- Instituto de Productos Lacteos de Asturias (IPLA), CSIC, Villaviciosa, Spain
Bacteriophages are a nuisance in the production of fermented dairy products driven by starter bacteria and strategies to reduce the risk of phage infection are permanently sought. Bearing in mind that the bacterial cell wall plays a pivotal role in host recognition and lysis, our goal was to elucidate to which extent modifications in the cell wall may alter endolysin activity and influence the outcome of phage infection in Lactococcus. Three lactococcal endolysins with distinct catalytic domains (CHAP, amidase and lysozyme) from phages 1,358, p2 and c2 respectively, were purified and their exolytic activity was tested against lactococcal mutants either overexpressing or lacking genes involved in the cell envelope stress (CES) response or in modifying peptidoglycan (PG) composition. After recombinant production in E. coli, Lys1358 (CHAP) and LysC2 (muramidase) were able to lyse lactococcal cells in turbidity reduction assays, but no activity of LysP2 was detected. The degree of PG acetylation, namely C6-O-acetylation and de-N-acetylation influenced the exolytic activity, being LysC2 more active against cells depleted of the PG deacetylase PgdA and the O-acetyl transferase OatA. On the contrary, both endolysins showed reduced activity on cells with an induced CES response. By measuring several growth parameters of phage c2 on these lactococcal mutants (lytic score, efficiency of plaquing, plaque size and one-step curves), a direct link between the exolytic activity of its endolysin and phage performance could not be stablished.
Introduction
Bacteriophages or phages are viruses that infect bacteria, they are ubiquitous and can be found in every ecosystem where bacteria exist. If present in the dairy factory environment, phage infection of lactic acid bacteria (LAB), specifically that of Lactococcus lactis and Lactococcus cremoris used in starter cultures, may interfere with bacterial growth and led to failures in milk fermentation. In order to reduce the risk, several measures have been implemented including the search for phage-resistant starter bacteria (Marcó et al., 2012).
The bacterial cell wall (CW) plays a pivotal role in host:phage interactions in LAB and its composition and architecture may determine resistance to phage infection (reviewed by Chapot-Chartier and Kulakauskas, 2014; Mahony et al., 2017; Martínez et al., 2020). Indeed, three of the infection steps (adsorption, DNA delivery and host lysis), involve CW components. Surface structures, e.g., cell wall polysaccharides (CWPS), membrane proteins and (lipo-)teichoic acids, act as discriminating recognition sites for phages and are prone to be modified and confer phage resistance (Viscardi et al., 2003; Chapot-Chartier and Kulakauskas, 2014; Bertozzi Silva et al., 2016). Once positioned, phages must eject their DNA inside the bacterial cell with the help of virion-associated enzymes that degrade CW polymers locally, allowing tail penetration and DNA passage without lysing the cell (Moak and Molineux, 2004; Latka et al., 2017). Later in the lytic cycle after building new phage particles, host lysis relies on the activity of phage-encoded endolysins that hydrolyze the peptidoglycan (PG), the main CW component in Eubacteria, made of glycan chains of N-acetyl-muramic acid (NAM) and N-acetyl-glucosamine (NAG) cross-linked through short peptide chains.
Endolysins from phages infecting Gram-positive bacteria often have a modular structure that includes a N-terminal domain, that specifies the catalytic activity of the enzyme, and a CW binding domain involved in substrate binding (Schmelcher et al., 2012; Oliveira et al., 2013). A recent insight into the diversity of lactococcal phage endolysins have uncovered 11 types of these modular enzymes (Oechslin et al., 2022). Identified catalytic domains include muramidases (phage_lysozyme, Glycohydro_25), N-acetyl-muramyl-L-Ala-amidases (Amidase_2 domain), γ-D-Glu-L-Lys-endopeptidases (Amidase_5) and CHAP, with both cysteine-histidine-dependent amidohydrolase and/or endopeptidase activity (Oliveira et al., 2013).
As described for host autolysins, resistance to PG-degrading enzymes may occur through chemical modifications of the CW components (Grishin et al., 2020). In Lactococcus, PG modifications such as NAG de-N-acetylation by the deacetylase PgdA and amidation of D-Asp in the PG cross bridge protects PG from hydrolysis by the major autolysin AcmA (Meyrand et al., 2007; Veiga et al., 2009), while changes in the composition of the lipoteichoic acid decreased substrate binding (Steen et al., 2008). However, resistance to the exolytic activity of phage endolysins is scarcely documented and even less is known if there are consequences for phage growth.
Exposure to harsh conditions or CW damaging agents may also change, at least, transiently, the structure of major CW components through the activation of signal transduction systems, mostly two-component systems (TCSs), that activate defense mechanisms, collectively known as the cell envelope stress (CES) response (Jordan et al., 2008). In Lactococcus cremoris MG1363, inhibition of CW biosynthesis by the lactococcal bacteriocin Lcn972 and PG hydrolysis by lysozyme are known to activate the TCS CesSR (Martínez et al., 2007; Veiga et al., 2007). Of interest for this work, the CES response has been shown to be also activated upon bacteriophage infection (Fallico et al., 2011). A main effector of CesSR is SpxB which, through binding to the RNA polymerase RpoA, drives transcription of oatA, encoding the O-acetyl transferase OatA that leads to O-acetylation at the C6-hydroxyl of N-acetylmuramoyl residues (Veiga et al., 2007). This is a widespread PG modification that sterically inhibits lysozyme but also the exolytic activity of the L. cremoris prophage TP712 endolysin (Escobedo et al., 2019).
Hence, aware of the importance of the bacterial CW for phage infection as well as the regulatory mechanisms dedicated to preserve its integrity, along with its multiple modifications, the aim of this work was to identify modifications of the lactococcal CW that could alter the exolytic activity of phage endolysins. As described above, these modifications could be either caused by mutations in genes coding for PG modifying enzymes or in those involved in the lactococcal CES response. The consequences for phage infection were also evaluated using the virulent phage c2, in order to decipher to which extent a thwarted endolysin activity may or may not restrict phage growth. The results show that while the CES response and the degree of PG-acetylation may indeed compromise or enhanced the exolytic activity of two endolysins, the impact on phage growth is negligible.
Materials and methods
Bacterial strains, bacteriophages, and growth conditions
Bacterial strains used in this study are listed in Table 1. L. cremoris strains were grown in M17 medium (Formedium, UK) supplemented with 0.5% glucose (GM17) at 30°C. Chloramphenicol (5 μg/ml) and erythromycin (2.5 μg/ml) were added as needed. Escherichia coli was grown in Luria Bertani (LB) or Terrific broth (TB; Sambrook et al., 1989) at 37°C with shaking (250 rpm) in the presence of 30 μg/ml kanamycin if needed.
To propagate phage c2, L. cremoris MG1363 was grown in GM17 with 10 mM Ca(NO3)2 and 10 mM MgS04 until an OD600 of 0.4 was reached and then infected at a MOI of 0.01. Incubation proceeded until lysis had occurred (≈6 h). The culture was then centrifugated, the lysate filtered through a polyethersulfone filter (0.2 μm) and stored at 4°C. Plaque-forming units (pfu/ml) were determined by the double-layer agar assay. Inoculating the top agar (0.7%) with 100 μl of overnight cultures of the bacterial host and appropriate phage dilutions prepared in SM buffer (20 mg/ml Tris–HCL, 10 mg/ml MgSO4, 10 mg/ml CaCl2, 100 mg/ml NaCl, pH 7.5).
