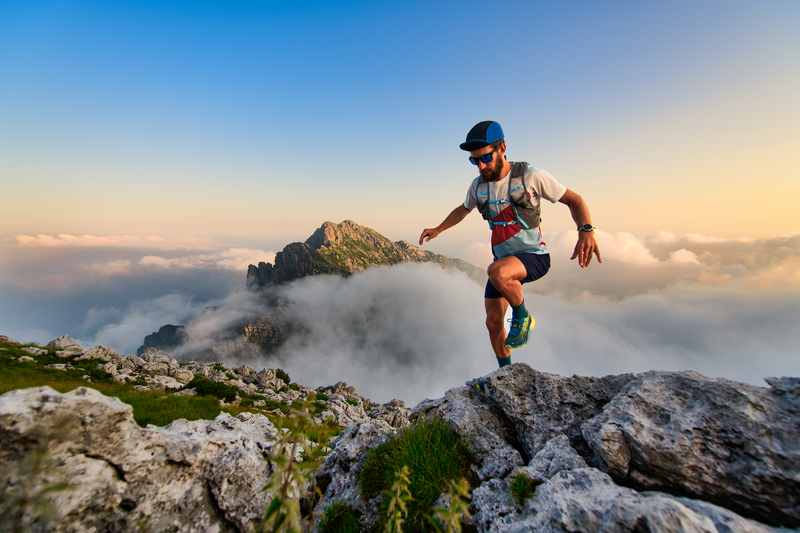
94% of researchers rate our articles as excellent or good
Learn more about the work of our research integrity team to safeguard the quality of each article we publish.
Find out more
ORIGINAL RESEARCH article
Front. Microbiol. , 13 March 2023
Sec. Food Microbiology
Volume 14 - 2023 | https://doi.org/10.3389/fmicb.2023.1106543
This article is part of the Research Topic Aspergillus-Derived Mycotoxins In The Feed And Food Chain, Volume II View all 9 articles
Aflatoxin contamination of the staples maize and groundnut is a concern for health and economic impacts across sub-Saharan Africa. The current study (i) determined aflatoxin levels in maize and groundnut collected at harvest in Burundi, (ii) characterized populations of Aspergillus section Flavi associated with the two crops, and (iii) assessed aflatoxin-producing potentials among the recovered fungi. A total of 120 groundnut and 380 maize samples were collected at harvest from eight and 16 provinces, respectively. Most of the groundnut (93%) and maize (87%) contained aflatoxin below the European Union threshold, 4 μg/kg. Morphological characterization of the recovered Aspergillus section Flavi fungi revealed that the L-morphotype of A. flavus was the predominant species. Aflatoxin production potentials of the L-morphotype isolates were evaluated in maize fermentations. Some isolates produced over 137,000 μg/kg aflatoxin B
1
. Thus, despite the relatively low aflatoxin levels at harvest, the association of both crops with highly toxigenic fungi poses significant risk of post-harvest aflatoxin contamination and suggests measures to mitigate aflatoxin contamination in Burundi should be developed. Over 55% of the L-morphotype A. flavus did not produce aflatoxins. These atoxigenic L-morphotype fungi were characterized using molecular markers. Several atoxigenic genotypes were detected across the country and could be used as biocontrol agents. The results from the current study hold promise for developing aflatoxin management strategies centered on biocontrol for use in Burundi to reduce aflatoxin contamination throughout the value chain.
Production of food crops including maize and groundnut in sub-Saharan Africa (SSA) faces a combination of challenges that reduce yields, including drought, pests, diseases, soil nutrient limitation, shortage of farm inputs and credit, climate change, low labor productivity, and high population pressure on farmland (Keya and Rubaihayo, 2013; Ludgate and Tata, 2015). Additionally, many crops are prone to contamination with aflatoxins, highly toxic secondary metabolites produced by Aspergillus section Flavi fungi (Pildain et al., 2008; Probst et al., 2010; Mutegi et al., 2012). Crop contamination with aflatoxins results in health and economic constraints in many regions (Probst et al., 2012). Also, the toxins increase mortality and reduce productivity in livestock (Ezekiel et al., 2014; Monson et al., 2015). Trade within and between countries is affected by the aflatoxin menace, leading to rejection of consignments, extra costs for product testing, and reduced marketable volumes (Ramesh et al., 2003; PACA, 2012; The Standard, 2019; Daily Nation, 2020).
There are four major aflatoxins: B1, B2, G1, and G2. Aflatoxin B1 is the most prevalent and toxic. Aspergillus flavus and A. parasiticus are the species most implicated in contamination events (Probst et al., 2007; Amaike and Keller, 2011). A. flavus produces B aflatoxins while A. parasiticus produces both B and G aflatoxins (Klich, 2007). A. flavus is subdivided in two morphotypes, L and S, which differ in genetic, physiological, and toxigenic characteristics (Cotty, 1989). Across SSA there are many types of aflatoxigenic fungi, several of them not formally described, that resemble the S-morphotype but that are not closely related (Frisvad et al., 2019; Singh et al., 2020). Some of those types of fungi produce B and G aflatoxins (Cotty and Cardwell, 1999). In the current paper, we use the term ‘fungi with S-morphology’ for all those Aspergillus resembling the S-morphotype of A. flavus, regardless of which aflatoxins they produce.
Demand for maize, an important aflatoxin-prone staple in Burundi, has been increasing due to rapid population growth. Maize production in the country reached over 260,000 tons in 2020 making it the sixth-most important crop after cassava, bananas, sweet potato, beans, and potato (Food and Agriculture Organization of the United Nations, 2022). Maize in Burundi is mainly grown by smallholder farmers (around 0.5 ha/farm) for household consumption (Keya and Rubaihayo, 2013; Ludgate and Tata, 2015). Groundnut, with an annual production of approximately 9,300 tons in Burundi (Food and Agriculture Organization of the United Nations, 2022), is a traditional food mainly consumed as a snack and as a constituent of salads, porridges, and soups. Vulnerability of crops to mycotoxins varies as do their distribution in various crop matrices.
Aflatoxin contamination can start in the field during crop development where it sometimes reaches dangerous levels (Mahuku et al., 2019). However, even when aflatoxin contamination is low at harvest, it can increase to dangerous levels under suboptimal storage (Seetha et al., 2017). In Burundi, aflatoxin content of maize and groundnut at harvest has not been carefully quantified. After harvest, farmers store maize and groundnut in their houses in pots, bags or spread on the floor. In the absence of drying, some such storage conditions can encourage growth of toxigenic fungi and subsequent mycotoxin production. Udomkun et al. (2018) reported high aflatoxin levels in cassava, maize and groundnut foodstuffs collected from local markets in Burundi. Sixty-eight per cent of the 300 collected samples (15% cassava, 77% maize and 84% groundnut) contained aflatoxins levels above the European Union Commission allowable threshold (EUC; 4 μg/kg; sum of aflatoxin B1, B2, G1, and G2) and 37% samples (39% maize and 51% groundnut) exceeded the East African Community (EAC) standard (10 μg/kg total aflatoxins). It is not known whether those levels were due to either pre- or post-harvest contamination, or a combination of both.
To better design appropriate aflatoxin management strategies in Burundi, the current study sought to (i) quantify aflatoxin levels in maize and groundnut collected at harvest across Burundi, (ii) characterize populations of Aspergillus section Flavi associated with the crops, (iii) quantify potentials of the recovered fungi to produce aflatoxins, and (iv) characterize molecularly the atoxigenic fungi. The obtained results provide knowledge on the extent of aflatoxin contamination in maize and groundnut in Burundi at harvest, as well as, the fungi responsible for aflatoxin contamination. A large number of atoxigenic fungi were detected, which can be put to use as components of aflatoxin management strategies across Burundi.
