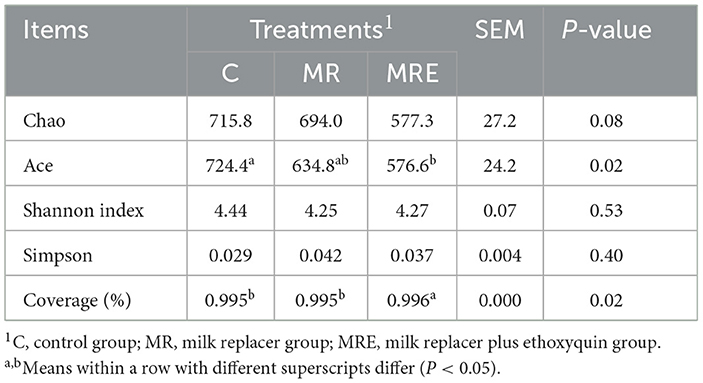
Table 6. Effects of milk, milk replacer and milk replacer plus ethoxyquin on fecal bacterial diversity at OTU level of Holstein calves (N = 5).
The differences in microbial compositions at the phylum and genus levels are shown in Figure 3C. At the phylum level, the abundance of Firmicutes was decreased and that of Bacteroidota was increased in the MRE group compared to the C group (P < 0.05, Figure 3D), and the ratio of Firmicutes to Bacteroidota was lower in both the MR and MRE groups. The abundance of Jeotgalicoccus was significantly reduced in both the MR and MRE groups, and the abundance in the MRE group was the lowest (Figure 3E, P < 0.05). The abundance of Alistipes was found to be decreased, while the abundance of Alloprevotella was found to be increased in the MRE group compared to the C group (P < 0.01). The abundance of Bacteroides was increased in both the MR and MRE groups compared to the C group (P < 0.05 and P < 0.01, respectively). Moreover, the abundance of Turicibacter was decreased in both the MR and MRE groups (P < 0.05 and P < 0.01, respectively). The MRE significantly reduced the abundance of Ruminococcaceae compared with the C and MR groups (P < 0.05).
As shown in Figure 3F, the taxonomic biomarkers were Jeotgalicoccus, Christensenellaceae_R-7_group, Jeotgalibaca, Alistipes, Dorea, Clostridium_sensu_stricto_1, Facklamia, and Ruminococcaceae in the C group. In the MRE group, the predominant bacteria were Bacteroides, Prevotella, Collinsella, Ruminococcus_gauvreauii_group, Syntrophococcus, Subdoligranulum, and Erysipelotrichaceae_UCG-003. Additionally, no taxonomic biomarkers were found in the MR group under this condition.
Relationship between bacterial and phenotypic variables
The correlations between fecal microbes, performance, and blood parameters were examined to further identify the underlying mechanisms. As shown in Figure 4, the concentration of MDA was positively correlated with Bacteroides and Prevotella. Alistipes showed a negative correlation with GSH-PX. Moreover, Succiniclasticum showed a positive correlation with CAT, T-AOC, NEFA, and isoacid proportions, while Ruminococcus was positively correlated with only isoacid proportions. Lachnospiraceae and Ruminococcaceae were positively linked with SOD. The results showed that the genera Psychrobacter, Atopostipes, Jeotgalibaca, Corynebacterium, Aerococcaceae, Bifidobacterium, Coprococcus, and Facklamia were positively related to the ADG.
