- 1National Institute of Animal Health, National Agriculture and Food Research Organization, Tsukuba, Japan
- 2Graduate School of Veterinary Science, Osaka Metropolitan University, Osaka, Japan
- 3Ehime Chuyo Livestock Hygiene Service Center, Ehime, Japan
- 4Kagoshima Central Livestock Hygiene Service Center, Kagoshima, Japan
- 5Miyazaki Livestock Hygiene Service Center, Miyazaki, Japan
- 6Niigata Chuo Livestock Hygiene Service Center, Niigata, Japan
- 7Kumamoto Chuo Livestock Hygiene Service Center, Kumamoto, Japan
- 8Department of Infectious Medicine, Kurume University School of Medicine, Fukuoka, Japan
- 9Department of Bacteriology, Faculty of Medical Sciences, Kyushu University, Fukuoka, Japan
- 10School of Veterinary Medicine, Rakuno Gakuen University, Ebetsu, Japan
Pathogenic Escherichia coli strains are important causes of several swine diseases that result in significant economic losses worldwide. In Japan, the use of antimicrobials in swine is much higher than that in other farm animals every year. Antimicrobial resistance in pathogenic E. coli strains also heavily impacts the swine industry due to the limited treatment options and an increase in the potential risk of the One Health crisis. In 2016, we investigated 684 Japanese isolates of swine pathogenic E. coli belonging to four major serogroups and reported the emergence and increase in the highly multidrug-resistant serogroups O116 and OSB9 and the appearance of colistin-resistant strains. In the present study, by expanding our previous analysis, we determined the serotypes and antimicrobial resistance of 1,708 E. coli strains isolated from diseased swine between 1991 and 2019 in Japan and found recent increases in the prevalences of multidrug-resistant strains and minor serogroup strains. Among the antimicrobials examined in this study that have been approved for animal use, a third-generation cephalosporin was found to be effective against the most isolates (resistance rate: 1.2%) but not against highly multidrug-resistant strains. We also analyzed the susceptibilities of the 1,708 isolates to apramycin and bicozamycin, both which are available for treating swine in Japan, and found that the rates of resistance to apramycin and bicozamycin were low (6.7% and 5.8%, respectively), and both antimicrobials are more effective (resistance rates: 2.7% and 5.4%, respectively) than third-generation cephalosporins (resistance rate: 16.2%) against highly multidrug-resistant strains.
1. Introduction
Pathogenic Escherichia coli strains are important causes of several diseases in swine worldwide, including neonatal diarrhea, postweaning diarrhea, edema disease, and septicemia. In particular, diarrhea and edema disease result in significant economic losses due to high morbidity and mortality, decreased weight gain, and the cost of treatment (Fairbrother and Gyles, 2012; Abubakar et al., 2017). Antimicrobial resistance (AMR) of pathogenic E. coli also heavily impacts the swine industry because of the limited treatment options and an increased public health crisis due to the potential transfer of AMR genes into food chains (Fairbrother and Gyles, 2012). The emergence of multidrug-resistant (MDR) bacteria, which are defined as bacteria that are resistant to at least one antimicrobial agent in each of three or more drug classes (Magiorakos et al., 2012), are widely recognized as one of the most important current threats to public health (van Duin and Paterson, 2020). In Japan, the use of antimicrobial drugs in farm animals, which has been calculated from the sales volume of veterinary medical products, is highest in swine, followed by poultry and cattle (Hosoi et al., 2013). We previously determined the susceptibilities of the E. coli strains O139, O149, O116, and OSB9 (684 in total), which represent the four major serogroups of E. coli strains isolated between 1991 and 2014 from diseased swine in Japan, to 21 antimicrobials selected from various drug classes and found that most of the 684 strains (91%) were resistant to one or more antimicrobial (Kusumoto et al., 2016a). The O116 and OSB9 strains showed resistance to a wider range of antimicrobials than the O139 and O149 strains, and surprisingly, all and 68% of the O116 and OSB9 strains, respectively, were resistant to fluoroquinolones (FQs) (Kusumoto et al., 2016a). We also investigated the susceptibility of these 684 strains to colistin and demonstrated that 309 (45%) were colistin resistant (Kusumoto et al., 2016b). The rates of colistin resistance among the O139, O149, O116, and OSB9 strains did not differ significantly. Because the isolation rate of colistin-resistant E. coli from healthy animals has been low (1%) in Japan (Suzuki et al., 2016), the recent emergence of colistin-resistant swine pathogenic E. coli was considered to be associated with the use of colistin to treat swine diseases. However, the AMR of swine pathogenic E. coli strains other than the four major serogroups remains unclear.
Colistin has recently become an important antimicrobial in human medicine for the treatment of infectious diseases caused by MDR Gram-negative bacteria such as Pseudomonas aeruginosa, Acinetobacter baumannii, and carbapenem-resistant Enterobacteriaceae (Ruppe et al., 2015). In contrast, colistin has been used in veterinary medicine for several decades, not only for the prevention and treatment of infectious diseases but also for growth promotion (Kempf et al., 2016). With the emergence of colistin resistance gene (mcr-1)-positive bacteria, its use in animals has been re-evaluated in many countries (Shen et al., 2020). Notably, the Food Safety Commission of Japan conducted a risk assessment in 2017 focusing on antimicrobial-resistant E. coli arising from the use of colistin (Food Safety Commission Japan, 2017). Based on this assessment, the Ministry of Agriculture, Forestry and Fisheries of Japan prohibited the use of feed additives, including colistin, and transferred colistin from a first-choice to second-choice drug for the treatment of diseased swine in 2018 (Makita et al., 2020). FQs are also regarded as second-choice drugs for swine in Japan because of their importance in human medicine, and FQ resistance has essentially remained at a low level in commensal E. coli isolated from healthy swine in Japan (Harada and Asai, 2010). As such, the emergence of E. coli strains O116 and OSB9 that are resistant to multiple drugs, including FQs, and colistin-resistant pathogenic E. coli strains are significant risks to animal production. Extensive assessment of the risk of antimicrobial use to select resistant E. coli strains and the development of novel antimicrobial agents effective against resistant strains must control the emergence and spread of AMR strains in both humans and animals under the One Health concept.
In the present study, we collected more extensive data on the AMR of swine pathogenic E. coli than that gathered in previous studies in terms of the number of isolates (1,708 strains) and antimicrobial agents (24 antimicrobials, which included the previously analyzed 22 antimicrobials plus two types of combination drugs composed of β-lactam and β-lactamase inhibitors), to obtain a wider view on the spread of AMR among these strains in Japan. The susceptibility of the 1,708 strains to apramycin and bicozamycin (previously known as bicyclomycin) was also analyzed to evaluate the effectiveness of these antimicrobials against swine pathogenic E. coli.