Burundi lies between latitudes 2.3°S to 4.5°S and longitudes 28.8°E to 31°E. The elevation across the country ranges between 770 and 2,670 m above sea level (DFID, 2009). The country has five agro-ecological zones (AEZ), which are described in Table 1. The highest average annual rainfall occurs in the Congo–Nile ridge while the lowest occurs in the East and Northern depressions (Niyongabo, 2008). Most of the topography in Burundi is hilly, which constrains cultivation practices due to soil erosion and makes mechanization difficult. There are two main cropping seasons in Burundi: a short-rain season from September to February and a long-rain season from February to May (DFID, 2009). Smallholder farmers produce maize and groundnut mainly during the short-rain season in Burundi (Collins et al., 2013).
Sampling was conducted across the major maize and groundnut production areas of Burundi (Figure 1). Maize was sampled from 2–3 communes (in parenthesis) in all 16 provinces: Bubanza (Gihanga, Mpanda), Bujumbura (Mutimbuzi, Nyabiraba, Mukike), Bururi (Burambi, Rumonge, Mugamba), Cankuzo (Cankuzo, Gisagara), Cibitoke (Rugombo, Mabayi, Mugina), Gitega (Makebuko, Mutaho), Karuzi (Buhiga, Nyabikere, Bugenyuzi), Kayanza (Gatara, Matongo, Muruta), Kirundo (Busoni, Kirundo), Makamba (Kayogoro, Mabanda, Vugizo), Muramvya (Bukeye, Rutegama), Muyiniga (Giteranyi, Muyinga), Mwaro (Kayokwe, Rusaka), Ngozi (Gashikanwa, Kiremba), Rutana (Bukemba, Musongati), and Ruyigi (Butaganzwa, Kinyinya). Groundnut was sampled from 8 provinces: Makamba, Rutana, Muyinga, Kirundo, Ruyigi, Cankuzo, Gitega, and Muramwa.
A total of 120 groundnut and 380 maize samples were collected from farmers’ fields at harvest, in two batches. The first batch (250) was collected in the lowlands and midlands in March 2014, after the end of the short-rain season. The samples were transported to KALRO Regional Mycotoxin Laboratory in Katumani, Kenya for processing. The second batch of samples (250) were harvested in highland areas in July 2014 at the end of the long-rain season and sent to Katumani for processing and analysis. Up to 10 maize cobs (~1 kg of grains after shelling) were sampled per field while groundnut pods were collected from up to 10 points in each farm (approximately 500 g when shelled). All samples were collected by moving in a zigzag line across each farm.
All cob/pod samples were sundried for 7 d, shelled manually and grain dried in a hot air oven (Memmert, United Kingdom) at 45°C for 48 h, to reach a moisture content of ≤ 13% for maize and ≤ 10% for groundnut. Moisture content was assessed using an Infratec™ 1,241 Grain Analyzer (Foss, Denmark). Then, maize grains were ground using a coffee mill (Bunn-O-Matic Corp., Springfield, IL, United States) and groundnut grains were milled using a blender (BL335, Kenwood Intl., China). Both the coffee mill and blender were washed with 70% ethanol between samples to prevent cross contamination. All samples were divided in two halves, one for microbial and the other for aflatoxin analyzes. Halves for microbial analyzes were stored in hermetic bags at 4°C while those for aflatoxin analyzes were stored at −20°C until analysis.
Aflatoxin levels in maize and groundnut were determined using Reveal Q+ for Aflatoxin kits and Accuscan Pro Reader (Neogen Corp., Lansing, MI, United States) following the manufacturer’s instructions. After homogenizing the sub-samples, 10 g were taken, mixed with 50 ml 65% ethanol, and shaken for 3 min using an orbital shaker (HS501 IKA-WERKE, Germany). The mixture was filtered through fluted Whatman No. 4 filter paper (Whatman Intl. Ltd., Maidstone, England) into a Tri-Pour® beaker. Thereafter, 500 μL sample diluent was transferred to a sample cup and 100 μL of sample filtrate was added and mixed by pipetting up and down seven times. A 100-μL of diluted sample extract was transferred into a new sample cup. A strip was placed into the sample cup and left for 6 min. Then, the strip was read in the Accuscan Pro Reader. The lower detection limit of the Reader was 2 μg/kg while the upper detection limit was 150 μg/kg. Samples with more than 150 μg/kg were serially diluted in 65% ethanol, re-analyzed and the dilution factor was considered during the interpretation of results.
For microbiological analyzes, the sub-samples from batch 1 were sent to IITA Pathology and Mycotoxin Laboratory in Ibadan, Nigeria under appropriate import/export permits provided by phytosanitary authorities. The sub-samples from batch 2 were analyzed in Katumani. Aspergillus section Flavi were isolated and identified as in previous studies in our research group (Atehnkeng et al., 2008; Agbetiameh et al., 2018). Modified Rose Bengal Agar (MRBA; 3 g sucrose, 3 g NaNO3, 0.75 g KH2PO4, 0.25 g K2HPO4, 0.5 g MgSO4.7H2O, 0.5 g KCl, 10 g NaCl, 1 mL A&M micronutrients, 0.025 g Rose Bengal stock solution, 0.05 g chloramphenicol, 1 L distilled water, pH = 6.5) was used for isolation while 5–2 agar (50 mL V-8™ juice, 950 mL distilled water, 20 g agar, pH = 6.0) was used for both identifying Aspergillus section Flavi fungi as well as for saving sporulating cultures. Both media were autoclaved for 20 min at 121°C and cooled to <60°C. After cooling, 0.01 g dichloran and 0.05 g streptomycin sulfate were added to MRBA before pouring into Petri dishes.
Briefly, 1 g of each maize and groundnut sample was obtained from a thoroughly mixed sub-sample and suspended in 10 mL sterile distilled water. The suspension was homogenized by vortexing for 30 s and aliquots of 100 μL were inoculated on MRBA in a biosafety cabinet. Inoculated plates were incubated in the dark (31°C, 3 d). Putative Aspergillus section Flavi colonies were transferred to 5–2 plates using sterile toothpicks. When the number of putative section Flavi colonies per plate exceeded 10, the sub-sample was serially diluted and re-plated. When section Flavi colonies were not detected in a sample, the sub-sample weight or aliquot was increased accordingly. Colony forming units (CFU) of Aspergillus section Flavi per g of maize and groundnut were calculated as follows: CFU/g = (number of colonies × dilution factor) / weight of samples.
Isolates with greenish-yellow colonies and no or large sclerotia were identified as A. flavus L-morphotype. Isolates with numerous small sclerotia were classified as fungi with S-morphology. Colonies showing dark green color and large, rough dark-green spores were assigned as A. parasiticus. Colonies showing brown color were identified as A. tamarii. Pure cultures of 12 isolates per sample were stored in 4 mL vials containing 2 mL sterile water and stored at room temperature (23 ± 2°C) for short-term storage. For long-term storage, cultures were stored on silica gel at 4°C.