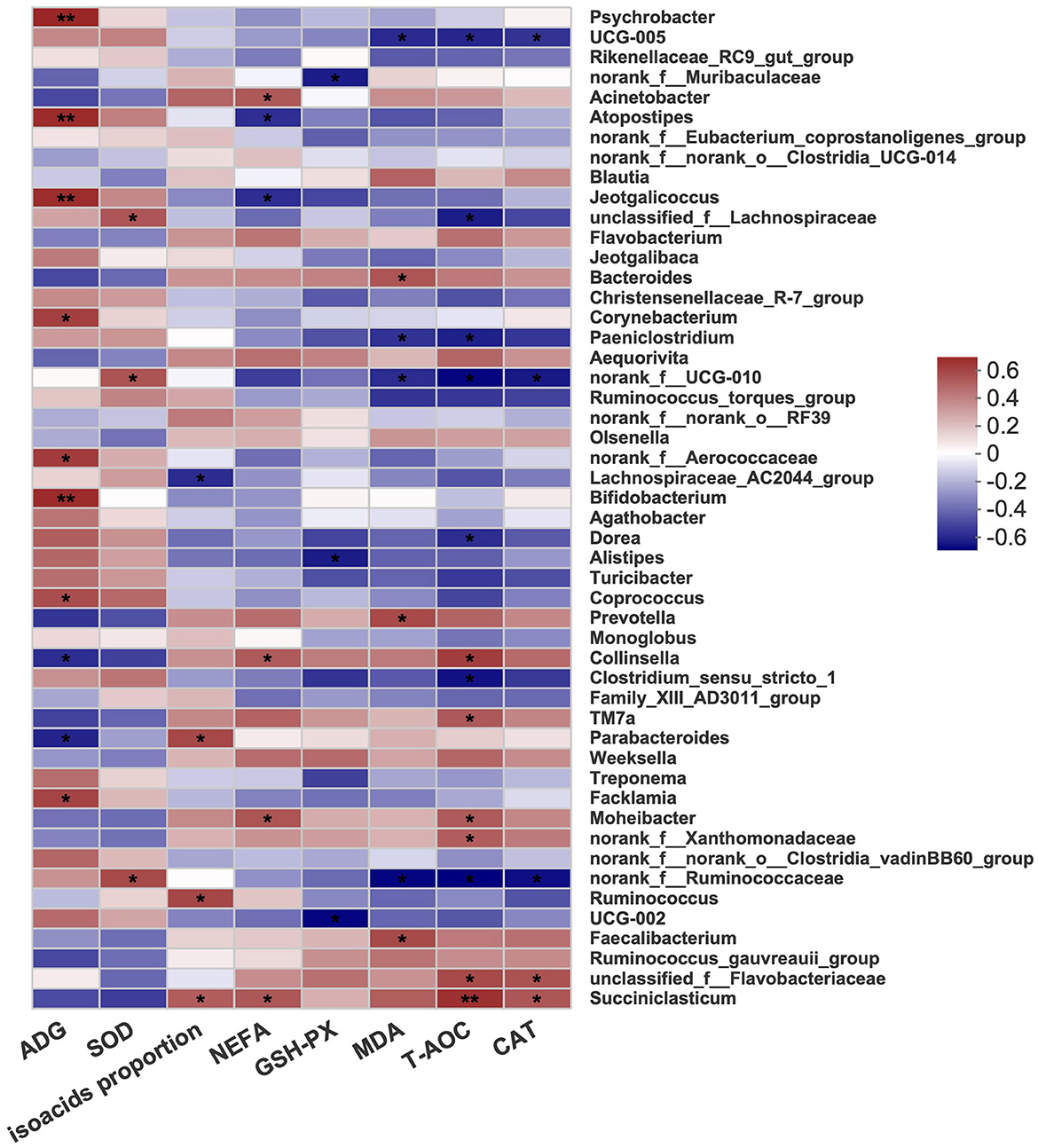
Figure 4. Heatmap diagram of correlations between fecal bacterial and performance and blood parameters at the genus level. Red was positively correlated and blue was negatively correlated. C, calves fed fresh milk; MR, calves fed milk replacer; MRE, calves fed milk replacer plus ethoxyquin. N = 5 in each group. Correlation significance P-value was indicated by “*”. *P < 0.05, **P < 0.01. ADG: average daily gain; SOD, superoxide dismutase; NEFA, nonesterified fatty acid; GSH-PX, glutathione peroxidase; MDA, malonaldehyde; T-AOC, total antioxidant capacity; CAT, catalase.
Discussion
This experiment evaluated the effects of milk, milk replacer, and ethoxyquin on growth performance, weaning stress, and fecal microbiota in dairy calves.
In this study, we found that the calves fed milk demonstrated superior growth performance and higher weaning weights.
The milk-fed calves showed better overall BW than those fed milk replacers (Zhang et al., 2019; Qadeer et al., 2021; Wang et al., 2022). The authors believe that this change might be due to the lower fat and protein contents and poor utilization of non-milk proteins. Moreover, milk might have better bioavailability of protein and energy along with minerals, enzymes, and growth factors (Lee et al., 2009). At the beginning and first few weeks, the BW of the dairy calves was not changed, while the milk replacer decreased the BW during weeks 4–7. A similar result was found in the study by Zhang et al. (2019), in which the BW of calves fed MR was significantly lower than that of those fed milk at 58 days of age. The nutrient contents of milk replacers were different in this study and that by Zhang et al. (2019). Previous studies demonstrated that the nutritional composition and the number of milk replacers provided significantly affect the BW of calves (Geiger et al., 2014; Chapman et al., 2016; Qadeer et al., 2021), suggesting that these factors are crucial.
The starter intake in the first 2 months was significantly associated with milk, fat, and protein production in the first lactation and lifetime production (Heinrichs and Heinrichs, 2011). Starter intake has been reported to be higher in milk-fed calves than those fed milk replacers, thus contributing to a higher growth rate (Qadeer et al., 2021). However, there was no difference in starter intake between the calves fed milk and those fed milk replacers in this study. The ethoxyquin helped to narrow the differences in BW and ADG between the calves fed milk and milk replacers. It might be due to the increased starter intake, as it could result in the compensation of nutrients to meet growth requirements. Feeding cows with ethoxyquin increased their DMI during mid and late lactation (Váquez-Añón et al., 2008).