2. Materials and methods
2.1. Bacterial strains and culture conditions
We investigated 1,708 E. coli strains that were isolated in Japan between 1991 and 2019 from swine that died of neonatal diarrhea, postweaning diarrhea, or edema disease (Table 1; see also Supplementary Table 1 for more detailed information), and 967 of these strains were described in our previous studies (Kusumoto et al., 2016a,b). These strains represent all of the swine disease-associated E. coli strains isolated from the 31 Japanese prefectural Livestock Hygiene Service Centers during this period. Samples collected by the prefectural centers were sent to the National Institute of Animal Health for diagnostic purposes. The bacterial strains were identified as E. coli by biochemical tests using the API20E system and the apiweb database (Sysmex-bioMerieux, Tokyo, Japan) according to the manufacturer’s instructions. The O serogroups of the strains were determined with agglutination tests using antisera that were obtained from the Statens Serum Institute (Copenhagen, Denmark) or Denka Seiken Co., Ltd. (Tokyo, Japan) according to the manufacturer’s instructions. All strains were grown in Mueller-Hinton broth (Becton, Dickinson and Company, Sparks, MD) for 18 h at 37°C.
2.2. Antimicrobial susceptibility testing
The 0.5 McFarland test strain suspensions were prepared using the Prompt System (Becton, Dickenson and Company). The following 23 antimicrobials were tested by the Kirby-Bauer disc diffusion test using Sensi-Disc Susceptibility Discs (Becton, Dickinson and Company) according to the recommendation of the Clinical and Laboratory Standards Institute (CLSI) (ClSI, 2016): ampicillin (10 μg), piperacillin (100 μg), cefazolin (30 μg), cefuroxime (30 μg), cefotaxime (30 μg), cefepime (30 μg), cefoxitin (30 μg), moxalactam (30 μg), aztreonam (30 μg), imipenem (10 μg), meropenem (10 μg), gentamicin (10 μg), kanamycin (30 μg), streptomycin (10 μg), tetracycline (30 μg), chloramphenicol (30 μg), nalidixic acid (30 μg), ciprofloxacin (5 μg), levofloxacin (5 μg), gatifloxacin (5 μg), trimethoprim-sulfamethoxazole (1.25/23.75 μg), ampicillin-sulbactam (10/10 μg), and piperacillin-tazobactam (100/10 μg). We included multiple antimicrobials from each drug class, as bacterial susceptibilities to antimicrobial agents often differ, even among agents belonging to the same class. The MICs of the other three antimicrobials, colistin (Merck KGaA, Darmstadt, Germany), apramycin (Merck), and bicozamycin (MSD Animal Health K.K., Osaka, Japan), were determined by the agar dilution method in accordance with the guidelines of the CLSI (2015). The isolates with a colistin MIC of ≥ 4 μg/ml were considered colistin resistant based on the European Committee on Antimicrobial Susceptibility Testing (EUCAST) criteria (EUCAST, 2021). When the MICs for apramycin and bicozamycin showed bimodal distribution, the breakpoints were set as the midpoint between the two peaks of each MIC distribution by reference to the previous report (Esaki et al., 2004). The E. coli reference strain ATCC 29522 was used as a quality control in the MIC determinations.
The AMR of 684 strains for 22 antimicrobials (ampicillin, piperacillin, cefazolin, cefuroxime, cefotaxime, cefepime, cefoxitin, moxalactam, aztreonam, imipenem, meropenem, gentamicin, kanamycin, streptomycin, tetracycline, chloramphenicol, nalidixic acid, ciprofloxacin, levofloxacin, gatifloxacin, trimethoprim-sulfamethoxazole, and colistin) was determined in our previous studies (Kusumoto et al., 2016a,b), and these data were integrated with the data determined in the present study.
2.3. Statistical analysis
The statistical significance of the observed differences in the percentages of the strains that were resistant to each antimicrobial agent was analyzed by Fisher’s exact test with Bonferroni correction and was calculated by EZR software (Kanda, 2013).
3. Results
3.1. Prevalence of O serogroups in swine pathogenic E. coli isolates
We analyzed 1,708 E. coli strains isolated from diseased swine in Japan between 1991 and 2019 (Table 1; see also Supplementary Table 1 for more details). Among the 1,708 strains, the O serogroups of 967 strains were determined in our previous study (Kusumoto et al., 2016a), and those of the remaining 741 strains were determined in this study. As shown in Table 1, 1,571 strains (92.0%) were classified into 69 O serogroups, while 137 (8.0%) were untypeable (OUT). O139 (25.8%), O149 (20.4%), O116 (13.6%), and OSB9 (7.8%) were predominant among the 69 serogroups, together representing 67.6% of the 1,708 strains examined.
