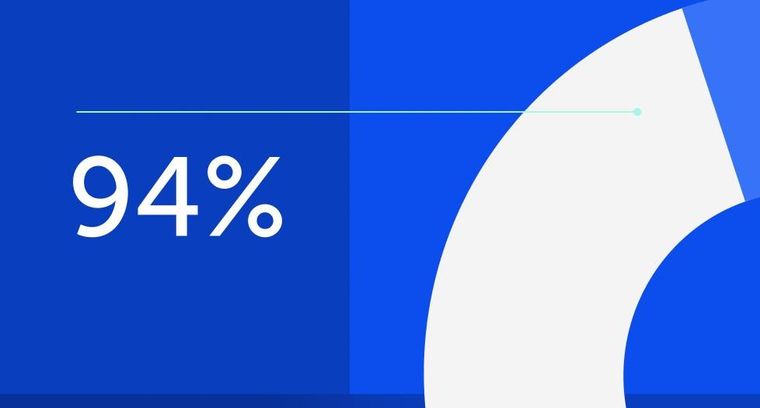
94% of researchers rate our articles as excellent or good
Learn more about the work of our research integrity team to safeguard the quality of each article we publish.
Find out more
ORIGINAL RESEARCH article
Front. Microbiol., 23 September 2022
Sec. Microbial Symbioses
Volume 13 - 2022 | https://doi.org/10.3389/fmicb.2022.994188
Microcystin-LR (MC-LR) is a hazardous substance that threaten the health of aquatic animals. Intestinal microbes and their metabolites can interact with hosts to influence physiological homeostasis. In this study, the shrimp Litopenaeus vannamei were exposed to 1.0 μg/l MC-LR for 72 h, and the toxic effects of MC-LR on the intestinal microbial metagenomic and metabolomic responses of the shrimp were investigated. The results showed that MC-LR stress altered the gene functions of intestinal microbial, including ABC transporter, sulfur metabolism and riboflavin (VB2) metabolism, and induced a significant increase of eight carbohydrate metabolism enzymes. Alternatively, intestinal metabolic phenotypes were also altered, especially ABC transporters, protein digestion and absorption, and the biosynthesis and metabolism of amino acid. Furthermore, based on the integration of intestinal microbial metagenomic and metabolome, four bacteria species (Demequina globuliformis, Demequina sp. NBRC 110055, Sphingomonas taxi and Sphingomonas sp. RIT328) and three metabolites (yangonin, α-hederin and soyasaponin ii) biomarkers were identified. Overall, our study provides new insights into the effects of MC-LR on the intestinal microbial functions of L. vannamei.
The Pacific white shrimp Litopenaeus vannamei is an important economic aquatic animal species. Harmful cyanobacteria are the dominant phytoplankton in shrimp ponds. With the increase of eutrophication level in the rearing water, cyanobacteria are easy to multiply, which not only pollutes the water quality, but also releases a large amount of cyanotoxins, threatening the health and food safety of shrimp (Magalhães et al., 2003; Pham and Utsumi, 2018). Microcystins (MCs) are one of the most common and highly toxic cyanotoxins, the concentration of which in cyanobacterial bloom ponds can reach 0.1–10 μg/l (Ye et al., 2014; Li et al., 2016). Microcystin-LR (MC-LR) is considered to be the most common and widely studied type of MCs, which are encountered in freshwater systems, estuarine and marine environments (Anderson et al., 1993; Ramos et al., 2005; Miller et al., 2010; Preece et al., 2017). It was reported that the MCs concentration of L. vannamei ponds was 0.53–2.25 μg/l at the initial stage, and reached 1.79–2.25 μg/l after 80 days of culture (Wang, 2018); the MC-LR content of L. vannamei ponds with dead individuals was as high as 45 μg/l (Zimba et al., 2006). MC-LR stress can cause immune disorder and induce disease susceptibility in shrimp (Chen et al., 2016, 2017; Yuan et al., 2016; Li et al., 2019a; Duan et al., 2020). Unfortunately, effective measures to prevent MC-LR stress in shrimp are still lacking.
The regulation of intestinal microecology has been proved to be beneficial to the prevention of shrimp diseases. The shrimp intestine inhabits a large microbial community including both beneficial and harmful bacteria, which can interact with their host. A stable intestinal microbiota is beneficial to the host health by participating in substance metabolism, promoting nutrient digestion and absorption, and acting as a biological barrier (Holt et al., 2021). However, intestinal microbiota unbalance will affect the host immune and metabolic homeostasis, and then induce the occurrence of diseases (Li et al., 2019b). Studies have shown that MC-LR stress can lead to intestinal microbiota composition variations and immune dysfunction in shrimp (Duan et al., 2020). Ten and forty microgram per liter MC-LR can induce a decrease in intestinal microbiota diversity and a change in community composition of Procambarus clarkii (Zhang et al., 2020b). Similar phenomena have been observed in mice (Chen et al., 2015; Zhang et al., 2016, 2020a; Zhuang et al., 2021) and zebrafish (Li et al., 2019a). Additionally, MC-LR stress can also alter the functional gene profiles of intestinal microbiota in rats, including chitin, starch and limonene metabolism (Lin et al., 2015). But the effects of MC-LR exposure on the intestinal microbial function of L. vannamei are absence.
Therefore, in this study, we aimed to explore the effects of MC-LR on the gene function and metabolic profile of intestinal microbial in L. vannamei using an integrated metagenomic and metabolomic approach. Our main goals were to reveal: (1) the changes of functional genes in intestinal microbial of MC-LR-stressed L. vannamei; (2) the metabolic characteristics in the intestines of MC-LR-stressed L. vannamei; (3) the changes of the major pathways and sensitive biomarkers that were closely associated with MC-LR stress. The results of this study are helpful to understand the response mechanism of intestinal microbial of L. vannamei to MC-LR stress.
Healthy L. vannamei juveniles with average body weights of 1.2 ± 0.2 g, were randomly collected from a local shrimp pond in Shenzhen, China. The shrimp were acclimated in 100 l experimental tanks for 7 days. Each tank was filled with 60 l of filtered seawater with adequate safety for shrimp and aerated continuously (salinity 30, temperature 30°C ± 0.2, pH 8.1 ± 0.1). The shrimp were fed commercial feed with 40% crude protein (Haida Feed, China), and the filtered seawater from the same source was renewed daily.
