- 1School of Hydraulic and Environmental Engineering, Changsha University of Science & Technology, Changsha, China
- 2Key Laboratory of Dongting Lake Aquatic Eco-Environmental Control and Restoration of Hunan Province, Changsha University of Science and Technology, Changsha, China
- 3Technology Center, Hunan Pilot Yanghu Reclaimed Water Co., Ltd., Changsha, China
- 4Technology Department, Hunan Rongantai Ecological Technology Co., Ltd., Changsha, China
- 5Technology and Information Department, CCCC-TDC Environmental Engineering Co., Ltd., Tianjin, China
- 6Engineering Department, China Railway Wuju Group the First Engineering Co., Ltd., Changsha, China
Constructed wetlands (CWs) are artificial systems that use natural processes to treat wastewater containing organic pollutants. This approach has been widely applied in both developing and developed countries worldwide, providing a cost-effective method for industrial wastewater treatment and the improvement of environmental water quality. However, due to the large organic carbon inputs, CWs is produced in varying amounts of CH4 and have the potential to become an important contributor to global climate change. Subsequently, research on the mitigation of CH4 emissions by CWs is key to achieving sustainable, low-carbon dependency wastewater treatment systems. This review evaluates the current research on CH4 emissions from CWs through bibliometric analysis, summarizing the reported mechanisms of CH4 generation, transfer and oxidation in CWs. Furthermore, the important environmental factors driving CH4 generation in CW systems are summarized, including: temperature, water table position, oxidation reduction potential, and the effects of CW characteristics such as wetland type, plant species composition, substrate type, CW-coupled microbial fuel cell, oxygen supply, available carbon source, and salinity. This review provides guidance and novel perspectives for sustainable and effective CW management, as well as for future studies on CH4 reduction in CWs.
Introduction
With rapid economic and industrial development, global climate change has become an increasingly critical concern, driven by the excessive emission of greenhouse gases (GHGs) such as carbon dioxide (CO2), methane (CH4) and nitrous oxide (Dreyfus et al., 2022). GHG emissions are continually increasing worldwide, resulting in a large amount of research which focused on methods to control GHG emissions, and the development of low-carbon systems (Nuamah et al., 2020).
Wastewater treatment is one of the most resource-intensive industrial practices. Constructed wetlands (CWs) are a well-established low-cost, energy-saving, multifunctional approach to sustainable wastewater treatment, that have been widely used for the treatment of various polluted water bodies (Yu et al., 2020, 2021). However, due to the large scale of wastewater discharged, the widespread use of CWs would have an obvious environmental consequence in terms of GHG emissions. Studies have shown that the amount of GHGs emitted from CWs is 2-to 10-fold higher than from natural wetlands (Maltais-Landry et al., 2009). Current atmospheric CH4 concentrations are more than 2.5-fold higher than pre-industrial levels (Zhang Q. et al., 2020), which is concerning as the global warming potential (GWP) of CH4 in the carbon cycle is 34-fold stronger than that of CO2. Therefore, despite CH4 being present at much lower atmospheric concentrations than CO2, its growth rate is considerably larger, making it one of the most important GHGs contributing to global warming (Guerrero-Cruz et al., 2021). Average CH4 emissions are extremely variable by reviewing 158 studies data, ranging from 0.15 to 5,220 mg/m2/h, which can disrupt earth radiation levels (Mander et al., 2014) It has been reported that a 1-fold increase in atmospheric CH4 concentration would lead to tropospheric surface warming by 0.2–0.3 degrees, presenting a serious risk to human and environmental health (Dreyfus et al., 2022). CH4 emissions originate from both anthropogenic and natural sources, with wetland ecosystems being the largest natural source, generating annual global CH4 emissions of 177–284 Tg (Zhang et al., 2021b), 82% of which originate from CWs worldwide (Nuamah et al., 2020). CH4 has become an important aspect of global carbon reduction, due to its potentially considerable role in future warming. According to the latest IPCC report (Dreyfus et al., 2022), in order to achieve the global temperature rise control target of 1.5°C, CH4 emissions should be reduced by one-third by 2030 and nearly half by 2050. Achieving this goal is a necessary requirement for sustainable social and economic development (Zhang Q. et al., 2020). and effectively controlling CH4 emissions from CWs is an essential aspect of reducing global CH4 emissions.
The CH4 emission by CWs is the terminal product of various processes in the production, transport, and oxidation of organic matter under anaerobic conditions (Shao et al., 2020). Methanogens and methanotrophs are important microorganisms that mediate functional communities in CWs, and are closely connected with the metabolism of CH4 and carbon cycle processes (Bridgham et al., 2013). Disruption to the CH4 source-sink balance of CWs is a direct driver of dramatic increases in atmospheric CH4. In CW ecosystems, plants use assimilation to fix inorganic carbon from both the air and the water column (converting it into organic carbon), while also fixing organic carbon in the water column through substrate sequestration and uptake, resulting in wetlands serving as a carbon sink (Liu et al., 2022). When wetlands are subjected to long-term anaerobic conditions, plant debris, litter and the substrate gradually convert macromolecular organic matter into CH4 and CO2, which is released into the atmosphere through anaerobic microbial metabolic activities, resulting in wetlands also serving as a carbon source (Yan et al., 2012). CO2 released from CWs can be captured from the atmosphere through photosynthesis, with the CO2 fixed within plant biomass no longer contributing to the long-term carbon sink, and CO2 emissions from CWs are not considered as GHG because they are the natural fate of organic matter (Guo et al., 2020). The abundance, composition and activity of methanogens and methanotrophs are important determinants of CH4 emissions from CWs (Ji et al., 2021). In view of this, the growth of methanogens should be inhibited in CWs, ensuring a suitable environment is provided for the survival of methanotrophs, maintaining maximum conversion of the generated CH4 to CO2 and subsequently, reducing the contribution of CWs to the GWP. To stimulate the growth of methanotrophs, plant cover serving as a powerful carbon sink is used in horizontal subsurface flow CWs (HSSFCWs) which allow O2 transported by the aerenchyma of plant roots (Mander et al., 2008). It is confirmed that plant diversity can also increase carbon sequestration in the substrate and substrate-based carbon sequestration not only completely offsets GWP based on CH4 and nitrous oxide emissions, but also simulates the conversion of CWs from a carbon source to a carbon sink (Du et al., 2018). Carbon uptake in vegetated wetlands decreases with increasing levels of salinity, mainly due to the inhibition of plant productivity (Sheng et al., 2015). Microbial transport and transformation are the main reason for the high carbon source consumption of CW-coupled microbial fuel cell (MFC) systems, with these processes regulated by microbial competition driven by environmental aspects, providing a novel approach to controlling CH4 emissions from wetland systems (Liu et al., 2022). The contributions of carbon “source” and “sink” functions in CWs, as well as their relationship and interactions, are crucial to the material and energy cycles within CW ecosystems, global carbon dynamics and global climate change trends (Dreyfus et al., 2022).
If CWs are designed only to consider pollutant removal, the process may not fit with the current double-carbon philosophy, highlighting the importance of constructing effective wetland systems, that can achieve high pollutant removal performance while minimizing CH4 emissions. There remains a lack of research on CH4 emissions from CWs, making it difficult to accurately determine the mechanisms and processes of CH4 emission from CWs. Furthermore, the important environmental control factors have not been comprehensively established, reducing the validity of CH4 emission reduction measures, and limiting the effectiveness of CWs. Therefore, this review was conducted to explore the available research in this field.
Bibliometric analysis
Data collection sources
Data was collected using the Web of Science (WOS) Core Collection database, with this review only considering the Science Citation Index Expanded (SCI-Expanded). Based on the findings of previous research by Yu et al. (2022), the keywords used to screen the available research on CH4 in CWs included (“constructed wetland*” OR “treatment wetland*” OR “engineered wetland*” OR “artificial wetland*” OR “reed bed*” OR “man-made wetland*”) and (“methane sequestration*” OR “methane capture*” OR “methane emission*” OR “CH4 capture*” OR “CH4 sequestration*” OR “CH4 emission*” OR “methane reduc*” OR “CH4 reduc*”). The literature type was restricted to “article” and “review,” with publications in English from 1991 to December 2021 included (1991 was the earliest publication date available in the database). After the initial data search, 108 articles investigating CH4 in CWs were selected. The analysis of these publications were performed using Microsoft Excel (2019), charts were generated using Origin (2019 learning version) and the S-curve was prepared using the logistic model in Loglet Lab 4. Analysis of keyword co-occurrence were performed using VOS viewer software (version 1.6.15). After exporting the 108 publications from the WOS platform in plain text form, the author keyword co-occurrence function of VOS viewer software was used to analyze keyword co-occurrence, with the thesaurus files then were merged (such as CH4, methanes replaced with methane) and the minimum number of co-occurrences set to 3.
Analysis
Publication trend
The publication trend for studies on CH4 in CWs is shown in Figure 1. In the long term, the number of publications has continually increased with fluctuations, reflecting the growing concern within the scientific community about the effect of CH4 emissions and the need to actively reduce CH4 emissions in order to maintain global ecological security (Kasak et al., 2020). Prior to 2004, the publication number was relatively low and did not increase significantly from 2005 to 2013. However, a notable increase was observed after 2014, finally reaching 108 articles in 2021. Although the number of publications on this topic remains relatively low, work in this field is gaining importance constantly, as shown by the S-curve (R2 = 0.984) in Figure 1, which indicates that research is in the growth stage and is expected to reach the inflection point in 2026 and remain stable until 2040. Therefore, publications in this field are not expected to reach saturation over the next 15 years, highlighting the high potential for innovation and development. In this sense, a comprehensive review of the current state of knowledge is essential to help stimulate and guide future development and research on CH4 in CWs.
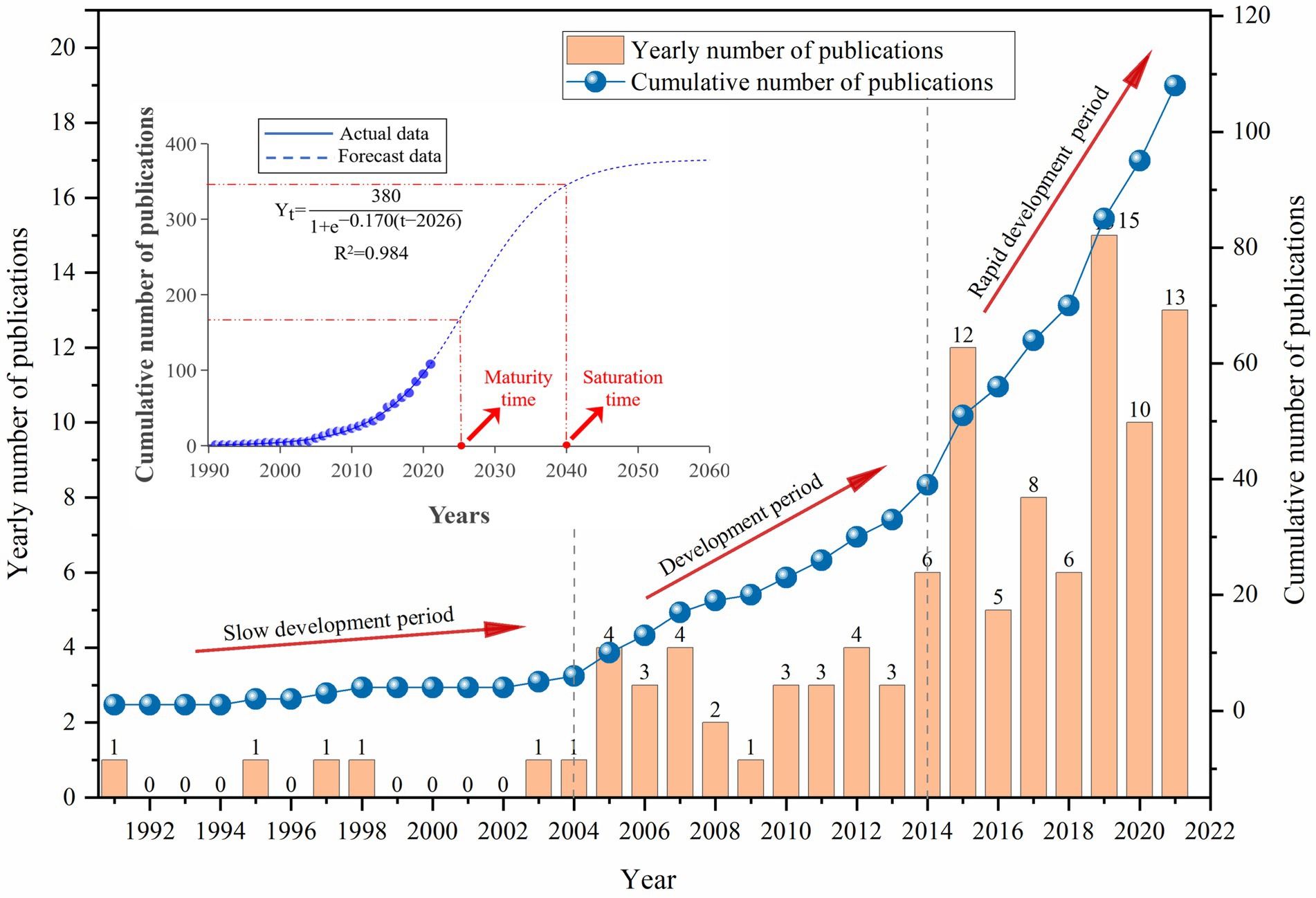
Figure 1. Temporal evolution of publications about CH4 in CWs, showing the total and cumulative trend, with the corresponding S-curve.
