- 1State Key Laboratory of Freshwater Ecology and Biotechnology, Key Laboratory of Algal Biology, Institute of Hydrobiology, Chinese Academy of Sciences, Wuhan, China
- 2College of Life Science, University of Chinese Academy of Sciences, Beijing, China
The diatom Skeletonema costatum and the haptophyte Phaeocystis globosa often form blooms in the coastal waters of the South China Sea. Skeletonemacostatum commonly dominates in nutrient enrichment coastal waters, whereas P. globosa starts flourishing after the diatom blooms when phosphorus (P) is limited. Therefore, P limitation was proposed to be a critical factor affecting diatom–haptophyte transition. To elucidate the tolerance to P limitation in P. globosa compared with S. costatum, the effect of P limitation on their photosystem II (PSII) performance was investigated and their photosynthesis acclimation strategies in response to P limitation were evaluated. P limitation did not affect the growth of P. globosa over 7 days but decreased it for S. costatum. Correspondingly, the PSII activity of S. costatum was significantly inhibited by P limitation. The decline in PSII activity in S. costatum under P limitation was associated with the impairment of the oxygen-evolving complex (the donor side of PSII), the hindrance of electron transport from QA− to QB (the acceptor side of PSII), and the inhibition of electron transport to photosystem I (PSI). The 100% decrease in D1 protein level of S. costatum after P limitation for 6 days and PsbO protein level after 2 days of P limitation were attributed to its enhanced photoinhibition. In contrast, P. globosa maintained its photosynthetic activity with minor impairment of the function of PSII. With accelerated PSII repair and highly increased non-photochemical quenching (NPQ), P. globosa can avoid serious PSII damage under P limitation. On the contrary, S. costatum decreased its D1 restoration under P limitation, and the maximum NPQ value in S. costatum was only one-sixth of that in P. globosa. The present work provides extensive evidence that a close interaction exists between the tolerance to P limitation and photosynthetic responses of S. costatum and P. globosa.
1. Introduction
The diatom Skeletonema costatum and the haptophyte Phaeocystis globosa are often detected in the coastal water of the South China Sea (SCS) and found to form intensive blooms frequently (Liu and Tang, 2012; Wang X. D. et al., 2021; Wang et al., 2022). Skeletonema costatum usually dominates in nutrient enrichment coastal waters and is the most frequently occurring bloom species in some areas of the SCS such as the eastern Guangdong coastal region (Li et al., 2019) and Xiamen Bay (Chen et al., 2021) over the past 30 years. Although S. costatum is nontoxic, its blooms generate huge biomass influencing not only biogeochemical cycling but also the food chain (Falkowski et al., 1998; Armbrust, 2009). Phaeocystis globosa is a haptophyte species with ecological significance and can form harmful algal blooms that cause gill damage and hypoxia of fish, and its production of hemolytic toxins can contaminate seafood or kill fish. P. globosa has caused blooms almost every year in Chinese coastal waters since 1997 when the first occurrence was recorded (Chen et al., 1999). Wang K. et al. (2021) estimated that 80.6% of the P. globosa blooms in China occur in the SCS. P. globosa blooms in SCS usually occur after diatom blooms in the period from November to March (Chen et al., 2009; Wang K. et al., 2021). This phytoplankton succession in spring from diatoms to P. globosa is also a common pattern observed in the North Sea coast (Peperzak et al., 1998; Cadee and Hegeman, 2002), the Belgian coastal zone (Lancelot et al., 2005), and the Wadden Sea (Ly et al., 2014). S. costatum is also one of the common early succession species of the diatom–P. globosa (Wang X. D. et al., 2021). The latest S. costatum–P. globosa succession in SCS was recorded in Mirs Bay in late January 2021 (Liu et al., 2021).
The succession of dominant species in water bodies is considered to be regulated by numerous environmental factors and the interplay of multiple factors (Deng et al., 2014; Lu et al., 2022). The “silicate-Phaeocystis hypothesis” has historically been a major explanation for the appearance of Phaeocystis because environmental silicate concentration may determine the duration and stability of the diatom community (Lancelot et al., 1987; Reid et al., 1990). Moreover, some authors have considered the effects of temperature (Jahnke, 1989), iron availability (Schoemann et al., 2005), irradiance (Escaravage et al., 1995), and the capacity to escape grazing (Peperzak et al., 1998) on the succession from diatoms to P. globosa. Phosphorus (P) limitation often occurs in various parts of the oceans (Thingstad et al., 2005; Lin et al., 2016) as P is rapidly consumed for photosynthesis in the euphotic zone and its resupply is slow (White and Dyhrman, 2013). P limitation may also be a critical factor affecting the diatom–P. globosa transition (Lin et al., 2016; Karasiewicz et al., 2018). Skeletonema. costatum often dominates in nutrient-rich water because of its rapid growth rate related to its higher photosynthetic rate per unit carbon (Chan, 1980; Armbrust, 2009). The growth of S. costatum collapsed soon after P limitation (Ou et al., 2008; Huang et al., 2020). Ecologically, diatoms and P. globosa usually coexist during the initial phase of the spring bloom (Wang et al., 2013), but P. globosa rapidly increases at the start of the late bloom although phosphate concentrations are low (Burson et al., 2016; Liu et al., 2021). P reduction negatively affects diatoms without negative effects on Phaeocystis (Gypens et al., 2007).
Phaeocystis globosa appears to be more tolerant to P limitation than S. costatum. One of the most important mechanisms for coping with P limitation is the utilization of dissolved organic phosphorus (DOP). Although S. costatum and P. globosa have not been directly compared, it is clear that both species can exploit DOP when orthophosphate is low (Ghyoot et al., 2015; Ou et al., 2015). S. costatum has a much lower affinity for DOP compared with the dinoflagellate Prorocentrum donghaiense (Ou et al., 2008), which also often succeeds after the S. costatum bloom (Lu et al., 2022). Compared with diatoms, more tolerance by P. globosa to P limitation is likely because of its lower P demand (Riegman et al., 1992). S. costatum has been thought to require more phosphate because it has a high ATP content associated with a rapid growth rate (Egge, 1998). In addition, the unusual heteromorphic life cycle of P. globosa that includes gelatinous colonies and solitary cells might also contribute to its better performance under P limitation. The colony matrix of P. globosa can act as an energy and nutrient reservoir (Veldhuis et al., 1991). The solitary cells of P. globosa can compete for limiting nutrients because of their large surface area/volume ratio and their ability to move to the deeper nutrient-rich water (Verity and Medlin, 2003; Zhuang et al., 2022). Recently, Koppelle et al. (2022) demonstrated that P. globosa can utilize a phago-mixotrophic feeding strategy to acquire P and other nutrients by ingesting bacteria, which may also contribute to its remarkable success in forming blooms under P-limited conditions.
Photosynthesis is one of the most critical processes in the primary production of phytoplankton. A close interaction exists between the tolerance to P limitation and photosynthesis. Photosynthetic activity may affect the tolerance to P limitation because the energy sources for inorganic phosphate (Pi) uptake and alkaline phosphatase (APase) synthesis, which participate in the hydrolysis of DOP into phosphate, in microalgae are mostly derived from photosynthesis (Pandey, 2006; Gorbunov and Falkowski, 2022). P limitation causes a decline in photosynthesis in several different ways, including decreasing CO2 assimilation by reducing the regeneration of ribulose-1,5-bisphosphate in the Calvin cycle (Lewis et al., 1994; Campbell and Sage, 2006) as well as inhibiting electron transport between photosystem II (PSII) and photosystem I (PSI; Van Rensen and Vredenberg, 2009; Carstensen et al., 2018). P limitation selectively affected the PSII machinery in the model green alga Chlamydomonas reinhardtii with retardation of electron flow from QA− to QB (with QA and QB being the first and second quinone electron acceptors of PSII, respectively; Wykoff et al., 1998) and a drop in the level of the reaction center protein D1 (Allen et al., 2007). In addition, some studies have demonstrated that the regulation of various strategies for photosynthesis acclimation affects the tolerance to P limitation of phytoplankton. Enhancement of non-photochemical quenching (NPQ) helped avoid photodamage from excess light energy for coping with P deprivation in dinoflagellate Karlodinium veneficum (Cui et al., 2017). However, decreased PSII repair capacity in Dunaliella tertiolecta increased its susceptibility to photoinhibition under P limitation (Heraud et al., 2005). Although the photosynthetic activity of S. costatum (Liu et al., 2013), P. donghaiense (Shi et al., 2017), and some other species (Li et al., 2021) are significantly inhibited by P limitation, the mechanisms for the decline in photosynthetic activity are poorly studied in marine species with ecological significance, and the photosynthetic response of P. globosa under P limitation remains to be explored.
This study hypothesized that P. globosa has a higher capacity to sustain its photosynthetic activity under P limitation compared with diatom S. costatum, which contributes to their different tolerance to P limitation and affects their survival, dominance, and succession.
2. Materials and methods
2.1. Culture conditions and experimental design
Experimental strains S. costatum and P. globosa were both isolated from the coastal water in the SCS and cultured in Erdschreiber’s medium (modified from the original Plymouth seawater recipe, Rosowski and Parker, 1971; Supplementary Table S1) at (24 ± 1)°C and 50 μmol photons m−2 s−1 under a 24 h light: 0 h dark cycle. Phaeocystis. globosa was kept in the solitary form throughout the experimental period, and colony formation was not observed. This could be explained by no environmental stresses, such as grazing, in the laboratory. Na2SiO3·9H2O with a final concentration of 106 μM was added to the culture of S. costatum. Erdschreiber’s medium lacking Na2HPO4 was used as a P-free medium in which Pi-containing compounds were substituted with NaCl. Algal cells in the logarithmic growth phase under P-repleted condition were collected by centrifugation, washed three times with P-free Erdschreiber’s medium, and resuspended into 500 mL Erlenmeyer flasks containing 250 mL Erdschreiber’s medium (PO43− concentration: 67.0 μM) as the control group (+P) and 250 mL P-free medium (PO43− concentration: 0.0 μM) as the treatment group (−P). The initial biomass of experimental algae was set to an OD730 of 0.15 corresponding to Chl a content of ~0.6 μg/mL in S. costatum and ~ 0.4 μg/mL in P. globosa. All cultures were shaken three times every day during the 7-day cultivation period. All treatments were performed in triplicates.
To test the effect of intracellular P on the growth of S. costatum and P. globosa, the contents of polyphosphate body (PolyP) in cells were quantified according to Martin and Van Mooy (2013). Cells were harvested by centrifugation (Eppendorf 5810R centrifuge, 4,000 × g, 3 min). 10 μL proteinase K (20 mg/mL) was added to the pellets and incubated at 37°C for 30 min. Then centrifuged for 1 min at 16100 × g and the supernatant was removed. PolyP contents of samples were measured after the addition of DAPI (final concentration: 100 μM) and made on a fluorescence spectrofluorometer (F-2700, Hitachi, Japan) at an excitation wavelength of 415 nm and emission wavelength of 550 nm, with an integration time of 0.5 s.
Various chlorophyll fluorescence tools combined with protein measurements were used to examine the different effects of P limitation on PSII in S. costatum and P. globosa. The photosynthetic acclimation mechanisms of S. costatum and P. globosa were explored in terms of induction of NPQ and D1 turnover.
2.2. Growth and maximum photochemistry efficiency (Fv/Fm)
Growth characteristics of cells were studied by monitoring the optical density at 730 nm (OD730) using a spectrophotometer. Fv/Fm was obtained from the JIP-test after dark-adapted for 15 min at room temperature according to Strasser et al. (2004) with a dual modulation kinetic fluorometer FL3500 (PSI, Brno, Czechia). These two values were obtained from cultures every day.
2.3. Rapid light response curve
The rapid light response curve included steps of actinic irradiance: 2, 7, 14, 28, 45, 64, 92, 131, 181, 245, 321, 412, 540, 684, 869, 1,109, and 1,410 μmol·m−2 s−1, with a 30-s interval between steps using a Dual-PAM 100 (Walz, Effeltrich, Germany) under the illumination with the measuring light at 460 nm and the red actinic light at 635 nm. The built-in fitting function was used to fit the rapid light response curves with the EP model and derive the maximum electron transport rate (ETRmax), light-harvesting efficiency (alpha), and the point of light saturation (Ik).
2.4. Polyphasic Chl a fluorescence transient
Samples were dark-adapted for 15 min at room temperature before fluorescence measurement using a dual modulation kinetic fluorometer FL3500 (PSI, Brno, Czechia). Under conditions of continuous red actinic light (630 nm) at a high intensity of 2000 μmol·m−2 s−1, the Chl a fluorescence transient was recorded up to 1 s on a logarithmic timescale, with data acquisition every 10 μs for the first 2 ms and for every 1 ms thereafter. The curves were normalized between Fo and Fm and plotted as Vt changes on a logarithmic scale [Vt = (Ft − Fo)/(Fm − Fo)], where Ft is the fluorescence in respective time, Fo is the minimal fluorescence and Fm is the maximum fluorescence. The quantum yield of electron transport (φE0), the reduction in end acceptors on the PSI electron acceptor side (RE0/RC), and the amount of active PSII RCs per CS (RC/CS0) values were obtained from the JIP-test.
2.5. Measurement of QA− reoxidation kinetics
Samples of 3 mL were adjusted to an OD730 of 0.7 and dark-adapted for 10 min before measurement. The QA− reoxidation kinetics after a single turnover flash was performed with a double-modulation fluorometer FL6600 (Photon Systems Instruments, Brno, Czechia; Eisenstadt et al., 2008). Both measuring flashes (4 μs) and actinic flashes (50 μs) were provided by computer-controlled light-emitting diodes. QA− reoxidation kinetic curves were normalized and plotted as [F(t)-F(0)] changes on a logarithmic scale, where F(t) is the fluorescence in respective time and F(0) is the initial fluorescence.
The relaxation of the flash-induced increase in Chl a fluorescence yield reflects the reoxidation of QA− via forward electron transport to QB and reverse reactions with the S2 state of the oxygen-evolving complex (OEC; Cao and Govindjee, 1990; Dau, 1994). The fast phase is attributable to the reoxidation of QA− by QB. The middle phase arises from QA− reoxidation in the PSII reaction center that has an empty QB site at the time of the flash and has to bind to a plastoquinone (PQ) molecule from the PQ pool. The slow phase reflects QA− reoxidation with the S2 state of the OEC, thus causing backward electron transport through the equilibrium of QA−QB and QAQB− (Zhang et al., 2017). When QA− reoxidation kinetics were determined in the presence of 20 μM 3-(3,4-dichlorophenyl)-1,1-dimethylurea (DCMU), the fluorescence decay reflects QA− reoxidation via charge recombination with the S2 state of the OEC (Vass et al., 1999).
According to Beauchemkin et al. (2007), the QA− reoxidation kinetics curves were fitted by the three-exponential component decay equation:
Where F(t) is the variable fluorescence yield, A0 to A3 are the amplitudes, and T1 to T3 are the time constants from which the half-life values can be calculated as t1/2 = ln 2 T.
2.6. S-state test
To further determine the proportion of inactive PSII centers (PSIIX), S-state tests were performed with FL6600 after concentrated and dark-adapted samples as described in section 2.5. The population of the PSIIX center was measured by the difference between Fo and the fluorescence level 100 ms after the fourth flash (F4 = F400ms/Fo − 1) because the fluorescence decay after the fourth flash is controlled almost entirely by inactive centers (Lavergne and Leci, 1993). Given the decreased relative variable fluorescence, a revised equation was proposed according to Pan et al. (2008) as follows: PSIIX(%) = F4 × 100/(F300ms/Fo − 1).