Expression and purification of lactococcal endolysins
The genes encoding the lactococcal endolysins LysP2, LysC2 and Lys1358 (Table 2) were optimized for E. coli codon usage by Twist DNA codon optimization technology (Twist Bioscience, San Francisco, CA, USA). The DNA fragments were then cloned into the NdeI and XhoI restriction sites of the pET29b + vector, which introduces a C-terminal His6 tag to yield pETLysP2, pETLysC2 and pETLys1358 (Table 1). Escherichia coli BL21 (DE3) harboring these plasmids were grown in 0.5 L of LB (pETLysP2, pETLys1358) or TB media (pETLysC2) with 30 μg/ml kanamycin at 37°C to an OD600 of 0.5. Gene expression was induced by addition of 1 mM IPTG. Following 18 h of incubation at 12°C (pETLysC2) or 16°C (pETLysP2, pETLys1358) bacterial cells were collected and resuspended in lysis buffer (50 mM NaH2PO4, 300 mM NaCl, 10 mM imidazole, pH 8) supplemented with 1 mg/ml of lysozyme (Merck, Germany) and 10 μg/ml of DNAse (Sigma, Spain). The cell suspension was then disrupted by sonication, and after centrifugation (20,000× g, 30 min), the supernatant was passed through a Ni-NTA superflow column (Qiagen, Germany) and eluted in a 50 mM NaH2PO4, 300 mM NaCl, 250 mM imidazole (pH 8) buffer according to the manufacturer’s instructions. Due to low yields, LysC2 was further concentrated and the buffer replaced with 50 mM sodium phosphate buffer, 150 mM NaCl (pH 7.5) using an Amicon Ultra-15 3-kDa-molecular-weight-cutoff (MWCO) filter unit (Merck KGaA, Germany). The proteins were stored at −20°C after the addition of glycerol at 25%. Protein concentration was determined by the Bradford assay (Bio-Rad Laboratories, Hercules, CA, USA) using bovine serum albumin as standard.
SDS-PAGE and Zymograms
Sodium dodecyl sulfate (SDS)-PAGE was performed in a BioRad Mini-Protean gel apparatus (BioRad) using 10% (w/v) polyacrylamide separating gels. As previously described for zymograms (Lepeuple et al., 1998), the polyacrylamide gels contained 0.2% (w/v) Micrococcus luteus ATCC 4698 (Sigma) or 0.4% (w/v) L. cremoris NZ9000 autoclaved cells. Gels were subsequently washed for 30 min in deionized H2O at room temperature and incubated overnight at 37°C in 5 mM Tris–HCl, pH 7.5, supplemented with 0.1% Triton X-100 as renaturing buffer. Zymograms were photographed without further staining and Precision Plus Protein™ All Blue Standards (BioRad) was used as a molecular weight marker.
Turbidity reduction assays
Quantitative detection of endolysin activity was performed via turbidity reduction assays. Lactococcal cells used as substrate were grown in 30 ml GM17 until an OD600 0.5. Then, they were centrifuged at 3,500× g for 15 min at 4°C, washed with 30 ml 50 mM sodium phosphate buffer, pH 7.5, adjusted to a final OD600 1.5 in the same buffer, aliquoted and frozen at −20°C. In a 96-well plate, 100 μl of the cell suspension was mixed with one volume of LysC2 and Lys1358 to reach a final concentration of 4.5 and 0.5 μM, respectively. OD600 was measured in a microtiter plate reader (Tecan Trading AG) at 30°C every 5 min for 1 h. Activity was defined as the decrease of mOD per minute (mOD/min). Two to seven replicates were carried out.
Phage infection assays
Lysis-in-broth assays were performed by infecting early exponentially growing cultures at OD600 of 0.2 with c2 in the presence of 10 mM Ca(NO3)2 and 10 mM MgS04 at a MOI of 0.2. Lysis was monitored in a 96-well plate microtiter plate reader at 10 min intervals for 290 min post-infection. All assays were performed in triplicate. To analyze the results, the area under the curve (AUC) of the treated cultures and the uninfected control for the first 140 min was calculated as previously described (Xie et al., 2018). The lytic score was defined as 1-AUC treated/AUC control. For comparisons, the lytic scores on the wild type strains were taken as 100%. The growth rate of the non-infected cultures (μ, h−1) was calculated by linear regression of Ln(OD) plotted against time.
The efficiency of plaquing (EOP) of c2 on the lactococcal mutants was defined as the ratio between the average number of plaques on the mutant strain by the average number of plaques of the wild type (MG1363 or NZ9000). EOP determination was carried out in four independent experiments. The diameter of five lysis plaques from each biological replicate (n = 20) was measured with a digital caliper implemented in ImageJ (https://imagej.nih.gov/ij/).
Phage adsorption
Adsorption of phage to host cells was performed as described (Madera et al., 2003). Phage c2 was added at a MOI of 0.001 to stationary-phase host cultures diluted to an OD600 of 0.8 in GM17 supplemented with 10 mM of Ca(NO3)2 and 10 mM MgSO4. Following a 10 min incubation at room temperature, the phage-host mixture was centrifuged for 5 min, and phage counts in the supernatant determined by standard double-layer agar assays. A sample without cells was equally treated to determine the initial phage titer. The percentage adsorption was calculated as (1-residual phage titer/initial phage titer) × 100. Experiments were carried out with two to five independent lactococcal cultures.
One-step growth curves
One-step growth curves were performed as previously described (Moineau et al., 1993), with some modifications. Briefly, 2 ml of log-phase cells (OD600 = 0.8) of each host was centrifuged at 8,000× g for 5 min at 4°C. The pellet was resuspended in 900 μl of GM17 with 10 mM of Ca(N03)2/10 mM of MgSO4, and 100 μl of phage solution were added to achieve a final MOI of 0.01. The mixture was left at room temperature for 5 min and then washed twice to remove unabsorbed phages. The mixture was diluted 104-fold and incubated at 30°C. Samples were withdrawn periodically over 50 min, diluted, and spotted on L. cremoris MG1363 lawn for phage counts. The burst size (pfu per infected cell) was calculated as phage titer at the end of the one-step growth curve minus the initial titer and divided by the initial titer. The latent period was defined at the starting of the exponential phase and the burst time as the time invested to complete one cycle (Chmielewska-Jeznach et al., 2020).
Statistical analyses
A two-tailed Student t-test as implemented in Microsoft Excel 2019 was applied for comparison. A value of p threshold of 0.05 was set for significance.
Results
Recombinant production of lactococcal phage endolysins
The endolysins LysP2, LysC2 and Lys1358 with different catalytic domains (Table 2) were produced as C-terminally His-tagged proteins. The induction conditions, namely the growth medium and the temperature of incubation, were optimized for each endolysin. LysP2 and Lys1358 were successfully produced in the soluble fraction in LB at 16°C, yielding 3 and 16.5 mg per 0.5 L of induced E. coli cultures after purification, respectively. However, under these inducing conditions, LysC2 was insoluble but purification could be achieved after induction in TB and at lower T (12°C), reaching up to 3.3 mg per 0.5 L of induced cultures.