Aflatoxin-free maize grains were sourced in both Katumani and Ibadan. Maize samples were analyzed using Accuscan Pro as above and considered as aflatoxin-free when no aflatoxin was detected in five tests. Five grams of aflatoxin-free grains were weighed into 40 mL clear glass vials, washed with tap water, and soaked overnight in 20 mL distilled water to adjust moisture content to 25%. The grains were then washed thrice with tap water to remove any fermentation product and thereafter autoclaved (20 min, 121°C, 15 psi). The sterile grains were independently inoculated with 500-μL spore suspension (approximately 106 spores/mL) of an Aspergillus section Flavi isolate. Cultures were incubated at 31°C (dark, 7 d) for fermentation of maize grains by the inoculated isolates. Vials containing sterile grains inoculated with sterile water were used as controls.
Methodologies reported by Atehnkeng et al. (2008) and Ezekiel et al. (2014) were used to extract and quantify aflatoxins. After incubation, maize fermentations were stopped by adding 50 mL 70% methanol and the colonized grains were ground for 3 min using a high-speed blender (Waring commercial, Springfield, IL, United States). The mixture was transferred to a 250 mL separating funnel and 25 mL distilled water added. Thereafter, aflatoxin was partitioned twice by adding 6.25 mL dichloromethane followed by 2.5 mL dichloromethane. The dichloromethane extracts were passed through a bed of anhydrous sodium sulfate contained in fluted Whatman No. 4 filter paper into a Tri-Pour beaker and evaporated to dryness in the dark in a fume hood. The dried extract was dissolved in 1 mL dichloromethane and poured into a 1.5 mL Eppendorf tube. The toxin was then evaporated to dryness and tubes were stored at 4°C in darkness. Extracts were sent to IITA-Ibadan via courier for quantification.
In Ibadan, aflatoxin extracts were re-dissolved in 1 mL dichloromethane. Then, extracts and aflatoxin standards of known concentrations were spotted on thin-layer chromatography (TLC) plates. The plates were developed in diethylene:methanol:water (96:3:1) solution and then visualized under UV light (365 nm). The presence or absence of aflatoxin B1, B2, G1, and G2 was scored visually. Then, aflatoxins were quantified using a TLC Scanner 3 (CAMAG, Muttenz, Switzerland) with winCATS 1.4.2 software (Camag AG, Muttenz, Switzerland). The limit of quantification for all experiments was 2 μg/kg.
A total of 1,335 A. flavus L-morphotype isolates that did not produce aflatoxins in maize fermentations (1,167 from maize and 168 from groundnut) were characterized using simple sequence repeats (SSRs) developed for A. flavus (Grubisha and Cotty, 2009). Previously described protocols were used to extract DNA from single-spored isolates and conduct the multiplex-PCR and microsatellite genotyping analyzes (Callicott and Cotty, 2015; Islam et al., 2018).
The ability of representative isolates of 11 selected atoxigenic SSR haplotypes to reduce aflatoxin when co-inoculated with a potent aflatoxin-producing A. flavus isolate (BUM009–08 from Burundi) was determined in laboratory competition assays as described earlier (Probst and Cotty, 2012; Agbetiameh et al., 2019). Briefly, inocula of single-spored isolates were grown on 5–2 agar. An equal amount of spore suspensions (1 × 106 spores/mL) of individual atoxigenic isolate and the common toxigenic isolate (1 mL each) were combined and inoculated on 10 g autoclaved maize grain. Maize grains inoculated individually with each atoxigenic isolate, the toxigenic isolate, and water served as controls. There were four replications for each co-inoculation and control treatment. Following inoculation, protocols for maize fermentation and aflatoxin quantification process were similar as described in the previous sections.
Data on CFU/g, frequency of Aspergillus section Flavi species, and aflatoxins produced by the recovered fungi were analyzed using a negative binomial generalized linear model in R studio v3.5.3. Means were separated using Fisher’s protected least significance difference (LSD; α = 0.05). Aspergillus section Flavi isolates were categorized into aflatoxigenic and atoxigenic based on their ability to produce aflatoxin in maize fermentations. Aflatoxin content in samples at harvest was categorized into four levels: (i) aflatoxin below LOD of the kits (no aflatoxin), (ii) aflatoxin below the EUC threshold (4 μg/kg), (iii) aflatoxin above 4 μg/kg but below the EAC threshold (10 μg/kg), and (iv) aflatoxin above the EAC threshold. A chi-square test of association between aflatoxin concentrations and sample type (maize or groundnut) was performed in SPSS v.22 (IBM Corp, New York, United States). Correlation analysis among the population of Aspergillus section Flavi and levels of aflatoxins produced by selected isolates was performed in SPSS v.22.
Before analysis of SSR data, amplicon sizes were converted to repeat number by subtracting the size of the flanking region from the total amplicon size and then dividing by the size of the repeat. Allele frequencies and haplotypes were assessed with GenoDive (Meirmans and Van Tienderen, 2004). Relationships among unique haplotypes were displayed with a Neighbor-Net network generated with SplitsTree4 (Huson and Bryant, 2006) based on chord distances calculated with GenoDive. After sample-correcting the data by removing duplicate, identical haplotypes found in the same sample, an AMOVA (analysis of molecular variance) was performed using Arlequin v3.5.2.2 (Excoffier and Lishcer, 2010) to examine genetic variation by province.
Although 76% of both maize and groundnut contaminated with aflatoxin met the EUC threshold ( < 4 μg/kg), and a few maize (3%) and groundnut (6%) samples contained aflatoxin above the EAC threshold (10 μg/kg; Figure 2). Each of Burambi, Gihanga, Kayogoro, Kinyinya, Mpanda, Rugombo, and Kirundo communes had a maize sample contaminated with aflatoxin above 10 μg/kg, while Mabayi and Butaganzwa had two samples each. On the other hand, four groundnut samples (out of 10) from Vugizo had > 10 μg/kg aflatoxin compared to one sample each from Muyinga, Giteranyi, and Busoni.
Figure 2. Proportion of maize and groundnut samples contaminated with different aflatoxin concentration categories.
There were four types of fungi within Aspergillus section Flavi recovered from maize and groundnut: the A. flavus L-morphotype, fungi with S-morphology, A. parasiticus, and A. tamarii. The Aspergillus section Flavi population densities were significantly (p < 0.001) higher in maize (mean = 1,153 CFU/g) than in groundnut (mean = 266 CFU/g). For maize, there were significant (p < 0.001) differences in fungal densities among provinces (Figure 3A; data of only 10 of the 17 provinces shown) and within communes of a province (data not shown). The fungal density was low (< 50 CFU/g) in Karuzi, Kayanza, Muramvya, Muyinga, Mwaro, and Ngozi. Also, fungal densities in groundnut significantly (p < 0.005) differed among provinces (Figure 3B).
Figure 3. Population (colony forming units per gram) of Aspergillus section Flavi in maize (A) and groundnut (B) sampled from major provinces in Burundi. The letters attached to the bars represent statistical significance at 95% confidence level. Aspergillus population in maize was low in some provinces (Karuzi, Kayanza, Muramvya, Muyinga, Mwaro and Ngozo) and hence data for these provinces not plotted.
The population of Aspergillus section Flavi in both maize and groundnut was dominated by the A. flavus L-morphotype. However, on an average, the proportion was significantly (p < 0.001) higher in maize (92%) than in groundnut (60%; Figures 4A,B). Proportions of Aspergillus section Flavi fungi significantly (p < 0.001) differed among provinces for both crops, with L-morphotype ranging from 85 to 98% in maize, and 45 to 85% in groundnut.
Figure 4. Proportion (%) of members of Aspergillus section Flavi recovered in maize (A) and groundnut (B) from major production provinces in Burundi.