Moreover, the higher consumption of starter intake may improve early rumen microbial development, leading to greater rumen capacity and metabolic activity (Anderson et al., 1987; Khan et al., 2011). Smith et al. (2003) reported that feeding antioxidants mixed with ethoxyquin improved the organic matter's digestibility. We speculated that the digestibility of DM and CP would be increased, and more research is needed to verify this fact.
In this study, the milk replacer did not change the blood metabolites, while the milk replacer plus ethoxyquin elevated the plasma concentration of NEFA. Feeding branched-chain VFA could decrease NEFA concentration in dairy cows (Liu et al., 2009), and a greater concentration of ruminal isovalerate might induce lower NEFA in calves (Zhang et al., 2019). Ethoxyquin increased the starter intake in this study, indicating that the rumen fermentation and bacterial community would be altered (Zhang et al., 2019). Moreover, the plasma NEFA is usually derived from fat stores as a response to energy mobilization; however, in this study, the BW and starter intake were increased in the MRE group compared to the MR group, indicating that an increase in NEFA by ethoxyquin would be due to the rumen metabolism but not the fat mobilization. A previous study demonstrated that antioxidants, such as vitamin E, can potentially prevent the “trans-10 shift” during biohydrogenation (Pottier et al., 2006). The ethoxyquin supplementation would preserve the oxidation of unsaturated fatty acids before absorption (Andrews et al., 2006) and increase the cis-18:1 in milk (Váquez-Añón et al., 2008), suggesting lower ruminal hydrogenation and trans-isomerization of 18:1 in some cases. The question of whether and how the ethoxyquin supplementation altered the rumen fermentation and microbiota profile will be evaluated in future studies.
Weaning is a potent stressor for dairy calves because of the extreme dietary shift, which induces elevated blood reactive oxygen species (ROS) production (Bordignon et al., 2019). Maintaining a balance between the defensive ability (enzymatic system and non-enzymatic antioxidants) and ROS production is important. Any disruption in this balance can lead to oxidative stress (Lykkesfeldt and Svendsen, 2007). The observed increase in antioxidant ability was expected, as ethoxyquin is one of the well-known feed antioxidant for both domestic animals and fish. It is widely used in animal feed due to its high antioxidant capacity and low production costs (Błaszczyk et al., 2013). Greater SOD and GSH-PX activities resulted from ethoxyquin supplementation in lactating primiparous cows (Váquez-Añón et al., 2008). As suggested by the authors, it is possible that ethoxyquin reduced a load of peroxides by removing reactive oxygen molecules, thereby relieving the endogenous antioxidant defense system. The elevated antioxidative ability we found in this study may have helped to mitigate the weaning impact on the calves.
In this study, the concentration of acetic acid was increased in the MR and MRE groups. The isoacids are the sum of isobutyric acid, isovaleric acid, and valeric acid. Branched-chain VFAs are markers of protein fermentation and are primarily derived from the fermentation of branched-chain amino acids such as valine and leucine (Smith and Macfarlane, 1998). In a study by Kumar et al. (2021), a higher concentration of milk replacer induced lower acetic acid and valeric acid proportions. Thus, we presumed that the higher concentrations of milk replacer-derived proteins and peptides would reach the intestine.
Despite the fecal fermentation, the microbiota was also evaluated in this study. The composition and balance of the microbiota are closely related to the nutritional and physiological functions of the host. Milk replacer has been shown to increase the diversity and richness of the microflora in the ileum (Wang et al., 2021). The results of Badman et al. (2019) proved that the differences in the nutritional composition of bovine milk replacers had a major impact on microbiota composition, diversity, and succession in pre-weaned dairy calves, further influencing the health of the gut and the whole animal. In this study, milk replacers did not affect the richness of the microflora, and milk replacer plus ethoxyquin decreased the richness with no difference in diversity. Combining the heatmap diagram and PCA, the results suggested that ethoxyquin might alter the composition of a milk replacer containing less widely utilized substrates for microbial fermentation.