Figure 1A shows the temporal changes in the prevalence of O serogroups between 1991 and 2019. O139 and O149, the two most predominant O serogroups, first appeared in 1991 and 1993, respectively, and their proportions were very high in the period from 1991 to 1999 (41.3% and 49.5%, respectively), but their proportions have gradually decreased over time (20.5% and 15.9%, respectively, in the period from 2015 to 2019). The proportions of O116 and OSB9, which first appeared in 2005 and 2000, respectively, were initially high but have decreased in recent years. In contrast, the proportions of other minor O serogroups have gradually increased in recent years, accounting for 41.5% of the strains isolated in the period from 2015 to 2019. In particular, O141, O2, O9, O86, O45, O142, O103, and O115 exhibited notable increases in prevalence (Supplementary Figure 1).
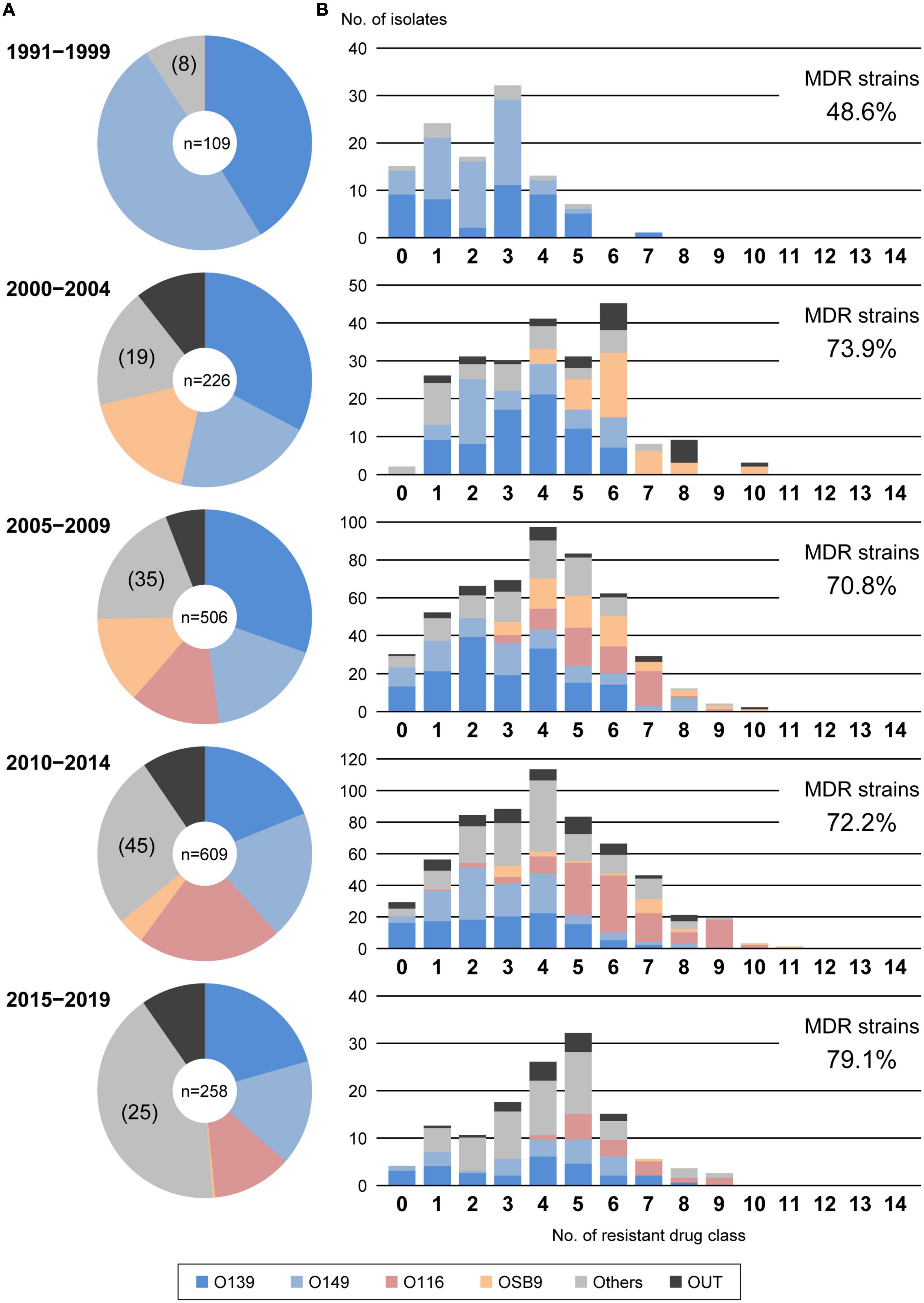
Figure 1. Temporal changes in O serogroups (A) and multidrug resistance levels among each O serogroup (B) in E. coli isolated from diseased swine in Japan. The O serogroup prevalences and multidrug resistance levels between 1991 and 1999, 2000 and 2004, 2005 and 2009, 2010 and 2014, and 2015 and 2019 were compared. O serogroups are indicated with the following colors: blue, O139; light blue, O149; pink, O116; orange, OSB9; dark gray, untypeable (OUT); and gray, O serogroups other than O139, O149, O116, OSB9, and OUT.
3.2. Antimicrobial susceptibility and multidrug resistance
We determined the susceptibility of the 1,708 strains to 24 antimicrobials and analyzed the proportion of strains resistant to each antimicrobial in the entire strain set (Supplementary Table 1). While no strains showed resistance to moxalactam, imipenem, meropenem, or piperacillin-tazobactam, 1,643 strains (96.2%) were resistant to one or more of the 24 antimicrobials. The rates of resistance to cefuroxime, cefotaxime, cefepime, and aztreonam in the entire strain set were extremely low (3.5%, 1.2%, 0.2%, and 1.0%, respectively). In contrast, more than half of the strains showed resistance to six important veterinary antimicrobials: ampicillin (60.5%), streptomycin (58.6%), tetracycline (77.2%), chloramphenicol (54.7%), trimethoprim-sulfamethoxazole (52.6%), and colistin (52.8%).
Analysis of the interserogroup differences in the proportions of strains resistant to each antimicrobial (Figure 2; see also Supplementary Figure 2 for the statistical significance of the differences between O139, O149, O116, and OSB9) revealed that the resistance profiles of O139 and O149 are similar in general, but the O149 strains showed significantly higher resistance rates to seven antimicrobials (cefuroxime, cefotaxime, kanamycin, nalidixic acid, ciprofloxacin, levofloxacin, and gatifloxacin; P < 0.05) and lower rates to colistin (P < 0.001). The profiles of O116 and OSB9 were also similar, but the O116 strains showed higher resistance rates to five antimicrobials (ampicillin, cefoxitin, ciprofloxacin, levofloxacin, and gatifloxacin; P < 0.05) and lower resistance rates to three antimicrobials (cefotaxime, gentamicin, and streptomycin; P < 0.05). Compared to both O139 and O149, O116 and OSB9 showed higher resistance rates to 11 antimicrobials (P < 0.05): ampicillin, cefuroxime, cefoxitin, gentamicin, kanamycin, tetracycline, nalidixic acid, ciprofloxacin, levofloxacin, gatifloxacin, and trimethoprim-sulfamethoxazole (Figure 1 and Supplementary Figure 1). Notable differences were observed for FQ resistance between O139/O149 and O116/OSB9 (P < 0.001), while only 0.5-0.7% and 10.3-12.6% of the O139 and O149 strains were resistant to the three FQs examined, respectively, and 70.0-98.3% and 43.6-81.2% of the O116 and OSB9 strains were resistant to these FQs, respectively.
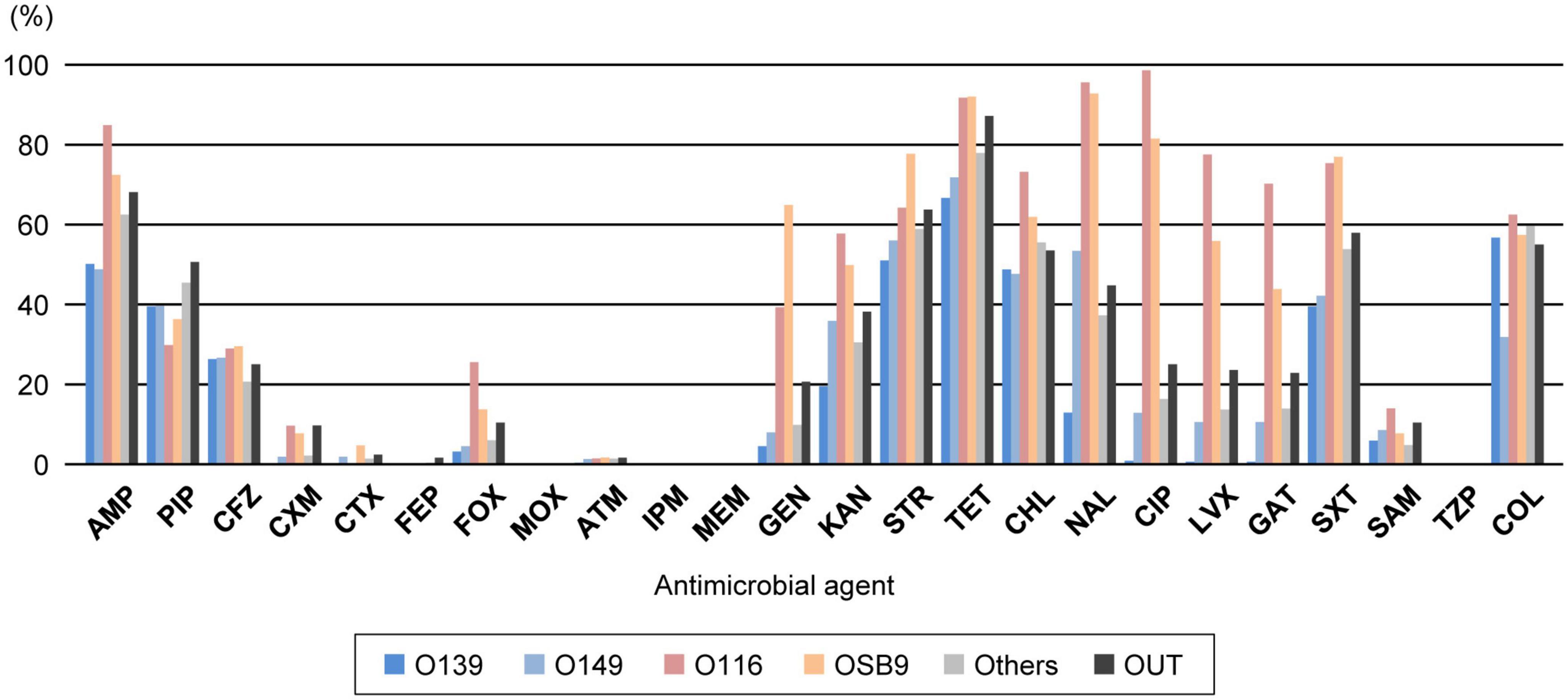
Figure 2. Comparison of the prevalence of O serogroups and rates of resistance to each antimicrobial. AMP, ampicillin; PIP, piperacillin; CFZ, cefazolin; CXM, cefuroxime; CTX, cefotaxime; FEP, cefepime; FOX, cefoxitin; MOX, moxalactam; ATM, aztreonam; IPM, imipenem; MEM, meropenem; GEN, gentamicin; KAN, kanamycin; STR, streptomycin; TET, tetracycline; CHL, chloramphenicol; NAL, nalidixic acid; CIP, ciprofloxacin; LVX, levofloxacin; GAT, gatifloxacin; SXT, trimethoprim-sulfamethoxazole; SAM, ampicillin-sulbactam; TZP, piperacillin-tazobactam; COL, colistin. O serogroups are indicated with the same colors as those in Figure 1.