Main topic
The results of keyword co-occurrence analysis are shown in Figure 2. The 6 most frequently co-occurring keywords were intercepted, showing that research on the effects of CH4 on GWP, plants, and microorganisms was closely linked to studies on CH4 emissions. Studies by Chen et al. (2020c) and Maucieri et al. (2019) attracted attention as they found that different plant species were able to exert varying effects on CH4 emissions. Furthermore, it has been shown that microbial diversity and abundance are a critical factor affecting CH4 fluxes, resulting in the need for further research and validation (Zhang et al., 2021a). MFC technology is a promising approach to the control of CH4 emissions from CWs. For example, Liu et al. (2022) reported that microbial competition in a CW-MFC system can convert unstable carbon sources to CO2 rather than CH4, which can considerably reduce the contribution of CWs to global CH4 emissions.
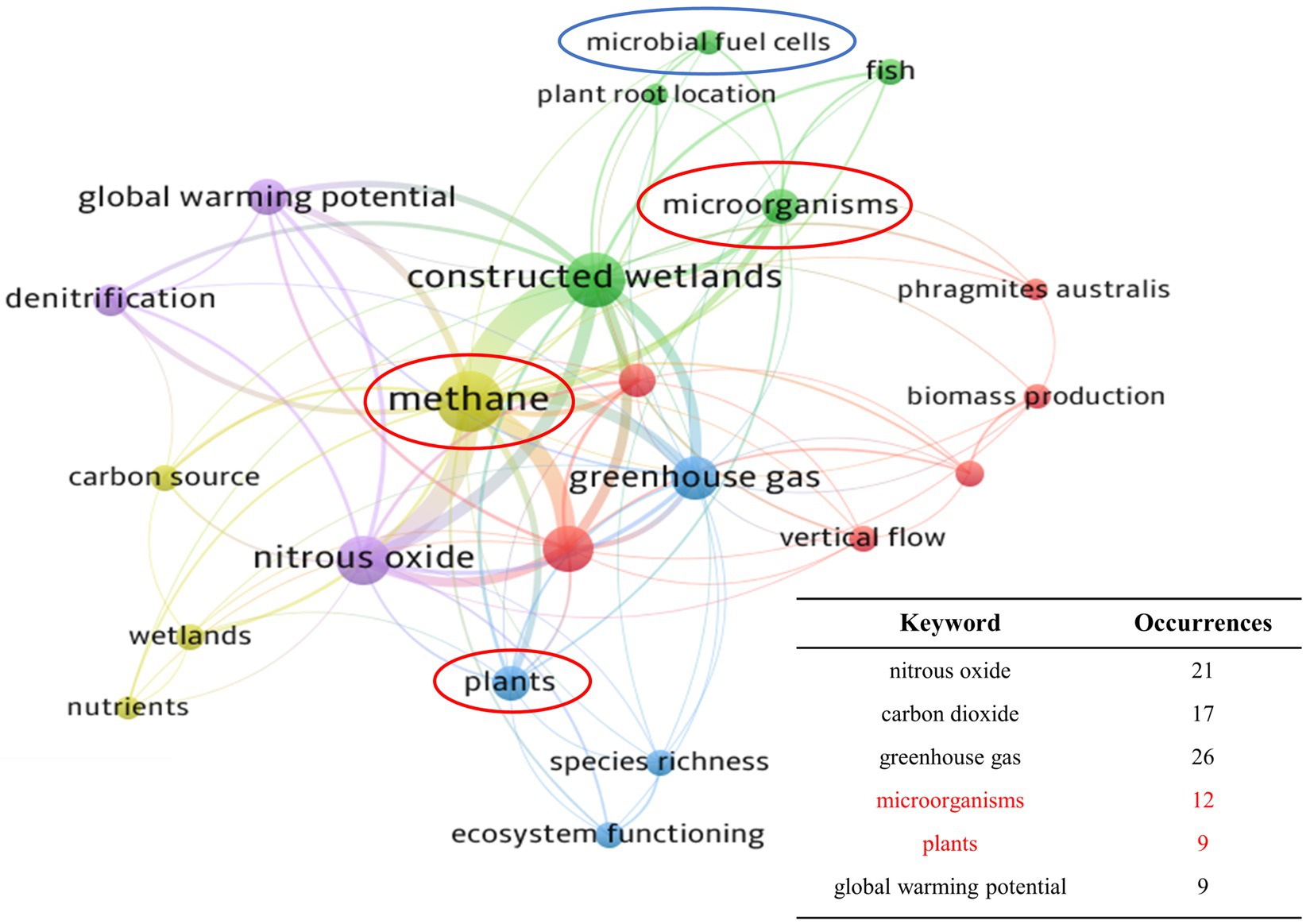
Figure 2. The bibliometric co-occurrence of keywords associated with CH4 in CWs. (Stronger degrees of connection between the keywords are indicated by closer locations and thicker lines, while a higher frequency of occurrence is shown by larger circles).
Production, transport, and oxidation of CH4 in CWs
Production of CH4 in CWs
Plants
Plants play a vital role in CH4 emissions, however due to contradictory results having been reported, the production of CH4 by plants was previously only considered in terms of a channel for soil-atmospheric gas exchange. However, some terrestrial plants were first demonstrated to release CH4 under aerobic conditions by Keppler et al. (2006), with more recent studies proving evidence that lignin, pectin, and cellulose can all serve as precursors for CH4 formation (Bruhn et al., 2012). The production of CH4 by plants has been reported as a defense strategy against environmental stress factors, such as cutting damage, increased temperatures, UV radiation, and the disturbance of cytochrome c oxidase activity (Zhang et al., 2012). Once plants trigger stress responses, ROS, such as O2˙− and H2O2, can be overproduced within plants, further exacerbating the degradation of cellular material, leading to the production of CH4 (Bruhn et al., 2012; Wang et al., 2023). In addition, some of the identified CH4 producing terrestrial plants are used in CW applications, such as Phragmites australis and Thalia dealbata. However, CH4 emissions from wetland hydrophyte plants under aerobic conditions have not been investigated to date, highlighting a key gap in knowledge that requires future research.
Microorganisms
Anaerobic microorganisms play the major role in CH4 production. Under anaerobic conditions, anaerobic hydrolytic microbes, fermentative microbes, and hydrogen-producing acetogens can decompose organic matter from wastewater, substrate materials, and plant biomass (Liu et al., 2022), forming simple inorganic (e.g., CO2 and H2) and organic compounds (e.g., acetate; López et al., 2019), that are subsequently converted to CH4 by methanogens (specialized anaerobic archaea; Malyan et al., 2016). Anaerobic environments occur widely in the substrate layer of CWs. For example, HSSFCWs are designed to purify wastewater through anaerobic pathways (Engida et al., 2020), while anaerobic microzones have been identified in surface flow CWs (SFCWs) due to water flow layering over substrate, and vertical subsurface flow CWs (VSSFCWs) due to long-term operation causing microbial oxygen (O2) consumption rates to exceed the reoxygenation rate (Maucieri et al., 2017). Methanogens are divided into seven orders, belonging to Euryarchaeota (Table 1). Most methanogens are hydrogenotrophic, with only Methanosarcinales being acetoclastic (Table 1; Bridgham et al., 2013; Zhang et al., 2021c). Methanosarcina are fast-growing organisms that can utilize high acetate concentrations, in contrast to Methanosaeta (Lu Y. et al., 2015). Newly discovered methanogens have been classified as belonging to Euryarchaeota (such as Methanomassiliicoccales, Methanofastidiosa, and Methanonatronarchaeia; Dridi et al., 2012; Nobu et al., 2016; Sorokin et al., 2017), as well as non-Euryarchaeota (such as Verstraetearchaeota, Bathyarchaeota, and Geoarchaeota (Evans et al., 2015; Vanwonterghem et al., 2016; Guo et al., 2020). Based on the results of this review, three methanogenic metabolic pathways exist in CWs: H2/CO2 reduction, methyl cracking, and acetate fermentation. The hydrogenotrophic pathway is more energetically favorable to methanogenesis than the acetoclastic pathway (Zhang et al., 2018a), although the acetoclastic pathway has been reported to dominate in freshwater wetland ecosystems, accounting for more than 67% of CH4 emissions (Zhang et al., 2018b). Novel methanogens have also been found to utilize a fourth methanogenic pathway, involving the reduction of methyl compounds by H2, as originally observed in Methanobacteriales (Dridi et al., 2012) and Methanomicrobiales (Sprenger et al., 2007), then later found in Methanomassiliicoccales (Dridi et al., 2012), Methanofastidiosa (Nobu et al., 2016), Bathyarchaeota (Evans et al., 2015), and Verstraetearchaeota (Vanwonterghem et al., 2016). The specific equations are shown in Eqs 1–7.
Transport of CH4 in CWs
The transport of CH4 occurs mainly via three processes: (1) direct CH4 transport through molecular diffusion from the water and substrate column; (2) direct CH4 transport through the ebullition flux process from substrate column; and (3) plant-mediated CH4 transport from the substrate column via plant aerenchym (Figure 3; Thangarajan et al., 2013; Kasak et al., 2020). Ebullition plays a major role in direct CH4 transport process, producing three-fold more CH4 fluxes than molecular diffusion by Bonetti et al. (2021), while plant-mediated CH4 transport is the dominant mode of CH4 release among three processes (Liu et al., 2017), accounting for about 70% of the total-CH4 emissions (Li et al., 2010). Moreover, the plant-mediated transport can also transport O2 to plant organ (Silvey et al., 2019). Therefore, investigations into the plant-mediated transport process are required to further our understanding of the role of plants in CW CH4 contributions. Plant-mediated CH4 transport mechanisms can be classified as molecular diffusion or convective transport processes. Molecular diffusion rates depend primarily on the CH4 gradient between plant roots and above-ground parts, along with the interior of the plant organ and the atmosphere, with the capacity for diffusion being susceptible to ambient temperatures (Joabsson et al., 1999). In contrast, gas movement by convective transport relies on the pressure difference between the inner-and outer-plant environment (Orr, 1992). Different plant species exhibit a diverse range of CH4 transport processes, with convective transport processes typically more effective than molecular diffusion processes (Xu H. et al., 2021). In a recent study by Feng et al. (2022), a novel plant-girdling method was developed, removing the epidermis and subepidermal sclerenchyma to disrupt the O2 transport pathway, suppressing O2 release and increasing CH4 emissions, verifying that wetland plants aerenchyma play a vital role in the transport of CH4.