2.7. Measurement of non-photochemical quenching
Samples were dark-adapted for 30 min before measurement using a Dual-PAM 100. The slow induction kinetic curves at a specific process (Delay: 30 s, Measure light: 46 μmol photons m−2 s−1, Saturable Pulse: 20000 μmol photons m−2 s−1, Width: 300 ms, Clock: 1 min). NPQ was calculated as Bilger and Bjorkman (1990): NPQ = (Fm − Fm′)/Fm′, where Fm′ is the maximum fluorescence measured after the samples are exposed to continuous red actinic light (635 nm) of 321 μmol photons m−2 s−1.
2.8. Total cellular protein extraction, SDS-PAGE analysis, and immunoblotting
Total cellular proteins were extracted as previously described by Thangaraj et al. (2021) with minor modifications. Cells were harvested and resuspended in lysis buffer (40 mM Tris–HCl, pH 8.0) supplemented with 1 mM phenylmethanesulfonyl fluoride (PMSF) as the protease inhibitor. The cells were lysed using a noise isolating chamber (3 s on, 3 s off, Scientz, China) for 20 min on ice with a whole-cell lysate and then centrifuged (Eppendorf 5810R centrifuge) at 1,800 × g for 10 min at 4°C to remove cell debris. Protein concentrations were determined using the BCA assay (Beyotime, China).
Protein samples were subjected to 12.5% SDS-PAGE where each gel lane was loaded with equal amounts of 10 μg, stained with Coomassie brilliant blue R250, or transferred to polyvinylidene fluoride membranes. Subsequently, each membrane was blocked for 1 h in 5% skimmed milk and probed using rabbit primary anti-D1 (1:4000, PhytoAB, United States) and anti-PsbO (1:2000, PhytoAB, United States) antibodies. Immunodetection was performed using a goat-anti-rabbit secondary antibody conjugated to horseradish peroxidase (1:5000). Proteins were visualized on the basis of the intensities of immunoreactions using an ImageQuant LAS 4000 Mini system (GE Healthcare) and semi-quantitated by ImageJ 1.52a (Wayne Rasband, United States).
The clearance of the D1 protein untreated and treated with lincomycin (block counteracting repair processes, Wu et al., 2012) was plotted and compared. Samples in triplicates were supplemented with 1,000 μg/mL lincomycin and incubated in the dark for 10 min, to allow the antibiotic to penetrate the cells and inhibit ribosome function. All samples were then shifted to 50 μmol photons m−2 s−1 and collected on days 0, 1 and 4 for later protein immunodetection. Newly synthesized D1 protein in PSII repair was calculated as D1newly = D1active − D1blocked, where D1active is the change in the active D1 level in the absence of lincomycin (PSII repair active), and D1blocked is the change in the active D1 level in the presence of lincomycin (PSII repair blocked).
2.9. Statistical analyses
All experiments were performed in triplicates, and the results are presented as means ± standard deviations (SD). Statistical significance of differences between treatments was compared using two-way ANOVA with the least-significant difference (LSD) by SPSS 18.0 (IBM, United States).
3. Results
3.1. The growth characteristics and contents of PolyP of Skeletonema costatum and Phaeocystis globosa
To test the endogenous phosphorus in initial experimental cells, intracellular PolyP in S. costatum and P. globosa in +P and − P groups on days 0, 3 and 7 were measured (Supplementary Figure S1). The contents of PolyP in S. costatum and P. globosa cells were both significantly lower in the +P group compared with the −P group on day 0 (p < 0.01, ANOVA), indicating that the above two strains have the ability to store phosphate and form the PolyP when P is sufficient. The contents of PolyP in S. costatum and P. globosa in −P groups were 0.005 ± 0.0016 and 0.003 ± 0.0003 nmol per OD730 on day 0, and 0.003 ± 0.0002 and 0.002 ± 0.0008 nmol per OD730 on day 7, respectively (Supplementary Figure S1), which indicated that the cells in −P groups were P-limited for the entire experiment duration.
The cell propagation of S. costatum was completely inhibited after exposure to P limitation. The OD730 of cells cultured in −P group was significantly lower after 4-day cultivation (Figure 1A, p < 0.01, ANOVA), implying that S. costatum experienced growth inhibition under the P-limited condition. In comparison, P. globosa cell growth in the absence of P showed OD730 similar to that of the control (p > 0.05), which could still maintain a logarithmic period (Figure 1B).
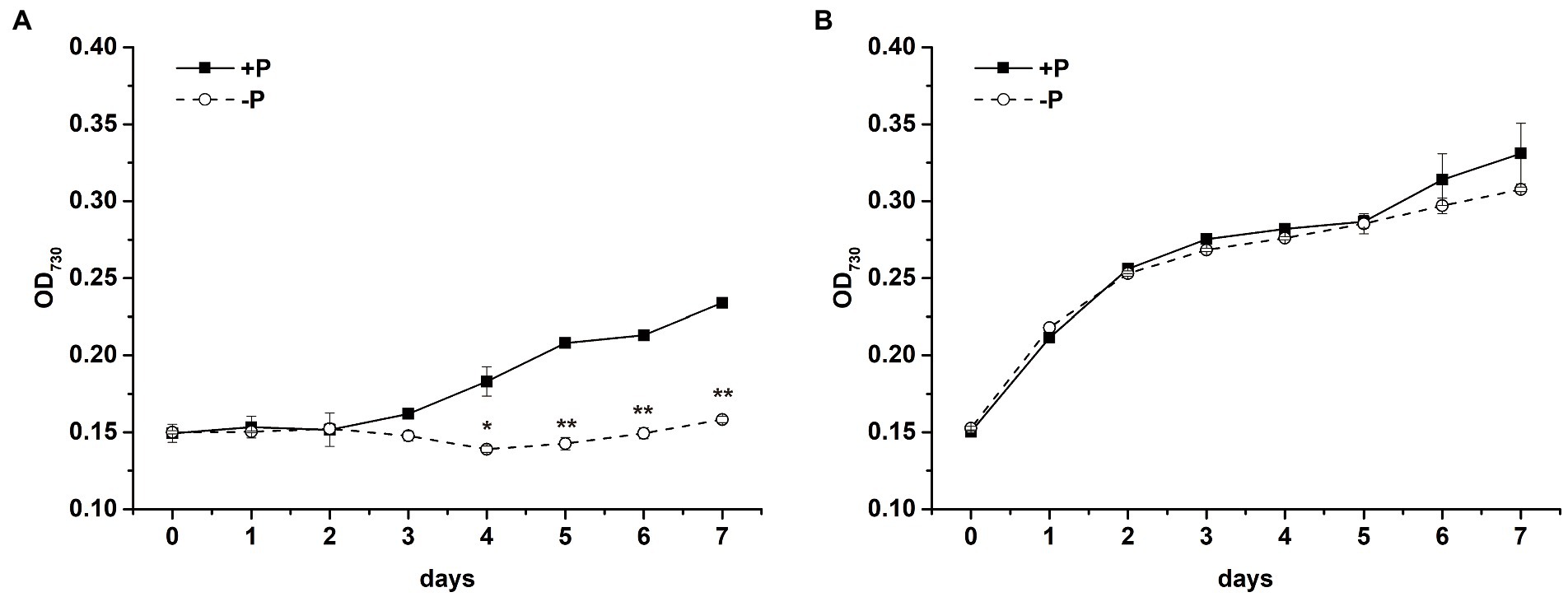
Figure 1. Growth curves of S. costatum (A) and P. globosa (B) in +P and −P groups. Error bars show the mean ± standard error (SE) of triplicate treatments. Superscripts indicate significant differences between +P group and −P group according to a two-way ANOVA with a post-hoc test (**p < 0.01, *p < 0.05).
3.2. Effects of P limitation on chlorophyll fluorescence in Skeletonema costatum and Phaeocystis globosa
3.2.1. Effects of P limitation on Fv/Fm
To explore the effect of P limitation on PSII, time courses were presented for Fv/Fm of PSII in S. costatum (Figure 2A) and P. globosa (Figure 2B). A difference in Fv/Fm values in the +P and − P groups was observed in S. costatum (Figure 2A). Fv/Fm of S. costatum decreased sharply after being cultured under P limitation (Figure 2A, p < 0.05, ANOVA), whereas that in the +P culture stayed at a relatively stable and higher level, indicating that P limitation led to lower photochemical efficiency in S. costatum. No significant difference in Fv/Fm of P. globosa was found in the +P and − P groups (Figure 2B, p > 0.05, ANOVA), even after 25 days of incubation (Supplementary Figure S2B), indicating that Fv/Fm of P. globosa could maintain stability under P limitation.
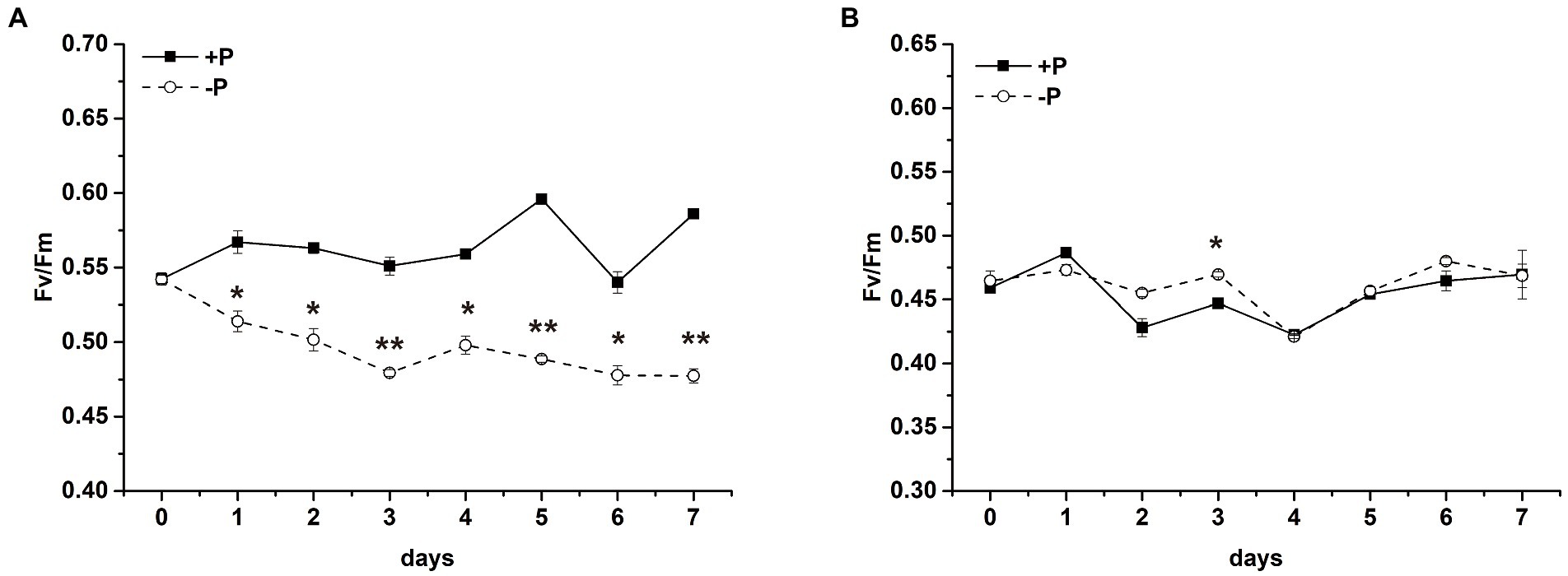
Figure 2. Maximum photochemistry efficiency (Fv/Fm) of S. costatum (A) and P. globosa (B) in +P and −P groups. Error bars show the mean ± standard error (SE) of triplicate treatments. Superscripts indicate significant differences between +P group and −P group according to a two-way ANOVA with a post-hoc test (**p < 0.01, *p < 0.05).
3.2.2. Effects of P limitation on the electron transport rate and light-harvesting efficiency
The maximal electron transport rates through PSII (ETRmax), light-harvesting efficiency (alpha), and the point of light saturation (Ik) were measured in the +P and − P groups on days 0, 2, and 6, respectively (Figure 3). After 2 days of cultivation, the ETRmax and alpha values of S. costatum were both significantly lower in the −P group compared with the +P group (Figures 3A,C, p < 0.01, ANOVA), indicating that the ability to transport the electron and to harvest the light of S. costatum cells decreased under P limitation. However, no significant decrease was observed in the above two values of the −P group compared with that of the +P group in P. globosa (Figures 3B,D). Thus, the electron transport and light-harvesting abilities of P. globosa were not limited by P limitation.
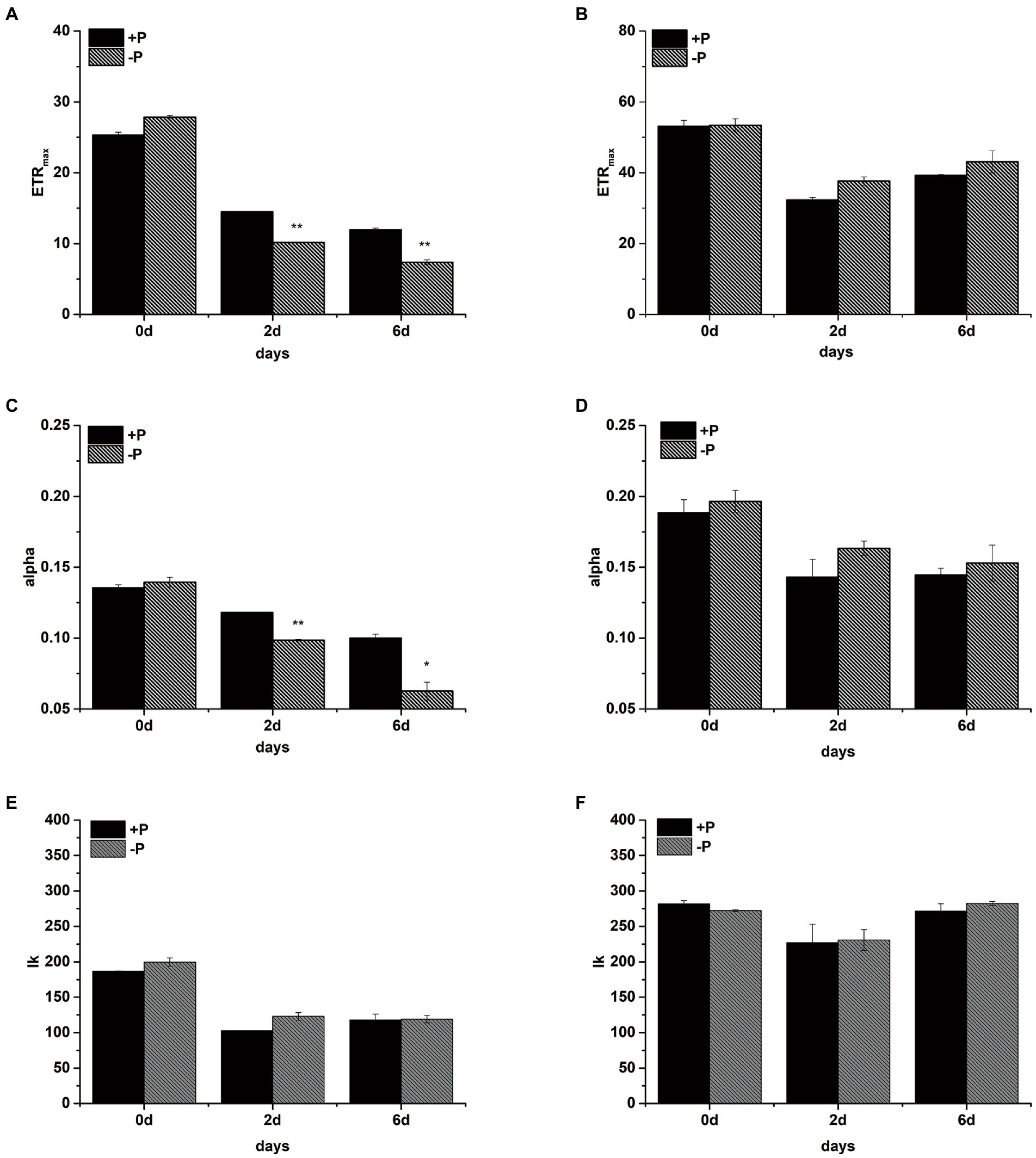
Figure 3. The maximum electron transport rate ETRmax (A: S. costatum, B: P. globosa), light-harvesting efficiency alpha (C: S. costatum, D: P. globosa), and the point of light saturation Ik (E: S. costatum, F: P. globosa) in +P and −P groups. Error bars show the mean ± standard error (SE) of triplicate treatments. Superscripts indicate significant differences between +P group and −P group according to a two-way ANOVA with a post-hoc test (**p < 0.01, *p < 0.05).