Despite the genus mentioned above, Firmicutes, Bacteroidetes, Proteobacteria, and Actinomycetes usually account for more than 90% of gut microbes (Sankar et al., 2015). Firmicutes, an important indicator of intestinal microflora's composition, can be converted into short-chain fatty acids by fermenting polysaccharides to provide energy (Mariat et al., 2009). Firmicutes were also shown to promote energy absorption and fat deposition (Turnbaugh et al., 2006). We found that the abundance of firmicutes decreased in the MRE group compared to the C group, which suggested that the energy absorption and fat deposition would be lower, further favoring the elevated NEFA concentration. A previous study showed an increase in the abundance of Bacteroidetes in obese animals fed high-fiber diets (de Wit et al., 2012). The children who consumed a diet rich in fiber had higher proportions of Bacteroidetes and fewer Firmicutes than those fed a diet that included large amounts of protein, fat, sugar, and starch (De Filippo et al., 2010). However, we are unsure of why ethoxyquin can cause these changes.
Moreover, the ratio of Firmicutes/Bacteroidetes was significantly decreased. A previous meta-analysis revealed that a higher Firmicutes/Bacteroidetes ratio suggested more energy extraction from food by the microbiota (Suzuki and Worobey, 2014). Thus, we speculated that milk replacer and milk replacer plus ethoxyquin might alter the energy extraction from feed through microbiota, which could, in some cases, explain the decreased BW and ADG in this study. Moreover, we found that the abundance of Bifidobacterium was strongly correlated with ADG. Bifidobacterium is often among the first colonizers of gut environments (Malmuthuge et al., 2015) and is known to be beneficial to physiological conditions within the gut, aiding intestinal development and preventing intestinal dysbiosis (Hidalgo-Cantabrana et al., 2017).
The VFAs are important metabolites of the microbiota. Generally, acetic acid, propionic acid, and butyric acid are the primary fermentation products of Ruminococcus albus, Prevotella ruminicola, and Butyrivibrio fibrisolvens, respectively (Emerson and Weimer, 2017; Liu et al., 2017). The lower abundance of Ruminococcaceae in the MRE group tend to decrease the concentration of acetic acid. A previous study showed that the fermentation of branched-chain amino acids is mainly carried out by members of the genera Clostridium, Peptostreptococcus, and Bacteroides (Smith and Macfarlane, 1998), and the abundance of Bacteroides was found elevated in the calves fed milk replacer plus ethoxyquin in this study. Both Ruminococcus albus and Butyrivibrio fibrisolvens species are the main consumers of branched-chain VFAs (Feng, 2004). We also found that the abundance of Ruminococcaceae was lower in the MRE group, and the abundance of Ruminococcus, Succiniciasticum, and Parabacteroides were positively related to the isoacids proportion. Therefore, the decreased abundance of these bacteria might explain the greater proportion of isoacids. Moreover, previous studies demonstrated that isoacids could increase the number of cellulolytic bacteria (fibrobacter succinogenes, Ruminococcus flavefaciens, and so on) (Bryant and Doetsch, 1954; Dehority et al., 1967). Some in vitro studies have shown that isoacids can accelerate the degradation of DM and NDF (Soofi et al., 1982; Roman-Garcia et al., 2021). They tended to increase the proportion of isoacids, further suggesting that milk replacer and milk replacer plus ethoxyquin might influence the degradation and usage of feedstuffs. These might explain the increased starter intake in the MRE group.
The concentration of NEFA, the T-AOC, and the enzyme activity of CAT was positively correlated with Succiniclasticum, which is involved in the production of propionate (van Gylswyk, 1995). A lower abundance of Ruminococcaceae was detected in rats that were fed a high-fat diet (Zhao et al., 2017). The serum indicators of inflammation, such as TNF-α and IL-6, significantly increased. In this study, we also found that the antioxidative ability was negatively related to Ruminococcaceae, and the lower abundance of Ruminococcaceae further indicated a better health condition with ethoxyquin supplementation. Moreover, Alistipes, a potential opportunistic pathogen in diseases and highly relevant to dysbiosis and inflammation (Kong et al., 2019; Parker et al., 2020), was found to decrease and be negatively correlated with GSH-PX. These results also suggested that ethoxyquin improved the bacterial community.
Conclusion
The results of this study suggest that milk replacers may not be sufficient to promote optimal growth performance in Holstein dairy calves during the early stages of life and that The addition of ethoxyquin could increase starter intake, thus narrowing the differences between the milk-fed and milk-replacer-fed calves. The results also suggested that milk replacer plus ethoxyquin enhanced the defensive ability and improved microbial composition to mitigate the negative effects of weaning.
Data availability statement
The datasets presented in this study have been deposited in the NCBI repository, accession number PRJNA914621 (https://www.ncbi.nlm.nih.gov/bioproject/PRJNA914621).
Ethics statement
The animal study was reviewed and approved by the Animal Care and Use Committee of Zhejiang A&F University, Zhejiang, China.
Author contributions
XW and CW designed and supervised the study and revised the manuscript. XW, JZ, JY, and YW conducted the experiments. JZ, YZ, and JW performed the data analysis. XW drafted the manuscript. All authors read and approved the final version of the manuscript.