MC-LR was purchased from Taiwan algal science Inc., the purity ≥95%. After acclimation, the shrimp were randomly divided into two groups: the control (CK), and the MC groups. Each group consisted of six replicate 100 l tanks that raised 40 shrimp in 60 l seawater per group. According to the report on the MC-LR toxicity in L. vannamei, 0.5–3.0 μg/l MC-LR can affect the immunity of L. vannamei (Chen et al., 2017b). In order to explore the toxicity of relatively low dose of MC-LR to L. vannamei, the concentration of MC-LR in this study was set as 1.0 μg/l, which was 1 folds of the WHO’s maximum standard for water (1.0 μg/l) (WHO, 2011). The CK group was normal seawater without MC-LR detection. The rearing water of the MC group contained 1.0 μg/l MC-LR, which were verified analytically. The water of each tank was all replaced every 24 h, and the MC-LR was re-added in the corresponding concentration. The culture conditions of the exposed stage were consistent with those of the acclimation stage.
After 72 h of MC-LR exposure, the intact intestines of 30 individual shrimps from each tank were collected. To reduce the impact of interindividual differences, the 30 intact intestines were homogenized and bisected into one metagenomic sample and one metabolome sample. Overall, six metagenomic samples and six metabolomic samples were collected from each group.
The genomic DNA was extracted using CTAB/SDS method and detected by 1.0% agarose gel electrophoresis. After DNA fragmentation, the Illumina NEBNext® Ultra™ DNA Library Prep Kit (NEB, USA) was used to construct the sequencing library according to the manufacturer’s instructions. The libraries were sequenced on Illumina Novoseq platform. The obtained raw reads were processed to obtain high-quality clean data using Readfq software (V8). Then the host gene contamination was removed according to the genome of L. vannamei using Bowtie2 software (V2.2.4). The Scaftigs were obtained by SOAPdenovo software (V2.04). MetaGeneMark (V2.10) was used to predict the ORF of Scaftigs, and CD-HIT software (V4.5.8) was used to remove the redundancy and obtain the initial gene catalogue. Bowtie2 software (V2.2.4) was used to compare with clean data of each sample to obtain the final gene set for subsequent analysis. According to the gene set, the gene abundance information in each sample was counted, and the gene number difference between groups and the Venn diagram were analyzed. DIAMOND software (V0.9.9.110) was used to align Unigenes with non-redundant (NR) database, annotate species information, and analyze non-metric multidimensional scaling (NMDS) dimensionality reduction at different classification levels using R Vegan software package (V2.15.3). DIAMOND software (V0.9.9.110) was used to annotate Unigenes with Kyoto Encyclopedia of Genes and Genomes (KEGG), evolutionary genealogy of genes: Non-supervised Orthologous Groups (eggNOG), and carbohydrate active enzyme (CAZyme) databases, and the parameter was set to E-value ≤1E-5. The functional differences between the groups were analyzed by Metastat software. All the raw data of intestinal microbiota has been submitted to the Sequence Read Archive (SRA) database (accession: PRJNA862309).
The samples were thawed slowly at 4°C, and homogenized with 200 μl of sterile water. The homogenized solution was mixed with 800 μl methanol/acetonitrile (1: 1, v/v) for metabolite extraction. The mixture was ultrasonicated at 4°C for 30 min, stood at–20°C for 10 min, and then centrifuged at 14,000g, 4°C for 20 min. The supernatant was vacuum dried and redissolved in 100 μl acetonitrile solution (acetonitrile:water = 1:1, v/v) for liquid chromatography-mass spectrometry (LC–MS) analysis.
Liquid chromatography was performed in an UHPLC (Agilent 1290 Infinity LC) coupled to a quadrupole time-of-flight (AB Sciex TripleTOF 6600). HILIC separation were analyzed using a ACQUIY UPLC BEH column (2.1 mm × 100 mm, 1.7 μm) (Waters, Ireland). Mass spectrometry was accomplished in an AB Triple TOF 6600 mass spectrometer to collect the primary and secondary spectra of the samples. The positive and negative ionization modes of electrospray ionization (ESI) were used for detection, respectively.
The raw MS data (wiff.scan files) were converted to MzXML files using ProteoWizard MSConvert before importing into freely available XCMS software. All the data were determined using total ion chromatogram (TIC) and quality control (QC). After sum-normalization, compound identification of metabolites was performed by comparing of accuracy m/z value (<25 ppm), and MS/MS spectra with an in-house database (Shanghai Applied Protein Technology) established with available authentic standards (Luo et al., 2017; Gu et al., 2018). All the metabolites were classified according to their chemical taxonomy. The variation trend of metabolite expression in each group was analyzed using fuzzy c-means (FCM) algorithm of Mfuzz packet. The visual volcano plot of the differential metabolites (DMs) was analyzed with an standard of fold-change (FC) > 1.5 or FC < 0.67, and p < 0.05. The metabolomic data were multiple compared with orthogonal partial least-squares discriminant analysis (OPLS-DA) using R package (ropls). The robustness of the model was evaluated by 7-fold cross-validation and response permutation test. The DMs of MC vs. CK were identified with an standard of OPLS-DA VIP > 1 and p < 0.05. The KEGG pathway of all the DMs was performed using KEGG software.1 The metabolite markers with significant changes were further screened and analyzed.
The KEGG pathways of differential genes and metabolites of intestinal bacteria were analyzed, and the pathway attribution of them were integrated. Based on the standardized data of scale, the spearman correlation coefficient between the differential bacteria and metabolites with linear discriminant analysis (LDA) > 2 were analyzed using R package psych software. The multilevel regulatory relationship among bacteria species, functional genes and metabolites was analyzed, and Sankey diagram was conducted using R package plotly software.