Protein bands of the expected sizes were detected in 10% SDS-PAGE gels (Figure 1A). In addition, their PG hydrolytic activity was also revealed by zymography. LysC2 and Lys1358 produced clear lytic bands in zymograms prepared with L. cremoris NZ9000 (Figure 1B) and Micrococcus luteus cells (Figure 1C), whereas for LysP2 we were unable to detect any lytic activity regardless of the substrate cells. Because the renaturing conditions, e.g., pH and cations, may be critical to detect the bacteriolytic activity (Lepeuple et al., 1998), other renaturation buffers such as 50 mM MES (pH 6.0) and 3 M sodium acetate (pH 5.2) with or without 10 mM CaCl2, were tested but failed to reveal LysP2 activity. Hence, the following experiments were carried out with LysC2 and Lys1358.
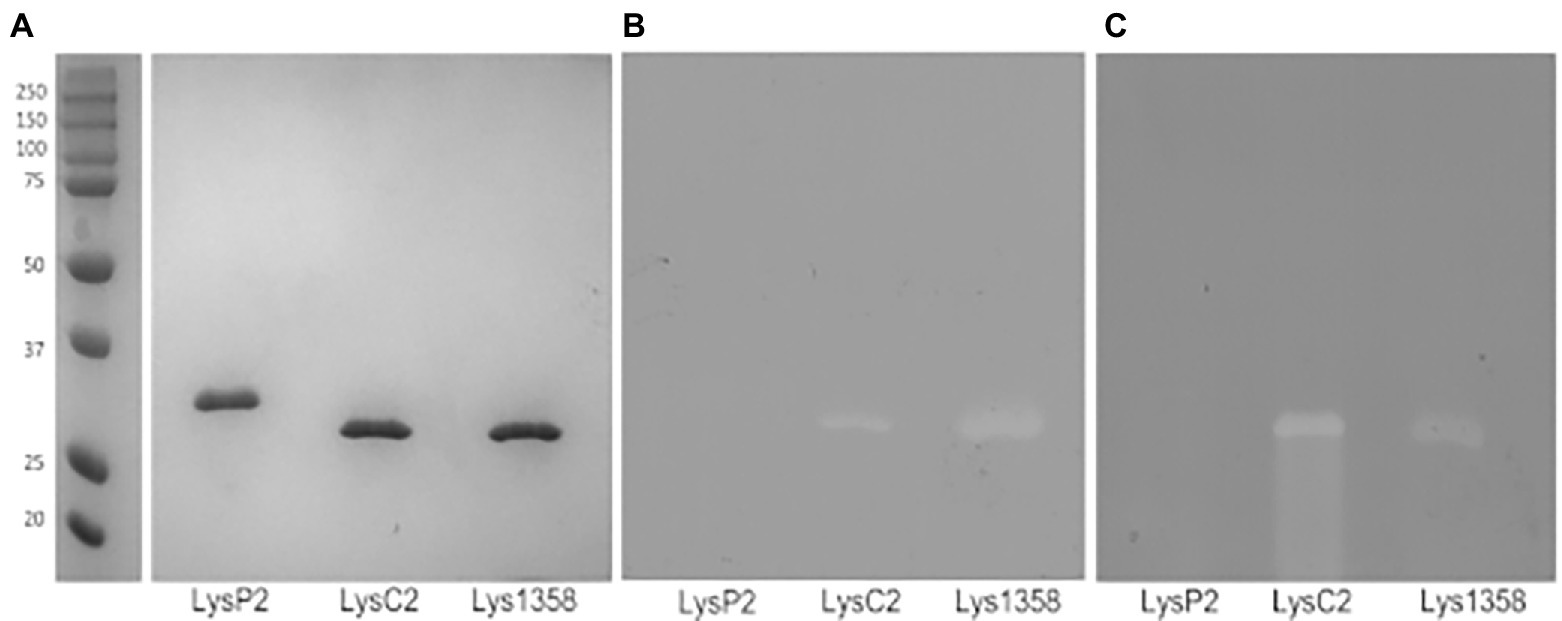
Figure 1. SDS-PAGE analysis of purified His-tagged LysP2, LysC2 and Lys1358 proteins (A) and zymograms prepared with Lactococcus cremoris NZ9000 (B) and Micrococcus luteus cells (C). Precision Plus Protein™ All Blue Standards were used as a marker (lane 1).
Exolytic activity of Lys1358 and LysC2 endolysins on CES and PG lactococcal mutants
To evaluate the impact on the exolytic activity of modifications in the PG or an altered CES response, two sets of available mutants were collected in either L. cremoris MG1363 or L. cremoris NZ9000 background (see Table 1). For clarity, plus and minus signs stand for mutants overexpressing or lacking a particular gene(s). So-called CES mutants were those overexpressing genes involved in the CES response including the TCS genes cesSR (cesSR+) or its main effector spxB (spxB+). The other group, PG mutants, comprised those in genes that code for the PG modifying enzymes N-acetylglucosamine deacetylase PgdA and the O-acetyl transferase OatA. An overview of the CES response and the changes in the PG composition introduced by the different enzymes is shown in Figure 2. In general, these mutants embody a different degree of PG acetylation of both NAM and NAG residues.
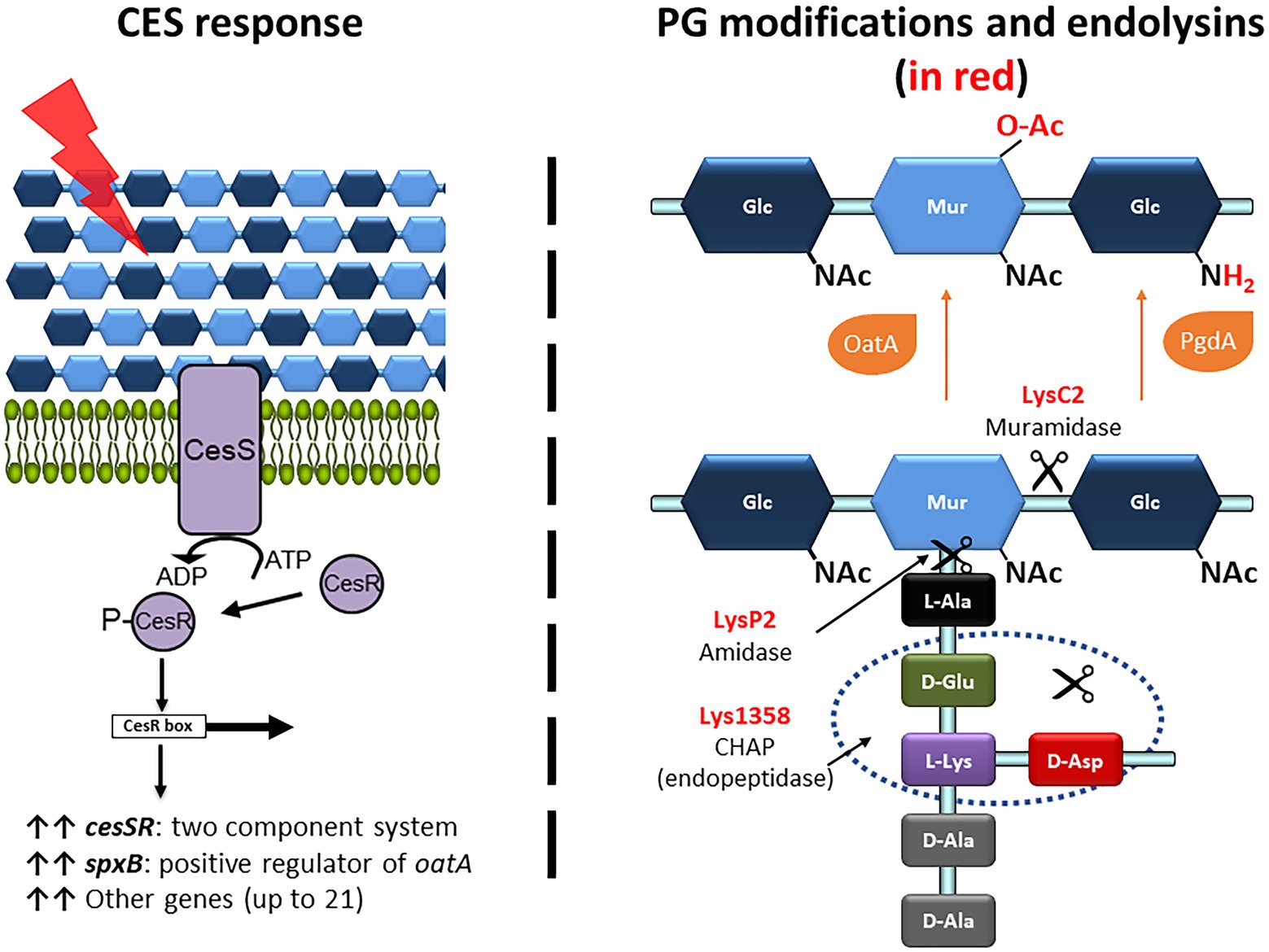
Figure 2. Overview of the CES response, the PG modifications and the PG bonds targeted by the lactococcal phage endolysins used in this work. (Right panel) The CES response is triggered upon damage (red ray) of the cell envelope that is sensed by the two-component system CesSR (purple proteins). The phosphorylated response regulator CesR binds to the CesR box and induces transcription of several genes, including cesSR and spxB (upright black arrows). SpxB, through binding to the RNA polymerase RpoA, drives transcription of oatA (not shown). (Left panel) The PG modifications of the N-acetyl-muramic acid (NAM) and N-acetyl-glucosamine (NAG) are shown in red. C6-O-acetylation and de-N-acetylation are mediated by OatA and PgdA, respectively (orange proteins). The bonds hydrolyzed by the endolysins LysC2, LysP2 and Lys1358, according to their conserved catalytic domains, are pointed by the scissors.