Overall, 44.4% of the Aspergillus section Flavi isolates were toxigenic (Table 2). Proportionally, there were more toxigenic fungi with S-morphology isolates while there were more atoxigenic A. flavus L-morphotype isolates (Table 2). The concentration of aflatoxin B1 was significantly (p < 0.001) higher in isolates recovered from groundnut (mean = 2,617 μg/kg) than in isolates from maize (mean = 1,728 μg/kg). Fungi with S-morphology generally produced higher concentrations of each type of aflatoxin than the other isolates; A. parasiticus produced higher concentrations of B2 in groundnut and of G1 in maize. Overall, 93.5% of the fungi with S-morphology produced aflatoxins (31.9% produced only B aflatoxins); only two isolates of A. parasiticus did not produce aflatoxins. The recovered A. tamarii fungi did not produce aflatoxin, as expected (Table 2).
Table 2. Proportion (%) of aflatoxigenic and atoxigenic fungi in four members of Aspergillus section Flavi recovered from maize and groundnuts in Burundi and aflatoxin-producing potential of the toxigenic fungi.
Concentrations of aflatoxins produced by selected A. flavus L-morphotype isolates had a negative correlation with the population of A. flavus L-morphotype in the samples from which the isolates were recovered (r = −0.508, p < 0.001). On the contrary, aflatoxin concentrations produced by selected fungi with S-morphology had a positive correlation with the population of this type of fungi in samples from which the isolates were recovered (r = 0.589, p < 0.001). These correlations were consistent for the types of aflatoxins produced by the two types of fungi. Moreover, there was a positive correlation between aflatoxin G1 (r = 0.315, p < 0.001) and G2 (r = 0.258, p = 0.002) produced by A. parasiticus with the population of the fungus in the samples from which the isolates were recovered.
Atoxigenic isolates of A. flavus were highly diverse. There were 376 SSR haplotypes among the 1,335 atoxigenic isolates, representing 85 unique SSR haplotypes in the 168 groundnut isolates and 336 unique SSR haplotypes in 1,167 maize isolates. Supplementary Table S1 provides allele sizes of 17 SSR loci (Grubisha and Cotty, 2009) for the 376 SSR haplotypes of atoxigenic A. flavus found in Burundi. A Neighbor-Net visualization of the unique haplotypes (Figure 5) color coded by whether the haplotype was found in maize, groundnut, or both crops, shows a highly diverse collection of genotypes, with no separation by crop origin. A few groups of haplotypes were found only in maize (Figure 5). An AMOVA of isolates by crop origin confirmed this lack of separation, with 99.94% of the genetic variation found within each crop and only 0.06% between the two crops, with a fixation index close to zero (FST = 0.00063; p = 0.25; Supplementary Table S2). Likewise, no significant difference was seen among populations. For example, the 20 most frequent haplotypes were found in, on an average, 10 of the 17 provinces (Supplementary Table S3). This lack of differentiation is also seen using AMOVA; only 0.76% of the variation is attributable to differences among provinces, and as in the crop comparison, the fixation index is very low (FST = 0.0075; p = 0.00, Supplementary Table S4).
Figure 5. Neighbor-Net tree of 376 unique non-aflatoxigenic haplotypes found in Burundi. Haplotypes seen only in maize are shaded red, those found only in groundnut are shaded blue, and those in both crops are shaded black.
Representative isolates of the SSR haplotypes were evaluated for their ability to limit aflatoxin when co-inoculated with the highly toxigenic A. flavus isolate BUM009-08, which produced 4,480 μg/kg aflatoxins when inoculated alone (Table 3). Aflatoxin reduction ranged from 28.3 to 96.0%. Four isolates (BUG241-03, BUG208-07, BUM134-06, and BUM184-12) reduced aflatoxin by > 90%. Two more isolates (BUG242-04 and BUM033-05) had statistically similar, though numerically less, aflatoxin reductions compared to the four isolates named earlier. No aflatoxin was produced in maize grains inoculated with the atoxigenic isolates alone and in water control.
Table 3. Occurrence and distribution of selected atoxigenic haplotypes of Aspergillus flavus in Burundi and aflatoxin reduction by a representative isolate of the 11 haplotypes in maize grains co-inoculated with a toxigenic strains.
The population genetic analyzes revealed several atoxigenic SSR haplotypes widely distributed across Burundi (Figure 6). Some SSR haplotypes were found exclusively in maize or groundnut, while others were found in both crops (Figure 5). The distribution of haplotypes varied within five regions: north, south, center, east, and west (Table 3). Five haplotypes were detected in all five regions while other specific haplotypes were found in 1 to 4 regions. Wide distribution and large number of members were used as criteria for selection, in addition to their abilities to reduce aflatoxin in the co-inoculation. The four isolates selected as active ingredients of the biocontrol product Aflasafe BU01 (BUG241-03, BUG208-07, BUM033-05, and BUM056-02) had medium to high ability to limit aflatoxin contamination (Table 3). Each of the four belonged to a unique SSR haplotype with frequent occurrence and wide distribution in at least 8 provinces located in 4 to 5 regions in Burundi (Figure 6A). Two other isolates (BUM184-12 and BUM021-05) were potentially good candidate active ingredients. Also, the analysis revealed that the genetic groups to which the active ingredient isolates of the biocontrol product Aflasafe KE01 belong to are also native to Burundi (Figure 6B; Supplementary Table S1), in addition to being common in Kenya, for which Aflasafe KE01 was originally developed. SSR fingerprints of the active ingredients of Aflasafe BU01 and Aflasafe KE01 are provided in Table 4.
Figure 6. Distribution across Burundi of the active ingredient atoxigenic Aspergillus flavus isolates composing the aflatoxin biocontrol product Aflasafe BU01 (A) and Aflasafe KE01 (B). Aflasafe KE01 was developed for use in Kenya but its active ingredients, apart from Burundi, have been detected in several other countries.
Table 4. Allele sizes of 17 simple sequence repeat loci (AF28 to AF55; Grubisha and Cotty, 2009) for active ingredients of biocontrol products Aflasafe BU01 and Aflasafe KE01.
In the current study, maize and groundnut produced in Burundi were examined for aflatoxin content at harvest. Over 75% of the crops contained aflatoxin levels considered by the EUC as safe for human consumption (less than 4 μg/kg total aflatoxin). Both crops were associated with various types of aflatoxin-producing fungi, including some with the capacity to contaminate crops with dangerous concentrations of aflatoxins. The fungal communities were dominated by the A. flavus L-morphotype and most members of this group were atoxigenic. Other members of the communities were fungi with S-morphology and A. parasiticus; both of which exhibited high capacity to produce aflatoxins. Within atoxigenic fungi (1,335 isolates), several genotypes associated with both maize and groundnut were detected across Burundi, including genotypes of active ingredients of the aflatoxin biocontrol product Aflasafe KE01already registered for use in Kenya. The results from the current study provide evidence of the extent of aflatoxin contamination of maize and groundnut at harvest in Burundi and revealed structures and compositions of fungal communities associated with the two crops, with some members of those communities posing a risk during the postharvest stage while others with potential for use in aflatoxin management. The identified fungi with potential for use as biocontrol agents in Burundi need to be tested across Burundi to determine their effectiveness in limiting aflatoxin contamination at pre- and post-harvest stages (Bandyopadhyay et al., 2022).