A total of 75,600 clean reads were obtained from 12 intestinal microbial samples in the two groups by metagenomic sequencing, with an average of 6,300 reads per sample; the effective were 96.63–99.75% (Supplementary Tables S1–S3). A total of 2,947,876 genes were obtained, and the number of genes in the MC group was more than that in the CK group (Figure 1A). Based on Venn analysis, 381,307 genes were co-owned by the two groups, and the unique genes of the MC group was higher than that of the CK group (Figure 1B).
Figure 1. Gene numbers analysis of intestinal microbial of L. vannamei after MC-LR exposure. (A) Comparison of non-redundant genes number between the CK and MC groups. (B) Venn diagram of gene numbers between the CK and MC groups.
Based on the eggNOG analysis, the highest functional categories was “amino acid transport and metabolism” followed by “energy production and conversion” “inorganic ion transport and metabolism” etc. (Supplementary Figure S1). At the level 2, the top categories were “ABC transporter” followed by “transcriptional regulator” “membrane” “histidine kinase” and “dehydrogenase” (Supplementary Figure S2). Compared with the CK group, “ABC transporter (COG1174)” was significantly increased in the MC group (Figure 2A). The KEGG analysis of all the functional genes was also performed, which were mainly “carbohydrate metabolism” “amino acid metabolism” “membrane transport” “translation” and “metabolism of cofactors and vitamins” (Supplementary Figures S3–S4). Compared with the CK group, the “KO_EC 1.5.1.38” pathway encoding “sulfur metabolism” and “riboflavin metabolism” was significantly increased in the MC group (Figure 2B).
Figure 2. Significantly altered gene function of intestinal microbial of L. vannamei after MC-LR exposure. (A) eggNOG function. (B) KEGG function. (C) CAZy categories. (D) CAZy species. * represents significant difference between the CK and MC groups (q < 0.05).
The CAZyme of intestinal microbe of the shrimp were further analyzed. The highest CAZyme taxa was glycoside hydrolases (GH), followed by glycosyltransferases (GT), carbohydrate-binding modules (CBM), carbohydrate esterases (CE), auxiliary activities (AA), and polysaccharide lyases (PL) (Supplementary Figure S5; Figure 2C). Compared with the CK group, the abundance of eight enzymes was significantly increased in the MC group, including α-mannosidase, glucan 1,3-β-glucosidase, glucan 1,4-β-glucosidase, coniferin β-glucosidase, β-N-acetylhexosaminidase, β-N-acetylglucosaminide phosphorylases, xylan 1,4-β-xylosidase, and exo-1,3-1,4-glucanase (Figure 2D).
Changes in intestinal microflora of the shrimp were analyzed. NMDS plot showed a clear visual separation in intestinal microbial composition between the CK and MC groups (Figure 3A). Several relatively high abundance bacterial species fluctuated in response to MC-LR exposure (Figure 3B). For example, the relative abundances of Demequina flava, Demequina sp. NBRC 110055, and Demequina sediminicola were significantly increased, while that of Photobacterium damselae and Shimia marina were significantly decreased. Additionally, Demequina globuliformis, Phyllobacterium sp. OV277, Ruegeria sp. 6PALISEP08, Sphingomonas taxi, Sphingomonas sp. RIT328, and Vibrio xuii were increased, while Flavobacteriaceae bacterium UJ101 and Nautella italica were decreased, but the differences were not significant.
Figure 3. Comparison of intestinal bacteria species of L. vannamei after MC-LR exposure. (A) NMDS analysis. (B) The relative abundance of bacteria species. * Indicates significant differences between the two groups (p < 0.05).
A total of 2,052 metabolites were identified, including 1,213 positive ion mode (pos) and 839 negative ion mode (neg). The chemical classification of these metabolites were mainly “organic acids and derivatives” (31.77%) and “lipids and lipid-like molecules” (23.59%) (Supplementary Figure S6). Multivariate statistical analysis of OPLS-DA under positive and negative ion modes showed that significant differences in metabolic patterns were existed between the CK and MC groups (Figure 4). Compared with the CK group, 103 DMs were identified under positive ion pattern, including 56 up-regulated and 47 down-regulated metabolites. The negative ion pattern identified 87 DMs, including 53 up-regulated and 34 down-regulated metabolites (Supplementary Figures S7–S9).
Figure 4. OPLS-DA analysis of intestinal metabolites of L. vannamei after MC-LR exposure. (A) Positive ion mode. (B) Negative ion mode
The metabolic pathways of the DMs were further analyzed (Figure 5A). The top five highly enriched pathways were mainly “ABC transporters” “biosynthesis of amino acids” “protein digestion and absorption” “neuroactive ligand-receptor interaction” “aminoacyl-tRNA biosynthesis” Additionally, many pathways of amino acid metabolism were also highly enriched, including “arginine and proline metabolism” “glycine, serine and threonine metabolism” “histidine metabolism” “alanine, aspartate and glutamate metabolism” and “taurine and hypotaurine metabolism” In the ABC transporters pathway, a total of 14 DMs were up-regulated, including DL-threonine, aspartic acid, L-alanine, glutamic acid, proline, DL-glutamic acid, glycine, taurine, betaine, N-acetyl-D-glucosamine, N-acetyl-glucosamine, 2′-deoxyinosine, deoxyinosine, and inosine (Figure 5B).
Figure 5. The pathways of differential metabolites in the intestinal of L. vannamei after MC-LR exposure. (A) The pathways of differential metabolites. (B) The differential metabolites of ABC transporters.
Certain organism health-related DMs were further identified (Table 1). For example, 7 carbohydrates abundance were increased, including pyruvate, muramic acid, N-acetyl-D-glucosamine, N-acetylglucosamine, N-acetyl-D-mannosamine, N-Acetylmannosamine, and DL-lactate. Fifteen amino acids abundance were altered, such as glycine, DL-threonine, DL-glutamic acid, D-pyroglutamic acid, proline, aspartic acid, L-alanine, L-methionine, glutamic acid, sarcosine, taurine, ergothioneine, γ-aminobutyric acid (GABA), and betaine were up-regulated; while γ-glutamylvaline was down-regulated. Five lipids such as linoleic acid, dodecanoic acid, myristic acid, taurocholate, and taurodeoxycholic acid were decreased. Additionally, 1 vitamin such as pyridoxine was decreased.