Oxidation of CH4 in CWs
During CH4 production and transport processes, CH4 oxidation is a significant factor affecting CH4 fluxes. CH4 oxidation can occur via aerobic or anaerobic pathways. Aerobic oxidation of CH4 (Table 2) mainly occurs at micro-interfaces where CH4 and O2 coexist, such as the substrate-air and water-air interfaces, the plant rhizosphere, and within plant tissues (Figure 3; Bonetti et al., 2021). Aerobic CH4 oxidation is a chemical process with rapid reaction rates, depending on the concentration of O2. Under anaerobic conditions, microbes use electron acceptors other than O2 to oxidize CH4, including sulfate (sulfate-reduction-dependent anaerobic methane oxidation, SAMO), (nitrite-dependent anaerobic methane oxidation), metal oxides (e.g., Fe3+ and Mn4+ anaerobic methane oxidation, metal-AOM), and direct interspecies electron transfer (Wegener et al., 2015; Guerrero-Cruz et al., 2021). Among these, SAMO is driven by anaerobic methanotrophs and sulfate-reducing bacteria, while nitrite-dependent AMO is performed by Candidatus Methylomirabilis oxyfera and Candidatus Methanoperedens nitroreducens (Cui et al., 2015). Additionally, metal-AMO is thermodynamically easier than SAMO (occurring at a 2-to 10-folds faster rate than SAMO) because metal-AMO produces more energy, accelerating the AMO process (Zhang et al., 2022). Mn-AMO has been estimated to reduce total-CH4 emissions by 66% (Liu et al., 2020) and Fe-AMO has the potential to decrease CH4 emissions by 2-fold if it was to oxidize 10% of CH4 worldwide (Egger et al., 2015). Among the different types of CWs, the use of VSSFCWs may be preferable for the reduction of CH4 emissions as they have a suitable aerobic/anaerobic interface due to intermittent flooding, which is beneficial for both aerobic oxidation and AOM processes (Zhang et al., 2022).
Methyl coenzyme M reductase (mcrA) and particulate methane monooxygenase (pmoA), are key enzymes in CH4 production and oxidation, resulting in their use as phylogenetic biomarkers for methanogens and methanotrophs (Chen et al., 2020b). Methanotrophs are the only known CH4 biosinks, oxidizing another component of methanogenesis as carbon sources and producing CO2, consuming at least 10% of atmospheric CH4 in the process (O'Connor et al., 2010). CH4 emissions have been found to positively correlate with the abundance of mcrA (Zhang et al., 2021d), and negatively correlate with the abundance of pmoA (Xu H. et al., 2021). However, no significant relationship was observed between pomA and CH4 emissions, suggesting that other factors also mediate their function and activity (Zhang et al., 2021d). The mcrA/pmoA ratio can be used to explore the quantitative relationship between CH4 production, oxidation and emissions, with a higher pmoA/mcrA ratio indicating higher CH4 oxidation potential and lower CH4 emissions (Zhao et al., 2022).
Environmental factors influencing CH4 reduction in CWs
Temperature
Temperature influences the production and oxidation of CH4. The optimum temperature for methanogenesis is typically 35°C–40°C (Ralf, 2007), with low temperatures impairing the activity of methanogens and fermentative bacteria (Barbera et al., 2015) by reducing the rate of organic matter degradation and hence, substrate availability for CH4 production (Zhu et al., 2007; Maucieri et al., 2019). CH4 production has been found to positively correlate with temperature to some extent under sufficient substrate availability conditions (Bhattacharyya et al., 2013; Zhao et al., 2022). For example, methanogenesis rate at 12°C is significantly lower than that at 30°C (Maucieri et al., 2019). Temperature not only influence microbial activity, but also affect the succession of dominant methanogenic archaea. According to Lu Y. et al. (2015), methanogens were dominated by Methanosarcinaceae (utilizing acetate and H2/CO2 substrates), while as temperature lower, Methanosaetaceae dominate the methanogens (using acetate for CH4 production). Methanotrophs is temperature non-sensitive species and their optimum temperature is 25°C. CH4 can be oxidized either at low to-2°C or at high to 30°C (Zhang et al., 2021a).
As a result of microbial activity influenced by temperature, CWs in warm season can release higher CH4 (3.4%–42%) than the cool or cold season (López et al., 2019; Maucieri et al., 2019; Shao et al., 2020). Johansson et al. (2004) found that CH4 fluxes in SFCWs were strongly driven by season, fluctuating from-375 mg/m2/d to 1739 mg/m2/d for the spring to autumn period. Wang et al. (2019) concluded that average CH4 emission in summer is 1.7 times higher than in winter, which was also proved by Zhao et al. (2014), D'Acunha and Johnson (2019), and Liu et al. (2019a). Therefore, in order to reduce the release of CH4, it is recommended to control the temperature at an appropriate time of higher temperatures in summer.
Water table position
Water table position determines the degree of anaerobiosis inside CWs. High water tables can exhibit slower rates of atmospheric O2 diffusion, creating a larger anoxic zone which is beneficial to CH4 production (McInerney and Helton, 2016). Henneberg et al. (2015) compared CH4 emissions in CW mesocosms with 0 cm, −10 cm, and −20 cm water tables, showing that CH4 emissions were much higher in the 0 cm water table treatment system than the −20 cm system both with and without vegetation. This phenomenon may partly be due to higher levels of CH4 dissolution occurring at increased water depths (Zhou et al., 2020). When the water table is below the substrate surface, the CH4 produced is oxidized as it travels through the water layer due to diffusion and ebullition, resulting in a reduction in CH4 emissions (Bonetti et al., 2021). When the water table is high, CH4 emissions are increased as most CH4 enters plant root systems in the deeper anaerobic layer, before being transported to the atmosphere through the aerenchyma (Henneberg et al., 2015). A significant positive correlation has been reported between CH4 emission rates and water table positions (Liu et al., 2009), with studies also documenting that net CH4 fluxes are reduced in systems with a lower water table (Bridgham et al., 2013).
Redox potential
Redox potential (Eh) is an indicator of the internal O2 level in CWs, which determines the activity of microbes and various enzymes, and is closely linked to CH4 production and oxidation processes (Liu et al., 2009). Different microbial groups require varying Eh conditions, with aerobic microbes generally requiring an Eh between +300 and + 400 mV. Parthenogenic anaerobic microbes typically have a cut-off Eh of +100 mV, with aerobic respiration occurring at Eh levels above this point and anaerobic respiration occurring at lower Eh levels. Specialized anaerobic bacteria typically require an Eh of-200 to-250 mV. The conversion of organic matter to CH4 occurs via the general anaerobic digestion pathway, while methanogens at the end of the respiratory chain require a strong reducing environment and very low Eh conditions (optimally-350 mV), with methanogenic processes initiated at Eh conditions < −200 mV (Chen et al., 2020b). The CH4 production potential of a system increases with decreasing Eh (Liu et al., 2019b; Shao et al., 2020), and principal component analysis studies have also demonstrated that CH4 fluxes are positively correlated with Eh conditions (Liu et al., 2019a).
The mechanisms and methods of CH4 reduction in design of CWs
CH4 generation, transport, and oxidation are the three main processes that contribute to net CH4 emissions from CWs (Bridgham et al., 2013), which are influenced by CWs design. Schemes implemented for the reduction of CH4 emissions have mainly depended on the reduction of CH4 generation and the promotion of CH4 oxidation (Mander et al., 2014). which are affect by CW types, plant species, substrate types, the effect of CW-MFC systems, O2 supply, available carbon source, and salinity. Therefore, a comprehensive analysis is required to provide a basis for the effective regulation and operation of CWs, while also actively mitigating GWP contributions (Figure 4; Liu et al., 2017; Maucieri et al., 2017).
Selection of suitable CW type
CWs are classified as SFCWs, HSSFCWs and VSSFCWs due to their varying structures and characteristics, resulting in significant differences in CH4 emission profiles (Zhang et al., 2021a). SFCW systems consist of wastewater flowing over a substrate layer (Maucieri et al., 2017), while VSSFCW systems are gradually infiltrated by wastewater being applied to the surface layer by intermittent feeding, with the wetland bed maintaining an aerobic state with strong reoxygenation capabilities (Liu et al., 2019a). In contrast, wastewater is applied to HSSFCWs through the substrate layer by horizontal percolation in a mixed environment with aerobic, anoxic, and anaerobic degradation pathways, where the bed is submerged for a prolonged period of time causing anoxia (Zhou et al., 2020).
CH4 emissions from SSFCWs have been found to be significantly lower than from SFCWs (Table 3). In a survey by VanderZaag et al. (2010), SFCWs were found to emit 2-to 3-fold more CH4 as a percentage of carbon removal than SSFCWs. The conditions associated with the highest CH4 fluxes from SFCWs and the lowest fluxes from VSSFCWs were studied by Liu et al. (2009). The reason for the observed variation was that SFCWs exhibit very low reoxygenation rates, that are insufficient for the complete oxidation of organic matter and readily create anaerobic conditions that promote CH4 release (Søvik et al., 2006), with this effect usually observed in wetland systems that do not have routine harvesting of above-ground plant biomass, providing a continual supply of organic carbon (Hernandez et al., 2018). However, SSFCWs increase the contact time in the aerobic headspace and rhizosphere, which hinders the movement of gases (Zhu et al., 2007), resulting in more CH4 oxidation occurring in SSFCWs than in SFCWs and lower CH4 fluxes. Liu et al. (2009) also discovered that Eh conditions < −100 mV were primarily found in SFCWs, while no Eh was identified in VSSFCWs, supporting the association between Eh conditions and the high CH4 fluxes observed in SFCWs.
CH4 fluxes from HSSFCWs were found to be higher than those from VSSFCWs (Table 3). For example, Bateganya et al. (2015) found that HSSFCWs emit more CH4 than VSSFCWs, irrespective of plant growth. Zhou et al. (2020) designed a hybrid CW system that generated about 4.8-fold lower CH4 fluxes in the VSSF bed section than the HSSF bed section. The reason for this difference may be attributed to VSSFCWs having efficient O2 transport, while HSSFCWs contain anoxic-anaerobic conditions (Mander et al., 2008), exhibiting negative Eh levels (−100 mV to-500 mV) and low dissolved oxygen (DO) concentrations (< 2 mg/L; López et al., 2015). In contrast, the VSSF system was found to be higher than the HSSF system in a study by Zhang et al. (2021a), due to the potential for CH4 emissions to be affected by competition between various methanogens. A life cycle assessment also concluded that the environmental impact of CH4 emissions from VSSFCWs was half or less than those from HSSFCWs (Fuchs et al., 2011).
CWs are increasingly being designed with composite structures that are more valid and robust for the treatment of a wide range of wastewater types (Liu et al., 2019a), However, these composite CW systems often have a negative effect on CH4 fluxes (Zhou et al., 2020). For example, a monitoring study found that CH4 emissions from VSSF-HSSF-SF CW was higher than from both VSSFCW and SFCW (Liu et al., 2009). Liu et al. (2019a,b) and Liu et al. (2018) designed integrated VSSFCWs that consist of alternating multifunctional layers of aerobic-anoxic-anaerobic-anoxic-aerobic conditions, leading to the accumulation of methanogens and increased CH4 emissions (Zhang et al., 2021a). Waste resource conservation and reducing environmental influence have become priorities in sustainable engineering design. Therefore, the small occupation area of VSSFCWs, along with their high treatment efficiency and relatively low level of CH4 fluxes, make VSSFCW systems more extensively used, and a more CH4 flux can be reduced via an enhanced O2 transfer approach (Liu et al., 2019b).
Plant species selection
The relationships between CH4 emissions and plant occurrence and diversity in CWs remain unknown (Han et al., 2019), as plants are able to both produce CH4 independently, and mediate or influence CH4 emission pathways. For example, organic matter and root exudates (such as sugars and amino acids) synthesized by plants via photosynthesis, can provide electron donors (Chen et al., 2020c; Liu et al., 2022), while root exudates also release degradable carbon which increases the number of methanogens and methanotrophs (Zhang et al., 2018b). Furthermore, plant root-secreted O2 can provide electron acceptors (Kasak et al., 2020), with the intensity of O2 secretion varying depending on the plant species, biomass, temperature, O2 concentration, and photointensity conditions (Feng et al., 2022). In typical VSSFCWs, O2 released by plant roots can provide approximately 0.43–1.12% of the biochemical oxygen demand (Zhang et al., 2014). The rhizosphere is a crucial zone for the production and oxidation of CH4, with plant species composition, diversity, and planting density affecting the release of CH4 (Chen et al., 2020c).