The exolytic activity of Lys1358 and LysC2 was tested in turbidity reduction assays, following the decrease in the optical density (OD600) of cell suspensions. As shown in Figure 3, the activity of Lys1358 (CHAP catalytic domain) at 0.5 μM was mostly compromised against L. cremoris CES mutants cesSR+ and spxB+ where the lytic activity was only 27 and 13% of that on wild type cells, respectively (Figure 3A). Lys1358 activity on the PG mutants (pgdA-, pgdA+ and oatA-) was also diminished, albeit to a lesser extent, and no differences were observed when the substrate cells exhibit opposite degrees of NAG de-acetylation (pgdA+/pgdA-). Hence, Lys1358 appears to be more sensitive to an activated CES response and to a higher degree of NAM O-acetylation.
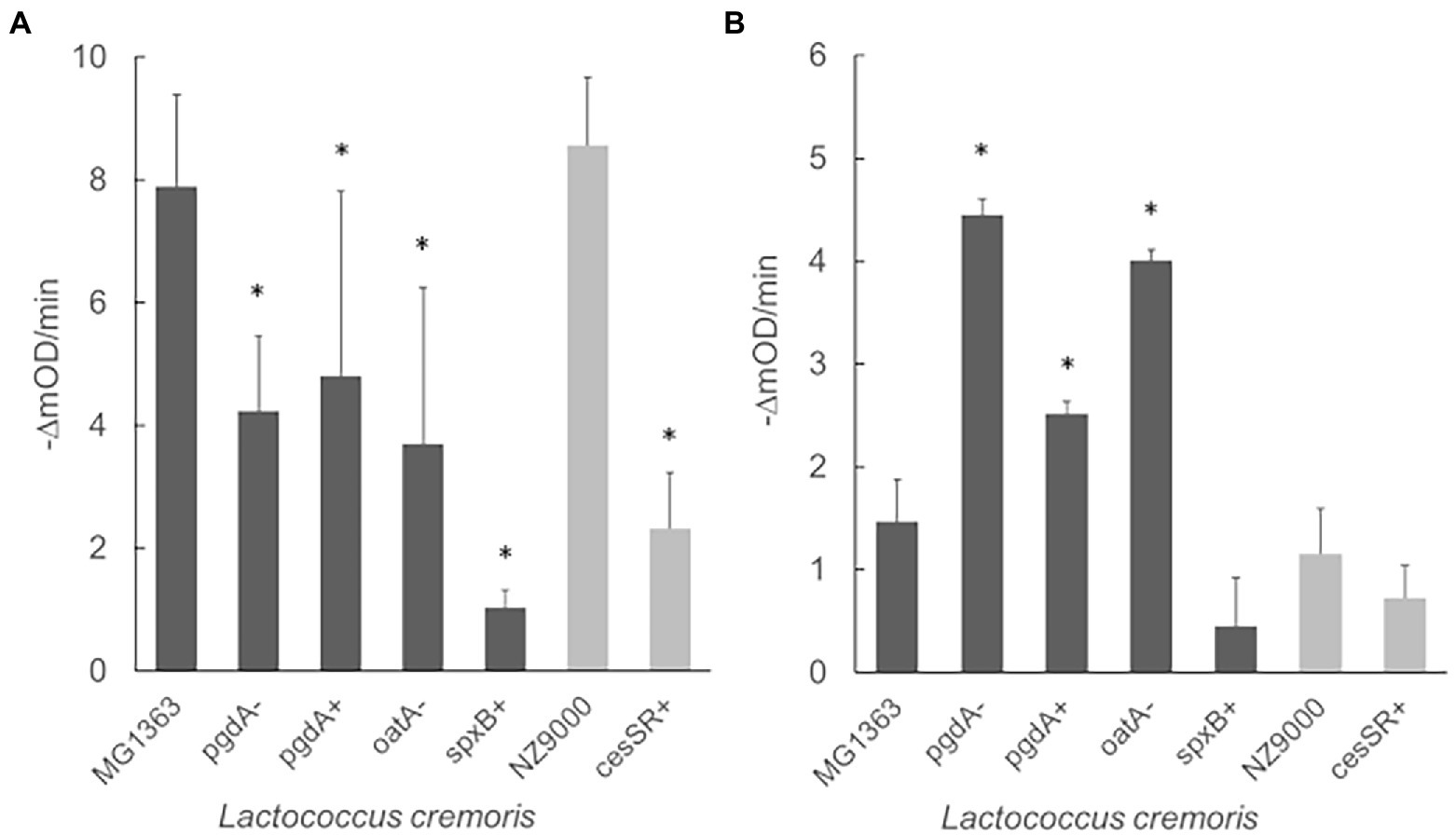
Figure 3. Exolytic activity of Lys1358 (A) and LysC2 (B) against CES and PG lactococcal mutants as determined by turbidity reduction assays. Purified Lys1358 (0.5 μM) and LysC2 (4.5 μM) were incubated with frozen cells and the decrease in OD600 (expressed in mili OD units, mOD) was monitored (-ΔmOD/min). Dark grey columns: L. cremoris MG1363 and derived mutants. Light grey columns: L. cremoris NZ9000 and derived mutants. *, Significantly different (p < 0.05) compared to the wild type L. cremoris MG1363 and NZ9000 cells.