The KEGG pathway of functional genes and metabolites with significant differences in abundance was statistically analyzed (Figure 6). The results showed that phenylpropanoid biosynthesis, and riboflavin, sulfur and cyanoamino acid metabolism were the high abundance pathways of intestinal microbial metabolism.
Figure 6. Correlation analysis of KEGG pathway of intestinal microbial metagenome and metabolome of L. vannamei.
The correlation between intestinal bacterial species and metabolites was further analyzed (Figure 7). For instance, D. sp. NBRC 110055 was positively correlated with linoleic acid, but negatively correlated with enniatin B and L-methionine. Sphingomonas melonis was negatively correlated with pantothenate and proline. S. taxi was positively correlated with α-hederin, asiaticoside, dodecanoic acid, linoleic acid, myristic acid, paxilline, soyasaponins, taurochenodeoxycholate, taurodeoxycholic acid, and yangonin. Sphingomonas sp. TDK1 was negatively correlated with glutamic acid, proline and pantothenate. Sphingomonas haliotis was negatively correlated with norharmane.
Figure 7. The correlation analysis between intestinal bacterial species and metabolites of L. vannamei. * represents a significant difference (*p < 0.05; **p < 0.01).
The correlation between intestinal bacterial species, functional genes and metabolites was further analyzed (Figure 8). The bacteria D. sp. NBRC 110055, S. taxi and S. sp. RIT328 were correlated with the metabolite yangonin through β-glucosidase (bglX) gene, while D. globuliformis was correlated with yangonin through flavin mononucleotide reductase (ssuE) gene. The bacteria S. taxi was correlated with the metabolites α-hederin and soyasaponin ii through (1 → 4)-α-D-glucan 1-α-D-glucosylmutase (treY) gene.
Figure 8. Correlation analysis of intestinal bacteria species, functional genes and metabolites of L. vannamei. Left: bacteria species, middle: functional genes, right: metabolites. The line between the bacteria species and functional gene indicates the contributing bacteria of the functional gene. The line between the functional genes and metabolites represents they have significant correlation (|r| > 0.8, p < 0.05).
The intestinal microbiota interacts with its host, thereby influencing many of the host physiological processes, and changes in their functions can affect host physiological homeostasis (Valdes et al., 2018; Holt et al., 2021). In this study, based on the functional annotations of eggNOG and KEGG, the gene functions of the intestinal microbial of L. vannamei were mainly amino acid and carbohydrate metabolism, which was consistent with the results of Gao et al. (2019). After MC-LR exposure, the functions of ABC transporter and “KO_EC 1.5.1.38” of intestinal microbial were increased, and “KO_EC 1.5.1.38” was mainly involved in sulfur and riboflavin metabolism. Microbial sulfur metabolism produces hydrogen sulfide (H2S), which is associated with colorectal cancer (Nguyen et al., 2021). Riboflavin, also known as vitamin B2, has antioxidant activity and is mainly involved in biological oxidation and energy metabolism in vivo (Ashoori and Saedisomeolia, 2014; Salman and Naseem, 2015). ABC transporters are membrane-binding proteins that participate in transmembrane transport of endogenous syncytic and xenobiotic compounds, contributing to the detoxification and excretion of toxic substances (Higgins, 1992; Wang et al., 2021). Therefore, it can be concluded that MC-LR exposure can induce the risk of H2S generation in intestinal microbial metabolism of L. vannamei, and the organism can improve antioxidant function and promote detoxification metabolism by enhancing ABC transporter and riboflavin metabolism.
Intestinal microbes can produce a large number of carbohydrate metabolism enzymes to influence digestion and metabolism of the host (Valdes et al., 2018). CAZymes encoded by intestinal microbes can catalyze the decomposition of glycoconjugates, oligosaccharides and polysaccharides into monosaccharides, and can also metabolize dietary fibers to produce short-chain fatty acids to promote the intestinal health of the host (Kaoutari et al., 2013). In this study, based on CAZy functional annotation, carbohydrate metabolism enzymes of intestinal microbial of L. vannamei were mainly glycoside hydrolase (GH) and glycosyltransferase (GT). After MC-LR exposure, the levels of α-mannosidase, glucan 1,3-β-glucosidase, glucan 1,4-β-glucosidase, coniferin β-glucosidase, β-N-acetylhexosaminidase, β-N-acetylglucosaminide phosphorylases, xylan 1,4-β-xylosidase, and exo-1,3-1,4-glucanase of intestinal microbial of the shrimp were increased, indicating that intestinal microbes respond to MC-LR stress by enhancing the capacity of carbohydrate hydrolysis.
Several potentially bacteria species exhibited variation after MC-LR exposure. Demequina is involved in starch degradation to glucose and dextrine (Al-naamani et al., 2015; Peruzzi et al., 2017). In this study, the relative abundances of three Demequina species (D. flava, D. sp. NBRC 110055 and D. sediminicola) were increased in response to MC-LR exposure, suggesting that these bacteria may facilitate carbohydrate degradation. P. damselae is an opportunistic pathogen, which has high pathogenicity and can cause photobacteriosis of shrimp (Song et al., 1993; Vaseeharan et al., 2007). S. marina was originally isolated from the biofilm of a coastal fish farm (Choi and Cho, 2006), and may have pathogenicity related to coral white diseases based on metagenomic studies (Godwin et al., 2012; Séré et al., 2013). In this study, the relative abundances of P. damselae and S. marina were decreased in the MC group, indicating MC-LR exposure might affect the abundance of potentially harmful bacteria in the shrimp intestines.
Previous studies have shown that MC-LR exposure induces hepatopancreas metabolic phenotype and lipid homeostasis in L. vannamei, such as citric acid cycle as well as amino acid, linoleic acid, and arachidonic acid metabolism. However, intestinal metabolic characteristics of L. vannamei under MC-LR stress are still unclear. In this study, untargeted metabonomics further revealed that MC-LR exposure caused significant fluctuations in the intestinal metabolites in L. vannamei. Interestingly, the differential metabolites were mainly enriched in the ABC transporters, protein digestion and absorption, and amino acid biosynthesis and metabolism. These phenomena indicate that amino acid metabolism is the main toxic mechanism of MC-LR in L. vannamei, and the metabolic response of intestinal and hepatopancreas to MC-LR stress is different.