According to the proposal by Magiorakos et al. (2012), we also evaluated the multidrug resistance of the 1,708 strains by counting the number of strains resistant to 14 antimicrobial drug classes (Supplementary Table 2) and identified 1,222 MDR E. coli strains (71.5%) resistant to three or more drug classes (Figure 3). The numbers of drugs to which the O116 and OSB9 strains showed resistance were significantly higher (median of 6 classes for each) than those for the O139 and O149 strains (median of 3 classes for each) (P < 0.001). Surprisingly, the prevalence of O116 MDR strains was 99.6%, and all OSB9 strains were MDR. Furthermore, 24 O116 (64.9%) and seven OSB9 (18.9%) strains were resistant to more than nine of the 14 drug classes, whereas the O139 and O149 strains did not show extremely high levels of multidrug resistance (Supplementary Table 3). The temporal changes in the level of multidrug resistance between 1991 and 2019 shown in Figure 1B indicate that there were fewer MDR strains in the period from 1991 to 1999 (48.6%) than the number of MDR strains isolated later than 2000 (70.8-79.1%). This increase in the prevalence of MDR strains is mainly attributable to the increase in MDR O116/OSB9 strains, but it is also notable that several minor O serogroups showed a high prevalence of MDR strains: 86.0% (O141), 97.1% (O147), 88.9% (O86), 84.6% (O180), 91.7% (O35), 81.8% (O103), 81.8% (O157), and 85.7% (O98) (Supplementary Figure 1). In addition, notable differences were observed for the temporal change in resistance rate between antimicrobials (Supplementary Figure 3). The prevalence of gentamicin-, nalidixic acid-, FQs (ciprofloxacin, levofloxacin, and gatifloxacin)-, trimethoprim- sulfamethoxazole-, and colistin-resistant strains has also significantly increased after 2000.
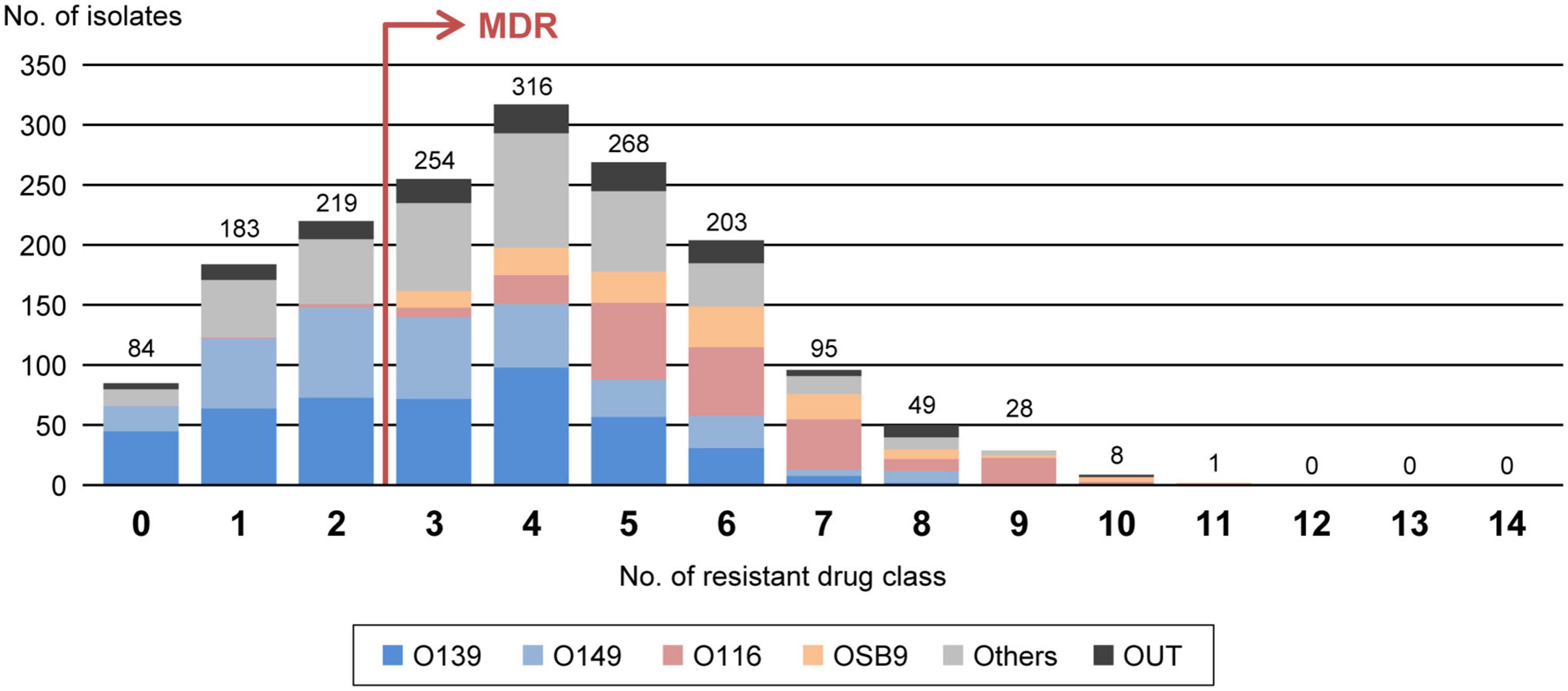
Figure 3. Comparisons of multidrug resistance levels among each O serogroup. The x-axis shows the number of drug classes to which the isolates in the serogroups are resistant. MDR isolates are defined as isolates that are resistant to three or more drug classes, as indicated by the red arrow. The numbers of isolates presenting resistance to each drug class are indicated above the bars. O serogroups are indicated with the same colors as those in Figure 1.