3.2.3. Polyphasic Chl a fluorescence transient
The polyphasic rise of the Chl a fluorescence transient (OJIP) in S. costatum and P. globosa cultured with −P groups were monitored to localize the action site of P limitation in the PSII transport chain. A typical curve characteristic has four phases of O, J, I, and P (Stirbet and Govindjee, 2012), but the peak in phase I was not observed in both species in this study. The fluorescence intensity of phase J represents the accumulation of QA−QB and QA−QB− (with QA and QB being the first and second quinone electron acceptors of PSII, respectively; Zhu et al., 2005). After normalizing the curves, the variable fluorescence of phase J increased continuously in the −P group, which was found in both S. costatum (Figure 4A) and P. globosa (Figure 4B), suggesting a reduction in the rate of QB-mediated QA− reoxidation (Haldimann et al., 1995). In addition, the increase in the K-step (300 μs) was pronounced in cells after P limitation in both S. costatum (Figure 4C) and P. globosa (Figure 4D), indicating OEC damage (Srivastava et al., 1997).
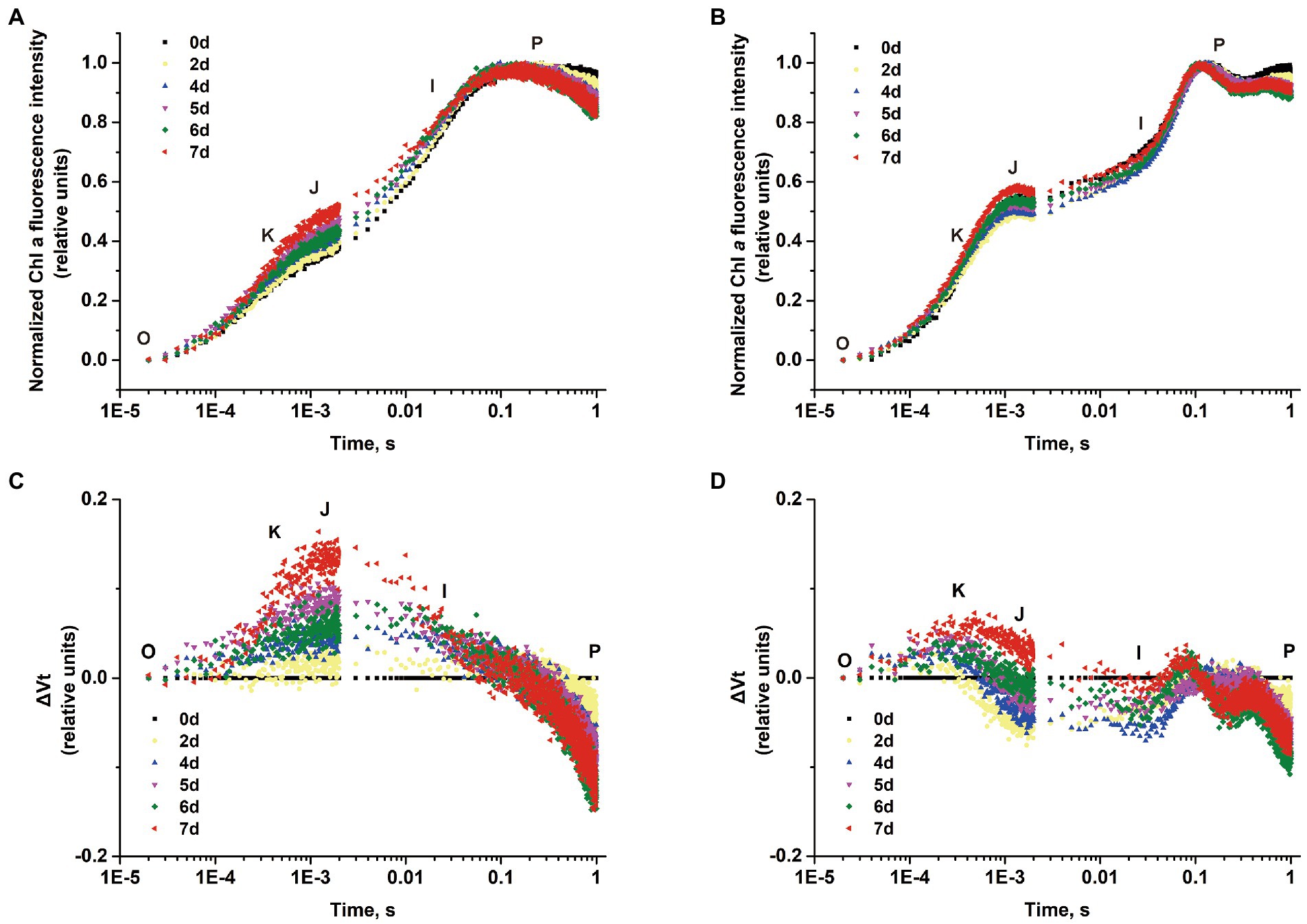
Figure 4. Normalized chlorophyll a fluorescence transient of S. costatum (A) and P. globosa (B) in −P group for various periods of time plotted by the mean of triplicate (Error bars omitted). Differential curves of ΔVt (obtained by subtracting the curve on day 0 from the samples for various periods of time) in S. costatum (C) and P. globosa (D) under P-limited condition.
φE0, RE0/RC, and RC/CS0 values were markedly decreased by P limitation compared with the corresponding values on day 0 in S. costatum (Figure 5A, p < 0.05, ANOVA) but not in P. globosa (Figure 5B, p > 0.05, ANOVA). Therefore, P limitation decreased the quantum yield of electron transport to intersystem electron acceptors and the electron flux for reducing terminal electron acceptors on the PSI side in S. costatum rather than P. globosa (Lin et al., 2009). In addition, the amount of active PSII RCs per excited CS decreased by P limitation in S. costatum (Lin et al., 2009).
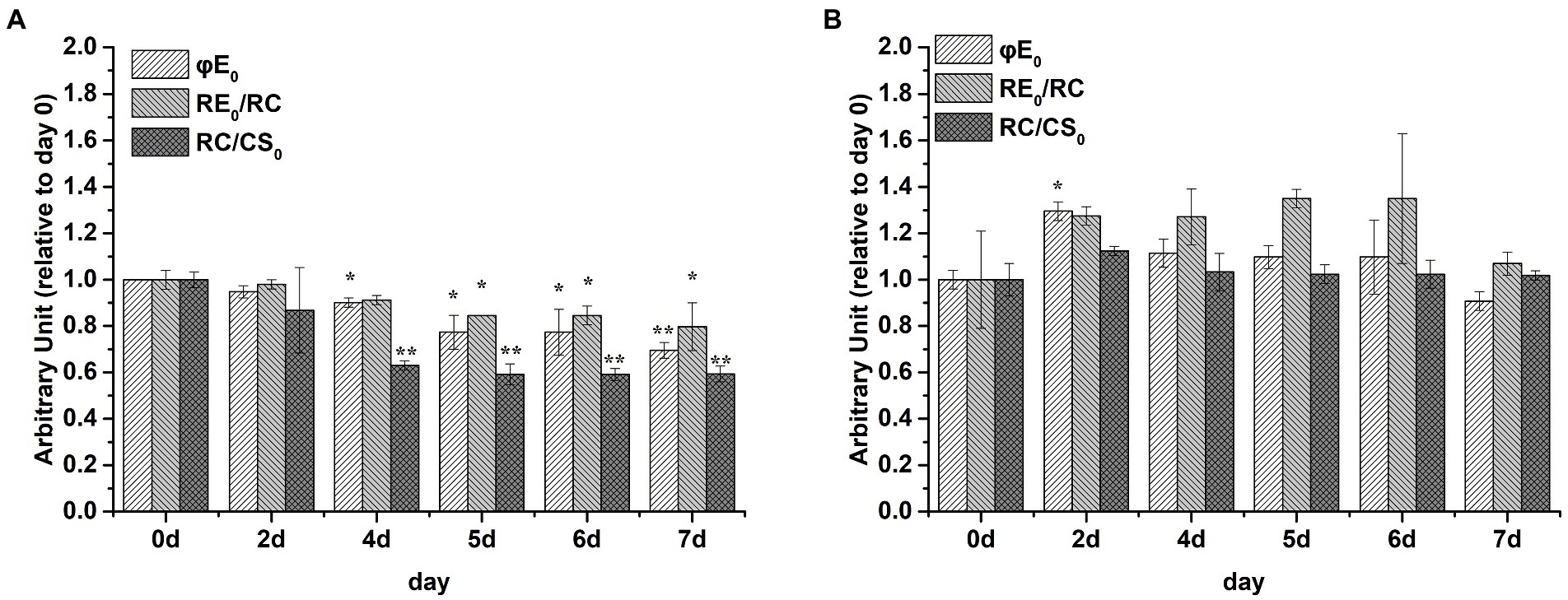
Figure 5. Photosynthetic parameters (relative to day 0) derived by the JIP-test of S. costatum (A) and P. globosa (B) under P-limited condition. φE0 = ET0/ABS = (Fv/Fm) × (1 – VJ), which is the quantum yield of electron transport to intersystem electron acceptors at t = 0; RE0/RC = (RE0/ET0) – (ET0/RC), denoting the reduction in end acceptors on the PSI electron acceptor side per RC at t = 0; RC/CS0 = φP0 × (ABS/CS0) × (VJ/M0), reflecting the amount of active PSII RCs per CS at t = 0. Error bars are mean ± standard error (SE) of triplicate treatments. Superscripts indicate significant differences between +P group and − P group according to a two-way ANOVA with a post-hoc test (**p < 0.01, *p < 0.05).
3.2.4. Effects of P limitation on QA− reoxidation kinetics
To verify the possibility of QA− reoxidation being retarded in response to P limitation, the QA− reoxidation kinetics test was performed. The curves were fitted by three-exponential decay without DCMU. The QA− reoxidation kinetic curves of S. costatum and P. globosa in the +P and − P groups for different periods are shown in Figure 6. The parameters of QA− reoxidation kinetics in cells exposed to P limitation are summarized in Table 1. P limitation increased the decay half-life of the fast phase (T1) in S. costatum, and it increased from 714.1 ± 58.4 μs on day 0 to 1010.0 ± 154.0 μs on day 6 in the −P group (Table 1, p < 0.05, ANOVA). For P. globosa, the decay half-life of the fast phase (T1) increased from 526.0 ± 17.0 μs on day 0 to 871.0 μs on day 6 (Table 1, p < 0.05, ANOVA). Therefore, P limitation retards QB− mediated QA− reoxidation in both S. costatum and P. globosa (Zhang et al., 2017), which is in accordance with the observed changes of phase J (Figure 4).
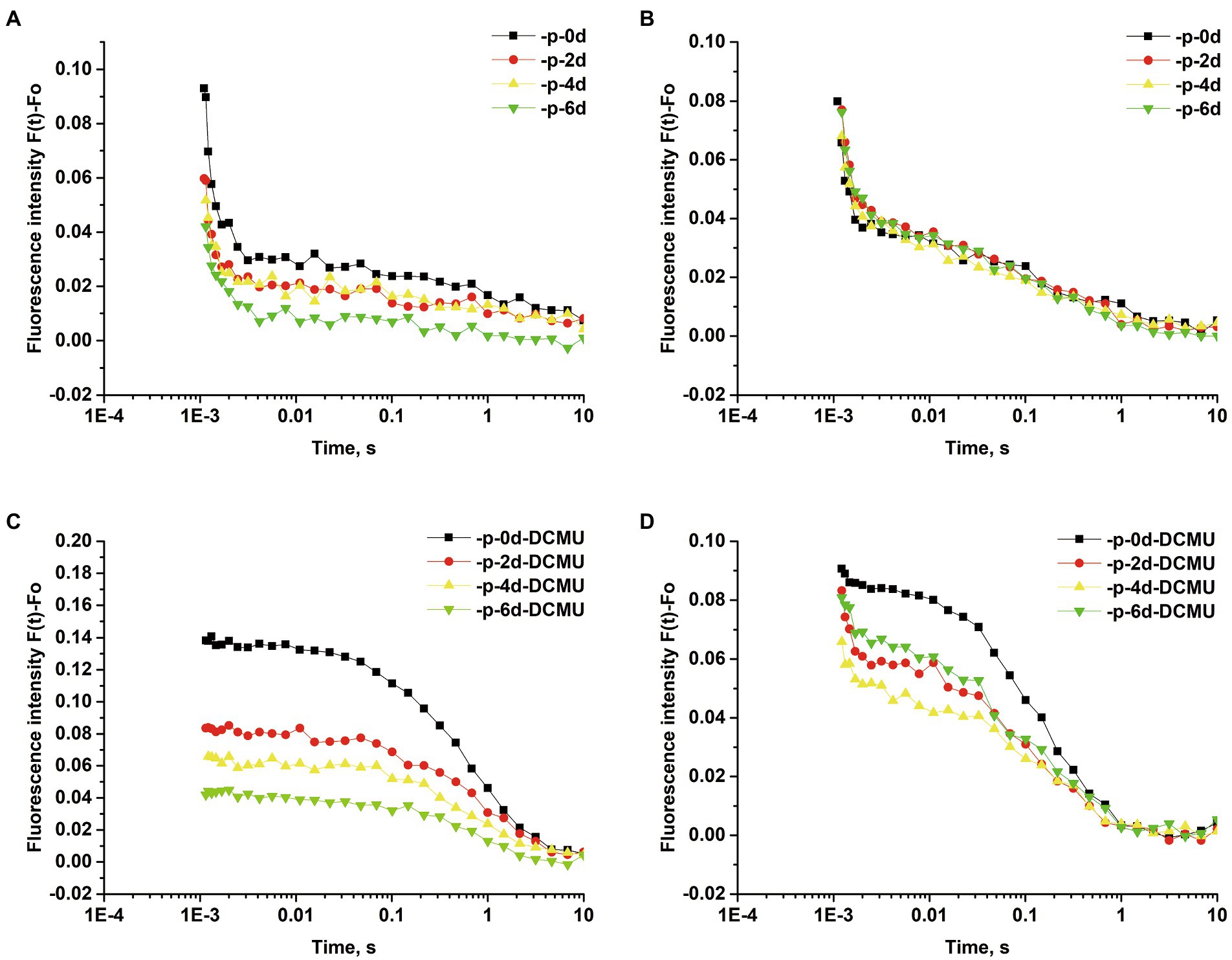
Figure 6. Normalized QA− reoxidation kinetic curves of S. costatum and P. globosa in +P and −P groups for various periods of time. Fluorescence decay in the absence of 3-(3,4-dichlorophenyl)-1,1-dimethylurea (DCMU) of S. costatum (A) and P. globosa (B). Fluorescence decay in the presence of 20 μM DCMU of S. costatum (C) and P. globosa (D). Each value represents the mean of the results from triplicates (Error bars omitted).