It has been observed that vegetation-covered CWs produce less CH4 than those without vegetative cover (Zhu et al., 2007; Bateganya et al., 2015). However, contradictory results have been reported by Silvey et al. (2019), with this variation potentially due to plant species variation. Chen et al. (2020c) reported that planting Cyperus alternifolius resulted in CWs having lower CH4 fluxes than unvegetated CWs, while planting Phragmites australis and Canna indica had the opposite effect, highlighting the varying effect of different species on CH4 fluxes. Firstly, some plants have been found to suppress CH4 fluxes (Table 4). A monitoring study by Bateganya et al. (2015) found that planting Cyperus papyrus was more effective for the suppression of CH4 fluxes regardless of the CW types, due to the extensive belowground rhizome network facilitating O2 transfer (Xu et al., 2019; Zhang et al., 2021a). Cyperus alternifolius is a ciliate with large root surface areas and root lengths of approximately 20 cm, providing them with the capacity to reach the bottom of CWs and release high amounts of root O2, leading to a more extensive aerobic environment (Chen et al., 2020c). The presence of Oenanthe javanicade has been shown to reduce the carbon concentration in wastewater, minimizing the production of CH4 (Zhao et al., 2016; Han et al., 2017). In contrast, some plant species enhance CH4 emissions (Table 4; Du et al., 2018; Maucieri et al., 2019). Juncus effusus has a significant capacity to transport CH4 (Henneberg et al., 2015), and Rumex japonicus possesses a high root biomass capable of secreting low molecular weight substances (Zhao et al., 2016), accelerating microbial activity and increasing CH4 emissions (Du et al., 2018). Overall, the contribution of plants to CH
4
emissions remains unclear. For example, Phragmites australis has an extensive rhizome system that typically penetrates substrate depths of 0.6–1.0 m, and has been reported to effectively reduce CH4 fluxes (Xu et al., 2019), while other studies have reported that Phragmites australis possesses highly developed aerenchyma that allow more efficient gas transfer and increase CH4 emissions (Sheng et al., 2015; Chen et al., 2020c). Moreover, some studies have proposed that certain plant species has no overall impact on CW CH4 emissions (Han et al., 2019; Maucieri et al., 2019). Instead, plant characteristics such as root porosity and belowground biomass can regulate microbial community competition, O2 transfer efficiency to the root system, and carbon source inputs, leading to varying levels of CH4 productivity in different species (Maucieri et al., 2017; Zhang et al., 2018b). The average CH4 fluxes of submerged plants are generally lower than those of emergent plants (Niu et al., 2015; Zhang et al., 2018a). O2 and carbon inputs from emergent plants can significantly affect the methanogenic community structure and methanogenic pathways, while submerged plants are primarily subject to regulation by DO and nitrogen levels (Zhang et al., 2018a). Furthermore, in contrast to the comparatively less-rigid forb species, some structurally rigid graminoids exhibit larger aerenchyma, which increases their ability to transport O2 between the roots. Mesocosms containing Asclepias incaranta were found to have average CH4 fluxes that were 8-fold higher than those of mesocosms containing Alisma triviale (Silvey et al., 2019). Therefore, the selection of suitable plant species composition is essential to minimize CH4 emissions from CWs.
Plant species diversity has been widely studied in recent years, and its contribution to CH4 emissions has increasingly being investigated. Several studies have observed a positive correlation between CH4 emissions and plant species diversity (Du et al., 2018), as high species diversity increases carbon source available and promotes CH4 emissions (Zhang et al., 2012). Some studies have also found that plant species diversity have no significant influence on CH4 emissions (Zhao et al., 2016; Han et al., 2019), as in high ammonium environments, denitrification has a higher capacity to produce thermodynamic processes than methanogenesis (Chen et al., 2020c). In addition, denitrifying bacteria have a competitive organic substrate advantage, limiting CH4 formation (Zhang et al., 2018a), in which high ammonium loading and plant biomass can perform CH4 offsetting functions, leaving CH4 emissions unchanged overall (Han et al., 2019). Moreover, plant density has been reported to have no influence on CH4 emissions (Hernandez et al., 2018). In conclusion, species characteristics remain a critical driver although more research is required in this field.
The contribution of plant harvesting to CH4 emissions is also significant (Feng et al., 2022). Non-harvested wetland plants can promote methanotrophic CH4 consumption by transporting O2 to the roots and substrate through aerenchyma (Zhu et al., 2007). However, the dying plant biomass provides an abundant source of bioenergy and promotes methanogenesis, with the potential to generate 10–40% of annual atmospheric CH4 emissions worldwide (Juutinen et al., 2003; Keppler et al., 2006), highlighting the need for plant harvesting to be carefully managed. The time and manner of harvesting of aboveground macrophytes also affects CH4 emissions from CWs (Kasak et al., 2020). Ensuring that harvesting occurs at the end of the growing season (i.e., before nutrient transfer to belowground plant structures) can significantly reduce CH4 emissions. However, biomass harvesting during peak periods of plant growth and soil microbial activity has been shown to significantly enhance CH4 emissions (Barbera et al., 2015), due to the rapid release of CH4 accumulated in the vascular system of plant stalks (Kasak et al., 2020). Therefore, effective planning to optimize harvesting time and method can effectively reduce CH4 emissions from managed wetlands, and thus enhance their multiple ecological benefits.
Substrate amendment
The substrate forms the backbone of CWs, providing support for the growth of plants and microbes (Ji et al., 2021). Recently, novel substrate amendment schemes have been applied in CWs, with the addition of substances such as biochar, iron oxides, manganese oxides, zeolite, walnut shell, activated alumina, and ferric-carbon, which are gradually displacing traditional substrates (such as sand, gravel, and ceramsite) and improving the treatment efficiency of CWs. Some reviews have focused on the impact of substrate on CH4 emission reduction. However, none of these have emphasized the role of enhanced substrates compared to conventional substrates (Yu et al., 2022; Zhao et al., 2022). Therefore, in order to achieve sustainable and low-environmental impact CW operations, we summarized multifarious functional substrates used in CWs for enhancing the CH4 emissions reduction.
Carbon-rich substrate types
Biochar is an organic carbon-enriched product that is considered a promising alternative substrate (Zhuang et al., 2022). Ji et al. (2021) showed that biochar-based CWs contained a higher pmoA/mcrA ratio than none-biochar CWs, with the addition of biochar having an inhibitory effect on CH4 fluxes, possibly due to biochar promoting the secretion of O2 from plant roots, increasing CH4 oxidation (Ji et al., 2020). However, Chen et al. (2020a) and Guo et al. (2020) proposed contrasting conclusions, finding that CH4 fluxes were consistently higher in biochar-added CWs than CWs without added biochar. This may be due to biochar enhancing direct interspecies electron transfer between methanogens and Geobacteraceae, while also providing organic matter to methanogens, resulting in the stimulation of CH4 emissions (Liu et al., 2012). Overall, the influences of biochar on CH4 emissions remains unclear (Table 5), with the reported differences primarily caused by variations in the raw biochar materials, operating conditions, and the properties of the microbial community within the system (Chen et al., 2020a), highlighting the need for further research to determine the role of biochar in regulating CH4 emissions (Zhuang et al., 2022). Walnut shell is also loaded with high concentrations of organic matter. Xu G. et al. (2021) showed that CH4 emissions from walnut shell substrate were 14.8-fold higher than from the control substrate, due to the release of large amounts of degradable organic carbon from walnut shell, resulting in high CH4 fluxes.
Electron-exchange substrate types
Highly crystalline iron oxides and manganese oxides are electron-exchange substrates that are abundant and readily available, making them highly suitable for use as CW substrate materials (Zhang et al., 2021b; Yu et al., 2022). Cheng et al. (2021a) reported that CWs using iron oxide substrates emitted less CH4, finding that iron oxide increased CH4 emissions by promoting electron transfer between Geobacter and methanogens, while also directly inhibiting the activity of methanogens and some enzymes involved in CO2 reduction, and promoting the AOM process under the influence of dissimilated metal-reducing bacteria, ultimately resulting in a reduction in CH4 emissions overall (Cheng et al., 2021b). Mn ore substrates have been found to reduce CH4 emissions from CWs (Liu et al., 2020). In addition, Cheng et al. (2021b) observed that both Mn ore and iron ore substrates inhibited CH4 emissions, with Mn ore reported to be more effective due to the fact that Mn ore promotes AOM processes mainly by competing for organic substrates and providing electron acceptors, almost completely inhibiting CH4 production (Xu G. et al., 2021). However, the use of iron oxide as a substrate has more complex implications, such as different forms and valences of iron affecting CH4 production (Cheng et al., 2021a).
Adsorption substrate types
Fe-C is widely used as a substrate in wastewater treatment, utilizing chemistry-coupled biological processes for the removal of pollutants (Dong et al., 2020). Zeolite, a common silicate mineral, is considered to be a high-performance gas adsorption material, due to its relatively well-developed pore network and skeletal configuration (Wang Y. et al., 2020), with well characterized CH4 adsorption, storage, and oxidation capabilities (Wang H. et al., 2020; Zhou et al., 2020). Zhao et al. (2022) monitored CH4 emissions from laboratory-scale CWs containing different substrates, showing that systems utilizing Fe-C and zeolite as substrates all exhibited lower average CH4 fluxes than the control groups (Table 5), as Fe-C can compete with methanogens for substrate in the presence of iron-reducing bacteria, inhibiting the production of CH4. In addition, Fe3+ has a high Eh as an electron acceptor (Bond and Lovley, 2002), and the larger surface area of activated carbon facilitates biofilm generation, promoting CH4 oxidation. Zeolite incorporation into the substrate has been found to significantly reduce CH4 emissions from CWs. Zhou et al. (2020) discovered that CWs containing zeolite substrate exhibited reduced CH4 fluxes by about 2-fold compared to those of gravel substrate CWs, due to the porous structure of zeolite improving local atmospheric DO concentrations and reducing methanogen activity. The results of these studies are further supported by the observation that Fe-C and zeolite substrates contained a reduced abundance of the functional gene mcrA and a significantly increased abundance of pmoA (Zhao et al., 2022). Activated alumina is also a powerful adsorption substrate, as shown in a study by Xu G. et al. (2021) in which activated alumina significantly reduced CH4 emissions from CWs due to its strong adsorption capacity, leading to reduction in organic matter content and inhibiting methanogenic microbial activity (Alvarez and Cervantes, 2012). In addition, sand, ceramsite and gravel have also been utilized as adsorption fillers (Table 5; Wang Y. et al., 2020), but they have been gradually replaced due to poor CH4 adsorption capacities (Ji et al., 2020; Cheng et al., 2021a; Zhao et al., 2022). With a survey of CWs finding that mcrA and pmoA were not detectable in all gravel substrates (Chen et al., 2020c), which was attributed to the fact that methanogens and methanotrophs do not easily attach to gravel so it is gradually replaced.
CW-coupled MFC systems
MFCs are a low-environmental impact energy utilization technology (Wang et al., 2019; Zhang et al., 2021d). CWs (especially VSSFCWs) have unique water quality conditions with substantial redox gradients across their vertical profile, with DO and Eh levels increasing from the subsurface to the surface zones (Maucieri et al., 2017). As CWs and MFC systems function under similar conditions, it is feasible to control CH4 emissions using CW-coupled MFC systems (Zhang et al., 2021b,c). Methanogens and electrogenic bacteria require similar living conditions, such as an anaerobic environment and low Eh, resulting in competition for the substrate at CW-MFC anodes, which may inhibit the power generation performance of the CW-MFC (Liu et al., 2017; Zhang K. et al., 2020). A comprehensive understanding of the competitive mechanisms between methanogens and electrogens would help maximize the potential advantages of CW-MFC systems for efficient biopower generation and the reduction of CH4 emissions (Zhang et al., 2021e), along with determining the influences of circuit condition, plant type, external resistance, substrate type, and hydraulic retention time (HRT).
Open/closed circuit in CW-MFC
Open or closed circuit systems are one of the essential factors affecting CH4 fluxes from CW-MFCs. Liu et al. (2022) found that the CH4 emissions from non-planted CW-MFCs, were about 0.21 ± 0.01 mg/m2/h higher in open-circuit systems than in closed-circuit systems, while in the planted open-circuit system the CH4 emissions were 0.46 ± 0.02 mg/m2/h higher than in the closed-circuit planted system. A study by Xu et al. (2021b) yielded CH4 fluxes of 6.37–7.28 mg/m2/h and 7.43–8.36 mg/m2/h for closed and open circuit CW-MFC systems, respectively. Zhang et al. (2021e) noted that running a MFC in CWs can suppress a third of all CH4 emissions. These studies show that CW-MFCs can effectively reduce CH4 emissions from CWs, with similar findings also reported by Zhang K. et al. (2020) and Zhang et al. (2021c), with the main contributory factor being the bioanode. In anaerobic environments, methanogens are dominant in CWs due to the absence of current transmission, resulting in an increase in the production of CH4 (Zhang et al., 2021d). In contrast, because organic matter is more readily available for electrochemically active bacteria (EAB) in closed-circuit CW-MFC systems, electrical stimulation of EAB growth results in a current that inhibits methanogens (Liu et al., 2022). In addition, electrons from the anode may compete with methanogens as the anode layer of the CW-MFC has a higher Eh, allowing electron-producing bacteria to capture electrons more easily than methanogens, leading to a reduction in CH4 emissions (Zhang et al., 2021e).