3.3. Apramycin and bicozamycin effectiveness against MDR strains
We analyzed the apramycin and bicozamycin resistance of the 1,708 strains by determining their MICs in the range of 0.125-512 μg/ml for apramycin and 0.5-2,048 μg/ml for bicozamycin (Figure 4). The MICs against apramycin showed bimodal distribution with two peaks at 4 and 512 μg/ml (Figure 4A). When the breakpoint was set to 64 μg/ml, the midpoint between the two peaks, 114 (6.7%) of the 1,708 strains were classified as apramycin resistant (Table 2). The MIC50 and MIC90 values were 4 and 8 μg/ml, respectively. The MICs against bicozamycin also showed bimodal distribution with two peaks at 16 and > 2,048 μg/ml (Figure 4B; note that it was difficult to test concentrations higher than 2,048 μg/ml due to the solubility of bicozamycin). Therefore, a breakpoint for bicozamycin was set to 256 μg/ml, the midpoint between the two peaks. With this breakpoint, 99 (5.8%) of the 1,708 strains were classified as bicozamycin resistant (Table 2). The MIC50 and MIC90 values were 16 and 32 μg/ml, respectively. Apramycin-resistant strains generally showed higher levels of multidrug resistance than bicozamycin-resistant strains, i.e., the median values of the drug classes to which the apramycin strains were resistant were significantly higher than those in bicozamycin-resistant strains (6 classes vs. 4 classes; P < 0.001). More importantly, there were only six strains (0.4%) resistant to both apramycin and bicozamycin in the strain set, meaning that the other 1,702 strains (99.6%) were susceptible to apramycin, bicozamycin, or both (Table 2).
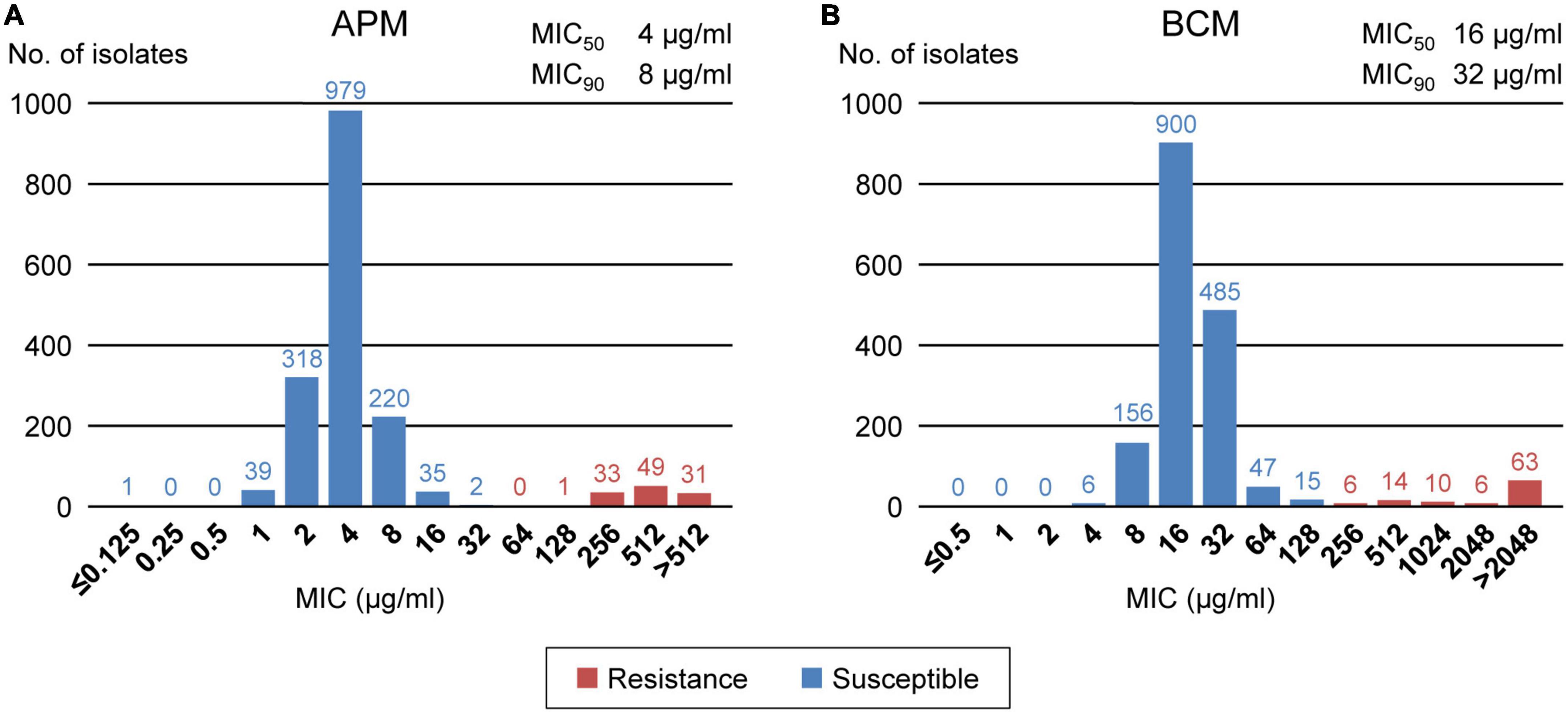
Figure 4. Distributions of the MICs of apramycin (A) and bicozamycin (B) for the 1,708 E. coli isolates from diseased swine in Japan between 1991 and 2019. The numbers of isolates presenting with each MIC are indicated above the bars. The susceptible and resistant isolates are shown in blue and red, respectively. The MIC50 and MIC90 values are shown at the upper right of each panel.

Table 2. Distributions of apramycin- and/or bicozamycin-resistant isolates in each number of resistant drug class.
4. Discussion
Through nationwide analysis of swine pathogenic E. coli strains isolated over 29 years (1991-2019) in Japan, we showed that there were temporal changes in the prevalence of four major serogroups and other serogroups and differences in the AMR profiles between serogroups (Figure 2). O139 and O149 have gradually decreased, and two later appearing serogroups (O116 and OSB9) once became dominant, but their prevalence has also decreased recently. However, these four major serogroups are still important swine pathogens because the O139, O149, and O116 strains are still predominant (altogether representing nearly half of the strains isolated between 2015 and 2019; Figure 1), and O116 and OSB9 include strains with extremely high levels of multidrug resistance (Figure 3). Contrary to the recent decreases in these four serogroups, the prevalence of other minor serogroups has recently increased (representing 41.5% of the strains isolated between 2015 and 2019; Figure 1), indicating that more diverse E. coli strains have recently caused diseases in swine. Notably, many of these strains, e.g., O141, O147, O86, O180, O35, O103, O157, and O98, include a high prevalence of MDR strains (Supplementary Figure 1). In addition, strains showing the same high multidrug resistance levels as the O116 and OSB9 strains were found among the O86, O159, and OUT strains (Figure 3 and Supplementary Table 3). Therefore, continuous monitoring of the prevalence and AMR of not only the major serogroups but also minor serogroups will be needed. The choice and use of proper antimicrobials is also important to achieve the maximum therapeutic benefit while minimizing the selection of resistant bacteria.