Amino acid metabolism can provide nutrients and participate in physiological processes in organisms (Eswarappa and Fox, 2013). ABC transporter can realize trans-membrane transport of various substances and has the detoxification function (Higgins, 1992; Wang et al., 2021). In this study, the up-regulation of amino acids also participated in ABC transporters. Hence, we speculated that the shrimp intestines might utilize ABC transporters and amino acid metabolism for adapting MC-LR stress, and the up-regulated amino acids could also compensate for the host’s requirements for amino acids. Pyridoxine (VB6) is a coenzyme component involved in the non-oxidative degradation of amino acids (Giri et al., 1997). In this study, the decreased level of pyridoxine further suggested that MC-LR influenced the homeostasis of amino acid metabolism in the shrimp intestines.
Lipid metabolism participates in fatty acid and energy sources for organisms (Lee et al., 2018). In this study, the decreased levels of linoleic acid, dodecanoic acid, myristic acid, taurocholate and taurodeoxycholic acid indicated that MC-LR affected intestinal lipid metabolism homeostasis. Taurocholate is considered to be a more efficient bile acid in fat absorption (Chesney et al., 1998). Taurine is involved in fat digestion as a bile acid conjugator (Kim et al., 2007). In this study, the decreased levels of taurocholate and taurodeoxycholic acid, and the increased level of taurine indicated that MC-LR might also affect the bile acid metabolism of the shrimp.
Additionally, several intestinal metabolites related to the health of the organism were also fluctuated after the shrimp exposed to MC-LR. For example, four amino acids including ergothioneine, betaine, GABA and taurine were all up-regulated. Ergothioneine is a natural antioxidant (Hartman, 1990). Betaine is a common feed additive that functions in anti-inflammatory, liver disease treatment, and intestinal health improvement (Santos et al., 2019; Huang et al., 2021). GABA is an neurotransmitter produced by microorganisms that enhances stress resistance in shrimp (Xie et al., 2015). Taurine can reduce inflammation and oxidative stress, and regulate blood sugar, lipids and energy metabolism by binding bile acids (Guizoni et al., 2020). In this study, the increased levels of ergothioneine, betaine, GABA and taurine indicated that the shrimp intestines might response positively to stress by producing these beneficial metabolites.
Host-microbiota interactions can affect the immunity of aquatic animals (Li et al., 2019b; Xiao et al., 2021a,b). The integrated analysis of intestinal microbial metagenome and metabolome is conducive to elucidate the relationship between intestinal microbe and metabolites, thus identifying key biomarkers. In this study, intestinal bacteria species D. globuliformis, D. sp. NBRC 110055, S. taxi and S. sp. RIT328 were correlated with the metabolites yangonin, α-hederin and soyasaponin ii. At present, there is a lack of study reports on these four bacteria species, their specific functions are still unclear and need to be further analyzed. α-hederin and soyasaponin have anti-tumor and inflammatory and immunomodulatory functions (Deng et al., 2019; Chitisankul et al., 2021). Yangonin has anti-cancer cell proliferation and antioxidant activity, which can also be used to treat liver diseases (Renchao et al., 2019). Thus speculate intestinal metabolites yangonin, α-hederin and soyasaponin ii might improve the resistance to MC-LR stress of L. vannamei, and can be used as potential functional metabolic markers for the development of biological agents. For these intestinal bacteria and metabolic markers identified in this study, their roles in the response of L. vannamei to MC-LR stress will be explored in the future, so as to lay the foundation for the research and development of biological agents.
We investigated the effects of MC-LR on the intestinal microbial function of L. vannamei using an integrated metagenomic and metabolomic approach. MC-LR stress altered the gene functions of intestinal microbial, including ABC transporter, sulfur metabolism and riboflavin (VB2) metabolism, and induced a significant increase of eight carbohydrate metabolism enzymes. Alternatively, the metabolic pattern variation occurred the intestine, involving ABC transporters, protein digestion and absorption, and the biosynthesis and metabolism of amino acid; several functional metabolites including 7 carbohydrates, 14 amino acids, 5 lipids, and 1 vitamin were identified. Further, based on the integration of intestinal microbial metagenomic and metabolome, four bacteria species (D. globuliformis, D. sp. NBRC 110055, S. taxi and S. sp. RIT328) and three metabolites (yangonin, α-hederin and soyasaponin ii) biomarkers were identified. ABC transporter is an important target of intestinal microbiota of the shrimp in response to MC-LR stress. Overall, our study provides new insights into the effects of MC-LR on the intestinal microbial functions of L. vannamei.
The datasets presented in this study can be found in online repositories. The names of the repository/repositories and accession number (PRJNA862309) can be found in the article/Supplementary material.
The protocols for collecting and handling the animals in this study were approved by the Animal Care and Use Committee of the South China Sea Fisheries Research Institute, Chinese Academy of Fishery Sciences (SCSFRI-CAFS, No. nhdf2022-12).
YD designed and performed the experiments, analyzed data, and wrote the manuscript. YX, SZ, and ZM contributed to sample collection and experimental analysis. XD, JZ, and YL assisted in resources, supervision, and project administration. All authors contributed to the article and approved the submitted version.
This study was supported by National Natural Science Foundation of China (31902343); Guangdong Natural Science Foundation (2021A1515012084); Hainan Provincial Natural Science Foundation of China (322QN436); Guangzhou Basic and Applied Basic Research Project (202102080246); Young Scientific Talents Lifting Planning Project of Guangzhou Science and Technology Association (X20210201039); Agricultural Research Outstanding Talents Training Program (13210308); Central Public-interest Scientific Institution Basal Research Fund, South China Sea Fisheries Research Institute, CAFS (2022RC01 and 2021SD19); Central Public-interest Scientific Institution Basal Research Fund, CAFS (2021XT0604); Guangdong Provincial Special Fund for Modern Agriculture Industry Technology Innovation Teams (2019KJ141); and China-ASEAN Maritime Cooperation Fund, China-ASEAN Modern Marine Fishery Technical Cooperation, Industrialization Development and Demonstration.