Plant rhizosphere location
CH4 emissions are significantly affected by plants in CW-MFC systems. Recently, an increasing number of studies have demonstrated that plant root location has an influential effect on CH4 emissions in CW-MFC systems (Liu et al., 2017). When the plant rhizosphere is in the cathode layer, electron acceptors provided by root-secreted O2 favor cathode reactions and power generation (Zhang et al., 2021e). Since CW-MFC anodes are typically in an anoxic state rather than an anaerobic state, the small amount of O2 secreted by plant roots has little effect on the O2 levels in the anode environment (Zhang et al., 2021d). Therefore, plant rhizospheres in the anodic zone can provide photosynthetic organic matter as an alternative energy source, increasing CH4 emissions. Zhang et al. (2021e) reported that CW-MFC systems with rhizospheres in the cathode zone emit less total-CH4 (29.21 mg/m2/d) than those with rhizospheres at the anode (33.01 mg/m2/d). Zhang et al. (2021d) observed that growing Typha orientalis and Cyperus alternifolius in the cathode zone of a reactor, resulted in lower CH4 emissions than when grown at the anode. Zhang et al. (2021c) reported a similar conclusion, with methanogens becoming more active as the organic matter content of the rhizosphere increases, resulting in an increase in CH4 emissions. Additionally, the O2 provided by plant roots has been found to have less of an impact on lowering CH4 emissions than the organic matter in the root system (Lu L. et al., 2015).
The presence of plants and the selected plant species also influence CH4 emissions in CW-MFC systems. Liu et al. (2022) reported that CH4 emissions increased by 0.48 ± 0.02 mg/m2/h in closed-circuit CW systems with plants, compared to the non-planted group. A similar conclusion was reached by Xu et al. (2021b), with a 21.79% reduction in average CH4 emissions from non-planted reactors, as compared to those with plants. Zhang et al. (2021d) reported lower CH4 emissions in systems with anode grown Typha orientalis (3.9 mg/m2/h), than with anode grown Cyperus alternifolius (4.5 mg/m2/h). A study comparing the effects of three plant species, Typha orientalis, Thalia dealbata, and Cyperus alternifolius, found that CH4 emissions from the CW-MFC were highest in the Typha orientalis system, and lowest in the Cyperus alternifolius system (Zhang K. et al., 2020). These results emphasize the importance of further research to determine the effect of different plant species on CH4 emissions from CW-MFC systems.
The important role that plants play in CH4 emissions from CW-MFC systems is becoming increasingly apparent, with both the plant species and root location influencing CH4 emissions, although root location appears to have a more pronounced effect (Zhang et al., 2021d). Therefore, focusing on plant selection to ensure that plants with inhibiting methanogenesis or poor CH4 transport are utilized, with their roots located in the cathodic zone, has been shown to be more advantageous for the sustainable development of wastewater treatment systems, in terms of reactor power generation and CH4 reduction.
External resistance
The long-term application of external resistance in CW-MFC systems affects the microbial community structure and biochemical metabolism in the anode biofilm (Zhang et al., 2021c). Liu et al. (2022) observed that an external resistance of 1,000 Ω leads to an increase in CH4 emissions by 0.67 ± 0.01 mg/m2/h compared to a 100 Ω resistance. Wang et al. (2019) found that CH4 emissions tended to increase with the addition of external resistances >500 Ω. This effect occurs due to variation in microbial metabolic activities, electron transfer rates and substrate utilization kinetics, under varying external resistance conditions (Liu et al., 2022). Higher external resistance loads slow down the flow of electrons to and from EAB, which encourages methanogens to consume more substrate and increases CH4 emissions (Liu et al., 2017). However, it has also been found that insufficiently low external resistances lead to rapid electron flow rates, which are not conducive to the sustainable utilization of CW-MFC systems, and eventually leads to a decline in power generation (Nikhil et al., 2018). Therefore, a suitable relatively low external resistance should be applied, ensuring that CW-MFC systems generate maximum levels of power, while releasing minimum CH4 (Liu et al., 2022).
Additional influencing factors
Substrate. CH4 emissions in CW-MFC systems are also driven by substrate type. A Mn CW-MFC system was shown to generate lower CH4 emissions (53.44–66.64 mg/m2/h) than a clinopyrite CW-MFC system (62.69–88.02 mg/m2/h; Zhang et al., 2021b). Furthermore, Xu et al. (2021b) observed a 25.42% reduction in average CH4 emissions from Mn-based reactors compared to graphite granule-based reactors. This may be due to the occurrence of Mn-driven Mn-AOM lowering CH4 emissions, while dissimilatory metal reduction processes encourage competition between EABs and methanogens, as well as increasing the growth of EABs on Mn ore anodes, further inhibiting the growth of methanogens (Li et al., 2019; Zhang et al., 2021b, 2022). Therefore, different substrate materials have varying electron acceptor functions and significantly affect CH4 emissions, with Mn exhibiting high prospects as a substrate for CH4 emissions reduction.
HRT. Due to the relatively high organic load in CW-MFCs, there is a positive correlation between CH4 emissions and HRT. Lower HRTs result in a higher load and increased CH4 emissions (Zhang et al., 2021e). It was observed that when the HRT in plant systems increased, CH4 fluxes tended to reduce, with higher organic matter contents stimulating the activity of inactive microorganisms, consuming DO, and promoting the growth of methanogens (Wang et al., 2019). HRT is an extremely vital operating parameter for conventional wetlands (Zhang K. et al., 2020). A longer HRT can prolong the contact time between microorganisms and wastewater (Zhang et al., 2021d), ensuring the effective removal of organic matter as well as the suppression of CH4 emissions. Finally, it has been found that CW-MFC systems have a stronger CH4 reduction effect when operated in continuous flow mode than in batch mode (Wang et al., 2019).
Other methods
Oxygen supply strategy
Shortage of DO in CWs due to prolonged saturation and rapid microbial metabolism is a major limitation to the removal of organic matter from conventional CWs (Wang et al., 2022). Intermittent aeration and tidal flow are considered to be the most effective oxygenation strategies to directly influence CH4 emissions, allowing the manipulation of DO conditions in CWs (Table 5; Ji et al., 2020), providing additional O2 for the inhibition of CH4 biochemical processes and the acceleration of CH4 oxidation, reducing CH4 fluxes (Ji et al., 2021). D'Acunha and Johnson (2019) found that CH4 fluxes could be reduced by 60.7% using intermittent microaeration under optimal aeration conditions. Furthermore, Zhao et al. (2022) observed increased CH4 fluxes in aerated sections of CWs compared to non-aerated sections, which was attributed to CH4 production primarily occurring in non-aerated sections, while CH4 emissions were enhanced by agitation and blowing in aerated sections, with the change in emissions consistent with the aeration rate and dissolved CH4 concentration. Ji et al. (2020) showed that only a slight decrease in CH4 fluxes occurred under tidal flow oxygenation conditions, although Ji et al. (2021) concluded that the total CH4 fluxes were slightly higher due to the loss of large amounts of CH4 from the empty substrate after drainage. However, the use of intermittent aeration increases operational costs and energy input requirements, making it more suitable for use in CWs in relatively concentrated communities or limited land areas. In contrast, the tidal flow process involves no significant additional costs or maintenance expenses.
Carbon source supplement
The available carbon sources in CWs can be supplied internally or externally. Internal carbon sources mainly include plant roots or microbial secretions, plant deadfall, organic matter decomposition, and substrates (Liu et al., 2019a), while external carbon sources include biodegradable carbon, natural plant material, and soluble carbon from natural organic matter (Liu et al., 2019b). To balance the nutritional ratio in CWs, additional carbon sources must be provided in some CWs, directly affecting CH4 emissions (Chen et al., 2020b). C/N ratios represent the relative amount of carbon and nitrogen available in the wastewater, with different C/N ratios promoting or inhibiting microbial activity (Guo et al., 2020). Yan et al. (2012) found that CH4 fluxes in CWs with C/N ratios of 2.5, 5, and 10 were 1.36 ± 0.09 mg/m2/h, 2.02 ± 0.07 mg/m2/h, and 2.34 ± 0.15 mg/m2/h, respectively, showing that CH4 fluxes were lowest with low C/N ratio conditions. Corbella and Puigagut (2015) also reported a positive correlation between CH4 emissions and influent C/N conditions, which may be attributed to increases in C/N causing rapid O2 consumption, resulting in a lower Eh and higher CH4 production (Guo et al., 2020). However, Chen et al. (2020b) analyzed CWs with influent C/N ratios of 0, 5, 10, 15, and 20, showing that CH4 emissions did not differ significantly, reaching a minimum value of −42.49 mg/m2/h at a C/N ratio of 10. Zhao et al. (2014) combined SSFCWs with an earthworm eco-filter and found that CH4 emissions increased in accordance with the influent C/N ratio regardless of the sequence of treatment, as the earthworm activity greatly increased the permeability of the SSFCW. Therefore, lower C/N ratio conditions are more favorable for CH4 emissions reduction. Liu et al. (2019a) observed that the addition of urea as an external carbon source at concentrations of 0, 12.1, 30, 45, 61, and 80 mmol/L, resulted in a trend of increasing and then decreasing CH4 emissions, achieving the lowest emission level with a urea concentration of 80 mmol/L. Similar results were obtained by Liu et al. (2019b) using ethanol as an external carbon source, with results showing that Eh values after the addition of ethanol (except for the highest ethanol concentration of 32 mmol/L) were higher than the control groups, resulting in more CH4 emissions. Excessive carbon source concentration increases the water purification load. Therefore, when external carbon sources are provided, the dosage should be adjusted to ensure optimal water purification performance with CH4 emission control.
Salinity control
With increasing levels of water scarcity, desalination, or the direct utilization of seawater in coastal areas is becoming increasingly common, generating large quantities of saline wastewater. CWs are increasingly being used for the treatment of saline wastewater in response to different regional treatment strategies (Shao et al., 2020). The salinity of the influent affects not only the growth of wetland plants but also the structure, abundance and activity of microbial communities (Sheng et al., 2015; Xiao et al., 2016). According to Shao et al. (2020), CH4 emissions were higher at salinities of 0 and 0.5%, continually decreasing with increasing salinity, with a substantial decrease occurring at 1.0%. Sheng et al. (2015) also observed that CH4 emissions decreased significantly with increasing salinity due to inhibition of methanogen growth and activity (Xiao et al., 2016). In addition, salinity has been repeatedly shown to inhibit CH4 emissions in tidal wetlands, due to competition between sulfate and methanogens for substrates in tidal waters (Poffenbarger et al., 2011; Marton et al., 2012). In summary, the effect of salinity on CH4 fluxes in CWs is pronounced, with emissions promoted by low salinity and inhibited by high salinity conditions.
Conclusions and perspectives
Global climate change is a complex phenomenon. CWs are a highly productive green technology for surface source pollution control, which are designed to treat wastewater using the natural processes of plants, substrates and microorganisms, utilizing carbon cycling processes that are an essential part of the global system, generating both environmental and economic benefits. Currently, CWs have the potential to be restored using known and innovative land management practices, providing significant opportunities for carbon sequestration and CH4 offsetting. CH4 emissions from SFCWs have been found to be significantly higher than from SSFCWs, while CH4 emissions from VSSFCWs were significantly lower than from HSSFCWs. Several species-specific communities have been shown to facilitate the operation of energy-efficient and low-emission CWs. In addition, the selection of an appropriate substrate is also critical for CH4 mitigation. In CW-MFCs, the combination of biological and bio-electrochemical methods can effectively control CH4 emissions from CWs, although it is essential to maintain a stable balance between the systems CH4 production rate and power generation capacity. In addition, the O2 supply strategy, carbon source concentrate, and salinity control are key operational aspects that should be optimized to reduce the CH4 production potential of the system.
As research has progressed in this field, tremendous advances have been made in the control of CH4 emissions from CWs. However, there are still some aspects that require further investigation:
•When construction budgets and operating conditions allow, the use of integrated CWs is more effective for wastewater treatment, and optimization of the design of integrated systems for CH4 reduction will increase the development potential of CWs.