In Japan, the following drug classes are not approved for animal use (Ministry of Agriculture, Forestry and Fisheries, 2019): fourth-generation cephalosporins, cephamycins, oxacephems, monobactams, carbapenems, and combinations of β-lactams and β-lactamase inhibitors. These classes include cefepime, cefoxitin, moxalactam, aztreonam, imipenem, meropenem, ampicillin-sulbactam, and piperacillin-tazobactam, which were analyzed in the present study. Therefore, cefuroxime and cefotaxime (second- and third-generation cephalosporins, respectively) are the only effective and approved antimicrobials among the 24 agents analyzed (Figure 2). Notably, the rate of resistance to cefotaxime among the 1,708 strains was extremely low (1.2%). However, in our recent study, we observed a clear association between the therapeutic use of third-generation cephalosporins by swine farms and the selection of swine pathogenic E. coli that show resistance to this and other classes of β-lactams (Misumi et al., 2021). Furthermore, some of the selected E. coli strains produce extended-spectrum β-lactamase (ESBL) encoded by genes such as blaCTX–M–14 and blaCTX–M–15, which have also been found in E. coli isolated from humans (23). ESBL-producing E. coli strains have increasingly been isolated from humans, animals, food, and the environment worldwide and have become a serious concern due to resistance to clinically important third- and fourth-generation cephalosporins (Dorado-Garcia et al., 2018; Bush and Bradford, 2020). Therefore, the use of third-generation cephalosporins is effective against swine pathogenic E. coli but poses a considerable risk to select highly MDR pathogenic E. coli strains.
The effective and approved antimicrobial candidates other than third-generation cephalosporins found in this study are apramycin and bicozamycin. Currently, apramycin and bicozamycin can be used in veterinary medicine in Japan, including to treat diseased swine (Ministry of Agriculture, Forestry and Fisheries, 2019). Apramycin is an aminocyclitol aminoglycoside with a unique chemical structure that differs from that of clinically relevant aminoglycosides (Juhas et al., 2019). Bicozamycin is a unique agent containing a diketopiperazine ring that is not found in any other antimicrobial (Bentley et al., 1993). Therefore, both compounds have a broad spectrum of action, including against MDR Gram-negative bacteria (Kohn and Widger, 2005; Wachino and Arakawa, 2012; Smith and Kirby, 2016; Kang et al., 2017). However, the criteria for determining resistance to them have not yet been defined, and their effectiveness against swine pathogenic E. coli has also yet to be investigated. We therefore investigated the prevalence of swine pathogenic E. coli resistance to these agents. We defined a breakpoint of apramycin at 64 μg/ml (Figure 4A) in the present study, and this breakpoint is reliable because the same concentration was reported by the National Veterinary Assay Laboratory (National Veterinary Assay Laboratory, 2009), Smith and Kirby (2016), and Yang et al. (2020). The National Veterinary Assay Laboratory also determined the MICs of E. coli strains against bicozamycin to be in the range of 0.125–512 μg/ml and set the breakpoint at 128 μg/ml (National Veterinary Assay Laboratory, 2009). We extended the range of MIC measurements to 2,048 μg/ml and found that resistance produced a broad distribution with a peak at > 2,048 μg/ml (Figure 4B). As it is difficult to analyze concentrations higher than that due to the solubility of bicozamycin, the breakpoint defined in the present study (256 μg/ml) is reasonable considering the measurable range of its MIC.
The prevalence of apramycin- and bicozamycin-resistant strains was low (6.7% and 5.8% among the total 1,708 strains, respectively). More importantly, both antimicrobials are effective against highly MDR pathogenic E. coli strains: the rates of resistance to apramycin and bicozamycin among the top 37 MDR isolates (Supplementary Table 3) were much lower (2.7% and 5.4%, respectively) than that the resistance to extended-spectrum cephalosporins (16.2%). In Japan, the sales volumes of apramycin and bicozamycin in 2019 were 2,228.8 kg and 0.2 kg, respectively (Ministry of Agriculture, Forestry and Fisheries, 2019). In contrast, the sales volumes of ampicillin, streptomycin, and oxytetracycline (a commonly used tetracycline for farm animals in Japan), to which many of the E. coli strains showed resistance (Figure 2), were 35.9 t, 27.2 t, and 169.8 t, respectively (Ministry of Agriculture, Forestry and Fisheries, 2019). Thus, apramycin and bicozamycin are currently good choices to treat E. coli infection in swine, particularly highly MDR strains. If the amounts of antimicrobials used in farm animals are estimated from these sales volumes, the effectiveness of apramycin and bicozamycin is due not only to their unique chemical structures but also their low usage on farms. In E. coli, the aminoglycoside 3-N-acetyltransferase type IV gene (aac(3)-IV) has been identified as an apramycin resistance gene (Hunter et al., 1993) and is carried by plasmids in most apramycin-resistant strains isolated from swine (Mathew et al., 2003, 2005) and humans (Zhang et al., 2009). Therefore, careful monitoring of the prevalence of aac(3)-IV in swine pathogenic E. coli strains is needed. In contrast, there are few reports of bicozamycin resistance genes. However, it should be noted that bcr1 was found in an integrative and conjugative element in P. aeruginosa (Fonseca et al., 2015). The World Organization for Animal Health (OIE) and the World Health Organization (WHO) have included bicozamycin in the lists “veterinary important antimicrobial agents” and “antimicrobial classes currently not used in humans”, respectively (OIE, 2019; WHO, 2019), and no bicozamycin-based animal drug or feed additive is currently used in the USA (U.S. Food and Drug Administration, 2003) or EU (European Medicines Agency, 2019).
In conclusion, our nationwide analysis of swine pathogenic E. coli strains isolated over 29 years in Japan revealed a recent increase in the prevalence of MDR and minor serogroup strains. We also showed that among the approved antimicrobials in Japan, cefuroxime and cefotaxime are effective, but apramycin and bicozamycin are more effective against pathogenic E. coli strains, including those showing a high level of multidrug resistance. However, as heavy use of antimicrobials is an important risk factor for AMR (Paterson and Bonomo, 2005), apramycin and bicozamycin (and third-generation cephalosporins such as cefotaxime) should be used prudently to achieve the maximum therapeutic benefit while minimizing the selection of resistant bacteria. Careful monitoring of the use of these antimicrobials and their resistance in swine pathogenic E. coli are also needed.
Data availability statement
The original contributions presented in this study are included in the article/Supplementary material, further inquiries can be directed to the corresponding author.
Author contributions
MK conceived and designed the study and wrote the manuscript. YT-A, YH-K, AM, EO, KS, YM, NA, AW-Y, and TI performed the experiments. YO, YG, KN, TH, and MA supervised and made an intellectual contribution to the work. All authors were responsible for acquisition and analysis of data, commented on the draft, and approved the final version of the manuscript.