The authors are grateful to the laboratory staff for technical assistance. We also thank the reviewers for their helpful suggestions to the manuscript.
The authors declare that the research was conducted in the absence of any commercial or financial relationships that could be construed as a potential conflict of interest.
All claims expressed in this article are solely those of the authors and do not necessarily represent those of their affiliated organizations, or those of the publisher, the editors and the reviewers. Any product that may be evaluated in this article, or claim that may be made by its manufacturer, is not guaranteed or endorsed by the publisher.
The Supplementary material for this article can be found online at: https://www.frontiersin.org/articles/10.3389/fmicb.2022.994188/full#supplementary-material
Al-naamani, L. S. H., Dobretsov, S., Al-sabahi, J., and Soussi, B. (2015). Identification and characterization of two amylase producing bacteria Cellulosimicrobium sp. and Demequina sp. isolated from marine organisms. JAMS 20, 8–15.
Anderson, R. J., Luu, H. A., Chen, D. Z. X., Holmes, C. F. B., Kent, M. L., Le Blanc, M., et al. (1993). Chemical and biological evidence links microcystins to salmon “netpen liver disease”. Toxicon 31, 1315–1323. doi: 10.1016/0041-0101(93)90404-7
Ashoori, M., and Saedisomeolia, A. (2014). Riboflavin (vitamin B2) and oxidative stress: a review. Br. J. Nutr. 111, 1985–1991. doi: 10.1017/S0007114514000178
Chen, C., Liu, W., Wang, L., Li, J., Chen, Y., Jin, J., et al. (2016). Pathological damage and immunomodulatory effects of zebrafish exposed to microcystin-LR. Toxicon 118, 13–20. doi: 10.1016/j.toxicon.2016.04.030
Chen, J., Xie, P., Lin, J., He, J., Zeng, C., and Chen, J. (2015). Effects of microcystin-LR on gut microflora in different gut regions of mice. J. Toxicol. Sci. 40, 485–494. doi: 10.2131/jts.40.485
Chen, Y., Huang, X., Wang, J., and Li, C. (2017). Effect of pure microcystin-LR on activity and transcript level of immune-related enzymes in the white shrimp (Litopenaeus vannamei). Ecotoxicology 26, 702–710. doi: 10.1007/s10646-017-1802-7
Chesney, R. W., Helms, R. A., Christensen, M., Budreau, A. M., Han, X., and Sturman, J. A. (1998). The role of taurine in infant nutrition. Adv. Exp. Med. Biol. 442, 463–476. doi: 10.1007/978-1-4899-0117-0_56
Chitisankul, W. T., Itabashi, M., Son, H., Takahashi, Y., Ito, A., Varanyanond, W., et al. (2021). Soyasaponin composition complexities in soyfoods relating nutraceutical properties and undesirable taste characteristics. LWT 146:111337. doi: 10.1016/j.lwt.2021.111337
Choi, D. H., and Cho, B. C. (2006). Shimia marina gen. Nov., sp. nov., a novel bacterium of the Roseobacter clade isolated from biofilm in a coastal fish farm. Int. J. Syst. Evol. Microbiol. 56, 1869–1873. doi: 10.1099/ijs.0.64235-0
Deng, H., Ma, J. J., Liu, Y. H., He, P. Z., and Dong, W. G. (2019). Combining α-hederin with cisplatin increases the apoptosis of gastric cancer in vivo and in vitro via mitochondrial related apoptosis pathway. Biomed. Pharmacother. 120:109477. doi: 10.1016/j.biopha.2019.109477
Duan, Y. F., Xiong, D. L., Wang, Y., Dong, H. B., Huang, J. H., and Zhang, J. S. (2020). Effects of Microcystis aeruginosa and microcystin-LR on intestinal histology, immune response, and microbial community in Litopenaeus vannamei. Environ. Pollut. 265:114774. doi: 10.1016/j.envpol.2020.114774
Eswarappa, S. M., and Fox, P. L. (2013). Citric acid cycle and the origin of MARS. Trends Biochem. Sci. 38, 222–228. doi: 10.1016/j.tibs.2013.01.005
Gao, S., Pan, L. Q., Huang, F., Song, M. S., Tian, C. C., and Zhang, M. Y. (2019). Metagenomic insights into the structure and function of intestinal microbiota of the farmed Pacific white shrimp (Litopenaeus vannamei). Aquaculture 499, 109–118. doi: 10.1016/j.aquaculture.2018.09.026
Giri, I. N. A., Teshima, S., Kanazawa, A., and Ishikawa, M. (1997). Effects of dietary pyridoxine and protein levels on growth, vitamin B6 content, and free amino acid profile of juvenile Penaeus japonicus. Aquaculture 157, 263–275. doi: 10.1016/S0044-8486(97)00157-9
Godwin, S., Bent, E., Borneman, J., and Pereg, L. (2012). The role of coral-associated bacterial communities in Australian subtropical white syndrome of Turbinaria mesenterina. PLoS One 7:e44243. doi: 10.1371/journal.pone.0044243
Gu, Z. B., Li, L., Tang, S. K., Liu, C. B., Fu, X. H., Shi, Z. X., et al. (2018). Metabolomics reveals that crossbred dairy buffaloes are more thermotolerant than Holstein cows under chronic heat stress. J. Agric. Food Chem. 66, 12889–12897. doi: 10.1021/acs.jafc.8b02862
Guizoni, D. M., Vettorazzi, J. F., Carneiro, E. M., and Davel, A. P. (2020). Modulation of endothelium-derived nitric oxide production and activity by taurine and taurine-conjugated bile acids. Nitric Oxide 94, 48–53. doi: 10.1016/j.niox.2019.10.008
Hartman, P. E. (1990). Ergothioneine as antioxidant. Methods Enzymol. 186, 310–318. doi: 10.1016/0076-6879(90)86124-E
Higgins, C. F. (1992). ABC transporters: from microorganisms to man. Annu. Rev. Cell Biol. 8, 67–113. doi: 10.1146/annurev.cb.08.110192.000435
Holt, C. C., Bass, D., Stentiford, G. D., and van der Giezen, M. (2021). Understanding the role of the shrimp gut microbiome in health and disease. J. Invertebr. Pathol. 186:107387. doi: 10.1016/j.jip.2020.107387
Huang, T. D., Yu, J. S., Luo, Z. P., Yu, L., Liu, S. Q., Wang, P., et al. (2021). Translatome analysis reveals the regulatory role of betaine in high fat diet (HFD)-induced hepatic steatosis. Biochem. Biophys. Res. Commun. 575, 20–27. doi: 10.1016/j.bbrc.2021.08.058