•The effect of different plant species on CH4 emissions in CW systems requires further research, with the rational application of plant litter as a substrate, such as biochar, may be beneficial to the overall sustainability and low-environmental impact of the CWs.
•Different anode materials can function as distinct types of electron acceptors, influencing the oxidation of CH4 in CW-MFC systems. Therefore, the mechanism of effect anode materials on CH4 production and emissions requires further investigation.
•Despite CWs being an essential ecosystem, most of the previously reported results were obtained from controlled mesoscale experiments, resulting in the need for future research to include field assessments conducted over long time spans.
Author contributions
GY was responsible for data curation, formal analysis, and investigation. JC wrote the manuscript draft. GW, HC, and JH contributed to conceptualization, formal analysis, and visualization. YL, WW, FS, YM, QW, MW, TL, ZS, JS, and ZY provided feedback on the manuscript. All authors contributed to the article and approved the submitted version.
Funding
This work was supported by the Hunan Provincial Natural Science Foundation of China (Nos. 2021JJ30728, 2021JJ40596, and 2019JJ50672), the Scientific Research Projects of Ecology and Environment Department of Hunan (No. HBKT-2021012), and the Water Conservancy Science and Technology Project of Hunan Province (No. XSKJ2022068-03).
Conflict of interest
WW and FS were employed by Hunan Pilot Yanghu Reclaimed Water Co., Ltd. YM was employed by Hunan Rongantai Ecological Technology Co., Ltd. QW and MW were employed by CCCC-TDC Environmental Engineering Co., Ltd. TL and ZS were employed by China Railway Wuju Group the First Engineering Co., Ltd.
The remaining authors declare that the research was conducted in the absence of any commercial or financial relationships that could be construed as a potential conflict of interest.
Publisher’s note
All claims expressed in this article are solely those of the authors and do not necessarily represent those of their affiliated organizations, or those of the publisher, the editors and the reviewers. Any product that may be evaluated in this article, or claim that may be made by its manufacturer, is not guaranteed or endorsed by the publisher.
References
Alvarez, L. H., and Cervantes, F. J. (2012). Assessing the impact of alumina nanoparticles in an anaerobic consortium: methanogenic and humus reducing activity. Appl. Microbiol. Biotechnol. 95, 1323–1331. doi: 10.1007/s00253-011-3759-4
Barbera, A. C., Borin, M., Cirelli, G. L., Toscano, A., and Maucieri, C. (2015). Comparison of carbon balance in Mediterranean pilot constructed wetlands vegetated with different C4 plant species. Environ. Sci. Pollut. Res. 22, 2372–2383. doi: 10.1007/s11356-014-2870-3
Bateganya, N. L., Mentler, A., Langergraber, G., Busulwa, H., and Hein, T. (2015). Carbon and nitrogen gaseous fluxes from subsurface flow wetland buffer strips at mesocosm scale in East Africa. Ecol. Eng. 85, 173–184. doi: 10.1016/j.ecoleng.2015.09.081
Bhattacharyya, P., Roy, K. S., Neogi, S., Dash, P. K., Nayak, A. K., Mohanty, S., et al. (2013). Impact of elevated CO2 and temperature on soil C and N dynamics in relation to CH4 and N2O emissions from tropical flooded rice (Oryza sativa L.). Sci. Total Environ. 461-462, 601–611. doi: 10.1016/j.scitotenv.2013.05.035
Bond, D. R., and Lovley, D. R. (2002). Reduction of Fe(III) oxide by methanogens in the presence and absence of extracellular quinones. Environ. Microbiol. 4, 115–124. doi: 10.1046/j.1462-2920.2002.00279.x
Bonetti, G., Trevathan-Tackett, S. M., Hebert, N., Carnell, P. E., and Macreadie, P. I. (2021). Microbial community dynamics behind major release of methane in constructed wetlands. Appl. Soil Ecol. 167:104163. doi: 10.1016/j.apsoil.2021.104163
Bridgham, S. D., Cadillo-Quiroz, H., Keller, J. K., and Zhuang, Q. (2013). Methane emissions from wetlands: biogeochemical, microbial, and modeling perspectives from local to global scales. Glob. Chang. Biol. 19, 1325–1346. doi: 10.1111/gcb.12131
Bruhn, D., Moller, I. M., Mikkelsen, T. N., and Ambus, P. (2012). Terrestrial plant methane production and emission. Physiol. Plant. 144, 201–209. doi: 10.1111/j.1399-3054.2011.01551.x
Chen, X., Zhu, H., Banuelos, G., Shutes, B., Yan, B., and Cheng, R. (2020a). Biochar reduces nitrous oxide but increases methane emissions in batch wetland mesocosms. Chem. Eng. J. 392:124842. doi: 10.1016/j.cej.2020.124842
Chen, X., Zhu, H., Yan, B., Shutes, B., Tian, L., and Wen, H. (2020b). Optimal influent COD/N ratio for obtaining low GHG emissions and high pollutant removal efficiency in constructed wetlands. J. Clean. Prod. 267:122003. doi: 10.1016/j.jclepro.2020.122003
Chen, X., Zhu, H., Yan, B., Shutes, B., Xing, D., Banuelos, G., et al. (2020c). Greenhouse gas emissions and wastewater treatment performance by three plant species in subsurface flow constructed wetland mesocosms. Chemosphere 239:124795. doi: 10.1016/j.chemosphere.2019.124795
Cheng, S., Qin, C., Xie, H., Wang, W., Hu, Z., Liang, S., et al. (2021a). A new insight on the effects of iron oxides and dissimilated metal-reducing bacteria on CH4 emissions in constructed wetland matrix systems. Bioresour. Technol. 320:124296. doi: 10.1016/j.biortech.2020.124296
Cheng, S., Qin, C., Xie, H., Wang, W., Zhang, J., Hu, Z., et al. (2021b). Comprehensive evaluation of manganese oxides and iron oxides as metal substrate materials for constructed wetlands from the perspective of water quality and greenhouse effect. Ecotoxicol. Environ. Saf. 221:112451. doi: 10.1016/j.ecoenv.2021.112451
Corbella, C., and Puigagut, J. (2015). Effect of primary treatment and organic loading on methane emissions from horizontal subsurface flow constructed wetlands treating urban wastewater. Ecol. Eng. 80, 79–84. doi: 10.1016/j.ecoleng.2014.09.071
Cui, M., Ma, A., Qi, H., Zhuang, X., and Zhuang, G. (2015). Anaerobic oxidation of methane: an "active" microbial process. Microbiol. Open 4, 1–11. doi: 10.1002/mbo3.232
D'Acunha, B., and Johnson, M. S. (2019). Water quality and greenhouse gas fluxes for stormwater detained in a constructed wetland. J. Environ. Manag. 231, 1232–1240. doi: 10.1016/j.jenvman.2018.10.106
de Klein, J. J. M., and van der Werf, A. K. (2014). Balancing carbon sequestration and GHG emissions in a constructed wetland. Ecol. Eng. 66, 36–42. doi: 10.1016/j.ecoleng.2013.04.060
Dong, C., Li, M., Zhuang, L.-L., Zhang, J., Shen, Y., and Li, X. (2020). The improvement of pollutant removal in the ferric-carbon micro-electrolysis constructed wetland by partial aeration. Water 12:389. doi: 10.3390/w12020389
Dreyfus, G. B., Xu, Y., Shindell, D. T., Zaelke, D., and Ramanathan, V. (2022). Mitigating climate disruption in time: a self-consistent approach for avoiding both near-term and long-term global warming. Proc. Natl. Acad. Sci. U. S. A. 119:e2123536119. doi: 10.1073/pnas.2123536119
Dridi, B., Fardeau, M.-L., Ollivier, B., Raoult, D., and Drancourt, M. (2012). Methanomassiliicoccus luminyensis gen. Nov., sp. nov., a methanogenic archaeon isolated from human faeces. Int. J. Syst. Evol. Microbiol. 62, 1902–1907. doi: 10.1099/ijs.0.033712-0
Du, Y., Pan, K., Yu, C., Luo, B., Gu, W., Sun, H., et al. (2018). Plant diversity decreases net global warming potential integrating multiple functions in microcosms of constructed wetlands. J. Clean. Prod. 184, 718–726. doi: 10.1016/j.jclepro.2018.02.273
Egger, M., Rasigraf, O., Sapart, C. J., Jilbert, T., Jetten, M. S. M., Rockmann, T., et al. (2015). Iron-mediated anaerobic oxidation of methane in brackish coastal sediments. Environ. Sci. Technol. 49, 277–283. doi: 10.1021/es503663z
Engida, T., Wu, J. M., Xu, D., and Wu, Z. B. (2020). Review paper on horizontal subsurface Flowconstructed wetlands: potential for their use in climate change mitigation and treatment of wastewater. Appl. Ecol. Environ. Res. 18, 1051–1089. doi: 10.15666/aeer/1801_10511089
Evans, P. N., Parks, D. H., Chadwick, G. L., Robbins, S. J., Orphan, V. J., Golding, S. D., et al. (2015). Methane metabolism in the archaeal phylum Bathyarchaeota revealed by genome-centric metagenomics. Science 350, 434–438. doi: 10.1126/science.aac7745
Feng, L., He, S., Yu, H., Zhang, J., Guo, Z., Wei, L., et al. (2022). A novel plant-girdling study in constructed wetland microcosms: insight into the role of plants in oxygen and greenhouse gas transport. Chem. Eng. J. 431:133911. doi: 10.1016/j.cej.2021.133911
Fuchs, V. J., Mihelcic, J. R., and Gierke, J. S. (2011). Life cycle assessment of vertical and horizontal flow constructed wetlands for wastewater treatment considering nitrogen and carbon greenhouse gas emissions. Water Res. 45, 2073–2081. doi: 10.1016/j.watres.2010.12.021
Guerrero-Cruz, S., Vaksmaa, A., Horn, M. A., Niemann, H., Pijuan, M., and Ho, A. (2021). Methanotrophs: discoveries, environmental relevance, and a perspective on current and future applications. Front. Microbiol. 12:678057. doi: 10.3389/fmicb.2021.678057
Gui, P., Inamori, R., Matsumura, M., and Inamori, Y. (2007). Evaluation of constructed wetlands by wastewater purification ability and greenhouse gas emissions. Water Sci. Technol. 56, 49–55. doi: 10.2166/wst.2007.517
Guo, F., Zhang, J., Yang, X., He, Q., Ao, L., and Chen, Y. (2020). Impact of biochar on greenhouse gas emissions from constructed wetlands under various influent chemical oxygen demand to nitrogen ratios. Bioresour. Technol. 303:122908. doi: 10.1016/j.biortech.2020.122908
Han, W., Luo, G., Luo, B., Yu, C., Wang, H., Chang, J., et al. (2019). Effects of plant diversity on greenhouse gas emissions in microcosms simulating vertical constructed wetlands with high ammonium loading. J. Environ. Sci. 77, 229–237. doi: 10.1016/j.jes.2018.08.001
Han, W., Shi, M., Chang, J., Ren, Y., Xu, R., Zhang, C., et al. (2017). Plant species diversity reduces N2O but not CH4 emissions from constructed wetlands under high nitrogen levels. Environ. Sci. Pollut. Res. 24, 5938–5948. doi: 10.1007/s11356-016-8288-3
Henneberg, A., Brix, H., and Sorrell, B. K. (2015). The interactive effect of Juncus effusus and water table position on mesocosm methanogenesis and methane emissions. Plant Soil 400, 45–54. doi: 10.1007/s11104-015-2707-y
Hernandez, M. E., Galindo-Zetina, M., and Carlos Hernandez-Hernandez, J. (2018). Greenhouse gas emissions and pollutant removal in treatment wetlands with ornamental plants under subtropical conditions. Ecol. Eng. 114, 88–95. doi: 10.1016/j.ecoleng.2017.06.001