Funding
This study was conducted under the research project on “Regulatory Research Projects for Food Safety, Animal Health, and Plant Protection (JPJ008617.22682153)” funded by the Ministry of Agriculture, Forestry and Fisheries of Japan. This work was also supported in part by a Grants-in-Aid for Scientific Research (B), KAKENHI Grant Number: JP19H03133 to MK and TH, from the Japan Society for the Promotion of Science (JSPS).
Conflict of interest
The authors declare that the research was conducted in the absence of any commercial or financial relationships that could be construed as a potential conflict of interest.
Publisher’s note
All claims expressed in this article are solely those of the authors and do not necessarily represent those of their affiliated organizations, or those of the publisher, the editors and the reviewers. Any product that may be evaluated in this article, or claim that may be made by its manufacturer, is not guaranteed or endorsed by the publisher.
Supplementary material
The Supplementary Material for this article can be found online at: https://www.frontiersin.org/articles/10.3389/fmicb.2023.1107566/full#supplementary-material
References
Abubakar, R. H., Madoroba, E., Adenubi, O., Morar-Leather, D., and Fasina, F. O. (2017). Bacterial pathogens of pigs with particular reference to Escherichia coli: a systematic review and meta-analysis. J. Vet. Med. Anim. Health 9, 159–185.
Bentley, J., Hyatt, L. S., Ainley, K., Parish, J. H., Herbert, R. B., and White, G. R. (1993). Cloning and sequence analysis of an Escherichia coli gene conferring bicyclomycin resistance. Gene 127, 117–120. doi: 10.1016/0378-1119(93)90625-d
Bush, K., and Bradford, P. A. (2020). Epidemiology of beta-lactamase-producing pathogens. Clin. Microbiol. Rev. 33:e00047-19. doi: 10.1128/CMR.00047-19
CLSI (2015). Methods for Dilution Antimicrobial Susceptibility Tests for Bacteria that Grow Aerobically; Approved Standard-Tenth Edition, Document M07-A10. Wayne, PA: Clinical and Laboratory Standards Institute.
ClSI (2016). Performance Standards for Antimicrobial Susceptibility Testing; Twenty-Sixth Informational Supplement, Document M100-S26. Wayne, PA: Clinical and Laboratory Standards Institute.
Dorado-Garcia, A., Smid, J. H., van Pelt, W., Bonten, M. J. M., Fluit, A. C., and van den Bunt, G. (2018). Molecular relatedness of ESBL/AmpC-producing Escherichia coli from humans, animals, food and the environment: a pooled analysis. J. Antimicrob. Chemother. 73, 339–347. doi: 10.1093/jac/dkx397
Esaki, H., Morioka, A., Ishihara, K., Kojima, A., Shiroki, S., Tamura, Y., et al. (2004). Antimicrobial susceptibility of Salmonella isolated from cattle, swine and poultry (2001-2002): report from the Japanese veterinary antimicrobial resistance monitoring program. J. Antimicrob. Chemother. 53, 266–270. doi: 10.1093/jac/dkh081
EUCAST (2021). Breakpoint Tables for Interpretation of MICs and Zone Diameters, Version 110. Available online at: http://www.eucast.org/clinical_breakpoints/ (accessed October 12, 2021).
European Medicines Agency (2019). Categorisation of Antibiotics in the European Union. Available online at: https://www.ema.europa.eu/en/documents/report/categorisation-antibiotics-european-union-answer-request-european-commission-updating-scientific_en.pdf (accessed October 12, 2021).
Fairbrother, J. M., and Gyles, C. L. (2012). “Colibacillosis,” in Diseases of Swine, 10th Edn, eds J. J. Zimmerman, L. A. Karriker, A. Ramirez, K. J. Schwartz, and G. W. Stevenson (Hoboken, NJ: Wiley-Blackwell), 723–749.
Fonseca, E. L., Marin, M. A., Encinas, F., and Vicente, A. C. (2015). Full characterization of the integrative and conjugative element carrying the metallo-beta-lactamase bla SPM-1 and bicyclomycin bcr1 resistance genes found in the pandemic Pseudomonas aeruginosa clone SP/ST277. J. Antimicrob. Chemother. 70, 2547–2550. doi: 10.1093/jac/dkv152
Food Safety Commission Japan (2017). Antimicrobial-resistant bacteria arising from the use of colistin sulfate in the livestock. Food. Saf. 5, 24–28. doi: 10.14252/foodsafetyfscj.2016033s
Harada, K., and Asai, T. (2010). Role of antimicrobial selective pressure and secondary factors on antimicrobial resistance prevalence in Escherichia coli from food-producing animals in Japan. J. Biomed. Biotechnol. 2010:180682. doi: 10.1155/2010/180682
Hosoi, Y., Asai, T., Koike, R., Tsuyuki, M., and Sugiura, K. (2013). Use of veterinary antimicrobial agents from 2005 to 2010 in Japan. Int. J. Antimicrob. Agents 41, 489–490. doi: 10.1016/j.ijantimicag.2013.01.002
Hunter, J. E., Hart, C. A., Shelley, J. C., Walton, J. R., and Bennett, M. (1993). Human isolates of apramycin-resistant Escherichia coli which contain the genes for the AAC(3)IV enzyme. Epidemiol. Infect. 110, 253–259. doi: 10.1017/s0950268800068175
Juhas, M., Widlake, E., Teo, J., Huseby, D. L., Tyrrell, J. M., Polikanov, Y. S., et al. (2019). In vitro activity of apramycin against multidrug-, carbapenem- and aminoglycoside-resistant Enterobacteriaceae and Acinetobacter baumannii. J. Antimicrob. Chemother. 74, 944–952. doi: 10.1093/jac/dky546
Kanda, Y. (2013). Investigation of the freely available easy-to-use software ‘EZR’ for medical statistics. Bone. Marrow. Transplant. 48, 452–458. doi: 10.1038/bmt.2012.244
Kang, A. D., Smith, K. P., Eliopoulos, G. M., Berg, A. H., McCoy, C., and Kirby, J. E. (2017). Invitro apramycin activity against multidrug-resistant Acinetobacter baumannii and Pseudomonas aeruginosa. Diagn. Microbiol. Infect. Dis. 88, 188–191. doi: 10.1016/j.diagmicrobio.2017.03.006
Kempf, I., Jouy, E., and Chauvin, C. (2016). Colistin use and colistin resistance in bacteria from animals. Int. J. Antimicrob. Agents 48, 598–606. doi: 10.1016/j.ijantimicag.2016.09.016
Kohn, H., and Widger, W. (2005). The molecular basis for the mode of action of bicyclomycin. Curr. Drug. Targets Infect. Disord. 5, 273–295. doi: 10.2174/1568005054880136
Kusumoto, M., Hikoda, Y., Fujii, Y., Murata, M., Miyoshi, H., Ogura, Y., et al. (2016a). Emergence of a multidrug-resistant Shiga toxin-producing enterotoxigenic Escherichia coli lineage in diseased swine in Japan. J. Clin. Microbiol. 54, 1074–1081. doi: 10.1128/JCM.03141-15
Kusumoto, M., Ogura, Y., Gotoh, Y., Iwata, T., Hayashi, T., and Akiba, M. (2016b). Colistin-resistant mcr-1-positive pathogenic Escherichia coli in swine. Japan, 2007-2014. Emerg. Infect. Dis. 22, 1315–1317. doi: 10.3201/eid2207.160234
Magiorakos, A. P., Srinivasan, A., Carey, R. B., Carmeli, Y., Falagas, M. E., and Giske, C. G. (2012). Multidrug-resistant, extensively drug-resistant and pandrug-resistant bacteria: an international expert proposal for interim standard definitions for acquired resistance. Clin. Microbiol. Infect. 18, 268–281. doi: 10.1111/j.1469-0691.2011.03570.x
Makita, K., Fujimoto, Y., Sugahara, N., Miyama, T., Usui, M., Asai, T., et al. (2020). Quantitative release assessment of mcr-mediated colistin-resistant Escherichia coli from Japanese pigs. Food Saf. 8, 13–33. doi: 10.14252/foodsafetyfscj.D-20-00004
Mathew, A. G., Arnett, D. B., Cullen, P., and Ebner, P. D. (2003). Characterization of resistance patterns and detection of apramycin resistance genes in Escherichia coli isolated from swine exposed to various environmental conditions. Int. J. Food. Microbiol. 89, 11–20. doi: 10.1016/s0168-1605(03)00124-7
Mathew, A. G., Garner, K. N., Ebner, P. D., Saxton, A. M., Clift, R. E., and Liamthong, S. (2005). Effects of antibiotic use in sows on resistance of E. coli and Salmonella enterica Typhimurium in their offspring. Foodborne Pathog. Dis. 2, 212–220. doi: 10.1016/S0168-1605(03)00124-7
Ministry of Agriculture, Forestry and Fisheries (2019). Annual Report of Sales Amount and Sales Volume of Veterinary Drugs, Quasi-Drugs and Medical Devices. Available online at: https://www.maff.go.jp/nval/iyakutou/hanbaidaka/pdf/R1_hanbaidaka.pdf (accessed October 12, 2021).