The curves were fitted by the single exponential decay under the presence of 20 μM DCMU. For S. costatum, there was a significant increase from 0.71 ± 0.03 μs (day 0) to 0.91 ± 0.07 μs (day 2) in the time constant for the slow phase (T3; p < 0.05, ANOVA), and the increase in the non-decaying component (A0) of fluorescence after P limitation (Table 1), which increased from 40.90% (day 0) to 42.34% (day 2), indicating that QA− was restricted to recombine with the S2 state after only 2 days of P-limited culture in S. costatum cells. However, no significant increase in the slow phase (T3) was observed in P. globosa, and the non-decaying component (A0) of fluorescence increased slightly from 38.56% (day 0) to 38.94% (day 6) under P limitation (Table 1).
3.2.5. Effects of P limitation on the proportion of PSIIX centers
Intracellular PSII exists in different forms, which is the so-called heterogeneity. According to the electron transfer capacity, PSII can be divided into PSIIA (active PSII center) and PSIIX (inactive PSII center; Lavergne and Leci, 1993). In PSIIA centers, the oxidation of QA− is rapid, whereas in PSIIX centers, the oxidation of QA− is much slower (Chylla and Whitmarsh, 1989). The proportion of PSIIX in S. costatum and P. globosa cells treated with +P and − P for various periods of time was measured by the S-state test (Supplementary Figure S3). The proportion of PSIIX centers increased in S. costatum under P limitation, which significantly increased from 18.34 ± 2.26% to 27.17 ± 0.38% after 7 days of P limitation (p < 0.01, Figure 7A, ANOVA), indicating that the proportion of QB that cannot oxidize QA− increased. Similarly, P limitation had significant effects on the proportion of PSIIX centers in P. globosa, which increased from 14.65 ± 0.92% on day 0 to 24.54 ± 1.53% on day 4 (Figure 7B, p < 0.01, ANOVA).
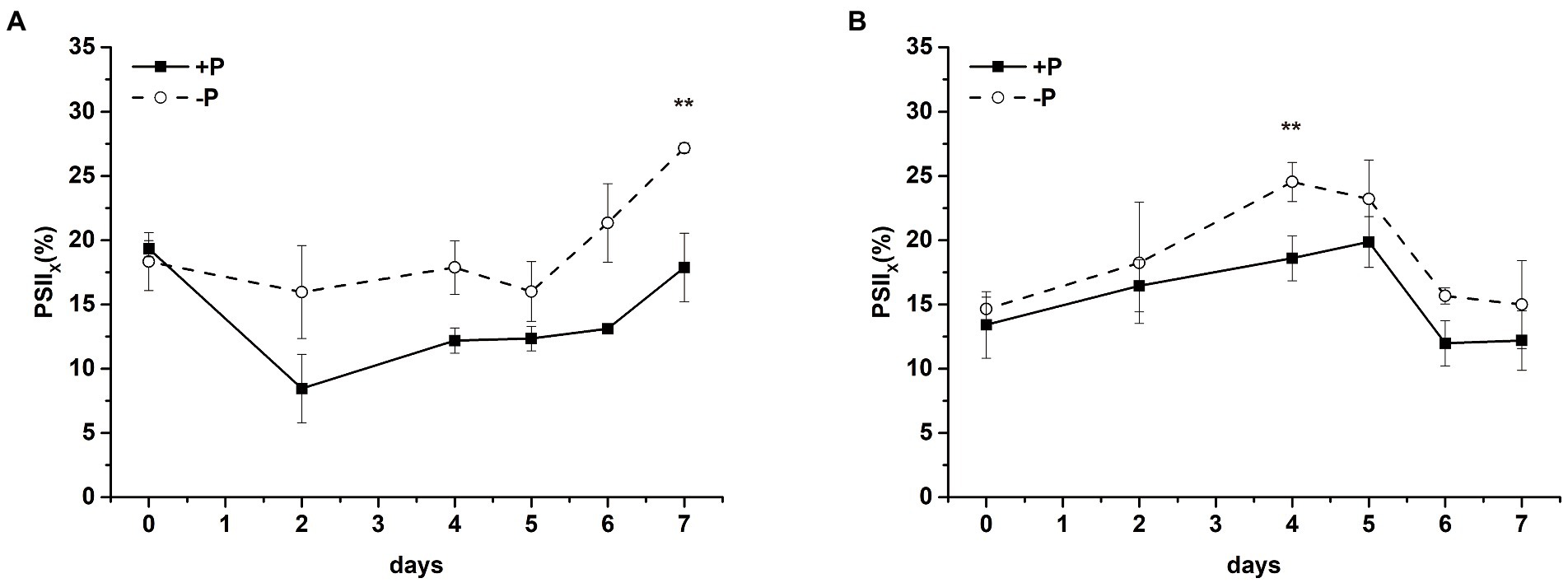
Figure 7. Proportion of PSIIX centers of S. costatum (A) and P. globosa (B) in +P and −P groups for various periods of time. Error bars show the mean ± standard error (SE) of triplicate treatments. Superscripts indicate significant differences between +P group and −P group according to a two-way ANOVA with a post-hoc test (**p < 0.01).
3.3. Effects of P limitation on their photosystem II proteins of Skeletonema costatum and Phaeocystis globosa
To investigate if the decreased PSII activity under P limitation was associated with the decrease in the PSII protein level, the effects of P limitation on PSII subunits D1 and PsbO were further examined. The decrease in D1 proteins in S. costatum and P. globosa in the +P group (Figures 8A,C) could be explained by the gradual consumption of nutrient elements in batch culture experiments (Keren et al., 1997). P limitation results in 100% loss of the D1 protein in S. costatum in 4 days (Figures 8A,C, p < 0.01, ANOVA). However, a 60% decrease was found in the D1 level of P. globosa under P limitation for 6 days (Figures 8A,C, p < 0.05, ANOVA). The PsbO level was stable at 25% of the original value in the later stage of growth under P limitation in P. globosa. However, a 100% decrease in PsbO protein level was observed in S. costatum after 2 days of P limitation (Figures 8B,D, p < 0.01, ANOVA). Therefore, P limitation resulted in a larger and faster decrease in D1 and PsbO in S. costatum than in P. globosa.
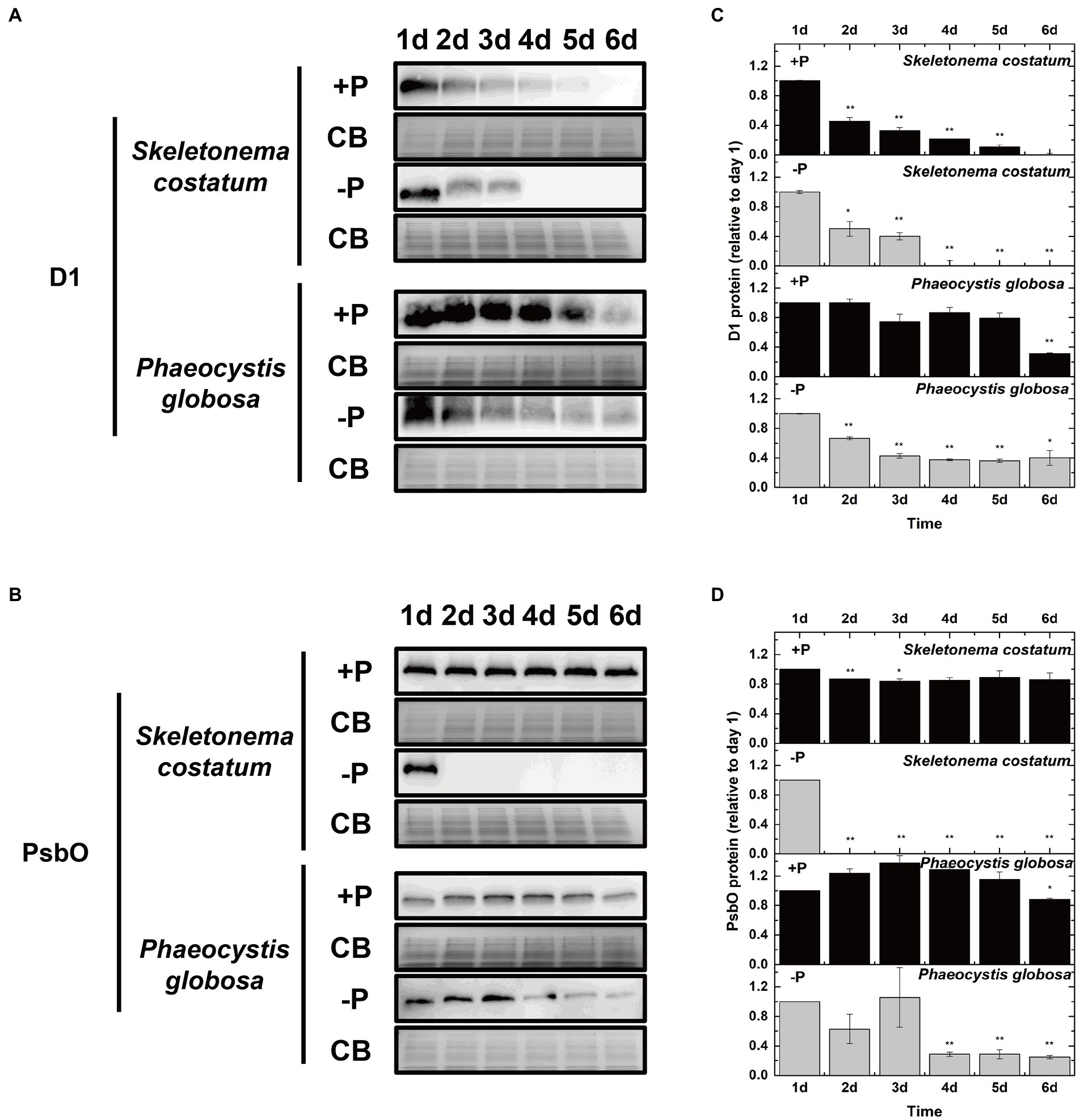
Figure 8. Immunoblot analysis of PSII proteins and stained with Coomassie blue (CB). (A) Immunoblot analysis was performed using antibodies specific to D1. (B) Immunoblot analysis was performed using an antibody specific to PsbO. (C) Ratios of the D1 protein level of P-limited cells were semi-quantitated by the immunoblot analysis relative to that of cells on day 1, which was set to 100% for easy comparison. (D) Ratios of the PsbO protein level of P-limited cells were semi-quantitated by the immunoblot analysis relative to that of cells on day 1, which was set to 100% for easy comparison. Error bars show mean ± standard error (SE) of triplicate treatments. Superscripts indicate significant differences between day 1 and other days according to a two-way ANOVA with a post-hoc test (**p < 0.01, *p < 0.05).
3.4. P limitation on their photosystem II repair in Skeletonema costatum and Phaeocystis globosa under P limitation
Cells can repair damaged PSII by replacing photo-damaged proteins in PSII with newly synthesized subunits (Nixon et al., 2010; Komenda et al., 2012). Wu et al. (2012) blocked the counteracting repair processes by adding lincomycin to estimate the newly synthesized D1 protein in PSII repair. To further investigate if stable PSII activity under P limitation in P. globosa was associated with PSII repair, the newly synthesized D1 was plotted and compared. In both S. costatum and P. globosa, treatment with lincomycin elicited a greater drop in the D1 protein level in the absence of P than in the presence of P (Figures 9A–C). The presence of lincomycin resulted in a greater loss of the D1 protein in S. costatum than in P. globosa under both P-repleted and P-limited conditions (Figures 9A–C). Without lincomycin, the loss of D1 protein content is still higher in S. costatum than in P. globosa under both two conditions (Figures 9A–C). These results indicated that the PSII was impaired more seriously in S. costatum than in P. globosa under both conditions. When comparing the newly synthesized D1 protein between P-limited and P-repleted conditions, we found that the newly synthesized D1 protein decreased in S. costatum (Figure 9D, p < 0.05, ANOVA) while increased in P. globosa after P limitation (Figure 9E, p < 0.01, ANOVA), These results suggested that P limitation inhibited the PSII repair in S. costatum but accelerated it in P. globosa.
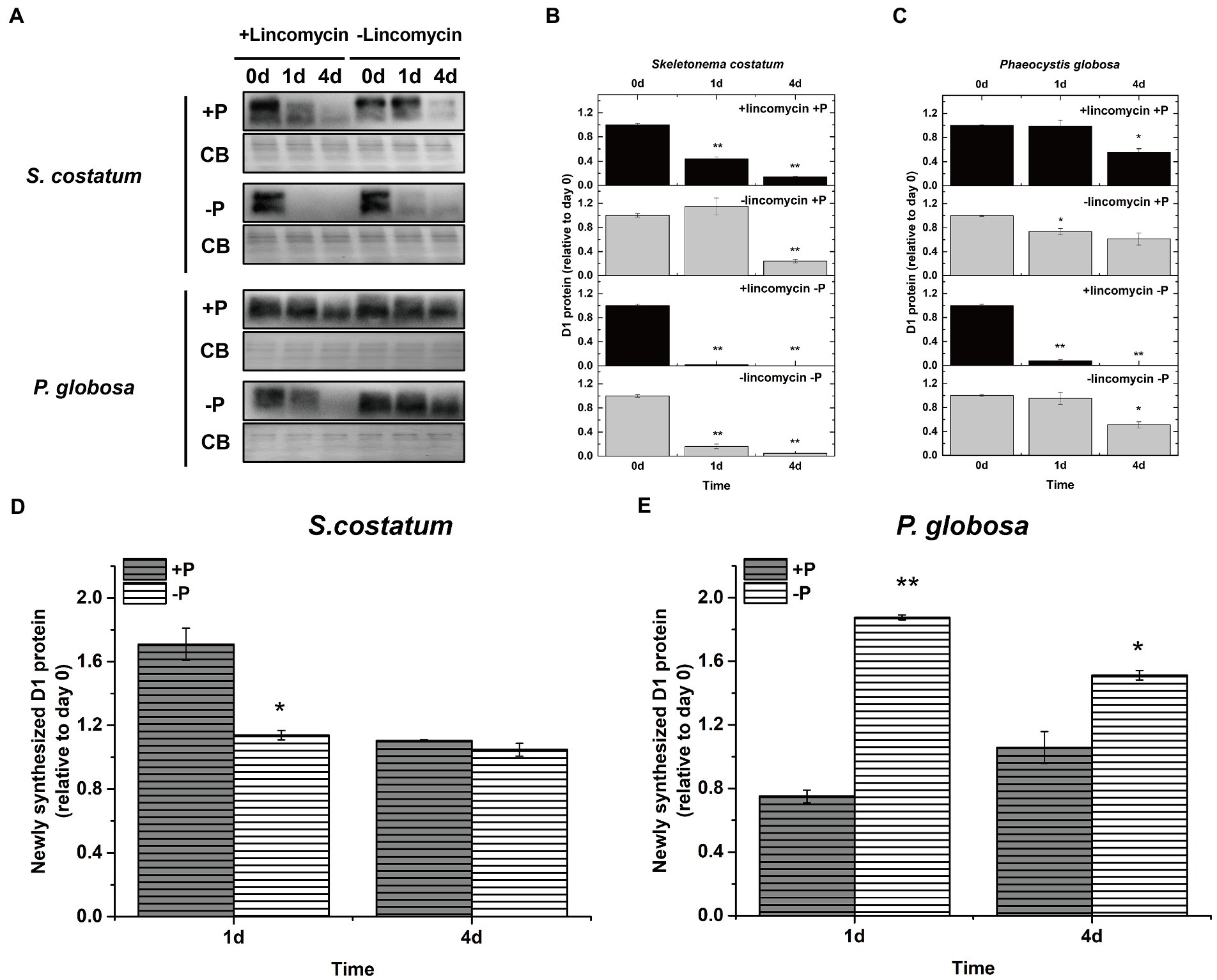
Figure 9. (A) Immunoblot analysis of D1 protein levels in S. costatum and P. globosa cultures treated with or without lincomycin in +P and −P groups. The blot was stained with Coomassie blue (CB). (B) Ratios of the D1 protein level of S. costatum cells semi-quantitated by the immunoblot analysis relative to that of cells on day 0, which was set to 100% for easy comparison. (C) Ratios of the D1 protein level of P. globosa cells semi-quantitated by the immunoblot analysis relative to that of cells on day 0. (D) Newly synthesized D1 protein (relative to day 0) in S. costatum in +P and −P groups. Newly synthesized D1 in PSII repair was measured as the change in the active D1 content in the absence of lincomycin (PSII repair active) minus the change in the active D1 content in the presence of lincomycin (PSII repair blocked). (E) Newly synthesized D1 protein (relative to day 0) in P. globosa in +P and −P groups. Newly synthesized D1 in PSII repair was measured as the change in the active D1 content in the absence of lincomycin (PSII repair active) minus the change in the active D1 content in the presence of lincomycin (PSII repair blocked). Error bars are mean ± SE of triplicate treatments. Superscripts indicate significant differences according to a two-way ANOVA with a post-hoc test (B,C: day 0 vs. other days; D,E: +P group vs.−P group. **p < 0.01, *p < 0.05).