Ji, B., Chen, J., Li, W., Mei, J., Yang, Y., and Chang, J. (2021). Greenhouse gas emissions from constructed wetlands are mitigated by biochar substrates and distinctly affected by tidal flow and intermittent aeration modes. Environ. Pollut. 271:116328. doi: 10.1016/j.envpol.2020.116328
Ji, B., Chen, J., Mei, J., Chang, J., Li, X., Jia, W., et al. (2020). Roles of biochar media and oxygen supply strategies in treatment performance, greenhouse gas emissions, and bacterial community features of subsurface-flow constructed wetlands. Bioresour. Technol. 302:122890. doi: 10.1016/j.biortech.2020.122890
Joabsson, A., Christensen, T. R., and Wallén, B. (1999). Vascular plant controls on methane emissions from northern peatforming wetlands. Trends Ecol. Evol. 14, 385–388. doi: 10.1016/S0169-5347(99)01649-3
Johansson, A. E., Gustavsson, A. M., Oquist, M. G., and Svensson, B. H. (2004). Methane emissions from a constructed wetland treating wastewater--seasonal and spatial distribution and dependence on edaphic factors. Water Res. 38, 3960–3970. doi: 10.1016/j.watres.2004.07.008
Juutinen, S., Larmola, T., Remus, R., Mirus, E., Merbach, W., Silvola, J., et al. (2003). The contribution of Phragmites australis litter to methane (CH4) emission in planted and non-planted fen microcosms. Biol. Fertil. Soils 38, 10–14. doi: 10.1007/s00374-003-0618-1
Kasak, K., Valach, A. C., Rey-Sanchez, C., Kill, K., Shortt, R., Liu, J., et al. (2020). Experimental harvesting of wetland plants to evaluate trade-offs between reducing methane emissions and removing nutrients accumulated to the biomass in constructed wetlands. Sci. Total Environ. 715:136960. doi: 10.1016/j.scitotenv.2020.136960
Keppler, F., Hamilton, J. T., Brass, M., and Rockmann, T. (2006). Methane emissions from terrestrial plants under aerobic conditions. Nature 439, 187–191. doi: 10.1038/nature04420
Li, T., Huang, Y., Zhang, W., and Song, C. (2010). CH4MODwetland: a biogeophysical model for simulating methane emissions from natural wetlands. Ecol. Model. 221, 666–680. doi: 10.1016/j.ecolmodel.2009.05.017
Li, H., Xu, H., Yang, Y.-L., Yang, X.-L., Wu, Y., Zhang, S., et al. (2019). Effects of graphite and Mn ore media on electro-active bacteria enrichment and fate of antibiotic and corresponding resistance gene in up flow microbial fuel cell constructed wetland. Water Res. 165:114988. doi: 10.1016/j.watres.2019.114988
Liu, S., Feng, X., and Li, X. (2017). Bioelectrochemical approach for control of methane emission from wetlands. Bioresour. Technol. 241, 812–820. doi: 10.1016/j.biortech.2017.06.031
Liu, X., Fu, X., Pu, A., Zhang, K., Luo, H., Anderson, B. C., et al. (2019a). Impact of external carbon source addition on methane emissions from a vertical subsurface-flow constructed wetland. Greenhouse Gases-Sci. Technol. 9, 331–348. doi: 10.1002/ghg.1847
Liu, F., Rotaru, A.-E., Shrestha, P. M., Malvankar, N. S., Nevin, K. P., and Lovley, D. R. (2012). Promoting direct interspecies electron transfer with activated carbon. Energy Environ. Sci. 5:8982. doi: 10.1039/c2ee22459c
Liu, X., Wang, J., Fu, X., Luo, H., Anderson, B. C., Zhang, K., et al. (2019b). Methane emissions driven by adding a gradient of ethanol as carbon source in integrated vertical-flow constructed wetlands. Water 11:1086. doi: 10.3390/w11051086
Liu, W., Xiao, H., Ma, H., Li, Y., Adyel, T. M., and Zhai, J. (2020). Reduction of methane emissions from manganese-rich constructed wetlands: role of manganese-dependent anaerobic methane oxidation. Chem. Eng. J. 387:123402. doi: 10.1016/j.cej.2019.123402
Liu, C., Xu, K., Inamori, R., Ebie, Y., Liao, J., and Inamori, Y. (2009). Pilot-scale studies of domestic wastewater treatment by typical constructed wetlands and their greenhouse gas emissions. Front. Environ. Sci. Eng. 3, 477–482. doi: 10.1007/s11783-009-0155-8
Liu, S., Xue, H., Wang, Y., Wang, Z., Feng, X., and Pyo, S.-H. (2022). Effects of bioelectricity generation processes on methane emission and bacterial community in wetland and carbon fate analysis. Bioresour. Bioprocess. 9:69. doi: 10.1186/s40643-022-00558-8
Liu, X., Zhang, K., Fan, L., Luo, H., Jiang, M., Anderson, B. C., et al. (2018). Intermittent micro-aeration control of methane emissions from an integrated vertical-flow constructed wetland during agricultural domestic wastewater treatment. Environ. Sci. Pollut. Res. 25, 24426–24444. doi: 10.1007/s11356-018-2226-5
López, D., Fuenzalida, D., Vera, I., Rojas, K., and Vidal, G. (2015). Relationship between the removal of organic matter and the production of methane in subsurface flow constructed wetlands designed for wastewater treatment. Ecol. Eng. 83, 296–304. doi: 10.1016/j.ecoleng.2015.06.037
López, D., Sepúlveda-Mardones, M., Ruiz-Tagle, N., Sossa, K., Uggetti, E., and Vidal, G. (2019). Potential methane production and molecular characterization of bacterial and archaeal communities in a horizontal subsurface flow constructed wetland under cold and warm seasons. Sci. Total Environ. 648, 1042–1051. doi: 10.1016/j.scitotenv.2018.08.186
Lu, Y., Fu, L., Lu, Y., Hugenholtz, F., and Ma, K. (2015). Effect of temperature on the structure and activity of a methanogenic archaeal community during rice straw decomposition. Soil Biol. Biochem. 81, 17–27. doi: 10.1016/j.soilbio.2014.10.031
Lu, L., Xing, D., and Ren, Z. J. (2015). Microbial community structure accompanied with electricity production in a constructed wetland plant microbial fuel cell. Bioresour. Technol. 195, 115–121. doi: 10.1016/j.biortech.2015.05.098
Maltais-Landry, G., Maranger, R., Brisson, J., and Chazarenc, F. (2009). Greenhouse gas production and efficiency of planted and artificially aerated constructed wetlands. Environ. Pollut. 157, 748–754. doi: 10.1016/j.envpol.2008.11.019
Malyan, S. K., Bhatia, A., Kumar, A., Gupta, D. K., Singh, R., Kumar, S. S., et al. (2016). Methane production, oxidation and mitigation: a mechanistic understanding and comprehensive evaluation of influencing factors. Sci. Total Environ. 572, 874–896. doi: 10.1016/j.scitotenv.2016.07.182
Mander, Ü., Dotro, G., Ebie, Y., Towprayoon, S., Chiemchaisri, C., Nogueira, S. F., et al. (2014). Greenhouse gas emission in constructed wetlands for wastewater treatment: a review. Ecol. Eng. 66, 19–35. doi: 10.1016/j.ecoleng.2013.12.006
Mander, Ü., Lõhmus, K., Teiter, S., Mauring, T., Nurk, K., and Augustin, J. (2008). Gaseous fluxes in the nitrogen and carbon budgets of subsurface flow constructed wetlands. Sci. Total Environ. 404, 343–353. doi: 10.1016/j.scitotenv.2008.03.014
Marton, J. M., Herbert, E. R., and Craft, C. B. (2012). Effects of salinity on denitrification and greenhouse gas production from laboratory-incubated tidal Forest soils. Wetlands 32, 347–357. doi: 10.1007/s13157-012-0270-3
Maucieri, C., Barbera, A. C., Vymazal, J., and Borin, M. (2017). A review on the main affecting factors of greenhouse gases emission in constructed wetlands. Agric. For. Meteorol. 236, 175–193. doi: 10.1016/j.agrformet.2017.01.006
Maucieri, C., Borin, M., and Barbera, A. C. (2014). Role of C3 plant species on carbon dioxide and methane emissions in Mediterranean constructed wetland. Ital. J. Agron. 9, 120–126. doi: 10.4081/ija.2014.601
Maucieri, C., Borin, M., Milani, M., Cirelli, G. L., and Barbera, A. C. (2019). Plant species effect on CO2 and CH4 emissions from pilot constructed wetlands in Mediterranean area. Ecol. Eng. 134, 112–117. doi: 10.1016/j.ecoleng.2019.04.019
McInerney, E., and Helton, A. M. (2016). The effects of soil moisture and emergent herbaceous vegetation on carbon emissions from constructed wetlands. Wetlands 36, 275–284. doi: 10.1007/s13157-016-0736-9
Nikhil, G. N., Krishna Chaitanya, D. N. S., Srikanth, S., Swamy, Y. V., and Venkata Mohan, S. (2018). Applied resistance for power generation and energy distribution in microbial fuel cells with rationale for maximum power point. Chem. Eng. J. 335, 267–274. doi: 10.1016/j.cej.2017.10.139
Niu, C., He, Z., Ge, Y., Chang, J., and Lu, Z. (2015). Effect of plant species richness on methane fluxes and associated microbial processes in wetland microcosms. Ecol. Eng. 84, 250–259. doi: 10.1016/j.ecoleng.2015.09.007
Nobu, M. K., Narihiro, T., Kuroda, K., Mei, R., and Liu, W.-T. (2016). Chasing the elusive Euryarchaeota class WSA2: genomes reveal a uniquely fastidious methyl-reducing methanogen. Int. Soc. Microb. Ecol. 10, 2478–2487. doi: 10.1038/ismej.2016.33
Nuamah, L. A., Li, Y., Pu, Y., Nwankwegu, A. S., Haikuo, Z., Norgbey, E., et al. (2020). Constructed wetlands, status, progress, and challenges. The need for critical operational reassessment for a cleaner productive ecosystem. J. Clean. Prod. 269:122340. doi: 10.1016/j.jclepro.2020.122340
O'Connor, F. M., Boucher, O., Gedney, N., Jones, C. D., Folberth, G. A., Coppell, R., et al. (2010). Possible role of wetlands, permafrost, and methane hydrates in the methane cycle under future climate change: a review. Rev. Geophys. 48, 1–33. doi: 10.1029/2010RG000326
Orr, H. B. B. K. S. P. T. (1992). Internal pressurization and convective gas flow in some emergent. Limnol. Oceanogr. 37, 1420–1433. doi: 10.4319/lo.1992.37.7.1420
Poffenbarger, H. J., Needelman, B. A., and Megonigal, J. P. (2011). Salinity influence on methane emissions from tidal marshes. Wetlands 31, 831–842. doi: 10.1007/s13157-011-0197-0
Ralf, C. (2007). Microbial ecology of methanogens and methanotrophs. Adv. Agron. 96, 1–63. doi: 10.1016/s0065-2113(07)96005-8
Shao, X., Zhao, L., Sheng, X., and Wu, M. (2020). Effects of influent salinity on water purification and greenhouse gas emissions in lab-scale constructed wetlands. Environ. Sci. Pollut. Res. 27, 21487–21496. doi: 10.1007/s11356-020-08497-7
Sheng, Q., Wang, L., and Wu, J. (2015). Vegetation alters the effects of salinity on greenhouse gas emissions and carbon sequestration in a newly created wetland. Ecol. Eng. 84, 542–550. doi: 10.1016/j.ecoleng.2015.09.047
Silvey, C., Jarecke, K. M., Hopfensperger, K., Loecke, T. D., and Burgin, A. J. (2019). Plant species and hydrology as controls on constructed wetland methane fluxes. Soil Sci. Soc. Am. J. 83, 848–855. doi: 10.2136/sssaj2018.11.0421
Sorokin, D. Y., Makarova, K. S., Abbas, B., Ferrer, M., Golyshin, P. N., Galinski, E. A., et al. (2017). Discovery of extremely halophilic, methyl-reducing euryarchaea provides insights into the evolutionary origin of methanogenesis. Nat. Microbiol. 2:17081. doi: 10.1038/nmicrobiol.2017.81
Søvik, A. K., Augustin, J., Heikkinen, K., Huttunen, J. T., Necki, J. M., Karjalainen, S. M., et al. (2006). Emission of the greenhouse gases nitrous oxide and methane from constructed wetlands in Europe. J. Environ. Qual. 35, 2360–2373. doi: 10.2134/jeq2006.0038