Overall, only a small fraction of the samples contained aflatoxins above 4 μg/kg, the EUC threshold (Figure 2). Aflatoxins were not detected in most (approximately 90%) of the groundnut and maize (50%) samples. The environmental conditions of the five AEZs in Burundi (Table 1) may not allow for aflatoxin production in the field despite the association of both crops with aflatoxin-producers (Figure 3) and the high aflatoxin-production potential of a significant proportion of the associated fungi (Table 2). However, apart from potential aflatoxin contamination in the field, crops associated with potent aflatoxin producers are at high risk of contamination, if stored under sub-optimal conditions (Hell et al., 2008; Seetha et al., 2017; Senghor et al., 2020), or if climate change result in conditions favorable for aflatoxin production. Unfortunately, models indicate that in large portions of East Africa, including Burundi, maize production will become severally affected by climate change (Ojara et al., 2021). In Burundi, as in many other countries in SSA, storage needs significant improvement to discourage post-harvest losses, including aflatoxin contamination (Udomkun et al., 2017). Most maize and groundnut samples met the EUC threshold (<4 μg/kg) and hence were regarded as safe for human consumption. However, low aflatoxin levels at harvest do not necessarily mean that the crops will remain safe, especially in the absence of integrated programs promoting food safety from field to fork.
The A. flavus L-morphotype was the predominant species in both maize and groundnut (Figure 4). Similarly, the L-morphotype composes ~80% of the maize and groundnut communities in various SSA countries (Atehnkeng et al., 2008; Mutegi et al., 2012; Probst et al., 2012). Dominance of the L-morphotype significantly corresponded with low levels of aflatoxin in most maize and groundnut, which concurs with findings of a study in Kenya reporting that the L-morphotype dominated non-aflatoxin-outbreak regions while fungi with S-morphology dominated aflatoxin outbreak regions (Probst et al., 2010). On the other hand, communities of the A. flavus L-morphotype have been reported to produce high aflatoxin levels (Atehnkeng et al., 2008; Okoth et al., 2012; Agbetiameh et al., 2019) and therefore high prevalence of the L-morphotype cannot be used as a conclusive proxy for the aflatoxin concentration of a crop. The incidence of A. parasiticus was relatively low in both groundnut and maize. In other reports, A. parasiticus has been reported to have a high association with groundnut fields (Horn and Dorner, 1998; Kachapulula et al., 2017). However, several studies from our research group have noticed low incidence of A. parasiticus in groundnut samples in certain countries: Ghana (Agbetiameh et al., 2019), Mali and Sudan (unpublished). It is unclear which factor led to relatively low levels of A. parasiticus in groundnut in Burundi.
Around 40% of A. flavus L-morphotype were toxigenic (Table 2). Over 2 decades ago, the same percentage of A. flavus isolated from foods in Burundi were toxigenic (Munimbazi and Bullerman, 1996). In addition, over 90% of both fungi with S-morphology and A. parasiticus exhibited high aflatoxin production potential (Table 2), which is a norm for both groups of fungi. The high levels of aflatoxins (>1,500 μg/kg) in some groundnut samples can be attributed to the presence of potent toxigenic strains. The levels of aflatoxin produced in vitro by fungi with S-morphology and A. parasiticus had a positive correlation with the population of each type of fungi. Similar studies (Jaime-Garcia and Cotty, 2006; Probst et al., 2007, 2012; Mauro et al., 2015) showed a positive correlation between the presence of fungi with S-morphology and high aflatoxin levels. Since the examined crops were collected at harvest, there is still a high risk of contamination during storage and subsequent exposure of consumers of the foodstuffs, if aflatoxin-conducive conditions occur throughout storage and before consumption.
Fungi with S-morphology were more toxigenic than A. flavus L-morphotype, as previously reported (Probst et al., 2007; Mutegi et al., 2012). Therefore, even low levels of fungi with S-morphology are a high risk to accumulation of unsafe aflatoxin levels in crops. Fungi with S-morphology were implicated in the high levels of aflatoxin contamination that claimed more than 125 lives in lower eastern Kenya in 2004 (Probst et al., 2012, 2014). However, Probst et al. (2011) also reported that 33% of A. flavus L-morphotype isolates recovered from Kenya were not toxigenic. The high prevalence of atoxigenic A. flavus L-morphotype isolates is encouraging, as it provides many potential aflatoxin biocontrol agents to protect maize and groundnut (Probst et al., 2011; Agbetiameh et al., 2019). Indeed, when the atoxigenic isolates were genotyped with SSR markers, a few SSR genotypes occurred in high frequency and in several provinces demonstrating wide distribution and hence potential of high adaptation in the country (Table 3; Supplementary Table S1). An extensive Aspergillus population distribution study in Kenyan soil showed that the active ingredients of the biocontrol product Aflasafe KE01 are widely distributed in Kenya (Islam et al., 2021). Isolates of some representative Burundi-specific SSR groups detected in the current study were tested in laboratory assays as potential candidates for the development of biocontrol products for aflatoxin management for use in Burundi (Table 3).
There are several criteria for selecting atoxigenic isolates to constitute biocontrol products containing multiple active ingredients, including frequent occurrence and wide distribution across the target region, membership to VCGs composed entirely of atoxigenic members, superior ability to reduce aflatoxins in laboratory experiments (Moral et al., 2020) and displacement ability in field experiments (Agbetiameh et al., 2019), among others. Recently, our group reported that in some cases, after application, some active ingredient fungi are found at higher proportions in the soil while others in the grain (Atehnkeng et al., 2022). Those results suggest that under some conditions some active ingredient isolates may be better displacers of aflatoxin producers in the soil than the others, and those others may competitively displace better in the grain that would appear as the major contributor on aflatoxin reduction. However, the displacement in the soil is important to prevent aflatoxin producers from reaching the maturing crop.
For biocontrol formulation for Burundi, a balanced selection took into consideration isolates belonging to SSR groups with frequent occurrence and wide distribution, and high aflatoxin reductions. Therefore, there was one isolate (BUM056-02) from a group with frequent occurrence, detected in many regions across Burundi, but with relatively less aflatoxin reduction in the competition experiments (Table 3). We hypothesize that the isolate will compensate its relatively lower ability to reduce aflatoxin in the laboratory with its ability to dominate crops and soils from the target areas. Indeed, initial field testing of the biocontrol product developed for Burundi reveals that treated crops contain lower aflatoxin levels than nontreated crops (data not shown). Thus, the rationale for selecting the active ingredient fungi appears to be correct. Nonetheless, an advantage of characterizing large numbers of A. flavus isolates in Burundi is that a rich germplasm of atoxigenic A. flavus is available to replace any active ingredient isolate of Aflasafe BU01, if performing poorly in the soil and the crop. It has also been argued that aflatoxin biocontrol products containing various active ingredient fungi should contain isolates with opposing mating-type idiomorphs (Moore, 2022). Three of the selected isolates (BUG241-03, BUG208-07, and BUM056-02) contain the MAT1-1 idiomorph while the other (BUM033-05) contains the MAT1-2 idiomorph (data not shown).
In various SSA countries, use of aflatoxin biocontrol products based on atoxigenic fungi have been developed, tested, registered, and transferred to the private sector for large scale use (Probst et al., 2011; Agbetiameh et al., 2019; Bandyopadhyay et al., 2022). Atoxigenic fungi competitively exclude aflatoxin-producing Aspergillus from the crop environment, and this results in low aflatoxin levels in crops at harvest and during storage, even under sub-optimal conditions (Senghor et al., 2020). While there were groups of atoxigenic haplotypes only seen in maize, it is likely that this is due to the much larger maize sample size (Table 2). We detected atoxigenic fungal genotypes never reported outside of Burundi (Figure 6A), but also atoxigenic genotypes native to Kenya (Figure 6B) that have been already registered for use in as active ingredients of the biocontrol product Aflasafe KE01 (Adhikari et al., 2016; Moral et al., 2020). Extension of the label for use of Aflasafe KE01 in groundnut and sorghum is ongoing. Extension of the registration to groundnut and sorghum is in the final stages.