LysC2 (lysozyme catalytic domain) turned out to be less active against wild type cells than Lys1358 and cell lysis could only be observed at 4.5 μM, the highest concentration possible in our assay. Despite of this, differences in its exolytic activity on PG and CES mutants was noticed (Figure 3B). Contrary to Lys1358, LysC2 showed enhanced lytic activity on the PG mutants, mainly pgdA- and oatA-, increasing up to twofold, while on pgdA+, the activity was reduced compared to pgdA-. LysC2 was also less active against the CES mutants, although the difference was not statistically significant (p > 0.05). Thereby, LysC2 is more at the mercy of the degree of PG acetylation, in line with its muramidase specificity.
Phage growth parameters on CES and PG lactococcal mutants
The results described above showed that the exolytic activity of phage endolysins may be either reduced or enhanced as function of the CES response and PG modifications. Hereinafter, we conducted experiments to evaluate their impact on several phage growth parameters. Since phage 1358 does not infect the reference strains L. cremoris MG1363 and NZ9000, the experiments were carried out with the virulent phage c2.
Phage infection experiments
The ability of phage c2 to propagate on the different mutants was assessed both in broth and solid medium. In these and the following experiments, a mutant devoid of the TCS CesSR (cesSR-) (Pinto et al., 2011) was also included. This mutant would be unable to turn on the TCS upon phage infection and would help us to fully appreciate the role of the CES response in phage growth.
To compare phage infection in broth, exponentially growing cells were challenged with phage c2 at a multiplicity of infection (MOI) of 0.2, and growth was followed until lysis had occurred, roughly up to 140 min post-infection. Following the method proposed by Xie et al. (2018), growth curves were integrated to determine the area under the curve and transformed into a single value, representing the differences in growth between the infected and the non-infected cultures. The lower the score, the poorer the performance of the phage on a particular mutant. This value (lytic score, Table 3) was normalized according to the reference strains L. cremoris MG1363 and NZ9000 to compare the lytic activity of phage c2 on the lactococcal mutants. The lytic scores were significantly reduced by half (p < 0.05) on spxB+ and by approximately 20% in cesSR+ and pgdA+. Noteworthy, the lytic score was restored on cesSR-, while there were no significant differences on phage performance on pgdA− and oatA− mutants (Table 3). Worth mentioning is that lower lytic scores were recorded in mutants that grow slower than their wild type counterparts. Therefore, the lower phage performance could be attributed, at least in part, to the physiology of the host rather than to their CW composition and its impact on the exolytic activity of LysC2.
The ability of phage c2 to propagate on the CES and PG mutants was further confirmed on solid medium. Phage c2 formed plaques on all the mutants, although the efficiency of plaquing (EOP) was again slightly lower on cesSR+ (0.81, p < 0.05) and spxB+ (0.85, p < 0.05; Table 3). The two CES mutants cesSR+ and cesSR- showed opposite trends, reinforcing the suggested defense role of this TCS against phage infection (Fallico et al., 2011). Remarkably, the plaque size of phage c2 varied depending on the mutants (Figure 4). The largest plaques were formed on those mutants susceptible to lysozyme (pgdA− and oatA−, see Table 1) with increments of 100 and 60%, respectively, compare to the wild type cells. Larger plaques were also noted on cesSR+ and pgdA+, although to a lesser extent, while the smallest c2 plaques were on spxB+, roughly 20% smaller than on the wild type host.
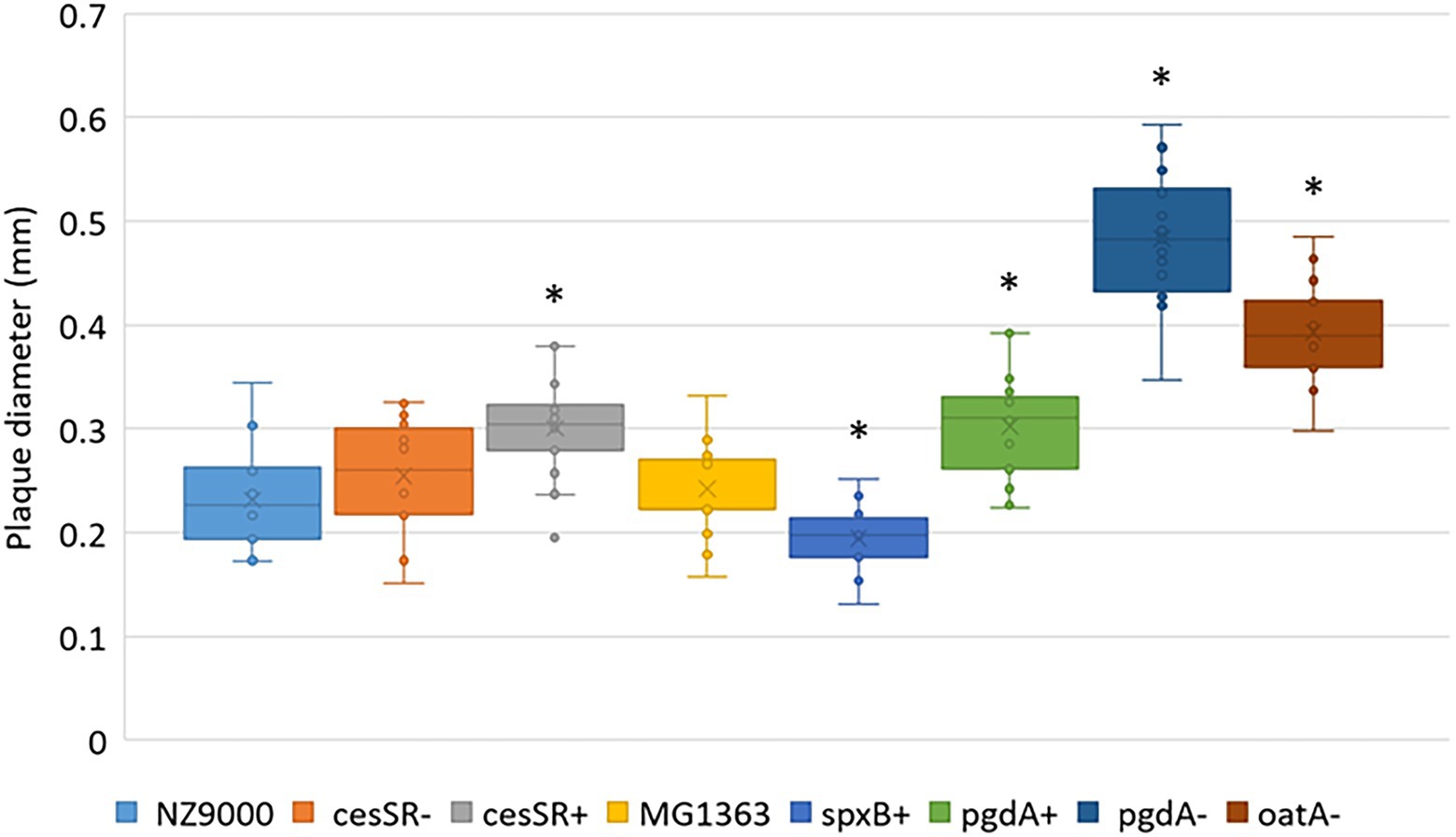
Figure 4. Average plaque diameter of phage c2 on lactococcal CES and PG mutants. *Significantly different (p < 0.05) compared to the wild type L. cremoris MG1363 and NZ9000.