Funding
The financial supports were received from the National Natural Science Foundation of China (32172742 and 32202688), Zhejiang Provincial Science and Technology Plan Project (2022C04038), Science and Technology Cooperation Plan of SanNongLiuFang of Zhejiang Province (2020SNLF019), and Zhejiang Animal Husbandry Industry Technology Project.
Acknowledgments
We appreciate the members of CW's laboratory for helping with animal care and sample collection.
Conflict of interest
YW was employed by Kemin (China) Technologies Co., Ltd. (Zhuhai, China).
The remaining authors declare that the research was conducted in the absence of any commercial or financial relationships that could be construed as a potential conflict of interest.
Publisher's note
All claims expressed in this article are solely those of the authors and do not necessarily represent those of their affiliated organizations, or those of the publisher, the editors and the reviewers. Any product that may be evaluated in this article, or claim that may be made by its manufacturer, is not guaranteed or endorsed by the publisher.
References
Anderson, K. L., Nagaraja, T. G., Morrill, J. L., Avery, T. B., Galitzer, S. J., and Boyer, J. E. (1987). Ruminal microbial development in conventionally or early weaned calves. J. Anim. Sci. 64, 1215–1226. doi: 10.2527/jas1987.6441215x
Andrews, J., Vazquez-Anon, M., and Bowman, G. (2006). Fat stability and preservation of fatty acids with AGRADO antioxidant in feed ingredients used in ruminant rations. J. Dairy Sci. 89, 60.
Badman, J., Daly, K., Kelly, J., Moran, A. W., Cameron, J., Watson, I., et al. (2019). The effect of milk replacer composition on the intestinal microbiota of pre-ruminant dairy calves. Front. Vet. Sci. 6, 371. doi: 10.3389/fvets.2019.00371
Bernabucci, U., Basiricò, L., Morera, P., Dipasquale, D., Vitali, A., Piccioli, C. F., et al. (2015). Effect of summer season on milk protein fractions in Holstein cows. J Dairy Sci. 98, 1815–1827. doi: 10.3168/jds.2014-8788
Błaszczyk, A., Augustyniak, A., and Skolimowski, J. (2013). Ethoxyquin: An antioxidant used in animal feed. Int. J. Food Sci. 2013, 585931. doi: 10.1155/2013/585931
Boerman, J. P., Preseault, C. L., and Lock, A. L. (2014). Effect of dietary antioxidant and increasing corn oil inclusion on milk fat yield and fatty acid composition in dairy cattle. J. Dairy Sci. 97, 7697–7705. doi: 10.3168/jds.2013-7701
Bordignon, R. A., Volpato, P., Glombowsky, C. F., Souza, M. D., Baldissera, R., Secco, W. A. B., et al. (2019). Nutraceutical effect of vitamins and minerals on performance and immune and antioxidant systems in dairy calves during the nutritional transition period in summer. J. Therm. Biol. 84, 451–459. doi: 10.1016/j.jtherbio.2019.07.034
Bryant, M. P., and Doetsch, R. N. (1954). Factors necessary for the growth of Bacteroides succinogenes in the volatile acid fraction of rumen fluid. Science. 120, 944–945. doi: 10.1126/science.120.3127.944.b
Chapman, C. E., Erickson, P. S., Quigley, J. D., Hill, T. M., Bateman, H. N., Suarez-Mena, F. X., et al. (2016). Effect of milk replacer program on calf performance and digestion of nutrients with age of the dairy calf. J. Dairy Sci. 99, 2740–2747. doi: 10.3168/jds.2015-10372
Chester-Jones, H., Heins, B., Ziegler, D., Schimek, D., Schuling, S., Ziegler, B., et al. (2017). Relationships between early-life growth, intake, and birth season with first-lactation performance of Holstein dairy cows. J. Dairy Sci. 100, 3697–3704. doi: 10.3168/jds.2016-12229
De Filippo, C., Cavalieri, D., Di Paola, M., Ramazzotti, M., Poullet, J. B., Massart, S., et al. (2010). Impact of diet in shaping gut microbiota revealed by a comparative study in children from Europe and rural Africa. Proc Natl Acad Sci U S A. 107, 14691–14696. doi: 10.1073/pnas.1005963107