East Africa has a broad diversity of agroecologies including both high elevation and low elevation production areas. Between Kenya and Burundi these agroecologies are well represented. Frequent association of the active ingredients of Aflasafe KE01 with maize and groundnut produced in Burundi, in addition to Uganda (G. Mahuku, personal communication) indicates that the Aflasafe KE01 active ingredients may be broadly effective across East Africa. On-going field testing of a country specific Aflasafe product for use in Burundi, Aflasafe BU01 (Figure 6A), and Aflasafe KE01 hold promise to manage aflatoxin contamination in Burundi. Both Burundi and Kenya belong to the EAC, which also includes Uganda, Rwanda, Tanzania, and DR Congo. EAC Partner States are promoting the use of biocontrol through ongoing harmonization of regional regulatory frameworks for biocontrol agents (Ortega-Beltran and Bandyopadhyay, 2021).1
Only a small proportion of samples (groundnut = 6%, maize = 3%) was contaminated with aflatoxins above the EAC threshold of 10 μg/kg. However, maize and groundnut were associated with highly toxigenic fungi, representing a risk of contamination during the post-harvest stages, which may last for more than 1 year. Further studies to assess contamination further up the value chain are necessary. The population of Aspergillus section Flavi in both maize and groundnut was composed of a significant proportion of aflatoxigenic strains. Fungi with S-morphology were the most toxigenic while A. flavus L-morphotype isolates were mostly atoxigenic. While the observed and potential contamination can be attributed to the fungi with S-morphology, the high proportion of A. flavus L-morphotype provides hope for developing biocontrol products for use in Burundi. Indeed, among the L-morphotype isolates of A. flavus, 55% were atoxigenic within which a few genetic groups were widely distributed in Burundi. The active ingredients of the biocontrol product registered for use in Kenya (Aflasafe KE01) were also found in Burundi and could be evaluated in Burundi. In addition, another biocontrol product, Aflasafe BU01, was formulated with four different widely distributed genetic groups specific to Burundi. However, biocontrol must be supported by other interventions including awareness creation, timely harvesting, rapid grain drying, appropriate storage structures, sorting, and processing and insect control at pre-and post-harvest stages.
The original contributions presented in the study are included in the article/Supplementary material, further inquiries can be directed to the corresponding author.
RB, PC, and CM designed the overall projects from which data are derived. GN, CM, JW, PN, JA, EN, PC, and RB contributed to the conception and design of the experiments. GN, PN, MN, and EN collected the grain samples. GN, CM, JW, AM, NN, JA, and AO-B conducted and analyzed the microbiology and aflatoxin studies. KC and PC collected and analyzed the molecular data. AO-B, KC, and RB performed distribution mapping of the genetic groups. CM, PC, AO-B, and RB provided guidance. GN, CM, and AO-B drafted the original manuscript followed by editing by other authors. RB and PC secured funds for the study. All authors contributed to the article and approved the submitted version.
The study was supported by the United States Agency for International Development (USAID), through the Aflatoxin Policy and Program for the East Africa Region (APPEAR) project that was implemented by the International Institute of Tropical Agriculture (IITA), Institut des Science Agronomiques du Burundi (ISABU), Kenya Agricultural and Livestock Research Organization (KALRO) and the East African Community. We acknowledge partial support from the Bill & Melinda Gate Foundation (OPP1133356). Support was also provided by USDA Agricultural Research Project 5347-42000-02-00D. We also gratefully acknowledge additional funding support from the CGIAR Research Program on Agriculture for Nutrition and Health (A4NH), and the CGIAR Plant Health Initiative by CGIAR Trust Fund contributors (https://www.cgiar.org/research/).
The authors are grateful to all farmers that provided the samples used in the current study. Technical assistance of staff of the Eastern Africa Regional Mycotoxin Research & Training Lab at Katumani and Pathology & Mycotoxin lab of IITA at Ibadan is appreciated.
The authors declare that the research was conducted in the absence of any commercial or financial relationships that could be construed as a potential conflict of interest.
All claims expressed in this article are solely those of the authors and do not necessarily represent those of their affiliated organizations, or those of the publisher, the editors and the reviewers. Any product that may be evaluated in this article, or claim that may be made by its manufacturer, is not guaranteed or endorsed by the publisher.
The use of trade, firm, or corporation names in these methods is for the information and convenience of the reader. Such use does not constitute an official endorsement or approval by the USDA Agricultural Research Service, of any product or service to the exclusion of others that may be suitable. In addition, USDA-ARS makes no warranties as to the merchantability or fitness of the methodologies described on these pages for any particular purpose, or any other warranties expressed or implied. These methodologies provide a guide and do not replace published work. USDA-ARS is not liable for any damages resulting from the use or misuse of these methodologies.
The Supplementary material for this article can be found online at: https://www.frontiersin.org/articles/10.3389/fmicb.2023.1106543/full#supplementary-material
Adhikari, B. N., Bandyopadhyay, R., and Cotty, P. J. (2016). Degeneration of aflatoxin gene clusters in Aspergillus flavus from Africa and North America. AMB Express 6:62. doi: 10.1186/s13568-016-0228-6
Agbetiameh, D., Ortega-Beltran, A., Awuah, R. T., Atehnkeng, J., Cotty, P. J., and Bandyopadhyay, R. (2018). Prevalence of aflatoxin contamination in maize and groundnut in Ghana: population structure, distribution, and toxigenicity of the causal agents. Plant Dis. 102, 764–772. doi: 10.1094/PDIS-05-17-0749-RE
Agbetiameh, D., Ortega-Beltran, A., Awuah, R. T., Atehnkeng, J., Islam, M. -S., Callicott, K. A., et al. (2019). Potential of atoxigenic Aspergillus flavus vegetative compatibility groups associated with maize and groundnut in Ghana as biocontrol agents for aflatoxin management. Front. Microbiol. 10:2069. doi: 10.3389/fmicb.2019.02069
Amaike, S., and Keller, N. P. (2011). Aspergillus flavus. Annu. Rev. Phytopathol. 49, 107–133. doi: 10.1146/annurev-phyto-072910-095221
Atehnkeng, J., Ojiambo, P. S., Ortega-Beltran, A., Augusto, J., Cotty, P. J., and Bandyopadhyay, R. (2022). Impact of frequency of application on the long-term efficacy of the biocontrol product Aflasafe in reducing aflatoxin contamination in maize. Front. Microbiol. 13:1049013. doi: 10.3389/fmicb.2022.1049013
Atehnkeng, J., Ojiambo, P. S., Donner, M., Ikotun, T., Sikora, R. A., Cotty, P. J., et al. (2008). Distribution and toxigenicity of Aspergillus species isolated from maize kernels from three agro-ecological zones in Nigeria. Int. J. Food Microbiol. 122, 74–84. doi: 10.1016/j.ijfoodmicro.2007.11.062
Bandyopadhyay, R., Ortega-Beltran, A., Konlambigue, M., Kaptoge, L., Falade, T. D. O., and Cotty, P. J. (2022). “Development and scale-up of bioprotectants to keep staple foods safe from aflatoxin contamination in Africa” in Microbial Bioprotectants for Plant Disease Management. eds. J. Köhl and W. J. Ravensberg (Cambridge, UK: Burleigh Dodds Science Publishing), 1–41. doi: 10.19103/AS.2021.0093.16
Callicott, K. A., and Cotty, P. J. (2015). Method for monitoring deletions in the aflatoxin biosynthesis gene cluster of Aspergillus flavus with multiplex PCR. Lett. Appl. Microbiol. 60, 60–65. doi: 10.1111/lam.12337
Collins, C., Magnani, R., and Ngomirakiza, E. (2013). USAID Office of Food for Peace-Food Security Country Framework. Washington D.C., U.S.A: FHI 360/FANTA.