3.5. Non-photochemical quenching induction in Skeletonema costatum and Phaeocystis globosa under P limitation
To explore whether S. costatum and P. globosa enhance their NPQ activity under P limitation to dissipate excess light as heat for protection from potential photo-oxidative damage, the NPQ values were obtained from the slow induction kinetic curves of S. costatum and P. globosa in the +P and − P groups for various periods of time (Supplementary Figure S4). The NPQ value of the −P group was significantly higher than that of the +P group, which was expressed in P. globosa (p < 0.05, ANOVA, Figure 10B), indicating that P limitation induced higher NPQ. The NPQ values of S. costatum were maintained at a low level for various periods, and the increase was not significant in the −P group compared with the +P group (p > 0.05, ANOVA, Figure 10A). Notably, P. globosa had >5-fold higher NPQ relative to the S. costatum at the same actinic light of 321 μmol photons m−2 s−1 red light (Figure 10), suggesting that the NPQ capacity of P. globosa cell was higher than that of S. costatum. Considering that the NPQ prevents photodamage of the D1 protein in PSII (Lepetit et al., 2012), we suggested that the above more active D1 turnover (Figure 9E) and stable PSII activity (Figure 3B) were due to the higher NPQ capacity P. globosa under P limitation.
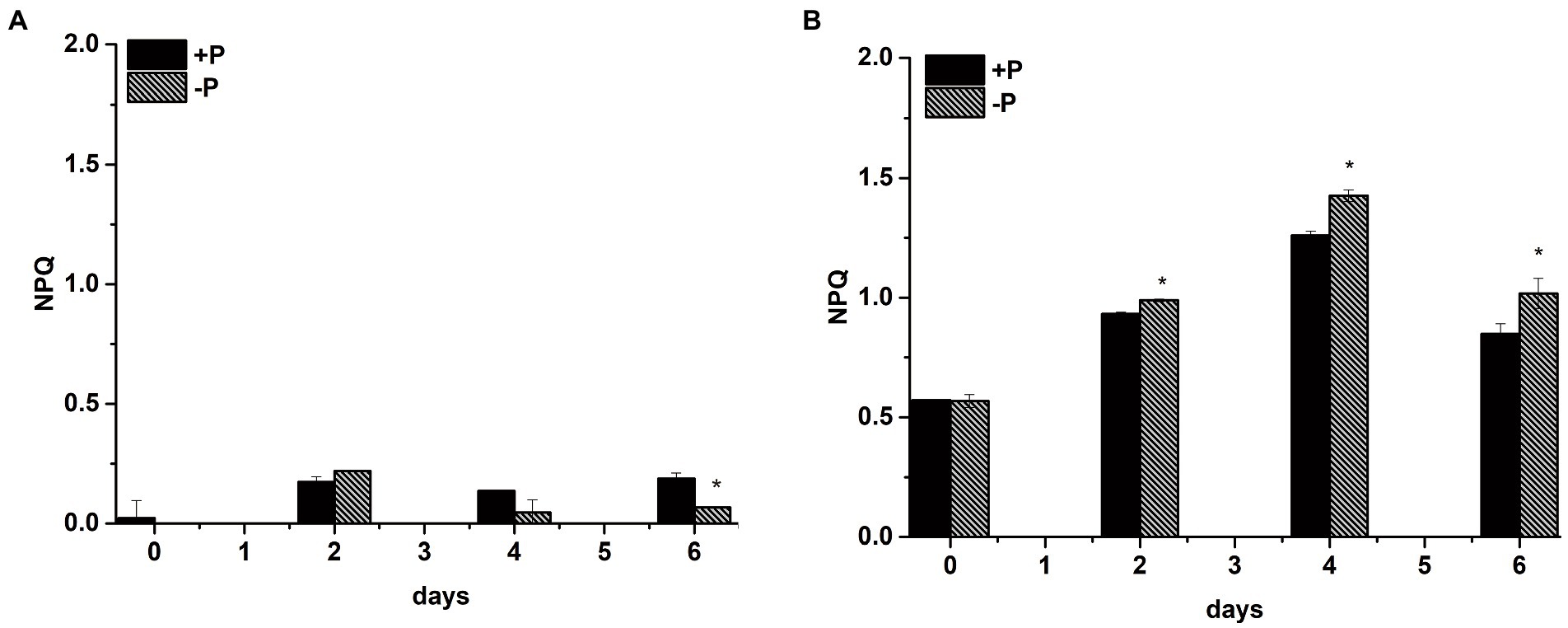
Figure 10. Non-photochemical quenching (NPQ) capacity of S. costatum (A) and P. globosa (B) in +P and −P groups. Error bars show the mean ± standard error (SE) of triplicate treatments. Superscripts indicate significant differences between +P group and −P group according to a two-way ANOVA with a post-hoc test (*p < 0.05).
4. Discussion
In this study, photosynthetic responses of the diatom S. costatum and the haptophyte P. globosa under P limitation were compared. S. costatum cells could not proliferate after P limitation with the impairment of OEC on the donor side, the inhibition of electron transport from QA− to QB, and the inability to provide electrons downstream of PSII. The fast and complete decrease in D1 and PsbO in S. costatum were associated with its enhanced photoinhibition. However, when exposed to P limitation, P. globosa could grow and retain its photosynthetic activity for at least 1 week with minor impairment of PSII function compared with S. costatum. In particular, the photosynthetic acclimation mechanisms adopted by P. globosa under P limitation to avoid greater photoinhibition of PSII were highlighted.
4.1. Effects of P limitation on growth and their photosystem II activity in Skeletonema costatum and Phaeocystis globosa
Phaeocystis globosa blooms usually occur after diatom blooms in winter to early spring in the SCS and similar trends were also observed in the coastal waters of high latitude North Sea (Lancelot et al., 2005). Karasiewicz et al. (2018) suggested both abiotic and biotic interactions favored Phaeocystis blooms with great contribution from the preceding diatoms. The silicate exhaustion has historically been thought to be a major explanation for the appearance of P. globosa (Lancelot et al., 1987; Reid et al., 1990). The role of P in the transition of phytoplankton communities was reviewed by Lin et al. (2016).
P is an essential nutrient for the growth and proliferation of phytoplankton. P limitation inhibited the growth of S. costatum remarkably but had no obvious effect on the growth of P. globosa over a period of 7 days (Figure 1), which strongly suggested that P. globosa has more tolerance to P limitation compared with S. costatum. Moreover, the PSII activity of S. costatum was inhibited by P limitation, whereas P. globosa maintained photosynthetic activity after P limitation based on the estimation of Fv/Fm and other chlorophyll fluorescence parameters (Figure 2). The obvious growth and photosynthetic activity inhibition of P limitation in S. costatum are in accordance with those reported previously (Zhang et al., 2016).
In this study, the pre-incubation in P-free medium before this experiment was not done since S. costatum cannot keep photosynthetic activity after the pre-incubation. P pools associated with cells were identified previously as intracellular P pool and cell surface-adsorbed P pool (Jin et al., 2021). Both S. costatum and P. globosa can take up P in excess of its immediate cellular demand under P-sufficient conditions and store it in the form of PolyP, so-called luxury uptake, and break down this PolyP store upon P stress (Schoemann et al., 2001; Solovchenko et al., 2019). As shown in Supplementary Figure S1, both of them have the similar ability to store PolyP. However, the maintenance of growth and photosynthetic activity in P. globosa under P limitation might be caused by its higher tolerance to the P limitation as it still kept a stable photosynthetic activity after 25 days of P limitation (Supplementary Figure S2).
Photosynthesis can be divided into the light reactions, in which light energy is stored as ATP and NADPH, and the dark reactions, in which the products of the light reactions are used to reduce inorganic C (Green and Durnford, 1996). Phosphorus is an essential element in compounds such as ATP, NADPH, nucleic acids, phospholipids and sugar phosphates, all of which play important roles in photosynthesis. Numerous groups have demonstrated that P limitation causes a decline in photosynthesis in a number of different ways (Heraud et al., 2005; Shelly et al., 2005). It was proposed that a decreased supply of Pi reduced the ATP synthesis/levels, thereby, reducing both phosphorylation and CO2 assimilation (Lewis et al., 1994; Campbell and Sage, 2006). Other studies also reported that the photosynthetic machinery composition or electron transport activity was also affected under P-limited condition (Van Rensen and Vredenberg, 2009; Carstensen et al., 2018). Wykoff et al. (1998) reported that P deprivation seriously inhibited the PSII activity but not that of PSI in C. reinhardtii. In this study, we mainly focused on the performance of PSII.
4.2. Different performance of P limitation on their photosystem II in Skeletonema costatum and Phaeocystis globosa under P limitation
P limitation on their photosystem II, a multiprotein complex present in the thylakoid membranes of oxygenic photosynthetic organisms, is the center of photosynthesis that uses light to drive water oxidation and PQ reduction (Iwata and Barber, 2004). PSII is artificially divided into the donor sides (OEC), reaction centers, and acceptor sides. The donor sides split water and evolve oxygen (Wilson and Jain, 2018). The reaction center of PSII carries out photochemical reactions, including the primary charge separation and subsequent electron transfer from water to PQ (Semenov et al., 2011). On the electron acceptor side of PSII, QA (a one-electron acceptor PQ) takes up one electron from PheD1− (a pheophytin molecule) and transfers it to QB (a two-electron acceptor PQ; Zhang et al., 2017). In this study, various chlorophyll fluorescence tools were used to examine the physiological response to P limitation in and around PSII.
P limitation inhibited electron transfer from QA− to QB in S. costatum and P. globosa, which was suggested by the increase in the fluorescence intensity of phase J (Figures 4A,B), the increase in the decay half-life of the fast phase obtained from the QA− reoxidation kinetics test (Table 1) and the increase in the proportion of PSIIX centers (Figures 7A,B). The increase in PSIIX centers, also called QB-nonreducing PSII centers, under P limitation was also observed in Chlamydomonas reinhardtii, Chlorella, and Scenedesmus (Wykoff et al., 1998; Dao and Beardall, 2016; Markou et al., 2017). These results provide circumstantial evidence that both S. costatum and P. globosa accumulate inactive PSII unable to reduce PQ under P limitation, which might be a way to acclimate to abiotic stress including P starvation. Previous studies have found that these centers are resistant to photoinhibition and capable of facilitating excess excitation energy dissipation for photosynthetic organisms under abiotic stress conditions (Neale and Melis, 1990; Hill and Ralph, 2006). A higher concentration of QB-nonreducing PSII centers was also shown in the triazine-resistant plant Chenopodium album (Van Rensen and Vredenberg, 2009).
The impairment of the donor side in PSII by P limitation has been reported in wheat, rice, citrus, and other higher plants (Lin et al., 2009; Veronica et al., 2017; Meng et al., 2021). From the QA− reoxidation kinetics test in the presence of DCMU (Table 1), the donor side of PSII in S. costatum was damaged after exposure to P limitation for 2 days. These results are in accordance with the observed positive K-step in the OJIP transients (Figure 4C) and a sharp decrease in RC/CS0 (Figure 5A) of S. costatum under P limitation. However, because the changes in these parameters resulting from P limitation were small or even negligible in P. globosa, the donor side of PSII in P. globosa might be damaged by P limitation but not seriously. The fact that P limitation caused more serious damage to OEC in S. costatum than in P. globosa was further confirmed by the different PsbO expression patterns in the two species under P limitation. PsbO is an extrinsic protein that plays a crucial role in the structure and function of the OEC of PSII (Popelkova and Yocum, 2011). Jain et al. (2005) reported that P limitation suppresses the expression of psbO (PsbO protein encoding gene) in Arabidopsis thaliana, thus impairing the structural integrity of OEC and consequently its function. In this study, a 100% decrease of PsbO in S. costatum was observed after exposure to P limitation for 2 days, which was in accordance with the physiological result that P limitation resulted in a serious impairment of the donor side in PSII in S. costatum. However, PsbO in P. globosa showed a slow decrease and remained at a certain level in the later stage of culture under P limitation (Figures 8B,D), reflecting smaller and slighter damage of OEC caused by P limitation in P. globosa.
Impairment of PSII finally led to the inhibition of electron transport to PSI. P limitation inhibiting electron transport to PSI has been demonstrated in barley (Carstensen et al., 2018) and dinoflagellate Peridinium bipes revealed by the decrease in φE0 and RE0/RC (Yang et al., 2020). P limitation resulted in the gradual and significant decline in φE0 and RE0/RC values in S. costatum (Figure 5A) rather than in P. globosa. These results confirmed that the P limitation only leads to the inhibition of electron transport after QA− to the PSI electron acceptor side of S. costatum. However, the electron transport to PSI in P. globosa was not affected by P limitation (Figure 5B).
Thus, P limitation caused serious PSII damage with obvious impairment of both donor and acceptor sides of PSII in S. costatum; P. globosa under P limitation showed minor impairment of OEC of PSII compared with S. costatum and no obvious inhibition of electron transport to PSI. D1 constitutes the core of the PSII reaction center. PSII inactivation in C. reinhardtii subjected to P deprivation is the result of enhanced photoinhibition shown by enhanced D1 degradation (Wykoff et al., 1998; Malnoe et al., 2014). In this study, P limitation resulted in a much faster and larger decrease in D1 in S. costatum than in P. globosa, which is associated with the worse performance of PSII in the former than in the latter.