Kaoutari, A. E., Armougom, F., Gordon, J. I., Raoult, D., and Henrissat, B. (2013). The abundance and variety of carbohydrate-active enzymes in the human gut microbiota. Nat. Rev. Microbiol. 11, 497–504. doi: 10.1038/nrmicro3050
Kim, S. K., Matsunari, H., Takeuchi, T., Yokoyama, M., Murata, Y., and Ishihara, K. (2007). Effect of different dietary taurine levels on the conjugated bile acid composition and growth performance of juvenile and fingerling Japanese flounder Paralichthys olivaceus. Aquaculture 273, 595–601. doi: 10.1016/j.aquaculture.2007.10.031
Lee, M. C., Park, J. C., and Lee, J. S. (2018). Effects of environmental stressors on lipid metabolism in aquatic invertebrates. Aquat. Toxicol. 200, 83–92. doi: 10.1016/j.aquatox.2018.04.016
Li, J., Chen, C. Y., Zhang, T. Z., Liu, W. J., Wang, L., and Chen, Y. Y. (2019a). μEvaluation of microcystin-LR absorption using an in vivo intestine model and its effect on zebrafish intestine. Aquat. Toxicol. 206, 186–194. doi: 10.1016/j.aquatox.2018.11.014
Li, X. M., Ringø, E., Hoseinifar, S. H., Lauzon, H. L., Birkbeck, H., and Yang, D. (2019b). The adherence and colonization of microorganisms in fish gastrointestinal tract. Rev. Aquacult. 11, 603–618. doi: 10.1111/raq.12248
Lin, J., Chen, J., He, J., Chen, J., Yan, Q., Zhou, J., et al. (2015). Effects of microcystin-LR on bacterial and fungal functional genes profile in rat gut. Toxicon 96, 50–56. doi: 10.1016/j.toxicon.2015.01.011
Li, X., Cheng, R., Shi, H., Tang, B., Xiao, H. S., and Zhao, G. H. (2016). A simple highly sensitive and selective aptamer-based colorimetric sensor for environmental toxins microcystin-LR in water samples. J. Hazard. Mater. 304, 474–480. doi: 10.1016/j.jhazmat.2015.11.016
Luo, D., Deng, T., Yuan, W., Deng, H., and Jin, M. (2017). Plasma metabolomic study in Chinese patients with wet age-related macular degeneration. BMC Ophthalmol. 17:165. doi: 10.1186/s12886-017-0555-7
Magalhães, V. F., Marinho, M. M., Domingo, P., Oliveira, A. C., Costa, S. M., Azevedo, L. O., et al. (2003). Microcystins (cyanobacteria hepatotoxins) bioaccumulation in fish and crustaceans from Sepetiba Bay (Brasil, RJ). Toxicon 42, 289–295. doi: 10.1016/S0041-0101(03)00144-2
Miller, M. A., Kudela, R. M., Mekebri, A., Crane, D., Oates, S. C., Tinker, M. T., et al. (2010). Evidence for a novel marine harmful algal bloom: cyanotoxin (microcystin) transfer from land to sea otters. PLoS One 5:e12576. doi: 10.1371/journal.pone.0012576
Nguyen, L. H., Cao, Y., Hur, J., Mehta, R. S., Sikavi, D. R., Wang, Y. Q., et al. (2021). The sulfur microbial diet is associated with increased risk of early-onset colorectal cancer precursors. Gastroenterology 161, 1423–1432.e4. doi: 10.1053/j.gastro.2021.07.008
Peruzzi, E., Franke-Whittle, I. H., Kelderer, M., Ciavatta, C., and Insam, H. (2017). Microbial indication of soil health in apple orchards affected by replant disease. Appl. Soil Ecol. 119, 115–127. doi: 10.1016/j.apsoil.2017.06.003
Pham, T. L., and Utsumi, M. (2018). An overview of the accumulation of microcystins in aquatic ecosystems. J. Environ. Manag. 213, 520–529. doi: 10.1016/j.jenvman.2018.01.077
Preece, E. P., Hardy, F. J., Moore, B. C., and Bryan, M. (2017). A review of microcystin detections in estuarine and marine waters: environmental implications and human health risk. Harmful Algae 61, 31–45. doi: 10.1016/j.hal.2016.11.006
Ramos, A. G., Martel, A., Codd, G. A., Soler, E., Coca, J., Redondo, L., et al. (2005). Bloom of the marine diazotrophic cyanobacterium Trichodesmium erythraeum in the northwest African upwelling. M.E.P.S. 301, 303–305. doi: 10.3354/meps301303
Renchao, D., Yang, X. B., Wang, C. Y., Liu, K. X., Liu, Z. H., Ma, X. D., et al. (2019). Yangonin protects against non-alcoholic fatty liver disease through farnesoid X receptor. Phytomedicine 53, 134–142. doi: 10.1016/j.phymed.2018.09.006
Salman, M., and Naseem, I. (2015). Riboflavin as adjuvant with cisplatin: study in mouse skin cancer model. Front. Biosci. 7, 278–291. doi: 10.2741/e731
Santos, T. T., Dassi, S. C., Franco, C. R. C., Costa, C. R. V., Lee, S. A., and Silva, A. V. F. (2019). Influence of fibre and betaine on development of the gastrointestinal tract of broilers between hatch and 14 d of age. Anim. Nutr. 5, 163–173. doi: 10.1016/j.aninu.2018.06.005
Séré, M. G., Tortosa, P., Chabanet, P., Turquet, J., Quod, J. P., and Schleyer, M. H. (2013). Bacterial communities associated with Porites white patch syndrome (PWPS) on three western Indian Ocean (WIO) coral reefs. PLoS One 8:e83746. doi: 10.1371/journal.pone.0083746
Song, Y. L., Cheng, W., and Wang, C. H. (1993). Isolation and characterization of vibrio damsela infections of cultural shrimp in Taiwan. J. Invert. Pathol. 61, 24–31. doi: 10.1006/jipa.1993.1005
Valdes, A. M., Walter, J., Segal, E., and Spector, T. D. (2018). Role of the gut microbiota in nutrition and health. BMJ Clin. Res. 361:k2179. doi: 10.1136/bmj.k2179
Vaseeharan, B., Sundararaj, S., Murugan, T., and Chen, J. (2007). Photobacterium damselae sp. Damselae associated with diseased black tiger shrimp Penaeus monodon Fabricius in India. Lett. Appl. Microbiol. 45, 82–86. doi: 10.1111/j.1472-765X.2007.02139.x
Wang, H. Z., Liu, S. Q., Xun, X. G., Li, M. L., Lou, J. R., Zhang, Y. H., et al. (2021). Toxin-and species-dependent regulation of ATP-binding cassette (ABC) transporters in scallops after exposure to paralytic shellfish toxin-producing dinoflagellates. Aquat. Toxicol. 230:105697. doi: 10.1016/j.aquatox.2020.105697
Wang, X. (2018). “The dynamic variation of microcystins in shrimp ponds and toxic mechanism to Litopenaeus vannamei,” in Dissertation, Vol. 22-23. China Jiliang University).