de Passillé, A. M., Borderas, T. F., and Rushen, J. (2011). Weaning age of calves fed a high milk allowance by automated feeders: effects on feed, water, and energy intake, behavioral signs of hunger, and weight gains. J Dairy Sci. 94, 1401–1408. doi: 10.3168/jds.2010-3441
de Wit, N., Derrien, M., Bosch-Vermeulen, H., Oosterink, E., Keshtkar, S., Duval, C., et al. (2012). Saturated fat stimulates obesity and hepatic steatosis and affects gut microbiota composition by an enhanced overflow of dietary fat to the distal intestine. Am. J. Physiol. Gastrointest. Liver Physiol. 303, G589–99. doi: 10.1152/ajpgi.00488.2011
Dehority, B. A., Scott, H. W., and Kowaluk, P. (1967). Volatile fatty acid requirements of cellulolytic rumen bacteria. J. Bacteriol. 94, 537. doi: 10.1128/jb.94.3.537-543.1967
Emerson, E. L., and Weimer, P. J. (2017). Fermentation of model hemicelluloses by Prevotella strains and Butyrivibrio fibrisolvens in pure culture and in ruminal enrichment cultures. Appl. Microbiol. Biotechnol. 101, 4269–4278. doi: 10.1007/s00253-017-8150-7
Emsen, E., Yaprak, M., Bilgin, O., and Ockermanb, H. (2004). Growth performance of Awassi lambs fed calf milk replacer. Small Ruminant Res. 53, 99–102. doi: 10.1016/j.smallrumres.2003.08.018
Geiger, A. J., Ward, S. H., Williams, C. C., Rude, B. J., Cabrera, C. J., Kalestch, K. N., et al. (2014). Short communication: Effects of increasing protein and energy in the milk replacer with or without direct-fed microbial supplementation on growth and performance of preweaned Holstein calves. J. Dairy Sci. 97, 7212–7219. doi: 10.3168/jds.2013-7000
Gensollen, T., Iyer, S. S., Kasper, D. L., and Blumberg, R. S. (2016). How colonization by microbiota in early life shapes the immune system. Science. 352, 539–544. doi: 10.1126/science.aad9378
Godbout, J. P., and Glaser, R. (2006). Stress-induced immune dysregulation: implications for wound healing, infectious disease and cancer. J. Neuroimmune Pharmacol. 1, 421–427. doi: 10.1007/s11481-006-9036-0
Heinrichs, A. J., and Heinrichs, B. S. (2011). A prospective study of calf factors affecting first-lactation and lifetime milk production and age of cows when removed from the herd. J. Dairy Sci. 94, 336–341. doi: 10.3168/jds.2010-3170
Hidalgo-Cantabrana, C., Delgado, S., Ruiz, L., Ruas-Madiedo, P., Sánchez, B., and Margolles, A. (2017). Bifidobacteria and their health-promoting effects. Microbiol Spectr. 5, 3. doi: 10.1128/microbiolspec.BAD-0010-2016
Iqbal, Z., Hayat, Z., Abdullah, M., Javed, K., and Ahamd, N. (2014). Milk and milk replacer performance in dairy calves. J. Anim. Plant Sci. 24, 52−54.
Khan, M. A., Lee, H. J., Lee, W. S., Kim, H. S., Kim, S. B., Ki, K. S., et al. (2007). Pre- and postweaning performance of Holstein female calves fed milk through step-down and conventional methods. J. Dairy Sci. 90, 876–885. doi: 10.3168/jds.S0022-0302(07)71571-0
Khan, M. A., Weary, D. M., and von Keyserlingk, M. A. G. (2011). Hay intake improves performance and rumen development of calves fed higher quantities of milk. J. Dairy Sci. 94, 3547–3553. doi: 10.3168/jds.2010-3871
Kim, E. T., Lee, H. G., Kim, D. H., Son, J. K., Kim, B. W., Joo, S. S., et al. (2020). Hydrolyzed yeast supplementation in calf starter promotes innate immune responses in Holstein calves under weaning stress condition. Animals (Basel). 10, 1468. doi: 10.3390/ani10091468
Kong, C., Gao, R., Yan, X., Huang, L., and Qin, H. (2019). Probiotics improve gut microbiota dysbiosis in obese mice fed a high-fat or high-sucrose diet. Nutrition. 60, 175–184. doi: 10.1016/j.nut.2018.10.002
Kumar, S., Khan, M. A., Beijer, E., Liu, J., Lowe, K. K., Young, W., et al. (2021). Effect of milk replacer allowance on calf facal bacterial community profiles and fermentation. Anim. Microbiome. 3, 27. doi: 10.1186/s42523-021-00088-2
Lee, H. J., Khan, M. A., Lee, W. S., Yang, S. H., Kim, S. B., Ki, K. S., et al. (2009). Influence of equalizing the gross composition of milk replacer to that of whole milk on performance of Holstein calves. J. Anim. Sci. 87, 1129–1137. doi: 10.2527/jas.2008-1110