Cotty, P. J. (1989). Virulence and cultural characteristics of two Aspergillus flavus strains pathogenic on cotton. Phytopathology 79, 808–814. doi: 10.1094/Phyto-79-808
Cotty, P. J., and Cardwell, K. F. (1999). Divergence of west African and north American communities of Aspergillus section Flavi. Appl. Environ. Microbiol. 65, 2264–2266. doi: 10.1128/aem.65.5.2264-2266.1999
Daily, Nation. (2020). Maize flour with high aflatoxin levels destroyed in Nakuru. Available at: https://www.nation.co.ke/kenya/counties/nakuru/maize-flour-with-high-aflatoxin-levels-destroyed-in-nakuru-248298. Date accessed: 2020-06-16
DFID. (2009). Economic Impacts of Climate Change: Kenya, Rwanda and Burundi. ICPAC, Kenya and SEI Oxford Office.
Excoffier, L., and Lishcer, H. E. L. (2010). Arlequin suite ver 3.5: a new series of programs to perform population genetic analyzes under Linux and windows. Mol. Ecol. Resour. 10, 564–567. doi: 10.1111/j.1755-0998.2010.02847.x
Ezekiel, C. N., Atehnkeng, J., Odebode, A. C., and Bandyopadhyay, R. (2014). Distribution of aflatoxigenic Aspergillus section Flavi in commercial poultry feed in Nigeria. Int. J. Food Microbiol. 189, 18–25. doi: 10.1016/j.ijfoodmicro.2014.07.026
Food and Agriculture Organization of the United Nations. (2022) FAOSTAT Statistical Database. Rome: FAO. (Accessed October 23, 2022)
Frisvad, J. C., Hubka, V., Ezekiel, C. N., Hong, S. B., Nováková, A., Chen, A. J., et al. (2019). Taxonomy of Aspergillus section Flavi and their production of aflatoxins, ochratoxins and other mycotoxins. Stud. Mycol. 93, 1–63. doi: 10.1016/j.simyco.2018.06.001
Grubisha, L. C., and Cotty, P. J. (2009). Twenty-four microsatellite markers for the aflatoxin-producing fungus Aspergillus flavus. Mol. Ecol. Resour. 9, 264–267. doi: 10.1111/j.1755-0998.2008.02378.x
Hell, K., Fandohan, P., Bandyopadhyay, R., Kiewnick, S., Sikora, R., and Cotty, P. J. (2008). “Pre-and postharvest management of aflatoxin in maize: an African perspective” in Mycotoxins: Detection Methods, Management, Public Health and agricultural trade. eds. J. F. Leslie, R. Bandyopadhyay, and A. Visconti (Wallingford UK: CABI), 219–229.
Horn, B. W., and Dorner, J. W. (1998). Soil populations of Aspergillus species from section Flavi along a transect through peanut-growing regions of the United States. Mycologia 90, 767–776. doi: 10.1080/00275514.1998.12026969
Huson, D. H., and Bryant, D. (2006). Application of phylogenetic networks in evolutionary studies. Mol. Biol. Evol. 23, 254–267. doi: 10.1093/molbev/msj030
Islam, M.-S., Callicott, K. A., Mutegi, C., Bandyopadhyay, R., and Cotty, P. J. (2018). Aspergillus flavus resident in Kenya: high genetic diversity in an ancient population primarily shaped by clonal reproduction and mutation-driven evolution. Fungal Ecol. 35, 20–33. doi: 10.1016/j.funeco.2018.05.012
Islam, M.-S., Callicott, K. A., Mutegi, C., Bandyopadhyay, R., and Cotty, P. J. (2021). Distribution of active ingredients of a commercial aflatoxin biocontrol product in naturally occurring fungal communities across Kenya. Microb. Biotechnol. 14, 1331–1342. doi: 10.1111/1751-7915.13708
Jaime-Garcia, R., and Cotty, P. J. (2006). Spatial distribution of Aspergillus flavus and its toxigenic strains on commercial cottonseed from South Texas and its relationship to aflatoxin contamination. Plant Pathol. 55, 358–366. doi: 10.1111/j.1365-3059.2006.01358.x
Kachapulula, P. W., Akello, J., Bandyopadhyay, R., and Cotty, P. J. (2017). Aspergillus section Flavi community structure in Zambia influences aflatoxin contamination of maize and groundnut. Int. J. Food Microbiol. 261, 49–56. doi: 10.1016/j.ijfoodmicro.2017.08.014
Keya, S., and Rubaihayo, P. (2013). Progress in on-farm production and productivity in the east African community: 50 years after independence. Kilimo Trust Technical Paper 8, 20–22.
Klich, M. A. (2007). Environmental and developmental factors influencing aflatoxin production by Aspergillus flavus and Aspergillus parasiticus. Mycoscience 48, 71–80. doi: 10.1007/s10267-006-0336-2
Ludgate, N., and Tata, S. J. (2015). Integrating gender and nutrition within agricultural extension services: Burundi landscape analysis. Feed the future: the US government’s global hunger and food security initiative Available at: www.ingenaes.illinois.edu
Mahuku, G., Nzioki, H. S., Mutegi, C., Kanampiu, F., Narrod, C., and Makumbi, D. (2019). Pre-harvest management is a critical practice for minimizing aflatoxin contamination of maize. Food Control 96, 219–226. doi: 10.1016/j.foodcont.2018.08.032
Mauro, A., Battilani, P., and Cotty, P. J. (2015). Atoxigenic Aspergillus flavus endemic to Italy for biocontrol of aflatoxins in maize. BioControl 60, 125–134. doi: 10.1007/s10526-014-9624-5
Meirmans, P. G., and Van Tienderen, P. H. (2004). GENOTYPE and GENODIVE: two programs for the analysis of genetic diversity of asexual organisms. Mol. Ecol. Notes 4, 792–794. doi: 10.1111/j.1471-8286.2004.00770.x
Monson, M., Coulombe, R., and Reed, K. (2015). Aflatoxicosis: lessons from toxicity and responses to aflatoxin B1 in poultry. Agriculture 5, 742–777. doi: 10.3390/agriculture5030742
Moore, G. G. (2022). Practical considerations will ensure the continued success of pre-harvest biocontrol using non-aflatoxigenic Aspergillus flavus strains. Crit. Rev. Food Sci. Nutr. 62, 4208–4225. doi: 10.1080/10408398.2021.1873731
Moral, J., Garcia-Lopez, M. T., Camiletti, B. X., Jaime, R., Michailides, T. J., Bandyopadhyay, R., et al. (2020). Present status and perspective on future use of aflatoxin biocontrol products. Agronomy 10:491. doi: 10.3390/agronomy10040491
Munimbazi, C., and Bullerman, L. B. (1996). Molds and mycotoxins in foods from Burundi. J. Food Prot. 59, 869–875. doi: 10.4315/0362-028X-59.8.869
Mutegi, C. K., Ngugi, H. K., Hendriks, S. L., and Jones, R. B. (2012). Factors associated with the incidence of Aspergillus section Flavi and aflatoxin contamination of peanuts in the Busia and Homa bay districts of western Kenya. Plant Pathol. 61, 1143–1153. doi: 10.1111/j.1365-3059.2012.02597.x
Niyongabo, I. (2008). Best practices for water harvesting and irrigation. Efficient water use for agricultural production project. Nile Basin Initiative, 13–14. Available at: https://nilebasin.org/nileis/system/files/burundi_best_practices_print.pdf
Ojara, M. A., Yunsheng, L., Ongoma, V., Mumo, L., Akodi, D., Ayugi, B., et al. (2021). Projected changes in east African climate and its impacts on climatic suitability of maize production areas by the mid-twenty-first century. Environ. Monit. Assess. 193, 1–24. doi: 10.1007/s10661-021-09547-4
Okoth, S., Nyongesa, B., Ayugi, V., Kan’gethe, E., Korhonen, H., and Joutsjoki, V. (2012). Toxigenic potential of Aspergillus species occurring on maize kernels from two agro-ecological zones in Kenya. Toxins 4, 991–1007. doi: 10.3390/toxins4110991
Ortega-Beltran, A., and Bandyopadhyay, R. (2021). Contributions of integrated aflatoxin management strategies to achieve the sustainable development goals in various African countries. Glob. Food Sec. 30:100559. doi: 10.1016/j.gfs.2021.100559
PACA. (2012). Aflatoxin impacts and potential solutions in agriculture, trade, and health: an introduction to aflatoxin impacts in Africa Available at: https://archives.au.int/handle/123456789/4985 (Accessed November 19, 2022).