Xiao, F. S., Liao, L. J., Xu, Q. Q., He, Z. L., Xiao, T. Y., Wang, J. J., et al. (2021b). Host-microbiota interactions and responses to grass carp reovirus infection in Ctenopharyngodon idellus. Environ. Microbiol. 23, 431–447. doi: 10.1111/1462-2920.15330
Xiao, F. S., Zhu, W. G., Yu, Y. H., He, Z. L., Wu, B., Wang, C., et al. (2021a). Host development overwhelms environmental dispersal in governing the ecological succession of zebrafish gut microbiota. NPJ Biofilms Microb. 7:5. doi: 10.1038/s41522-020-00176-2
Xie, S. W., Li, Y. T., Zhou, W. W., Tian, L. X., Li, Y. M., Zeng, S. L., et al. (2015). Effect of γ-aminobutyric acid supplementation on growth performance, endocrine hormone and stress tolerance of juvenile Pacific white shrimp, Litopenaeus vannamei, fed low fishmeal diet. Aquac. Nutr. 23, 54–62. doi: 10.1111/anu.12358
Ye, R., Shan, K., Gao, H., Zhang, R., Xiong, W., Wang, Y., et al. (2014). Spatio-temporal distribution patterns in environmental factors, chlorophyll-a and microcystins in a large shallow lake, lake Taihu, China. Int. J. Environ. Res. Publ. Health 11, 5155–5169. doi: 10.3390/ijerph110505155
Yuan, J. L., Gu, Z. M., Zheng, Y., Zhang, Y. Y., Gao, J. C., Chen, S., et al. (2016). Accumulation and detoxification dynamics of microcystin-LR and antioxidant responses in male red swamp crayfish Procambarus clarkii. Aquat. Toxicol. 177, 8–18. doi: 10.1016/j.aquatox.2016.05.004
Zhang, G., Zhang, P., Li, W., Hao, F., Chen, F., Yu, Y., et al. (2020a). Reduction of gut microbial diversity and short chain fatty acids in BALB/c mice exposure to microcystin-LR. Ecotoxicology 29, 1347–1357. doi: 10.1007/s10646-020-02254-9
Zhang, Y., Li, Z., Kholodkevich, S., Sharov, A., Feng, Y., Ren, N., et al. (2020b). Microcystin-LR-induced changes of hepatopancreatic transcriptome, intestinal microbiota, and histopathology of freshwater crayfish (Procambarus clarkii). Sci. Total Environ. 711:134549. doi: 10.1016/j.scitotenv.2019.134549
Zhang, Z., Zhang, X., Wu, B., Yin, J., Yu, Y., and Yang, L. (2016). Comprehensive insights into microcystin-LR effects on hepatic lipid metabolism using cross-omics technologies. J. Hazard. Mater. 315, 126–134. doi: 10.1016/j.jhazmat.2016.05.011
Zhuang, L., Jin, Z., Li, H., Wu, S., Tong, X., Wang, H., et al. (2021). Effects of chronic exposure to microcystin-LR on the gut microbiota of male mice. Int. J. Toxicol. 40, 171–177. doi: 10.1177/1091581820972311
Keywords: shrimp, microcystin-LR, intestinal microbial, gene function, metabolites
Citation: Duan Y, Xing Y, Zeng S, Dan X, Mo Z, Zhang J and Li Y (2022) Integration of metagenomic and metabolomic insights into the effects of microcystin-LR on intestinal microbiota of Litopenaeus vannamei. Front. Microbiol. 13:994188. doi: 10.3389/fmicb.2022.994188
Received: 15 July 2022; Accepted: 05 September 2022;
Published: 23 September 2022.
Edited by:
Jin Zhou, Tsinghua University, ChinaCopyright © 2022 Duan, Xing, Zeng, Dan, Mo, Zhang and Li. This is an open-access article distributed under the terms of the Creative Commons Attribution License (CC BY). The use, distribution or reproduction in other forums is permitted, provided the original author(s) and the copyright owner(s) are credited and that the original publication in this journal is cited, in accordance with accepted academic practice. No use, distribution or reproduction is permitted which does not comply with these terms.
*Correspondence: Jiasong Zhang, amlhc29uZ3poYW5nQGhvdG1haWwuY29t; Yanwei Li, eWFud2VpbGlAc2NhdS5lZHUuY24=
Disclaimer: All claims expressed in this article are solely those of the authors and do not necessarily represent those of their affiliated organizations, or those of the publisher, the editors and the reviewers. Any product that may be evaluated in this article or claim that may be made by its manufacturer is not guaranteed or endorsed by the publisher.
Research integrity at Frontiers
Learn more about the work of our research integrity team to safeguard the quality of each article we publish.