Li, A., Yang, Y., Qin, S., Lv, S., Jin, T., Li, K., et al. (2021). Microbiome analysis reveals gut microbiota alteration of early-weaned Yimeng black goats with the effect of milk replacer and age. Microb. Cell Fact. 20, 78. doi: 10.1186/s12934-021-01568-5
Li, B., Zhang, K., Li, C., Wang, X., Chen, Y., and Yang, Y. (2019). Characterization and comparison of microbiota in the gastrointestinal tracts of the goat (Capra hircus) during preweaning development. Front. Microbiol. 10, 2125. doi: 10.3389/fmicb.2019.02125
Li, C., Wang, W., Liu, T., Zhang, Q., Wang, G., Li, F., et al. (2018). Effect of early weaning on the intestinal microbiota and expression of genes related to barrier function in lambs. Front. Microbiol. 9, 1431. doi: 10.3389/fmicb.2018.01431
Liu, Q., Wang, C., Yang, W. Z., Zhang, B., Yang, X. M., He, D. C., et al. (2009). Effects of isobutyrate on rumen fermentation, lactation performance and plasma characteristics in dairy cows. Anim. Feed Sci. Technol. 154, 58–67. doi: 10.1016/j.anifeedsci.2009.08.001
Liu, Q., Yao, S., Chen, Y., Gao, S., Yang, Y., Deng, J., et al. (2017). Use of antimicrobial peptides as a feed additive for juvenile goats. Sci Rep. 7, 12254. doi: 10.1038/s41598-017-12394-4
Lykkesfeldt, J., and Svendsen, O. (2007). Oxidants and antioxidants in disease: Oxidative stress in farm animals. Vet. J. 173, 502-511. doi: 10.1016/j.tvjl.2006.06.005
Malmuthuge, N., Griebel, P. J., and Guan Le, L. (2015). The gut microbiome and its potential role in the development and function of newborn calf gastrointestinal tract. Front. Vet. Sci. 2, 36. doi: 10.3389/fvets.2015.00036
Mariat, D., Firmesse, O., Levenez, F., Guimarăes, V., Sokol, H., Dor,é, J., et al. (2009). The Firmicutes/Bacteroidetes ratio of the human microbiota changes with age. BMC Microbiol. 9, 123. doi: 10.1186/1471-2180-9-123
Mattioli, G. A., Rosa, D. E., Turic, E., Picco, S. J., Raggio, S. J., Minervino, A. H. H., et al. (2020). Effects of parenteral supplementation with minerals and vitamins on oxidative stress and humoral immune response of weaning calves. Animals (Basel). 10, 1298. doi: 10.3390/ani10081298
Parker, B. J., Wearsch, P. A., Veloo, A. C. M., and Rodriguez-Palacios, A. (2020). The genus Alistipes: gut bacteria with emerging implications to inflammation, cancer, and mental health. Front. Immunol. 11, 906. doi: 10.3389/fimmu.2020.00906
Pottier, J. M., Focant, C., Debier, G., De Buysser, C., Goffe, E., Mi- gnolet, E., et al. (2006). Effect of dietary vitamin E on rumen biohydrogenation pathways and milk fat depression in dairy cows fed high-fat diets. J. Dairy Sci. 89, 685–692. doi: 10.3168/jds.S0022-0302(06)72131-2
Qadeer, M. K., Bhatti, S. A., Nawaz, H., and Khan, M. S. (2021). Effect of milk or milk replacer offered at varying levels on growth performance of Friesian veal calves. Trop. Anim. Health Prod. 53, 290. doi: 10.1007/s11250-021-02666-7
Roman-Garcia, Y., Mitchell, K. E., Denton, B. L., Lee, C., Socha, M. T., Wenner, B. A., et al. (2021). Conditions stimulating neutral detergent fiber degradation by dosing branched-chain volatile fatty acids. II: relation with solid passage rate and pH on neutral detergent fiber degradation and microbial function in continuous culture. J. Dairy Sci. 104. 9, 9853–9867. doi: 10.3168/jds.2021-20335
Sankar, S. A., Lagier, J. C., Pontarotti, P., Raoult, D., and Fournier, P. E. (2015). The human gut microbiome, a taxonomic conundrum. Syst. Appl. Microbiol. 4, 276–286. doi: 10.1016/j.syapm.2015.03.004
Smith, E. A., and Macfarlane, G. T. (1998). Enumeration of amino acid fermenting bacteria in the human large intestine: effects of pH and starch on peptide metabolism and dissimilation of amino acids. FEMS Microbiol. Ecol. 25, 355–368. doi: 10.1111/j.1574-6941.1998.tb00487.x
Smith, J. L., Sheffield, L. G., and Saylor, D. (2003). Impact of ethoxyquin on productivity of dairy cattle. J. Dairy Sci. 85, 358.