Pildain, M. B., Frisvad, J. C., Vaamonde, G., Cabral, D., Varga, J., and Samson, R. A. (2008). Two novel aflatoxin-producing Aspergillus species from Argentinean peanuts. Int. J. Syst. Evol. Microbiol. 58, 725–735. doi: 10.1099/ijs.0.65123-0
Probst, C., Bandyopadhyay, R., and Cotty, P. J. (2014). Diversity of aflatoxin-producing fungi and their impact on food safety in sub-Saharan Africa. Int. J. Food Microbiol. 174, 113–122. doi: 10.1016/j.ijfoodmicro.2013.12.010
Probst, C., Bandyopadhyay, R., Price, L. E., and Cotty, P. J. (2011). Identification of atoxigenic Aspergillus flavus isolates to reduce aflatoxin contamination of maize in Kenya. Plant Dis. 95, 212–218. doi: 10.1094/pdis-06-10-0438
Probst, C., Callicott, K. A., and Cotty, P. J. (2012). Deadly strains of Kenyan Aspergillus are distinct from other aflatoxin producers. Eur. J. Plant Pathol. 132, 419–429. doi: 10.1007/s10658-011-9887-y
Probst, C., and Cotty, P. J. (2012). Relationships between in vivo and in vitro aflatoxin production: reliable prediction of fungal ability to contaminate maize with aflatoxins. Fungal Biol. 116, 503–510. doi: 10.1016/j.funbio.2012.02.001
Probst, C., Njapau, H., and Cotty, P. J. (2007). Outbreak of an acute aflatoxicosis in Kenya in 2004: identification of the causal agent. Appl. Environ. Microbiol. 73, 2762–2764. doi: 10.1128/AEM.02370-06
Probst, C., Schulthess, F., and Cotty, P. J. (2010). Impact of Aspergillus section Flavi community structure on the development of lethal levels of aflatoxins in Kenyan maize (Zea mays). J. Appl. Microbiol. 108, 600–610. doi: 10.1111/j.1365-2672.2009.04458.x
Ramesh, V., Bhat, R. V., and Vasanthi, S. (2003). “Mycotoxin food safety risk in developing countries” in Food Safety in Food Security and Food Trade. ed L. Unnevehr (Washington D.C., U.S.A.: IFPRI). Brief 3. https://ebrary.ifpri.org/digital/collection/p15738coll2/id/92787/rec/2 (Accessed November 19, 2022).
Seetha, A., Munthali, W., Msere, H. W., Swai, E., Muzanila, Y., Sichone, E., et al. (2017). Occurrence of aflatoxins and its management in diverse cropping systems of Central Tanzania. Mycotoxin Res. 33, 323–331. doi: 10.1007/s12550-017-0286-x
Senghor, L. A., Ortega-Beltran, A., Atehnkeng, J., Callicott, K. A., Cotty, P. J., and Bandyopadhyay, R. (2020). The atoxigenic biocontrol product Aflasafe SN01 is a valuable tool to mitigate aflatoxin contamination of both maize and groundnut cultivated in Senegal. Plant Dis. 104, 510–520. doi: 10.1094/PDIS-03-19-0575-RE
Singh, P., Mehl, H. L., Orbach, M. J., Callicott, K. A., and Cotty, P. J. (2020). Phenotypic differentiation of two morphologically similar aflatoxin-producing fungi from West Africa. Toxins 12:656. doi: 10.3390/toxins12100656
The Standard. (2019). State to destroy 9.4 tonnes of contaminated maize in Mombasa. Newspaper Article Available at: https://www.standardmedia.co.ke/article/2001309356/state-to-destroy-9-4-tonnes-of-contaminated-maize-in-mombasa (Accessed June 16, 2020).
Udomkun, P., Mutegi, C., Wossen, T., Atehnkeng, J., Nabahungu, N. L., Njukwe, E., et al. (2018). Occurrence of aflatoxin in agricultural produce from local markets in Burundi and eastern Democratic Republic of Congo. Food Sci. Nutr. 6, 2227–2238. doi: 10.1002/fsn3.787
Keywords: atoxigenic, biocontrol, toxigenic, Aspergillus section Flavi, diversity
Citation: Nsabiyumva G, Mutegi CK, Wagacha JM, Mohamed AB, Njeru NK, Ndayihanzamaso P, Niyuhire MC, Atehnkeng J, Njukwe E, Callicott KA, Cotty PJ, Ortega-Beltran A and Bandyopadhyay R (2023) Aflatoxin contamination of maize and groundnut in Burundi: Distribution of contamination, identification of causal agents and potential biocontrol genotypes of Aspergillus flavus. Front. Microbiol. 14:1106543. doi: 10.3389/fmicb.2023.1106543
Received: 23 November 2022; Accepted: 21 February 2023;
Published: 13 March 2023.
Edited by:
István Pócsi, University of Debrecen, HungaryReviewed by:
Giancarlo Perrone, National Research Council (CNR), ItalyCopyright © 2023 Nsabiyumva, Mutegi, Wagacha, Mohamed, Njeru, Ndayihanzamaso, Niyuhire, Atehnkeng, Njukwe, Callicott, Cotty, Ortega-Beltran and Bandyopadhyay. This is an open-access article distributed under the terms of the Creative Commons Attribution License (CC BY). The use, distribution or reproduction in other forums is permitted, provided the original author(s) and the copyright owner(s) are credited and that the original publication in this journal is cited, in accordance with accepted academic practice. No use, distribution or reproduction is permitted which does not comply with these terms.
*Correspondence: Ranajit Bandyopadhyay, ci5iYW5keW9wYWRoeWF5QGNnaWFyLm9yZw==
†Present addresses: Charity K. Mutegi, Rootooba, Nairobi, Kenya
Emmanuel Njukwe, CORAF, Dakar, Senegal
Disclaimer: All claims expressed in this article are solely those of the authors and do not necessarily represent those of their affiliated organizations, or those of the publisher, the editors and the reviewers. Any product that may be evaluated in this article or claim that may be made by its manufacturer is not guaranteed or endorsed by the publisher.
Research integrity at Frontiers
Learn more about the work of our research integrity team to safeguard the quality of each article we publish.