4.3. Regulation of D1 turnover and non-photochemical quenching in Skeletonema costatum and Phaeocystis globosa under P limitation
PSII repair involves partial disassembly of the damaged complex, selective proteolytic degradation, and replacement of the damaged subunit (predominantly the D1 reaction center subunit) by de novo synthesized copy and reassembly. Turnover of the D1 protein is required for PSII repair and restoration of PSII photochemical activity after photoinhibition (Nixon et al., 2010). P limitation causes photodamage of PSII in S. costatum by not only directly accelerating photoinactivation but also inhibiting D1 restoration. Inhibited D1 restoration under P starvation has also been reported in D. tertiolecta (Heraud et al., 2005). This may be because protein synthesis (protein turnover) is energetically costly, requiring 10.8 ATP per peptide bond, and P limitation often causes a decline in nucleotides such as ATP and GTP (Murata and Nishiyama, 2018). In contrast, P. globosa under P limitation showed increased D1 restoration obtaining a smaller decrease in the D1 level compared with S. costatum. It remains to be explained how P. globosa synthesizes a high amount of D1 under P limitation. P. globosa still maintains active D1 synthesis while ceasing synthesis of most other proteins, including the photosynthetic apparatus subunits in P limitation (Feng et al., 2015). Under unfavorable P-limited conditions, P. globosa invests much more energy in optimization and maintaining of light harvesting (such as de novo D1 synthesis), but not under P-replete conditions. This flexibility of P. globosa provides an advantage in the fluctuating environment. Similarly, the acceleration of D1 synthesis has been reported in Emiliania huxleyi under short-term P depletion (Loebl et al., 2010) and in the cyanobacterium Synechocystis sp. PCC 6803 under oxidative stress (Yu et al., 2014). Loebl et al. (2010) also found that the superior ability of PSII repair in E. huxleyi helps maintain significant PSII function over at least 38 days under nitrogen depletion. Undoubtedly, P. globosa, but not S. costatum, could counteract photoinactivation by the acceleration of PSII repair, thus maintaining stable photosynthetic activity under P limitation.
The photosynthetic apparatus showed higher resistance in P. globosa, which might also be caused by more effective energy dissipative mechanisms. NPQ allows for the safe dissipation of excess excitation energy as heat in light-harvesting antenna complexes (LHCs) of PSII and prevents over-reduction in the electron transport chain that may lead to reactive oxygen species (ROS) production (Muller et al., 2001). It thereby prevents photodamage of the D1 protein in PSII, which is the primary target of ROS-generated oxidative stress (Wu et al., 2011; Lepetit et al., 2012). The main part of NPQ in microalgae is called qE, which relies on the interconversion of specific pigments by the xanthophyll cycle. The xanthophyll cycle in green and brown algae is composed of violaxanthin, antheraxanthin, and zeaxanthin (VAZ cycle), while in dinoflagellates, diatoms, and haptophytes, it consists of diadinoxanthin and diatoxanthin (Dd-Dt cycle; Eisenstadt et al., 2008; Goss and Jakob, 2010).
Both P. globosa and S. costatum possess the Dd-Dt cycle and can promote NPQ (Lavaud et al., 2002; Harris et al., 2005). From the induction kinetics of NPQ (Figure 10B), P. globosa cells could enhance their NPQ activity as an adaptive strategy under P limitation. This can be explained as follows: P limitation reduces the orthophosphate concentration in the chloroplast stroma and causes the inhibition of ATP synthase activity. Consequently, protons accumulate in the thylakoids and cause a high trans-thylakoidal proton gradient activating the NPQ mechanism (Lavaud et al., 2004; Lavaud and Lepetit, 2013). Enhancement of NPQ is also an adaptive strategy under P limitation in the dinoflagellate Karlodinium veneficum (Cui et al., 2017), diatom Thalassiosira pseudonana (Li et al., 2021), and chlorophytes D. tertiolecta (Petrou et al., 2008) and C. reinhardtii (Wykoff et al., 1998). Indeed, the induction of LHCSR (Light Harvesting Complex Stress Related), which controls the recruitment of NPQ in C. reinhardtii, has also been reported under P deprivation (Moseley et al., 2006). Thus, NPQ, as a photosynthetic mechanism to cope with P limitation, plays an important role in maintaining the balance of the energy budget in P-limited P. globosa cells.
Su et al. (2012) and Lavaud et al. (2016) pointed out that S. costatum had limited capacity for induction of NPQ. Previous studies suggested two molecular explanations for the low NPQ in S. costatum (Lavaud and Lepetit, 2013): (1) a lower amount of Dt molecules involved in NPQ, and (2) lower capacity to form large functionally disconnected oligomeric fucoxanthin–chlorophyll protein complexes caused by the different organization and composition of LHC of PSII. Ultimately, the inability of S. costatum to promote a similarly strong NPQ as in P. globosa leads to a higher susceptibility of S. costatum to photoinhibition during exposure to P limitation.
4.4. Photosynthetic responses to P limitation are associated with the survival, dominance, and succession of marine microalgae under P limitation
P limitation was considered one of the factors favoring the population shift from diatoms or other early succession species to late succession species such as dinoflagellates, haptophytes, and pelagophytes, which are better adapted to this new set of environmental conditions (Lin et al., 2016). Considering that photosynthesis is the most important driver of cell growth and other physiological processes in phytoplankton, these different photosynthetic responses in algal bloom species may also contribute to their collapse or well-being under P limitation, thus affecting the transition of phytoplankton communities from early succession species to late succession species.
Liu et al. (2013) suggested that the photosynthetic capacity of S. costatum was limited by P limitation, and Fv/Fm decreased in S. costatum under P limitation (Li and Sun, 2016). This study demonstrated that P limitation seriously impaired PSII in S. costatum, and this species did not have a strong ability to induce high NPQ and accelerate the PSII repair cycle to decrease photosynthetic impairment resulting from P limitation. From the results of the present study, P. globosa could maintain relatively stable photosynthetic activity under P limitation by inducing high NPQ and accelerating D1 restoration (Figure 11). The photosynthesis activity might affect the P limitation tolerance because the energy sources for Pi uptake and the synthesis of APase in microalgae are mostly derived from photosynthesis (Pandey, 2006; Gorbunov and Falkowski, 2022). The superior photosynthetic performance of P. globosa under P limitation was undoubtedly conducive to its well-being, which is consistent with previous ecological investigations (Wang K. et al., 2021; Wang X. D. et al., 2021).
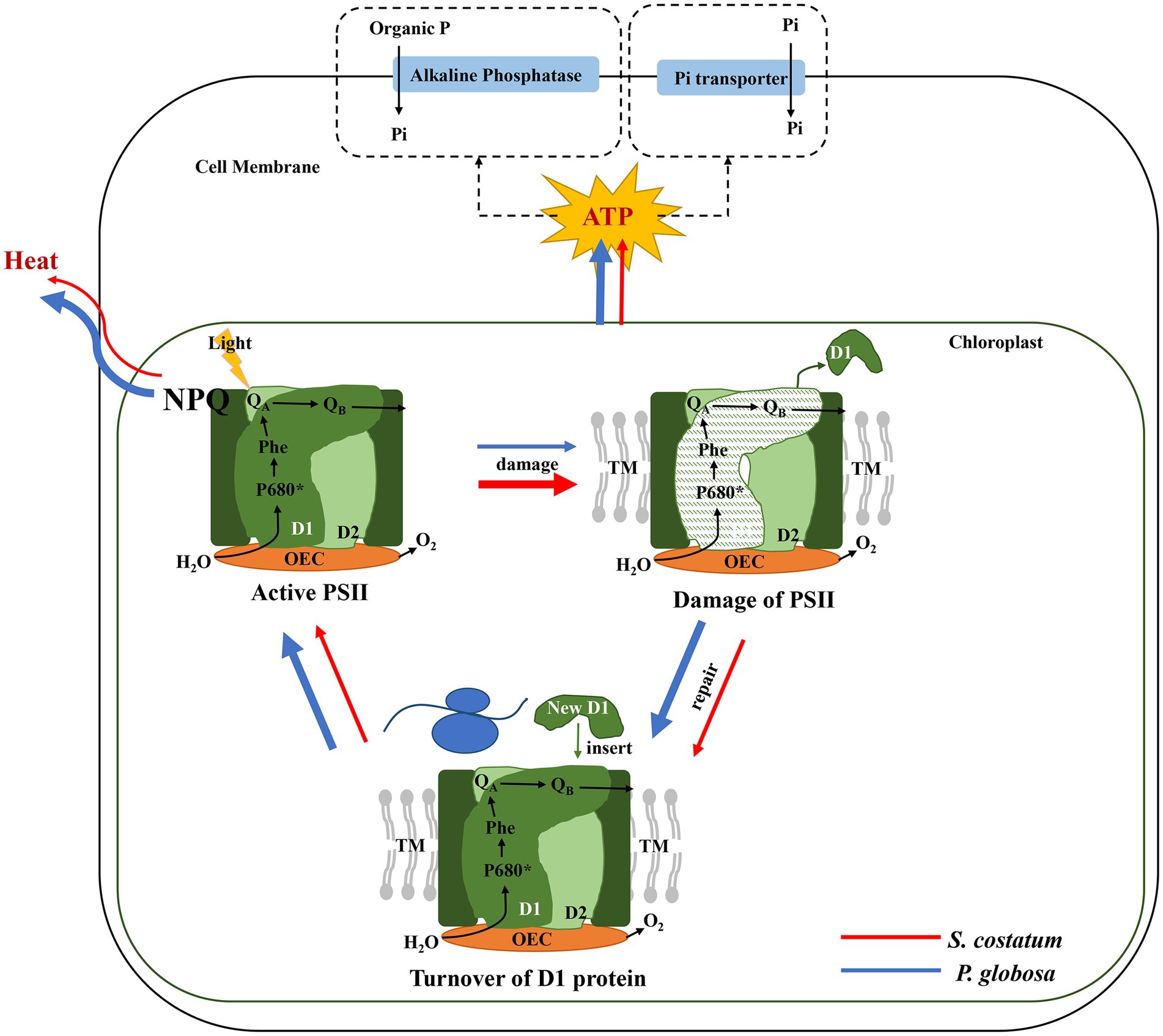
Figure 11. The photosynthetic responses of S. costatum (red line) and P. globosa (blue line) under P limitation (the thickness of the line indicates the strength of the effect).
The succession of other species under P limitation has also been reported to be closely correlated with photosynthetic responses. An apparent bloom-forming species succession with the shifting from diatom blooms (mainly S. costatum) in the early spring to long-lasting and large-scale dinoflagellate blooms dominated by Prorocentrum donghaiense was observed in the coastal waters of the East China Sea (Lu et al., 2022), which is also reported to be in accordance with the different response of S. costatum and P. donghaiense to the phosphate exhaustion (Li et al., 2011; Ou et al., 2020). Although the study about the comparison of photosynthesis response to P limitation of these two strains is still limited, it is clear that P. donghaiense had higher photosynthetic activity and potential than S. costatum under eutrophic but relatively P-limited conditions according to Li and Sun (2016). P limitation is also one of the critical factors allowing the coccolithophorid E. huxleyi to bloom after diatom blooms in temperate coastal and oceanic areas (Tyrrell and Merico, 2004; Lessard et al., 2005; Oguz and Merico, 2006), although these conditions are not mandatory for E. huxleyi blooms (Lessard et al., 2005). Zhao et al. (2015) reported that P limitation resulted in greater damage to the photosynthetic apparatus in Phaeodactylum tricornutum, while E. huxleyi under P limitation showed increased xanthophyll cycle pigment accumulation and more active transformation from diadinoxanthin to diatoxanthin than P. tricornutum. Hence, the different photosynthetic responses to P limitation in some algal bloom species might be associated with their survival, dominance, and succession under P limitation.
5. Conclusion
The photosynthetic responses for the different tolerance to P limitation in the diatom S. costatum and the haptophyte P. globosa were investigated and compared. When exposed to P limitation, S. costatum could not proliferate with obvious impairment of both donor and acceptor sides of PSII, and the inhibition of electron transport to PSI. The fast and complete decrease in the D1 and PsbO levels of S. costatum were associated with its enhanced photoinhibition. On the contrary, P. globosa could decrease susceptibility to photoinactivation of PSII with minor impairment of the donor side and thus the reaction center of PSII compared with S. costatum. By the induction of NPQ and a faster D1 turnover in PSII repair, P. globosa avoided further photoinhibition and maintained its stable photosynthetic capacity under P limitation. In addition, although not specifically investigated in this study, the different photosynthetic responses to P limitation in some algal bloom species might be crucial to explain their survival, dominance, and succession under P limitation. Overall, these findings can enhance understanding of the effect on the photosynthetic apparatus of PSII by P limitation in marine algae, elucidate the photosynthetic responses for superior tolerance to P limitation in P. globosa than in S. costatum when cells undergo P limitation, and provide additional evidence explaining the transition of phytoplankton communities from diatom to late succession haptophytes.
Data availability statement
The original contributions presented in the study are included in the article/Supplementary material, further inquiries can be directed to the corresponding author.
Author contributions
LS conceived and initiated the project. XC performed all the experiments and analyzed the data. JZ and LS designed and supervised all the experiments. LZ helped to isolate the strains used in the manuscript. JL provided resources and methodology. JZ and XC wrote the article. All authors provided comments and approved the final version of the article.
Funding
This work was supported by the Funds for International Cooperation and Exchange of the National Natural Science Foundation of China (Grant No. 3201101157), National Key Research and Development Program of China (Grant No. 2016YFE0202100), and National Natural Science Foundation of China (Grant No. 32270136).
Conflict of interest
The authors declare that they have no known competing financial interests or personal relationships that could have appeared to influence the work reported in this paper.
Publisher’s note
All claims expressed in this article are solely those of the authors and do not necessarily represent those of their affiliated organizations, or those of the publisher, the editors and the reviewers. Any product that may be evaluated in this article, or claim that may be made by its manufacturer, is not guaranteed or endorsed by the publisher.
Supplementary material
The Supplementary material for this article can be found online at: https://www.frontiersin.org/articles/10.3389/fmicb.2023.1085176/full#supplementary-material
References
Allen, M. D., Kropat, J., Tottey, S., Del Campo, J. A., and Merchant, S. S. (2007). Manganese deficiency in Chlamydomonas results in loss of photosystem II and MnSOD function, sensitivity to peroxides, and secondary phosphorus and iron deficiency. Plant Physiol. 143, 263–277. doi: 10.1104/pp.106.088609
Armbrust, E. V. (2009). The life of diatoms in the world's oceans. Nature 459, 185–192. doi: 10.1038/nature08057
Beauchemkin, R., Gauthier, A., Harnois, J., Boisvert, S., Govindachary, S., and Carpentier, R. (2007). Spen-nine and spermidine inhibition of photosystem II: disassembly of the oxygen evolving complex and consequent perturbation in electron donation from Tyrz to P680(+) and the quinone acceptors Q(a)(−) to Q(B). BBA-Bioenergetics 1767, 905–912. doi: 10.1016/j.bbabio.2007.04.001
Bilger, W., and Bjorkman, O. (1990). Role of the xanthophyll cycle in photoprotection elucidated by measurements of light-induced absorbency changes, fluorescence and photosynthesis in leaves of Hedera canariensis. Photosynth. Res. 25, 173–185. doi: 10.1007/Bf00033159
Burson, A., Stomp, M., Akil, L., Brussaard, C. P. D., and Huisman, J. (2016). Unbalanced reduction of nutrient loads has created an offshore gradient from phosphorus to nitrogen limitation in the North Sea. Limnol. Oceanogr. 61, 869–888. doi: 10.1002/lno.10257
Cadee, G. C., and Hegeman, J. (2002). Phytoplankton in the Marsdiep at the end of the 20th century; 30 years monitoring biomass, primary production, and Phaeocystis blooms. J. Sea Res. 48, 97–110. doi: 10.1016/S1385-1101(02)00161-2
Campbell, C. D., and Sage, R. F. (2006). Interactions between the effects of atmospheric CO2 content and P nutrition on photosynthesis in white lupin (Lupinus albus L.). Plant Cell Environ. 29, 844–853. doi: 10.1111/j.1365-3040.2005.01464.x
Cao, J., and Govindjee, M. (1990). Chlorophyll-a fluorescence transient as an indicator of active and inactive photosystem II in thylakoid membranes. Biochim. Biophys. Acta 1015, 180–188. doi: 10.1016/0005-2728(90)90018-Y
Carstensen, A., Herdean, A., Schmidt, S. B., Sharma, A., Spetea, C., Pribil, M., et al. (2018). The impacts of phosphorus deficiency on the photosynthetic electron transport chain. Plant Physiol. 177, 271–284. doi: 10.1104/pp.17.01624
Chan, A. T. (1980). Comparative physiological study of marine diatoms and dinoflagellates in relation to irradiance and cell-size 2: Relationship between photosynthesis, growth, and carbon/chlorophyll a ratio. J. Phycol. 16, 428–432. doi: 10.1111/j.1529-8817.1980.tb03056.x
Chen, T. G., Chen, Z. R., Tang, C. L., and Song, D. (2009). Trend of mean winter sea surface temperature (SST) along eastern Guangdong coastal waters during 1898-2009. Guangdong Meteorol. 4, 1–3. doi: 10.3969/j.issn.1007-6190.2009.04.001
Chen, B. H., Kang, W., Xu, D., and Hui, L. (2021). Long-term changes in red tide outbreaks in Xiamen Bay in China from 1986 to 2017. Estuar. Coast. Shelf 249:107095. doi: 10.1016/j.ecss.2020.107095
Chen, F. J., Xu, N., Jiang, T. J., Wang, Y., Wang, Z. H., and Qi, Y. Z. (1999). A report of Phaeocystis globosa bloom in coastal water of Southeast China. J. Jinan Univ. 20, 124–129.