Adsorption and one-step growth curves
To get a deeper insight into the phage infection cycle in each of the lactococcal mutants, adsorption experiments and one-step curves were performed (Table 3). For phage c2, reversible adsorption occurs through interactions with an unidentified saccharide component of the CW and later, irreversible binding to the phage infection protein Pip followed by DNA ejection (Monteville et al., 1994). Under our experimental conditions, total adsorption was not largely affected, although lower adsorption values (p < 0.05) were recorded for cesSR+, spxB+ and pgdA+, the mutants with the lower lytic scores in infections in broth (Table 3).
The results of the one-step growth curves revealed that phage c2 behaved very much alike regardless the host. The same latent period (15 min) was recorded in all lactococcal mutants but oatA-, the later with a latent period 5 min shorter and the lowest burst time (Table 3). Burst sizes were also comparable (100–150 pfu per infected cell) with the exception of cesSR+ where the burst size was reduced by 50% (Table 3).
Discussion
The bacterial CW is highly dynamic and can be either transiently modified in response to external cues or subjected to chemical modification through the activity of various enzymes. In this work, we asked the question if CW modifications could hinder the activity of endolysins from phages infecting dairy starter Lactococcus, so that phage propagation on these “endolysin-resistant” strains might be contained. Host lysis is a critical step for phage survival and previous reports had already shown that it can be targeted either by anti-phage systems such as AbiZ, that causes premature lysis of infected cells (Durmaz and Klaenhammer, 2007) or by host factors which are required for optimal lysis (São-José et al., 2000; Frías et al., 2009; Roces et al., 2013).
To identify such CW modifications, we initially opted to test the exolytic activity of three endolysins with different catalytic specificities (amidase, muramidase and CHAP) against a battery of lactococcal mutants, although turbidity reduction assays do not recapitulate fully the natural context of phage infection. Unfortunately, we were unable to find the right experimental conditions to measure the activity of LysP2 bearing an amidase_2 catalytic domain, one of the most abundant among lactococcal phages, according to a recent survey (Oechslin et al., 2022). The absence of LysP2 activity could be related to a putative multimeric nature of the enzyme. Whereas many endolysins produced by phages that infect Gram positive bacteria are monomeric, some require the assembly of additional domains which are synthesized from internal translational start sites within the endolysin gene, a situation more common than previously anticipated (Pinto et al., 2022). Inspection of the E. coli codon-optimized lysP2 gene revealed the presence of an internal ATG start codon but the consensus ribosome binding site had been lost (see Supplementary Table S1). This absence could explain the lack of LysP2 activity, although this has not been experimentally demonstrated, and other factors (e.g., specific metal ion requirements, buffer composition or substrate specificity) cannot be disregarded.
The results from the turbidity assays showed that indeed modifications of the bacterial CW may compromise, but also improve, the exolytic activity of endolysins and underlined the difference and similarities within phage endolysins bearing CHAP (Lys1358) and muramidase (LysC2) catalytic domains. On one hand, the impact of the degree of PG acetylation (i.e., de-N-acetylation and C6-O-acetylation) had opposite effects on each endolysin. The exolytic activity of LysC2 was enhanced when tested on mutants such as those depleted of pgdA and oatA, known to be susceptible to egg-white lysozyme (Meyrand et al., 2007; Veiga et al., 2007). In contrast, Lys1358 was negatively affected. These two mutations modify defined PG sites which are specifically important for lysozyme activity (Bera et al., 2005) but other surface properties may also be altered. For example, anchoring of rhamnan is postulated to occur at the same site that is acetylated by OatA (Sadovskaya et al., 2017), anticipating an altered cell envelope configuration, which could be relevant for Lys1358 activity.
On the other hand, both endolysins showed a reduced exolytic activity on cesSR+ and spxB+, being susceptibility to Lys1358 lytic action more heavily reduced. This could be explained by a more broader modification of the cell envelope caused by these mutations. The TCS CesSR regulates up to 21 genes, supposedly involved in protecting cells from CW damage, and SpxB may compete with other Spx proteins, leading to so far unknown phenotypes (reviewed by Martínez et al., 2020). Therefore, it is plausible that other modifications are responsible for the altered exolytic activity of the two lactococcal phage endolysins. Moreover, such modifications may also influence endolysin binding to the substrate cells, accounting for their overall lytic activity, as observed when engineering cell wall binding domains (Schmelcher et al., 2011). We have previously reported reduced binding of the cell wall binding domain of LysTP712 to L. lactis mutants lacking ftsH (Roces et al., 2016).
As for the consequences for phage infection, no drastic impacts were seen on phage c2 in terms of resistance. Nonetheless, the two CES mutants cesSR+ and spxB+ tend to curb phage growth slightly, as shown by the lowest lytic scores and EOP values. However, it is not possible to directly infer that this is due to the reduced activity of its endolysin. Firstly, because these mutations did not strongly inhibit LysC2. Secondly, at least, other two factors could account for the lower phage fitness such as the lower growth rate of these mutants and the lower phage adsorption values. Both factors are known to influence phage infection dynamics (Hadas et al., 1997; Dennehy and Abedon, 2020). In the particular case of the cesSR+ mutant, the burst size was remarkably reduced. This may reflect the contribution of this TCS to overcome the stress caused upon phage infection, compromising phage replication and protein synthesis (Fallico et al., 2011). The results for the pgdA+ mutant also exemplify the difficulties encountered in stablishing a correlation between the exolytic activity and phage infection. In this case, in spite of the enhanced exolytic activity of LysC2, the lytic score is reduced, likely due to the lower phage adsorption values.
Mutations that enhanced the exolytic activity of LysC2 such as the lack of NAG de-N-acetylation (pgdA-) and PG O-acetylation (oatA-) did have an impact on phage growth on solid media. On these mutants, phage c2 forms considerably larger plaques (see Figure 4) which could be explained by the shorter latent period, at least, in the case of oatA-. In this scenario, phage virions would be released quicker increasing the number of replicative cycles, so more bacteria would become infected and, consequently, plaques are enlarged (Abedon and Culler, 2007; Liu et al., 2022).
In summary, this work has demonstrated that certain CW modifications have an impact on the exolytic activity of endolysins in a similar fashion as described for autolysins or innate immunity factors such as lysozyme. While in the context of milk fermentations, this may not have important implications, so it does when endolysins targeting pathogenic bacteria are proposed as antimicrobials to fight infections or as food biopreservatives (Schmelcher et al., 2012; Murray et al., 2021). Based on our data, limited to a single endolysin/phage pair, hindering the exolytic activity of endolysins does not appear to impose a major burden to phage growth.
Data availability statement
The datasets presented in this study can be found in online repositories. The names of the repository/repositories and accession number(s) can be found below: Raw data are available at the institutional repository of the Spanish National Research Council, Digital.CSIC https://doi.org/10.20350/digitalCSIC/14766.
Author contributions
AR and BM contributed to conception and design of the study. SE, MP, and CR performed the experiments. SE and BM carried out formal analysis and wrote the first draft of the manuscript. All authors contributed to manuscript revision, read, and approved the submitted version.
Funding
This work has been funded by grants AYUD/2021/52120 (Program of Science, Technology and Innovation 2018–2022, Principado de Asturias, FICYT, FEDER-UE), grant BIO2017-88147-R (MCIN/AEI/10.13039/501100011033 and by “ERDF A way of making Europe”) and grant PID2020-119697RB-I00 (MCIN/AEI/10.13039/501100011033). CR is a fellow of the program “Ayudas Severo Ochoa” of the Principality of Asturias (BP20 006).