Soofi, R., Fahey, G. C., Berger, L. L., and Hinds, F. C. (1982). Effect of branched chain volatile fatty acids, trypticase, urea, and starch on in vitro dry matter disappearance of soybean stover. J. Dairy Sci. 65, 1748–1753. doi: 10.3168/jds.S0022-0302(82)82411-9
Suzuki, T. A., and Worobey, M. (2014). Geographical variation of human gut microbial composition. Biol. Lett. 10, 20131037. doi: 10.1098/rsbl.2013.1037
Toral, P. G., Chilliard, Y., Rouel, J., Leskinen, H., Shingfield, K. J., and Bernard, L. (2015). Comparison of the nutritional regulation of milk fat secretion and composition in cows and goats. J. Dairy Sci. 98, 7277–7297. doi: 10.3168/jds.2015-9649
Turnbaugh, P. J., Ley, R. E., Mahowald, M. A., Magrini, V., Mardis, E. R., and Gordon, J. I. (2006). An obesity-associated gut microbiome with increased capacity for energy harvest. Nature. 444, 1027–1031. doi: 10.1038/nature05414
Ungerfeld, R., Hötzel, M. J., Scarsi, A., and Quintans, G. (2011). Behavioral and physiological changes in early-weaned multiparous and primiparous beef cows. Animal. 5, 1270–1275. doi: 10.1017/S1751731111000334
van Gylswyk, N. O. (1995). Succiniclasticum ruminis gen. nov., sp. nov., a ruminal bacterium converting succinate to propionate as the sole energy-yielding mechanism. Int. J. Syst. Bacteriol. 45, 297–300. doi: 10.1099/00207713-45-2-297
Váquez-Añón, M. J., Nocek, G., Bowman, T., Hampton, C., Atwell, P., Vazquez, T., et al. (2008). Effect of feeding a dietary antioxidant in diets with oxidized fat on lactation performance and antioxidant status of the cow. J. Dairy Sci. 91, 3165–3172. doi: 10.3168/jds.2007-0737
Wang, S., Hu, F., Diao, Q., Li, S., Tu, Y., and Bi, Y. (2022). Comparison of growth performance, immunity, antioxidant capacity, and liver transcriptome of calves between whole milk and plant protein-based milk replacer under the same energy and protein levels. Antioxidants (Basel). 11, 270. doi: 10.3390/antiox11020270
Wang, Y., Li, A., Zhang, L., Waqas, M., Mehmood, K., Iqbal, M., et al. (2019). Probiotic potential of Lactobacillus on the intestinal microflora against Escherichia coli induced mice model through high- throughput sequencing. Microb. Pathog. 137, 103760. doi: 10.1016/j.micpath.2019.103760
Wang, Y., Yang, Y., Zhang, Y., Kulyar, M. F., Waqas, M., Han, Z., et al. (2021). Milk replacer supplementation in early life optimizes the development of intestinal microbes in goats. Microb Pathog. 161, 105210. doi: 10.1016/j.micpath.2021.105210
Wang, Y., and Zuo, M. (2015). Nicotinamide improves sevoflurane-induced cognitive impairment through suppression of inflammation and anti-apoptosis in rat. Int. J. Clin. Exp. Med. 8, 20079–20085.
Zhang, R., Zhang, W. B., Bi, Y. L., Tu, Y., Beckers, Y., Du, H. C., et al. (2019). Early feeding regime of waste milk, milk, and milk replacer for calves has different effects on rumen fermentation and the bacterial community. Animals (Basel). 9, 443. doi: 10.3390/ani9070443
Keywords: milk replacer, ethoxyquin, fecal fermentation, bacteria, body weight, weaning
Citation: Wei X, Zou J, Zhang Y, Yang J, Wang J, Wang Y and Wang C (2023) Effects of milk, milk replacer, and milk replacer plus ethoxyquin on the growth performance, weaning stress, and the fecal microbiota of Holstein dairy calves. Front. Microbiol. 14:1113518. doi: 10.3389/fmicb.2023.1113518
Received: 01 December 2022; Accepted: 09 February 2023;
Published: 13 March 2023.
Edited by:
Ming Fan, University of Guelph, CanadaReviewed by:
Qi Yu Diao, Feed Research Institute (CAAS), ChinaZhijun Cao, China Agricultural University, China
Copyright © 2023 Wei, Zou, Zhang, Yang, Wang, Wang and Wang. This is an open-access article distributed under the terms of the Creative Commons Attribution License (CC BY). The use, distribution or reproduction in other forums is permitted, provided the original author(s) and the copyright owner(s) are credited and that the original publication in this journal is cited, in accordance with accepted academic practice. No use, distribution or reproduction is permitted which does not comply with these terms.
*Correspondence: Chong Wang, d2FuZ2Nvbmc5OTJAMTYzLmNvbQ==