Misumi, W., Funamori, T., Hamada, K., Iwamoto, J., Fujisono, S., Chitose, K., et al. (2021). Association between antimicrobial treatment and resistance of pathogenic Escherichia coli isolated from diseased swine in Kagoshima Prefecture. Japan. J. Vet. Med. Sci. 83, 358–369. doi: 10.1292/jvms.20-0338
National Veterinary Assay Laboratory (2009). Report of the Japanese Veterinary Antimicrobial Resistance Monitoring System 2000 to 2007. Available online at: https://www.maff.go.jp/nval/english/pdf/jvarm2000_2007_final_201005.pdf (accessed October 12, 2021).
OIE, (2019). OIE List of Antimicrobial Agents of Veterinary Importance. Available online at: https://www.woah.org/app/uploads/2021/03/oie-list-antimicrobials.pdf (accessed October 12, 2021).
Paterson, D. L., and Bonomo, R. A. (2005). Extended-spectrum beta-lactamases: a clinical update. Clin. Microbiol. Rev. 18, 657–686. doi: 10.1128/CMR.18.4.657-686.2005
Ruppe, E., Woerther, P. L., and Barbier, F. (2015). Mechanisms of antimicrobial resistance in Gram-negative bacilli. Ann. Intensive Care 5:61. doi: 10.1186/s13613-015-0061-0
Shen, Y., Zhang, R., Schwarz, S., Wu, C., Shen, J., Walsh, T. R., et al. (2020). Farm animals and aquaculture: significant reservoirs of mobile colistin resistance genes. Environ. Microbiol. 22, 2469–2484. doi: 10.1111/1462-2920.14961
Smith, K. P., and Kirby, J. E. (2016). Evaluation of apramycin activity against carbapenem-resistant and -susceptible strains of Enterobacteriaceae. Diagn. Microbiol. Infect. Dis. 86, 439–441. doi: 10.1016/j.diagmicrobio.2016.09.002
Suzuki, S., Ohnishi, M., Kawanishi, M., Akiba, M., and Kuroda, M. (2016). Investigation of a plasmid genome database for colistin-resistance gene mcr-1. Lancet Infect. Dis. 16, 284–285. doi: 10.1016/S1473-3099(16)00008-6
U.S. Food and Drug Administration (2003). Guidance for Industry #152. Evaluating the Safety of Antimicrobial new Animal Drugs with Regard to their Microbiological Effects on Bacteria of Human Health Concern. Available online at: https://www.fda.gov/regulatory-information/search-fda-guidance-documents/cvm-gfi-152-evaluating-safety-antimicrobial-new-animal-drugs-regard-their-microbiological-effects (accessed October 12, 2021).
van Duin, D., and Paterson, D. L. (2020). Multidrug-resistant bacteria in the community: an update. Infect. Dis. Clin. North Am. 34, 709–722. doi: 10.1016/j.idc.2020.08.002
Wachino, J., and Arakawa, Y. (2012). Exogenously acquired 16S rRNA methyltransferases found in aminoglycoside-resistant pathogenic gram-negative bacteria: an update. Drug. Resist. Updat. 15, 133–148. doi: 10.1016/j.drup.2012.05.001
WHO (2019). Critically Important Antimicrobials for Human Medicine: 6th Revision. Available online at: https://www.who.int/publications/i/item/9789241515528 (accessed October 12, 2021).
Yang, Y., Xiao, T., Li, J., Cheng, P., Li, F., Yu, H., et al. (2020). Wild-type cutoff for apramycin against Escherichia coli. BMC Vet. Res. 16:309. doi: 10.1186/s12917-020-02522-0
Keywords: apramycin, bicozamycin, multidrug-resistant, pathogenic Escherichia coli, swine
Citation: Kusumoto M, Tamamura-Andoh Y, Hikoda-Kogiku Y, Magome A, Okuhama E, Sato K, Mizuno Y, Arai N, Watanabe-Yanai A, Iwata T, Ogura Y, Gotoh Y, Nakamura K, Hayashi T and Akiba M (2023) Nationwide analysis of antimicrobial resistance in pathogenic Escherichia coli strains isolated from diseased swine over 29 years in Japan. Front. Microbiol. 14:1107566. doi: 10.3389/fmicb.2023.1107566
Received: 25 November 2022; Accepted: 06 March 2023;
Published: 17 March 2023.
Edited by:
Etienne Giraud, Institut National de la Recherche Agronomique de Toulouse, FranceReviewed by:
Chang-Wei Lei, Sichuan University, ChinaYuqi Yang, Northeast Agricultural University, China
Copyright © 2023 Kusumoto, Tamamura-Andoh, Hikoda-Kogiku, Magome, Okuhama, Sato, Mizuno, Arai, Watanabe-Yanai, Iwata, Ogura, Gotoh, Nakamura, Hayashi and Akiba. This is an open-access article distributed under the terms of the Creative Commons Attribution License (CC BY). The use, distribution or reproduction in other forums is permitted, provided the original author(s) and the copyright owner(s) are credited and that the original publication in this journal is cited, in accordance with accepted academic practice. No use, distribution or reproduction is permitted which does not comply with these terms.
*Correspondence: Masahiro Kusumoto, a3VzdTU1NUBhZmZyYy5nby5qcA==