Acknowledgments
The authors wish to thank S. Kulakauskas (INRAE, France) for providing lactococcal mutants (pgdA+, pgdA-, oatA-) and templates for Figure 2, and J. Kok (RuG, The Netherlands) for the cesSR mutants.
Conflict of interest
The authors declare that the research was conducted in the absence of any commercial or financial relationships that could be construed as a potential conflict of interest.
Publisher’s note
All claims expressed in this article are solely those of the authors and do not necessarily represent those of their affiliated organizations, or those of the publisher, the editors and the reviewers. Any product that may be evaluated in this article, or claim that may be made by its manufacturer, is not guaranteed or endorsed by the publisher.
Supplementary material
The Supplementary material for this article can be found online at: https://www.frontiersin.org/articles/10.3389/fmicb.2023.1106049/full#supplementary-material
References
Abedon, S. T., and Culler, R. R. (2007). Optimizing bacteriophage plaque fecundity. J. Theor. Biol. 249, 582–592. doi: 10.1016/j.jtbi.2007.08.006
Bera, A., Herbert, S., Jakob, A., Vollmer, W., and Götz, F. (2005). Why are pathogenic staphylococci so lysozyme resistant? The peptidoglycan O-acetyltransferase OatA is the major determinant for lysozyme resistance of Staphylococcus aureus. Mol. Microbiol. 55, 778–787. doi: 10.1111/j.1365-2958.2004.04446.x
Bertozzi Silva, J., Storms, Z., and Sauvageau, D. (2016). Host receptors for bacteriophage adsorption. FEMS Microbiol. Lett. 363:fnw002. doi: 10.1093/femsle/fnw002
Chapot-Chartier, M. P., and Kulakauskas, S. (2014). Cell wall structure and function in lactic acid bacteria. Microb. Cell Factories 13:S9. doi: 10.1186/1475-2859-13-S1-S9
Chmielewska-Jeznach, M., Bardowski, J. K., and Szczepankowska, A. K. (2020). Lactococcus Ceduovirus phages isolated from industrial dairy plants-from physiological to genomic analyses. Viruses 12:280. doi: 10.3390/v12030280
Dennehy, J. J., and Abedon, S. T. (2020). “Phage infection and lysis” in Bacteriophages. eds. D. R. Harper, S. T. Abedon, B. H. Burrowes, and M. L. McConville (Springer: Cham) doi: 10.1007/978-3-319-40598-8_53-1
Durmaz, E., and Klaenhammer, T. R. (2007). Abortive phage resistance mechanism AbiZ speeds the lysis clock to cause premature lysis of phage-infected Lactococcus lactis. J. Bacteriol. 189, 1417–1425. doi: 10.1128/JB.00904-06
Escobedo, S., Campelo, A. B., Wegmann, U., García, P., Rodríguez, A., and Martínez, B. (2019). Insight into the lytic functions of the lactococcal prophage TP712. Viruses 11:881. doi: 10.3390/v11100881
Fallico, V., Ross, R. P., Fitzgerald, G. F., and McAuliffe, O. (2011). Genetic response to bacteriophage infection in Lactococcus lactis reveals a four-strand approach involving induction of membrane stress proteins, D-alanylation of the cell wall, maintenance of proton motive force, and energy conservation. J. Virol. 85, 12032–12042. doi: 10.1128/JVI.00275-11
Frías, M. J., Melo-Cristino, J., and Ramírez, M. (2009). The autolysin LytA contributes to efficient bacteriophage progeny release in Streptococcus pneumoniae. J. Bacteriol. 191, 5428–5440. doi: 10.1128/JB.00477-09
Gasson, M. J. (1983). Plasmid complements of Streptococcus lactis NCDO 712 and other lactic streptococci after protoplast-induced curing. J. Bacteriol. 154, 1–9. doi: 10.1128/jb.154.1.1-9.1983
Grishin, A. V., Karyagina, A. S., Vasina, D. V., Vasina, I. V., Gushchin, V. A., and Lunin, V. G. (2020). Resistance to peptidoglycan-degrading enzymes. Crit. Rev. Microbiol. 46, 703–726. doi: 10.1080/1040841X.2020.1825333
Hadas, H., Einav, M., Fishov, I., and Zaritsky, A. (1997). Bacteriophage T4 development depends on the physiology of its host Escherichia coli. Microbiology 143, 179–185. doi: 10.1099/00221287-143-1-179
Jordan, S., Hutchings, M. I., and Mascher, T. (2008). Cell envelope stress response in gram-positive bacteria. FEMS Microbiol. Rev. 32, 107–146. doi: 10.1111/j.1574-6976.2007.00091.x
Kuipers, O. P., De Ruyter, P. G. G. A., Kleerebezem, M., and De Vos, W. M. (1998). Quorum sensing-controlled gene expression in lactic acid bacteria. J. Biotech. 64, 15–21. doi: 10.1016/S0168-1656(98)00100-X
Latka, A., Maciejewska, B., Majkowska-Skrobek, G., Briers, Y., and Drulis-Kawa, Z. (2017). Bacteriophage-encoded virion-associated enzymes to overcome the carbohydrate barriers during the infection process. Appl. Microbiol. Biotechnol. 101, 3103–3119. doi: 10.1007/s00253-017-8224-6
Lepeuple, A. S., Van Gemert, E., and Chapot-Chartier, M. P. (1998). Analysis of the bacteriolytic enzymes of the autolytic Lactococcus lactis subsp. cremoris strain AM2 by renaturing polyacrylamide gel electrophoresis: identification of a prophage-encoded enzyme. Appl. Environ. Microbiol. 64, 4142–4148. doi: 10.1128/AEM.64.11.4142-4148.1998
Liu, C., Hong, Q., Chang, R. Y. K., Kwok, P. C. L., and Chan, H. K. (2022). Phage-antibiotic therapy as a promising strategy to combat multidrug-resistant infections and to enhance antimicrobial efficiency. Antibiotics 11:570. doi: 10.3390/antibiotics11050570
Madera, C., García, P., Janzen, T., Rodríguez, A., and Suárez, J. E. (2003). Characterization of technologically proficient wild Lactococcus lactis strains resistant to phage infection. Int. J. Food Microbiol. 86, 213–222. doi: 10.1016/s0168-1605(03)00042-4
Mahony, J., Cambillau, C., and van Sinderen, D. (2017). Host recognition by lactic acid bacterial phages. FEMS Microbiol. Rev. 41, S16–S26. doi: 10.1093/femsre/fux019
Marcó, M. B., Moineau, S., and Quiberoni, A. (2012). Bacteriophages and dairy fermentations. Bacteriophage 2, 149–158. doi: 10.4161/bact.21868
Martínez, B., Rodríguez, A., Kulakauskas, S., and Chapot-Chartier, M. P. (2020). Cell wall homeostasis in lactic acid bacteria: threats and defences. FEMS Microbiol. Rev. 44, 538–564. doi: 10.1093/femsre/fuaa021
Martínez, B., Zomer, A. L., Rodríguez, A., Kok, J., and Kuipers, O. P. (2007). Cell envelope stress induced by the bacteriocin Lcn972 is sensed by the Lactococcal two-component system CesSR. Mol. Microbiol. 64, 473–486. doi: 10.1111/j.1365-2958.2007.05668.x
Meyrand, M., Boughammoura, A., Courtin, P., Mézange, C., Guillot, A., and Chapot-Chartier, M. P. (2007). Peptidoglycan N-acetylglucosamine deacetylation decreases autolysis in Lactococcus lactis. Microbiology 153, 3275–3285. doi: 10.1099/mic.0.2007/005835-0
Moak, M., and Molineux, I. J. (2004). Peptidoglycan hydrolytic activities associated with bacteriophage virions. Mol. Microbiol. 51, 1169–1183. doi: 10.1046/j.1365-2958.2003.03894.x
Moineau, S., Durmaz, E., Pandian, S., and Klaenhammer, T. R. (1993). Differentiation of two abortive mechanisms by using monoclonal antibodies directed toward lactococcal bacteriophage capsid proteins. Appl. Environ. Microbiol. 59, 208–212. doi: 10.1128/aem.59.1.208-212.1993
Monteville, M. R., Ardestani, B., and Geller, B. L. (1994). Lactococcal bacteriophages require a host cell wall carbohydrate and a plasma membrane protein for adsorption and ejection of DNA. Appl. Environ. Microbiol. 60, 3204–3211. doi: 10.1128/aem.60.9.3204-3211.1994
Murray, E., Draper, L. A., Ross, R. P., and Hill, C. (2021). The advantages and challenges of using endolysins in a clinical setting. Viruses 13:680. doi: 10.3390/v13040680
Oechslin, F., Zhu, X., Dion, M. B., Shi, R., and Moineau, S. (2022). Phage endolysins are adapted to specific hosts and are evolutionarily dynamic. PLoS Biol. 20:e3001740. doi: 10.1371/journal.pbio.3001740
Oliveira, H., Melo, L. D., Santos, S. B., Nóbrega, F. L., Ferreira, E. C., Cerca, N., et al. (2013). Molecular aspects and comparative genomics of bacteriophage endolysins. J. Virol. 87, 4558–4570. doi: 10.1128/JVI.03277-12
Pillidge, C. J., and Jarvis, A. W. (1988). DNA restriction maps and classification of the lactococcal bacteriophages c2 and skl. J. Dairy. Sci. Technol. 23, 411–416.