Sprenger, W. W., Hackstein, J. H. P., and Keltjens, J. T. (2007). The competitive success of Methanomicrococcus blatticola, a dominant methylotrophic methanogen in the cockroach hindgut, is supported by high substrate affinities and favorable thermodynamics. FEMS Microbiol. Ecol. 60, 266–275. doi: 10.1111/j.1574-6941.2007.00287.x
Ström, L., Lamppa, A., and Christensen, T. R. (2006). Greenhouse gas emissions from a constructed wetland in southern Sweden. Wetl. Ecol. Manag. 15, 43–50. doi: 10.1007/s11273-006-9010-x
Thangarajan, R., Bolan, N. S., Tian, G., Naidu, R., and Kunhikrishnan, A. (2013). Role of organic amendment application on greenhouse gas emission from soil. Sci. Total Environ. 465, 72–96. doi: 10.1016/j.scitotenv.2013.01.031
VanderZaag, A. C., Gordon, R. J., Burton, D. L., Jamieson, R. C., and Stratton, G. W. (2010). Greenhouse gas emissions from surface flow and subsurface flow constructed wetlands treating dairy wastewater. J. Environ. Qual. 39, 460–471. doi: 10.2134/jeq2009.0166
Vanwonterghem, I., Evans, P. N., Parks, D. H., Jensen, P. D., Woodcroft, B. J., Hugenholtz, P., et al. (2016). Methylotrophic methanogenesis discovered in the archaeal phylum Verstraetearchaeota. Nat. Microbiol. 1:16170. doi: 10.1038/nmicrobiol.2016.170
Wang, Y., Cai, Z., Sheng, S., Pan, F., Chen, F., and Fu, J. (2020). Comprehensive evaluation of substrate materials for contaminants removal in constructed wetlands. Sci. Total Environ. 701:134736. doi: 10.1016/j.scitotenv.2019.134736
Wang, J., Long, Y., Yu, G., Wang, G., Zhou, Z., Li, P., et al. (2022). A review on microorganisms in constructed wetlands for typical pollutant removal: species, function, and diversity. Front. Microbiol. 13:845725. doi: 10.3389/fmicb.2022.845725
Wang, X., Tian, Y., Liu, H., Zhao, X., and Peng, S. (2019). The influence of incorporating microbial fuel cells on greenhouse gas emissions from constructed wetlands. Sci. Total Environ. 656, 270–279. doi: 10.1016/j.scitotenv.2018.11.328
Wang, H., Xu, J., and Sheng, L. (2020). Purification mechanism of sewage from constructed wetlands with zeolite substrates: a review. J. Clean. Prod. 258:120760. doi: 10.1016/j.jclepro.2020.120760
Wang, G., Yu, G., Chi, T., Li, Y., Zhang, Y., Wang, J., et al. (2023). Insights into the enhanced effect of biochar on cadmium removal in vertical flow constructed wetlands. J. Hazard. Mater. 443:130148. doi: 10.1016/j.jhazmat.2022.130148
Wegener, G., Krukenberg, V., Riedel, D., Tegetmeyer, H. E., and Boetius, A. (2015). Intercellular wiring enables electron transfer between methanotrophic archaea and bacteria. Nature 526, 587–590. doi: 10.1038/nature15733
Xiao, K., Guo, C., Maspolim, Y., Zhou, Y., and Ng, W. J. (2016). The role of methanogens in acetic acid production under different salinity conditions. Chemosphere 161, 53–60. doi: 10.1016/j.chemosphere.2016.06.112
Xu, G., Li, Y., Hou, W., Wang, S., and Kong, F. (2021). Effects of substrate type on enhancing pollutant removal performance and reducing greenhouse gas emission in vertical subsurface flow constructed wetland. J. Environ. Manag. 280:111674. doi: 10.1016/j.jenvman.2020.111674
Xu, G., Li, Y., Wang, S., Kong, F., and Yu, Z. (2019). An overview of methane emissions in constructed wetlands: how do plants influence methane flux during the wastewater treatment? J. Freshw. Ecol. 34, 333–350. doi: 10.1080/02705060.2019.1588176
Xu, H., Song, H.-L., Singh, R. P., Yang, Y.-L., Xu, J.-Y., and Yang, X.-L. (2021). Simultaneous reduction of antibiotics leakage and methane emission from constructed wetland by integrating microbial fuel cell. Bioresour. Technol. 320:124285. doi: 10.1016/j.actatropica.2020.124285
Yan, C., Zhang, H., Li, B., Wang, D., Zhao, Y., and Zheng, Z. (2012). Effects of influent C/N ratios on CO2 and CH4 emissions from vertical subsurface flow constructed wetlands treating synthetic municipal wastewater. J. Hazard. Mater. 203-204, 188–194. doi: 10.1016/j.jhazmat.2011.12.002
Yu, G., Li, P., Wang, G., Wang, J., Zhang, Y., Wang, S., et al. (2021). A review on the removal of heavy metals and metalloids by constructed wetlands: bibliometric, removal pathways, and key factors. World J. Microbiol. Biotechnol. 37:157. doi: 10.1007/s11274-021-03123-1
Yu, G., Wang, G., Chi, T., Du, C., Wang, J., Li, P., et al. (2022). Enhanced removal of heavy metals and metalloids by constructed wetlands: a review of approaches and mechanisms. Sci. Total Environ. 821:153516. doi: 10.1016/j.scitotenv.2022.153516
Yu, G., Wang, G., Li, J., Chi, T., Wang, S., Peng, H., et al. (2020). Enhanced Cd2+ and Zn2+ removal from heavy metal wastewater in constructed wetlands with resistant microorganisms. Bioresour. Technol. 316:123898. doi: 10.1016/j.biortech.2020.123898
Zhang, Q., Li, T.-T., Zhang, Q., Wang, G.-C., Yu, L.-J., Guo, B., et al. (2020). Accuracy analysis in CH4MODwetland in the simulation of CH4 emissions from Chinese wetlands. Adv. Clim. Chang. Res. 11, 52–59. doi: 10.1016/j.accre.2020.06.003
Zhang, K., Liu, Y., Chen, Q., Luo, H., Zhu, Z., Chen, W., et al. (2018a). Effect of submerged plant species on CH4 flux and methanogenic community dynamics in a full-scale constructed wetland. Ecol. Eng. 115, 96–104. doi: 10.1016/j.ecoleng.2018.02.025
Zhang, K., Luo, H., Zhu, Z., Chen, W., Chen, J., and Mo, Y. (2018b). CH4 flux and methanogen community dynamics from five common emergent vegetations in a full-scale constructed wetland. Environ. Sci. Pollut. Res. 25, 26433–26445. doi: 10.1007/s11356-018-2692-9
Zhang, C.-B., Sun, H.-Y., Ge, Y., Gu, B.-J., Wang, H., and Chang, J. (2012). Plant species richness enhanced the methane emission in experimental microcosms. Atmos. Environ. 62, 180–183. doi: 10.1016/j.atmosenv.2012.08.034
Zhang, K., Wang, J., Liu, X., Fu, X., Luo, H., Li, M., et al. (2021a). Methane emissions and methanogenic community investigation from constructed wetlands in Chengdu City. Urban Clim. 39:100956. doi: 10.1016/j.uclim.2021.100956
Zhang, K., Wu, X., Chen, J., Wang, W., Luo, H., Chen, W., et al. (2021b). The role and related microbial processes of Mn-dependent anaerobic methane oxidation in reducing methane emissions from constructed wetland-microbial fuel cell. J. Environ. Manag. 294:112935. doi: 10.1016/j.jenvman.2021.112935
Zhang, J., Wu, H., Hu, Z., Liang, S., and Fan, J. (2014). Examination of oxygen release from plants in constructed wetlands in different stages of wetland plant life cycle. Environ. Sci. Pollut. Res. Int. 21, 9709–9716. doi: 10.1007/s11356-014-2905-9
Zhang, K., Wu, X., Luo, H., Li, X., Chen, W., Chen, J., et al. (2020). CH4 control and associated microbial process from constructed wetland (CW) by microbial fuel cells (MFC). J. Environ. Manag. 260:110071. doi: 10.1016/j.jenvman.2020.110071
Zhang, K., Wu, X., Wang, W., Chen, J., Chen, J., and Luo, H. (2021c). Roles of external circuit and rhizosphere location in CH4 emission control in sequencing batch flow constructed wetland-microbial fuel cell. J. Environ. Chem. Eng. 9:106583. doi: 10.1016/j.jece.2021.106583
Zhang, K., Wu, X., Wang, W., Chen, J., Luo, H., Chen, W., et al. (2022). Anaerobic oxidation of methane (AOM) driven by multiple electron acceptors in constructed wetland and the related mechanisms of carbon, nitrogen, sulfur cycles. Chem. Eng. J. 433:133663. doi: 10.1016/j.cej.2021.133663
Zhang, K., Wu, X., Wang, W., Luo, H., Chen, W., and Chen, J. (2021d). Effects of the bioelectrochemical technique on methane emission and energy recovery in constructed wetlands (CWs) and related biological mechanisms. Environ. Technol. 1–12. doi: 10.1080/09593330.2021.1976846
Zhang, K., Wu, X., Wang, W., Luo, H., Chen, W., Ma, D., et al. (2021e). Effects of plant location on methane emission, bioelectricity generation, pollutant removal and related biological processes in microbial fuel cell constructed wetland. J. Water Process Eng. 43:102283. doi: 10.1016/j.jwpe.2021.102283
Zhao, Z., Chang, J., Han, W., Wang, M., Ma, D., Du, Y., et al. (2016). Effects of plant diversity and sand particle size on methane emission and nitrogen removal in microcosms of constructed wetlands. Ecol. Eng. 95, 390–398. doi: 10.1016/j.ecoleng.2016.06.047
Zhao, Z., Hao, Q., Ma, R., Chen, X., Xiong, Y., Hu, J., et al. (2022). Ferric-carbon micro-electrolysis and zeolite reduce CH4 and N2O emissions from the aerated constructed wetland. J. Clean. Prod. 342:130946. doi: 10.1016/j.jclepro.2022.130946
Zhao, Y., Zhang, Y., Ge, Z., Hu, C., and Zhang, H. (2014). Effects of influent C/N ratios on wastewater nutrient removal and simultaneous greenhouse gas emission from the combinations of vertical subsurface flow constructed wetlands and earthworm eco-filters for treating synthetic wastewater. Environ. Sci. Process. Impacts 16, 567–575. doi: 10.1039/c3em00655g
Zhou, S., Wang, C., Liu, C. E., Sun, H., Zhang, J., Zhang, X., et al. (2020). Nutrient removal, methane and nitrous oxide emissions in a hybrid constructed wetland treating anaerobic digestate. Sci. Total Environ. 733:138338. doi: 10.1016/j.scitotenv.2020.138338
Zhu, N., An, P., Krishnakumar, B., Zhao, L., Sun, L., Mizuochi, M., et al. (2007). Effect of plant harvest on methane emission from two constructed wetlands designed for the treatment of wastewater. J. Environ. Manag. 85, 936–943. doi: 10.1016/j.jenvman.2006.11.004
Zhu, J., Wang, Q., Yuan, M., Tan, G.-Y. A., Sun, F., Wang, C., et al. (2016). Microbiology and potential applications of aerobic methane oxidation coupled to denitrification (AME-D) process: a review. Water Res. 90, 203–215. doi: 10.1016/j.watres.2015.12.020
Keywords: constructed wetland, methane reduction, greenhouse gas, methanogen, methanotrophy main topic
Citation: Yu G, Chen J, Wang G, Chen H, Huang J, Li Y, Wang W, Song F, Ma Y, Wang Q, Wang M, Ling T, Shu Z, Sun J and Yu Z (2023) Recent advances in constructed wetlands methane reduction: Mechanisms and methods. Front. Microbiol. 14:1106332. doi: 10.3389/fmicb.2023.1106332
Edited by:
Tian Li, Nankai University, ChinaReviewed by:
Huijun He, Guilin University of Technology, ChinaJiachao Zhang, Hunan Agricultural University, China
Wenjing Chen, Chengdu University of Information Technology, China
Copyright © 2023 Yu, Chen, Wang, Chen, Huang, Li, Wang, Song, Ma, Wang, Wang, Ling, Shu, Sun and Yu. This is an open-access article distributed under the terms of the Creative Commons Attribution License (CC BY). The use, distribution or reproduction in other forums is permitted, provided the original author(s) and the copyright owner(s) are credited and that the original publication in this journal is cited, in accordance with accepted academic practice. No use, distribution or reproduction is permitted which does not comply with these terms.
*Correspondence: Wenming Wang, ✉ dy5tLndhbmdAMTI2LmNvbQ==