Chylla, R. A., and Whitmarsh, J. (1989). Inactive photosystem II complexes in leaves–turnover rate and quantitation. Plant Physiol. 90, 765–772. doi: 10.1104/pp.90.2.765
Cui, Y. D., Zhang, H., and Lin, S. J. (2017). Enhancement of non-photochemical quenching as an adaptive strategy under phosphorus deprivation in the dinoflagellate Karlodinium veneficum. Front. Microbiol. 8:43. doi: 10.1016/j.ecoenv.2018.04.043
Dao, L. H. T., and Beardall, J. (2016). Effects of lead on two green microalgae chlorella and Scenedesmus: photosystem II activity and heterogeneity. Algal Res. 16, 150–159. doi: 10.1016/j.algal.2016.03.006
Dau, H. (1994). Molecular mechanisms and quantitative models of variable photosystem II fluorescence. Photochem. Photobiol. 60, 1–23. doi: 10.1016/j.algal.2016.03.006
Deng, J. M., Qin, B. Q., Paerl, H. W., Zhang, Y. L., Wu, P., Ma, J. R., et al. (2014). Effects of nutrients, temperature and their interactions on spring phytoplankton community succession in Lake Taihu, China. PLoS One 9:e113960. doi: 10.1371/journal.pone.0113960
Egge, J. K. (1998). Are diatoms poor competitors at low phosphate concentrations? J. Mar. Syst. 16, 191–198. doi: 10.1016/S0924-7963(97)00113-9
Eisenstadt, D., Ohad, I., Keren, N., and Kaplan, A. (2008). Changes in the photosynthetic reaction center II in the diatom Phaeodactylum tricornutum result in non-photochemical fluorescence quenching. Environ. Microbiol. 10, 1997–2007. doi: 10.1111/j.1462-2920.2008.01616.x
Escaravage, V., Peperzak, L., Prins, T. C., Peeters, J. C. H., and Joordens, J. C. A. (1995). The development of a Phaeocystis bloom in a mesocosm experiment in relation to nutrients, irradiance and coexisting algae. Ophelia 42, 55–74. doi: 10.1080/00785326.1995.10431497
Falkowski, P. G., Barber, R. T., and Smetacek, V. (1998). Biogeochemical controls and feedbacks on ocean primary production. Science 281, 200–206. doi: 10.1126/science.281.5374.200
Feng, T. Y., Yang, Z. K., Zheng, J. W., Xie, Y., Li, D. W., Murugan, S. B., et al. (2015). Examination of metabolic responses to phosphorus limitation via proteomic analyses in the marine diatom Phaeodactylum tricornutum. Sci. Rep. 5:373. doi: 10.1038/srep10373
Ghyoot, C., Gypens, N., Flynn, K. J., and Lancelot, C. (2015). Modeling alkaline phosphatase activity in microalgae under orthophosphate limitation: the case of Phaeocystis globosa. J. Plankton Res. 37, 869–885. doi: 10.1038/srep10373
Gorbunov, M. Y., and Falkowski, P. G. (2022). Using chlorophyll fluorescence to determine the fate of photons absorbed by phytoplankton in the World's oceans. Annu. Rev. Mar. Sci. 14, 213–238. doi: 10.1146/annurev-marine-032621-122346
Goss, R., and Jakob, T. (2010). Regulation and function of xanthophyll cycle-dependent photoprotection in algae. Photosynth. Res. 106, 103–122. doi: 10.1007/s11120-010-9536-x
Green, B. R., and Durnford, D. G. (1996). The chlorophyll-carotenoid proteins of oxygenic photosynthesis. Annu. Rev. Plant Physiol. Plant Mol. Biol. 47, 685–714. doi: 10.1146/annurev.arplant.47.1.685
Gypens, N., Lacroix, G., and Lancelot, C. (2007). Causes of variability in diatom and Phaeocystis blooms in Belgian coastal waters between 1989 and 2003: a model study. J. Sea Res. 57, 19–35. doi: 10.1016/j.seares.2006.07.004
Haldimann, P., Fracheboud, Y., and Stamp, P. (1995). Carotenoid composition in Zea mays developed at sub-optimal temperature and different light intensities. Physiol. Plantar. 95, 409–414. doi: 10.1111/j.1399-3054.1995.tb00856.x
Harris, G. N., Scanlan, D. J., and Geider, R. J. (2005). Acclimation of Emiliania huxleyi (Prymnesiophyceae) to photon flux density. J. Phycol. 41, 851–862. doi: 10.1111/j.1529-8817.2005.00109.x
Heraud, P., Roberts, S., Shelly, K., and Beardall, J. (2005). Interactions between UV-B exposure and phosphorus nutrition. II. Effects on rates of damage and repair. J. Phycol. 41, 1212–1218. doi: 10.1111/j.1529-8817.2005.00149.x
Hill, R., and Ralph, P. J. (2006). Photosystem II heterogeneity of in hospite zooxanthellae in scleractinian corals exposed to bleaching conditions. Photochem. Photobiol. 82, 1577–1585. doi: 10.1562/2006-04-13-Ra-871
Huang, K. X., Feng, Q. L., Zhang, Y., Ou, L. J., Cen, J. Y., Lu, S. H., et al. (2020). Comparative uptake and assimilation of nitrate, ammonium, and urea by dinoflagellate Karenia mikimotoi and diatom Skeletonema costatum s.l. in the coastal waters of the East China Sea. Mar. Pollut. Bull. 155:111200. doi: 10.1016/j.marpolbul.2020.111200
Iwata, S., and Barber, J. (2004). Structure of photosystem II and molecular architecture of the oxygen-evolving center. Curr. Opin. Struct. Biol. 14, 447–453. doi: 10.1016/j.sbi.2004.07.002
Jahnke, J. (1989). The light and temperature dependence of growth rate and elemental composition of Phaeocystis globosa Scherffel and Phaeocystis pouchetii (Har) Lagerh in batch cultures. Neth. J. Sea Res. 23, 15–21. doi: 10.1016/0077-7579(89)90038-0
Jain, A., Cao, A., Karthikeyan, A. S., Baldwin, J. C., and Raghothama, K. G. (2005). Phosphate deficiency suppresses expression of light-regulated psbO and psbP genes encoding extrinsic proteins of oxygen-evolving complex of PSII. Curr. Sci. 89, 1592–1596.
Jin, J., Liu, S. M., and Ren, J. L. (2021). Phosphorus utilization by phytoplankton in the Yellow Sea during spring bloom: cell surface adsorption and intracellular accumulation. Mar. Chem. 231:103935. doi: 10.1016/j.marchem.2021.103935
Karasiewicz, S., Breton, E., Lefebvre, A., Farinas, T. H., and Lefebvre, S. (2018). Realized niche analysis of phytoplankton communities involving HAB: Phaeocystis spp. as a case study. Harmful Algae 72, 1–13. doi: 10.1016/j.hal.2017.12.005
Keren, N., Berg, A., VanKan, P. J. M., Levanon, H., and Ohad, I. (1997). Mechanism of photosystem II photoinactivation and D1 protein degradation at low light: the role of back electron flow. P. Natl. Acad. Sci. U. S. A. 94, 1579–1584. doi: 10.1073/pnas.94.4.1579
Komenda, J., Sobotka, R., and Nixon, P. J. (2012). Assembling and maintaining the photosystem II complex in chloroplasts and cyanobacteria. Curr. Opin. Plant Biol. 15, 245–251. doi: 10.1016/j.pbi.2012.01.017
Koppelle, S., Lopez-Escardo, D., Brussaard, C. P. D., Huisman, J., Philippart, C. J. M., Massana, R., et al. (2022). Mixotrophy in the bloom-forming genus Phaeocystis and other haptophytes. Harmful Algae 117:102292. doi: 10.1016/j.hal.2022.102292
Lancelot, C., Billen, G., Sournia, A., Weisse, T., Colijn, F., Veldhuis, M. J. W., et al. (1987). Phaeocystis blooms and nutrient enrichment in the continental coastal zones of the North-Sea. Ambio 16, 38–46.
Lancelot, C., Spitz, Y., Gypens, N., Ruddick, K., Becquevort, S., Rousseau, V., et al. (2005). Modeling diatom and Phaeocystis blooms and nutrient cycles in the southern bight of the North Sea: the MIRO model. Mar. Ecol. Prog. Ser. 289, 63–78. doi: 10.3354/meps289063
Lavaud, J., and Lepetit, B. (2013). An explanation for the inter-species variability of the photoprotective non-photochemical chlorophyll fluorescence quenching in diatoms. BBA-Bioenergetics 1827, 294–302. doi: 10.1016/j.bbabio.2012.11.012
Lavaud, J., Rousseau, B., and Etienne, A. L. (2004). General features of photoprotection by energy dissipation in planktonic diatoms (Bacillariophyceae). J. Phycol. 40, 130–137. doi: 10.1046/j.1529-8817.2004.03026.x
Lavaud, J., Rousseau, B., van Gorkom, H. J., and Etienne, A. L. (2002). Influence of the diadinoxanthin pool size on photoprotection in the marine planktonic diatom Phaeodactylum tricornutum. Plant Physiol. 129, 1398–1406. doi: 10.1104/pp.002014
Lavaud, J., Six, C., and Campbell, D. A. (2016). Photosystem II repair in marine diatoms with contrasting photophysiologies. Photosynth. Res. 127, 189–199. doi: 10.1007/s11120-015-0172-3
Lavergne, J., and Leci, E. (1993). Properties of inactive photosystem II centers. Photosynth. Res. 35, 323–343. doi: 10.1007/Bf00016563
Lepetit, B., Goss, R., Jakob, T., and Wilhelm, C. (2012). Molecular dynamics of the diatom thylakoid membrane under different light conditions. Photosynth. Res. 111, 245–257. doi: 10.1007/s11120-011-9633-5
Lessard, E. J., Merico, A., and Tyrrell, T. (2005). Nitrate: phosphate ratios and Emiliania huxleyi blooms. Limnol. Oceanogr. 50, 1020–1024. doi: 10.4319/lo.2005.50.3.1020
Lewis, J. D., Griffin, K. L., Thomas, R. B., and Strain, B. R. (1994). Phosphorus supply affects the photosynthetic capacity of loblolly pine grown in elevated carbon-dioxide. Tree Physiol. 14, 1229–1244. doi: 10.1093/treephys/14.11.1229
Li, Z. K., Li, W., Zhang, Y., Hu, Y. Y., Sheward, R., Irwin, A. J., et al. (2021). Dynamic photophysiological stress response of a model diatom to ten environmental stresses. J. Phycol. 57, 484–495. doi: 10.1111/jpy.13072
Li, L., Lu, S. H., and Cen, J. Y. (2019). Spatio-temporal variations of harmful algal blooms along the coast of Guangdong, southern China during 1980-2016. J. Oceanol. Limnol. 37, 535–551. doi: 10.1007/s00343-019-8088-y
Li, Y., Lu, S. H., Jiang, T. J., Xiao, Y. P., and You, S. P. (2011). Environmental factors and seasonal dynamics of Prorocentrum populations in Nanji Islands National Nature Reserve East China Sea. Harmful Algae 10, 426–432. doi: 10.1016/j.hal.2010.08.002
Li, J. L., and Sun, X. X. (2016). Effects of different phosphorus concentrations and N/P ratios on the growth and photosynthetic characteristics of Skeletonema costatum and Prorocentrum donghaiense. Chin. J. Oceanol. Limn. 34, 1158–1172. doi: 10.1007/s00343-016-5169-z
Lin, Z. H., Chen, L. S., Chen, R. B., Zhang, F. Z., Jiang, H. X., and Tang, N. (2009). CO2 assimilation, ribulose-1,5-bisphosphate carboxylase/oxygenase, carbohydrates and photosynthetic electron transport probed by the JIP-test, of tea leaves in response to phosphorus supply. BMC Plant Biol. 9:43. doi: 10.1186/1471-2229-9-43
Lin, S. J., Litaker, R. W., and Sunda, W. G. (2016). Phosphorus physiological ecology and molecular mechanisms in marine phytoplankton. J. Phycol. 52, 10–36. doi: 10.1111/jpy.12365
Liu, Y., Li, L., Zhai, X. H., Zhou, J., Ye, P. H., and Huang, S. D. (2021). Analysis of the bloom caused by colonial Phaeocystis globosa in Mirs Bay. J. Trop. Oceanogr. 41, 164–171. doi: 10.11978/2021148
Liu, Y., Song, X. X., Cao, X. H., and Yu, Z. M. (2013). Responses of photosynthetic characters of Skeletonema costatum to different nutrient conditions. J. Plankton Res. 35, 165–176. doi: 10.1093/plankt/fbs080
Liu, C. L., and Tang, D. L. (2012). Spatial and temporal variations in algal blooms in the coastal waters of the western South China Sea. J. Hydro Environ. Res. 6, 239–247. doi: 10.1016/j.jher.2012.02.002
Loebl, M., Cockshutt, A. M., Campbell, D. A., and Finkel, Z. V. (2010). Physiological basis for high resistance to photoinhibition under nitrogen depletion in Emiliania huxleyi. Limnol. Oceanogr. 55, 2150–2160. doi: 10.4319/lo.2010.55.5.2150
Lu, S. H., Ou, L. J., Dai, X. F., Cui, L., Dong, Y. L., Wang, P. B., et al. (2022). An overview of Prorocentrum donghaiense blooms in China: species identification, occurrences, ecological consequences, and factors regulating prevalence. Harmful Algae 114:102207. doi: 10.1016/j.hal.2022.102207
Ly, J., Philippart, C. J. M., and Kromkamp, J. C. (2014). Phosphorus limitation during a phytoplankton spring bloom in the western Dutch Wadden Sea. J. Sea Res. 88, 109–120. doi: 10.1016/j.seares.2013.12.010
Malnoe, A., Wang, F., Girard-Bascou, J., Wollman, F. A., and de Vitry, C. (2014). Thylakoid FtsH protease contributes to photosystem II and cytochrome b(6)f remodeling in Chlamydomonas reinhardtii under stress conditions. Plant Cell 26, 373–390. doi: 10.1105/tpc.113.120113
Markou, G., Dao, L. H. T., Muylaert, K., and Beardall, J. (2017). Influence of different degrees of N limitation on photosystem II performance and heterogeneity of Chlorella vulgaris. Algal Res. 26, 84–92. doi: 10.1016/j.algal.2017.07.005
Martin, P., and Van Mooy, B. A. S. (2013). Fluorometric quantification of polyphosphate in environmental plankton samples: extraction protocols, matrix effects, and nucleic acid interference. Appl. Environ. Microb. 79, 273–281. doi: 10.1128/Aem.02592-12
Meng, X., Chen, W. W., Wang, Y. Y., Huang, Z. R., Ye, X., Chen, L. S., et al. (2021). Effects of phosphorus deficiency on the absorption of mineral nutrients, photosynthetic system performance and antioxidant metabolism in Citrus grandis. PLoS One 16:e0246944. doi: 10.1371/journal.pone.0246944
Moseley, J. L., Chang, C. W., and Grossman, A. R. (2006). Genome-based approaches to understanding phosphorus deprivation responses and PSR1 control in Chlamydomonas reinhardtii. Eukaryot. Cell 5, 26–44. doi: 10.1128/EC.5.1.26-44.2006
Muller, P., Li, X. P., and Niyogi, K. K. (2001). Non-photochemical quenching. A response to excess light energy. Plant Physiol. 125, 1558–1566. doi: 10.1104/pp.125.4.1558
Murata, N., and Nishiyama, Y. (2018). ATP is a driving force in the repair of photosystem II during photoinhibition. Plant Cell Environ. 41, 285–299. doi: 10.1111/pce.13108
Neale, P. J., and Melis, A. (1990). Activation of a reserve pool of photosystem II in Chlamydomonas reinhardtii counteracts photoinhibition. Plant Physiol. 92, 1196–1204. doi: 10.1104/pp.92.4.1196
Nixon, P. J., Michoux, F., Yu, J. F., Boehm, M., and Komenda, J. (2010). Recent advances in understanding the assembly and repair of photosystem II. Ann. Bot. 106, 1–16. doi: 10.1093/aob/mcq059
Oguz, T., and Merico, A. (2006). Factors controlling the summer Emiliania huxleyi bloom in the Black Sea: a modeling study. J. Marine Syst. 59, 173–188. doi: 10.1016/j.jmarsys.2005.08.002
Ou, L. J., Huang, X. Y., Huang, B. Q., Qi, Y. Z., and Lu, S. H. (2015). Growth and competition for different forms of organic phosphorus by the dinoflagellate Prorocentrum donghaiense with the dinoflagellate Alexandrium catenella and the diatom Skeletonema costatum s.l. Hydrobiologia 754, 29–41. doi: 10.1007/s10750-014-1994-2
Ou, L. J., Qin, X. L., Shi, X. Y., Feng, Q. L., Zhang, S. W., Lu, S. H., et al. (2020). Alkaline phosphatase activities and regulation in three harmful Prorocentrum species from the coastal waters of the East China Sea. Microb. Ecol. 79, 459–471. doi: 10.1007/s00248-019-01399-3
Ou, L. J., Wang, D., Huang, B. Q., Hong, H. S., Qi, Y. Z., and Lu, S. H. (2008). Comparative study of phosphorus strategies of three typical harmful algae in Chinese coastal waters. J. Plankton Res. 30, 1007–1017. doi: 10.1093/plankt/fbn058
Pan, X. L., Deng, C. N., Zhang, D. Y., Wang, J. L., Mu, G. J., and Chen, Y. (2008). Toxic effects of amoxicillin on the photosystem II of Synechocystis sp characterized by a variety of in vivo chlorophyll fluorescence tests. Aquat. Toxicol. 89, 207–213. doi: 10.1016/j.aquatox.2008.06.018
Pandey, M. (2006). Nutrient modulated alkaline phosphatase and associated processes in diazotrophic cyanobacteria. Pol. J. Microbiol. 55, 53–62.