Pinto, D., Gonçalo, R., Louro, M., Silva, M. S., Hernandez, G., Cordeiro, T. N., et al. (2022). On the occurrence and multimerization of two-polypeptide phage endolysins encoded in single genes. Microbiol. Spectr. 10:e0103722. doi: 10.1128/spectrum.01037-22
Pinto, J. P., Kuipers, O. P., Marreddy, R. K., Poolman, B., and Kok, J. (2011). Efficient overproduction of membrane proteins in Lactococcus lactis requires the cell envelope stress sensor/regulator couple CesSR. PLoS One 6:e21873. doi: 10.1371/journal.pone.0021873
Roces, C., Campelo, A. B., Escobedo, S., Wegmann, U., García, P., Rodríguez, A., et al. (2016). Reduced binding of the endolysin LysTP712 to Lactococcus lactis ΔftsH contributes to phage resistance. Front. Microbiol. 7:138. doi: 10.3389/fmicb.2016.00138
Roces, C., Wegmann, U., Campelo, A. B., García, P., Rodríguez, A., and Martínez, B. (2013). Lack of the host membrane protease FtsH hinders release of the Lactococcus lactis bacteriophage TP712. J. Gen. Virol. 94, 2814–2818. doi: 10.1099/vir.0.057182-0
Sadovskaya, I., Vinogradov, E., Courtin, P., Armalyte, J., Meyrand, M., Giaouris, E., et al. (2017). Another brick in the wall: a rhamnan polysaccharide trapped inside peptidoglycan of Lactococcus lactis. mBio 8:e01303-17. doi: 10.1128/mBio.01303-17
Sambrook, J., Fritsch, E. R., and Maniatis, T. (1989). Molecular Cloning: A Laboratory Manual, 2nd Edn Cold Spring Harbor, NY: Cold Spring Harbor Laboratory Press.
São-José, C., Parreira, R., Vieira, G., and Santos, M. A. (2000). The N-terminal region of the Oenococcus oeni bacteriophage fOg44 lysin behaves as a bona fide signal peptide in Escherichia coli and as a cis-inhibitory element, preventing lytic activity on oenococcal cells. J. Bacteriol. 182, 5823–5831. doi: 10.1128/JB.182.20.5823-5831.2000
Schmelcher, M., Donovan, D. M., and Loessner, M. J. (2012). Bacteriophage endolysins as novel antimicrobials. Future Microbiol. 7, 1147–1171. doi: 10.2217/fmb.12.97
Schmelcher, M., Tchang, V. S., and Loessner, M. J. (2011). Domain shuffling and module engineering of listeria phage endolysins for enhanced lytic activity and binding affinity. Microb. Biotechnol. 4, 651–662. doi: 10.1111/j.1751-7915.2011.00263.x
Steen, A., Buist, G., Kramer, N. E., Jalving, R., Benus, G. F., Venema, G., et al. (2008). Reduced lysis upon growth of Lactococcus lactis on galactose is a consequence of decreased binding of the autolysin AcmA. Appl. Environ. Microbiol. 74, 4671–4679. doi: 10.1128/AEM.00103-08
Veiga, P., Bulbarela-Sampieri, C., Furlan, S., Maisons, A., Chapot-Chartier, M. P., Erkelenz, M., et al. (2007). SpxB regulates O-acetylation-dependent resistance of Lactococcus lactis peptidoglycan to hydrolysis. J. Biol. Chem. 282, 19342–19354. doi: 10.1074/jbc.M611308200
Veiga, P., Erkelenz, M., Bernard, E., Courtin, P., Kulakauskas, S., and Chapot-Chartier, M. P. (2009). Identification of the asparagine synthase responsible for D-Asp amidation in the Lactococcus lactis peptidoglycan interpeptide crossbridge. J. Bacteriol. 191, 3752–3757. doi: 10.1128/JB.00126-09
Viscardi, M., Capparelli, R., Di Matteo, R., Carminati, D., Giraffa, G., and Iannelli, D. (2003). Selection of bacteriophage-resistant mutants of Streptococcus thermophilus. J. Microbiol. Methods 55, 109–119. doi: 10.1016/s0167-7012(03)00146-5
Keywords: bacteriophage, endolysins, Lactococcus, peptidoglycan, cell envelope stress
Citation: Escobedo S, Pérez de Pipaon M, Rendueles C, Rodríguez A and Martínez B (2023) Cell wall modifications that alter the exolytic activity of lactococcal phage endolysins have little impact on phage growth. Front. Microbiol. 14:1106049. doi: 10.3389/fmicb.2023.1106049
Edited by:
Marcin Łoś, University of Gdansk, PolandReviewed by:
Daniel Nelson, University of Maryland, College Park, United StatesCarlos São-José, University of Lisbon, Portugal
Malgorzata Barbara Lobocka, Institute of Biochemistry and Biophysics (PAN), Poland
Copyright © 2023 Escobedo, Pérez de Pipaon, Rendueles, Rodríguez and Martínez. This is an open-access article distributed under the terms of the Creative Commons Attribution License (CC BY). The use, distribution or reproduction in other forums is permitted, provided the original author(s) and the copyright owner(s) are credited and that the original publication in this journal is cited, in accordance with accepted academic practice. No use, distribution or reproduction is permitted which does not comply with these terms.
*Correspondence: Beatriz Martínez, Ym1mMUBpcGxhLmNzaWMuZXM=