Peperzak, L., Colijn, F., Gieskes, W. W. C., and Peeters, J. C. H. (1998). Development of the diatom-Phaeocystis spring bloom in the Dutch coastal zone of the North Sea: the silicon depletion versus the daily irradiance threshold hypothesis. J. Plankton Res. 20, 517–537. doi: 10.1093/plankt/20.3.517
Petrou, K., Doblin, M. A., Smith, R. A., Ralph, P. J., Shelly, K., and Beardall, J. (2008). State transitions and non-photochemical quenching during a nutrient-induced fluorescence transient in phosphorus-starved Dunaliella tertiolecta. J. Phycol. 44, 1204–1211. doi: 10.1111/j.1529-8817.2008.00585.x
Popelkova, L., and Yocum, C. F. (2011). PsbO, the manganese-stabilizing protein: analysis of the structure-function relations that provide insights into its role in photosystem II. J. Photochem. Photobiol. B 104, 179–190. doi: 10.1016/j.jphotobiol.2011.01.015
Reid, P. C., Lancelot, C., Gieskes, W. W. C., Hagmeier, E., and Weichart, G. (1990). Phytoplankton of the North Sea and its dynamics–a review. Neth. J. Sea Res. 26, 295–331. doi: 10.1016/0077-7579(90)90094-W
Riegman, R., Noordeloos, A. A. M., and Cadee, G. C. (1992). Phaeocystis blooms and eutrophication of the continental coastal zones of the North Sea. Mar. Biol. 112, 479–484. doi: 10.1007/BF00356293
Rosowski, J. R., and Parker, B. C. (1971). Selected papers in phycology. Lincoln: Department of Botany, University of Nebraska.
Schoemann, V., Becquevort, S., Stefels, J., Rousseau, W., and Lancelot, C. (2005). Phaeocystis blooms in the global ocean and their controlling mechanisms: a review. J. Sea Res. 53, 43–66. doi: 10.1016/j.seares.2004.01.008
Schoemann, V., Wollast, R., Chou, L., and Lancelot, C. (2001). Effects of photosynthesis on the accumulation of Mn and Fe by Phaeocystis colonies. Limnol. Oceanogr. 46, 1065–1076. doi: 10.4319/lo.2001.46.5.1065
Semenov, A. Y., Kurashov, V. N., and Mamedov, M. D. (2011). Transmembrane charge transfer in photosynthetic reaction centers: some similarities and distinctions. J. Photochem. Photobiol. B. 104, 326–332. doi: 10.1016/j.jphotobiol.2011.02.004
Shelly, K., Roberts, S., Heraud, P., and Beardall, J. (2005). Interactions between UV-B exposure and phosphorus nutrition. I. Effects on growth, phosphate uptake, and chlorophyll fluorescence. J. Phycol. 41, 1204–1211. doi: 10.1111/j.1529-8817.2005.00148.x
Shi, X. G., Lin, X., Li, L., Li, M. Z., Palenik, B., and Lin, S. J. (2017). Transcriptomic and microRNAomic profiling reveals multi-faceted mechanisms to cope with phosphate stress in a dinoflagellate. ISME J. 11, 2209–2218. doi: 10.1038/ismej.2017.81
Solovchenko, A. E., Ismagulova, T. T., Lukyanov, A. A., Vasilieva, S. G., Konyukhov, I. V., Pogosyan, S. I., et al. (2019). Luxury phosphorus uptake in microalgae. J. Appl. Phycol. 31, 2755–2770. doi: 10.1007/s10811-019-01831-8
Srivastava, A., Guisse, B., Greppin, H., and Strasser, R. J. (1997). Regulation of antenna structure and electron transport in photosystem II of Pisum sativum under elevated temperature probed by the fast polyphasic chlorophyll a fluorescence transient: OKJIP. BBA Bioenergetics 1320, 95–106. doi: 10.1016/S0005-2728(97)00017-0
Stirbet, A., and Govindjee, N. R. (2012). Chlorophyll a fluorescence induction: a personal perspective of the thermal phase, the J-I-P rise. Photosynth. Res. 113, 15–61. doi: 10.1007/s11120-012-9754-5
Strasser, R. J., Tsimilli-Michael, M., and Srivastava, A. (2004). Analysis of the chlorophyll a fluorescence transient: Chlorophyll a fluorescence. Sign. Photosyn. 19, 321–362. doi: 10.1007/978-1-4020-3218-9_12
Su, W. W., Jakob, T., and Wilhelm, C. (2012). The impact of nonphotochemical quenching of fluorescence on the photon balance in diatoms under dynamic light conditions. J. Phycol. 48, 336–346. doi: 10.1111/j.1529-8817.2012.01128.x
Thangaraj, S., Palanisamy, S. K., Zhang, G. C., and Sun, J. (2021). Quantitative proteomic profiling of marine diatom Skeletonema dohrnii in response to temperature and silicate induced environmental stress. Front. Microbiol. 11:4832. doi: 10.3389/fmicb.2020.554832
Thingstad, T. F., Krom, M. D., Mantoura, R. F. C., Flaten, G. A. F., Groom, S., Herut, B., et al. (2005). Nature of phosphorus limitation in the ultraoligotrophic eastern Mediterranean. Science 309, 1068–1071. doi: 10.1126/science.1112632
Tyrrell, T., and Merico, A. (2004). Emiliania huxleyi: bloom observations and the conditions that induce them. Coccolithophores: from molecular processes to global impact eds. H. R. Thierstein and J. R. Young (Berlin, Heidelberg: Springer), 75–97.
Van Rensen, J. J. S., and Vredenberg, W. J. (2009). Higher concentration of Q(B)-nonreducing photosystem II centers in triazine-resistant Chenopodium album plants as revealed by analysis of chlorophyll fluorescence kinetics. J. Plant Physiol. 166, 1616–1623. doi: 10.1016/j.jplph.2009.04.011
Vass, I., Kirilovsky, D., and Etienne, A. L. (1999). UV-B radiation-induced donor-side and acceptor-side modifications of photosystem II in the cyanobacterium Synechocystis sp PCC 6803. Biochemistry 38, 12786–12794. doi: 10.1021/bi991094w
Veldhuis, M. J. W., Colijn, F., and Admiraal, W. (1991). Phosphate utilization in Phaeocystis pouchetii (Haptophyceae). Pszni. Mar. Ecol. 12, 53–62. doi: 10.1111/j.1439-0485.1991.tb00083.x
Verity, P. G., and Medlin, L. K. (2003). Observations on colony formation by the cosmopolitan phytoplankton genus Phaeocystis. J. Mar. Syst. 43, 153–164. doi: 10.1016/j.jmarsys.2003.09.001
Veronica, N., Subrahmanyam, D., Kiran, T. V., Yugandhar, P., Bhadana, V. P., Padma, V., et al. (2017). Influence of low phosphorus concentration on leaf photosynthetic characteristics and antioxidant response of rice genotypes. Photosynthetica 55, 285–293. doi: 10.1007/s11099-016-0640-4
Wang, K., Chen, B. H., Gao, Y. H., and Lin, H. (2021). Harmful algal blooms caused by Phaeocystis globosa from 1997 to 2018 in Chinese coastal waters. Mar. Pollut. Bull. 173:112949. doi: 10.1016/j.marpolbul.2021.112949
Wang, Y., Deng, K., and Wang, X. D. (2013). The effects of light, nutrient and co-existing diatom on colony formation of Phaeocystis globosa. Ecol. Sci. 32, 165–170.
Wang, X. D., Huo, Y. P., Yang, F., and Wang, Y. (2021). Induced Allelopathic effects of Thalassiosira weissflogii on Colony formation in Phaeocystis globosa. Water-Sui 13:581. doi: 10.3390/w13050581
Wang, Z. H., Liu, L., Tang, Y. L., Li, A., Liu, C., Xie, C., et al. (2022). Phytoplankton community and HAB species in the South China Sea detected by morphological and metabarcoding approaches. Harmful Algae 118:102297. doi: 10.1016/j.hal.2022.102297
White, A., and Dyhrman, S. (2013). The marine phosphorus cycle. Front. Microbiol. 4:105. doi: 10.3389/fmicb.2013.00105
Wilson, A. J., and Jain, P. K. (2018). Structural dynamics of the oxygen-evolving complex of photosystem II in water-splitting action. J. Am. Chem. Soc. 140, 5853–5859. doi: 10.1021/jacs.8b02620
Wu, H. Y., Cockshutt, A. M., McCarthy, A., and Campbell, D. A. (2011). Distinctive photosystem II photoinactivation and protein dynamics in marine diatoms. Plant Physiol. 156, 2184–2195. doi: 10.1104/pp.111.178772
Wu, H., Roy, S., Alami, M., Green, B. R., and Campbell, D. A. (2012). Photosystem II photoinactivation, repair, and protection in marine centric diatoms (vol 160, pg 464, 2012). Plant Physiol. 160:1146. doi: 10.1104/pp.112.900447
Wykoff, D. D., Davies, J. P., Melis, A., and Grossman, A. R. (1998). The regulation of photosynthetic electron transport during nutrient deprivation in Chlamydomonas reinhardtii. Plant Physiol. 117, 129–139. doi: 10.1104/pp.117.1.129
Yang, Y. J., Shi, J. Q., Jia, Y. L., Bai, F., Yang, S. Q., Mi, W. M., et al. (2020). Unveiling the impact of glycerol phosphate (DOP) in the dinoflagellate Peridinium bipes by physiological and transcriptomic analysis. Environ. Sci. Eur. 32:38. doi: 10.1186/s12302-020-00317-6
Yu, Z. B., Lu, Y., Du, J. J., Peng, J. J., and Wang, X. Y. (2014). The chloroplast protein LTO1/AtVKOR is involved in the xanthophyll cycle and the acceleration of D1 protein degradation. J. Photochem. Photobiol. B. 130, 68–75. doi: 10.1016/j.jphotobiol.2013.11.003
Zhang, X., Ma, F., Zhu, X., Zhu, J. Y., Rong, J. F., Zhan, J., et al. (2017). The acceptor side of photosystem II is the initial target of nitrite stress in Synechocystis sp Strain PCC 6803. Appl. Environ. Microbiol. 83:16. doi: 10.1128/AEM.02952-16
Zhang, S. F., Yuan, C. J., Chen, Y., Chen, X. H., Li, D. X., Liu, J. L., et al. (2016). Comparative transcriptomic analysis reveals novel insights into the adaptive response of Skeletonema costatum to changing ambient phosphorus. Front. Microbiol. 7:1476. doi: 10.3389/fmicb.2016.01476
Zhao, Y., Wang, Y., and Quigg, A. (2015). Comparison of population growth and photosynthetic apparatus changes in response to different nutrient status in a diatom and a coccolithophore. J. Phycol. 51, 872–884. doi: 10.1111/jpy.12327
Zhu, X. G., Govindjee,, Baker, N. R., deSturler, E., Ort, D. R., and Long, S. P. (2005). Chlorophyll a fluorescence induction kinetics in leaves predicted from a model describing each discrete step of excitation energy and electron transfer associated with photosystem II. Planta 223, 114–133. doi: 10.1007/s00425-005-0064-4
Keywords: Skeletonema costatum, Phaeocystis globosa, succession, phosphorus limitation, photosynthetic response
Citation: Chai X, Zheng L, Liu J, Zhan J and Song L (2023) Comparison of photosynthetic responses between haptophyte Phaeocystis globosa and diatom Skeletonema costatum under phosphorus limitation. Front. Microbiol. 14:1085176. doi: 10.3389/fmicb.2023.1085176
Edited by:
Ondrej Prasil, Institute of Microbiology, Academy of Sciences of the Czech Republic (ASCR), CzechiaReviewed by:
Tomáš Zavrel, Global Change Research Centre (ASCR), CzechiaLi Tangcheng, Shantou University, China
Copyright © 2023 Chai, Zheng, Liu, Zhan and Song. This is an open-access article distributed under the terms of the Creative Commons Attribution License (CC BY). The use, distribution or reproduction in other forums is permitted, provided the original author(s) and the copyright owner(s) are credited and that the original publication in this journal is cited, in accordance with accepted academic practice. No use, distribution or reproduction is permitted which does not comply with these terms.
*Correspondence: Jiao Zhan, ✉ emhhbmppYW9AaWhiLmFjLmNu